Abstract
We present the broadband (0.3–40.0 keV) X-ray analysis of a unique intermediate polar Paloma using simultaneous data from XMM-Newton and NuSTAR observatories. The X-ray power spectra show strong modulations over orbital period compared to spin period. The orbit-folded light curves show a single broad hump-like structure with strong dips for soft to medium X-rays (0.3–10.0 keV). The energy-dependent dips at ϕ ∼ 0.16 and 0.5 arise as a result of a complex intrinsic absorber, strong enough to have an effect around 15 keV. The absorber could potentially be contributed from accretion curtain/accretion stream and absorbing material produced by stream–disk/stream–magnetosphere interactions. We notice significant variation of the absorber with orbital phase, with maximum absorption during orbital phase 0.1–0.22. The absorber requires more than one partial covering absorber component, specifying the necessity to use distribution of column densities for spectral modeling of the source. The isobaric cooling flow component is utilized to model the emission from the multitemperature post-shock region, giving a shock temperature of keV, which corresponds to a white dwarf mass of
. We have used both the neutral absorber and warm absorber models, which statistically give a similarly good fit, but with different physical implications. Among the Fe Kα line complex, the neutral line is the weakest. We probed the Compton reflection and found a minimal statistical contribution in the spectral fitting, suggesting the presence of weak reflection in Paloma.
Export citation and abstract BibTeX RIS

Original content from this work may be used under the terms of the Creative Commons Attribution 4.0 licence. Any further distribution of this work must maintain attribution to the author(s) and the title of the work, journal citation and DOI.
1. Introduction
Intermediate polars (IPs) are a type of magnetic cataclysmic variable (mCV) in which the white dwarf (WD) magnetic field is relatively weaker than the polars. Unlike the polars, the magnetic locking between the WD and the companion star is absent in IPs, giving rise to a lack of synchronicity between the spin period (Pω ) and the orbital period (PΩ). In most IPs, the spin period is much shorter than the orbital period (Pω ≲ 0.1 × PΩ).
In IPs, a weaker magnetic field may allow formation of a partial accretion disk before the magnetic field disrupts the disk. During the final stretch of the accretion, the accreting material falls on the WD following the magnetic field lines of the WD. The infalling material with supersonic velocity creates strong shock over the WD surface. Subsequently, the material cools down in the post-shock region (PSR) by radiating bremsstrahlung emission in X-rays and cyclotron emission in optical wavelengths, and it finally settles down on the WD surface. The X-rays emitted from the PSR interact with the intrinsic absorber like accretion stream or accretion curtain in the source, as well as any intervening medium like the interstellar medium (ISM), while reaching the observer. A part of the X-rays can get Compton reflected by the WD surface and produce an excess (hump-like feature) in the 10–30 keV range of the spectrum. In addition, a part of the emitted X-rays will undergo photoelectric absorption, giving rise to fluorescence emissions, most notably an iron fluorescence line at 6.4 keV. It is believed that the strength of the iron fluorescence line is correlated to the Compton reflection, as both physical processes can take place in a similar region of the WD surface.
The source Paloma (RX J0524+42, 1RXS J052430.2+424449) is a special IP in which the asynchronism is much less (∼14%) compared to other IPs (typically ∼90%). Schwarz et al. (2007) have studied the source using optical photometry on multiple nights, extended over a duration of several years, and ROSAT X-ray observation. They detected three strong periodic signatures in the optical light curve and commented on two possible values of the spin period. Recently, Littlefield et al. (2022) specified one of the two values of Schwarz et al. (2007) for the spin period of the WD using nearly a month-long TESS observation. This source was studied by Joshi et al. (2016) in the soft to medium X-rays (0.3–10 keV) using XMM-Newton. Their spectral modeling hinted at the presence of strong reflection amplitude. Paloma is yet to receive its proper classification among mCVs. Here we should note that the rate of change of spin period () is not yet determined for Paloma. Given the asynchronosity that is much lower than conventional IPs and slightly higher than traditional asynchronous polars (APs; ∼1%–2%; e.g., V1432 Aql, CD Ind, BY Cam, V1500 CYg) for which
is measured, it is hard to distinctly classify Paloma as an IP or AP. Schwarz et al. (2007) and Joshi et al. (2016) described Paloma as an IP, whereas Littlefield et al. (2022) considered it to be an AP.
Using the broadband X-ray data, simultaneously obtained from XMM-Newton and NuSTAR, we tried to give an overall description of the spectral properties of the system, along with the temporal behavior of the system extending all the way up to 40 keV. The strong reflection amplitude should manifest a reflection hump in the range of 10–30 keV, which we could not unambiguously detect in the NuSTAR spectra. We argued for an alternative picture to the strong reflection hypothesis, using a complicated absorber model for the source. We also probed for X-ray periodicity in the power spectra. In the following section we describe the reduction of the obtained data. In Section 3, we present our results based on the timing and the spectral analysis of the broadband X-ray data. In Section 4 we discuss our results, and we summarize the results in Section 5.
2. Observation and Data Reduction
Paloma was observed simultaneously by the XMM-Newton (Jansen et al. 2001) and NuSTAR (Harrison et al. 2013) observatories. XMM-Newton can observe the source in the 0.3–10.0 keV band with good spectral resolution, whereas NuSTAR is capable of imaging the source in the 3.0–78.0 keV band with high sensitivity in the hard X-rays. Thereby simultaneous observation by these two observatories provides us with broadband data in the range of 0.3–78.0 keV. This enables us to probe the properties of the source in the soft X-rays (e.g., constraining the absorption parameters) as well as in the hard X-rays (e.g., detecting the shock temperature and Compton reflection).
NuSTAR observed (ObsID 30601019002) the source on UTC 2021-3-2 01:10:08. The focal plane modules (FPMA and FPMB) recorded the source for an exposure time of ∼47 ks. We have selected a source region using a circle of radius 40'' and a background region of radius 80'' in the same detector as that of the source. We used NUPIPELINE to extract the cleaned event files with default screening criteria and then NUPRODUCTS to obtain the final science products such as light curves and the spectra, along with detector response files. We performed barycenter correction while deriving the science products. The time bin size of the extracted light curves is chosen to be 1 s. We have grouped the spectra for a minimum number of counts of 25 per bin to use χ2 statistics to test goodness of fit.
The XMM-Newton observation (ObsID 0870800201) was taken on UTC 2021-3-2 01:06:28 for a duration of ∼33 ks. The observation was carried out in full window mode using the medium filter for both the PN (Struder et al. 2001) and MOS (Turner et al. 2001) cameras of the European Photon Imaging Camera (EPIC) instruments. We have selected a circular source region of radius 25'' and a circular background region of radius 50'' in the same detector as that of the source. We used XMMSAS v19.1.0 (Gabriel et al. 2004) for data reduction and extraction of the light curves and the spectra following the SAS analysis thread. 1 The calibration files are used from the current calibration file (CCF) repository 2 of XMM-Newton, the latest during the analysis. The XMM data are contaminated with background flaring in the initial part of the observation, so we discarded an initial few kiloseconds of data, which neatly eliminates the flaring part and leaves us with nearly ∼29 ks of continuous data from all the EPIC cameras. Using SAS tool EPATPLOT, we have also checked for pileup in the data, and we did not find any significant contribution of it. The products have been barycenter corrected. We chose the 1 s binning for the extracted light curves. The SAS tool SPECGROUP is used to group the data with minimum counts per bin of at least 25 for using the χ2 statistics. The oversample parameter is set at 3 so that minimum group width is not less than 1/3 of the FWHM of the instrument resolution at that energy.
We have also extracted the spectrum from the reflection grating spectrometer (RGS) on board XMM-Newton. We have used the RGSPROC tool and eliminated the background flaring to obtain the grating spectra. The spectra are also grouped into 25 minimum counts per bin to use χ2 statistics.
3. Data Analysis and Results
3.1. Timing Analysis
We have the continuous data from XMM-Newton for ∼29 ks and a total duration of ∼96 ks data from NuSTAR including the gaps due to Earth occultation and the South Atlantic Anomaly (SAA). The duration of XMM-Newton and NuSTAR observation gives us the opportunity to study the timing properties of the source for ∼3 and 10 cycles, respectively. We have shown the background-subtracted light curves from XMM-Newton and NuSTAR in the top and middle panels of Figure 1, respectively. In the bottom panel of Figure 1, the light curves in the 3–10 keV band from both the observatories are overplotted. In this section, we have calculated the power spectra for the source and subsequently folded the light curves based on the orbital period of the system. We have presented the results from NuSTAR-FPMA and XMM-Newton EPIC-PN in the following subsections, as the results from FPMB for NuSTAR and MOS1 and MOS2 for XMM-Newton EPIC are also similar.
Figure 1. Background-subtracted light curves from XMM-Newton (top) and NuSTAR (middle) and overlapping 3–10 keV light curves from both the observatories for comparison (bottom).
Download figure:
Standard image High-resolution image3.1.1. Power Spectra
We have implemented two methods to search for the period: the Lomb–Scargle method (Lomb 1976; Scargle 1982) and the CLEAN algorithm (Roberts et al. 1987). The first one is widely used to find the frequencies present in an unevenly sampled time series. The later one uses the algorithm to deconvolve the effect of the window function from the discrete Fourier transform (DFT) spectra and thereby minimize the effect of spectral leakage. This method has been used efficiently for other IP sources with unevenly sampled data (Norton et al. 1992, 1997).
In the NuSTAR-FPMA power spectra using the Lomb–Scargle method (top panel of Figure 2), we can clearly identify the peak at orbital frequency Ω, corresponding to a period value of 9384 ± 229 s, present in all the energy bands (3–40 keV, 3–10 keV, and 10–40 keV). We notice that the peaks are stronger in the 3–10 keV band compared to the 10–40 keV band. This period is also identified by Schwarz et al. (2007) at ∼9430.35 s in their optical photometric campaign and Thorstensen et al. (2017) at ∼9434.88 s using radial velocity spectroscopy. We see the spin frequency peak ω, corresponding to a period value of 8365 ± 181 s, immediately next to the orbital frequency in the Lomb–Scargle power spectra. This value agrees with one of the spin period candidate (∼8175.37 s) by Schwarz et al. (2007) and spin period (∼8229 s) detected by Littlefield et al. (2022). We notice another weak peak at 2ω − Ω, with a period value of 7545 ± 148 s. In the FPMA power spectra, these two peaks (ω, 2ω − Ω) appear with a much lower power compared to the Ω. Joshi et al. (2016) obtained two low-power peaks for ω and 2ω − Ω adjacent to the strongest peak at orbital period in their power spectra, obtained using previous XMM-Newton observation, but with smaller period values (7800 and 6660 s, respectively) than ours. In Figure 2 we have also marked the expected positions of several harmonics and sideband frequencies. We notice a relatively strong peak (2850 ± 21 s) near Ω + 2ω (expected at 2893 ± 48 s). We also observe peaks at 4474 ± 52 s and 4183 ± 46 s, which respectively agree with Ω + ω (expected at 4423 ± 72 s) and 2ω (expected at 4182 ± 90 s) within error bars. Note that the resolution of our power spectra, which depends on the total observation duration, data gaps, etc., creates a hindrance to resolve all the harmonics and sidebands precisely. Another possible candidate of spin period at ∼8758.12 s according to Schwarz et al. (2007), which is very close to peak Ω, could not be confirmed owing to the limitation of the resolution of the power spectra. We also notice that the peaks in the FPMA power spectra are stronger in the 3–10 keV band compared to the 10–40 keV band.
Figure 2. Power spectra obtained from NuSTAR-FPMA using the Lomb–Scargle algorithm (top) and CLEAN algorithm (bottom). We have identified the two fundamental peaks, Ω and ω, along with the location of a few sidebands and harmonics.
Download figure:
Standard image High-resolution imageIn the bottom panel of Figure 2, we have plotted the CLEANed power spectra. Before performing the CLEAN operation, we have detrended the light curve for the presence of dc bias, if any, by subtracting a mean value and dividing by the standard deviation. The CLEANed algorithm requires two parameters—number of iterations and loop gain—to deconvolve the window power spectra from the DFT spectra iteratively with the gain fraction in each iteration. We chose gain 0.1, typically used (Norton et al. 1992; Rana et al. 2004) for this algorithm. Iteration number was set at 1000. We find that the strong Ω peak and the Ω + 2ω peak are present; however, other weak peaks at ω, 2ω − Ω, and 2ω, which were in seen in the Lomb–Scargle power spectra, are almost diminished in the CLEANed power spectra.
A similar exercise is done for the XMM-Newton PN power spectra (Figure 3). Though the PN light curves are continuous, the power spectral resolution is poor owing to the shorter duration of the observation. We have found that the peak corresponding to Ω is evidently present, with a period value of 9400 ± 761 s, agreeing with that of the FPMA value. We are unable to distinguish either ω or 2ω − Ω in the PN power spectra because of the broad peak of Ω. It is interesting to note that the peak strength of Ω is much lower in the 0.3–3.0 keV band than in the 3–10 keV band. However, for all other peaks in the power spectra, peak strength decreases in the higher energy band. By our previous definition of orbital and spin periods from FPMA, we have marked their expected positions in the PN power spectra, along with harmonics and sideband frequencies. We notice that there is significantly strong power near the position marked by Ω + 2ω, as we found from FPMA power spectra.
Figure 3. Power spectra obtained from XMM-Newton PN using the Lomb–Scargle algorithm (top figure) and CLEAN algorithm (bottom figure). The Ω peak is identified, along with the expected (from FPMA power spectra) position of ω. Few sideband frequencies and harmonics are labeled.
Download figure:
Standard image High-resolution image3.1.2. Folded Light Curves
Based on our measured orbital period (9384 ± 229 s), we have folded the PN and FPMA light curves to study the rotational properties of the source. The folding is performed based on the ephemeris TJD = 19,275, chosen arbitrarily close to the start time of the NuSTAR observation. The folded light curves in different energy bands and the hardness ratios (HRs) are shown in Figure 4. In the soft X-rays, the 0.3–3.0 keV band obtained from PN (left panel of Figure 4), there is strong modulation in the light curve; however, the entire light curve can be described by one broad hump (ϕ = 0.7–1.7), with several dips in between. There are three prominent minima, appearing at ϕ ∼ 0.16, 0.50, and 0.70, with the first one being the strongest. In the 3.0–10.0 keV band, the similar broad hump-like feature is visible, with the minimum at ϕ ∼ 0.70 being the strongest one. A direct comparison of PN light curve with the FPMA light curve (right panel of Figure 4) in the same energy band, 3–10 keV, shows the similar nature of the hump and the presence of an overall minimum at ϕ ∼ 0.70. The pulse fraction (PF) of modulation in the 3–10 keV energy band is 72% ± 4% and 72% ± 6% from PN and FPMA, respectively, using the definition PF , where I denotes the count rate. The hard X-ray in the 3–10 keV band of FPMA follows the same pattern of the light curve as that of 10–40 keV.
Figure 4. Folded light curve, based on period 9384 s, from different energy bands, using PN (left) and FPMA (right). The bin size is 188 s. In the bottom panels, HR1 (left) and HR2 (right) are shown. A constant model is fitted to HR2, represented by a black line.
Download figure:
Standard image High-resolution imageAs we inspect the hardness ratio 1 (HR1), defined as HR1 = I3−10 keV/I0.3−3.0 keV, obtained from the PN light curve, we notice a strong peak around ϕ ∼ 0.16 (bottom left panel of Figure 4). This corresponds to the dip at the same phase, seen in the 0.3–3.0 keV and 3.0–10.0 keV bands of the PN light curve. HR1 can roughly be divided into four intervals: (a) ϕ ∼ 0.10–0.22, (b) ϕ ∼ 0.22–0.50, (c) ϕ ∼ 0.50–0.80, and (d) ϕ ∼ 0.80–1.10. In HR1, phase interval (a) shows the strong peak, whereas interval (c) covers the lowest valley region. Intervals (b) and (d) represent the phase intervals before and after the peak region in the HR1, respectively.
Hardness ratio 2 (HR2), defined similarly to HR1, but for the 10–40 keV and 3–10 keV bands of FPMA, exhibit a rather flat profile (bottom right panel of Figure 4). We have fit a constant model that gives a mean value of 0.34, with an acceptable fit statistic of χ2(dof) = 141.5(100).
We did not attempt detailed analysis based on the period ω, since this period appears very weakly in the FPMA power spectra and could not be resolved in PN power spectra.
3.2. Spectral Analysis
For the spectral analysis, the X-ray Spectral Analysis Software package XSPEC v12.12.0 (Arnaud 1996) is used. We have followed the abundance table defined by Wilms and McCray (Wilms et al. 2000) and the photoelectric cross-section table set after Verner et al. (1996). All the spectral models used for analysis are part of the XSPEC package. The errors on the parameter values are quoted within 90% confidence levels. In this section we have first attempted the spectral analysis with NuSTAR data and XMM-Newton data separately, to have an idea of how the spectra can be modeled in the hard X-ray part and the soft X-ray part, along with detecting the strong line emissions present in the spectra. Finally, we have employed the complete spectral model to jointly fit the broadband spectral data obtained from both the observatories.
3.2.1. Phenomenological Fit to NuSTAR Spectra
The FPMA and FPMB data in the 3–40 keV range are employed for probing the shock temperature of the PSR and the presence of the Compton reflection hump. Beyond 40 keV the background dominates over the source counts; hence, it is not included for the spectral analysis. Initially we have used an absorbed bremsstrahlung model, with a single Gaussian component for modeling the iron line complex, which could not be resolved by NuSTAR data. We obtain a good fit with χ2/dof = 463(439). The column density of the absorber is . This value is larger than the total galactic column density along the direction of the source, 0.42 × 1022 cm−2 (HI4PI Collaboration et al. 2016). This indicates that a strong absorption specific to the source is present in the spectra. The obtained continuum temperature is
keV. The Gaussian component shows a line energy of
keV, with a
eV, indicating the presence of significant ionized Fe Kα lines.
Since the PSR is multitemperature in nature, the obtained bremsstrahlung temperature indicates an average temperature of the entire PSR. Therefore, we used the isobaric cooling flow model mkcflow (Mushotzky & Szymkowiak 1988) to model the PSR (Mukai et al. 2003). This model also incorporates the ionized line emissions, including the ionized Fe Kα lines (He-like and H-like). We kept the switch parameter set at 2 to determine the spectra based on AtomDB 3.0.9.
3
The redshift parameter is fixed at 1.35 × 10−7 following the Gaia DR2 distance of 578 ± 35 pc (Gaia Collaboration et al. 2018). We have also incorporated the absorption component tbabs. For the neutral Fe Kα line at 6.4 keV, we have added a Gaussian component with line central energy fixed at 6.4 keV and line width σ fixed at 0 (as suggested by spectral analysis of XMM-Newton EPIC data, discussed in the next section). We obtained an acceptable fit with χ2(dof) = 470(440). The overall absorber column density remains similar to that of the bremsstrahlung fit within statistical uncertainty. The lower temperature reached the lower limit allowed by the model, so we fixed it at 0.0808 keV. The upper temperature comes out to be keV. To check the presence of Compton reflection, we convolved the reflect component with the multitemperature cooling flow model. It slightly improves the fit statistic to χ2(dof) = 464(439), but the reflection amplitude gives a high value
with large uncertainty associated with it. The upper temperature is somewhat lowered in this case, standing at
keV. The values of the other parameters remain similar to the no-reflection fit within statistical uncertainty. A strong and complex absorption can affect the spectra beyond 7 keV, and thereby the continuum itself. Therefore, we can comment on reflection only after properly modeling the absorption in the source by including soft X-rays from XMM-Newton data.
3.2.2. Phenomenological Fit to XMM-Newton Spectra
First, we have utilized the EPIC (PN, MOS1, and MOS2) spectra in the 5–8 keV range to study the Fe Kα emission lines. We have used the absorbed bremsstrahlung model to fit the underlying continuum. We have added three Gaussians to model the three Fe Kα lines: neutral, He-like, and H-like. The best-fit parameter values are reported in Table 1, with the spectral fitting plotted in Figure 5. The line widths are kept fixed at 0 since all the Fe Kα lines are consistent with the instrumental resolution limit of EPIC (∼120 eV at 6.0 keV). This shows that lines are narrow. We have fixed the bremsstrahlung temperature and column density of the absorber at 19.9 keV and 3.76 × 1022 cm−2, as obtained with the NuSTAR-only fit using the bremsstrahlung model (see Section 3.2.1), which otherwise could not be constrained. We notice that the best-fit values of the line central energies are slightly redshifted; however, that is not statistically important, since they agree with their expected theoretical values within error bars.
Figure 5. Fe Kα lines are fitted with three Gaussian components over an absorbed bremsstrahlung continuum using EPIC data in the 5–8 keV range. We show the spectra and data-to-model ratio in the top and bottom panels, respectively.
Download figure:
Standard image High-resolution imageTable 1. Probing Iron Lines Using EPIC Data (5.0–8.0 keV)
Parameter | Unit | Value |
---|---|---|
nH,tb | ×1022 cm−2 | 3.76f |
Tbr | keV | 19.9f |
Nbr | ×10−3 |
![]() |
EL, Neutral | keV |
![]() |
σNeutral | eV | 0f |
eqwNeutral | eV |
![]() |
NL, Neutral | ×10−5 |
![]() |
EHe−Like | keV |
![]() |
σHe−Like | eV | 0f |
eqwHe−Like | eV |
![]() |
NHe−Like | ×10−5 |
![]() |
EH−Like | keV |
![]() |
σH−Like | eV | 0f |
eqwH−Like | eV |
![]() |
NH−Like | ×10−5 |
![]() |
χ2(dof) | 123(93) |
Note. nH,tb represents column density of the absorber. Tbr and Nbr denote the temperature and normalization of bremsstrahlung continuum, respectively. EL , σ, NL , and "eqw" represent line central energy, line width, normalization, and equivalent width of the corresponding Fe Kα line, respectively. f denotes that the line parameter is fixed.
Download table as: ASCIITypeset image
We have next looked into the RGS spectra. The spectra from RGS1 and RGS2 are simultaneously fit in the range of 0.45–2.0 keV. The signal-to-noise ratio of the spectral data is poor, with only 47% of the total count being contributed from source. However, the ionized oxygen line (O vii) in the spectra is clearly present. To get an overview of the ionized line, we fitted the line with a Gaussian component on top of an absorbed bremsstrahlung continuum. The spectra are shown in Figure 6. The line center appears at keV, with a line width (σ) of
eV and an equivalent width of
eV. We note that the O vii line is composed of fine atomic transition lines (intercombination, resonance, and forbidden lines), which RGS cannot resolve, thereby giving rise to a broad line width. There is visibly some excess residual around 0.5 keV, expected from the N vii line. But a poor signal-to-noise ratio for that line makes it statistically unimportant.
Figure 6. Fitting the O vii Kα line in RGS spectra. The spectral quality is poor, but the O vii line appears with significant strength and fitted with a Gaussian over an absorbed bremsstrahlung continuum. The spectra and data-to-model ratio are shown in the top and bottom panels, respectively. Expected locations of O viii and N vii Kα lines are marked.
Download figure:
Standard image High-resolution imageIn order to constrain the absorption parameters, as well as the nature of the multitemperature PSR, the spectral data from all three EPIC cameras were simultaneously fit in the 0.3–10.0 keV band. We have used ISM absorption model tbabs for modeling the overall absorption near the source, as well as any intervening galactic absorption. The multitemperature plasma of the PSR has been modeled with the cooling flow model, implemented by mkcflow. The neutral Fe Kα line is described by a narrow (σ = 0) Gaussian component. The absorbed cooling flow model with a Gaussian component gives an extremely poor fit statistic χ2(dof) = 5447(424). Inclusion of a partial covering absorber dramatically improves the fit, with χ2(dof) = 604(421). The overall column density appears to be ∼6.3 × 1020 cm−2. The column density of the partial covering absorber comes out as ∼6.08 × 1022 cm−2 with a covering fraction of ∼65%. However, the upper temperature of the cooling flow model remains unconstrained (79 keV), the overall abundance is very large (∼3.77), and the fit statistic is still poor and deserves further improvement. Inclusion of a reflect model substantially improves the fit to χ2(dof) = 501(420), but the reflection amplitude turns out to be unphysically large (∼8.24). A poor fit with a single partial covering component suggests that absorption should require more elaborate modeling. In addition, getting such a high value of reflection amplitude indirectly shows that absorption has an extended effect until the energy range 7 keV and beyond, which can also be mimicked by the tail of the reflection hump for an alleged strong Compton reflection. Therefore, instead of reflect, we introduced another partial covering absorber (pcfabs 1 ∗pcfabs 2 ). Two partial covering absorbers mathematically describe abosorbers with three column densities and corresponding covering fraction (Equation (1))
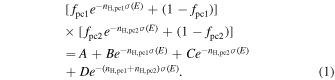
Here σ(E) stands for photoelectric cross section, and nH,pc1, fpc1 and nH,pc2, fpc1 represent column densities and covering fraction contributed from each partial absorber model component. B, C, and D are the functions of fpc1 and fpc2 and represent the respective fraction of each of the resulting three column densities, and A = 1 − (B + C + D).
The fit with two partial covering absorbers improves the fit statistic significantly (χ2(dof) = 478(419)) in comparison to the single partial absorber model. The column density of the overall absorber is . The column density and covering fraction of the two pcfabs components become
with 65% ± 3% and
with 50% ± 5%, respectively. The upper temperature of the cooling flow model is constrained with a value of
keV. Though the error bars are large, it is expected owing to the limited coverage of hard X-rays by XMM-Newton data. The lower temperature of
keV represents some mean temperature near the bottom region of the PSR. At this point we observe slight excess in the soft X-rays, possibly arising as a result of line emissions of low Z and Fe L-shell emissions. The main excess is around 0.57 keV, representing the O vii line, which is also observed from RGS spectra. To model that, we introduced an extra optically thin plasma emission component (mekal), appearing with a temperature
keV, which decreased the fit statistic further to χ2(dof) = 466(417). The corresponding F-statistic probability for inclusion of this extra component is 4.9 × 10−3.
It is evident that the source spectra involve a complicated absorption scenario, where it is paramount to model it appropriately. Motivated by the fact that a single partial absorber is not enough to model the local intrinsic absorber, which indicated a distribution of column densities, we introduced the pwab (Done & Magdziarz 1998) component to model the intrinsic absorber. This component assumes a power-law distribution of the covering fraction as a function of column density of neutral absorbers. The fit statistic is χ2(dof) = 470(418), closely similar to the fit statistic obtained from the previous two partial absorber fits. We kept the of the power-law absorber fixed to 1 × 1015 cm−2, which could not be constrained otherwise. The
provides a value of
, along with a power-law index of
. We could not constrain the column density of the overall absorber either, which provided us with an upper limit of <7.0 × 1019 cm−2. However, in this fit, the emission components parameters remain similar with that of the two partial covering absorber fit scenario within statistical uncertainty. Here we notice that the F-test probability of introducing the extra optically thin component to model the emission features below 1 keV is 1.5 × 10−18, indicating a much stronger necessity for this component than the case of two partial absorbers.
Given the complex intrinsic absorber scenario of Paloma and emission features in soft X-rays, we have also performed an alternate modeling using zxipab (Islam & Mukai 2021). This component can describe the absorption in the pre-shock flow as the power-law distribution of the warm photoionized absorber, hence providing an alternate description of the soft X-ray features. In this case, we do not introduce an extra low-temperature plasma emission component. We obtain a similarly good fit statistic as in previous cases, χ2(dof) = 467(420). The overall absorber presents itself with a column density of , consistent with the two-partial-absorber scenario. The warm absorber shows a column density value of
for
, and we kept the
frozen at the lowest value permissible for this parameter (1015 cm−2), because of it becoming unconstrained. The low temperature of the cooling flow component now goes to the lower limit allowed by the model, so fixed at 0.0808 keV. The upper temperature (
keV) is consistent with the value obtained from the previous two scenarios within statistical uncertainty.
3.2.3. Simultaneous Fit to Broadband XMM-Newton and NuSTAR Spectra
Equipped with our understanding of the complex absorber and the overall continuum from the XMM-only and NuSTAR-only fits, we devised three model variants M1, M2, and M3 to model the broadband spectra in the range of 0.3–40.0 keV. The description of the model variants is given in Table 2.
Table 2. Model Description in XSPEC Notation
Abbreviation | Model |
---|---|
M1 | constant∗tbabs∗pcfabs∗pcfabs∗(mckflow+mekal+Gauss) |
M1-R | constant∗tbabs∗pcfabs∗pcfabs∗(reflect∗(mckflow+mekal)+Gauss) |
M2 | constant∗tbabs∗pwab∗(mckflow+mekal+Gauss) |
M2-R | constant∗tbabs∗pwab∗(reflect∗(mckflow+mekal)+Gauss) |
M3 | constant∗tbabs∗zxipab∗(mckflow+Gauss) |
M3-R | constant∗tbabs∗zxipab∗(reflect∗mckflow+Gauss) |
Download table as: ASCIITypeset image
We have quoted the best-fit values of the parameters in Table 3. For representation, the spectral and ratio (data/model) plots using model M1 are shown in the top and middle panels of Figure 7. The spectral parameters agree within the statistical uncertainty for all three models. However, for model M2 we could not constrain the column density of the overall absorber, and an upper limit (<0.7 × 1020 cm−2) is obtained. For a low value of the overall absorber that includes the galactic absorption, it may be hard to constrain this parameter, given that we already have a complex intrinsic absorber component to describe the spectra. Note also that the neutral power-law distribution of absorber pwab and the ionized version zxipab have significant differences in predicting the model flux below <0.8 keV (Figure 1 of Islam & Mukai 2021). This gives rise to differences in the obtained column densities of the overall absorber between models M2 and M3. M1, M2, and M3 give us consistently statistically acceptable fits, with a χ2(dof) = 952(859), 956(862), and 952(863), respectively. In addition, the emission parameters for all three model variants give statistically similar values.
Figure 7. The top and middle panels show the plots of the best-fit model and ratio (data/model) for the broadband spectra using model variant M1. We In the bottom panel we show the ratio plot using the "reflect" version of model M1, i.e., M1-R.
Download figure:
Standard image High-resolution imageTable 3. Best-fit Parameters Using Simultaneous Broadband Spectra in the 0.3–40.0 keV Range
Parameter d | Unit | M1 | M1-R | M2 | M2-R | M3 | M3-R |
---|---|---|---|---|---|---|---|
nH,tb | 1020 cm−2 |
![]() |
![]() | <0.7 | <0.7 |
![]() |
![]() |
nH,pc1 | 1022 cm−2 |
![]() |
![]() | ⋯ | ⋯ | ⋯ | ⋯ |
pcf1/log(xi) a |
![]() |
![]() | ⋯ | ⋯ |
![]() |
![]() | |
nH,pc2/![]() | 1022 cm−2 |
![]() |
![]() |
![]() |
![]() |
![]() |
![]() |
pcf2/β c |
![]() |
![]() |
![]() |
![]() |
![]() |
![]() | |
Tme | keV |
![]() |
![]() |
![]() |
![]() | ⋯ | ⋯ |
Nme | 10−4 |
![]() |
![]() |
![]() |
![]() | ⋯ | ⋯ |
T1,mkc | keV |
![]() |
![]() |
![]() |
![]() | 0.0808f e | 0.0808f |
T2,mkc | keV |
![]() |
![]() |
![]() |
![]() |
![]() |
![]() |
Nmkc | 10−11 |
![]() |
![]() |
![]() |
![]() |
![]() |
![]() |
Z | Z⊙ |
![]() |
![]() |
![]() |
![]() |
![]() |
![]() |
Ω/2π | ⋯ |
![]() | ⋯ |
![]() | ⋯ |
![]() | |
EL | keV |
![]() |
![]() |
![]() |
![]() |
![]() |
![]() |
σ | eV | 0f | 0f | 0f | 0f | 0f | 0f |
N L | 10−5 |
![]() |
![]() |
![]() |
![]() |
![]() |
![]() |
χ2(dof) | 952(859) | 948(858) | 956(862) | 952(861) | 952(863) | 951(862) |
Notes.
a pcf1 (covering fraction for first partial absorber) for model M1 or log(xi) (ionization parameter of warm power-law absorber) for model M3. b nH,pc2 (column density of second partial absorber) for model M1 or
Download table as: ASCIITypeset image
Once our spectral model has satisfactorily constrained the absorption parameters and the continuum, we attempted to find the contribution of Compton reflection. We adopted model variants M1-R, M2-R, and M3-R (for model description, see Table 2), with the abundance parameters of the reflect component linked to that of emission components. We used the default value of the inclination angle (i), set at . The three models produced a reflection amplitude of
,
, and
, along with an improvement in fit statistic, however not large, of Δχ2(Δdof) = 4(1), 4(1), and 1(1), respectively (Table 3). The ratio (data/model) plot using model M1-R is shown in the bottom panel of Figure 7. Though mutually agreeing values of reflection amplitudes are obtained in all three models, the large statistical uncertainty and small improvement in fit-stat put us in a delicate situation to comment about its "robust" presence in our spectra.
3.2.4. Phase-resolved Spectroscopy
Our orbit-folded light curve (Figure 4) showed prominent variation in the HR1, indicating the presence of the change in the complex intrinsic absorber. We extracted phase-resolved spectra in the four phase intervals as defined in Section 3.1.2. We have quoted the best-fit parameters for the phase-resolved spectra in Table 4. We kept the column density of the overall absorber fixed at the best-fit value from the phase-averaged spectral fitting. The temperatures of the plasma emission components remain the same within statistical uncertainty for all the phases, as well as with the phase-averaged value, for any particular model variant. Though the column density of the complex absorbers also remains the same within error bars, our spectral fitting clearly reveals the change in the covering fraction parameters. For phase (a) model M1 shows the highest value of the covering fraction (pcf1 and pcf2) for the intrinsic absorbers ( and
), whereas for phase (c) the lowest values of those parameters are obtained (
and
). The parameter values (pcf1 and pcf2) for the other two phases, phase (b) (
and
) and phase (d) (
and
), are consistent with each other. Models M2 and M3 follow the same trend with the power-law index for the complex absorber. We obtain the highest value of the power-law index (∼−0.3) for phase (a) and the lowest value (∼−0.7) for phase (c), whereas phases (b) and (d) give intermediate values (∼−0.5), similar to each other. For model M3, we also obtain a low value of ionization parameter (∼−1.3) during phase (c), compared to the other three phases (∼0.7, 0.7, and 0.5 for (a), (b), and (d), respectively).
Table 4. Best-fit Parameters for Phase-resolved Spectra in the 0.3–40.0 keV Range
Parameter | Unit | M1 | M2 | M3 | |||||||||
---|---|---|---|---|---|---|---|---|---|---|---|---|---|
(a) | (b) | (c) | (d) | (a) | (b) | (c) | (d) | (a) | (b) | (c) | (d) | ||
0.10–0.22 | 0.22–0.50 | 0.50–0.80 | 0.80–1.10 | 0.10–0.22 | 0.22–0.50 | 0.50–0.80 | 0.80–1.10 | 0.10–0.22 | 0.22–0.50 | 0.50–0.80 | 0.80–1.10 | ||
nH,tb | 1020 cm−2 | 4.5f | 4.5f | 4.5f | 4.5f | 0.7f | 0.7f | 0.7f | 0.7f | 4.7f | 4.7f | 4.7f | 4.7f |
nH,pc1 | 1022 cm−2 |
![]() |
![]() |
![]() |
![]() | ⋯ | ⋯ | ⋯ | ⋯ | ⋯ | ⋯ | ⋯ | ⋯ |
pcf1/log(xi) |
![]() |
![]() |
![]() |
![]() | ⋯ | ⋯ | ⋯ | ⋯ |
![]() |
![]() |
![]() |
![]() | |
nH,pc2/![]() | 1022 cm−2 |
![]() |
![]() |
![]() |
![]() |
![]() |
![]() |
![]() |
![]() |
![]() |
![]() |
![]() |
![]() |
pcf2/β |
![]() |
![]() |
![]() |
![]() |
![]() |
![]() |
![]() |
![]() |
![]() |
![]() |
![]() |
![]() | |
Tme | keV | <0.27 | <0.19 | 0.18f |
![]() |
![]() |
![]() | <![]() |
![]() | ⋯ | ⋯ | ⋯ | ⋯ |
Nme | 10−4 |
![]() |
![]() |
![]() |
![]() |
![]() |
![]() |
![]() |
![]() | ⋯ | ⋯ | ⋯ | ⋯ |
T1,mkc | keV | <1.28 | <0.98 | <0.90 | <0.86 | <1.41 |
![]() | <0.87 |
![]() | 0.0808f | 0.0808f | 0.0808f | 0.0808f |
T2,mkc | keV |
![]() |
![]() |
![]() |
![]() |
![]() |
![]() |
![]() |
![]() |
![]() |
![]() |
![]() |
![]() |
Nmkc | 10−11 |
![]() |
![]() |
![]() |
![]() |
![]() |
![]() |
![]() |
![]() |
![]() |
![]() |
![]() |
![]() |
Z | Z⊙ |
![]() |
![]() |
![]() |
![]() |
![]() |
![]() |
![]() |
![]() |
![]() |
![]() |
![]() |
![]() |
LineE | keV |
![]() |
![]() |
![]() |
![]() |
![]() |
![]() |
![]() |
![]() |
![]() |
![]() |
![]() |
![]() |
σ | eV | 0f | 0f | 0f | 0f | 0f | 0f | 0f | 0f | 0f | 0f | 0f | 0f |
Nline | 10−5 |
![]() |
![]() |
![]() |
![]() |
![]() |
![]() |
![]() |
![]() |
![]() |
![]() |
![]() |
![]() |
χ2(dof) | 200(203) | 393(427) | 347(304) | 503(463) | 203(205) | 399(429) | 345(305) | 506(465) | 210(207) | 405(431) | 355(307) | 502(467) |
Note. Symbols carry the same meaning as in Table 3.
Download table as: ASCIITypeset image
4. Discussion
Paloma, a unique source among IPs, has been observed simultaneously in the broadband X-rays (0.3–40 keV) for the first time. We have studied the X-ray properties of the source, and in the following section we discuss our results.
4.1. Modulations in the Light Curve
The single hump-like structure in the orbit-folded light curve covering a full cycle denotes that we are seeing emissions from at least one pole at any point of time during the orbital motion of the WD. The energy-dependent dips arise owing to the presence of a complex inhomogeneous absorber. During the dip, around phase (a) (ϕ = 0.10–0.22), we believe that the column density of the absorber is maximum along the line of sight, causing the soft X-rays below 3 keV to undergo maximum absorption. This is what causes the peak in HR1 (Figure 4) at the same phase. This absorption feature also appears in the 3–10 keV band, indicating that the column density of the absorber is very high during this phase. However, the amplitude of the dip decreases with increasing energy. The complex intrinsic absorber also describes another extra dip at ϕ ∼ 0.5 in the soft X-rays below 3 keV but does not impact the light curve above 3 keV.
The periodic absorption features present at ϕ ∼ 0.16 and 0.5 in the orbital cycle for the soft to medium X-rays (0.3–10.0 keV) of the PN light curve can be contributed from absorbing material produced by stream–disk or stream–magnetosphere interactions (Norton et al. 1996; Parker et al. 2005). However, due to the close proximity of the orbital and spin periods and the short duration of the PN light curve (covering a few spin and orbital cycles), the periodic absorption features from the accretion curtain or accretion stream arising in the spin cycle can also contribute to the features in the orbit-folded PN light curve.
The significant power at orbital frequency Ω is representative of strong periodic variation at this period. The minima at ϕ ∼ 0.7 during phase (c) is present in all the energy bands in the orbit-folded light curves, obtained from both the telescopes. The presence of a dense blob of material fixed in the orbital frame along the line of sight, capable of obstructing hard X-rays, can be a possible explanation for this feature during phase (c). We also note a relatively weaker peak at Ω in the soft 0.3–3.0 keV band with respect to the medium 3.0–10.0 keV band. This can happen because the features coming from the absorbers fixed in spin phase affect the soft X-rays (0.3–3.0 keV) in the PN light curve more dominantly and get superposed with the modulation features arising in the orbital cycle, thereby reducing the power at Ω in the soft energy band.
The earlier photometric observations revealed variation of spin modulations over a complete beat cycle. Littlefield et al. (2022) found that the spin profile changes from single-peak to double-peak structure, and they proposed pole switching or grazing eclipse. Schwarz et al. (2007) showed the change in modulations of the double-hump structure (their case B). They argued for the pole-shifting scenario. These kinds of variations in spin modulations over a beat cycle weaken the power at spin frequency in the NuSTAR power spectra (both 3–10 keV and 10–40 keV energy bands), derived from more than a beat-period long light curve. In the pole-switching case the power at the frequency peak can be shifted from ω to Ω and 2ω − Ω approximately equally (Littlefield et al. 2019). But in the NuSTAR power spectra we notice that the 2ω − Ω peak is even weaker than ω. Therefore, for a pole-switching scenario, if some power is transferred from ω to Ω in the NuSTAR power spectra, then the amount of that power should not be substantial. It indicates that the strong orbital frequency peak is a distinguishing feature for Paloma, irrespective of weaker spin frequency peak. However, this is not commonplace in IPs, since, for example, in a systematic study of several IPs by Parker et al. (2005), orbital peaks appear to be weaker than or comparable to spin peaks.
4.2. Nature of the Intrinsic Absorber and the Variation with Orbital Phase
The inherently complex nature of the intrinsic absorber is supported from spectral analysis. The simplistic scenario, implemented using two multiplicative partial covering model components as described by model M1, mathematically represents intrinsic inhomogeneous absorbers with at least three distinct column densities with their respective fractional contribution. From the fit to the phase-averaged spectra, we calculate three such column densities as 2.2 × 1022 cm−2, 20.0 × 1022 cm−2, and 22.2 × 1022 cm−2 appearing with fractional contributions of 0.30, 0.18, and 0.35 (following Equation (1)). We can physically explain this as the X-ray emission encountering the intrinsic absorbers which are mentioned in earlier subsection (Section 4.1). These absorbers are inhomogeneous and do not have a unique column density, but rather a distribution of column densities. This scenario is supported by models M2 and M3, where we assume a power-law distribution of the column densities of the intrinsic absorber. The maximum values of the column density are ∼28 × 1022 cm−2, consistent with model M1.
During orbital phase (a), the high value of the covering fractions for model M1, or the high values of the power-law indices (β) of the complex absorber for models M2 and M3, agree with the sharp dip that we see in the folded light curve. This sharp excess in absorption is contributed when a large part of emission encounters the absorber. During the other two phases (b) and (d), we obtain similar values of the covering fraction (model M1) or the β of the complex absorber (models M2 and M3). This indicates the situation when lesser and lesser amount of emission pass through the absorber, as the system rotates and during phase (c), the least value of the covering fractions (model M1) or β (model M2 and M3) are obtained.
4.3. Fe Kα Lines and Compton Reflection
The XMM-Newton EPIC spectra in Figure 5 present three Fe Kα line complexes, where the He-like Fe Kα line appears with maximum strength (equivalent width eV), and the neutral line is the weakest one (equivalent width
eV). All three lines are narrow, with line width being consistent with instrument resolution.
The contribution of the neutral Fe Kα line comes from both the intrinsic absorber and the Compton reflection of the X-rays from the WD surface (Ezuka & Ishida 1999). A strong and complex absorber, with a weak neutral Fe Kα line, suggests that the absorber is likely to be the main contributor for the strength of this line for Paloma; however, there is a possibility that some amount is contributed via Compton reflection.
In the broadband fitting, the "reflect" versions of the models (i.e., M1-R, M2-R, M3-R) display a small improvement in fit statistic. This suggests that reflection is weak in the source.
In order to check the degree of degeneracy between absorption and reflection, we plotted (Figure 8) the model spectra (using best-fit values of parameters of the M1-R fit, obtained in Table 3) when both the absorption and reflection are present and when either one of them is absent. This plot shows that the strong intrinsic absorber in Paloma have effect well up to 20 keV (where black and blue curves merge), whereas the lower end of the reflection hump tail is comfortably extended until 7 keV (where red and blue curves merge). This indicates the degeneracy of the reflection and absorption in this energy range, which can be eliminated by securely detecting the reflection hump beyond 15 keV. The model spectra show a reflection hump feature in 10–30 keV that we could not robustly detect in the observed spectra, as the improvement in fit statistics is minimal. Note that Mukai et al. (2015) have detected strong reflections in IPs. Hence, reflection in IPs is an important physical process and can be studied in detail provided that the spectral quality is such that the detection is robust.
Figure 8. Effect of complex absorber and Compton reflection in the spectral modeling. Spectral parameters from model M1-R have been used. The blue curve represents the model spectrum including both reflection and absorption. For the black curve, the absorption is set to zero, but reflection is present. The red curve denotes the spectra when absorption is present but reflection is not.
Download figure:
Standard image High-resolution imageThe reflection amplitude (Ω/2π) physically denotes the shock height (h) over the surface of the WD. Theoretically, reflection amplitude of 1 denotes that the shock is happening just over the WD surface, whereas a reflection amplitude of 0 denotes a tall shock (Mukai 2017). If we consider that there is a certain contribution of Compton reflection in Paloma, though we agree that the statistical improvement to the fit is small, we obtain physically acceptable values of the reflection amplitudes ∼0.39, 0.47, and 0.26 from models M1-R, M2-R, and M3-R, respectively. These values are much less than the value calculated by Joshi et al. (2016; ∼2.4) using XMM-Newton-only data. However, remembering the large statistical uncertainty associated with Ω/2π for any of our model variants, we refrain from deducing quantitative information, like the value of the shock height, based on that.
4.4. Case of Collisionally Excited Plasma and Photoionized Absorber
The RGS spectra show the signature of a strong O vii line. Due to the poor quality of the data, we could not detect any other lines with statistical significance. Agreeing with RGS spectra, the ionized oxygen line also appears as a small hump around 0.57 keV in the EPIC spectra and other features in the soft X-rays. These soft X-ray features are not properly taken care of by the cooling flow component, for which we modeled (models M1 and M2) them after an extra optically thin collisionally ionized plasma emission component. The temperature of this component signifies that this emission is coming from the base of the PSR.
As an alternative scenario, the photoionized warm absorber describes the soft X-ray features without the necessity of extra collisionally excited emission. Using Chandra grating data, Islam & Mukai (2021) have shown the presence of photoionized pre-shock flow for two IPs (V1223 Sgr and NY Lup). Ionization parameter (ξ = L/nr2) is defined as a ratio of ionization flux to ionization density where emission with flux L/r2 (modulo 4π) ionizes the cloud of density n at distance r. This parameter is independent of the specific mass accretion rate. The 4π factor denotes the geometrical effect for an extended cloud covering an extended source. Geometrical effects can reduce the value of the ionization parameter significantly. Based on the photoionized warm absorber model developed by Islam & Mukai (2021), we modeled our spectra using model M3 and found a similarly good fit in comparison with models M1 and M2. The phase-resolved spectral fitting using model M3 gives consistent values of ionization parameter for phases (a), (c), and (d) within statistical uncertainty. The low value for this parameter (∼−1.3) during the phase of the lowest covering fraction of complex absorber, i.e., (c), is because of the geometrical effects, like the orientation and fraction of the absorber available to be ionized.
At this point, within the limited resolution of EPIC spectra and poor signal-to-noise ratio of RGS data, the two scenarios—presence of photoionized absorption by warm pre-shock flow, and neutral absorber with extra collisionally ionized emission from the bottom of the PSR—seem equally plausible from a statistical perspective. High-quality grating data will reveal ionized emissions and absorption edges, which are important for securing the presence of a complex warm absorber.
4.5. White Dwarf Mass
The upper temperature of the cooling flow model represents the shock temperature, i.e., the temperature of the shock front on top of the PSR. For all three model variants (M1, M2, and M3), the values of this parameter are mutually consistent with each other and well constrained. Assuming the shock temperature ( keV) from model M3 (minimum value of reduced χ2), we estimate a WD mass of
and a WD radius of
. We have used the WD mass–radius relationship from Nauenberg (1972) and the formula for shock temperature with WD mass and radius (e.g., Mukai 2017). Since this value is measured from the shock temperature, obtained using broadband spectra, it is expected to give a fine estimate of the WD mass. However, for a negligible value of reflection amplitude, indicating a tall shock height, this mass can be somewhat underestimated (Lopes de Oliveira & Mukai 2019).
The unabsorbed flux in the 0.3–40.0 keV range is , of which ∼33% is contributed from the 10–40 keV band. The corresponding luminosity (0.3–40.0 keV) is ∼8.44 × 1032 erg s−1, where distance to the source d = 578 pc (Gaia Collaboration et al. 2018).
Accretion luminosity can be represented in terms of the mass accretion rate () as (Frank et al. 2002)

Using our obtained values of luminosity, mass, and radius of the WD, we calculate . This value is in line with the M⊙ seen for other asynchronous polars, for example, V1432 Aql (∼3 × 1015 g s−1; Rana et al. 2005), CD Ind (∼8 × 1014 g s−1; Dutta & Rana 2022), V1500 Cyg (∼6 × 1014 g s−1; Harrison & Campbell 2016), and BY Cam (∼6 × 1016 g s−1; Done & Magdziarz 1998).
4.6. Possibility of Formation of Disk
Past studies of Paloma (Schwarz et al. 2007; Littlefield et al. 2022) have favored a diskless scenario mainly based on the presence of a 2ω − Ω peak (Wynn & King 1992) in their periodgram obtained from photometric observation. We also notice the presence of a 2ω − Ω peak in the FPMA Lomb–Scargle power spectra (Figure 2), but that peak is much weaker compared to the Ω peak and also suppressed in CLEANed power spectra. However, the power spectra presented in Figures 2 and 3 show the presence of a Ω + 2ω peak that appears with relatively higher power in power spectra of both telescopes, as well as in the Lomb–Scargle and CLEANed algorithms. This frequency peak is among the set of frequency peaks that can be produced in a scenario with a purely disk-fed system with an asymmetric dipole condition and additional orbital modulations (like stream–disk impact) (Norton et al. 1996). There are other expected frequency peaks for this scenario, for example, ω + Ω is noticed in the FPMA power spectra (but unresolved in PN power spectra). Surprisingly, the expected lower orbital sideband counterpart ω − Ω is not noticed in the FPMA power spectra (PN power spectra are anyway unable to detect it owing to the smaller duration of the light curve), and the spin harmonic 2ω and its lower orbital sideband 2ω − Ω appear with small power in the FPMA Lomb–Scargle power spectra. Therefore, it is difficult to conclusively argue for a disk-fed or diskless system, as evidenced from our power spectra. Another alternate argument for the formation of a disk could be made by comparing the magnetospheric radius with the other binary parameters (circularization radius, distance of closest approach of freefall stream). Unfortunately, we could not do so because of the unavailability of the measured magnetic fields of the WD in Paloma, as of now.
5. Summary and Conclusions
The X-ray broadband data of Paloma reveal interesting properties of the source, some of which open up new possibilities related to the accretion mechanism for this source. In light of our current data, we summarize our findings in this section.
- 1.Emission from at least one WD pole is visible at any point during the orbital cycle. The complex intrinsic absorbers play a crucial role in the system, which is most likely contributed from the accretion curtain/accretion stream and absorbing material due to stream–disk/stream-magnetospheric interaction.
- 2.The contribution of the complex intrinsic absorber changes significantly over a complete orbital cycle with maximum contribution during orbital phase ϕ = 0.1–0.22 and minimum during phase ϕ = 0.5–0.8.
- 3.Power spectra show that the orbital frequency peak is much stronger than the spin frequency peak. Strong orbital modulation possibly resulted from presence of a dense blob fixed in the orbital frame. Literature on this source showed that spin modulations change significantly over the beat cycle, which is the reason behind weak power at spin frequency in the power spectra.
- 4.From the power spectra of the source, it is difficult to conclusively argue for the presence or absence of a partial accretion disk.
- 5.The WD mass is estimated to be
using the shock temperature, measured directly from the broadband spectra using the multitemperature emission model for the PSR.
- 6.The features in the soft X-rays can be explained with similar statistical goodness either by the extra optically thin collisionally excited cold plasma emission from the PSR or by using the photoionized absorption from the warm pre-shock. To definitively favor one possibility, good-quality high-resolution data are required in the soft to medium X-rays.
- 7.The O vii Kα emission line is noticed in soft X-rays. The neutral Fe Kα is weaker compared to ionized lines, and presumably the neutral line strength is mostly contributed from a strong intrinsic absorber. We also measured reflection amplitude but with a poor constraint, as well as a small statistical improvement to the broadband fitting, suggesting the presence of weak reflection in the system.
We would like to thank the referee for valuable comments that further improved the manuscript. This research has made use of the data obtained from the NuSTAR telescope, operated jointly by Caltech and NASA, and the XMM-Newton telescope, operated by ESA. We thank the NuSTAR science operation team and XMM-Newton science operation team for providing the data. We acknowledge the members at the helpdesk maintained by the High Energy Astrophysics Science Archive Research Center (HEASARC) and the XMM-Newton helpdesk team members for providing necessary support. A.D. thanks Nazma Islam for providing useful infomation regarding zxipab. A.D. also mentions other helps provided by T.G., H.M., and K.R. during the work.
The data used for analysis in this article are publicly available in NASA's High Energy Astrophysics Science Archive Research Center (HEASARC) archive (https://heasarc.gsfc.nasa.gov/docs/archive.html) and XMM-Newton Science archive (http://nxsa.esac.esa.int/nxsa-web/#search).
Facility: XMM-Newton - , NuSTAR - The NuSTAR (Nuclear Spectroscopic Telescope Array) mission.
Software: HEASoft (NASA High Energy Astrophysics Science Archive Research Center (Heasarc), 2014, https://heasarc.gsfc.nasa.gov/docs/software/heasoft/), XMM-Newton SAS (https://www.cosmos.esa.int/web/xmm-newton/sas), STARLINK (Currie et al. (2014) https://starlink.eao.hawaii.edu/starlink).
Footnotes
- 1
- 2
- 3
The same setting is used for subsequent spectral analysis, whenever required.