- Molecular Plant Biology, Department of Biochemistry, University of Turku, Turku, Finland
Absence of the Proton Gradient Regulation 5 (PGR5) protein from plant chloroplasts prevents the induction of strong trans-thylakoid proton gradient (ΔpH) and consequently also the thermal dissipation of excess energy (NPQ). The absence of the PSBS protein likewise prevents the formation of ΔpH-dependent NPQ. This component of NPQ is called qE, which is nearly exclusively responsible for induction of NPQ upon increase in light intensity. On the other hand, the pgr5 mutant is not only deficient in induction of strong NPQ but it also lacks the capability to oxidize P700 upon increase in light intensity. This, in turn, results from uncontrolled electron flow toward photosystem I (PSI), which has been proposed to be caused by the lack of PSII down-regulation by NPQ and by a poor control of electron flow via the Cytochrome b6f (Cyt b6f) complex. Here we asked whether NPQ really is a component of such regulation of electron flow from PSII to PSI at high light. To this end, the two NPQ mutants pgr5 and npq4, the latter lacking the PSBS protein, were characterized. It is shown that the npq4 mutant, despite its highly reduced Plastoquinone pool, does not inhibit but rather enhances the oxidation of P700 in high light as compared to wild type. This clearly demonstrates that the control of electron flow from PSII to PSI cannot be assigned, even partially, to the down-regulation of PSII by NPQ but apparently takes place solely in Cyt b6f. Moreover, it is shown that the pgr5 mutant can induce NPQ in very high light, but still remains deficient in P700 oxidation. These results challenge the suggestion that NPQ, induced by PGR5-dependent cyclic electron transfer, would have a key role in regulation of electron transfer from PSII to PSI. Instead, the results presented here are in line with our recent suggestion that both PSII and PSI function under the same light harvesting machinery regulated by ΔpH and the PSBS protein (Tikkanen and Aro, 2014; Grieco et al., 2015).
Introduction
Solar energy is converted into chemical form by photosynthetic light reactions, which in plants and green algae take place in the thylakoid membrane inside the chloroplasts. Safe and efficient function of the photosynthetic light reactions is based on synchronized function of the light-driven enzymes photosystem II (PSII) and photosystem I (PSI), the former splitting water to protons and electrons and the latter using electrons to reduce NADP to NADPH. Electron transfer from PSII to PSI takes place via the intersystem electron transfer chain composed of Plastoquinone (PQ), Cytochrome b6f (Cyt b6f), and Plastocyanin (PC). PQ accepts electrons from PSII and the electrons are then transferred to PSI via Cyt b6f and PC. The electron transfer reactions in Cyt b6f are coupled to transfer of protons from chloroplast stroma to thylakoid lumen (Q cycle). This reaction not only facilitates the generation of trans-thylakoid proton gradient (ΔpH) but also allows the ΔpH to control the rate of electron transfer to PSI (Joliot and Johnson, 2011; Tikhonov, 2014; Tikkanen and Aro, 2014). This is because the oxidation of plastoquinol at the Qo site is the rate limiting step of the electron transfer (Stiehl and Witt, 1969), making the rate of electron transfer dependent on ΔpH.
Photosystem II and Photosystem I have their own minor light harvesting antennae, but the energy capture to both photosystems is largely based on the major light harvesting system that is embedded in the thylakoid membrane and composed of LHCII trimers (Wientjes et al., 2013; Grieco et al., 2015). The distribution of excitation energy from the LHCII system to PSII and PSI is redox regulated (See for review: Allen et al., 1981; Murata, 2009). This regulation is based on phosphorylation of thylakoid proteins and required to maintain the functional balance between PSII and PSI upon changes in light quality (Allen et al., 1981; Mekala et al., 2015) and quantity (Mekala et al., 2015). Efficiency of the LHCII system, in turn, is regulated by ΔpH and is dependent on the PSBS protein (Li et al., 2000; Niyogi and Truong, 2013). The ΔpH generated by PSII and the Q cycle is released by ATP synthase in a reaction utilizing the proton motive force. Thus, ΔpH is eventually determined by the ratio between the ΔpH generation and release mechanisms according to the energetic state of the chloroplast (Kanazawa and Kramer, 2002; Kohzuma et al., 2013).
It is not fully understood how the regulation of trans-thylakoid ΔpH actually occurs according to the light intensity and the energetic state of the chloroplast. Nevertheless, it has been clearly demonstrated that strengthening of ΔpH upon increase in light intensity is dependent on proteins Proton Gradient Regulation 5 (PGR5) and Proton Gradient Regulation Like 1 (PGRL1; Munekage et al., 2002; DalCorso et al., 2008). Traditionally, the PGR5 protein is linked to the cyclic electron flow around PSI (CET) via putative Ferredoxin (FD) -PQ oxidoreductase (FQR; Munekage et al., 2002). By this mechanism, PGR5 is supposed to enhance the generation of ΔpH and thereby accelerate the induction of NPQ and slow down the Q cycle. This model, however, is paradoxical since it states that the slowdown of electron transfer (occurring in the Cyt b6f complex) results from acceleration of electron transfer via Cyt b6f complex (in CET). Based on this paradox, it has also been proposed that PGR5 simply prevents the leaking of protons from the lumen to chloroplast stroma by a still uncharacterized mechanism (Avenson et al., 2005). Whatever the mechanism, it is clear that the pgr5 mutant cannot increase the trans-thylakoid ΔpH and consequently fails in the PSBS protein-dependent thermal dissipation of excess excitation energy (NPQ) upon increase in light intensity (Munekage et al., 2002; Suorsa et al., 2012). Due to the impaired control of electron transfer via Cyt b6f and low thermal dissipation of excess excitation energy, PSI of the pgr5 mutant is sensitive for photoinhibition and has reduced amount of PSI (Munekage et al., 2002). The amount of PSI is adjusted according to the acceptor side limitation of PSI, which in turn is dependent on the light intensity, the capacity of carbon metabolism and the amount of active PSII (Munekage et al., 2002, 2008; Suorsa et al., 2012; Tikkanen et al., 2014).
Generally, NPQ is considered as a mechanism that specifically down-regulates the activity of PSII and therefore is supposed to limit electron transfer to PSI. However, based on the behavior of mutants disturbed in the distribution of excitation energy from the LHCII system to PSII and PSI (Tikkanen et al., 2010, 2011; Grieco et al., 2012), it seems highly likely that NPQ downregulates both photosystems to similar extent. Indeed, only in a specific case of the stn7 mutant when energy distribution from the LHCII system to PSI is impaired, the relaxation of NPQ in low light selectively affects only PSII leading to high reduction of the PQ pool (Tikkanen et al., 2010, 2011; Grieco et al., 2012). This challenges the idea that NPQ is required for oxidation of P700 in high light. Moreover, it has been reported that PSBS mutants can oxidize P700 upon increase in light intensity (Grieco et al., 2012; Roach and Krieger-Liszkay, 2012) indicating that the PSBS-dependent mechanism does not specifically downregulate PSII, but rather affects both photosystems. Previously the interaction between proton gradient-dependent regulation of electron transfer and NPQ was studied in Chlamydomonas reinhardtii (Kukuczka et al., 2014). It was shown that the two mechanisms are complementary, both of them being needed for high light acclimation in oxygen limiting conditions.
Here, we demonstrate that the PSBS protein- and ΔpH-dependent NPQ are needed to prevent over-reduction of the PQ pool at high light, but importantly, NPQ is not required for oxidation of P700 at high light. On the contrary, the oxidation of P700 at high light is even enhanced in the npq4 mutant as compared to wild type (WT), indicating that in the absence of PSBS-dependent NPQ the excitation energy transfer to PSI is enhanced. This also points out that the deficiency of the pgr5 mutant to oxidize P700 cannot result from the deficient NPQ, but more likely solely from the missing photosynthetic control via Cyt b6f.
Materials and Methods
Wild type (ecotype Columbia) and mutant lines pgr5 (Munekage et al., 2002) and npq4 (Li et al., 2000) of Arabidopsis thaliana were grown at 23°C and in 60% relative humidity under an 8-h photoperiod of constant white light (110–120 μmol photons m-2 s-1) with OSRAM PowerStar HQIT 400/D Metal Halide lamps as a light source. Leaves from 5-weeks-old plants were used for the experiments. Detached leaves with petioles submerged in tap water were incubated 10 min in darkness before the measurements. For each lineage, leaves from three different plants were analyzed and SD was calculated with formula .
Chlorophyll a fluorescence and signal from oxidized P700 (Klughammer and Schreiber, 1994, 2008) were detected with Dual-PAM-100 (Heinz Walz). A saturating pulse (5000 μmol photons m-2 s-1 for 500 ms) was applied in every 1 min with increasing 635-nm actinic light (50, 127, 274, 661, and 1595 μmol photons m-2 s-1 or 127 and 1953 μmol photons m-2 s-1). Chlorophyll a fluorescence was detected with 460-nm measuring light (19 μmol photons m-2 s-1) and oxidation state of P700 was determined based on the difference of intensities 875 nm and 830 nm of pulse-modulated measuring light reaching the photodetector (Klughammer and Schreiber, 2008). PSI redox state (P/Pm) was obtained by normalizing the signal of oxidized P700 at a given light phase (P) to the signal of maximal proportion of oxidized P700 under a saturating pulse with far-red background (Pm; Klughammer and Schreiber, 1994). Relative reduction of QA (F′/Fm) was determined by normalizing the fluorescence under actinic light (F′) to the maximal fluorescence of dark-adapted leaf (Fm). Induction of NPQ (1–Fm′/Fm) was calculated by reversing the maximal fluorescence from a light-adapted leaf (Fm′) normalized to the maximal fluorescence of a dark-adapted leaf (Fm).
Results
To clarify the differential roles of ΔpH-dependent control of Cyt b6f and NPQ in regulation of electron flow from PSII to PSI at high light, we investigated WT, pgr5, and npq4 with respect to the redox state of PSI, redox state of PSII electron acceptors and induction of NPQ upon changes in illumination conditions. The functional phenotypes of the mutants were addressed by applying saturating pulses with actinic light intensity increasing either gradually (50, 127, 274, 661, and 1595 μmol photons m-2 s-1; Figure 1) or in two steps from darkness to light slightly higher that growth light (127 μmol photons m-2 s-1) and subsequently to very high light (1953 μmol photons m-2 s-1; Figure 2).
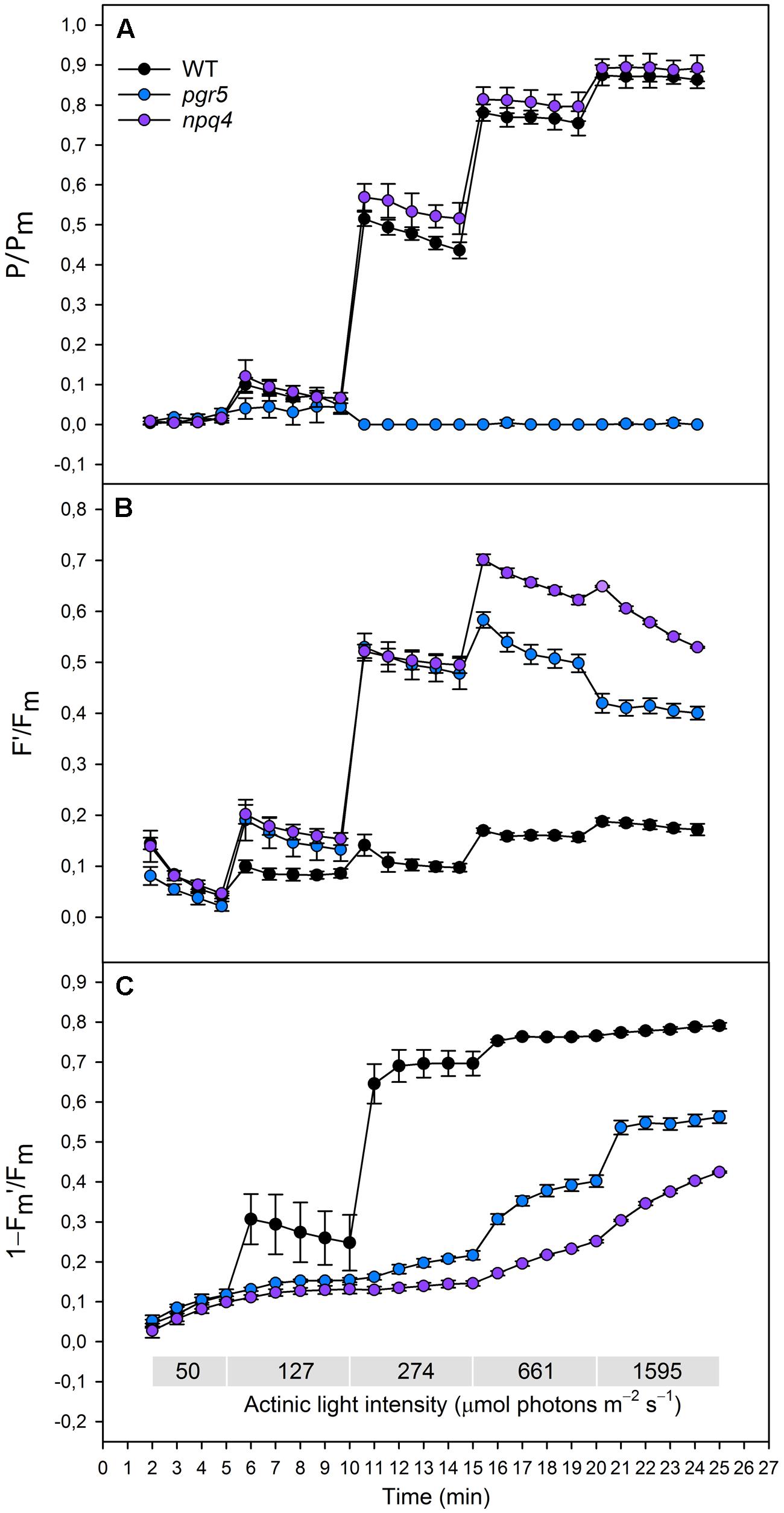
FIGURE 1. (A)(P/Pm), (B) (F′/Fm), (C) (1–Fm′/Fm) in wild type (black dots), pgr5 (blue dots), and npq4 (purple dots) during a stepwise increase in actinic light intensity. Saturating pulse was applied in every 1 min with gradually increasing actinic light intensity (50, 127, 274, 661, and 1595 μmol photons m-2 s-1). Detached leaves were incubated in darkness 10 min before measurements. Representative data is shown with three different plants measured of each lineage.
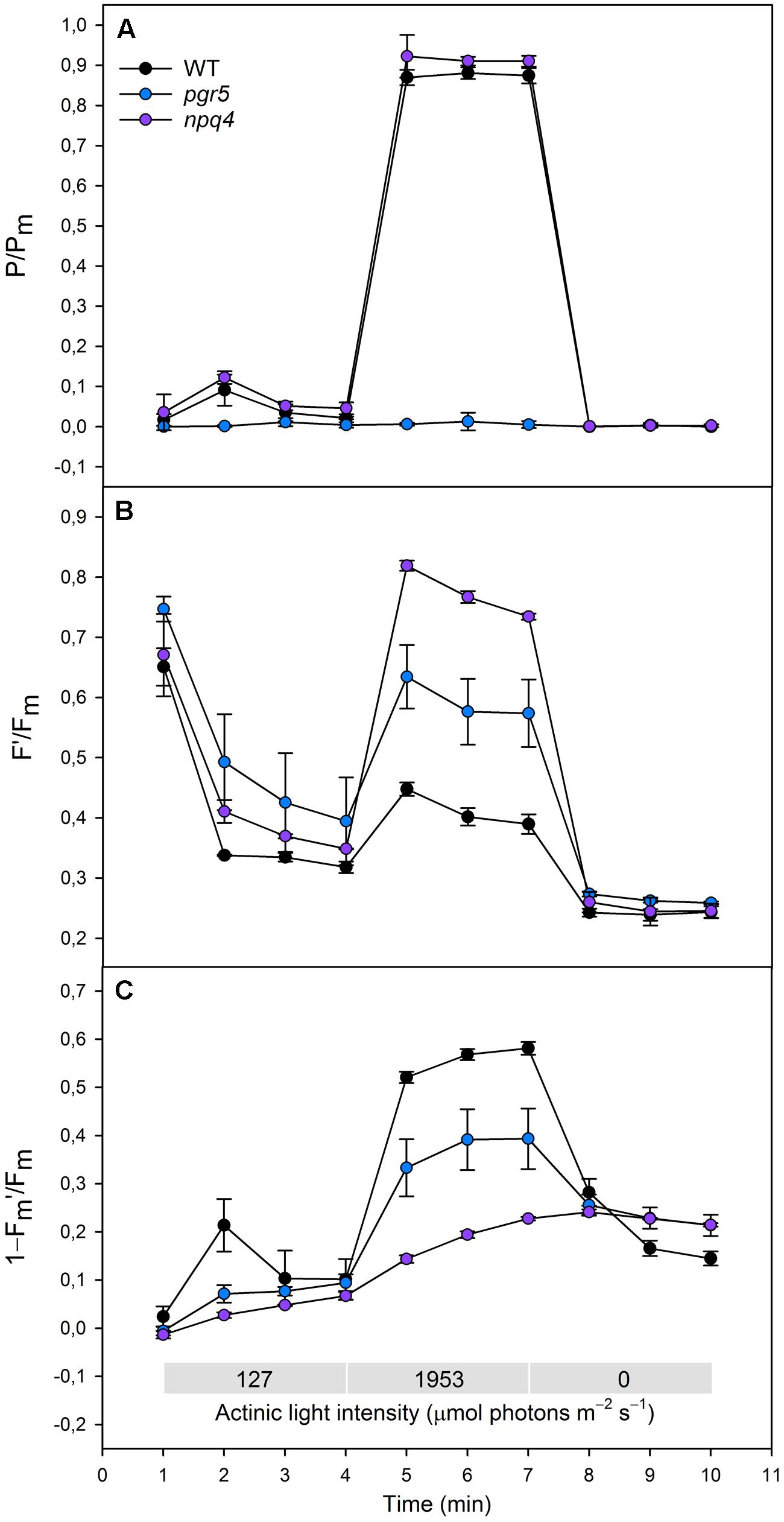
FIGURE 2. (A)(P/Pm), (B) (F′/Fm), (C) (1–Fm′/Fm) in WT (black dots), pgr5 (blue dots), and npq4 (purple dots) during a steep increase in actinic light intensity. Saturating pulse was applied in every 1 min with a non-gradual increase in the actinic light intensity (0, 127, and 1953 μmol photons m-2 s-1). Detached leaves were incubated in darkness 10 min before measurements. Representative data is shown with three different plants measured of each lineage.
Redox state of PSI was determined by normalizing the signal of oxidized P700 to the signal of maximal proportion of oxidized P700 (P/Pm). When increasing the actinic light intensity stepwise, the npq4 mutant showed a higher oxidation level of P700 at moderate high light intensities (274 and 661 μmol photons m-2 s-1), whereas no difference between npq4 and WT was detected at lower or higher intensities (Figure 1A). The steep increase in actinic light intensity, on the other hand, resulted in more substantial difference in the oxidation of P700 between npq4 and WT (Figure 2A). In pgr5, P700 oxidized slightly during the low actinic light intensities (50 and 127 μmol photons m-2 s-1), but remained reduced under higher intensities (Figure 1A) and throughout the drastic increase of actinic light intensity (Figure 2A).
To study the redox state PSII acceptor side, the redox state of QA was estimated by normalizing fluorescence to the maximal fluorescence (F′/Fm). Although the fluorescence normalized to the maximal fluorescence does not linearly correlate with [QA-] due to the antenna connectivity (Lavergne and Trissl, 1995; Joliot and Joliot, 2003), the parameter F′/Fm is the best parameter for the mutants with severely altered behaviour of both Fm′ and F′. During the gradual increase of actinic light intensity, WT maintained its F′/Fm level, whereas pgr5 and npq4 showed a drastic increase in the parameter already at light slightly higher that growth light and throughout the experiment (Figure 1B). In addition, npq4 reached a still higher level of F′/Fm than pgr5 at the high light intensities (661, 1595 μmol photons m-2 s-1), during which the QA begun to return to its oxidized state (Figure 1B). The differential reduction pattern of pgr5 and npq4 recurred during the steep increase of actinic light intensity (Figure 2B).
Induction of NPQ (1–Fm′/Fm) was analyzed in order to clarify the relationship between thermal dissipation of excess excitation energy and redox state of ETC. NPQ was almost non-existent in the npq4 mutant, whereas pgr5 was capable of inducing a relatively high NPQ compared to npq4 especially in very high light (Figures 1C and 2C). In addition to the slower induction, both mutants showed an impaired relaxation of NPQ during the subsequent phase of darkness (Figure 2C).
Discussion
Limitation of electron flow to PSI upon increase in light intensity has been shown to be crucial for protection of PSI against photodamage (Munekage et al., 2002; Suorsa et al., 2012). The mechanisms involved in such a regulation of electron flow have, however, remained elusive. Here, we compared the putative effects of NPQ and the reduction state of the PQ pool on P700 oxidation in WT and in the pgr5 and npq4 mutants (Schematic model, Figure 3). It has been proposed that the deficiency of pgr5 in generation of ΔpH upon increase in light intensity is due to incomplete cycling of electrons from PSI acceptor side back to the PQ pool by a putative FQR (Munekage et al., 2002). This obviously has not been considered to be a problem because the simultaneously induced energy-dependent NPQ is believed to selectively downregulate PSII, thus leading to PSII limitation of electron transfer, and consequent oxidation of PSI. We tested this hypothesis and demonstrated (Figure 1) that despite the absence of NPQ, the npq4 mutant perfectly oxidizes P700, even more efficiently than WT. Importantly, P700 is oxidized (Figures 1A and 2A) despite the fact that the PQ pool is at the same time strongly reduced (Figures 1B and 2B). The concomitant reduction of the PQ pool and oxidation of P700 strongly suggest that the electron transfer is controlled by Cyt b6f. Moreover, the fact that oxidation of P700 is facilitated in the npq4 mutant indicates that in the absence of the PSBS protein, PSI has more excitation energy to oxidize P700 as compared to WT. This difference, however, decreases at extremely high light (Figure 1A), indicating that when the light intensity is strong enough, the capacity of PSBS-dependent quenching mechanism to limit excitation pressure becomes saturated.
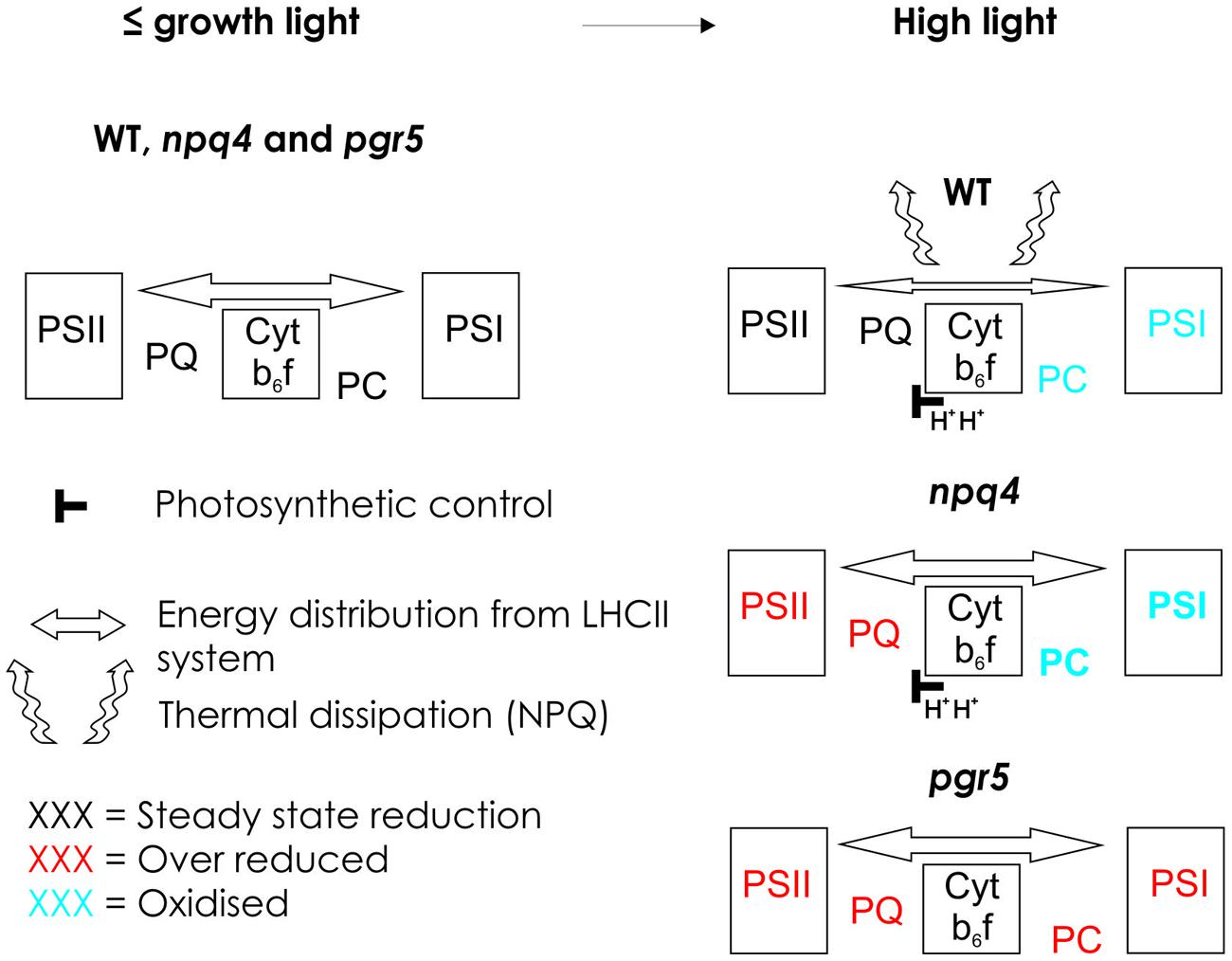
FIGURE 3. Schematic model presenting the redox state of electron transfer chain in WT and in npq4 and pgr5 mutants in growth light and in high light. In growth light or light intensities below the growth light, WT, npq4, and pgr5 can keep the intersystem electron transfer chain optimally oxidized. Increase in light intensity enhances ΔpH in WT and npq4 but not in pgr5. Increased ΔpH slows down electron flow via Cyt b6f in WT and npq4, but induces NPQ only in WT. In WT, NPQ prevents the over-reduction of Plastoquinone (PQ) pool and slows down the Cyt b6f leading to oxidation of plastocyanin (PC) and photosystem I (PSI). In the npq4 mutant, with low NPQ but normal photosynthetic control, high light leads to high reduction of PQ pool, and enhanced oxidation of PC and PSI. This indicates that in the absence of PSBS protein-dependent NPQ, the photochemical capacity of the both photosystems in improved. In the pgr5 mutant, that cannot raise the ΔpH upon increase in light intensity, the entire electron transfer chain becomes over-reduced. This results from the incapability to slow down the Cyt b6f rather than from the low level of NPQ.
The pgr5 mutant is severely deficient in induction of NPQ when the increase in light intensity is not extreme (Figure 1C). Nevertheless, when the increase in light intensity is strong enough, the pgr5 mutant can induce a reasonably high NPQ that in WT occurs concomitantly with oxidation of P700 (Figure 2A). Despite the induction of NPQ, the pgr5 mutant cannot oxidize P700 (Figures 1A and 2A), which further confirms that NPQ is not a mechanism to control the electron flow to PSI. Further support to this conclusion is provided by experiments (Tikkanen et al., 2010; Grieco et al., 2012) conducted with the stn7 mutant deficient in excitation energy transfer to PSI. Comparison of WT and stn7 with respect to the reduction state of the electron transfer chain, as affected by both the induction and relaxation of NPQ, revealed two distinct phenomena. First, the redox state of the PQ pool in WT remains relatively stable despite the induction or relaxation of NPQ. Second, in the stn7 plants, the relaxation of NPQ leads to reduction of the PQ pool (Tikkanen et al., 2010; Grieco et al., 2012). Taken together, it can be concluded that when the excitation energy distribution from the LHCII system to PSII and PSI is in balance, NPQ does not change the relative capacity of PSII and PSI electron transfer (Tikkanen et al., 2011). It is worth noting here that opposite to the independence between NPQ and oxidation of PSI, already a moderate photoinhibition of PSII leads to selective down-regulation of PSII and consequent oxidation of PSI (P700; Tikkanen et al., 2014).
Importantly, pgr5 is more efficient in oxidation of P700 in low light than in high light (Figure 1). This may suggest that in the absence of PGR5-provided ΔpH and resistance against proton extrusion from the lumen, the NDH-1-dependent cyclic is enhanced. Similar to bacterial and mitochondrial complex I (Efremov et al., 2010), a transfer of electron is coupled with translocation of four protons into thylakoid lumen via the NDH-1 complex (For a review: Battchikova et al., 2011; Kramer and Evans, 2011). This increases the amount of translocated protons in relation to transported electrons as compared to linear electron transfer and the FQR-CET. The additional ΔpH generated by NDH-1-CET may increase the resistance against LET via Cyt b6f, leading to enhanced oxidation of P700 in pgr5 in low light. Oxidation of P700 is, however, lost when the actinic light exceeds the intensity of growth light. This indicates that the NDH-1-dependent protonation of lumen is not capable of compensating the function of the PGR5 protein in high light. Indeed, in high light the PGR5 protein is essential in controlling the rate of the intersystem electron transfer.
In our opinion, based on the facts that NPQ plays no role in oxidation of P700 in vivo and the FQR-CET model is paradoxical in requiring simultaneous acceleration and deceleration of the electron transfer via Cyt b6f, it seems highly unlikely that the function of the PGR5 protein in PSI CET is to keep P700 oxidized. Therefore, we assume that there is a still uncharacterized PGR5-dependent mechanism that controls proton translocation across the thylakoid membrane and allows synchronized induction of NPQ together with Cyt b6f-dependent mechanism to control electron flow to PSI. A good candidate for such a mechanism is the regulation ATP synthase according to the redox state of electron transfer components between light reactions and carbon assimilation reactions (Kohzuma et al., 2013). It is known that PGR5 increases the resistance against proton translocation from thylakoid lumen to chloroplast stroma (Avenson et al., 2005). Interestingly, the PGR5-PGRL1 complex has redox active thiol groups being able to accept electrons from ferredoxin (Hertle et al., 2013). It is conceivable that the PGR5-PGRL1 complex senses the redox state of PSI electron acceptors and accordingly exerts feedback-regulation on photosynthetic light reactions, by tuning the resistance of proton translocation via ATP synthase by a mechanism that remains to be characterized.
Conflict of Interest Statement
The authors declare that the research was conducted in the absence of any commercial or financial relationships that could be construed as a potential conflict of interest.
Acknowledgment
Research was supported by the Academy of Finland (projects 271832, 273870, and 260094).
References
Allen, J. F., Bennett, J., Steinback, K. E., and Arntzen, C. J. (1981). Chloroplast protein phosphorylation couples plastoquinone redox state to distribution of excitation energy between photosystems. Nature 291, 25–29. doi: 10.1038/291025a0
Avenson, T. J., Cruz, J. A., Kanazawa, A., and Kramer, D. M. (2005). Regulating the proton budget of higher plant photosynthesis. Proc. Natl. Acad. Sci. U.S.A. 102, 9709–9713. doi: 10.1073/pnas.0503952102
Battchikova, N., Eisenhut, M., and Aro, E. M. (2011). Cyanobacterial NDH-1 complexes: novel insights and remaining puzzles. Biochim. Biophys. Acta 1807, 935–944. doi: 10.1016/j.bbabio.2010.10.017
Efremov, R. G., Baradaran, R., and Sazanov, L. A. (2010). The architecture of respiratory complex I. Nature 65, 441–445. doi: 10.1038/nature09066
DalCorso, G., Pesaresi, P., Masiero, S., Aseeva, E., Schünemann, D., Finazzi, G., et al. (2008). A complex containing PGRL1 and PGR5 is involved in the switch between linear and cyclic electron flow in Arabidopsis. Cell 132, 273–285. doi: 10.1016/j.cell.2007.12.028
Grieco, M., Suorsa, M., Jajoo, A., Tikkanen, M., and Aro, E. M. (2015). Light-harvesting II antenna trimers connect energetically the entire photosynthetic machinery - including both photosystems II and I. Biochim. Biophys. Acta 1847, 607–619. doi: 10.1016/j.bbabio.2015.03.004
Grieco, M., Tikkanen, M., Paakkarinen, V., Kangasjarvi, S., and Aro, E. M. (2012). Steady-state phosphorylation of light-harvesting complex II proteins preserves photosystem I under fluctuating white light. Plant Physiol. 160, 1896–1910. doi: 10.1104/pp.112.206466
Hertle, A. P., Blunder, T., Wunder, T., Pesaresi, P., Pribil, M., Armbruster, U., et al. (2013). PGRL1 is the elusive ferredoxin-plastoquinone reductase in photosynthetic cyclic electron flow. Mol. Cell. 49, 511–523. doi: 10.1016/j.molcel.2012.11.030
Joliot, P., and Johnson, G. N. (2011). Regulation of cyclic and linear electron flow in higher plants. Proc. Natl. Acad. Sci. U.S.A. 108, 13317–13322. doi: 10.1073/pnas.1110189108
Joliot, P., and Joliot, A. (2003). Excitation transfer between photosynthetic units: the 1964 experiment. Photosynth. Res. 76, 241–245. doi: 10.1023/A:1024908829819
Kanazawa, A., and Kramer, D. M. (2002). In vivo modulation of nonphotochemical exciton quenching (NPQ) by regulation of the chloroplast ATP synthase. Proc. Natl. Acad. Sci. U.S.A. 99, 12789–12794. doi: 10.1073/pnas.182427499
Klughammer, C., and Schreiber, U. (1994). An improved method, using saturating light-pulses, for the determination of photosystem I quantum yield via P700+-absorbance changes at 830nm. Planta 192, 261–268. doi: 10.1007/BF01089043
Klughammer, C., and Schreiber, U. (2008). Saturation pulse method for assessment of energy conversion in PS I. PAM Application Notes 1, 11–14.
Kohzuma, K., Dal Bosco, C., Meurer, J., and Kramer, D. M. (2013). Light- and metabolism-related regulation of the chloroplast ATP synthase have distinct mechanisms and functions. J. Biol. Chem. 288, 13156–13163. doi: 10.1074/jbc.M113.453225
Kramer, D. M., and Evans, J. R. (2011). The importance of energy balance in improving photosynthetic productivity. Plant Phys. 155, 170–178. doi: 10.1104/pp.110.166652
Kukuczka, B., Magneschi, L., Petroutsos, D., Steinbeck, J., Bald, T., Powikrowska, M., et al. (2014). Proton Gradient regulation5-like1-mediated cyclic electron flow is crucial for acclimation to anoxia and complementary to non-photochemical quenching in stress adaptation. Plant Physiol. 165, 1604–1617. doi: 10.1104/pp.114.240648
Lavergne, J., and Trissl, H. W. (1995). Theory of fluorescence induction in photosystem II: derivation of analytical expressions in a model including exciton-radical-pair equilibrium and restricted energy transfer between photosynthetic units. Biophys. J. 68, 2474–2492. doi: 10.1016/S0006-3495(95)80429-7
Li, X. P., Bjorkman, O., Shih, C., Grossman, A. R., Rosenquist, M., Jansson, S., and Niyogi, K. K. (2000). A pigment-binding protein essential for regulation of photosynthetic light harvesting. Nature 403, 391–395. doi: 10.1038/35000131
Mekala, N. R., Suorsa, M., Rantala, M., Aro, E. M., and Tikkanen, M. (2015). Plants actively avoid state-transitions upon changes in light intensity - role of light-harvesting complex II protein dephosphorylation in high light. Plant Physiol. 168, 721–734. doi: 10.1104/pp.15.00488
Munekage, Y. N., Genty, B., and Peltier, G. (2008). Effect of PGR5 impairment on photosynthesis and growth in Arabidopsis thaliana. Plant Cell Physiol. 49, 1688–1698. doi: 10.1093/pcp/pcn140
Munekage, Y., Hojo, M., Meurer, J., Endo, T., Tasaka, M., and Shikanai, T. (2002). PGR5 is involved in cyclic electron flow around photosystem I and is essential for photoprotection in Arabidopsis. Cell 110, 361–371. doi: 10.1016/S0092-8674(02)00867-X
Murata, N. (2009). The discovery of state transitions in photosynthesis 40 years ago. Photosynth. Res. 99, 155–160. doi: 10.1007/s11120-008-9389-8
Niyogi, K. K., and Truong, T. B. (2013). Evolution of flexible non-photochemical quenching mechanisms that regulate light harvesting in oxygenic photosynthesis. Curr. Opin. Plant Biol. 16, 307–314. doi: 10.1016/j.pbi.2013.03.011
Roach, T., and Krieger-Liszkay, A. (2012). The role of the PsbS protein in the protection of photosystems I and II against high light in Arabidopsis thaliana. Biochim. Biophys. Acta 1817, 2158–2165. doi: 10.1016/j.bbabio.2012.09.011
Stiehl, H. H., and Witt, H. T. (1969). Quantitative treatment of the function of plastoquinone in phostosynthesis. Z. Naturforsch. B 24, 1588–1598. doi: 10.1515/znb-1969-1219
Suorsa, M., Jarvi, S., Grieco, M., Nurmi, M., Pietrzykowska, M., Rantala, M., et al. (2012). Proton gradient regulation5 is essential for proper acclimation of Arabidopsis photosystem I to naturally and artificially fluctuating light conditions. Plant Cell 24, 2934–2948. doi: 10.1105/tpc.112.097162
Tikhonov, A. N. (2014). The cytochrome b6f complex at the crossroad of photosynthetic electron transport pathways. Plant Physiol. Biochem. 81, 163–183. doi: 10.1016/j.plaphy.2013.12.011
Tikkanen, M., and Aro, E. M. (2014). Integrative regulatory network of plant thylakoid energy transduction. Trends Plant Sci. 19, 10–17. doi: 10.1016/j.tplants.2013.09.003
Tikkanen, M., Grieco, M., and Aro, E. M. (2011). Novel insights into plant light-harvesting complex II phosphorylation and ‘state transitions’. Trends Plant Sci. 16, 126–131. doi: 10.1016/j.tplants.2010.11.006
Tikkanen, M., Grieco, M., Kangasjarvi, S., and Aro, E. M. (2010). Thylakoid protein phosphorylation in higher plant chloroplasts optimizes electron transfer under fluctuating light. Plant Physiol. 152, 723–735. doi: 10.1104/pp.109.150250
Tikkanen, M., Mekala, N. R., and Aro, E. M. (2014). Photosystem II photoinhibition-repair cycle protects Photosystem I from irreversible damage. Biochim. Biophys. Acta 1837, 210–215. doi: 10.1016/j.bbabio.2013.10.001
Keywords: regulation of photosynthetic electron transfer chain, cyclic electron transfer, thermal dissipation, NPQ, trans-thylakoid proton gradient, control of Cyt b6f, P700 oxidation
Citation: Tikkanen M, Rantala S and Aro E-M (2015) Electron flow from PSII to PSI under high light is controlled by PGR5 but not by PSBS. Front. Plant Sci. 6:521. doi: 10.3389/fpls.2015.00521
Received: 05 May 2015; Accepted: 26 June 2015;
Published: 08 July 2015.
Edited by:
Wei Huang, Kunming Institute of Botany – Chinese Academy of Sciences, ChinaReviewed by:
Michael Hippler, University of Muenster, GermanyJean Alric, Centre National de la Recherche Scientifique, France
Copyright © 2015 Tikkanen, Rantala and Aro. This is an open-access article distributed under the terms of the Creative Commons Attribution License (CC BY). The use, distribution or reproduction in other forums is permitted, provided the original author(s) or licensor are credited and that the original publication in this journal is cited, in accordance with accepted academic practice. No use, distribution or reproduction is permitted which does not comply with these terms.
*Correspondence: Eva-Mari Aro and Mikko Tikkanen, Molecular Plant Biology, Department of Biochemistry, University of Turku, Turku 20014, Finland, evaaro@utu.fi; misati@utu.fi