- 1Guangdong Laboratory of Lingnan Modern Agriculture, Guangzhou, China
- 2Laboratory of Plant Nematology, South China Agricultural University, Guangzhou, China
- 3Guangdong Eco-Engineering Polytechnic, Guangzhou, China
Plant-parasitic nematodes secrete an array of cell-wall-degrading enzymes to overcome the physical barrier formed by the plant cell wall. Here, we describe a novel pectate lyase gene Mg-PEL1 from M. graminicola. Quantitative real-time PCR assay showed that the highest transcriptional expression level of Mg-PEL1 occurred in pre-parasitic second-stage juveniles, and it was still detected during the early parasitic stage. Using in situ hybridization, we showed that Mg-PEL1 was expressed exclusively within the subventral esophageal gland cells of M. graminicola. The yeast signal sequence trap system revealed that it possessed an N-terminal signal peptide with secretion function. Recombinant Mg-PEL1 exhibited hydrolytic activity toward polygalacturonic acid. Rice plants expressing RNA interference vectors targeting Mg-PEL1 showed an increased resistance to M. graminicola. In addition, using an Agrobacterium-mediated transient expression system and plant immune response assays, we demonstrated that the cell wall localization of Mg-PEL1 was required for the activation of plant defense responses, including programmed plant cell death, reactive oxygen species (ROS) accumulation and expression of defense-related genes. Taken together, our results indicated that Mg-PEL1 could enhance the pathogenicity of M. graminicola and induce plant immune responses during nematode invasion into plants or migration in plants. This provides a new insight into the function of pectate lyases in plants-nematodes interaction.
Introduction
In nature, plants live in complex environments where they are continually exposed to various beneficial or harmful microbes (Rodriguez et al., 2019). To survive, plants have evolved a sophisticated immune system against pathogenic microbes. It is now clear that plants have two layers of the immune system. The first line of immunity relies on the perception of pathogen-associated molecular patterns (PAMPs) by pattern recognition receptors (PRRs), leading to PAMP-triggered immunity (PTI) (Jones and Dangl, 2006). Such a recognition triggers a cascade of immune events, including calcium influx, the burst of reactive oxygen species (ROS), deposition of callose and expression of defense-related genes (Couto and Zipfel, 2016). For a successful infection, pathogens need to subvert PTI. Adapted pathogens employ numerous effector proteins, which are secreted into plant cells to evade or interfere with PTI, resulting in effector-triggered susceptibility (Win et al., 2012). In the second line of plant immunity, plants make use of nucleotide-binding leucine-rich repeat (NB-LRR) receptor proteins to recognize pathogen effectors, resulting in effector-triggered immunity (ETI) (Jones and Dangl, 2006). In general, ETI leads to a strong defense response associated with a local hypersensitive cell death response (HR). HR-based cell death is believed to effectively limit biotrophic pathogens spread within plant cells (Wilkinson et al., 2019). Besides pathogen-derived PAMPs, the plant innate immune system can recognize some endogenous molecules of the host once the molecules are released into the extracellular environment during tissue disruption (Hou et al., 2019). These host-derived molecules are referred to as damage-associated molecular patterns (DAMPs), which are endogenous indicators of damage and can act as early and general activators of the plant immune system (Choi and Klessig, 2016; Quintana-rodriguez et al., 2018).
The plant cell wall is considered as the first barrier that protects against invading pathogens (Soitamo et al., 2011). The primary plant cell wall is composed of cellulose, hemicellulose, and pectin (Lerouxel et al., 2006). Correspondingly, pathogens including phytopathogenic bacteria, fungi and plant-parasitic nematodes (PPNs) have also evolved numerous kinds of cell-wall-degrading enzymes (CWDEs) to destroy plant cell wall-based defense structure during infection (Kikot et al., 2009; Kubicek et al., 2014). Among the components of a plant cell wall, pectin is the most complex family of acidic polysaccharides, which are classified into three groups: homogalacturonan (α-D-galacturonan or polygalacturonate, PG), rhamnogalacturonan I (RGI), and rhamnogalacturonan II (RGII) (Lerouxel et al., 2006). Therefore, complete pectin degradation requires various pectinases, including pectin lyases, pectate disaccharide-lyases, and pectate lyases (Pereira et al., 2014). Of these, pectate lyase is an important hydrolytic enzyme that cleaves α-1,4-polygalacturonic acid randomly and releasing pectin products, that may induce immune responses within plants (Gui et al., 2017). In the past several decades, some pectate lyases from plant pathogens were found to contribute to virulence and inducing host defense responses (Hugouvieux-Cotte-Pattat et al., 2014), such as endopolygalacturonase 1 T4BcPG1 of Botrytis cinerea fungus, pectate lyases from Erwinia carotovora bacterium and the oomycete pathogen Phytophthora capsici (Wegener et al., 1996; Poinssot et al., 2003; Fu et al., 2015).
PPNs are the only known animal with the ability to produce endogenous pectate lyases (Popeijus et al., 2000). Intriguingly, PPN pectate lyases are believed to originate from bacterial or fungal sources by the horizontal gene transfer (Vanholme et al., 2007). They also play a role in cleaving α-1,4-polygalacturonic acid of pectin, loosen plant cell wall and facilitate penetration and migration of PPNs (Doyle and Lambert, 2002). The first PPN-derived pectate lyase, named Gr-PEL-1, was isolated from the potato cyst nematode Globodera rostochiensis (Popeijus et al., 2000). So far pectate lyases have been found in many economically important PPNs, such as Meloidogyne incognita, M. javanica, M. enterolobii, Heterodera glycines, H. schachtii, Bursaphelenchus xylophilus, and Aphelenchus avenae (Doyle and Lambert, 2002; Huang et al., 2005; Kikuchi et al., 2006; Kudla et al., 2007; Vanholme et al., 2007; Karim et al., 2009; Zhuo et al., 2011). Some pectate lyases have been demonstrated to fulfill the virulence function and promote nematode infection (Vanholme et al., 2007; Peng et al., 2016). However, whether PPN pectate lyases can induce host immunity is unknown. In this study, a pectate lyase gene Mg-PEL1 was identified from M. graminicola. Here, we showed that the pectate lyase Mg-PEL1 not only plays a role in M. graminicola infection as an effector, but also induces plant immune responses.
Materials and Methods
Nematode Culture and Collection
M. graminicola population was isolated from cultivated rice in Hainan, China, purified using a single egg mass and maintained on rice (Oryza sativa cv. “Nipponbare”) roots at 28°C in sterilized sand and soil mixture (3:1). Pre-parasitic second-stage juveniles (pre-J2s) were collected as described previously (Huang et al., 2020).
Gene Cloning and Sequence Analysis
Total RNA and DNA were extracted from approximately 10,000 pre-J2s using RNAprep Pure Micro Kit (TianGen Biotech, Beijing, China) and TIANamp Micro DNA Kit (TianGen Biotech, Beijing, China), respectively. The first-stand cDNA was synthesized using the TransScript One-Step gDNA Removal and cDNA Synthesis Super Mix (TransGen Biotech, Beijing, China) according to the manufacturer’s instructions. Mg-PEL1 full-length cDNA sequence and genomic sequence were amplified by the primers Mg-PEL1_F and Mg-PEL1_R, which were designed according to a pectate lyase gene from M. graminicola transcription data (Haegeman et al., 2013) and the other pectate lyase gene (AID59201) deposited in GenBank. The PCR conditions were as follows: pre-denaturation at 94°C for 4 min, and 30 cycles of denaturation at 98°C for 10 s, annealing at 55°C for 30 s and polymerization at 68°C for 1 min. Primers used in this study were synthesized by Guangzhou IGE biotechnology, Ltd. (IGE biotechnology, Guangzhou, China) and listed in Supplementary Table 1.
The alignment of pectate lyase sequences from different PPNs was produced by ClustalW1 and visualized by Boxshade software2. Signal peptide (SP) and transmembrane domain of Mg-PEL1 were predicted using SignalP 5.03 and TMHMM Server v.2.04. The phylogeny tree was reconstructed using Neighbor-Joining (NJ) method by MEGA 7 software with a bootstrap test (1000 replicates) (Kumar et al., 2016). Protein molecular weight was predicted using DNAstar software.
In situ Hybridization and Developmental Expression Analysis
A 632-bp fragment of Mg-PEL1 from 46 to 677 was selected and amplified using the primer pairs Mg-insitu_F/Mg-insitu_R. Then, the fragment sequence of Mg-PEL146–677 was used to synthesize digoxigenin (DIG)-labeled cDNA sense and antisense probes by the PCR DIG Probe Synthesis Kit (Roche, Rotkreuz, Switzerland) according to the manufacturer’s instructions. Approximately 10,000 pre-J2s of M. graminicola were prepared for fixation and hybridization as described previously (de Boer et al., 1998; Haegeman et al., 2013), and the signals were visualized and captured using Nikon ECLIPSE Ni microscope (Nikon, Tokyo, Japan).
Transcript levels of Mg-PEL1 were measured at different developmental stages of M. graminicola by quantitative real-time PCR (qRT-PCR). Total RNA isolation and first-strand cDNA synthesis were performed as described above. qRT-PCR was performed using the primer pairs Mg-PEL1_qPCR_F/Mg-PEL1_qPCR_R and Mgactin2-F/Mgactin2-R for amplifying the gene Mg-PEL1 and the endogenous reference gene Mgactin-2 (Haegeman et al., 2013). qRT-PCR was performed using SYBR Premix Ex Taq II (Tli RNaseH Plus) (Takara, Tokyo, Japan) on a Thermal Cycler Dice Real-Time System (Takara, Tokyo, Japan). Fold changes in gene expression were determined using the 2–△ △ CT method (Livak and Schmittgen, 2001). Three independent experiments with three technical replicates in each experiment were performed.
Yeast Signal Sequence Trap System
The vector pSUC2 contains a truncated invertase gene, which lacks Methionine (Met) and SP sequence. The SP sequence of Mg-PEL1 was cloned into pSUC2 to generate pSUC2:Mg-PEL1(SP)-Invertase. And the SP of Phytophthora sojae RXLR effector Avr1b was also cloned into pSUC2 to generate pSUC2:Avr1b(SP)-Invertase as a positive control. This SP has been proven to have secretion function in yeast (Dou et al., 2008). pSUC2: Mg-PEL1(SP)-Invertase and pSUC2:Avr1b (SP)-Invertase were transformed into the yeast strain YTK12 by the lithium acetate method (Gietz and Schiestl, 2007; Yin et al., 2018). The yeast strain YTK12 and YTK12 carrying pSUC2 empty vector were used as negative controls. YTK12 and YTK12 containing pSUC2-derived plasmids were grown on the medium CMD-W (0.67% yeast N base without amino acids, 0.075% W dropout supplement, 2% sucrose, 0.1% glucose, and 2% agar) to detect the expression of pSUC2-derived plasmids. Yeast transformants were grown on the YPRAA plate [1% yeast extract, 2% peptone, 2% raffinose, and antimycin A (2 mg/ml)] to detect invertase secretion. Enzymic activity of invertase was further confirmed by the reduction of the dye 2, 3, 5-triphenyltetrazolium chloride (TTC) to the insoluble red-colored triphenylformazan. The detailed procedure was described previously (Yin et al., 2018).
Western Blot
Total proteins were extracted using RIPA lysis buffer (2% SDS, 80 mM Tris/HCl, pH 6.8, 10% glycerol, 0.002% bromophenol blue, 5% β-mercaptoethanol, and complete protease inhibitor cocktail). Sample proteins were denatured and separated on a 12% SDS-PAGE gel, and transferred to a nitrocellulose membrane (PALL, Washington, NY, United States). After blocking with 5% (w/v) non-fat milk for 1 h at room temperature, the membranes were incubated with a primary mouse anti-Flag, anti-Strep or anti-GFP antibody (1:5,000 dilution) (Roche, Rotkreuz, Switzerland) in blocking solution for 2 h. Then membranes were incubated with an anti-mouse horseradish peroxidase-conjugated secondary antibody at a 1:5,000 dilution (Biosynthesis Biotechnology Co., Beijing, China). The proteins were visualized using the Immobilon Western Chemiluminescent system (Merck, Darmstadt, Germany), and Ponceau S staining was used to show equal loading (Goldman et al., 2016).
Enzymatic Activity Assay
Extracellular enzyme activity of pectate lyase was monitored using a titrimetric stop reaction method (Starr et al., 1977). In brief, the full-length sequence of Mg-PEL1 was cloned into the yeast expression vector pSUC2 to generate a pSUC2: Mg-PEL1: Strep fusion construct. The Mg-PEL1: Strep fusion protein was concentrated by immunoprecipitation with Strep beads. Subsequently, Coomassie brilliant blue staining and western blots were used to confirm the expression of Mg-PEL1: Strep fusion protein in supernatant solution. The purified fusion proteins were mixed with 0.5% (wt/vol) polygalacturonic acid (PGA) (Sigma-Aldrich, MO, United States) solution (0.05 M Tris-HCl, 1 mM Ca2+, pH 8.5) and inoculated at 25°C for 0 h and 16 h, respectively. Then, 0.5 ml I2 (100 mM) solution and 0.1 ml Na2CO3 (1 M) were added to the reaction mixture and incubated in the dark for 20 min. The mixtures were then acidified by adding 0.2 ml of H2SO4 (1 M). The free iodine was titrated with continuous stirring against 50 mM Na2S2O3 using 1.0% (w/v) starch as an indicator.
Construction of RNAi Expression Vector and Plant Transformation
A 336 bp fragment of Mg-PEL1 from 368 to 703 bp was selected as the template for RNAi-mediated silencing in rice. CaMV35S-promotor of the pCAMBIA1305.1 vector was replaced with the maize ubiquitin promoter to generate the binary vector pUbi (Chen et al., 2017). The complete RNAi hairpin structure containing Mg-PEL1368–703 (both sense and antisense orientation, separated by a β-glucuronidase (GUS) intron) under the control of maize ubiquitin promoter (Ubi) was transformed into A. tumefaciens strain EHA105 by electroporation. Rice callus induction and co-cultivation with A. tumefaciens were performed as described previously (Kim et al., 2017). The transgenic seedlings were screened on an N6 medium containing 50 mg/L hygromycin. Semi-quantitative reverse-transcriptase polymerase chain reaction (Semi-qRT-PCR) was used to measure the expression level of RNAi construct in each transgenic rice line using the GUS intron as a target fragment. To evaluate the expression level of Mg-PEL1 in M. graminicola parasitizing rice lines expressing the RNAi expression vector, total RNA was isolated from root segments containing nematodes at 3 d after nematode inoculation. qRT-PCR was performed using the primer pairs Mg-PEL1_qPCR_F/Mg-PEL1_qPCR_R and Mgactin2-F/Mgactin2-R for amplifying the target gene Mg-PEL1 and the endogenous reference gene Mgactin-2 of M. graminicola, respectively. In addition, two other genes, Mg-CRT and Mg-expansin, were also evaluated to determine the specificity of Mg-PEL1 silencing (Chen et al., 2017). All mRNA samples were extracted from three independent samples and quantitative or semi-qRT-PCR were performed with three technical replicates for each sample.
Plant Material and Infection Assay
Rice plants were grown in a growth chamber at 28°C under 16 h light and 8 h dark conditions in Quartz sand. 2 week-old rice seedlings were inoculated with about 300 pre-J2s. Fifteen days after nematode inoculation, the rice roots were collected, washed and stained by acid fuchsine (Naalden et al., 2018b), and the number of nematodes and adult females were counted. For phenotypic analysis of transgenic rice, 4 week-old rice seedlings were collected, and plant height, root length and root weight were measured. These experiments were performed two times. Statistically significant differences between transgenic lines and wild type (WT) were determined by a Student’s t-test.
Subcellular Localization
The Mg-PEL1 sequence with or without the native SP was amplified and cloned into pCAMBIA1305.1 vector to generate Mg-PEL1: GFP and Mg-PEL1–SP: GFP, respectively. The SP sequence of pathogenesis-related protein 1a (PR1a) (Supplementary Figure 1) was amplified from N. benthamiana and fused with Mg-PEL1 lacking the native SP to generate Mg-PEL1PR1a: GFP. PR1a is an important defense protein of plants and accumulates predominantly in the apoplastic space (Pecenkova et al., 2017). All constructs were transformed into an A. tumefaciens strain GV3101 and transiently expressed in N. benthamiana leaves by agroinfiltration. To confirm the apoplastic localization, N. benthamiana leaves expressing constructs were infiltrated with 30% glycerol for 20 min to induce plasmolysis (Liu et al., 2016). Subsequently, photographs were taken using an SP5 Leica confocal microscope (Nikon, Tokyo, Japan).
Plant Immune Response Assay
N. benthamiana was grown in a controlled greenhouse at 25°C under 16 h light and 8 h dark conditions for a month. One day before agroinfiltration, plants were transferred into a growth chamber and maintained at 20–25°C, 35–50% humidity, with a 16 h light/8 h dark cycle. A. tumefaciens strain GV3101 carrying four constructs Mg-PEL1:Flag, Mg-PEL1–SP:Flag, Mg-PEL1PR1a:Flag and Flag were transiently expressed in N. benthamiana leaves. Images were taken for the cell death symptom analysis at 5 d after infiltration. To detect the accumulation of hydrogen peroxide, four constructs Mg-PEL1:Flag, Mg-PEL1–SP:Flag, Mg-PEL1PR1a:Flag and Flag were infiltrated into leaves of 4 week-old N. benthamiana. Two days after infiltration, N. benthamiana leaves were stained by using 3,3’-diaminobenzidine (DAB) solution as described previously (Daudi and O’Brien, 2012). To determine the defense-related gene expression levels, A. tumefaciens strains carrying the constructs Mg-PEL1:Flag, Mg-PEL1–SP:Flag, Mg-PEL1PR1a:Flag and Flag were infiltrated into N. benthamiana leaves. Two days after infiltration, the transcript abundance of three representative defense-related genes, PR-5, PAL, and NPR1, was determined by semi-qRT-PCR, and NbEF1α (Heese et al., 2007) was used as the reference gene.
Results
Sequence Analysis of the Mg-PEL1 Gene From M. graminicola
A 972-bp genomic DNA sequence of Mg-PEL1 (GenBank accession number MW266124) was amplified from M. graminicola. Mg-PEL1 contains a 795 bp coding region, interrupted by two introns of 140 and 37 bp (Supplementary Figure 2). The complete open read frame (ORF) encodes a 264-amino-acid (aa) protein with a predicted molecular mass of 29.3 kDa. Signal analysis of Mg-PEL1 identified an 18-aa N-terminal SP, and no putative transmembrane domain was predicted.
A multiple sequence alignment of the deduced amino acid sequence of Mg-PEL1 with pectate lyase sequences from other PPNs is present in Figure 1. Mg-PEL1 contains highly conserved regions that are common to microbial class III pectate lyases (Figure 1) and shows 100% identity with a pectate lyase (AID59201) from M. graminicola transcriptome and 99.6% identity (263/264, without insertions/deletions) with the other pectate lyase (KAF7637017) from M. graminicola genome. It also shows similarities to three Meloidogyne pectate lyases, Me-PEL1 of M. enterolobii (ADN87334), Mj-PEL1 of M. javanica (AAL66022), Mi-PEL1 of M. incognita (AAQ09004), by a protein BLAST search. However, the identities between Mg-PEL1 and these three pectate lyases are only 58.0–59.0%. In addition, Mg-PEL1 displays somewhat similar to Rs-PEL3 of Radopholus similis (QIC04078, 50.4% identity) and several pectate lyases from bacteria and fungi, such as Streptomyces kanasensis (WP_058944121, 42.0% identity) and Anthracocystis flocculosa PF-1 (XP_007881659, 41.1% identity). Between Mg-PEL1 and other PPN pectate lyases, the identities are less than 40.6%. A NJ tree (Supplementary Figure 3) was constructed to examine the relationships among 37 pectate lyases. This phylogenetic tree shows that Mg-PEL1, Me-PEL1, Mj-PEL1, Mi-PEL1, and Rs-PEL3 are placed within a well-supported monophyletic clade; this clade is then sister to the other clade comprising some pectate lyase sequences from bacteria and fungi, but far from other PPN pectate lyase sequences.
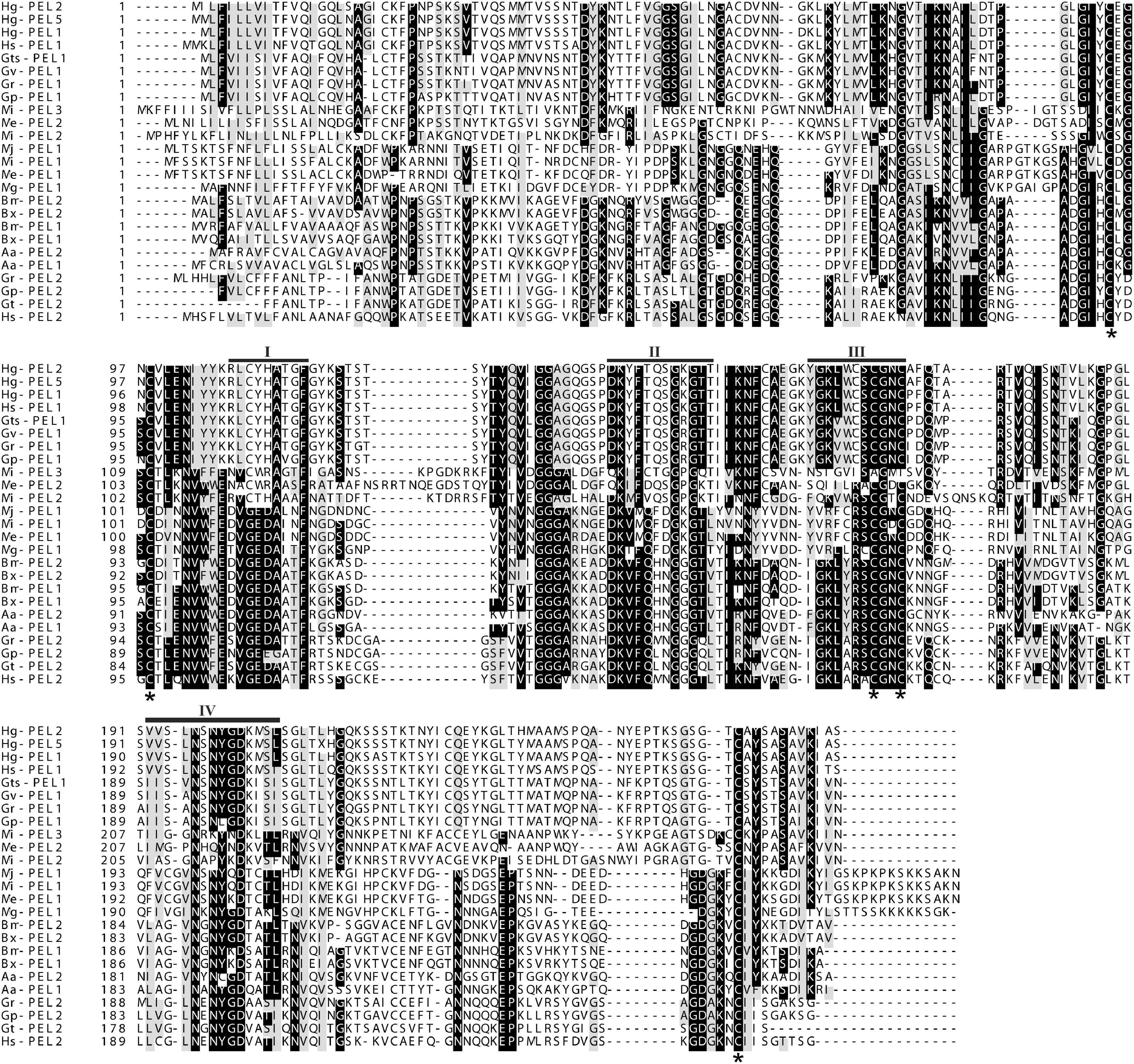
Figure 1. Multiple sequence alignment of the predicted Mg-PEL1 protein with other pectate lyase sequences from plant-parasitic nematodes. Hg-PEL2 (AAM74954), Hg-PEL5 (ADW77536), and Hg-PEL1 (AAK08974) from Heterodera glycines; Hs-PEL1 (ABN14273) and Hs-PEL2 (ABN14272) from H. schachtii; Gts-PEL1 (AEA08834) and Gt-PEL2 (ACU64859) from Globodera tabacum; Gv-PEL1 (AEA08828) from G. virginiae; Gr-PEL1 (AAF80746) and Gr-PEL2 (AAM21970) from G. rostochiensis; Gp-PEL2 (ACU64845) and Gp-PEL1 (AEA08862) from G. pallida; Mi-PEL1 (AAQ09004), Mi-PEL2 (AAQ97032) and Mi-PEL3 (AAW56829) from Meloidogyne incognita; Me-PEL1 (ADN87334) and Me-PEL2 (ALB38961) from M. enterolobii; Mj-PEL1 (AAL66022) from M. javanica; Mg-PEL1 (MW266124) from M. graminicola; Bm-PEL1 (BAE48374) and Bm-PEL2 (BAE48375) from Bursaphelenchus mucronatus; Bx-PEL1 (BAE48371) and Bx-PEL2 (BAE48372) from B. xylophilus; Aa-PEL1 (BAI44499) and Aa-PEL2 (BAI44497) from Aphelenchus avenae. Black bars (I–IV) indicate the conserved regions. Black stars indicate the conserved cysteine residues.
Mg-PEL1 Is Expressed in the Subventral Esophageal Gland Cells of M. graminicola and Contains a Signal Peptide With Secretion Function
Tissue localization of Mg-PEL1 in M. graminicola was determined by in situ hybridization. Hybridization signal was observed in the subventral esophageal gland cells with the DIG-labeled antisense cDNA probe of Mg-PEL1 (Figure 2 and Supplementary Figure 4). No hybridization signal was found in the tissue of M. graminicola with the sense cDNA probe of Mg-PEL1 (Figure 2).
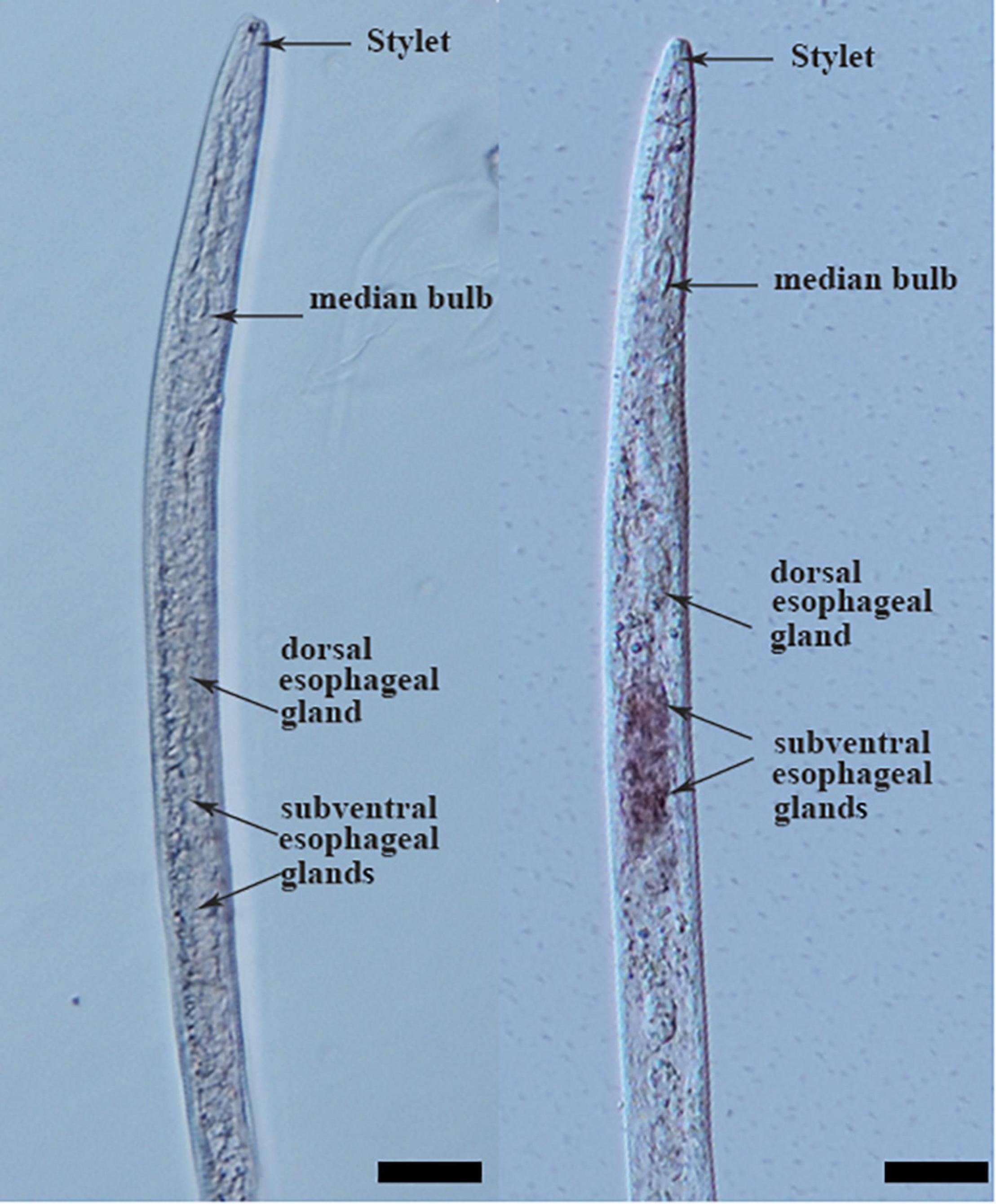
Figure 2. Subventral gland localization of Mg-PEL1 in the pre-parasitic second stage juveniles of Meloidogyne graminicola. Fixed nematodes were hybridized with digoxigenin-labeled Mg-PEL1 sense cDNA probe (left) and antisense cDNA probe (right). Scale bars, 20 μm.
The 20-aa N-terminal sequence of Mg-PEL1, i.e., the 18-aa predicted SP sequence along with the subsequent two amino acids, was cloned into the yeast vector pSUC2. The yeast YTK12 carrying pSUC2:Mg-PEL1(SP)-Invertase and the positive control pSUC2:Avr1b (SP)-Invertase can grow on CMD-W and YPRAA media plates (Figure 3). Invertase enzymatic activity was further confirmed by the reduction of the dye TTC to the insoluble red colored triphenylformazan (Figure 3). By contrast, negative controls, i.e., YTK12 strain carrying pSUC2 empty vector and YTK12 strain, were not able to grow on YPRAA media, and culture filtrates were treated with TTC remained colorless (Figure 3). These results suggested that Mg-PEL1 carries a functional secretory SP.
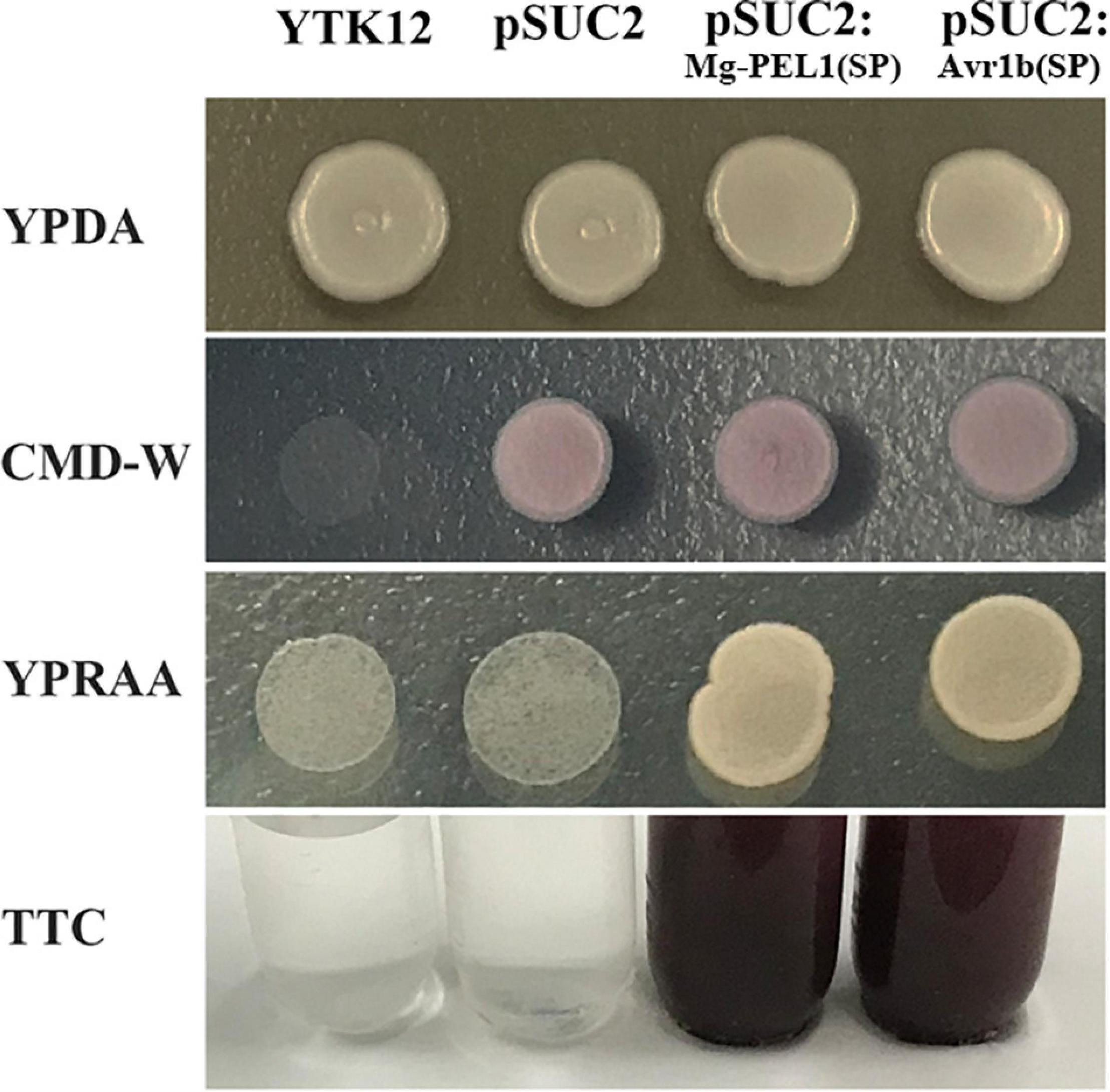
Figure 3. Secretion function analysis of Mg-PEL1 signal peptide. The predicted signal peptide of Mg-PEL1 was cloned into the yeast vector pSUC2 to generate pSUC2:Mg-PEL1(SP)-invertase construct, and pSUC2:Avr1b (SP)-Invertase was used as a positive control. The YTK12 strain and YTK12 carrying the empty pSUC2 vector were used as negative controls. YTK12 can grow on YPDA plates. CMD-W media was used to ensure the expression of pSUC2-derived plasmids. Yeast transformants were grown on the YPRAA media to detect invertase secretion. Meanwhile, enzymic activity of invertase was further confirmed by the reduction of the dye 2, 3, 5-triphenyltetrazolium chloride (TTC) to the insoluble red-colored triphenylformazan. The experiment was repeated three times independently.
Developmental Expression Analysis
To analyze the developmental expression pattern of Mg-PEL1, qRT-PCR was performed by using RNA isolated from different developmental stages of M. graminicola. Transcripts at the egg stage was set unity as a reference to calculate the relative fold changes in other stages. The highest transcription level of Mg-PEL1 appeared at the pre-J2 stage, representing 112-fold increases in expression compared with eggs. After the pre-J2 stage, the transcript level of Mg-PEL1 declined dramatically. However, the relative fold changes for transcripts in parasitic nematodes at 2 and 5 day post-inoculation (dpi) still reached approximately 13-fold compared that in eggs (Figure 4). The findings suggested that Mg-PEL1 may play a role in the invasion and early migration stages of M. graminicola, especially in the invasion stage.
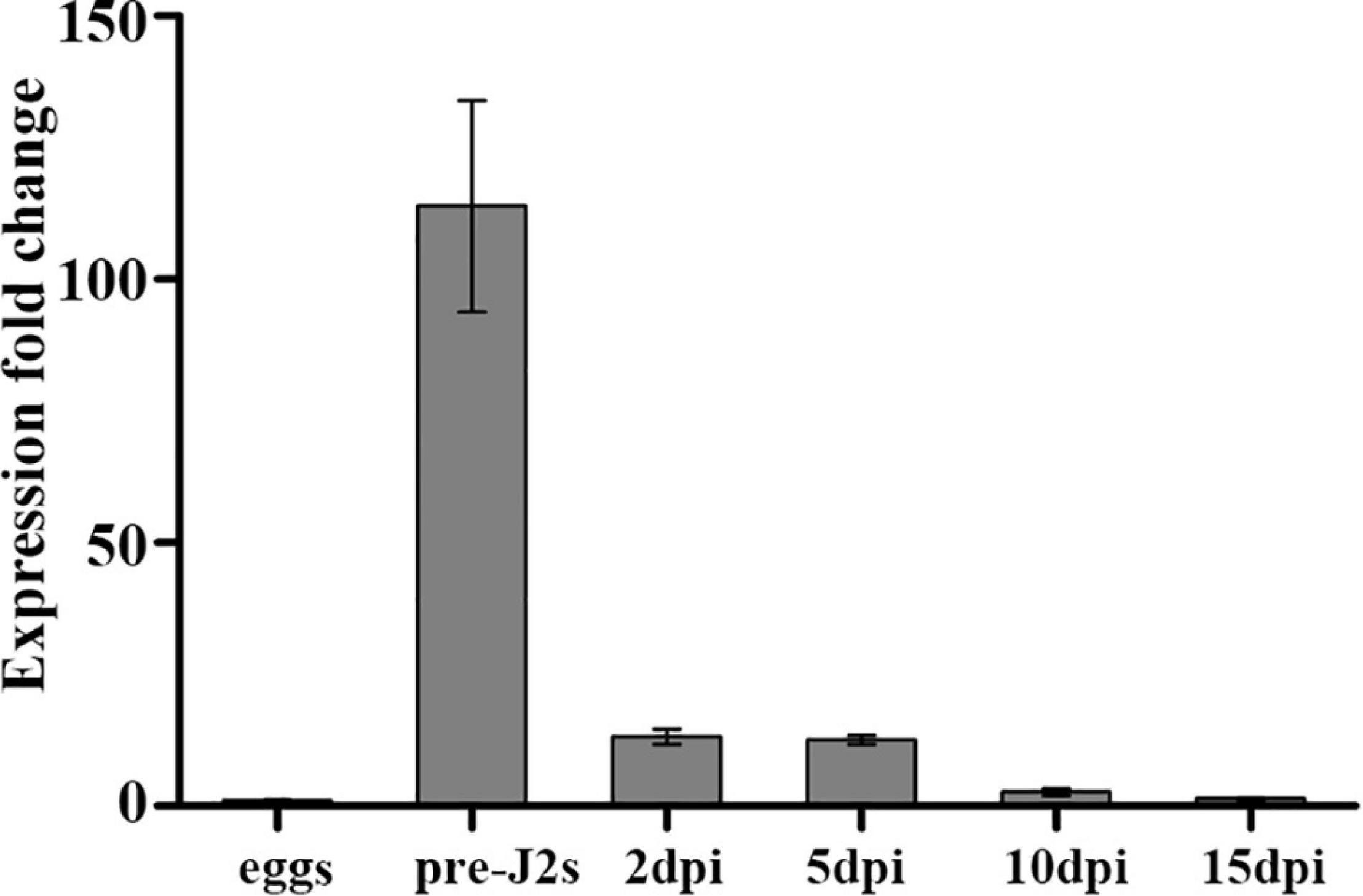
Figure 4. Expression of Mg-PEL1 in Meloidogyne graminicola at different life stages. The fold change values were presented as the change in expression relative to the egg. Data shown are the means of three repeats plus standard deviation (± SD). Three independent experiments were performed with similar results. dpi, day post-infection; pre-J2s, pre-parasitic second-stage juveniles.
Mg-PEL1 Exhibits a Hydrolytic Activity Toward PGA
To investigate enzymatic activity of Mg-PEL1, the yeast system was used to express the recombinant Mg-PEL1: Strep construct and empty vector (EV). Culture supernatants were purified using an anti-Strep bead and verified by Coomassie blue staining. A band at approximately 36 kDa molecular weight was observed in the Mg-PEL1: Strep construct culture supernatant, which corresponds to the predicted molecular weight of Mg-PEL1: Strep fusion protein. Western blot further confirmed the expression of Mg-PEL1: Strep. As a control, no bands were observed in the EV sample (Figure 5A), showing the correct expression of recombinant protein Mg-PEL1: Strep. For enzymatic activity analysis, culture supernatants of Mg-PEL1: Strep protein and EV were purified and incubated with free iodine and PGA solution for 0 and 16 h, respectively. The rest amount of free iodine was titrated against Na2S2O3 using soluble starch as the indicator. Titration results showed that the free iodine in the mixture containing Mg-PEL1: Strep protein at 16 h after incubation was lower than in other mixtures (Figure 5B). These results indicated that Mg-PEL1:Strep fusion protein has hydrolytic activity and can degrade PGA into oligogalacturonic acids, which are covalently bound to free iodine.
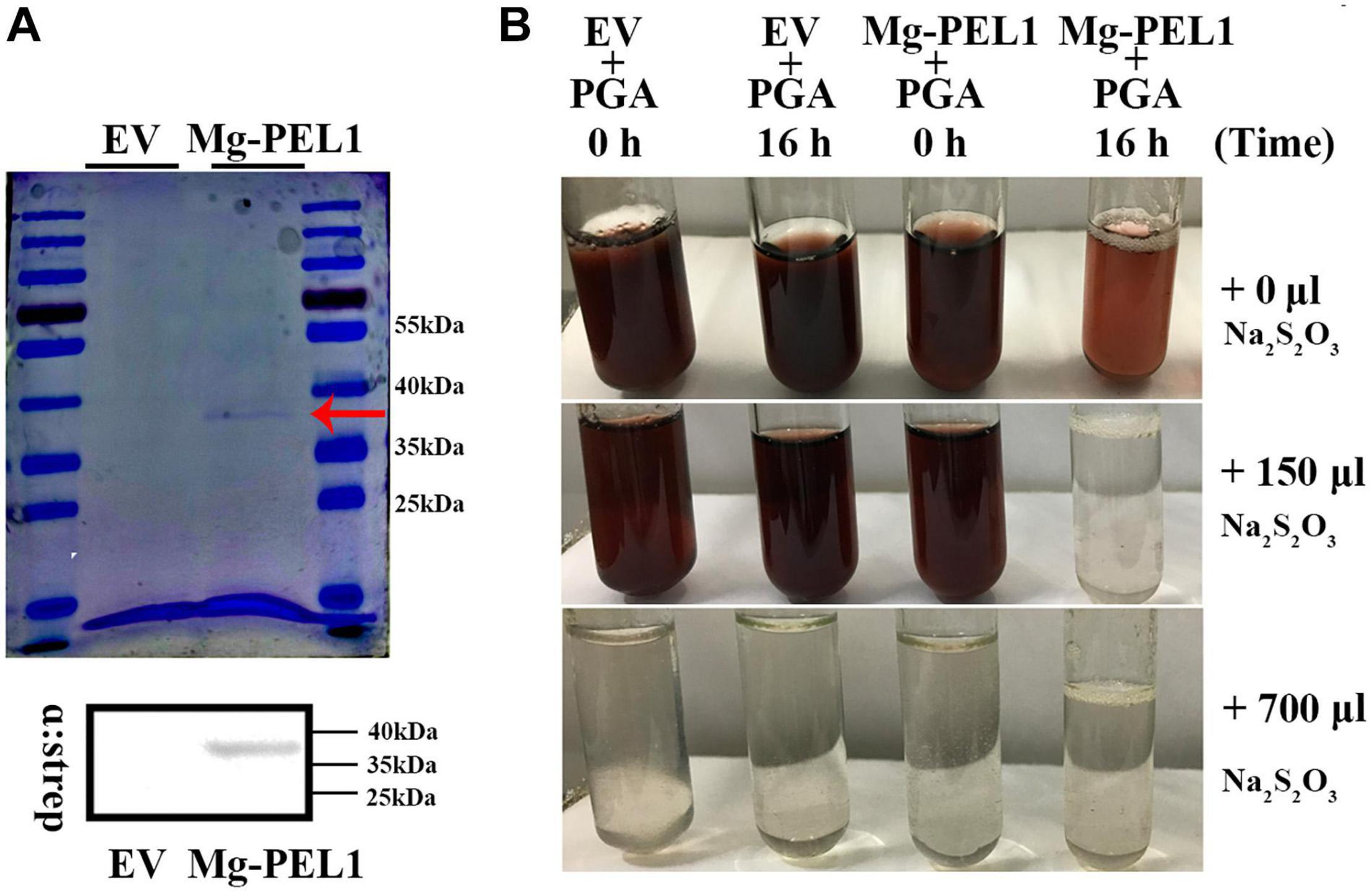
Figure 5. Enzyme activity analysis of recombinant protein Mg-PEL1. (A) Expression and purification of recombinant Mg-PEL1: Strep. Mg-PEL1: Strep was purified by anti-Strep beads and separated by SDS PAGE with Coomassie brilliant blue staining (red arrow). Western blot was used to confirm the correct expression of recombinant protein Mg-PEL1: Strep in culture supernatants. (B) Enzyme activity of Mg-PEL1 was measured using a titrimetric stop reaction assay. Mg-PEL1 possesses enzyme activity to hydrolyze PGA into oligogalacturonic acids, which can bind to free iodine. The rest amount of free iodine was titrated against Na2S2O3 using soluble starch as the indicator. The experiment was repeated three times. Similar results were obtained from three independent experiments. EV, empty vector; PGA, polygalacturonic acid.
Mg-PEL1 Is a Virulence Factor During M. graminicola Infection
To demonstrate the contribution of Mg-PEL1 on M. graminicola virulence, host-mediated artificial double strands RNA (dsRNA) approach was utilized to silence the target gene Mg-PEL1 within the feeding nematodes. The sense and antisense fragments of 368–703 bp within Mg-PEL1 were separated by a GUS intron to construct a hairpin dsRNA vector targeting Mg-PEL1 (Supplementary Figure 5), and the vector was transformed into rice. A 363-bp GUS intron fragment was detected by semi-qRT-PCR in two transgenic rice lines (#10 and #28), suggesting that these two lines may express Mg-PEL1368–703 dsRNA. As control, no GUS fragments were amplified from WT plants (Figure 6A). The transgenic rice lines showed no significant differences compared with the WT plants (Supplementary Figure 6). To demonstrate the RNAi effect, qRT-PCR was used to detect the expression level of Mg-PEL1 in nematodes at 3 dpi. The results showed that the transcription of Mg-PEL1 in nematodes from RNAi lines was significantly reduced compared with that from the WT plants (Figure 6B). Because no similar sequences of Mg-PEL1 were found in the genome and transcriptome of M. graminicola, two other M. graminicola effector genes MgCRT and MgExpansin, which have similar transcriptional expression patterns to Mg-PEL1 were used to verify the specificity of this Mg-PEL1-targeting RNAi. qRT-PCR showed that these two non-targeting genes were not affected by this Mg-PEL1-targeting RNAi (Figure 6B). These results suggested that this Mg-PEL1-targeting RNAi was effective and specific. Meanwhile, RNAi lines, and WT plants were used for nematode infection assays. PCR was used to confirm the positive RNAi transgenic rice seedlings prior to nematode inoculation (Supplementary Figure 7). The results of infection assays indicated that two RNAi lines displayed a significant reduction in the number of adult females per root system compared with the WT plants at 15 dpi with M. graminicola. The number of adult females were found to be reduced by 22.6–25.7% (Figure 6C). These results suggested that Mg-PEL1 affects M. graminicola parasitism.
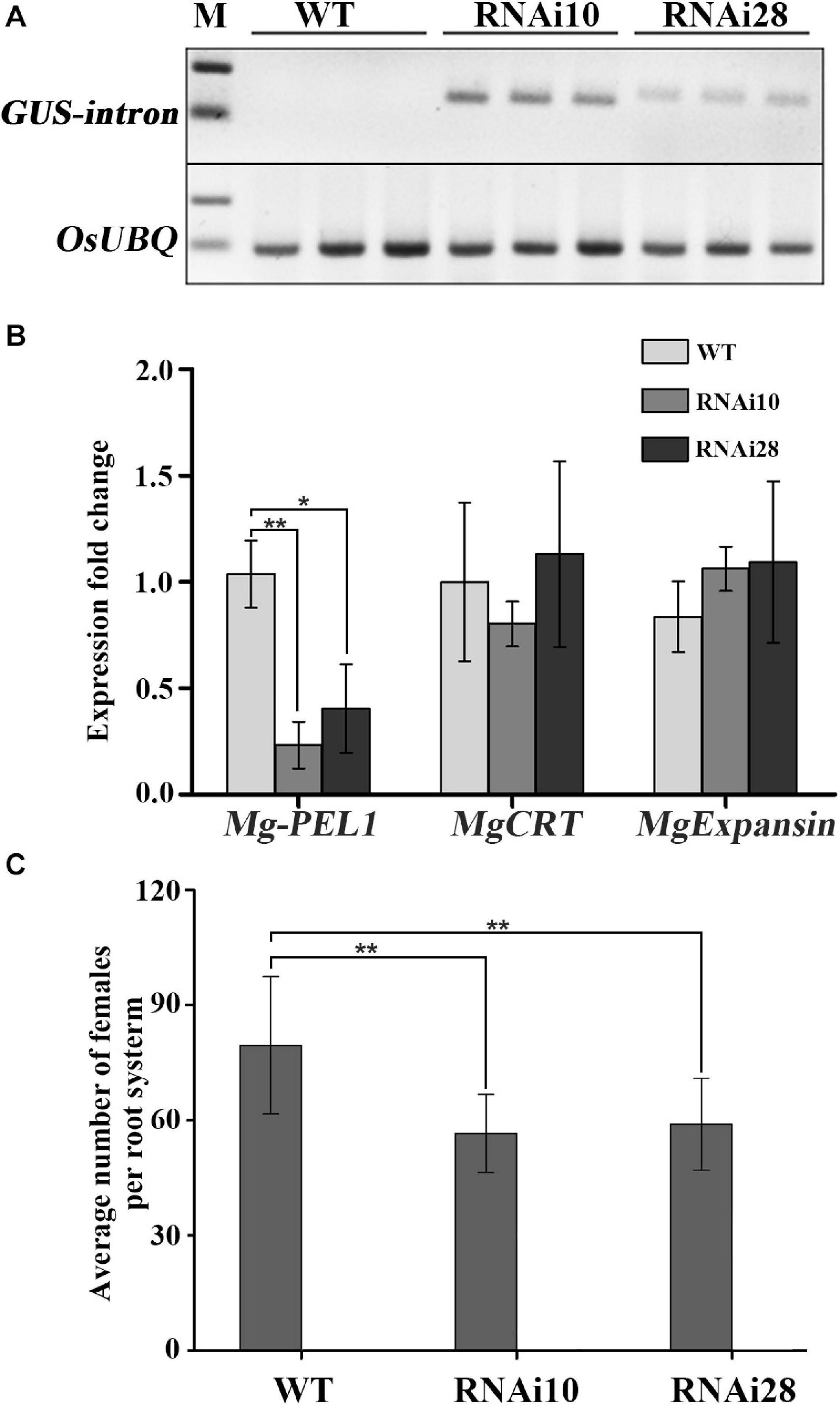
Figure 6. In planta RNA interference (RNAi) of Mg-PEL1 reduces pathogenicity of Meloidogyne graminicola. (A) Semi-quantitative PCR for the detection of the β-glucuronidase (GUS) intron fragment was used to confirm the expression levels of RNAi construct in roots of transgenic RNAi lines. Three independent samples from different plants of WT, RNAi10 and RNAi28 were used for the analysis. The experiment was repeated three times. OsUBQ (Os03g13170) gene was selected as the reference gene for semi-quantitative PCR. (B) qRT-PCR assays of the expression levels of Mg-PEL1 in M. graminicola collected from RNAi lines and WT at 3 day post-inoculation (dpi). The expression levels of MgCRT and MgExpansin from M. graminicola were used to determine the specificity of the Mg-PEL1-targeting RNAi. Similar results were obtained from three independent experiments. (C) Transgenic RNAi lines showed a significant reduction in the number of adult females compared with the WT. Two-week-old seedlings were inoculated with M. graminicola pre-J2s, and the number of adult females per plant was counted at 15 dpi. Data are presented as the means ± standard deviation (SD) from 9 to 10 plants. Two independent experiments were performed with similar results. All data revealed normal distribution and homogeneity of variance. One-way ANOVA Dunnett’s t-tests, *P < 0.05; **P < 0.01. RNAi10 and 28, different RNAi transgenic rice lines. WT, wild type.
Cell Wall Localization of Mg-PEL1 Is Required for Activation of Plant Defense Responses
Pectate lyases from pathogens usually target to plant cell wall and degrade plant cell wall polysaccharides (Hugouvieux-Cotte-Pattat et al., 2014). To investigate whether Mg-PEL1 can function in plant cell wall, three constructs Mg-PEL1–SP:GFP, Mg-PEL1:GFP and Mg-PEL1PR1a:GFP were first generated and transiently expressed in N. benthamiana leaves (Figure 7A). At 2 d post-infiltration, Mg-PEL1: GFP and Mg-PEL1–SP: GFP fusion proteins were observed in the cytoplasm and nucleus, while the Mg-PEL1PR1a: GFP fusion protein was accumulated in the edge of tobacco cells (Figure 7B). Plasmolysis was further performed to distinguish the plasma membrane from the cell wall. After the plasmolysis treatment, Mg-PEL1: GFP and Mg-PEL1–SP:GFP fusion proteins were still observed in the cytoplasm and nucleus, no signals observed in the extracellular space between plasma membrane and cell wall (Figure 7C). However, the Mg-PEL1PR1a: GFP fusion protein was found to localize in the cell wall and cytoplasm (Figure 7C and Supplementary Figure 8). Western blot performed on two dpi infiltrated leaves further confirmed the correct expression of these fusion proteins in tobacco cells (Figure 7D).
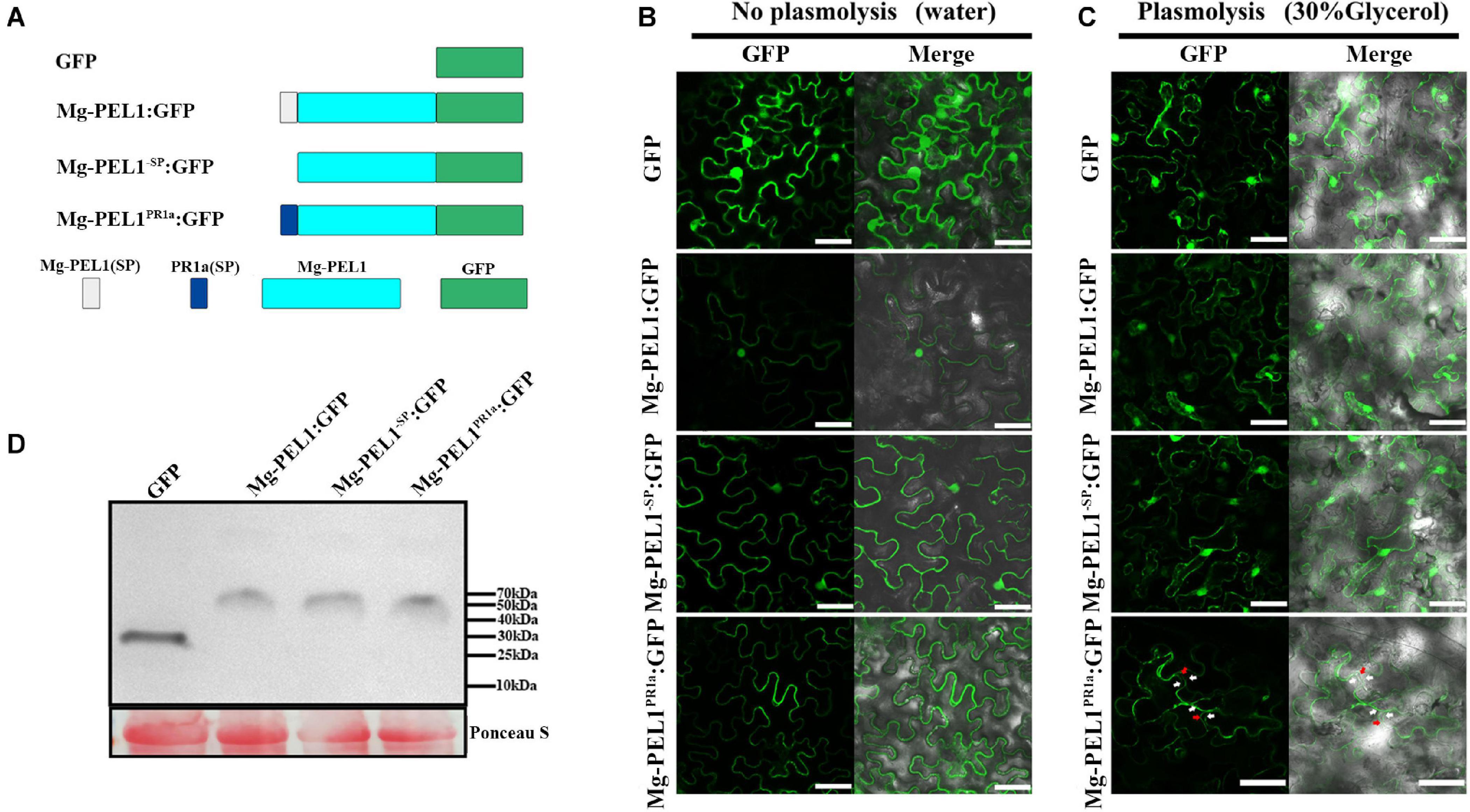
Figure 7. Subcellular localization of Mg-PEL1 in plant cells. (A) Schematic diagram showing the protein structures Mg-PEL1:GFP, Mg-PEL1– SP:GFP, Mg-PEL1PR1a:GFP and GFP. (B) Agrobacterium stain GV3101 carrying fusion constructs Mg-PEL1: GFP, Mg-PEL1– SP: GFP, Mg-PEL1PR1a:GFP and the GFP control were transiently expressed in Nicotiana benthamiana leaves. (C) N. benthamiana leaves expressing Mg-PEL1: GFP, Mg-PEL1– SP: GFP, Mg-PEL1PR1a: GFP and GFP were treated with 30% glycerol for plasmolysis. GFP signals were observed at 2 d after infiltration. Red arrows indicate plant cell wall, and white arrows indicate plasma membrane. Scale bar = 100μm. (D) Western blotting confirmed the expression of Mg-PEL1: GFP, Mg-PEL1– SP: GFP, Mg-PEL1PR1a: GFP and GFP in N. benthamiana leaves. Ponceau S staining was used to show equal loading.
To investigate whether Mg-PEL1 can induce the defense responses when it accumulates in plant cell wall, four constructs Mg-PEL1–SP:Flag, Mg-PEL1:Flag, Mg-PEL1PR1a:Flag and Flag were then generated and transiently expressed in N. benthamiana leaves. First, the ability of Mg-PEL1 in induction of plant cell death was investigated. At 5 d post-infiltration, 23 of 30 infiltrated sites of Mg-PEL1PR1a: Flag displayed necrosis, whereas sites infiltrated with Mg-PEL1–SP: Flag, Mg-PEL1: Flag and Flag did not induce necrosis (Figure 8A). Second, the ability of Mg-PEL1 in hydrogen peroxide induction was detected. The DAB straining result showed that light-brown color was visible in the infiltration areas expressing Mg-PEL1PR1a: Flag, but invisible in infiltration regions with Mg-PEL1–SP: Flag, Mg-PEL1: Flag and Flag (Figure 8B). The results indicated that the expression of Mg-PEL1PR1a: Flag markedly promoted hydrogen peroxide production. Third, transcriptional levels of defense-related genes including PR-5, PAL, and NPR1 were examined. Semi-qRT-PCR showed that the transcript abundances of the three defense-related genes were significantly up-regulated in N. benthamiana leaves expressing Mg-PEL1PR1a: Flag when compared to those in N. benthamiana leaves expressing Mg-PEL1–SP: Flag, Mg-PEL1: Flag and Flag (Figure 8C). The expression of all proteins was verified by western blot (Figure 8D). These results illustrated that Mg-PEL1 can activate plant defense responses only when it targets to plant cell wall.
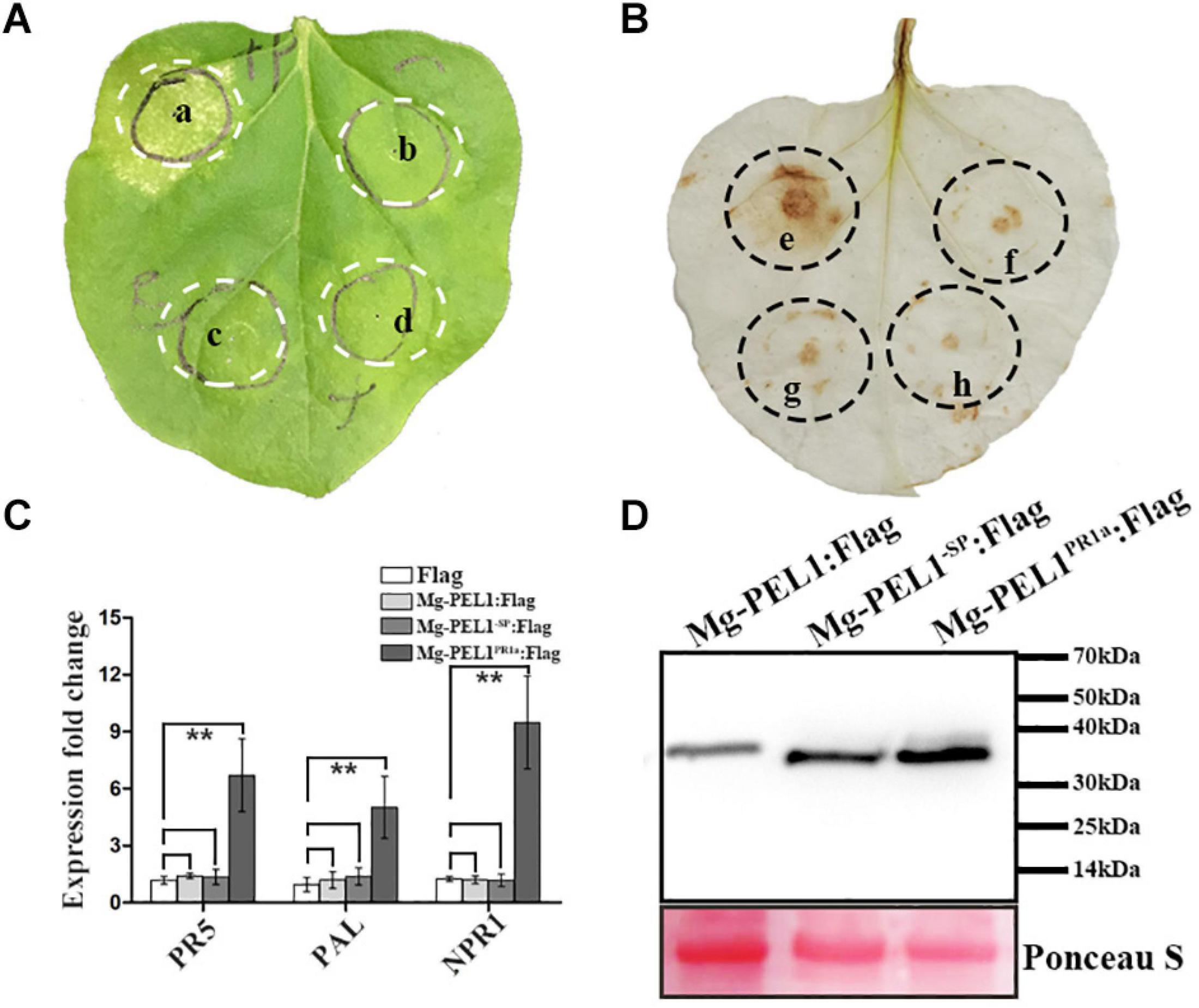
Figure 8. Cell wall localization of Mg-PEL1 is required for the activation of plant defenses. (A) Mg-PEL1 triggered cell death in Nicotiana benthamiana. Agrobacterium strain GV3101 carrying constructs Mg-PEL1PR1a: Flag (a), Mg-PEL1– SP: Flag (b), Mg-PEL1: Flag (c) and Flag (d) were transiently expressed in N. benthamiana leaves. At 5 d after infiltration, infiltrated sites were taken photos for cell death phenotype analysis. (B) Mg-PEL1 induced hydrogen peroxide production in N. benthamiana. Agrobacterium strain GV3101 carrying constructs Mg-PEL1PR1a: Flag (e), Mg-PEL1– SP: Flag (f), Mg-PEL1: Flag (g) and Flag (h) were transiently expressed in N. benthamiana leaves. At 2 d after infiltration, hydrogen peroxide production in infiltrated leaves was detected by DAB staining. (C) Mg-PEL1 induced the expression of defense related genes in N. benthamiana. The transcript levels of three defense response genes PR-5, PAL and NPR1 were measured in N. benthamiana leaves at 2 d after infiltration with Mg-PEL1– SP:Flag, Mg-PEL1:Flag, Mg-PEL1PR1a:Flag and Flag constructs. All data revealed normal distribution and homogeneity of variance. One-way ANOVA Dunnett’s t-tests, *P < 0.05; **P < 0.01. (D) Western blotting confirmed the expression of Mg-PEL1– SP: Flag, Mg-PEL1: Flag and Mg-PEL1PR1a: Flag in N. benthamiana leaves. Ponceau S staining were used to show equal loading.
Discussion
In this study, we cloned and characterized a pectate lyase gene Mg-PEL1 from the rice root-knot nematode, M. graminicola. Mg-PEL1 contains four highly conserved regions as well as five conserved cysteine residues, according to the class III pectate lyase (Shevchik et al., 1997). When compared with all the pectate lyase genes of PPNs, we found Mg-PEL1 at amino acid level shares <60% identities with them. Intriguingly, the phylogenetic analysis revealed that Mg-PEL1 has a closer relationship to several pectate lyases from fungi and bacteria than to some pectate lyases from PPNs, which is in line with the general idea that PPNs got pectate lyases from fungi or bacteria through horizontal gene transfer (Vanholme et al., 2007). These findings suggest that Mg-PEL1 may be a novel member of the class III pectate lyase.
Plant cell wall presents a primary defensive barrier against plant pathogens (Kubicek et al., 2014). As one of the important plant pathogens, PPNs can secrete various CWDEs to macerate the plant cell wall, which is essential during nematode invasion into plants or migration in plants (Kudla et al., 2005). Among these CWDEs, pectate lyases play a major role in the degradation of plant pectin, which is one of the most abundant components in plant cell wall (Caffall and Mohnen, 2009). Pectate lyases are widely distributed in diverse families of microorganisms and are considered as an important pathogenicity or virulence factor (Payasi et al., 2009). Recently, evidence emerged that PPN pectate lyases can promote nematode infection. For example, silencing of pectate lyase genes Me-PEL1, Hs-PEL2, and Hg-PEL6 significantly reduced the ability of nematodes to infect host plants (Vanholme et al., 2007; Zhuo et al., 2011; Peng et al., 2016). In the present study, we showed that Mg-PEL1 has hydrolytic activity against PGA, a major component of pectin. Meanwhile, we observed that there was a significant decrease in the number of females in transgenic rice roots expressing the hairpin dsRNA vector targeting Mg-PEL1. These results demonstrated that Mg-PEL1 could be a virulence factor attacking the pectin of plant cell wall to promote nematode infection.
As a pathogenicity or virulence factor attacking plant cell walls, pectate lyases are usually secreted into the plant apoplast via the secretion system of pathogens (Blackman et al., 2014). For example, using immunolocalization analysis, M. incognita-derived pectate lyase Mi-pel3 was found to be localized within the subventral esophageal glands and secreted in the plant apoplast during root invasion of nematodes (Vieira et al., 2011). In PPNs, one of the most important secretory organs is the pharyngeal glands, which are composed of two subventral gland cells and one dorsal gland cell (Haegeman et al., 2012). The subventral glands are more active during nematode penetration and migration in roots (Davis et al., 2008). In our study, Mg-PEL1 is specifically expressed in the subventral esophageal gland cells of M. graminicola by in situ hybridization. Additionally, the developmental expression profile of Mg-PEL1 showed that the highest expression level occurred in the pre-J2 stage, and high expression level persisted in the par-J2 stage. Therefore, combining with the fact of Mg-PEL1 with an N-terminal SP, Mg-PEL1 may probably be secreted during nematode invasion and migration in the roots, therefore playing a role in the penetration and migration of J2 in the host plant root, especially in penetration. It is believed that effector proteins with SP are cotranslationally transported into the endoplasmic reticulum of nematode esophageal gland cells, in which the SP is cleaved and the mature proteins are delivered into host tissue through the stylet of nematode (Elling et al., 2007). This is in line with our experiment that confirms the secretion function of the Mg-PEL1 SP using a yeast trap system.
In Eukaryotes, hydrophobic character of secretion signals is conserved and can be divided into three regions: a positively charge near the N-terminal end (n-region), a hydrophobic stretch of amino acids (h-region) and a polar C-terminal region leading up to the signal peptide cleavage site (Emanuelsson et al., 2000). The hydrophobic character of SP could be conserved between plant and animal (Schaaf et al., 2005). Several native SP containing-effector proteins from root-knot nematodes, including M. incognita-derived effector Mi-CRT, M. graminicola-derived effectors Mg16820 and Mg01965, have also been found to localize in the apoplast of host plant cells when transiently expressed (Jaouannet et al., 2013; Naalden et al., 2018a; Zhuo et al., 2019). However, several other factors besides hydrophobicity can also affect the complex interaction between SP and signal recognition particle, plants and animals are not exactly interchangeable (Matoba and Ogrydziak, 1998; Schaaf et al., 2005), raising an interesting question of whether the SP of Mg-PEL1 can be recognized by plants. Therefore, we used an Agrobacterium-mediated transient expression system to produce Mg-PEL1 with and without its native SP (Mg-PEL1SP and Mg-PEL1–SP) in N. benthamiana. The results showed that they were present both in the cytoplasm and nucleus. This demonstrates that plants may not be able to perceive the native SP of Mg-PEL1 and Mg-PEL1SP could not be transported to the plant apoplast.
The extracellular (apoplast) as a critical battleground for plants-microbes interaction is composed of intercellular space and plant cell wall (Ma Z. et al., 2017). Pathogen-secreted CWDEs including pectate lyases usually localize in the apoplast and degrade plant cell wall (D’Ovidio et al., 2004; Ma Y. et al., 2015; Gui et al., 2017). To deliver Mg-PEL1 into plant extracellular space, the SP of Mg-PEL1 was replaced with an endogenous SP sequence of PR1a (Mg-PEL1PR1a) from N. benthamiana. Subcellular localization showed that Mg-PEL1PR1a can be accumulated at tobacco cells edge. Plasmolysis experiments also indicated that the Mg-PEL1PR1a can target to the cell wall. Interestingly, Mg-PEL1PR1a was capable of triggering host immune responses, including the induction of cell death, activation of defense-related genes and accumulation of hydrogen peroxide. However, neither Mg-PEL1 nor Mg-PEL1–SP can induce host immunity. Plant innate immune system has been known to monitor the apoplastic environment through pattern recognition receptors that can detect fragments of host-derived molecules (DAMPs) as inducers of immune responses (Quintana-rodriguez et al., 2018). For instance, oligogalacturonides (OGs), a well-known fragment of the pectic polysaccharide homogalacturonan, were recognized as DAMPs by the receptor Wall Associated Kinase 1 to activate plant immune responses (De Lorenzo et al., 2011; Ferrari et al., 2013). Pectate lyases from Xanthomonas campestris pv. campestris with enzyme activity hydrolyzed plant pectate and produced OGs, which were subsequently recognized as a DAMP by the plant (Vorholter et al., 2012). In PPN-plant interaction, Arabidopsis polygalacturonase-inhibiting proteins (PGIP) mediated damage-associated responses during cyst nematode invasion of roots (Shah et al., 2017). PPNs secrete cell wall degrading enzymes to release cellular components that act as self-danger molecules known as DAMPs (Kaloshian and Teixeira, 2019; Sato et al., 2019). Combining with the results of Mg-PEL1 with hydrolytic activity toward PGA and immune activation ability relying on cell wall localization, Mg-PEL1 is likely to mediate the degradation of pectin in the plant cell wall, releasing pectin hydrolysis products to trigger defense responses in plants. In contrast, few studies such as HrpW protein belonging to pectin lyases 3 family from Pseudomonas syringae pv. tomato (Charkowski et al., 1998) showed that pectate lyases directly involved in plant immunity elicitation. As such, the possibility of plant immunity elicitation directly by Mg-PEL1 cannot be excluded. In PPNs, effectors GpPDI1 from G. pallida, MgPDI2 from M. graminicola and MG599854 from M. arenaria have been reported to induce cell death in the plant (Grijalva-Mañay et al., 2019; Gross et al., 2020; Tian et al., 2020). Recent studies also showed that pectate lyases have different functions in PPNs. Such as, silencing of Hg-pel-2 that changed the sexual rate in favor of males (Bakhetia et al., 2007). Gr-pel2 transient expression in Nicotiana benthamiana leaves led to severe malformations phenotype (Kudla et al., 2007). Either way, our study demonstrated the first example of PPN pectate lyases experimentally to trigger plant immune responses, although it has been shown previously that oomycete, fungal and bacterial pectate lyases play a role in plant immunity elicitation (Wegener et al., 1996; Poinssot et al., 2003; Fu et al., 2015; Yang et al., 2018).
Despite the ability of Mg-PEL1 to induce plant cell death, conversely, it also acts as an essential virulence factor during M. graminicola infection. It seems to be contradictory with the fact that root-knot nematodes are obligate biotrophic parasites and require living plant cells. Effector proteins with the ability to induce cell death are not preferred as a virulence factor for nematode parasitism. The similar contradictory phenomenon was also observed in several plant-pathogenic oomycetes effectors, such as PsXEG1 from Phytophthora sojae, Avh238 from P. essential, PpE4 from P. parasitica and PlAvh142 from Peronophythora litchii (Ma Z. et al., 2015; Yang et al., 2017; Huang et al., 2019; Situ et al., 2020). Two hypotheses have been raised to explain this. First, the accumulation of effectors or host-derived productions is insufficient to induce plant immune responses under natural conditions. Second, plant immune responses activated by effectors or host-derived productions could be suppressed by other effectors during successful parasitism of pathogens. As a typical example, the immune responses induced by the glycoside hydrolase family 12 protein PsXEG1 from P. sojae can be in turn suppressed by other RXLR effectors (Ma Z. et al., 2015). It will be interesting to determine whether M. graminicola can produce specific effectors to inhibit plant immunity induced by Mg-PEL1. And further studies on the exact mechanisms of plant immunity elicitation by Mg-PEL1 are also needed.
Data Availability Statement
The datasets presented in this study can be found in online repositories. The names of the repository/repositories and accession number(s) can be found in the article/Supplementary Material.
Author Contributions
KZ, JC, and BL: conceived and designed the experiments. JC and ZL: performed the experiments and data analysis. KZ, JC, and JL: manuscript preparation and submission. KZ, JC, and ZL: data curation and investigation. JC and BL: methodology. All authors contributed to the article and approved the submitted version.
Funding
This work was supported by the grants from the National Natural Science Foundation of China (31672007, 31801713, and 31772136).
Conflict of Interest
The authors declare that the research was conducted in the absence of any commercial or financial relationships that could be construed as a potential conflict of interest.
Acknowledgments
We acknowledge Guanghui Kong (South China Agricultural University, China) for providing the plasmid pSUC2 and yeast strain YTK12.
Supplementary Material
The Supplementary Material for this article can be found online at: https://www.frontiersin.org/articles/10.3389/fpls.2021.651627/full#supplementary-material
Footnotes
- ^ https://www.genome.jp/tools-bin/clustalw
- ^ http://sourceforge.net/projects/boxshade/
- ^ http://www.cbs.dtu.dk/services/SignalP/
- ^ http://www.cbs.dtu.dk/services/TMHMM/
References
Bakhetia, M., Urwin, P. E., and Atkinson, H. J. (2007). qPCR analysis and RNAi define pharyngeal gland cell-expressed genes of Heterodera glycines required for initial interactions with the host. Mol. Plant Microbe Interact. 20, 306–312. doi: 10.1094/MPMI-20-3-0306
Blackman, L. M., Cullerne, D. P., and Hardham, A. R. (2014). Bioinformatic characterization of genes encoding cell wall degrading enzymes in the Phytophthora parasitica genome. BMC Genomics 15:785. doi: 10.1186/1471-2164-15-785
Caffall, K. H., and Mohnen, D. (2009). The structure, function, and biosynthesis of plant cell wall pectic polysaccharides. Carbohydr. Res. 344, 1879–1900. doi: 10.1016/j.carres.2009.05.021
Charkowski, A. O., Alfano, J. R., Preston, G., Yuan, J., He, S. Y., and Collmer, A. (1998). The Pseudomonas syringae pv. tomato HrpW protein has domains similar to harpins and pectate lyases and can elicit the plant hypersensitive response and bind to pectate. J. Bacteriol. 180, 5211–5217. doi: 10.1128/JB.180.19.5211-5217.1998
Chen, J., Lin, B., Huang, Q., Hu, L., Zhuo, K., and Liao, J. (2017). A novel Meloidogyne graminicola effector, MgGPP, is secreted into host cells and undergoes glycosylation in concert with proteolysis to suppress plant defenses and promote parasitism. PLoS Pathog. 13:e1006301. doi: 10.1371/journal.ppat.1006301
Choi, H. W., and Klessig, D. F. (2016). DAMPs, MAMPs, and NAMPs in plant innate immunity. BMC Plant Biol. 16:232. doi: 10.1186/s12870-016-0921-2
Couto, D., and Zipfel, C. J. (2016). Regulation of pattern recognition receptor signalling in plants. Nat. Rev. Immunol. 16, 537–552. doi: 10.1038/nri.2016.77
Daudi, A., and O’Brien, J. A. (2012). Detection of hydrogen peroxide by DAB staining in Arabidopsis leaves. Bio. Protoc. 2:e263. doi: 10.21769/BioProtoc.263
Davis, E. L., Hussey, R. S., Mitchum, M. G., and Baum, T. J. (2008). Parasitism proteins in nematode–plant interactions. Curr. Opin. Plant Biol. 11, 360–366. doi: 10.1016/j.pbi.2008.04.003
de Boer, J. M., Yan, Y., Smant, G., Davis, E. L., and Baum, T. J. (1998). In-situ hybridization to messenger RNA in Heterodera glycines. J. Nematol. 30, 309–312.
De Lorenzo, G., Brutus, A., Savatin, D. V., Sicilia, F., and Cervone, F. (2011). Engineering plant resistance by constructing chimeric receptors that recognize damage-associated molecular patterns (DAMPs). FEBS Lett. 585, 1521–1528. doi: 10.1016/j.febslet.2011.04.043
Dou, D., Kale, S. D., Wang, X., Jiang, R. H., Bruce, N. A., Arredondo, F. D., et al. (2008). RXLR-mediated entry of Phytophthora sojae effector Avr1b into soybean cells does not require pathogen-encoded machinery. Plant Cell 20, 1930–1947. doi: 10.1105/tpc.107.056093
D’Ovidio, R., Mattei, B., Roberti, S., and Bellincampi, D. (2004). Polygalacturonases, polygalacturonase-inhibiting proteins and pectic oligomers in plant-pathogen interactions. Biochim. Biophys. Acta 1696, 237–244. doi: 10.1016/j.bbapap.2003.08.012
Doyle, E. A., and Lambert, K. N. (2002). Cloning and characterization of an esophageal-gland-specific pectate lyase from the root-knot nematode Meloidogyne javanica. Mol. Plant Microbe Interact. 15, 549–556. doi: 10.1094/MPMI.2002.15.6.549
Elling, A. A., Davis, E. L., Hussey, R. S., and Baum, T. J. (2007). Active uptake of cyst nematode parasitism proteins into the cell nucleus. Int. J. Parasitol. 37, 1269–1279. doi: 10.1016/j.ijpara.2007.03.012
Emanuelsson, O., Nielsen, H., Brunak, S., and Von Heijne, G. (2000). Predicting subcellular localization of proteins based on their N-terminal amino acid sequence. J. Mol. Biol. 300, 1005–1016. doi: 10.1006/jmbi.2000.3903
Ferrari, S., Savatin, D. V., Sicilia, F., Gramegna, G., Cervone, F., and Lorenzo, G. D. (2013). Oligogalacturonides: plant damage-associated molecular patterns and regulators of growth and development. Front. Plant Sci. 4:49. doi: 10.3389/fpls.2013.00049
Fu, L., Zhu, C., Ding, X., Yang, X., Morris, P. F., Tyler, B. M., et al. (2015). Characterization of cell-death-inducing members of the pectate lyase gene family in Phytophthora capsici and their contributions to infection of pepper. Mol. Plant Microbe Interact. 28, 766–775. doi: 10.1094/MPMI-11-14-0352-R
Gietz, R. D., and Schiestl, R. H. (2007). Large-scale high-efficiency yeast transformation using the LiAc/SS carrier DNA/PEG method. Nat. Protoc. 2, 38–41. doi: 10.1038/nprot.2007.15
Goldman, A., Harper, S., and Speicher, D. W. (2016). Detection of proteins on blot membranes. Curr. Protoc. Protein Sci. 86, 11–10. doi: 10.1002/cpps.15
Grijalva-Mañay, R., Dorca-Fornell, C., Enríquez-Villacreses, W., Miño-Castro, G., Oliva, R., Ochoa, V., et al. (2019). DnaJ molecules as potential effectors in Meloidogyne arenaria. An unexplored group of proteins in plant parasitic nematodes. Commun. Integr. Biol. 12, 151–161. doi: 10.1080/19420889.2019.1676138
Gross, R., Zhang, S., Wei, L., Caplan, A., Kuhl, J., Dandurand, L. M., et al. (2020). The Globodera pallida effector GpPDI1 is a functional thioredoxin and triggers defense-related cell death independent of its enzymatic activity. Phytopathology 110, 1838–1844. doi: 10.1094/PHYTO-02-20-0038-R
Gui, Y. J., Chen, J. Y., Zhang, D. D., Li, N. Y., Li, T. G., Zhang, W. Q., et al. (2017). Verticillium dahliae manipulates plant immunity by glycoside hydrolase 12 proteins in conjunction with carbohydrate-binding module 1. Environ. Microbiol. 19, 1914–1932. doi: 10.1111/1462-2920.13695
Haegeman, A., Bauters, L., Kyndt, T., Rahman, M. M., and Gheysen, G. (2013). Identification of candidate effector genes in the transcriptome of the rice root knot nematode Meloidogyne graminicola. Mol. Plant Pathol. 14, 379–390. doi: 10.1111/mpp.12014
Haegeman, A., Mantelin, S., Jones, J. T., and Gheysen, G. (2012). Functional roles of effectors of plant-parasitic nematodes. Gene 492, 19–31. doi: 10.1016/j.gene.2011.10.040
Heese, A., Hann, D. R., Gimenez-Ibanez, S., Jones, A. M., He, K., Li, J., et al. (2007). The receptor-like kinase SERK3/BAK1 is a central regulator of innate immunity in plants. Proc. Natl. Acad. Sci U.S.A. 104, 12217–12222. doi: 10.1073/pnas.0705306104
Hou, S., Liu, Z., Shen, H., and Wu, D. (2019). Damage-associated molecular pattern-triggered immunity in plants. Front. Plant Sci. 10:646. doi: 10.3389/fpls.2019.00646
Huang, G., Dong, R., Allen, R., Davis, E. L., Baum, T. J., and Hussey, R. S. (2005). Developmental expression and molecular analysis of two Meloidogyne incognita pectate lyase genes. Int. J. Parasitol. 35, 685–692. doi: 10.1016/j.ijpara.2005.01.006
Huang, G., Liu, Z., Gu, B., Zhao, H., Jia, J., Fan, G., et al. (2019). An RXLR effector secreted by Phytophthora parasitica is a virulence factor and triggers cell death in various plants. Mol. Plant Pathol. 20, 356–371. doi: 10.1111/mpp.12760
Huang, Q., Song, H., Lin, B., Zheng, X., Wang, W., Liao, J., et al. (2020). Arabidopsis thaliana as a model plant to study host-Meloidogyne graminicola interactions. Nematology 1, 1–10. doi: 10.1163/15685411-bja10008
Hugouvieux-Cotte-Pattat, N., Condemine, G., and Shevchik, V. E. (2014). Bacterial pectate lyases, structural and functional diversity. Environ. Microbiol Rep. 6, 427–440. doi: 10.1111/1758-2229.12166
Jaouannet, M., Magliano, M., Arguel, M. J., Gourgues, M., Evangelisti, E., Abad, P., et al. (2013). The root-knot nematode calreticulin Mi-CRT is a key effector in plant defense suppression. Mol. Plant Microbe Interact. 26, 97–105. doi: 10.1094/MPMI-05-12-0130-R
Jones, J. D., and Dangl, J. L. (2006). The plant immune system. Nature 444, 323–329. doi: 10.1038/nature05286
Kaloshian, I., and Teixeira, M. (2019). Advances in plant-nematode interactions with emphasis on the notorious nematode genus Meloidogyne. Phytopathology 109, 1988–1996. doi: 10.1094/PHYTO-05-19-0163-IA
Karim, N., Jones, J. T., Okada, H., and Kikuchi, T. (2009). Analysis of expressed sequence tags and identification of genes encoding cell-wall-degrading enzymes from the fungivorous nematode Aphelenchus avenae. BMC Genomics 10:525. doi: 10.1186/1471-2164-10-525
Kikot, G. E., Hours, R. A., and Alconada, T. M. (2009). Contribution of cell wall degrading enzymes to pathogenesis of Fusarium graminearum: a review. J. Basic Microbiol. 49, 231–241. doi: 10.1002/jobm.200800231
Kikuchi, T., Shibuya, H., Aikawa, T., and Jones, J. T. (2006). Cloning and characterization of pectate lyases expressed in the esophageal gland of the pine wood nematode Bursaphelenchus xylophilus. Mol. Plant Microbe Interact. 19, 280–287. doi: 10.1094/MPMI-19-0280
Kim, J. S., Chae, S., Jun, K. M., Pahk, Y. M., Lee, T. H., Chung, P. J., et al. (2017). Genome-wide identification of grain filling genes regulated by the OsSMF1 transcription factor in rice. Rice 10:16. doi: 10.1186/s12284-017-0155-4
Kubicek, C. P., Starr, T. L., and Glass, N. L. (2014). Plant cell wall-degrading enzymes and their secretion in plant-pathogenic fungi. Annu. Rev. Phytopathol. 52, 427–451. doi: 10.1146/annurev-phyto-102313-045831
Kudla, U., Milac, A. L., Qin, L., Overmars, H., Roze, E., Holterman, M., et al. (2007). Structural and functional characterization of a novel, host penetration-related pectate lyase from the potato cyst nematode Globodera rostochiensis. Mol. Plant Pathol. 8, 293–305. doi: 10.1111/j.1364-3703.2007.00394.x
Kudla, U., Qin, L., Milac, A., Kielak, A., Maissen, C., Overmars, H., et al. (2005). Origin, distribution and 3D-modeling of Gr-EXPB1, an expansin from the potato cyst nematode Globodera rostochiensis. FEBS Lett. 579, 2451–2457. doi: 10.1016/j.febslet.2005.03.047
Kumar, S., Stecher, G., and Tamura, K. (2016). MEGA7: molecular evolutionary genetics analysis version 7.0 for bigger datasets. Mol. Biol. Evol. 33, 1870–1874. doi: 10.1093/molbev/msw054
Lerouxel, O., Cavalier, D., Liepman, A. H., and Keegstra, K. J. (2006). Biosynthesis of plant cell wall polysaccharides–a complex process. Curr. Opin. Plant Biol. 9, 621–630. doi: 10.1016/j.pbi.2006.09.009
Liu, J., Peng, H., Cui, J., Huang, W., Kong, L., Clarke, J. L., et al. (2016). Molecular characterization of a novel effector expansin-like protein from Heterodera avenae that induces cell death in Nicotiana benthamiana. Sci. Rep. 6:35677. doi: 10.1038/srep35677
Livak, K. J., and Schmittgen, T. D. (2001). Analysis of relative gene expression data using real-time quantitative PCR and the 2(-Delta Delta C(T)) Method. Methods 25, 402–408. doi: 10.1006/meth.2001.1262
Ma, Y., Han, C., Chen, J., Li, H., He, K., Liu, A., et al. (2015). Fungal cellulase is an elicitor but its enzymatic activity is not required for its elicitor activity. Mol. Plant Pathol. 16, 14–26. doi: 10.1111/mpp.12156
Ma, Z., Song, T., Zhu, L., Ye, W., Wang, Y., Shao, Y., et al. (2015). A Phytophthora sojae glycoside hydrolase 12 protein is a major virulence factor during soybean infection and is recognized as a PAMP. Plant Cell 27, 2057–2072. doi: 10.1105/tpc.15.00390
Ma, Z., Zhu, L., Song, T., Wang, Y., Zhang, Q., Xia, Y., et al. (2017). A paralogous decoy protects Phytophthora sojae apoplastic effector PsXEG1 from a host inhibitor. Science 355, 710–714. doi: 10.1126/science.aai7919
Matoba, S., and Ogrydziak, D. M. (1998). Another factor besides hydrophobicity can affect signal peptide interaction with signal recognition particle. J. Biol. Chem. 273, 18841–18847. doi: 10.1074/jbc.273.30.18841
Naalden, D., Haegeman, A., de Almeida-Engler, J., Birhane Eshetu, F., Bauters, L., and Gheysen, G. (2018a). The Meloidogyne graminicola effector Mg16820 is secreted in the apoplast and cytoplasm to suppress plant host defense responses. Mol. Plant Pathol. 19, 2416–2430. doi: 10.1111/mpp.12719
Naalden, D., Verbeek, R., and Gheysen, G. (2018b). Nicotiana benthamiana as model plant for Meloidogyne graminicola infection. Nematology 20, 491–499. doi: 10.1163/15685411-00003154
Payasi, A., Sanwal, R., and Sanwal, G. G. (2009). Microbial pectate lyases: characterization and enzymological properties. World J. Microbiol Biotechnol. 25, 1–14. doi: 10.1007/s11274-008-9870-8
Pecenkova, T., Pleskot, R., and Zarsky, V. (2017). Subcellular localization of Arabidopsis pathogenesis-related 1 (PR1) protein. Int. J. Mol. Sci. 18:825. doi: 10.3390/ijms1804082
Peng, H., Cui, J., Long, H., Huang, W., Kong, L., Liu, S., et al. (2016). Novel pectate lyase genes of Heterodera glycines play key roles in the early stage of parasitism. PLoS One 11:e0149959. doi: 10.1371/journal.pone.0149959
Pereira, E. O., Kolotilin, I., Conley, A. J., and Menassa, R. (2014). Production and characterization of in planta transiently produced polygalacturanase from Aspergillus niger and its fusions with hydrophobin or ELP tags. BMC Biotechnol. 14:59. doi: 10.1186/1472-6750-14-59
Poinssot, B., Vandelle, E., Bentejac, M., Adrian, M., Levis, C., Brygoo, Y., et al. (2003). The endopolygalacturonase 1 from Botrytis cinerea activates grapevine defense reactions unrelated to its enzymatic activity. Mol. Plant Microbe Interact. 16, 553–564. doi: 10.1094/MPMI.2003.16.6.553
Popeijus, H., Overmars, H., Jones, J., Blok, V., Goverse, A., Helder, J., et al. (2000). Degradation of plant cell walls by a nematode. Nature 406, 36–37. doi: 10.1038/35017641
Quintana-rodriguez, E., Duranflores, D., Heil, M., and Camacho-Coronel, X. J. (2018). Damage-associated molecular patterns (DAMPs) as future plant vaccines that protect crops from pests. Sci. Hortic. 237, 207–220. doi: 10.1016/j.scienta.2018.03.026
Rodriguez, P. A., Rothballer, M., Chowdhury, S. P., Nussbaumer, T., Gutjahr, C., and Falter-Braun, P. (2019). Systems biology of plant-microbiome interactions. Mol. Plant. 12, 804–821. doi: 10.1016/j.molp.2019.05.006
Sato, K., Kadota, Y., and Shirasu, K. (2019). Plant immune responses to parasitic nematodes. Front. Plant Sci. 10:1165. doi: 10.3389/fpls.2019.01165
Schaaf, A., Tintelnot, S., Baur, A., Reski, R., Gorr, G., and Decker, E. L. (2005). Use of endogenous signal sequences for transient production and efficient secretion by moss (Physcomitrella patens) cells. BMC Biotechnol. 5:30. doi: 10.1186/1472-6750-5-30
Shah, S. J., Anjam, M. S., Mendy, B., Anwer, M. A., Habash, S. S., Lozano-Torres, J. L., et al. (2017). Damage-associated responses of the host contribute to defence against cyst nematodes but not root-knot nematodes. J. Exp. Bot. 68, 5949–5960.
Shevchik, V. E., Robert-baudouy, J., and Hugouvieux-cotte-pattat, N. J. (1997). Pectate lyase PelI of Erwinia chrysanthemi 3937 belongs to a new family. J. Bacteriol. 179, 7321–7330. doi: 10.1128/jb.179.23.7321-7330.1997
Situ, J., Jiang, L., Fan, X., Yang, W., Li, W., Xi, P., et al. (2020). An RXLR effector PlAvh142 from Peronophythora litchii triggers plant cell death and contributes to virulence. Mol. Plant Pathol. 21, 415–428. doi: 10.1111/mpp.12905
Soitamo, A. J., Jada, B., and Lehto, K. (2011). HC-Pro silencing suppressor significantly alters the gene expression profile in tobacco leaves and flowers. BMC Plant Biol. 11:68. doi: 10.1186/1471-2229-11-68
Starr, M. P., Chatterjee, A. K., Starr, P. B., and Buchanan, G. E. (1977). Enzymatic degradation of polygalacturonic acid by Yersinia and Klebsiella species in relation to clinical laboratory procedures. J. Clin. Microbiol. 6, 379–386.
Tian, Z., Wang, Z., Munawar, M., and Zheng, J. (2020). Identification and characterization of a novel protein disulfide isomerase gene (MgPDI2) from Meloidogyne graminicola. Int. J. Mol. Sci. 21:9586. doi: 10.3390/ijms21249586
Vanholme, B., Van Thuyne, W., Vanhouteghem, K., De Meutter, J., Cannoot, B., and Gheysen, G. J. (2007). Molecular characterization and functional importance of pectate lyase secreted by the cyst nematode Heterodera schachtii. Mol. Plant Pathol. 8, 267–278. doi: 10.1111/j.1364-3703.2007.00392.x
Vieira, P., Danchin, E. G., Neveu, C., Crozat, C., Jaubert, S., Hussey, R. S., et al. (2011). The plant apoplasm is an important recipient compartment for nematode secreted proteins. J. Exp. Bot. 62, 1241–1253. doi: 10.1093/jxb/erq352
Vorholter, F. J., Wiggerich, H. G., Scheidle, H., Sidhu, V. K., Mrozek, K., Kuster, H., et al. (2012). Involvement of bacterial TonB-dependent signaling in the generation of an oligogalacturonide damage-associated molecular pattern from plant cell walls exposed to Xanthomonas campestris pv. campestris pectate lyases. BMC Microbiol. 12:239. doi: 10.1186/1471-2180-12-239
Wegener, C., Bartling, S., Olsen, O., Weber, J., and Von Wettstein, D. (1996). Pectate lyase in transgenic potatoes confers pre-activation of defence against Erwinia carotovora. Physiol. Mol. Plant. 49, 359–376. doi: 10.1006/pmpp.1996.9998
Wilkinson, S. W., Magerøy, M. H., López Sánchez, A., Smith, L. M., Furci, L., Cotton, T. A., et al. (2019). Surviving in a hostile world: plant strategies to resist pests and diseases. Annu. Rev. Phytopathol. 57, 505–529. doi: 10.1146/annurev-phyto-082718-095959
Win, J., Chaparro-Garcia, A., Belhaj, K., Saunders, D. G., Yoshida, K., Dong, S., et al. (2012). Effector biology of plant-associated organisms: concepts and perspectives. Cold Spring Harb. Symp. Quant Biol. 77, 235–247. doi: 10.1101/sqb.2012.77.015933
Yang, B., Wang, Q., Jing, M., Guo, B., Wu, J., Wang, H., et al. (2017). Distinct regions of the Phytophthora essential effector Avh238 determine its function in cell death activation and plant immunity suppression. New Phytol. 214, 361–375. doi: 10.1111/nph.14430
Yang, Y., Zhang, Y., Li, B., Yang, X., Dong, Y., and Qiu, D. (2018). A Verticillium dahliae pectate lyase induces plant immune responses and contributes to virulence. Front. Plant Sci. 9:1271. doi: 10.3389/fpls.2018.01271
Yin, W., Wang, Y., Chen, T., Lin, Y., and Luo, C. (2018). Functional evaluation of the signal peptides of secreted proteins. Bio. Protoc. 8:e2839. doi: 10.21769/BioProtoc.2839
Zhuo, K., Chi, Y., Hu, L., Luo, M., and Liao, J. (2011). Cloning and RNA interference analysis of a pectate lyase gene of Meloidogyne enterolobii. Acta Phytopathol. Sin. 41, 473–481.
Keywords: Meloidogyne graminicola, pectate lyase, enzymatic activity, plant immunity elicitation, RNAi
Citation: Chen J, Li Z, Lin B, Liao J and Zhuo K (2021) A Meloidogyne graminicola Pectate Lyase Is Involved in Virulence and Activation of Host Defense Responses. Front. Plant Sci. 12:651627. doi: 10.3389/fpls.2021.651627
Received: 13 January 2021; Accepted: 22 February 2021;
Published: 22 March 2021.
Edited by:
Carolina Escobar, University of Castilla-La Mancha, SpainReviewed by:
Michaël Quentin, Université Côte d’Azur, FranceLander Bauters, Ghent University, Belgium
Copyright © 2021 Chen, Li, Lin, Liao and Zhuo. This is an open-access article distributed under the terms of the Creative Commons Attribution License (CC BY). The use, distribution or reproduction in other forums is permitted, provided the original author(s) and the copyright owner(s) are credited and that the original publication in this journal is cited, in accordance with accepted academic practice. No use, distribution or reproduction is permitted which does not comply with these terms.
*Correspondence: Kan Zhuo, zhuokan@scau.edu.cn