- 1Department of Horticulture, Zhejiang University, Hangzhou, China
- 2Key Laboratory of Horticultural Plants Growth, Development, and Quality Improvement, Agricultural Ministry of China, Hangzhou, China
Calvin cycle is a sequence of enzymatic reactions that assimilate atmospheric CO2 in photosynthesis. Multiple components are known to participate in the induction or suppression of the Calvin cycle but the mechanism of its regulation by phytohormones is still unclear. Brassinosteroids (BRs) are steroid phytohormones that promote photosynthesis and crop yields. In this study, we study the role of BRs in regulating Calvin cycle genes to further understand the regulation of the Calvin cycle by phytohormones in tomatoes. BRs and their signal effector BRASSINAZOLE RESISTANT 1 (BZR1) can enhance the Calvin cycle activity and improve the photosynthetic ability. BRs increased the accumulation of dephosphorylated form of BZR1 by 94% and induced an 88–126% increase in the transcription of key genes in Calvin cycle FBA1, RCA1, FBP5, and PGK1. BZR1 activated the transcription of these Calvin cycle genes by directly binding to their promoters. Moreover, silencing these Calvin cycle genes impaired 24-epibrassinolide (EBR)-induced enhancement of photosynthetic rate, the quantum efficiency of PSII, and Vc,max and Jmax. Taken together, these results strongly suggest that BRs regulate the Calvin cycle in a BZR1-dependent manner in tomatoes. BRs that mediate coordinated regulation of photosynthetic genes are potential targets for increasing crop yields.
Introduction
The crop yields need to be increased by 70% to meet the food demand of the world’s population by 2050 (Zhu et al., 2010; Simkin et al., 2019). Enhancing photosynthetic efficiency is one of the crucial approaches to increase crop yields (Long et al., 2015). Photosynthesis is composed of light reactions and dark reactions. The light reactions involve light capture, photochemical reactions, and photosynthetic electron transport chain that drives the formation of ATP and reducing equivalents. The dark reactions mainly involve a series of biochemical reactions in the Calvin cycle, which are affected by temperature, ionic concentration, and light environment. Sugars that produced by photosynthesis can be temporally stored as starch in the chloroplast during the day and consumed at night or be transported to sink tissues to support active growth. When the production of sugars exceeds plants’ demand, the metabolites can feedback downregulate the photosynthetic capacity (Paul and Pellny, 2003). In addition, stomata also influence photosynthesis by gating the entry of CO2 into the chloroplast (Lawson and Vialet-Chabrand, 2019; Xiong and Flexas, 2020).
The light reactions and Calvin cycle are two mechanistically different but closely coupled parts. It was reported that the enzymes in the Calvin cycle were activated by reduced thioredoxin (Trx) produced by the photosynthetic electron and proton transport chain (Cheng et al., 2014; Okegawa and Motohashi, 2015). Ruuska et al. (2000) found that decreasing the amount of cytochrome b/f complex led to a decrease in the intermediate metabolites in the Calvin cycle (Ruuska et al., 2000). Meanwhile, a missense mutation of the large subunit of Rubisco interrupted the Calvin cycle and suppressed the de novo synthesis of D1 and D2 proteins (Takahashi and Murata, 2005). Recently, abundant evidence shows that the regulation of enzyme activity in the Calvin cycle is a key way for crops to improve photosynthetic efficiency (Lin et al., 2014; Simkin et al., 2015, 2017; Driever et al., 2017; Lopez-Calcagno et al., 2020). For example, in tobacco, overexpression of sedoheptulose-1,7-bisphosphatase (SBPase), fructose-1,6-bisphosphate aldolase (FBPase), and cyanobacterial putative inorganic carbon transporter B (ictB) had a positive effect on the photosynthetic rate and biomass accumulation. Besides, altering Rubisco properties by inserting the genes of the Se7942 enzyme and RbcX or CcmM35 can activate Rubisco and increase CO2 assimilation in tobacco.
Brassinosteroids (BRs) are a group of steroid hormones that play essential roles in plant growth and development (Nolan et al., 2020). It was known that BRs could induce a phosphorylation cascade and ultimately activate the BR signaling effector, BRASSINAZOLE RESISTANT1 (BZR1) and BRI1 EMS SUPPRESSOR1 (BES1), which are responsible for most of the BR responses (Kim and Russinova, 2020). Activated BZR1 and BES1 can bind to the E-box (CANNTG) and BRRE motif (CGTGT/CG) in the promoters of BR-responsive genes and regulate their expression (He et al., 2005; Yin et al., 2005). Enhancing the endogenous BR biosynthesis specifically in vegetative tissues enlarged the sugar pools in flag leaves and promoted grain filling and yield in rice (Wu et al., 2008). Furthermore, exogenous EBR treatment enhances the plant fitness in response to the suboptimal light and temperature environments (Shu et al., 2016) and nutrient stresses (dos Santos et al., 2020, 2021b). Brassinosteroids can also increase the yields of monocot and dicot crops (Vriet et al., 2012). It is well known that the increase of crop yields is largely attributed to the promotion of photosynthesis. We previously found that BRs enhance photosynthetic CO2 assimilation by regulating the ribulose-1,5-bisphosphate (RuBP) carboxylation and regeneration (Yu et al., 2004). Specifically, BRs can activate Rubisco, the rate limiting enzyme of RuBP carboxylation (Spreitzer and Salvucci, 2002). However, whether BRs regulate other enzymes in the Calvin cycle remains unclear and the underlying molecular mechanism needs further studies.
In this study, we show that the BR signaling effector BZR1 plays a crucial role in the regulation of photosynthesis. Enhancement of endogenous BR levels led to increased stability of BZR1, which directly activated the transcription of several Calvin cycle genes including FBA1, RCA1, FBP5, and PGK1 by binding to their promoters. The increase in the transcript abundance of Calvin cycle genes led to the promotion of RuBP carboxylation and regeneration. Meanwhile, silencing of these Calvin cycle genes resulted in the decrease of RuBP carboxylation and regeneration and subsequently compromised BR-induced photosynthesis. In summary, these results provide strong evidence that BRs enhance photosynthesis through BZR1-mediated transcriptional regulation of the Calvin cycle in tomato plants.
Materials and Methods
Plant Materials
The tomato (Solanum lycopersicum L.) genotype ‘‘Condine Red’’ was used as the wild type (WT) in this study. Its BR synthesis impaired point mutant dwf (accession number LA0571), which has a mutation in the BR biosynthetic gene DWARF, was obtained from the Tomato Genetics Resource Center (University of California, Davis, CA, United States).1 Its BR synthesis increased transgenic plant DWF: OE was obtained as described before (Li et al., 2016a). 35Spro:BZR1-HA (transgenic line with HA tag) overexpression line and CRISPR/Cas9 transgenic lines of bzr1 generated in our laboratory were used in this study (Yin et al., 2018; Wang et al., 2019).
The virus-induced gene silencing (VIGS) constructs for silencing of FBA1, RCA1, FBP5, and PGK1 genes were generated by PCR amplification using specific primers (Supplementary Table 1), digested by EcoRI and KpnI, and ligated into empty TRV2 vector who was digested by EcoRI and KpnI, too. The recombined plasmids were transformed into A. tumefaciens strain GV3101 through electroporation. A. tumefaciens-mediated infection was executed as described before (Ekengren et al., 2003). Plants infected by empty TRV2 vector were used as controls in this study, named TRV. All the infected plants were kept at 23°C for 5 weeks before use. Leaflets whose transcript levels were less than 30% of the TRV plants were used in this study (Supplementary Figure 1).
Growth Conditions and Treatments
Tomato seeds were shaken in a flask at 28°C for 2 days and then sowed into a tray that contains a mixture of peat and vermiculite (2:1/vol:vol). Seedlings were watered with Hoagland nutrition solution every 2 days. When the first true leaf was fully expanded, seedlings were transplanted into plastic pots containing the same matrix and watered with Hoagland nutrition solution every day. The growth conditions in the growth chambers were as follows: a 12-h photoperiod, the temperature of 23°C/20°C (day/night), and PPFD of 300 μmol/m2/s photosynthetic photon flux density. Five-week-old seedlings were used for subsequent experiments. The photographs of the phenotype were also taken at this time.
To study how exogenous BR treatment effects photosynthesis, seedlings were sprayed with 24-epibrassinolide (EBR, 200 nM, Sigma, United States) at 8:00 a.m. As EBR was dissolved in ethanol beforehand, seedlings sprayed with distilled water containing the same amount of ethanol were used as controls. Leaf samples were collected 6 h after treatment and frozen in liquid nitrogen immediately. As for seedlings that were used for control, samples were collected at the same time as those EBR-treated plants. All the samples taken were later used for protein extraction or analysis of gene expression.
Leaf Gas Exchange and Chlorophyll Fluorescence Measurements
The fourth leaf (from top to down) of seedlings at the seven-leaf stage was used for all the measurements in this study. Gas exchange measurements were performed by a portable photosynthesis measurement system (LI-6400; LI-COR, Lincoln, NE, United States) with a fluorescent light source. All the measurements were taken between 8:00 a.m. and 8:00 p.m. The air temperature, relative humidity, CO2 concentration, and PPFD used for measurements were as follows: 23°C, 85%, 440 ± 20 μmol/mol and 1,000 μmol/m2/s photosynthetic photon flux density and kept for all the cases unless otherwise stated. The CO2 assimilation vs. intercellular CO2 concentration (A/Ci) curves were fitted as described before (von Caemmerer and Farquhar, 1981). The setting of the CO2 concentrations of the A/Ci curves was as follows: 400 μmol/mol at first and then decreased gradually to 50 μmol/mol, afterward returned to 400 μmol/mol for stability and then increased stepwise to 1,500 μmol/mol. The maximum ribulose-1,5-bisphosphate carboxylase/oxygenase (Rubisco) carboxylation rate (Vc,max) and maximum ribulose-1,5-bisphosphate(RuBP) regeneration rate (Jmax) were estimated according to the A/Ci curves using the method described before (Dubois et al., 2007).
The quantum efficiency of PSII (ΦPSII), photochemical quenching coefficient (qP), and the efficiency of excitation energy captured by open PSII centers (Fv′/Fm′) were measured using LI-6400 according to the manuscripts supplied by LI-COR. The maximum quantum yield of PSII (Fv/Fm) was measured as described before, using the Dual-PAM-100 system (Walz, Germany) (Li et al., 2016b). Before Fv/Fm was measured, seedlings were put in darkness for adaption for 30 min. For seedlings treated with EBR, gas exchange and chlorophyll fluorescence were measured 24 h after treatment, and A/Ci curves were measured 48 h after treatment.
Total RNA Extraction and Gene Expression Analysis
Total RNA was extracted from the second leaf (from top to down) of a seven-leaf seedling, following the method described previously (Wang et al., 2020). Total RNA (1 μg) was reverse-transcribed into cDNA as described before (Hu et al., 2021).
Quantitative real-time PCR (qRT-PCR) analysis was conducted in a Light Cycler 480 II real-time PCR system (Roche, Basel, Switzerland) using ChamQ Universal SYBR qPCR Master Mix kits (Vazyme Biotech Co., Ltd., Nanjing, China). Each reaction (20 μL) consists of 10 μl SYBR qPCR Master Mix, 8.2 μl ddH2O, 1 μl cDNA, 0.4 μl forward and reverse primer. The PCR condition was started with predenaturation at 95°C for 3 min, followed by denaturation at 95°C for 30 s for 40–45 cycles and then annealing at 58°C for 30 s and extension at 72°C for 1 min. The Actin and α-Tubulin 3 genes were used as internal controls. Gene-specific primers used in this study are shown in Supplementary Table 2. Relative gene expression was estimated as described previously (Livak and Schmittgen, 2001).
Protein Extraction and Western Blotting
For protein extraction, 0.1 g of leaf samples was harvested and ground into powder in liquid nitrogen. Then, the powder was homogenized in 300 μl 2 × loading buffer containing 0.1 M Tris-Base (pH = 6.8), 10% SDS, 20% glycerol, and the appropriate amount of bromophenol blue. In total, 100 mM dithiothreitol was added just before the use. After fully vortexed, the mixture was heated at 95°C for 10 min and then centrifugated at 4°C, 13,000 g for 10 min. Then, the supernatant was transferred into a new 1.5-ml tube and centrifugated at 4°C, 13,000 g for another 5 min. Subsequently, the extracted proteins were separated by 10% SDS-PAGE and transferred to a nitrocellulose membrane. After that, the membrane was blocked overnight at 4°C in TBST buffer (20 mM Tris, pH 7.5, 150 mM NaCl, and 0.1% Tween 20) with 5% BSA (Amresco 0332). After being washed in TBST at room temperature for half an hour, the membrane was incubated in TBST buffer with 1% BSA containing a rabbit anti-BZR1 multiclonal antibody (Labgic Technology Co., Ltd., Hefei, China) for another 12 h. The secondary antibody was goat anti-rabbit horseradish peroxidase (HRP)-linked antibody (Cell Signaling Technology, Danvers, MA, United States). When the incubation finished, the signal on the membrane was observed following the method described before (Chi et al., 2020).
Yeast One-Hybrid Assays
Yeast one-hybrid (Y1H) screening assay was conducted using Matchmatch Gold Yeast One-Hybrid System (Clontech) as described before (Fang et al., 2019). The promoters of FBA1, RCA1, FBP5, and PGK1 and the full-length coding region of BZR1 was amplified with specific primers (Supplementary Table 3) and ligated into the pAbAi vector and the pGADT7 vector, respectively. Combined pAbAi vectors were linearized and transformed to Y1H Gold yeast strain together with empty AD vector or pGADT7-BZR1. The transformed yeast cells were screened on SD/Leu-media supplemented with 50 ng/ml or 60 ng/ml aureobasidin A (AbA).
Dual-Luciferase Assay
Dual-luciferase assay was performed according to the previous study (Min et al., 2012). The full-length coding region of BZR1 was amplified and inserted into the pGreen II 0029 62-SK vector whereas the promoters of FBA1 (1513 bp), RCA1 (1953 bp), FBP5 (1978 bp), and PGK1 (2017 bp) were cloned and fused into pGreen II 0800-LUC vector. Primers used for conducting vectors are supplied in Supplementary Table 4. Both vectors were electroporated into A. tumefaciens strain GV3101. Agrobacterium cultures were infiltrated in MES buffer (10 mM MES, 10 mM MgCl2, 150 mM acetosyringone, pH 5.6) and adjusted to an OD600 varied from 0.75 to 0.8. Afterward, agrobacterium culture mixtures of transcription factor and genes (10:1/vol:vol) were infiltrated into Nicotiana benthamiana leaves through needle-less syringes. Three days after infiltration, tobacco leaf disks were taken and used for the analysis of the enzyme activity of firefly luciferase (LUC) and renilla luciferase (REN). The empty SK vector mixed with the gene promoters was considered as controls, and the whole test system was performed using the dual-LUC reporter assay system (Promega, Madison, WI, United States).
Chromatin Immunoprecipitation Assay
Chromatin immunoprecipitation (ChIP) assays were performed with an EpiQuik™ Plant ChIP Kit (Epigentek, Farmingdale, NY, United States) as described previously (Fang et al., 2019). Briefly, 1.5 g of leaf samples was collected from 5-week-old BZR1: OE and WT plants. After being fixed in 1% formaldehyde, chromatin was isolated and sonicated into pieces from 150 to 1,000 bp. BZR1 protein with pieces of DNA on it was immunoprecipitated by an anti-HA antibody (Pierce; Rockford, IL, United States). The enriched DNA was amplified by qPCR using specific primers (Supplementary Table 5). Enrichment was calculated by the radio of the immunoprecipitated DNA relative to the input. The goat anti-mouse IgG antibody (Millipore, Darmstadt, Germany) was used as the negative control.
Statistical Analysis
At least three independent biological replicates sampled from different plants were used for each determination. Statistical analysis of the bioassays was performed with SPSS statistical software (version 19.0, SPSS Inc., Chicago, IL, United States). ANOVA was used to test for significance. The means between different treatments or genotypes were evaluated by Tukey’s test at a level of p < 0.05.
Accession Numbers
Sequence data for the genes studied in this work are available in Sol Genomics Network2 under the following accession numbers: Actin (Solyc11g005330), α-Tubulin 3 (Solyc08g006890), FBA1 (Solyc01g110360), FBA2 (Solyc02g062340), FBA3 (Solyc02g084440), FBA4 (Solyc05g008600), FBA5 (Soly10g054390), FBA6 (Solyc07g065900), FBA7 (Solyc09g009260), FBA8 (Solyc1 0g083570), FBA9 (Solyc10g054380), FBA11 (Solyc09g059040), PGK1 (Solyc07g066610), PGK2 (Solyc07g066600), PGK3 (Solyc0 9g008130), SBP (Solyc05g052600), RCA1 (Solyc09g011080), RCA2 (Solyc10g086580), RCA3 (Solyc03g117850), FBP1 (Solyc1 2g056530), FBP2 (Solyc04g071340), FBP3 (Solyc09g011810), FBP4 (Solyc10g086730), FBP5 (Solyc10g086720), FBP6 (Solyc01g106010).
Results
Brassinosteroids Enhance the Photosynthetic Capacity of Tomato Plants
The dwf mutant we used in this research is allelic to the strong BR-deficient mutant dx that is impaired in the BR biosynthesis gene DWARF (DWF) (Bishop et al., 1999). Additionally, it contains a point mutation in the right border of the 8th intron of DWF (from “ag” to “tg”) which affects the mRNA splicing and ultimately leads to protein conformation change (Figure 1A). As indicated by our previous study, the dwf mutant contained decreased levels of endogenous BR (Li et al., 2016b). Corresponding to this, the dwf mutant showed significant inhibition of plant growth, while overexpression of DWF (DWF: OE) improved the plant growth (Figure 1B). The growth correlated well with the net photosynthetic rate (Pn) measured at the ambient CO2, with the Pn promoted by increased endogenous BR levels (Figure 1C). Similar to Pn, the stomatal conductance (Gs) and transpiration rate (Tr) were significantly decreased in dwf plants and were increased in DWF: OE plants (Supplementary Figures 2A,C). However, the intercellular CO2 concentration (Ci) was not significantly affected by BRs (Supplementary Figure 2B).
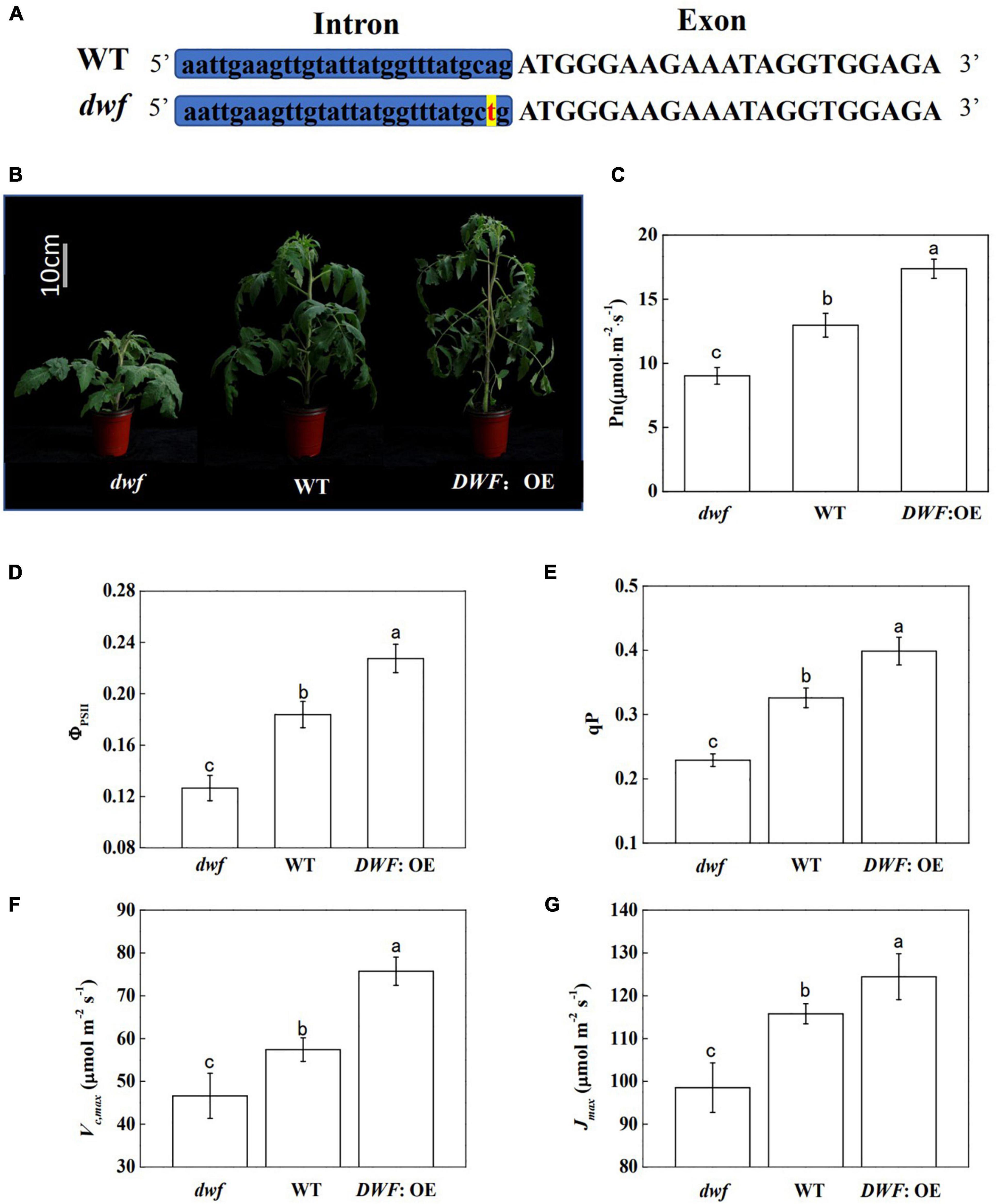
Figure 1. Influence of brassinosteroids (BRs) on plant growth and photosynthesis in tomato plants. BR-deficient mutant (dwf), wild type (WT; “Condine Red”), and plants overexpressing the BR biosynthetic DWARF gene (DWF: OE) were used for this study. Five-week-old plants were used for photographs taken and parameters measured in this analysis. (A) The mutation site of dwf. (B) Plant phenotype. (C) Net photosynthetic rate (Pn). (D) The actual quantum yield of PSII (ΦPSII). (E) Photochemical quenching coefficient (qP). (F) Maximum ribulose-1,5-bisphosphate carboxylase/oxygenase (Rubisco) carboxylation rate (Vc,max). (G) Maximum ribulose-1,5-bisphosphate (RuBP) regeneration rate (Jmax). For (C–G), data are the means ± SD of four biological replicates. Different letters represent significant differences (p < 0.05) according to Tukey’s test. Three independent experiments were performed with similar results.
Consistent with the Pn, the actual quantum efficiency of PSII (ΦPSII) in dwf plants was decreased by 31.1%, while the ΦPSII of DWF: OE was increased by 23.8% compared to WT (Figure 1D). Photochemical quenching coefficient (qP) showed similar changes to ΦPSII (Figure 1E), whereas the maximum photochemical efficiency of PSII under dark and light (Fv/Fm and Fv′/Fm′), which reflects the intrinsic activity of PSII, was not affected by BR levels (Supplementary Figure 3). Then, we evaluated the photosynthetic capacity of plants with different BR levels by analyzing the curve of CO2 assimilation rate vs. Ci (A/Ci). The in vivo maximum Rubisco carboxylation rate (Vc,max) and RuBP regeneration rate (Jmax) can be determined by fitting the A/Ci curve according to von Caemmerer and Farquhar’s model (von Caemmerer and Farquhar, 1981). The results showed that defects in BR biosynthesis inhibited Vc,max and Jmax, whereas overexpression of DWF significantly increased Vc,max and Jmax compared to WT (Figures 1F,G). Taken together, these results indicated that endogenous BR levels enhance photosynthetic capacity, possibly by regulating the Calvin cycle.
BRASSINAZOLE RESISTANT 1 Is Involved in the Brassinosteroid-Mediated Enhancement of Photosynthesis
BRASSINAZOLE RESISTANT 1 (BZR1), as the central transcription factor of the BR signaling pathway, determined the BR signaling output by regulating the expression of hundreds of BR-responsive genes (Wang et al., 2006). In this study, we found that the protein abundance of BZR1, particularly the active dephosphorylated form dBZR1, was decreased in the BR-deficient dwf mutant, but was increased by the overexpression of DWF (Figure 2A). Similar to the dwf mutant, the loss of function bzr1 mutant showed a decline in photosynthetic capacity, as indicated by lower Pn. Additionally, unlike WT plants, this decline cannot be recovered by 24-epibrassinolide (EBR) application (Figure 2B). Similar to Pn, the Gs and Tr were lower in bzr1 plants and barely response to EBR treatment (Supplementary Figures 4A,C). However, the intercellular CO2 concentration (Ci) was not significantly affected by BZR1 levels or EBR treatment (Supplementary Figure 4B).
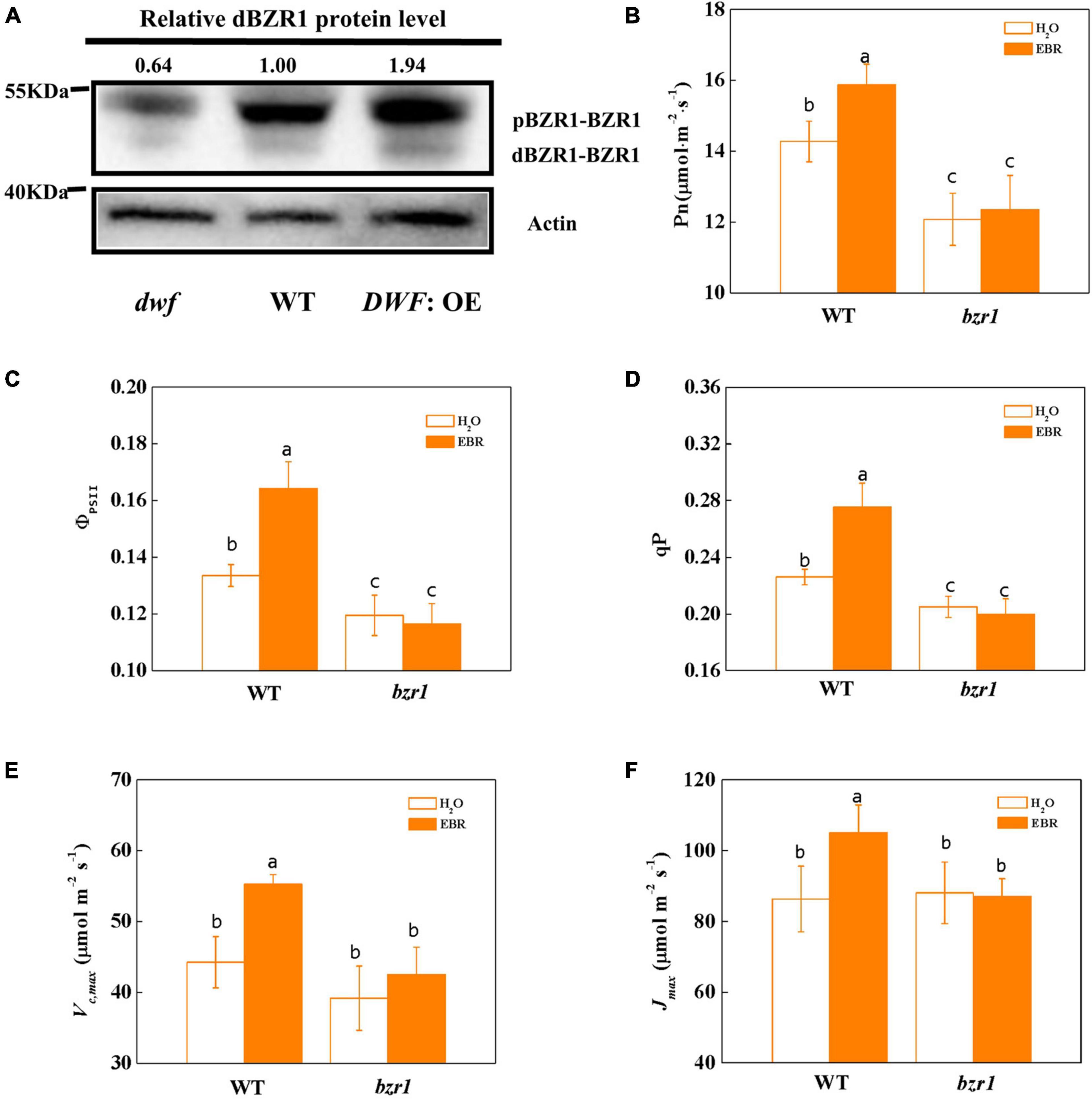
Figure 2. Influence of BZR1 on photosynthesis in tomato plants. (A) Induction of dephosphorylation of BZR1 by endogenous BRs. The phosphorylated and dephosphorylated forms of BZR1 are termed by pBZR1 and dBZR1, respectively. Total proteins were probed using an anti-BZR1 multiclonal antibody. The ratio reflects the relative abundance of dBZR1 to total protein, expressed as a number. Actin was used as the loading control. The signal intensity of dBZR1 protein was analyzed with Image Lab. The dBZR1 level of WT plants was set as one. (B) Net photosynthetic rate (Pn). (C) The actual quantum yield of PSII (ΦPSII). (D) Photochemical quenching coefficient (qP). (E) Maximum ribulose-1,5-bisphosphate carboxylase/oxygenase (Rubisco) carboxylation rate (Vc,max). (F) Maximum ribulose-1,5-bisphosphate (RuBP) regeneration rate (Jmax). Five-week-old bzr1 mutant and wild type (WT; “Condine Red”) plants were used for this analysis. EBR (200 nM) was applied at 8:00 in the morning. For (B–D), values were measured 24 h after EBR treatment. For (E,F), values were measured 48 h after EBR treatment. For (B–F), the data represent the means ± SD of at least three biological replicates. Different letters represent significant differences (p < 0.05) according to Tukey’s test. Three independent experiments were performed with similar results.
BRASSINAZOLE RESISTANT 1 deficiency also resulted in the impaired quantum efficiency of PSII. There was a decline of the ΦPSII and qP in bzr1 mutant compared to WT plants. Consistent with the enhancement of photosynthesis in DWF: OE plants, treatment with EBR promoted the ΦPSII and qP in WT plants, but had no promotion effect on bzr1 mutant (Figures 2C,D). Consistent with our earlier findings, both Fv/Fm and Fv′/Fm′ were not affected by BZR1 levels or the application of exogenous EBR (Supplementary Figure 5). Besides, Vc,max and Jmax in two plant materials showed no significant difference under control conditions but were improved only in WT plants after EBR treatment (Figures 2E,F).
BRASSINAZOLE RESISTANT 1 Regulates the Transcription of Calvin Cycle Genes
To get a better insight into the mechanism by which BRs regulated photosynthesis, we analyzed the transcription of genes encoding Calvin cycle enzymes, including Rubisco activase (RCA) (3), glycerate-3-phosphate kinase (PGK) (3), fructose-1,6-bisphosphate aldolase (FBA) (10), sedoheptulose-1,7-bisphosphatase (SBPase) (1), and fructose 1,6-bisphosphatase (FBPase) (6). Among these genes, more than half were induced in DWF: OE plants, with the accumulation of transcripts increased by 32%-295% as compared to WT plants. Notably, the expression of FBA1, PGK1, RCA1, RCA3, FBP1, FBP2, and FBP5 was consistently suppressed in the dwf mutant (Figure 3A). EBR treatment also induced a 24–103% increase in the transcription of FBA1, FBA3, FBA4, FBA5, FBA7, FBA8, FBA9, FBA11, PGK1, RCA1, FBP2, FBP4, and FBP5, and FBP5 was most significantly induced by EBR. Among these EBR-induced Calvin cycle genes, FBA1, FBA7, FBA8, FBA9, PGK1, RCA1, and FBP5 could not be transcriptionally induced in bzr1 mutant (Figure 3B). In combination with those genes regulated by endogenous BR levels, FBA1, RCA1, FBP5, and PGK1 were thought to be regulated by BZR1-mediated BR signaling and were selected for further study.
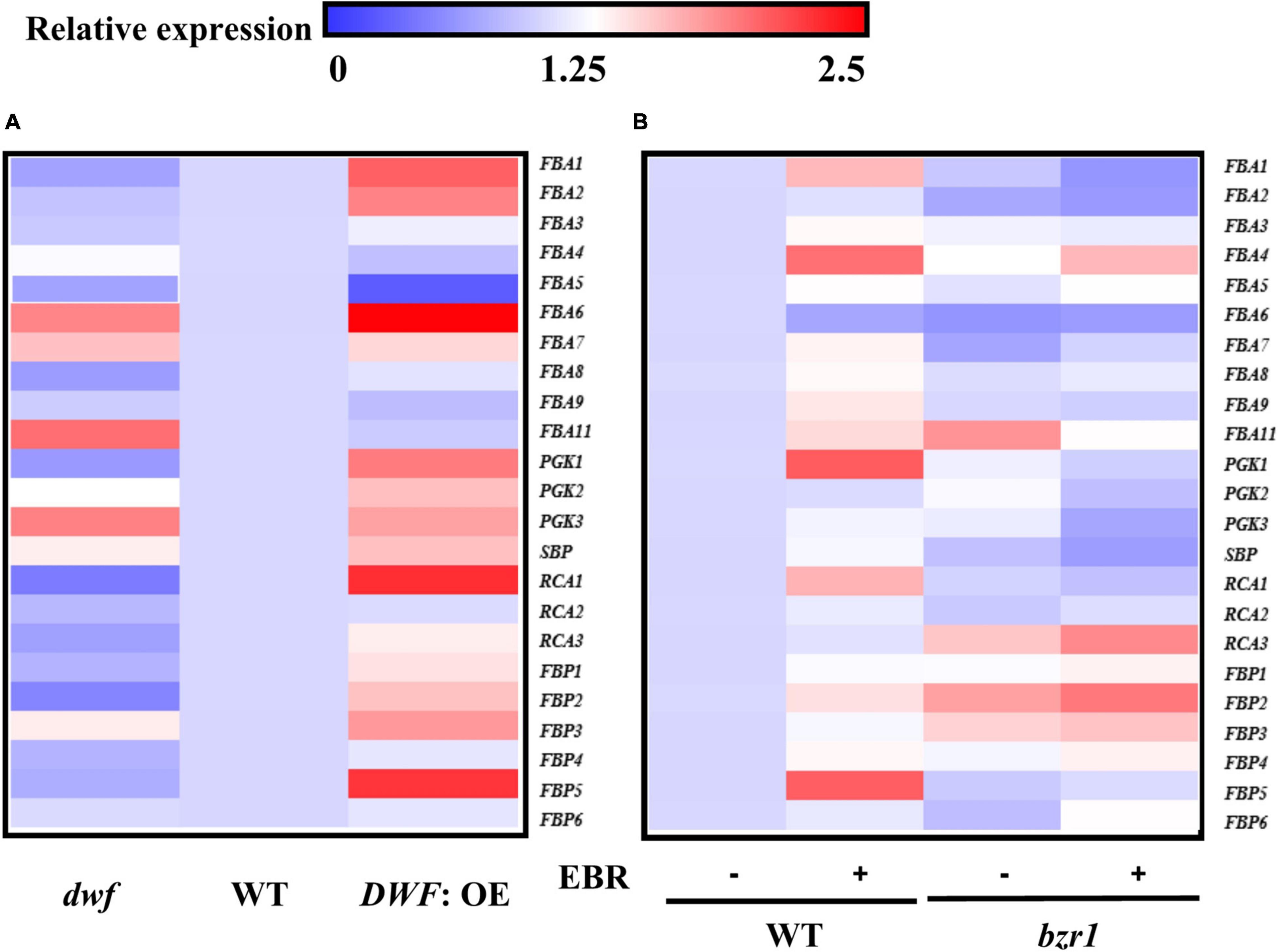
Figure 3. Influence of brassinosteroids (BRs) and BZR1 levels on the transcription of Calvin cycle genes. (A) Transcription of Calvin cycle genes in different endogenous BR levels plants. (B) Transcription of Calvin cycle genes in different BZR1 levels plants. EBR (200 nM) was applied at 8:00 in the morning. Overall, 6 h after the application, leaf samples were collected for gene expression analysis. Actin and α-tubulin 3 were used as the reference genes here. The data of transcription of genes represent the means ± SD of three biological replicates. Three independent experiments were performed with similar results.
To further detect the regulation of Calvin cycle genes by BZR1, the promoter regions (∼2 kb) located upstream of the transcriptional start sites of FBA1, RCA1, FBP5, and PGK1 were analyzed and found to contain 4∼11 E-box motifs (CANNTG), the dBZR1 binding sites (Figure 4A). The yeast one-hybrid assay showed that the yeast cells that contained the bait vector combined with the promoter regions of FBA1, RCA1, FBP5, and PGK1 grew on the selective medium when transformed with BZR1-AD; however, when transformed with the empty pGADT7 vector, the yeast cells did not grow on the same selective medium (Figure 4B). This indicates that BZR1 could directly bind to the promoters of FBA1, RCA1, FBP5, and PGK1 in vitro. The BZR1 binding to these genes was confirmed by dual-luciferase assays. As shown in Figure 4C, the promoter activity of FBA1, RCA1, FBP5, and PGK1 was induced by 1.5- to 2.2-folds by transient overexpression of BZR1. Furthermore, a ChIP-qPCR assay was used to verify the BZR1 binding to the promoters of FBA1, RCA1, FBP5, and PGK1 in vivo. As shown in Figure 4D, the promoter sequences of FBA1, RCA1, FBP5, and PGK1 were significantly enriched by immunoprecipitation via an anti-HA antibody in plants overexpressing the HA-BZR1 recombinant protein but not in WT plants. However, no difference in the efficiency of the pull-down of the promoter sequences was found using the IgG control antibody. Taken together, these results demonstrate that BRs upregulated the transcription of Calvin cycle genes such as FBA1, RCA1, FBP5, and PGK1 via BZR1 binding to their promoters.
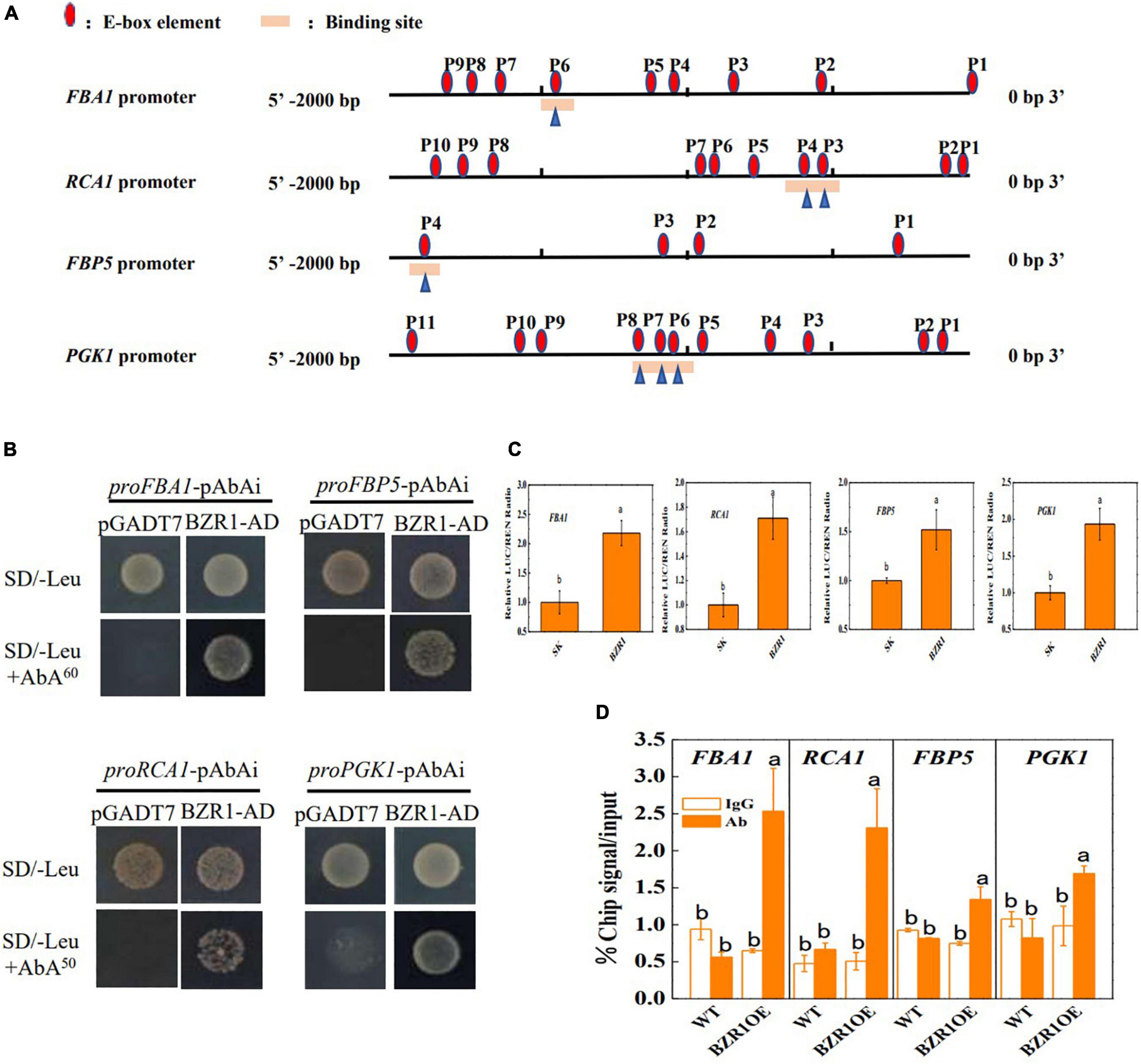
Figure 4. BZR1 binds to the promoters of FBA1, RCA1, FBP5, and PGK1 in vitro and in vivo. (A) E-box elements in the promoters of FBA1, RCA1, FBP5, and PGK1 genes. The numbering is counted from the predicted transcriptional start sites. (B) Yeast one-hybrid (Y1H) experiment showing the binding of BZR1-AD to the promoters of FBA1, RCA1, FBP5, and PGK1. Binding sites of every gene were shown in (A). (C) Dual-luciferase assay for the regulatory effect of BZR1 on the expression of FBA1, RCA1, FBP5, and PGK1. The ratio of LUC/REN of the combination of empty SK vectors mixed with the FBA1, RCA1, FBP5, and PGK1 promoters was set as one. (D) Direct binding of BZR1 to the promoters of FBA1, RCA1, FBP5, and PGK1 by ChIP–qPCR in 35S-BZR1-HA overexpressing (BZR1: OE) plants. Data present the means of three replicates ± SD. Different letters indicate significant differences (p < 0.05) according to Tukey’s test. Three independent experiments were performed with similar results.
Silencing of FBA1, RCA1, FBP5, and PGK1 Compromised Brassinosteroid-Induced Photosynthesis
To further clarify whether BRs promote photosynthesis through transcriptional regulation of Calvin cycle genes, the transcription of BZR1-regulated Calvin cycle genes was suppressed through VIGS. The efficiency of gene silencing was confirmed by RT-qPCR and shown in Supplementary Figure 1. The results showed that the suppression of transcription of FBA1, RCA1, FBP5, and PGK1 led to stunted growth and inhibition of photosynthesis (Figure 5 and Supplementary Table 6). Pn, ΦPSII, and qP were significantly decreased by gene silencing, whereas Gs, Tr, and Ci were not significantly affected (Figures 5B–D and Supplementary Figure 6). In addition, Fv/Fm and Fv′/Fm′ were not affected by gene silencing, indicating that inhibition of FBA1, RCA1, FBP5, and PGK1 did not result in damages to PSII (Supplementary Figure 7). EBR application enhanced the Pn, ΦPSII, and qP in TRV control plants; however, it had no influence on FBA1-, RCA1-, FBP5-, or PGK1- silencing plants. Interestingly, EBR-induced increase in Gs and Tr was not affected by the silencing of FBA1. Meanwhile, silencing of FBA1, RCA1, FBP5, and PGK1 compromised EBR-mediated increase in both Vc,max and Jmax (Figure 6A,B). These results indicated that transcriptional regulation of Calvin cycle genes is involved in BR-induced enhancement of photosynthesis.
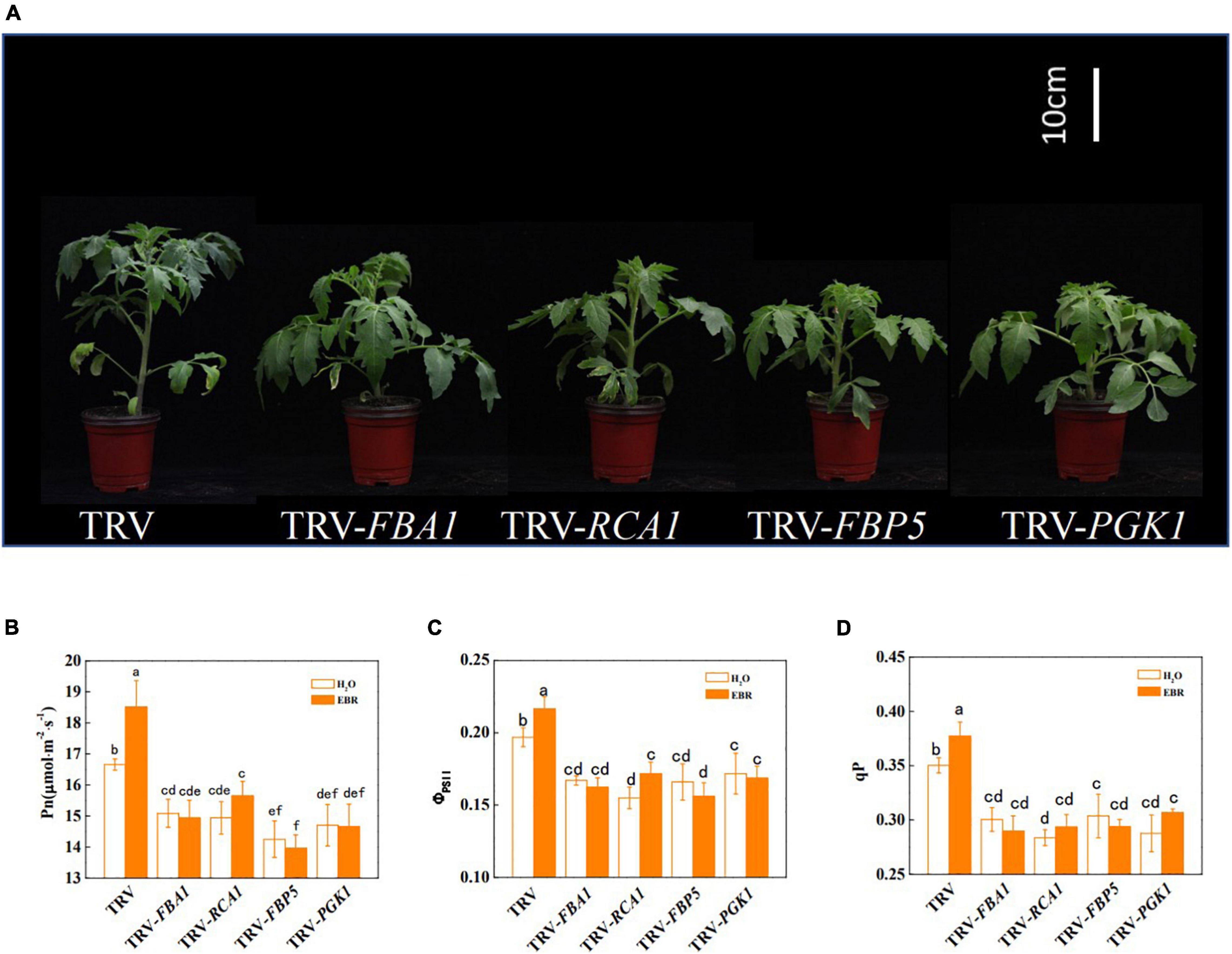
Figure 5. Influence of FBA1, RCA1, FBP5, and PGK1 silencing on plant growth and photosynthesis in tomato plants. (A) The phenotype of FBA1-, RCA1-, FBP5-, and PGK1-silencing plants. (B) Net photosynthetic rate (Pn). (C) The actual quantum yield of PSII (ΦPSII). (D) Photochemical quenching coefficient (qP). Five-week-old plants were used for photographs taken and parameters measured in this analysis. Plants, which were infected with an empty TRV2 vector named TRV, were used as controls here. EBR (200 nM) was applied at 8:00 in the morning. For (B–D), values were measured 24 h after EBR treatment. Data are the means ± SD of four biological replicates. Different letters represent significant differences (p < 0.05) according to Tukey’s test. Three independent experiments were performed with similar results.
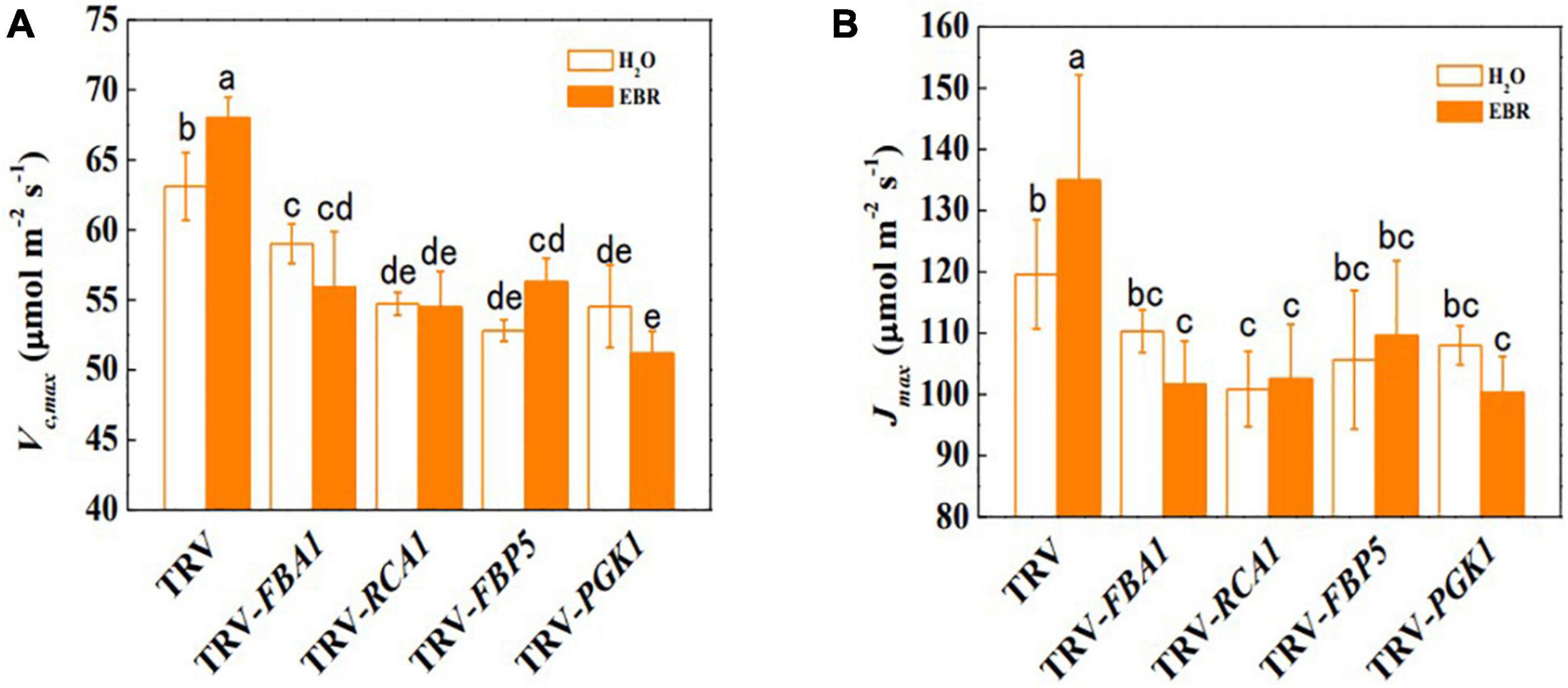
Figure 6. FBA1, RCA1, FBP5, and PGK1 are important in the BR-induced Calvin cycle. (A) Maximum ribulose-1,5-bisphosphate carboxylase/oxygenase (RuBisco) carboxylation rate (Vc,max). (B) Maximum ribulose-1,5-bisphosphate (RuBP) regeneration rate (Jmax). Five-week-old plants were used in this analysis. EBR (200 nM) was applied at 8:00 in the morning. For (A) and (B), values were measured 48 h after EBR treatment. Data are the means ± SD of four biological replicates. Different letters represent significant differences (p < 0.05) according to Tukey’s test. Three independent experiments were performed with similar results.
Discussion
In this study, we provide evidence that BZR1-mediated BR signaling enhanced the photosynthetic capacity of tomato plants by transcriptional regulation of Calvin cycle genes (Figure 7). BRs enhance crop yields by regulating multiple aspects of plant growth and development (Tong and Chu, 2018). Moreover, photosynthesis as the basis of crop yields is also regulated by BRs. The inhibition of BR biosynthesis leads to the decrease in CO2 assimilation and the suppression of carbohydrate metabolism (Li et al., 2016b), whereas enhancing the BR biosynthesis increases the sugar pools in leaves and was thought to stimulate the carbon to flow into yield-forming organs (Li et al., 2016a). In this study, we studied the role of BR signaling in the regulation of the Calvin cycle. First, BRs enhanced photosynthetic rate, the quantum efficiency of PSII, and Vc,max and Jmax which indicate the state of the Calvin cycle (Figures 1C–G). Second, BRs induced the accumulation of BZR1 (Figure 2A), the central effector of BR signaling, and BZR1 was involved in BR-induced enhancement of photosynthetic capacity (Figures 2B–F). Third, BRs and BZR1 regulated the transcription of Calvin cycle genes such as FBA1, RCA1, FBP5, and PGK1 (Figures 3A,B). Meanwhile, BZR1 can directly bind to the promoters of these Calvin cycle genes and activate their expression (Figure 4). Besides, decreasing the transcription of FBA1, RCA1, FBP5, and PGK1 by VIGS abolished BR-enhanced photosynthesis (Figures 5B–D, 6A,B). All these results demonstrate that BR signaling transcriptionally activates the Calvin cycle in response to photosynthesis.
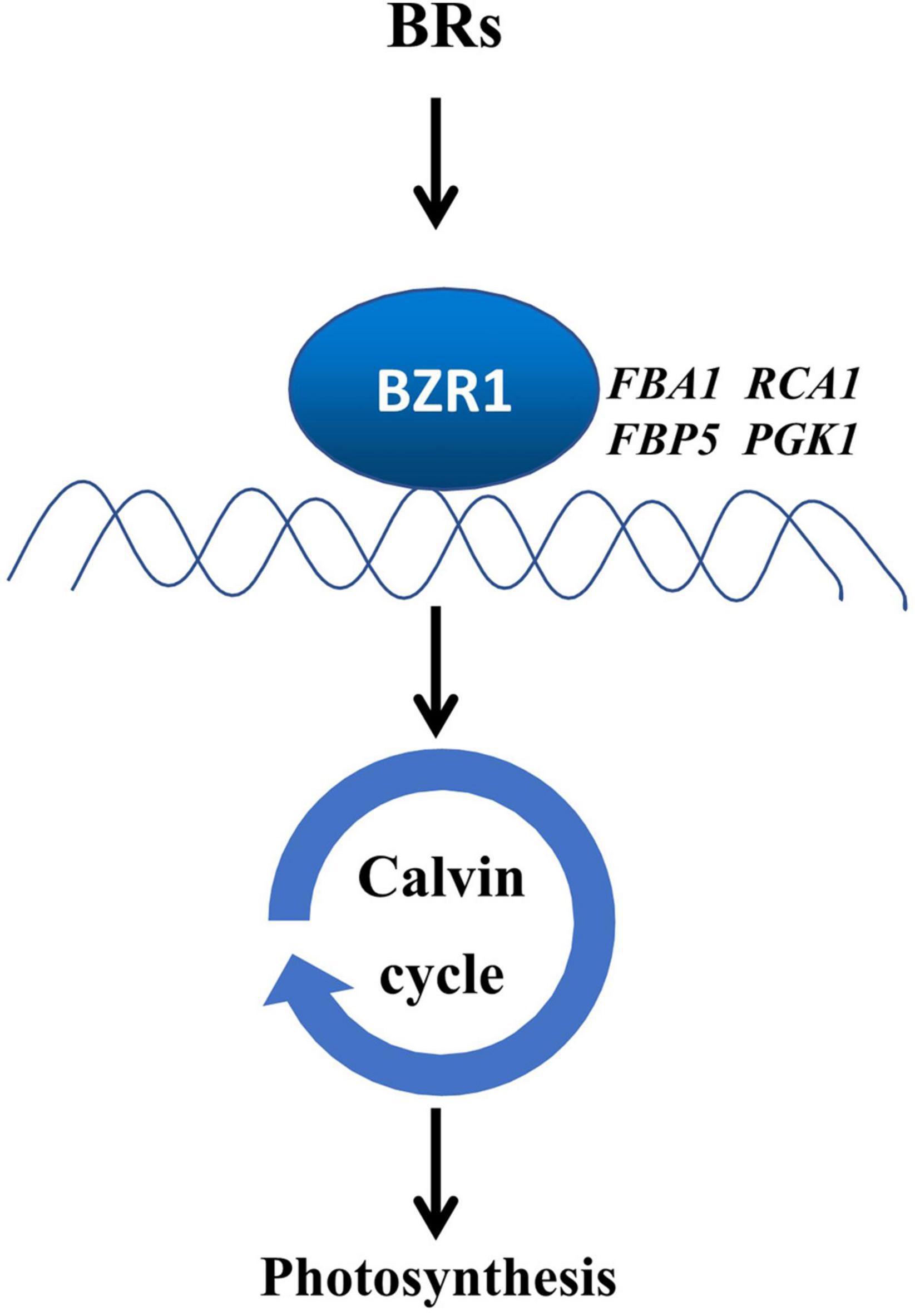
Figure 7. A proposed model for the induction of photosynthesis by BRs in a BZR1-dependent way. BZR1 regulates photosynthesis through the activation of the Calvin cycle in tomatoes. BRs induced the accumulation of BZR1 protein, especially the dephosphorylated forms of BZR1, which activates the transcription of the Calvin cycle genes such as FBA1, RCA1, FBP5, and PGK1 by directly binding to their promoters, subsequently enhancing the Calvin cycle. The induction of the Calvin cycle promotes photosynthesis. Arrows denote positive regulation.
Our previous studies demonstrated that BRs enhance CO2 assimilation by regulating the activity of Rubisco but not by increasing the content of Rubisco (Yu et al., 2004; Xia et al., 2009), and the activation state of Rubisco was associated with the protein level of RCA in plants differing in BR biosynthesis capacity (Li et al., 2016b). RCA is required to release the sugar phosphates from the active site of Rubisco and improve the activity of Rubisco (Portis et al., 2008). The light induction of photosynthesis was accelerated in the transgenic plants with the increased level of RCA and was inhibited by the antisense suppression of the RCA gene (Yamori et al., 2012). These studies demonstrated that regulation of the expression of the RCA gene is an effective way of improving photosynthetic efficiency. In this study, we found that BZR1 increased the mRNA level of RCA1 through direct transcriptional regulation. This can partially explain the BR-induced accumulation of RCA protein (Xia et al., 2009) and suggest that BRs activate Rubisco by regulating the transcription of the RCA gene.
Similar to RCA1, other Calvin cycle genes such as FBA1, FBP5, and PGK1 were also transcriptionally regulated by BR signaling, which might increase the activities of these enzymes. PGK catalyzes the conversion of the RuBP carboxylation product 3-phosphoglycerate to 1,3-glyceraldehyde diphosphate, which may play a role in the metabolic feedback regulation of Rubisco. FBA and FBPase control metabolic flux in the regeneration of RuBP. Transgenic plants with increased activities of FBA and FBPase showed improved CO2 assimilation and biomass yield (Ma et al., 2007). In contrast, VIGS silencing of FBA1 and FBP5 in tomatoes inhibited photosynthetic capacity and plant growth (Figures 5A,B). In this and previous studies, BRs have been shown to induce an increase in Jmax, which reflects the regeneration of RuBP in the Calvin cycle (Yu et al., 2004; Xia et al., 2009; Li et al., 2016b). The results suggested that BR signaling may involve in the control over the carbon flow through the Calvin cycle by regulating the activities of FBA and FBPase. It should be noted that Jmax was only slightly affected by single gene silencing of FBA and FBPase. It is likely that FBA and FBPase-mediated reactions are connected and both are required to support the full activation of the Calvin cycle. At ambient growth conditions in this study, the photosynthesis in the silencing plants was still limited as indicated by the reduced Vc,max, which ultimately led to a stunning growth. Taken together, BR signaling enhances both Vc,max and Jmax through coordinate regulation of key genes controlling multiple sites in the Calvin cycle.
The all-year-round production in the greenhouse is challenged by frequent changes in light and temperature conditions. The photosynthesis of warm-climate crops is sensitive to low temperature and dim light in winter and early spring. The inhibition of CO2 assimilation resulted from reduced light utilization efficiency, and low temperature leads to serious impairment of crop yields. The regulation of RCA by BZR1 is of particular importance under these conditions, since the increase in RCA protein level enhanced the photosynthetic efficiency at low temperature and weak light (Bi et al., 2017). In addition, BRs and BZR1 positively regulate the photoprotection mechanism (Fang et al., 2019). Besides, the application of EBR can alleviate the damage caused by drought and nutrient stresses (dos Santos et al., 2020, 2021a; Barros Junior et al., 2021; Sousa et al., 2021). Thus, BRs increase the fitness of crops in response to a fluctuating environment, and BR signaling is a promising target of engineering and/or gene editing for sustainable production of greenhouse crops. It should be noted that the enzymes of Calvin cycle are activated via redox regulation. Cheng et al. (2014) showed that silencing of chloroplast thioredoxins genes inhibited the activation of Calvin cycle enzymes and impaired the BR-induced increase in CO2 assimilation (Cheng et al., 2014). Brassinosteroids have also been shown to interact with the reactive oxygen species (ROS) signaling (Tian et al., 2018). Importantly, BRs induced ROS production via BZR1-dependent transcriptional regulation of RESPIRATORY BURST OXIDASE HOMOLOG (RBOH) (Yan et al., 2020), while ROS induced a reducing cellular redox status that increases the stability of RCA (Jiang et al., 2012). Hence, BRs are likely to involve in the posttranslational regulation of Calvin cycle enzymes. Photosynthesis also undergoes feedback regulation by carbon state, which is determined by sugar synthesis, metabolism, and transport (Paul and Foyer, 2001). In addition, carbon and nitrogen metabolism in plants are interconnected. Recently, BR signaling was shown to impinge on the nitrate response pathway (Chu et al., 2021). It is unclear whether nitrogen metabolism is involved in BR-mediated increase in photosynthesis. We propose that BRs regulate photosynthesis through the Calvin cycle, and further studies are required to get a whole picture of how BR signaling regulates photosynthesis and C/N metabolism to fuel plant growth.
Data Availability Statement
The original contributions presented in the study are included in the article/Supplementary Material, further inquiries can be directed to the corresponding author.
Author Contributions
JY conceived, designed, and supervised the experiments. XY conducted the experiments and analyzed the data. XY and XX prepared the first draft. MT contributed to the final editing of the manuscript. All authors contributed to the article and approved the submitted version.
Funding
This study was supported by the National Key Research and Development Program (2019YFD1001900), the National Natural Science Foundation of China (31872153), and the Fundamental Research Funds for the Central Universities (2021FZZX001-30).
Conflict of Interest
The authors declare that the research was conducted in the absence of any commercial or financial relationships that could be construed as a potential conflict of interest.
Publisher’s Note
All claims expressed in this article are solely those of the authors and do not necessarily represent those of their affiliated organizations, or those of the publisher, the editors and the reviewers. Any product that may be evaluated in this article, or claim that may be made by its manufacturer, is not guaranteed or endorsed by the publisher.
Acknowledgments
We are grateful to Engineer Li Xu and Engineer Ning Shi from Beijing Ecotek Technology Company for the guidance of utilization of photosynthesis measurement system LI-6400.
Supplementary Material
The Supplementary Material for this article can be found online at: https://www.frontiersin.org/articles/10.3389/fpls.2021.811948/full#supplementary-material
Footnotes
References
Barros Junior, U. O., Lima, M. D. R., Alsahli, A. A., and Lobato, A. K. S. (2021). Unraveling the roles of brassinosteroids in alleviating drought stress in young Eucalyptus urophylla plants: implications on redox homeostasis and photosynthetic apparatus. Physiol. Plant. 172, 748–761. doi: 10.1111/ppl.13291
Bi, H. A., Liu, P. P., Jiang, Z. S., and Ai, X. Z. (2017). Overexpression of the rubisco activase gene improves growth and low temperature and weak light tolerance in Cucumis sativus. Physiol. Plant. 161, 224–234. doi: 10.1111/ppl.12587
Bishop, G. J., Nomura, T., Yokota, T., Harrison, K., Noguchi, T., Fujioka, S., et al. (1999). The tomato DWARF enzyme catalyses C-6 oxidation in brassinosteroid biosynthesis. Proc. Natl. Acad. Sci. U. S. A. 96, 1761–1766. doi: 10.1073/pnas.96.4.1761
Cheng, F., Zhou, Y. H., Xia, X. J., Shi, K., Zhou, J., and Yu, J. Q. (2014). Chloroplastic thioredoxin-f and thioredoxin-m1/4 play important roles in brassinosteroids-induced changes in CO2 assimilation and cellular redox homeostasis in tomato. J. Exp. Bot. 65, 4335–4347. doi: 10.1093/jxb/eru207
Chi, C., Li, X. M., Fang, P. P., Xia, X. J., Shi, K., Zhou, Y. H., et al. (2020). Brassinosteroids act as a positive regulator of NBR1-dependent selective autophagy in response to chilling stress in tomato. J. Exp. Bot. 71, 1092–1106. doi: 10.1093/jxb/erz466
Chu, X. Q., Wang, J. G., Li, M. Z., Zhang, S. J., Gao, Y. Y., Fan, M., et al. (2021). HBI transcription factor-mediated ROS homeostasis regulates nitrate signal transduction. Plant Cell 33, 3004–3021. doi: 10.1093/plcell/koab165
dos Santos, L. A., Da Da Silva, B. R. S., and Lobato, A. K. D. (2021b). Foliar-applied 24-epibrassinolide systemically triggers tolerance to magnesium stress in soybean plants: plausible responses focused on root and leaf structures. Bot. Lett. 168, 558–569. doi: 10.1080/23818107.2021.1911844
dos Santos, L. A., Batista, B. L., and Lobato, A. K. D. (2021a). 24-Epibrasinolide Delays Chlorophyll Degradation and Stimulates the Photosynthetic Machinery in Magnesium-Stressed Soybean Plants. J. Plant Growth Regul. doi: 10.1007/s00344-021-10539-4
dos Santos, L. R., Paula, L. D. S., Pereira, Y. C., da Silva, B. R. S., Batista, B. L., Alsahli, A. A., et al. (2020). Brassinosteroids-Mediated Amelioration of Iron Deficiency in Soybean Plants: beneficial Effects on the Nutritional Status, Photosynthetic Pigments and Chlorophyll Fluorescence. J Plant Growth Regul. 40, 1803–1823. doi: 10.1007/s00344-020-10232-y
Driever, S. M., Simkin, A. J., Alotaibi, S., Fisk, S. J., Madgwick, P. J., Sparks, C. A., et al. (2017). Increased SBPase activity improves photosynthesis and grain yield in wheat grown in greenhouse conditions. Philos. Trans. R. Soc. Lond. B Biol. Sci. 372, 1–10. doi: 10.1098/rstb.2016.0384
Dubois, J. J. B., Fiscus, E. L., Booker, F. L., Flowers, M. D., and Reid, C. D. (2007). Optimizing the statistical estimation of the parameters of the Farquhar-von Caemmerer-Berry model of photosynthesis. New Phytol. 176, 402–414. doi: 10.1111/j.1469-8137.2007.02182.x
Ekengren, S. K., Liu, Y. L., Schiff, M., Dinesh-Kumar, S. P., and Martin, G. B. (2003). Two MAPK cascades, NPR1, and TGA transcription factors play a role in Pto-mediated disease resistance in tomato. Plant J. 36, 905–917. doi: 10.1046/j.1365-313X.2003.01944.x
Fang, P. P., Yan, M. Y., Chi, C., Wang, M. Q., Zhou, Y. H., Zhou, J., et al. (2019). Brassinosteroids Act as a Positive Regulator of Photoprotection in Response to Chilling Stress. Plant Physiol. 180, 2061–2076. doi: 10.1104/pp.19.00088
He, J. X., Gendron, J. M., Sun, Y., Gampala, S. S. L., Gendron, N., Sun, C. Q., et al. (2005). BZR1 is a transcriptional repressor with dual roles in brassinosteroid homeostasis and growth responses. Science 307, 1634–1638. doi: 10.1126/science.1107580
Hu, C. Y., Wei, C. Y., Ma, Q. M., Dong, H., Shi, K., Zhou, Y. H., et al. (2021). Ethylene response factors 15 and 16 trigger jasmonate biosynthesis in tomato during herbivore resistance. Plant Physiol. 185, 1182–1197. doi: 10.1093/plphys/kiaa089
Jiang, Y. P., Cheng, F., Zhou, Y. H., Xia, X. J., Mao, W. H., Shi, K., et al. (2012). Cellular glutathione redox homeostasis plays an important role in the brassinosteroid-induced increase in CO2 assimilation in Cucumis sativus. New Phytol. 194, 932–943. doi: 10.1111/j.1469-8137.2012.04111.x
Kim, E. J., and Russinova, E. (2020). Brassinosteroid signalling. Curr. Biol. 30, R294–R298. doi: 10.1016/j.cub.2020.02.011
Lawson, T., and Vialet-Chabrand, S. (2019). Speedy stomata, photosynthesis and plant water use efficiency. New Phytol. 221, 93–98. doi: 10.1111/nph.15330
Li, X. J., Chen, X. J., Guo, X., Yin, L. L., Ahammed, G. J., Xu, C. J., et al. (2016a). DWARF overexpression induces alteration in phytohormone homeostasis, development, architecture and carotenoid accumulation in tomato. Plant Biotechnol. J. 14, 1021–1033. doi: 10.1111/pbi.12474
Li, X. J., Guo, X., Zhou, Y. H., Shi, K., Zhou, J., Yu, J. Q., et al. (2016b). Overexpression of a brassinosteroid biosynthetic gene Dwarf enhances photosynthetic capacity through activation of Calvin cycle enzymes in tomato. BMC Plant Biol. 16:33. doi: 10.1186/s12870-016-0715-6
Lin, M. T., Occhialini, A., Andralojc, P. J., Parry, M. A., and Hanson, M. R. (2014). A faster Rubisco with potential to increase photosynthesis in crops. Nature 513, 547–550. doi: 10.1038/nature13776
Livak, K. J., and Schmittgen, T. D. (2001). Analysis of relative gene expression data using real-time quantitative PCR and the 2(T)(-Delta Delta C) method. Methods 25, 402–408. doi: 10.1006/meth.2001.1262
Long, S. P., Marshall-Colon, A., and Zhu, X. G. (2015). Meeting the global food demand of the future by engineering crop photosynthesis and yield potential. Cell 161, 56–66. doi: 10.1016/j.cell.2015.03.019
Lopez-Calcagno, P. E., Brown, K. L., Simkin, A. J., Fisk, S. J., Vialet-Chabrand, S., Lawson, T., et al. (2020). Stimulating photosynthetic processes increases productivity and water-use efficiency in the field. Nat. Plants 6, 1054–1063. doi: 10.1038/s41477-020-0740-1
Ma, W. M., Wei, L. Z., Wang, Q. X., Shi, D. J., and Chen, H. B. (2007). Increased activity of the tandem fructose-1,6-bisphosphate aldolase, triosephosphate isomerase and fructose-1,6-bisphosphatase enzymes in Anabaena sp. strain PCC 7120 stimulates photosynthetic yield. J. Appl. Phycol. 20, 437–443. doi: 10.1007/s10811-007-9286-0
Min, T., Yin, X. R., Shi, Y. N., Luo, Z. R., Yao, Y. C., Grierson, D., et al. (2012). Ethylene-responsive transcription factors interact with promoters of ADH and PDC involved in persimmon (Diospyros kaki) fruit de-astringency. J. Exp. Bot. 63, 6393–6405. doi: 10.1093/jxb/ers296
Nolan, T. M., Vukasinovic, N., Liu, D. R., Russinova, E., and Yin, Y. H. (2020). Brassinosteroids: multidimensional Regulators of Plant Growth, Development, and Stress Responses. Plant Cell 32, 295–318. doi: 10.1105/tpc.19.00335
Okegawa, Y., and Motohashi, K. (2015). Chloroplastic thioredoxin m functions as a major regulator of Calvin cycle enzymes during photosynthesis in vivo. Plant J. 84, 900–913. doi: 10.1111/tpj.13049
Paul, M. J., and Foyer, C. H. (2001). Sink regulation of photosynthesis. J. Exp. Bot. 52, 1383–1400. doi: 10.1093/jexbot/52.360.1383
Paul, M. J., and Pellny, T. K. (2003). Carbon metabolite feedback regulation of leaf photosynthesis and development. J. Exp. Bot. 54, 539–547. doi: 10.1093/jxb/erg052
Portis, A. R. Jr., Li, C., Wang, D., and Salvucci, M. E. (2008). Regulation of Rubisco activase and its interaction with Rubisco. J. Exp. Bot. 59, 1597–1604. doi: 10.1093/jxb/erm240
Ruuska, S. A., Andrews, T. J., Badger, M. R., Price, G. D., and von Caemmerer, S. (2000). The role of chloroplast electron transport and metabolites in modulating rubisco activity in tobacco. Insights from transgenic plants with reduced amounts of cytochrome b/f complex or glyceraldehyde 3-phosphate dehydrogenase. Plant Physiol. 122, 491–504. doi: 10.1104/pp.122.2.491
Shu, S., Tang, Y. Y., Yuan, Y. H., Sun, J., Zhong, M., and Guo, S. R. (2016). The role of 24-epibrassinolide in the regulation of photosynthetic characteristics and nitrogen metabolism of tomato seedlings under a combined low temperature and weak light stress. Plant Physiol. Biochem. 107, 344–353. doi: 10.1016/j.plaphy.2016.06.021
Simkin, A. J., Lopez-Calcagno, P. E., Davey, P. A., Headland, L. R., Lawson, T., Timm, S., et al. (2017). Simultaneous stimulation of sedoheptulose 1,7-bisphosphatase, fructose 1,6-bisphophate aldolase and the photorespiratory glycine decarboxylase-H protein increases CO2 assimilation, vegetative biomass and seed yield in Arabidopsis. Plant Biotechnol. J. 15, 805–816. doi: 10.1111/pbi.12676
Simkin, A. J., Lopez-Calcagno, P. E., and Raines, C. A. (2019). Feeding the world: improving photosynthetic efficiency for sustainable crop production. J. Exp. Bot. 70, 1119–1140. doi: 10.1093/jxb/ery445
Simkin, A. J., McAusland, L., Headland, L. R., Lawson, T., and Raines, C. A. (2015). Multigene manipulation of photosynthetic carbon assimilation increases CO2 fixation and biomass yield in tobacco. J. Exp. Bot. 66, 4075–4090. doi: 10.1093/jxb/erv204
Sousa, V. Q., Messias, W. F. S., Pereira, Y. C., da Silva, B. R. S., Lobato, E. M. S. G., Alyemeni, M. N., et al. (2021). Pretreatment with 24-Epibrassinolide Synergistically Protects Root Structures and Chloroplastic Pigments and Upregulates Antioxidant Enzymes and Biomass in Na+-Stressed Tomato Plants. J. Plant Growth Regul. 66. doi: 10.1007/s00344-021-10481-5
Spreitzer, R. J., and Salvucci, M. E. (2002). Rubisco: structure, regulatory interactions, and possibilities for a better enzyme. Annu. Rev. Plant Biol. 53, 449–475. doi: 10.1146/annurev.arplant.53.100301.135233
Takahashi, S., and Murata, N. (2005). Interruption of the Calvin cycle inhibits the repair of Photosystem II from photodamage. Biochim. Biophys. Acta 1708, 352–361. doi: 10.1016/j.bbabio.2005.04.003
Tian, Y. C., Fan, M., Qin, Z. X., Lv, H. J., Wang, M. M., Zhang, Z., et al. (2018). Hydrogen peroxide positively regulates brassinosteroid signaling through oxidation of the BRASSINAZOLE-RESISTANT1 transcription factor. Nat. Commun. 9:1063. doi: 10.1038/s41467-018-03463-x
Tong, H. N., and Chu, C. C. (2018). Functional Specificities of Brassinosteroid and Potential Utilization for Crop Improvement. Trends Plant Sci. 23, 1016–1028. doi: 10.1016/j.tplants.2018.08.007
von Caemmerer, S., and Farquhar, G. D. (1981). Some relationships between the biochemistry of photosynthesis and the gas exchange of leaves. Planta 153, 376–387. doi: 10.1007/BF00384257
Vriet, C., Russinova, E., and Reuzeau, C. (2012). Boosting Crop Yields with Plant Steroids. Plant Cell 24, 842–857. doi: 10.1105/tpc.111.094912
Wang, J., Zheng, C. F., Shao, X. Q., Hu, Z. J., Li, J. X., Wang, P., et al. (2020). Transcriptomic and genetic approaches reveal an essential role of the NAC transcription factor SlNAP1 in the growth and defense response of tomato. Hortic. Res. 7:209. doi: 10.1038/s41438-020-00442-6
Wang, Y., Cao, J. J., Wang, K. X., Xia, X. J., Shi, K., Zhou, Y. H., et al. (2019). BZR1 Mediates Brassinosteroid-Induced Autophagy and Nitrogen Starvation in Tomato. Plant Physiol. 179, 671–685. doi: 10.1104/pp.18.01028
Wang, Z. Y., Wang, Q. M., Chong, K., Wang, F. R., Wang, L., Bai, M. Y., et al. (2006). The brassinosteroid signal transduction pathway. Cell Res. 16, 427–434. doi: 10.1038/sj.cr.7310054
Wu, C. Y., Trieu, A., Radhakrishnan, P., Kwok, S. F., Harris, S., Zhang, K., et al. (2008). Brassinosteroids regulate grain filling in rice. Plant Cell 20, 2130–2145. doi: 10.1105/tpc.107.055087
Xia, X. J., Huang, L. F., Zhou, Y. H., Mao, W. H., Shi, K., Wu, J. X., et al. (2009). Brassinosteroids promote photosynthesis and growth by enhancing activation of Rubisco and expression of photosynthetic genes in Cucumis sativus. Planta 230, 1185–1196. doi: 10.1007/s00425-009-1016-1
Xiong, D. L., and Flexas, J. (2020). From one side to two sides: the effects of stomatal distribution on photosynthesis. New Phytol. 228, 1754–1766.
Yamori, W., Masumoto, C., Fukayama, H., and Makino, A. (2012). Rubisco activase is a key regulator of non-steady-state photosynthesis at any leaf temperature and, to a lesser extent, of steady-state photosynthesis at high temperature. Plant J. 71, 871–880. doi: 10.1111/j.1365-313X.2012.05041.x
Yan, M. Y., Xie, D. L., Cao, J. J., Xia, X. J., Shi, K., Zhou, Y. H., et al. (2020). Brassinosteroid-mediated reactive oxygen species are essential for tapetum degradation and pollen fertility in tomato. Plant J. 102, 931–947. doi: 10.1111/tpj.14672
Yin, Y. H., Vafeados, D., Tao, Y., Yoshida, S., Asami, T., and Chory, J. (2005). A new class of transcription factors mediates brassinosteroid-regulated gene expression in Arabidopsis. Cell. 120, 249–259. doi: 10.1016/j.cell.2004.11.044
Yin, Y. L., Qin, K. Z., Song, X. W., Zhang, Q. H., Zhou, Y. H., Xia, X. J., et al. (2018). BZR1 Transcription Factor Regulates Heat Stress Tolerance Through FERONIA Receptor-Like Kinase-Mediated Reactive Oxygen Species Signaling in Tomato. Plant Cell Physiol. 59, 2239–2254. doi: 10.1093/pcp/pcy146
Yu, J. Q., Huang, L. F., Hu, W. H., Zhou, Y. H., Mao, W. H., Ye, S. F., et al. (2004). A role for brassinosteroids in the regulation of photosynthesis in Cucumis sativus. J. Exp. Bot. 55, 1135–1143. doi: 10.1093/jxb/erh124
Keywords: brassinosteroids, BRASSINAZOLE RESISTANT 1 (BZR1), Calvin cycle, Calvin cycle genes, photosynthesis, Solanum lycopersicum (tomato)
Citation: Yin X, Tang M, Xia X and Yu J (2022) BRASSINAZOLE RESISTANT 1 Mediates Brassinosteroid-Induced Calvin Cycle to Promote Photosynthesis in Tomato. Front. Plant Sci. 12:811948. doi: 10.3389/fpls.2021.811948
Received: 09 November 2021; Accepted: 20 December 2021;
Published: 20 January 2022.
Edited by:
Agepati S. Raghavendra, University of Hyderabad, IndiaReviewed by:
Allan Lobato, Federal Rural University of the Amazon, BrazilPadmanabh Dwivedi, Banaras Hindu University, India
Copyright © 2022 Yin, Tang, Xia and Yu. This is an open-access article distributed under the terms of the Creative Commons Attribution License (CC BY). The use, distribution or reproduction in other forums is permitted, provided the original author(s) and the copyright owner(s) are credited and that the original publication in this journal is cited, in accordance with accepted academic practice. No use, distribution or reproduction is permitted which does not comply with these terms.
*Correspondence: Xiaojian Xia, xiaojianxia@zju.edu.cn