- Institut national de la santé et de la recherche médicale (Inserm) U955, Institut Mondor de Recherche Biomédicale (IMRB), Créteil, France
Cystic Fibrosis (CF) is a recessive genetic disease due to mutations of the Cystic Fibrosis Transmembrane Conductance Regulator (CFTR) gene encoding the CFTR chloride channel. The ion transport abnormalities related to CFTR mutation generate a dehydrated airway surface liquid (ASL) layer, which is responsible for an altered mucociliary clearance, favors infections and persistent inflammation that lead to progressive lung destruction and respiratory failure. The inflammatory response is normally followed by an active resolution phase to return to tissue homeostasis, which involves specialized pro-resolving mediators (SPMs). SPMs promote resolution of inflammation, clearance of microbes, tissue regeneration and reduce pain, but do not evoke unwanted immunosuppression. The airways of CF patients showed a decreased production of SPMs even in the absence of pathogens. SPMs levels in the airway correlated with CF patients’ lung function. The prognosis for CF has greatly improved but there remains a critical need for more effective treatments that prevent excessive inflammation, lung damage, and declining pulmonary function for all CF patients. This review aims to highlight the recent understanding of CF airway inflammation and the possible impact of SPMs on functions that are altered in CF airways.
Introduction
CF is a multisystemic disorder with the lung disease being the main cause of morbidity and mortality. It is commonly acknowledged that the reduced ASL height in CF, is responsible for an altered mucociliary clearance which favors infections and persistent inflammation, leading to progressive lung destruction and respiratory failure (Davis, 2006). However, CF airway inflammation is excessive and ineffective against pathogens, can occur very early in the development of the disease and in some cases without any sign of infection (Balough et al., 1995; Khan et al., 1995; Armstrong et al., 1997; Balázs and Mall, 2019).
While acute inflammation is protective, excessive swarming of polymorphonuclear neutrophils (PMN) amplifies inflammation with collateral tissue damage. Therefore, the inflammatory response is normally followed by an active resolution phase to return to tissue homeostasis in which among other mediators (cytokines, chemokines, immune cells), bioactive lipids might play a crucial role. Prostaglandins and leukotrienes stimulate the initiation and propagation phases of inflammation (Samuelsson et al., 1987; Funk, 2001) and the role of other lipid mediators called specialized pro-resolving mediators (SPMs) such as lipoxins, resolvins, protectins, and maresins has been demonstrated in the resolution phase (for recent review (Serhan and Levy, 2018)). SPMs promote resolution of inflammation, clearance of microbes, tissue regeneration and reduce pain, but do not evoke unwanted immunosuppression.
Abnormal SPM production or function have been related to widely occurring disorders, including CF airway disease. The airways of CF patients showed a decreased production of SPMs even in the absence of pathogens, which is consistent with other reports showing that inflammation in CF might not only be a consequence of chronic infection but could be related to intrinsic abnormalities of the inflammatory response (Muhlebach et al., 1999; Muhlebach and Noah, 2002; Karp et al., 2004; Ringholz et al., 2014; Bartlett et al., 2016; Isopi et al., 2020). The two SPMs, lipoxin A4 (LXA4) and resolvin D1 (RvD1), have the unique ability to restore the airway surface hydration, to damp the pro-inflammatory program and to fight infection in CF airways circumventing the most difficult aspects of CF pathophysiology (Karp et al., 2004; Grumbach et al., 2009; Verrière et al., 2012; Buchanan et al., 2013; Al-Alawi et al., 2014; Higgins et al., 2014; Higgins et al., 2016; Codagnone et al., 2017; Ringholz et al., 2018; Isopi et al., 2020). Furthermore, SPM levels in the airway correlate with CF patients lung function (Chiron et al., 2008; Eickmeier et al., 2017).
The prognosis for CF has greatly improved but it remains a severe and lethal disease. One promising therapy recently emerged, with small molecule correctors of mutated CFTR, which improve CFTR function and trafficking to the plasma-membrane [for recent review, see (Lopes-Pacheco, 2019)]. However, these therapies are gene mutation specific and their long-term impact on airway inflammation is still controversial (Jarosz-Griffiths et al., 2020; Volkova et al., 2020). To date there remains a critical need for more effective treatments that prevent excessive inflammation, lung damage, and declining pulmonary function for all CF patients.
This review highlights the current understanding of CF airway inflammation in the context of the recently described role of SPMs in chronic inflammation and the possible benefits of exogenous SPMs treatment in CF airways.
Acute Inflammation and Specialized Pro-Resolving Mediators
Specialized Pro-resolving Mediators (SPMs) are a new family of lipid mediators involved in the acute inflammatory process.
Excessive inflammation is considered a common component of a vast range of chronic diseases including vascular diseases, metabolic syndrome, cancer, and neurological and airway diseases. Chronic inflammation is a pathological condition characterized by a persistent inflammatory process which ultimately leads to tissue degradation and/or remodeling (Kotas and Medzhitov, 2015; Uluçkan and Wagner, 2017). Either innate or adaptive immune responses can be involved in chronic inflammation. Chronic inflammation stems from a failure to eliminate the excessive pathogen load and/or from a dysfunctional immune system including failure to resolve inflammation (Nathan, 2002; Nathan and Ding, 2010).
In contrast, acute inflammation is a protective immune response against microbial pathogens, tissue injury or other harmful stimuli which evolved to eliminate invading organisms and to enhance tissue repair (Germolec et al., 2018). The inflammatory process is thus normally self-limited without progressing to chronic inflammation and fibrosis, leading to a return to tissue homeostasis. Resolution was considered a passive process in which the mediators involved in generating the inflammatory response would just dilute and dissipate. With identification of the family of bioactive lipid mediators SPMs, Serhan’s team provided evidence that resolution of inflammation is an active, programmed response and not simply a process of diluting chemoattractant gradients (Samuelsson et al., 1987; Brady et al., 1995; Nathan, 2002; Serhan and Levy, 2018).
Therefore, inflammatory response is currently described as a physiological process divided into initiation and resolution phases, where lipid mediators, namely prostaglandins (PG), leukotrienes (LT), and SPMs play pivotal roles (Samuelsson et al., 1980; Funk, 2001). PGE2 and PGI2 induce vascular dilatation and permeability allowing PMN trafficking from blood circulation to enter the site of inflammation (Ley et al., 2007). LTB4 plays a role as a main chemoattractant driving PMNs to the inflammatory site to phagocytose pathogens. The resolution phase, orchestrated by SPMs in concert with other mediators, starts with cessation of PMN influx (Figures 1A, B) (Serhan et al., 2018). The families of SPMs identified to date are lipoxins (LX), resolvins (Rv), protectins (PD), and maresins (MaR). Their roles have been described in microbial defense, pain, organ protection and tissue regeneration, wound healing, cancer, reproduction, and neurobiology-cognition. SPMs play a crucial role in inhibiting the nuclear factor κB (NF-κB) (Chiang et al., 2006; Liao et al., 2012) and the synthesis of pro-inflammatory cytokines (Schwab et al., 2007; Krishnamoorthy et al., 2010; Chiang et al., 2012). SPMs also inhibit leukocyte chemotaxis and migration (Lee et al., 1989; Soyombo et al., 1994; Serhan et al., 1995; Papayianni et al., 1996; Sun et al., 2007; Isobe et al., 2012) (Figures 1A, B) as well as enhance innate microbial killing and clearance by stimulating leukocytes’ phagocytosis of bacteria (Chiang et al., 2012; Chiang et al., 2015; Codagnone et al., 2018). They enhance macrophages’ efferocytosis of apoptotic immune cells and cell debris (Godson et al., 2000; Schwab et al., 2007; Dalli and Serhan, 2012; Rogerio et al., 2012; Grabiec and Hussell, 2016) (Figure 1C). The repair of tissue damages generated by the inflammatory process also involves SPMs in promoting tissue regeneration (Dalli et al., 2014; Dalli et al., 2016) (Figure 1D). Beyond innate phagocyte responses to resolve acute inflammation, SPMs appear to play critical roles in regulating adaptive immunity (Barnig et al., 2013; Krishnamoorthy et al., 2015; Chiurchiù et al., 2016).
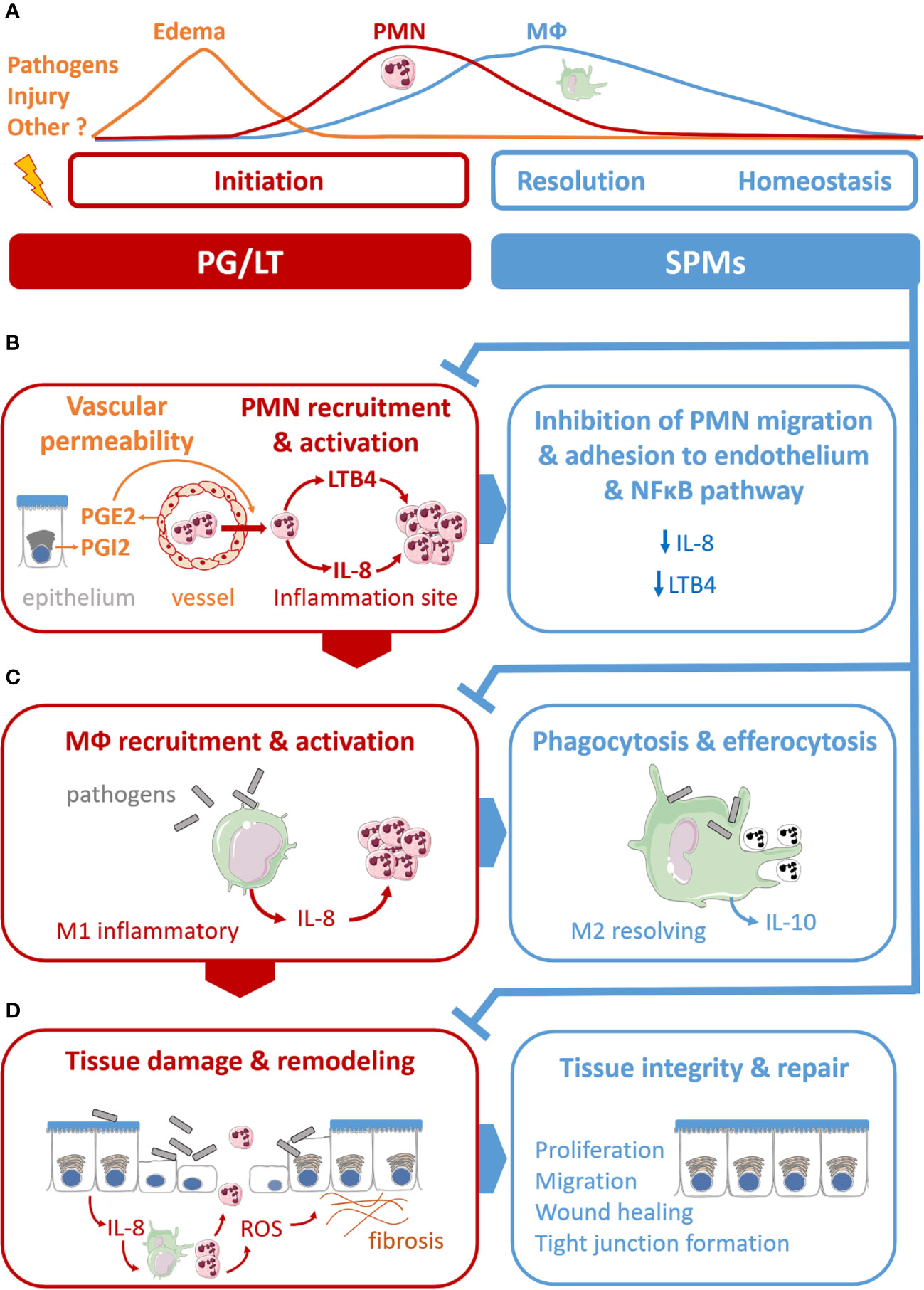
Figure 1 Illustration of specialized pro-resolving mediators’ (SPMs) multiple functions in the acute resolution of inflammation. (A) Acute inflammation evolved as a sequence of initiation and resolution phases that aim to drive back tissues toward homeostasis. The role of lipid mediators, prostaglandins (PG), leukotriene (LT), and SPMs can be related to each phase. (B) PGE2 and PGE2 and LTB4 play pivotal roles in the vascular response and leukocyte trafficking during the initiation phase. The SPMs, lipoxins, resolvins, protectins, and maresins produced at the inflammation, stimulate the cellular events that counter-regulate pro-inflammatory mediators and regulate polymorphonuclear (PMN) chemotaxis and migration, inhibiting LTB4 and pro-inflammatory cytokines synthesis (IL-8). (C) SPMs regulate monocyte and macrophage (MФ) response. SPMs drive differentiation from inflammatory MФ (M1) to resolving MФ (M2), inhibit pro-inflammatory cytokine production (IL-8) induced by bacteria, stimulate anti-inflammatory cytokines synthesis (IL-10), enhance MФ phagocytic function and efferocytosis of apoptotic PMN. (D) SPMs promote regeneration of tissue damaged by the inflammatory process.
SPMs exert their multiple actions through different cellular pathways involving diverse receptors (Chiang and Serhan, 2017). The first SPM receptor to be described is the N-formyl peptide receptor 2 (FPR2), a G protein-coupled receptor (GPCR) expressed in PMNs (Fiore et al., 1992) and other leukocytes (Chiang et al., 2006) as well as epithelial cells with high affinity for LXA4 (Fiore et al., 1994; Maddox et al., 1997; Chiang et al., 2003). Besides LXA4 and Aspirin-triggered 15-epi-LXA4 (AT-LXA4), FPR2 is also activated by Annexin A1 (ANXA1) (Perretti et al., 2002a; Bena et al., 2012). To date, five other specific SPM GPCRs have been identified in human and murine cells: GPR32, ChemR23, GPR18, GPR37, and GPR148. FPR2 and GPR32 mediate the RvD1, RvD3, and RvD5 immuno resolving actions (Krishnamoorthy et al., 2010; Hellmann et al., 2011; Recchiuti et al., 2011; Chiang et al., 2012; Gavins et al., 2012; Barnig et al., 2013; Buchanan et al., 2013; Dalli et al., 2013b; Lee and Surh, 2013; Recchiuti et al., 2014; Norling et al., 2016). Human GPR32 is mostly expressed in PMNs, monocytes, macrophages, and endothelial cells (Krishnamoorthy et al., 2010). Of note, RvD1 contributes to resolving inflammation by regulating a set of microRNAs specific to each receptor, FPR2 and GPR32 (Krishnamoorthy et al., 2010; Recchiuti et al., 2011). RvE1 and RvE2 exert potent and cell-specific actions on leukocytes via Chem32 (Tjonahen et al., 2006; Oh et al., 2012). GPR18 has been identified as the receptor for RvD2 (Chiang et al., 2015; Chiang et al., 2017), GPR37 for PD1 (Bang et al., 2018), and GPR148 for MaR1 (Chiang et al., 2019).
The cell signaling pathways activated by SPMs appear to be cell specific and do not depend solely on the receptor they bind to. In human epithelial cells, LXA4, and Mar1 stimulate a large increase in intracellular calcium promoting ASL height increase and epithelial repair in airway epithelia, and mucin secretion in conjunctival goblet cells (Lee et al., 1989; Nigam et al., 1990; Romano et al., 1996; Bonnans et al., 2003; Grumbach et al., 2009; Buchanan et al., 2013; Higgins et al., 2014; Hodges et al., 2017; Ringholz et al., 2018; Olsen et al., 2020). In contrast, in PMNs, LXA4 only induces a small increase in calcium and inhibits intracellular calcium mobilization induced by LTB4 in a context of PMN migration (Lee et al., 1989; Nigam et al., 1990; Romano et al., 1996; Grumbach et al., 2009; Buchanan et al., 2013; Higgins et al., 2014; Hodges et al., 2017; Ringholz et al., 2018). In human PMNs, stimulation of the LXA4 receptor inhibits phospholipase D (PLD) activity and superoxide anion generation (Levy et al., 1999; Levy and Serhan, 2000; Levy et al., 2005b), while in rat conjunctival goblet cells, LXA4 stimulates PLD activity in order to increase mucin secretion (Hodges et al., 2017). Binding to FPR2 can mediate both pro-inflammatory or anti-inflammatory responses depending on its ligand. In PMNs, while its ligand, serum amyloid A (SAA) induces a pro-inflammatory response with increased IL-8 secretion, LXA4 triggers the resolution characterized by a decreased IL-8 synthesis (He et al., 2003).
A distinct category of SPMs is the aspirin-triggered (AT) SPMs, which have a close but distinct structure. The AT-LXA4 and AT-LXB4 (15-epi-LXB4) differ only in the S and R chirality of their 15-hydroxyl residue. Theses endogenous SPMs' biosynthesis is induced by acetylsalicylic acid (ASA, or aspirin). To date, AT-LXs, AT-Rvs of the D series, and AT-PDs have been identified (Clària and Serhan, 1995; Chiang and Serhan, 2004; Serhan et al., 2004). They exert similar pro-resolving properties (Eickmeier et al., 2013; Wang et al., 2017; Liu et al., 2018; Hu et al., 2019).
Other mediators have been shown to play a role in the resolution of inflammation [for recent reviews, see (Perretti and D’Acquisto, 2009; Wallace et al., 2015; Schett and Neurath, 2018)]. ANXA1 and its derived peptides display a wide range of pro-resolving actions, among which inhibition of neutrophil recruitment, neutrophil/endothelium interaction and efferocytosis enhancement (Perretti et al., 2002b; Hayhoe et al., 2006; Scannell et al., 2007; D’Acquisto et al., 2008; Dalli et al., 2013a). In macrophages, the purine nucleoside adenosine promotes macrophage polarization toward resolution phenotype (M2), inhibits production of pro-inflammatory cytokines, and increases VEGF (Pinhal-Enfield et al., 2003; Csóka et al., 2012). Gaseous mediators such as carbon monoxide (CO), hydrogen sulfide (H2S), and nitric oxide (NO) also display anti-inflammatory and pro-resolving properties. Among them, NO possesses pro-apoptotic qualities, H2S reduces leukocyte-endothelium interaction through activation of KATP channels, CO inhibits NF-κB activation and proinflammatory cytokines secretion in colonic epithelial cells. However, NO and adenosine, can also display pro-inflammatory effects depending on the concentration, localization, receptors involved, and timing in the inflammatory response [reviewed in (Brüne, 2005; Vass and Horvath, 2008)]. In addition, these mediators share common anti-inflammatory pathways with SPM signaling pathways. The ANXA1’s anti-inflammatory actions rely on its ligation to the ALX/FPR2 receptor (Perretti et al., 2002b). Adenosine mediates Cl- secretion in epithelial cells through intracellular calcium signaling in CF airway epithelium (Chao et al., 1994). The pro-resolution neuromodulator netrin-1 contributes to SPM production in mice peritoneal inflammation (Mirakaj et al., 2014). CO stimulates SPM biosynthesis through the regulation of 15LO1 in vivo in mice and baboons as well as in vitro in human cells (Chiang et al., 2013; Dalli et al., 2015). Physiologic hypoxia also stimulates SPMs synthesis by M2 macrophages-neutrophils interaction (Norris et al., 2019).
In humans, SPMs which are rapidly metabolized and degraded are found at pico to nanogram levels in blood, breastmilk, the airways (breath condensate and sputum), urine, and tears (Gangemi et al., 2003; Levy et al., 2007; Psychogios et al., 2011; Mas et al., 2012; Weiss et al., 2013; Colas et al., 2014; Ringholz et al., 2014; Sasaki et al., 2015; Barden et al., 2016; Dalli et al., 2017; Eickmeier et al., 2017; English et al., 2017). The short half-life of SPMs, whether they are endogenous or synthetic, led to the manufacturing of the first analogs of LXA4 by Serhan and his collaborators have made it easier to study their biological effects. These analogs differ structurally to prevent their metabolization and thus have the advantage of being more stable (Serhan et al., 1995). Other generations of synthetic stable analogs have since been developed and have shown similar pro-resolving effects to native SPMs, such as LXA4 analogs (Maddox et al., 1997; Mitchell et al., 2002), RvD1 analog (Orr et al., 2015), and RvE1 analog (Arita et al., 2006).
SPMs levels are significantly increased in inflammatory exudates in rheumatoid arthritis, skin blisters, bronchoalveolar lavage (BAL) fluids from patients with airway diseases (Lee et al., 1990; Karp et al., 2004; Planagumà et al., 2008; Morris et al., 2009; Ringholz et al., 2014; Norling et al., 2016; Motwani et al., 2018a; Motwani et al., 2018b). However, SPMs levels are shown to be decreased and unbalanced compared to pro-inflammatory eicosanoids in patients with chronic conditions including airway diseases. Lower LXA4 and LXB4 levels in sputum, BAL, and blood correlate with a more severe asthma phenotype. LXA4 levels have been found to be reduced in the sputum of Chronic Obstructive Pulmonary Disease (COPD) patients during exacerbation (Levy et al., 2005a; Vachier et al., 2005; Levy et al., 2007, 1; Schwab et al., 2007; Planagumà et al., 2008; Balode et al., 2012; Liao et al., 2012; Karra et al., 2015; Codagnone et al., 2018; Serhan et al., 2018). Altogether, SPMs, which are conserved structures, are now strong candidates for the role of main actors of the resolution phase of acute inflammation. Reports of decreased SPM biosynthesis or function in inflammatory chronic diseases suggest the involvement of SPMs in their pathogenesis. From these observations, additional fundamental studies aim to shed more light on the precise role of SPMs in these conditions. The next chapter will tackle the known alterations in lipid metabolism and SPMs biosynthesis in CF.
Abnormal Lipid Metabolism and SPMS Biosynthesis in CF
Along with ANXA1 and NO, an abnormal SPMs biosynthesis was reported in CF (Karp et al., 2004; Dalli et al., 2010; Ringholz et al., 2014; Güney et al., 2019).
SPMs biosynthesis results from the interaction of enzymes called lipoxygenases (LOX) and cyclooxygenases (COX) to metabolize essential n-3 (omega 3) and n-6 (omega 6) polyunsaturated fatty acids (PUFA). The main PUFA substrates are arachidonic acid (AA, n-6), eicosapentaenoic acid (EPA, n-3), and docosahexaenoic acid (DHA, n-3) (Serhan et al., 1984; Samuelsson et al., 1987). Different cell types that differentially express LOX and COX enzymes cooperate by exchanging intermediates to produce the final active metabolites (Papayianni et al., 1996; Folco and Murphy, 2006; Sala et al., 2010). While free AA gives rise to the lipoxin family of SPMs, it is also the precursor of pro-inflammatory mediators PG and LT depending on which metabolic enzyme is involved (Samuelsson et al., 1987; Folco and Murphy, 2006). The DHA and EPA metabolites resolvins, protectins, and maresins exert mainly resolving actions (Figure 2).
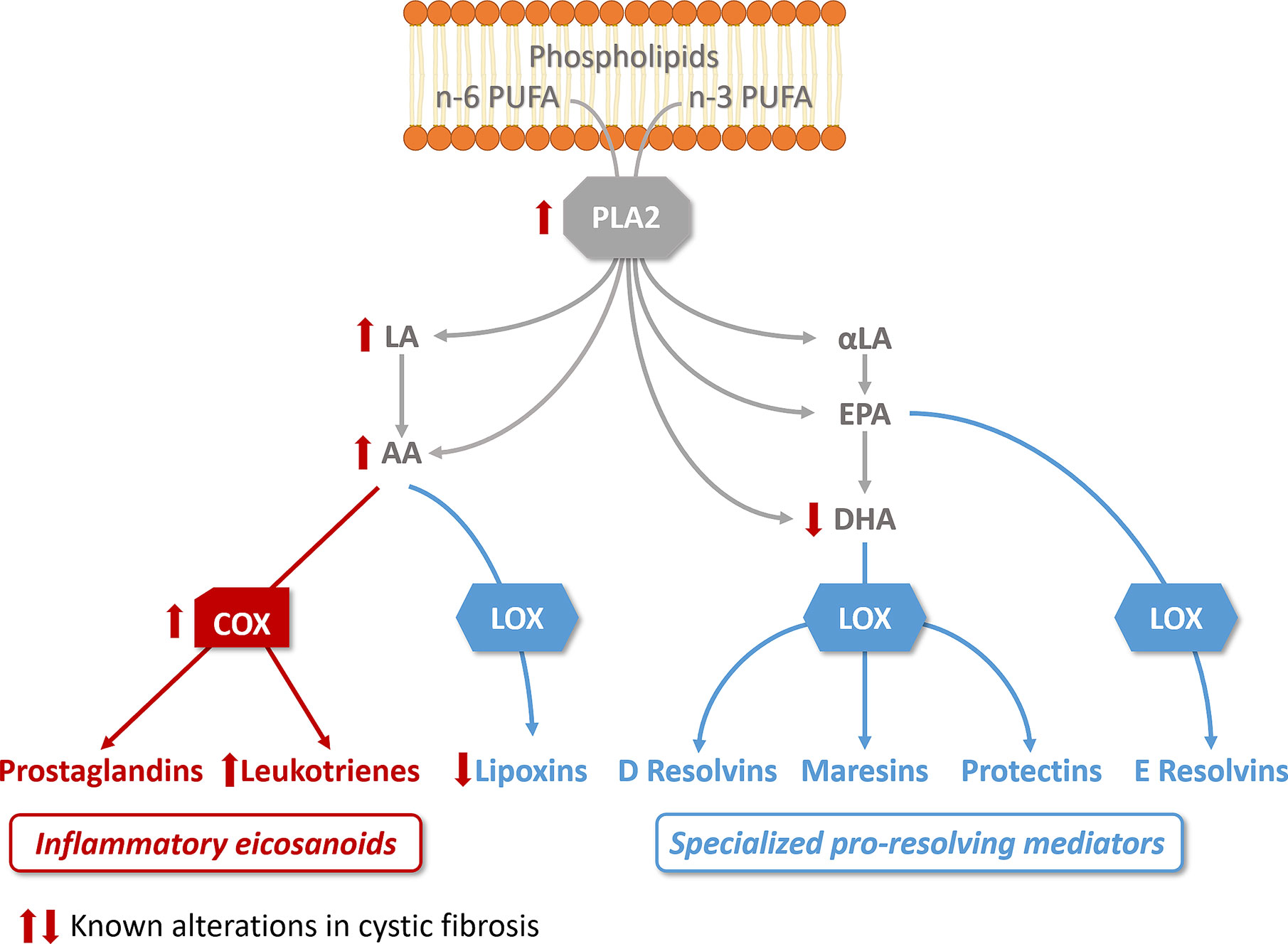
Figure 2 Altered lipid metabolism in cystic fibrosis. The activity of phospholipase A2 (PLA2), which releases essential fatty acid (n-6 and n-3 polyunsaturated fatty acids, PUFA) from phospholipids, is increased in CF bronchial epithelial cells. Free arachidonic acid (AA) which can be released from phospholipids or metabolized from n-6 linoleic acid (LA), is the precursor of prostaglandins and leukotrienes via cyclooxygenase activity (COX), which is increased in CF. AA is also a substrate for lipoxygenases (LOX) to produce lipoxins, found reduced in CF. The n-3 PUFA, α-linoleic acid (α-LA) is metabolized to ecosapentanoic acid (EPA) and docosahexaenoic acid (DHA), found decreased in CF. EPA and DHA are the precursors of resolvins, protectins, and maresins. The pathways involved in the biosynthesis of pro-inflammatory eicosanoids are illustrated in red, the pathways involved in the biosynthesis of specialized pro-resolving mediators in blue.
Acute inflammation is the result of a temporal eicosanoids class switching where COX-derived prostaglandins precede biosynthesis of lipoxins. This eicosanoids class switching, characterized by an increased biosynthesis of SPMs and a decrease of eicosanoids involved in the initiation of inflammation, results from the coordinated action of enzymes such as the different isoforms of lipoxygenases (15LO, 5LO, and 12LO), of COX (COX1 and COX2), as well as the leukotriene A4 hydrolase (LT4H) (Figure 3). These enzymes are selectively expressed in the cell types involved in the inflammatory process. The 15LO expressed in airway epithelial cells and macrophages and the 12LO in platelets play a central role in the class-switching from the pro-inflammatory lipid mediator LTB4 toward SPMs. Isolated PMNs exposed to PGE2 (to mimic exudates) switched eicosanoid biosynthesis from predominantly LTB4 and 5LO-initiated pathways to LXA4, which is a 15LO product that stops PMN infiltration (Levy et al., 2001). Indeed, the activity of 15LO favors LXA4 synthesis at the expense of LTB4 synthesis that involves LTA4H activity (Haeggström and Funk, 2011) (Figure 3). The cellular location of 5LO also plays a crucial role in the LTB4/SPM ratio. Inhibition of the CaM kinases by RvD1 favors the extra-nuclear location of 5LO, and the LXA4 biosynthesis at the expense of LTB4 (Fredman et al., 2014). Finally, while COX1 and COX2 metabolize AA into the inflammatory eicosanoids PGE2 and PGI2, acetylsalicylic acid (ASA) inhibits COX1 and modifies COX2 by acetylation (ASA-COX), leading to a shift from production of the precursor of PG, to 15-R-HpETE which is converted by the 5LO to AT-LXA4 (15-epi-LXA4) (Birnbaum et al., 2007) (Figure 3).
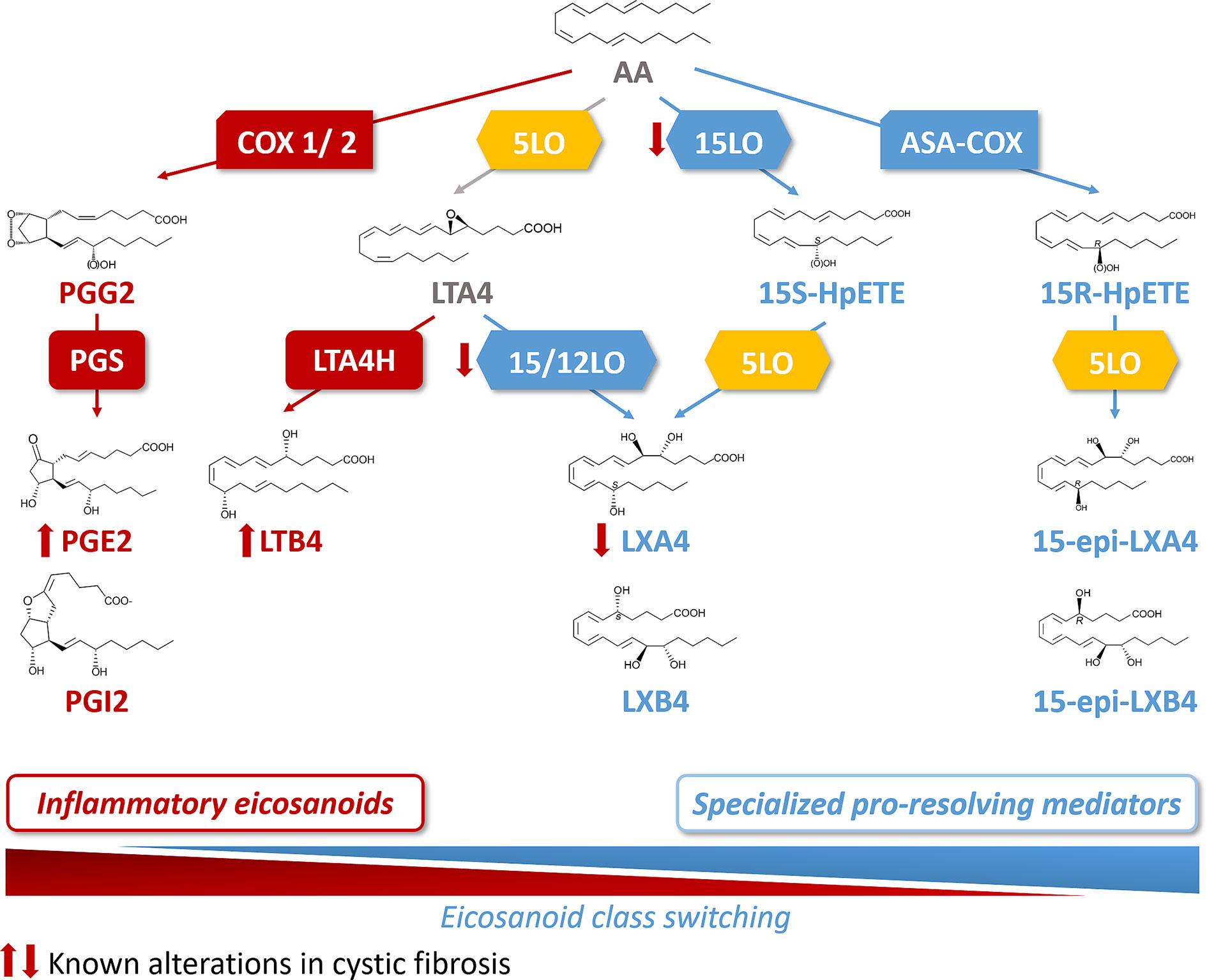
Figure 3 Arachidonic acid (AA) metabolome and abnormal class switching in CF. Arachidonic acid (AA) is metabolized by cyclooxygenase 1 and 2 (COX1/2) and prostaglandin synthase (PGS) into the inflammatory eicosanoids, prostaglandins (PGE2 and PGI2). AA is also a substrate for 5LO to produce leukotriene A4 (LTA4). The 15 lipoxygenase (15LO) activity drives then the SPM, lipoxin A4 (LXA4) synthesis (from LTA4) at the expense of the production of the inflammatory lipid, leukotriene B4 (LTB4) that involves leukotriene A4 hydrolase (LTA4H) activity. The sequential activities of 15LO and 5LO drive the biosynthesis of LXA4, with an intermediate product, 15S hydroperoxyeicosatetraenoic (15S-HpETE). Acetylsalicylic acid inhibited COX (ASA-COX) leads to the production of 15R hydroperoxyeicosatetraenoic acid (15-R-HpETE) which is converted by the 5LO to the SPM, 15-epi-LXA4. In CF, the abnormal class switching from inflammatory biosynthetic pathway to the pro-resolving pathway results into an increased LTB4 and decreased LXA4 biosynthesis in the airway which are responsible for the sustained inflammation.
Before the gene defect responsible for CF was identified, it was suggested that fatty acid metabolism abnormalities were responsible for the clinical symptoms of the CF disease and this has been confirmed in numerous further studies (Kuo et al., 1962) [for recent review, (Wheelock and Strandvik, 2020)]. Lower levels of palmitic, stearic, and linoleic acid (LA), were reported in plasma of CF patients compared to healthy controls (Hubbard et al., 1977; Gibson et al., 1986; Thompson, 1989; Roulet et al., 1997; Strandvik et al., 2001; Al-Turkmani et al., 2007). Initially thought as the result of malabsorption by CF patients (Hubbard et al., 1977), an abnormal lipid metabolism related to CFTR dysfunction was further demonstrated. An imbalance of PUFAs with increased release of AA and decreased levels of DHA in CF was established (Underwood et al., 1972; Bhura-Bandali et al., 2000; Freedman et al., 2004) (Figure 2). However, DHA supplementation in CF patients, in order to correct the imbalance between AA and DHA gave a controversial outcome (Lloyd-Still et al., 2006; Coste et al., 2007; Oliver and Watson, 2016; Teopompi et al., 2019). The direct link between the altered fatty acid levels and CFTR dysfunction is unclear. The activity of phospholipase A2 (PLA2), which releases AA from the membrane phospholipids and can interact with CFTR, is increased in human CF bronchial epithelial cells and in CFTR-knockout mice (Miele et al., 1997; Six and Dennis, 2000; Ghosh et al., 2006; Borot et al., 2009; Dif et al., 2010). Free AA is led down the PG metabolic pathway by the COX enzymes, which are upregulated in sinonasal mucosa of CF patients (Roca-Ferrer et al., 2006; Owens et al., 2008) (Figure 2). Consistent with this, polymorphisms downregulating COX1 and COX2 expression in CF patients are associated with a better clinical outcome (Czerska et al., 2010). The first studies focused on LXA4 levels and LXA4 to PMN ratio measured in BAL fluids of CF subjects compared to non-CF subjects and suggested a relative decrease of LXA4 to PMN concentrations (Karp et al., 2004). In contrast, Starosta et al. observed no differences in the absolute LXA4 BAL fluids concentrations between CF patients and controls with a similar degree of neutrophilic airway inflammation. Concentrations were also similar in CF patients with mild versus more severe airway inflammation (Starosta et al., 2006). Another study showed that CFTR defects dampen LXA4 biosynthesis during platelets-PMN interaction (Mattoscio et al., 2010). The ratio of SPMs to the pro-inflammatory eicosanoid LTB4 has also been evaluated by Ringholz et al. in BAL fluid samples from children with CF. An imbalance between resolving (LXA4) and pro-inflammatory eicosanoids (LTB4) was found, suggesting a defect in eicosanoid class switching (Ringholz et al., 2014). Furthermore, a reduced expression of the 12/15LO in the airway of a CF mice model as well as a reduced 15LO2 isoform level in the BAL fluid of patients with F508del mutation (Karp et al., 2004; Ringholz et al., 2014). Similarly, 15LO2 expression has been shown to be decreased by 50% in the nasal polyps of CF patients compared to non-CF (Jeanson et al., 2014) (Figure 3). A defective activity of 12LO, another lipoxygenase expressed in platelets, has been shown when inhibiting CFTR (Mattoscio et al., 2010). Although the cellular mechanism by which CFTR could affect LOX levels and/or activities remains unclear, these data are consistent with an abnormal SPMs biosynthesis in CF.
Taken together, the altered lipid metabolism that could result in an imbalance of eicosanoids with higher pro-inflammatory mediators as compared to SPMs would be coherent with the observations of the sustained and inefficient CF airway inflammation as detailed below.
Inflammation in CF Airway
CF is a recessive monogenic disease due to mutations in the Cystic Fibrosis Transmembrane conductance Regulator (CFTR) gene (Kerem et al., 1989; Riordan et al., 1989; Rommens et al., 1989). Over 2,000 mutations of CFTR are known, although around two thirds of patients have at least one copy of the F508del mutation (Cystic Fibrosis Mutation Database; Bobadilla et al., 2002). While CFTR dysfunction may affect many organs, the inflammatory airway disease leading to progressive lung damage is the main cause of morbidity and mortality of CF patients (Davis, 2006). Multiple pathways involved in CF airway inflammation have been described.
Ion Transport and Airway Surface Liquid Abnormalities
The dehydrated airway surface liquid (ASL) resulting from ion transport abnormalities is a critical feature of the CF airway pathogenesis (Boucher, 2007). The main CFTR function is to transport chloride from the cytosol to the lumen (Anderson et al., 1991; Linsdell, 2017). CFTR also regulates other epithelial functions (Stutts et al., 1995; Greger et al., 1996), including the activity of the sodium channel (ENaC) located in the same membrane (Hanukoglu and Hanukoglu, 2016). Although several reports suggested no change in ENaC activity associated with CFTR dysfunction (Chen et al., 2010; Itani et al., 2011; Collawn et al., 2012; Fisher et al., 2013; Sun et al., 2014; Tuggle et al., 2014), other functional studies have brought evidence supporting CFTR role in inhibiting sodium absorption by ENaC (Kunzelmann et al., 1995; Ismailov et al., 1996; Kunzelmann et al., 2000; König et al., 2001; Kunzelmann, 2003; Gentzsch et al., 2010; Lazrak et al., 2011). At the expression level, while the α- and β-ENaC subunit amount was reported to be increased in CF nasal epithelium the γ-ENaC was decreased (Bangel et al., 2008). Consistent with a role for ENaC in CF, similar features of human CF lung disease have been reproduced when ENaC is overexpressed in mice (Mall et al., 2004).
Both transepithelial chloride secretion involving chloride channels such as CFTR and the transepithelial sodium absorption via ENaC, finely regulate cellular and paracellular water movements generating the ASL layer (Tarran et al., 2001; Tarran, 2004). The ASL constitutes the first line of innate defense in controlling the mucociliary clearance process, trapping and neutralizing inhaled foreign particles in the mucus layer that are then removed from the airways by the ciliated cells (Knowles and Boucher, 2002; Button et al., 2012; Shei et al., 2018). In CF, ion transport abnormalities lead to a reduced ASL height (Boucher, 2007) and result in an impaired ciliary beating, favors mucus plugging in the airways, which constitutes a viscous and nutritive medium for pathogens proliferation in the respiratory tract, especially bacteria.
The content of the ASL layer of CF patients is also affected by dysregulation of bicarbonate transport. Firstly observed in pancreas, decreased bicarbonate secretion has been related to abnormal chloride secretion (Marino et al., 1991). CFTR has been shown to regulate bicarbonate secretion; either directly by being permeant (Poulsen et al., 1994; Illek et al., 1997; Kim et al., 2014), or by interacting with other transporters, such as the chloride/bicarbonate exchangers, SLC26A4 also named pendrin, and/or the ATP12A, a non-gastric form of H+/K+-ATPase expressed at the apical side of airway epithelia (Garnett et al., 2011; Scudieri et al., 2018). Indeed, H+-K+-ATPase and CFTR-dependent bicarbonate secretion regulate extracellular pH (Coakley et al., 2003). The lack of bicarbonate secretion due to altered CFTR activity or ATP12A increased expression in CF human airways secondary to inflammation and infection are consistent with a more acidic ASL pH in CF (Song et al., 2006; Cho et al., 2011; Scudieri et al., 2018). Furthermore, ASL acidification in CF bronchial epithelial cells can be driven by hyperglycemia and P. aeruginosa-related lactate secretion which monitors H+ secretion (Garnett et al., 2016). Although ASL pH is difficult to measure and ASL pH decrease in CF is still controverted (McShane et al., 2003; Schultz et al., 2017), more acidic ASL could explain some aspect of the pathogenesis of the disease. Indeed, an acidified environment is beneficial for bacteria survival. In vivo studies on CF newborn pigs and in vitro data obtained on human airway epithelial cells have demonstrated that bacterial killing is impaired in CF due to more acidic ASL pH (Pezzulo et al., 2012; Simonin et al., 2019).
Chronic Airway Infection and Inflammation
In CF, the lung function decline correlates with the chronic colonization by pathogens, including the bacteria Pseudomonas aeruginosa (Nixon et al., 2001; Emerson et al., 2002; Konstan et al., 2007) which are potent triggers of PMN response. However, in CF airways challenged by bacteria or viruses, inflammation appears disproportionate to the degree of infection, with a high PMN infiltration and release of pro-inflammatory molecules, such as TNF-α, IL-8, IL-6, IL-1β, proteases, oxidants, PG, and LT, together leading to bronchiectasis and fibrosis (Konstan et al., 1993; Balough et al., 1995; Bonfield et al., 1995; Khan et al., 1995; Noah et al., 1997; Muhlebach et al., 1999; Tirouvanziam et al., 2000; Muhlebach and Noah, 2002). As the first line of defense against microbial agents, macrophages can induce inflammation by stimulating the immune system but they also contribute to ending the inflammation process and to the return to tissue homeostasis, by clearing microbes and dead PMNs for instance. These polar roles define two macrophage subtypes with distinct gene signatures, a pro-inflammatory subtype (M1) and a pro-resolving one (M2) (Mills et al., 2000). M1 cells produce high levels of the pro-inflammatory TNFα, IL-1β, IL-6, and IL-12, while M2 cells secrete high levels of immune-resolving IL-10 and TGFβ1 compared with M1 cells (Bystrom et al., 2008). It has been suggested that altered macrophage function contributes to the sustained inflammation in CF airways (Bruscia and Bonfield, 2016). CF macrophages are hyper-responsive, producing a high amount of pro-inflammatory cytokines when exposed to bacterial stimuli (Bruscia et al., 2009; Bruscia et al., 2011) but showed a defective ability to clear bacteria (Di et al., 2006; Deriy et al., 2009; Porto et al., 2011). There have been reports of CF macrophages failing to polarize into anti-inflammatory M2 and M1 macrophages displaying a hypermetabolic state (Tarique et al., 2017; Lara-Reyna et al., 2019).
Early reports indicated signs of inflammation in the airways of young CF patients even in the absence of infection and questioned the nature of the relationship between infection and inflammation in the CF airways (Balough et al., 1995; Khan et al., 1995; Armstrong et al., 1997). Thanks to systematic newborn screening and methodical, precise phenotyping from longitudinal studies, the early lung disease onset and progression in CF has been better characterized. As early as infancy, CF patients’ lower airways show signs of mucus plugging and altered lung structure (Martínez et al., 2005; Stick et al., 2009). Furthermore, BAL fluid samples collected from infants bear evidence of active inflammation with high PMN counts and neutrophil elastase and pro-inflammatory cytokine levels, even before patients encounter their first infection by the conventional CF pathogens (Pillarisetti et al., 2011; Montgomery et al., 2017; Ranganathan et al., 2017; Balázs and Mall, 2019). The presence of sterile inflammation has also been observed in animal models, notably in CFTR-knockout ferrets that show signs of neutrophil-mediated inflammation despite being infection-free (Keiser et al., 2015; Rosen et al., 2018).
Despite the identification of the CFTR gene and the growing mutation database, establishing a correlation between CF genotype and clinical phenotype remains difficult, considering the vastly different disease courses between patients, even between twin siblings sharing the same CFTR mutations (Kerem et al., 1990; Mekus et al., 2000). Genome-wide association studies with large patient cohorts have identified genetic variants of specific loci associated with disease severity, called CF modifier genes, that contribute to phenotypic variability [see recent reviews (Lim et al., 2018; Shanthikumar et al., 2019)]. Among the proteins encoded by the CF modifier genes, some have direct protein-protein interaction with CFTR and/or are involved in immune response (Tugores et al., 2001; Wright et al., 2011; Stanke et al., 2014; Corvol et al., 2015). The trachea of CF and non-CF newborn pigs when challenged by an inflammatory stimulus revealed differential transcriptomes (Bartlett et al., 2016). Therefore, beyond ion transport abnormalities, intrinsic immune abnormalities related to CFTR dysfunction could be involved in the pathogenesis of the CF airway disease.
Dysregulated Calcium Homeostasis
Calcium is a major intracellular second messenger playing a key role in immune functions, among which cytokines secretion and PMN recruitment (Berridge et al., 2003; Immler et al., 2018). Calcium homeostasis has long been known to be dysregulated in CF (Katz et al., 1984; Cabrini and De Togni, 1985).
Initial studies supported the hypothesis of an acquired response from the airway epithelial cells to exogenous pathogens such as P. aeruginosa leading to calcium mobilization and hyperinflammation (Denning et al., 1998; Hybiske et al., 2007). Further studies demonstrated that the endoplasmic reticulum (ER), a major intracellular calcium storage compartment, undergoes stress and expansion in CF airway epithelial cells as the result of chronic infection and inflammation (Ribeiro et al., 2005a; Ribeiro et al., 2005b; Ribeiro, 2006). Other studies have suggested that ER stress, NF-κB activation, and IL-8 related expressions are closely linked to CFTR channel mistrafficking and its retention in the ER of different CF models (Weber et al., 2001; Knorre et al., 2002; Antigny et al., 2008a). The ER stress normally results in unfolded protein response (UPR) mediated by proteins located in the ER membrane. However, atypical UPR activation fails to resolve the ER stress in CF and sensitizes the innate immune system to respond more vigorously to microbial challenge (Ribeiro et al., 2005b; Ribeiro, 2006; Kerbiriou et al., 2007; Lin et al., 2008; Blohmke et al., 2012). Finally, calcium transporters are also reported to be altered in CF. In the ER, the retention of the F508del CFTR protein leads to impaired activity of SERCA pump and IP3 receptor, altering calcium exchange between the ER and with the cytosol (Antigny et al., 2008a; Antigny et al., 2008b; Philippe et al., 2015). Calcium influx and efflux at the plasma-membrane have been shown to be impaired by dysfunctional coupling of mutated CFTR with transient receptor potential canonical channels 6 (TRPC6), altered plasma-membrane calcium pump and upregulated complex Orai1/STIM1 formation that increases IL-8 secretion (Antigny et al., 2011; Balghi et al., 2011; Philippe et al., 2015).
Mucus
Mucus was for long observed to be more viscous and thick, in CF airways in in vitro and in situ studies (Derichs et al., 2011). In addition to altered ion and fluid transport that result in a dehydrated mucous layer, the CF airways are characterized by goblet cell and glandular hyperplasia and subsequent overproduction of the two secreted mucins MUC5B and MUC5AC, which has been reported in both CF patients and CF model systems (Henderson et al., 2014; Esther et al., 2019). Furthermore, structural mucus abnormalities have been described in CF. Analysis of airway mucins from newborn CF pigs’ freshly excised airways revealed that MUC5B strands remained attached to submucosal glands and MUC5AC, secreted by goblet cells, is more present and forms sheets that cover MUC5B strands (Ostedgaard et al., 2017). The early work of Inglis, Ballard et al. on excised porcine distal bronchi enlightened the role of chloride and water transport on mucus rheological properties (Inglis et al., 1997; Inglis et al., 1998; Inglis et al., 1999; Ballard et al., 2002). In addition, Perez-Vilar suggested that lower pH would favor interchain disulfide bonds and the ASL volume depletion may increase MUC5B and MUC5AC concentrations and favor interactions (Perez-Vilar and Boucher, 2004). Other studies suggested that mucus swelling and hydration is driven by the Donnan effects (unbalanced repartition of charged particles over a porous barrier) and that HCO3- plays a key role in the swelling of the mucins favoring a decrease content of calcium associated mucins (Quinton, 2008; Chen et al., 2010). From the new-born CF piglets and human bronchial epithelium studies, the impaired mucocilliary clearance and rheological properties of mucus appeared dependent on both chloride and pH (Hoegger et al., 2014; Gorrieri et al., 2016; Hill et al., 2018; Hughes et al., 2019). Finally, a pilot study realized on 12 CF volunteers supported that nebulized NaHCO3 was safe and well tolerated and it permit to increase ASL pH and change the rheology of the sputum (Gomez et al., 2020).
Oxidative Stress
Oxidative stress which arises from the loss of the oxidant/antioxidant balance shifted toward oxidant production is described in many airway diseases including CF and triggers lung injuries (Brown et al., 1996) and pro-inflammatory mechanisms (Brown et al., 1996; MacNee, 2001; Boncoeur et al., 2008; Chen et al., 2008; Bartling and Drumm, 2009; Park et al., 2009; Kelly-Aubert et al., 2011; Pongnimitprasert et al., 2012). Increased levels of reactive oxygen species (ROS), such as superoxide anion, and involvement of its main producer, the NADPH oxidase (NOX) enzyme, have been demonstrated in CF mice models and human cell lines (Esposito et al., 1999; Velsor et al., 2006; Pongnimitprasert et al., 2012). In CF airway epithelial cells, the morphology and functions of mitochondria, the main cellular component involved in ROS production (Favia et al., 2019) are altered (Feigal et al., 1982; Von Ruecker et al., 1984; Antigny et al., 2009) and restored by correction of F508del CFTR mutation (Valdivieso et al., 2007; Taminelli et al., 2008; Valdivieso et al., 2012; Atlante et al., 2016). Furthermore, P. aeruginosa infection and inflammation-related production of ROS by PMNs and macrophages exacerbate alterations of mitochondrial functions, DNA, and morphology in normal lung epithelial cells. All of these mitochondrial alterations contribute to the establishment of oxidative stress in the airways (Maurice et al., 2019; Causer et al., 2020). Studies from CF mice model, human cell lines, or BAL fluids have shown a reduced uptake, synthesis or activity of antioxidant defenses, such as superoxide dismutase, peroxidases, catalases, and reduced glutathione (GSH) which further enhances oxidative stress (Farrell et al., 1977; Roum et al., 1993; Velsor et al., 2006; Schwarzer et al., 2007; Rottner et al., 2011). In addition to CFTR permeability for GSH, polymorphisms in regulatory genes of the metabolic pathway of GSH were associated with different phenotypes of CF and its severity (Linsdell and Hanrahan, 1998; Hudson, 2001; Jungas et al., 2002; Marson et al., 2013; Marson et al., 2014). Finally, low levels of GSH in BAL could also result from its oxidation by pro-inflammatory products, such as hypochlorous acid, coming from infection-activated PMNs (Kettle et al., 2014).
Abnormal Epithelial Repair
In CF, impaired mucociliary clearance, long-term infection, ineffective inflammation, increased ROS secretion and PMN products contribute to epithelial injury, amplifying airway inflammation (Chmiel and Davis, 2003). Altered CF airway epithelia repair has been more directly related to CFTR dysfunction and expression (Schiller et al., 2010; Itokazu et al., 2014). While CFTR pharmacologic inhibition, repression or mutation affect epithelial cell proliferation and migration and wound healing, CFTR rescue with transfection or CFTR modulators (correctors, potentiators) improves epithelial repair in airway epithelial cultures from patients with the most common mutations (Trinh et al., 2012; Dong et al., 2015; Adam et al., 2018). Other ion channels are also involved in the altered repair processes in CF. Indeed, epidermal growth factor (EGF) and its receptor EGFR signaling, along with potassium channel function are impaired in CF and contribute to reduced cell migration and proliferation (Trinh et al., 2008). The activity and expression of the calcium activated chloride channel, Anoctamin 1 (ANO1), shown to be reduced in human and mice CF airway epithelial cells, could also delay CF airway epithelial cell proliferation and migration (Ruffin et al., 2013).
Dysregulated MicroRNA
MicroRNAs have emerged as important regulators in human physiological and pathological cell processes including the immune system, providing negative feedback regulation of inflammation and ion transport. In CF, microRNA profiling studies have shown that miR155, miR145, miR223, miR494, miR99b, let-7e, miR181b, and miR125a are increased in CF airway epithelial cell lines, CF bronchial brushing samples, and macrophages, compared to non-CF controls (McKiernan and Greene, 2015; Pierdomenico et al., 2017). In contrast, miR126, miR31, miR17 expression is decreased in CF airway epithelial cells. Decreased levels of miR126 correlate with up-regulation of TOM1, a negative regulator of pro-inflammatory cytokines. Decrease of miR31 in CF airways contributes to increased pulmonary cathepsin S production that activates the epithelial sodium channel and inactivates antimicrobial proteins (McKiernan and Greene, 2015). CFTR expression is under control of miR101 (Viart et al., 2015). Therefore, dysregulated microRNAs as critical regulators of epithelial immune responses can play a role in the intrinsic abnormalities of airway inflammation in CF.
Impact of SPMS Demonstrated in CF Models
Abnormal epithelial ion transport, excessive and non-resolving inflammation, chronic bacterial infection, and progressive lung destruction are the main features of CF airway disease, whether they are directly or indirectly related to CFTR misexpression or malfunction. Among the multiple cellular and molecular pathways involved in CF airway pathogenesis, the abnormal SPMs biosynthesis could play a central role. Indeed, SPMs have been demonstrated to regulate several distinct dysfunctions of CF airways that result in excessive and sustained inflammation (Table 1, Figure 4). Unless specified otherwise, the SPMs used in the studies described from this point on are synthetic exogenous SPMs which have the same chemical structure and half-life as their endogenous counterpart.
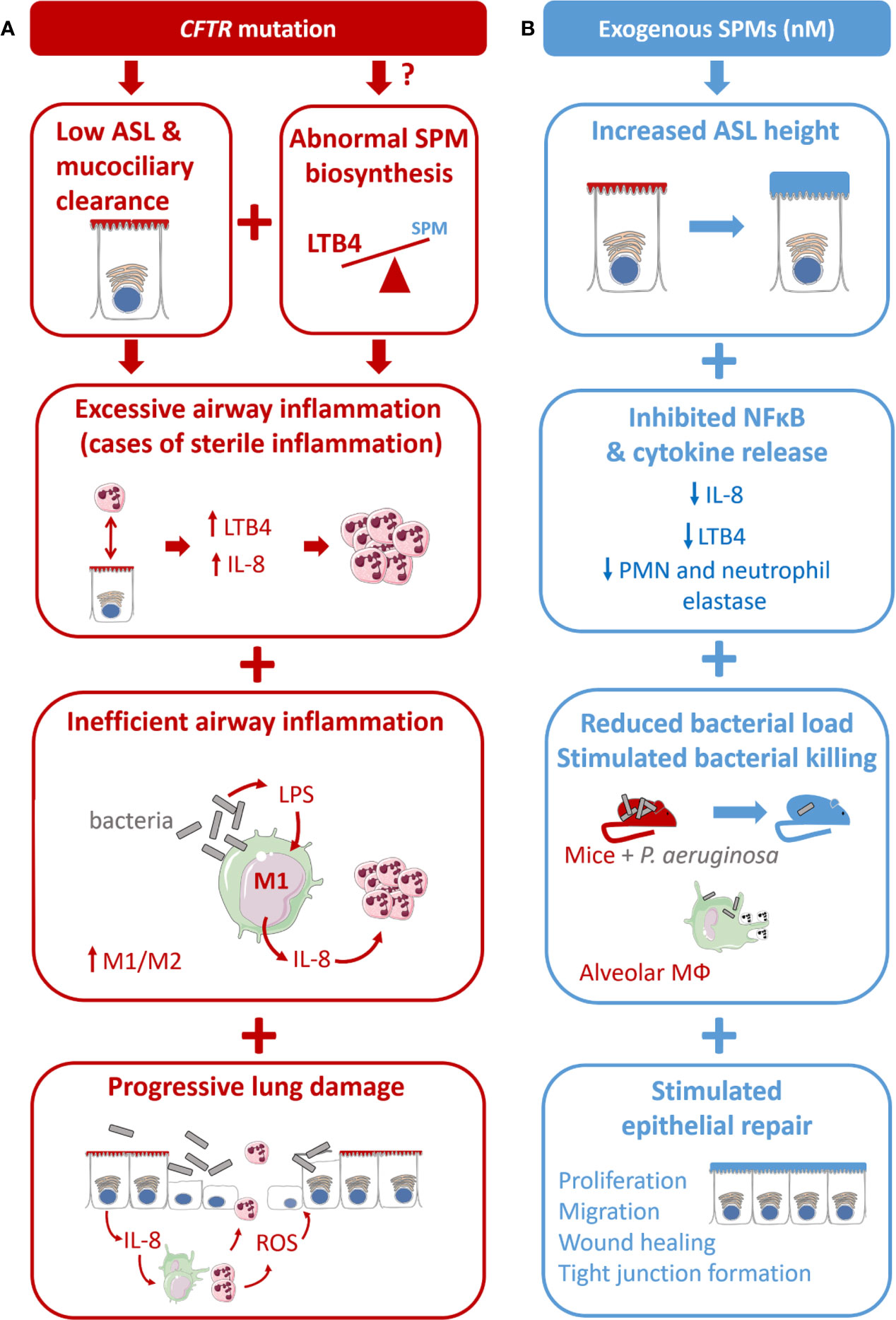
Figure 4 Proposed model for CF airway disease and beneficial impact of exogenous SPM exposure. (A) CF is due to the CFTR gene mutation leading to a reduced airway surface liquid layer (ASL) height and abnormal SPM biosynthesis. CF is characterized by an excessive airway inflammation with high amount of PMN and release of pro-inflammatory mediators (cytokines and LTB4). Inflammation in CF is inefficient to clear bacteria due to altered differentiation from M1 proinflammatory macrophages to M2 pro-resolving. Chronic inflammation and sustained inflammation, including oxidative stress (ROS) lead to progressive lung damage and respiratory failure. (B) The benefit of SPMs exposure at nM concentration is reported in different models of the CF airway disease. SPMs enhance the ASL height increase in CF bronchial epithelial cells. SPM treatment inhibits IL-8 release induced by TNF in CF airway epithelial cells. SPMs reduce bacterial load in mice models of P. aeruginosa infection and stimulate phagocytosis and bacterial killing in isolated CF alveolar macrophages. SPMs enhance tight junction formation and wound healing by stimulating CF airway epithelial proliferation and migration.
Epithelial Ion Transport, ASL and Calcium
Several in vivo and in vitro studies have provided evidence for SPMs’ involvement in regulating airway epithelial ionic transports and ASL layer height in models of airway epithelial diseases, including CF. In a rat model of acute lung injury (ALI), LXA4, RvD1, Mar1 enhance the expression of CFTR, ENaC (ENaC-α and ENaC-γ subunits), Na,K-ATPase (β1 subunit), and aquaporins leading to the stimulation of alveolar fluid clearance (AFC) (Yang et al., 2013; Wang Q. et al., 2014; Zhang et al., 2017) (Table 2).
In vitro studies of ion transport in CF airway epithelial cells. In human CF bronchial epithelial cells, LXA4 and RvD1 stimulate an increase of the ASL to a normal height (7 µm) (Verrière et al., 2012; Al-Alawi et al., 2014; Higgins et al., 2014; Ringholz et al., 2018). Stimulation of the surface airway hydration by these SPMs can be inhibited by the FPR2 receptor antagonist, BOC-2 and by BAPTA-AM, indicating this process to be mediated by the FPR2 receptor and highly dependent on intracellular calcium. Furthermore, LXA4 induces a large and sustained intracellular calcium increase in CF airway epithelial cells involving the stimulation of an apical ATP secretion by pannexin channels (PANX1). The subsequent activation of purino-receptors localized in these cells’ apical membrane generate calcium entry and calcium release from intracellular stores. A role for the P2Y11, a GPCR purino-receptor leading to a rise in both intracellular cAMP (stimulation of adenylate cyclase [AC]) and calcium mobilization (increased inositol 3 phosphate [IP3]) has been demonstrated upon exposure of CF airway epithelia cells to LXA4 (Figure 5) (Bonnans et al., 2003; Verrière et al., 2012; Higgins et al., 2014). In human CF airway epithelial cells, patch-clamp studies have demonstrated that LXA4 stimulates a calcium-activated chloride secretion (Verrière et al., 2012). Another study reported that the LXA4 effect in restoring the ASL height in CF airway epithelial cells also implicates ENaC inhibition (Al-Alawi et al., 2014) (Figure 5, Table 1).
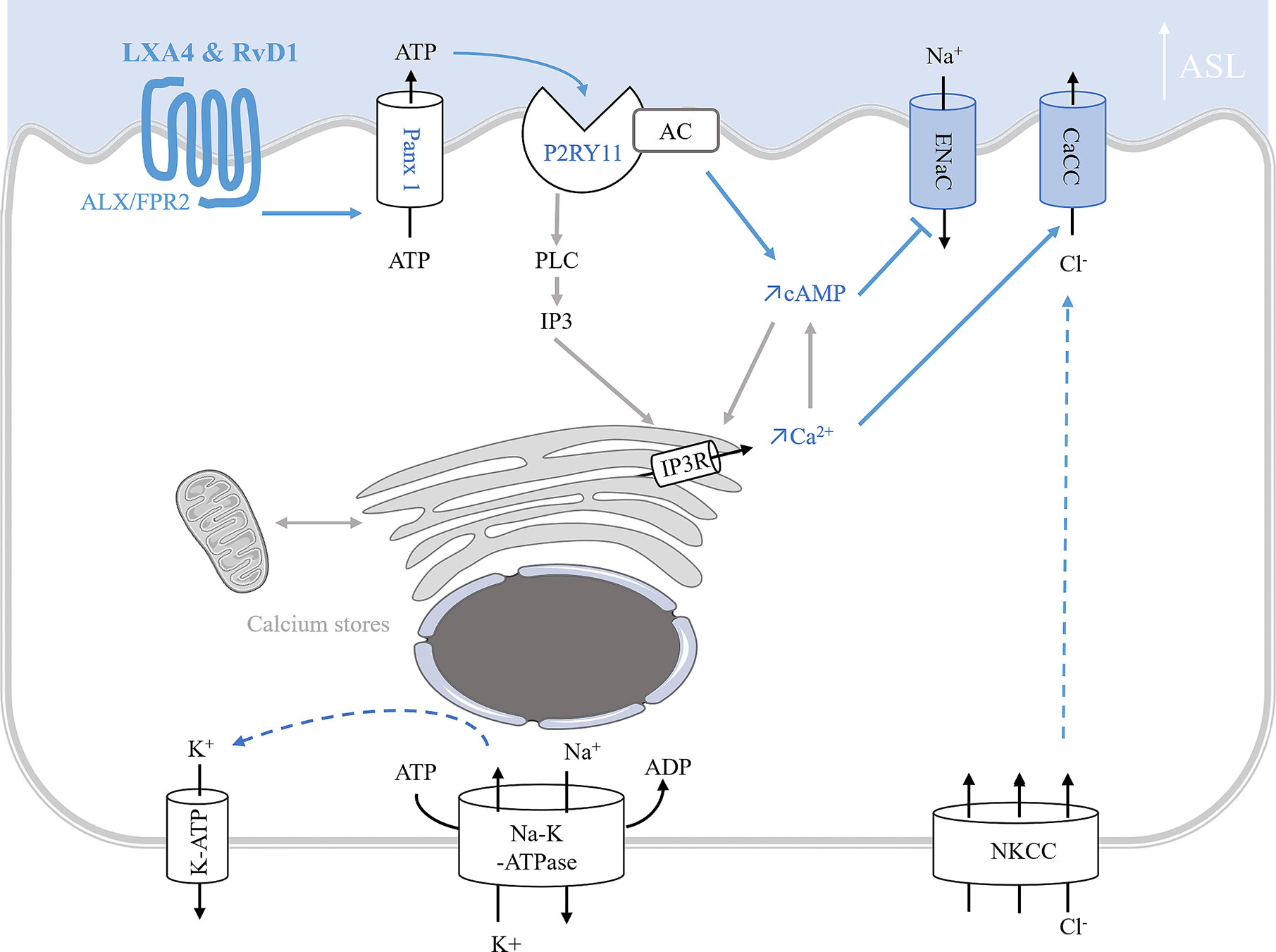
Figure 5 Model of ion transport regulation by LXA4 and RvD1 enabling the restoration of ASL height in CF human bronchial epithelial cells. LXA4 and RvD1 induce a calcium-dependent airway surface liquid (ASL) layer height increase in human CF bronchial epithelial cell lines and primary cultures (Bonnans et al., 2003; Al-Alawi et al., 2014; Higgins et al., 2014; Ringholz et al., 2018; Philippe and Urbach, 2018). This effect involves binding to the FPR2 receptor, stimulation of an ATP secretion into the airway lumen via pannexin 1 channels (Panx1), and subsequent activation of P2Yx purino-receptors, including P2Y11 which leads to a rise in both intracellular cAMP and calcium mobilization by stimulation of adenylate cyclase (AC) activity and inositol 3 phosphate (IP3) signaling pathway. Intracellular calcium and cAMP increases induce stimulation of calcium-activated chloride channel and inhibition of ENaC channel inhibition.
In vivo studies of ion transport in CF airway epithelial cells. The role of LXA4 and RvD1 on chloride secretion and sodium absorption was further confirmed in vivo by the recovery of nasal transepithelial potential difference in homozygous F508del CFTR mice (Ringholz et al., 2018) (Table 1).
Cytokine Production and Release
As part of the inflammatory response, the role of SPMs in inhibiting pro-inflammatory cytokines production (e.g.: IL-6, IL-8, IL-1β, and TNFα, IL-13) and stimulating anti-inflammatory cytokines (IL-4, IL-10) as well as inhibiting leukocyte chemotaxis and migration has been demonstrated in many chronic diseases. Their role has been established in models of acute lung injury, asthma, and COPD (Lee et al., 1989; Serhan et al., 1995; Papayianni et al., 1996; Hachicha et al., 1999; Bonnans et al., 2002; Vachier et al., 2005; Bonnans et al., 2006; Sun et al., 2007; Aoki et al., 2008; Isobe et al., 2012; Yang et al., 2013; Croasdell et al., 2015; Codagnone et al., 2018) as well as cellular models of viral and bacterial inflammation, or oxidative stress (Chiang et al., 2012; Hsiao et al., 2014; Wang L. et al., 2014; Wang Q. et al., 2014; Cox et al., 2015) and cigarette smoke-induced inflammation (Takamiya et al., 2012; Jiajia et al., 2014) (Table 3). The pathways involved in SPM’s regulation of inflammation are presented in Figure 6.
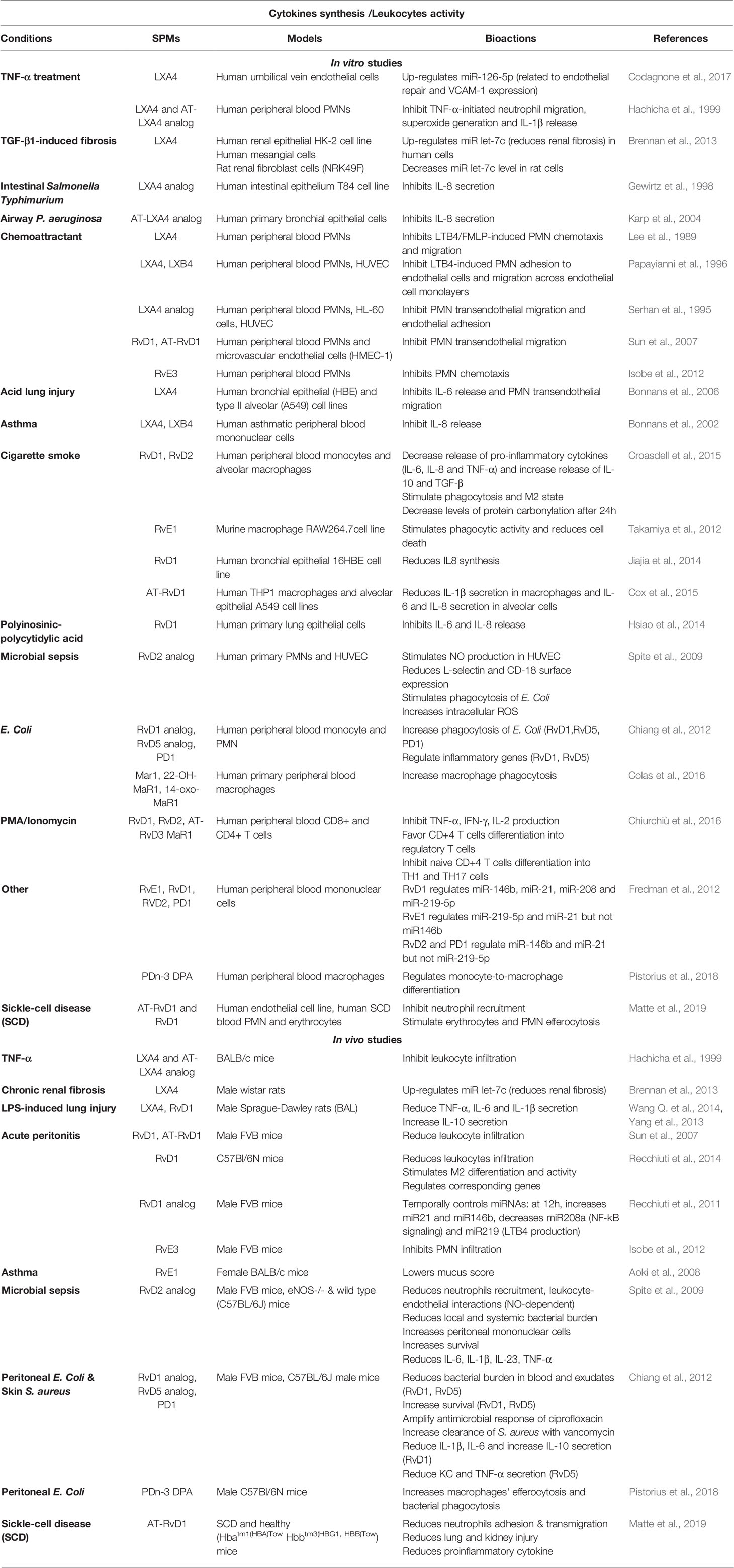
Table 3 Bioactions of SPMs on cytokine secretion and leukocytes activity, demonstrated in non-CF models.
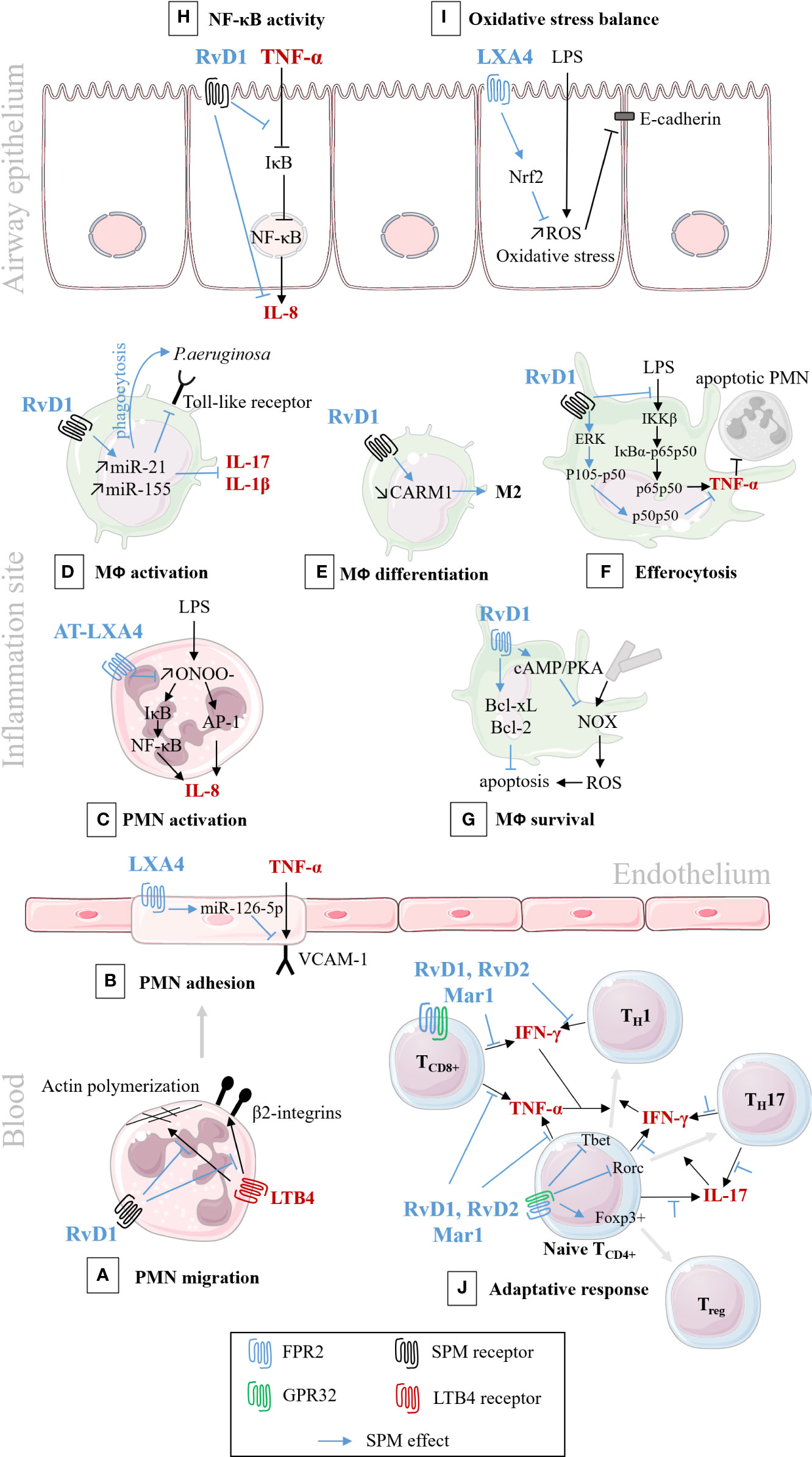
Figure 6 Schematic illustration of SPMs cellular pathways involved in the resolution of inflammation/infection. In black: pro-inflammatory pathways; In blue: bioactions of SPM. (A) RvD1 analog down-regulates the migration of PMNs isolated from human peripheral blood, by decreasing actin polymerization, and blocking LTB4-regulated adhesion molecules, 2 integrins. (Krishnamoorthy et al., 2010); (B) LXA4 down-regulates VCAM-1 receptor and PMN adhesion to human endothelial cells (Codagnone et al., 2017); (C) AT-LXA4 (15-epi-LXA4) down-regulates IL-8 synthesis and secretion, in human PMNs isolated from venous blood (Jozsef et al., 2002); (D) RvD1 down-regulates toll-like receptor (TLR), cytokines synthesis and enhances phagocytosis of murine lung macrophage (Codagnone et al., 2018); and enhances phagocytosis and bacterial killing in human CF alveolar macrophage (Ringholz et al., 2018); (E) RvD1 stimulates murine macrophage differentiation from M1 to M2 state (Recchiuti et al., 2014); (F) RvD1 stimulates efferocytosis of apoptotic PMNs, through the regulation of NF-κB pathways, in murine macrophage (Lee et al., 2013); (G) RvD1 inhibits ROS production and increases murine macrophage survival after efferocytosis (Lee and Surh, 2013); (H) RvD1 inhibits cytokines secretion by preventing IκB degradation in CF human airway epithelial cell (Ringholz et al., 2018); (I) LXA4 inhibits oxidative stress and protects E-cadherin, in human airway epithelial cell (Cheng et al., 2016); (J) RvD1, RvD2, and Mar1 regulate the adaptive response, enhance the differentiation to Treg rather than TH1 and TH17, in human peripheral blood lymphocytes (Chiurchiù et al., 2016).
In vitro studies in CF. In CF airway epithelial cells, LXA4 and RvD1 inhibit IL-8 synthesis by preventing IκB degradation induced by TNFα, thus resulting in NF-κB (Ringholz et al., 2018). RvD1 regulates specific genes and proteins involved in leukocyte chemotaxis and infiltration (e.g. CXCL1 [the mice IL-8]) in lung macrophages isolated from mice infected with P. aeruginosa (Codagnone et al., 2018; Isopi et al., 2020) (Table 1).
In vivo studies in P. aeruginosa infected mice. AT-LXA4 stable analog, reduces IL-8 levels and PMN recruitment in a murine model of short-term P. aeruginosa lung infection (Karp et al., 2004). RvD1 treatment also produces a significant decrease in PMN, IL-1β, and CXCL1 levels in vivo in the lungs of mice infected with P. aeruginosa (Codagnone et al., 2018) (Table 1).
Human studies in CF. Although, not a direct demonstration of SPMs’ impact on cytokine secretion, it is worth noting that human studies reveal that the levels of pro-inflammatory cytokines (IL-6 and IL-8) are inversely correlated with LXA4 and RvD1 in the sputum of patients with CF (Chiron et al., 2008; Ringholz et al., 2014; Eickmeier et al., 2017; Isopi et al., 2020).
Infection
Many actions exerted by SPMs to limit infection have been described in non-CF cells isolated from human and mice macrophages, (Chiang et al., 2012; Colas et al., 2016; Pierdomenico et al., 2017; Codagnone et al., 2018) (Table 3). In addition, SPMs (PDn-3DPA, RvD1, and RvD2) stimulate macrophage differentiation from a pro-inflammatory (M1) to a pro-resolutive phenotype (M2) in non-CF models (Dalli and Serhan, 2012; Recchiuti et al., 2014; Croasdell et al., 2015; Pistorius et al., 2018) and enhance the expression of surface receptors involved in the uptake of apoptotic cells (Matte et al., 2019) (Table 3). RvD2 also reduces polymicrobial sepsis severity in mice (Spite et al., 2009) and MaR1 and RvD3 enhance E. coli phagocytosis by macrophages (Colas et al., 2016) (Table 3).
In vitro studies in CF model of infection. The role of SPMs in regulating infection was also demonstrated in CF. RvD1 enhances phagocytosis of P. aeruginosa by human CF alveolar macrophages (Ringholz et al., 2018). LXA4 also stimulates CF bronchial epithelial cells’ protective functions by delaying P. aeruginosa invasion and migration (Higgins et al., 2016) (Table 1).
In vivo studies in CF model of infection. During P. aeruginosa lung infection in mice, exogenous administration of SPMs significantly decreases bacterial load, and improves clinical outcome. An AT-LXA4 stable analog, reduces bacterial burden in a murine model of short-term P. aeruginosa lung infection (Karp et al., 2004). RvD1 significantly diminishes bacterial growth, mucus metaplasia and lung inflammation resulting from long term exposure of mice to P. aeruginosa (Codagnone et al., 2018) (Table 1).
Epithelial Repair
In vitro studies have shown that SPMs (LXA4, RvD1, AT-RvD1 RvD2, and RvE1) protect tissues from lung injury in numerous models including CF airway epithelium (Zhang et al., 2010; Kenchegowda et al., 2011; Kakazu et al., 2012; Odusanwo et al., 2012; Buchanan et al., 2013; Higgins et al., 2014: Cheng et al., 2016; Higgins et al., 2016; Zhang et al., 2018; Zheng et al., 2018). LXA4 stimulated wound healing by enhancing cell proliferation, migration in human CF bronchial epithelial cells (Buchanan et al., 2013; Higgins et al., 2014) through stimulation of the ALX/FPR2 receptor, intracellular calcium mobilization, KATP potassium channel activation, and the mitogen-activated protein kinase ERK1/2 phosphorylation (Buchanan et al., 2013; Higgins et al., 2014). Moreover, LXA4 restores transepithelial resistance in CF airway epithelial cells by enhancing tight junction formation via upregulation of expression of the proteins ZO-1, occludin, claudin-1 during bacterial infection by P. aeruginosa (Grumbach et al., 2009; Higgins et al., 2016) (Figure 7) (Table 1). In corneal epithelium, the role of mitogen-activated protein kinase has also been reported to mediate the epithelial repair induced SPMs. The serine/threonine kinase Akt and NrF2 signaling pathways have been described in mediating the response to SPMs in corneal and other epithelial models (Zhang et al., 2010; Kenchegowda et al., 2011; Odusanwo et al., 2012; Wang L. et al., 2014; Posso et al., 2018; Zhang et al., 2018) (Table 4).
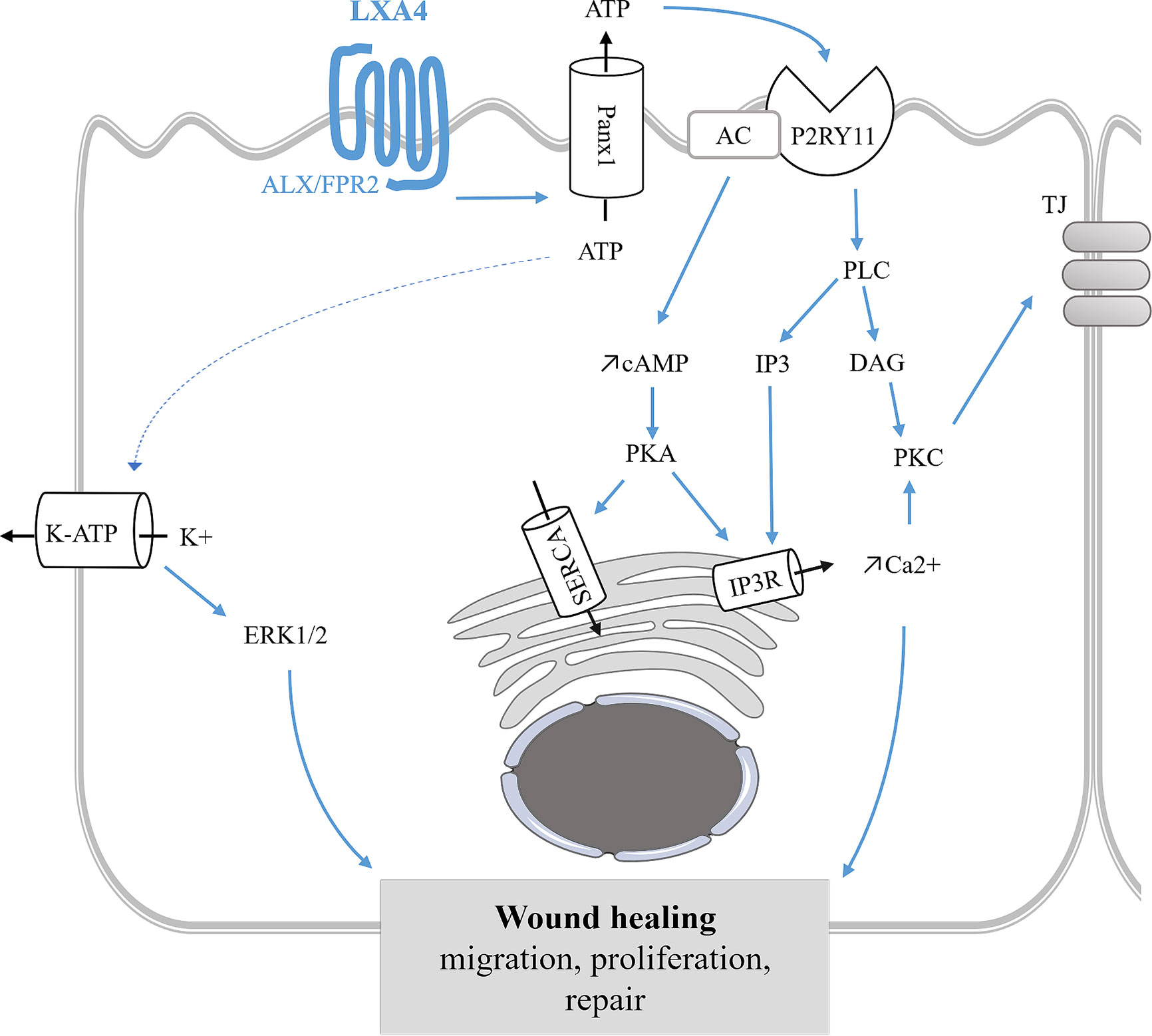
Figure 7 Epithelial repair mediated by LXA4 in bronchial epithelium. LXA4 stimulates wound healing by enhancing cell proliferation, migration of human CF bronchial epithelial cells. This mechanism is mediated by the FPR2 receptor, an apical ATP release and the subsequent stimulation of P2Yx receptors leading to intracellular calcium mobilization. The CF airway epithelial repair induced by LXA4 also involves ERK1/2 phosphorylation and KATP channel stimulation (Buchanan et al., 2013; Higgins et al., 2014). LXA4 maintains airway epithelial structure by stimulating tight junction (TJ) proteins synthesis and trafficking to the apical membrane leading to an increase of transepithelial electrical resistance (Grumbach et al., 2009). SPMs stimulation of the ERK pathway has also been demonstrated in corneal epithelial repair (Xu et al., 2009; Zhang et al., 2010; Kenchegowda et al., 2011; Zhang et al., 2018).
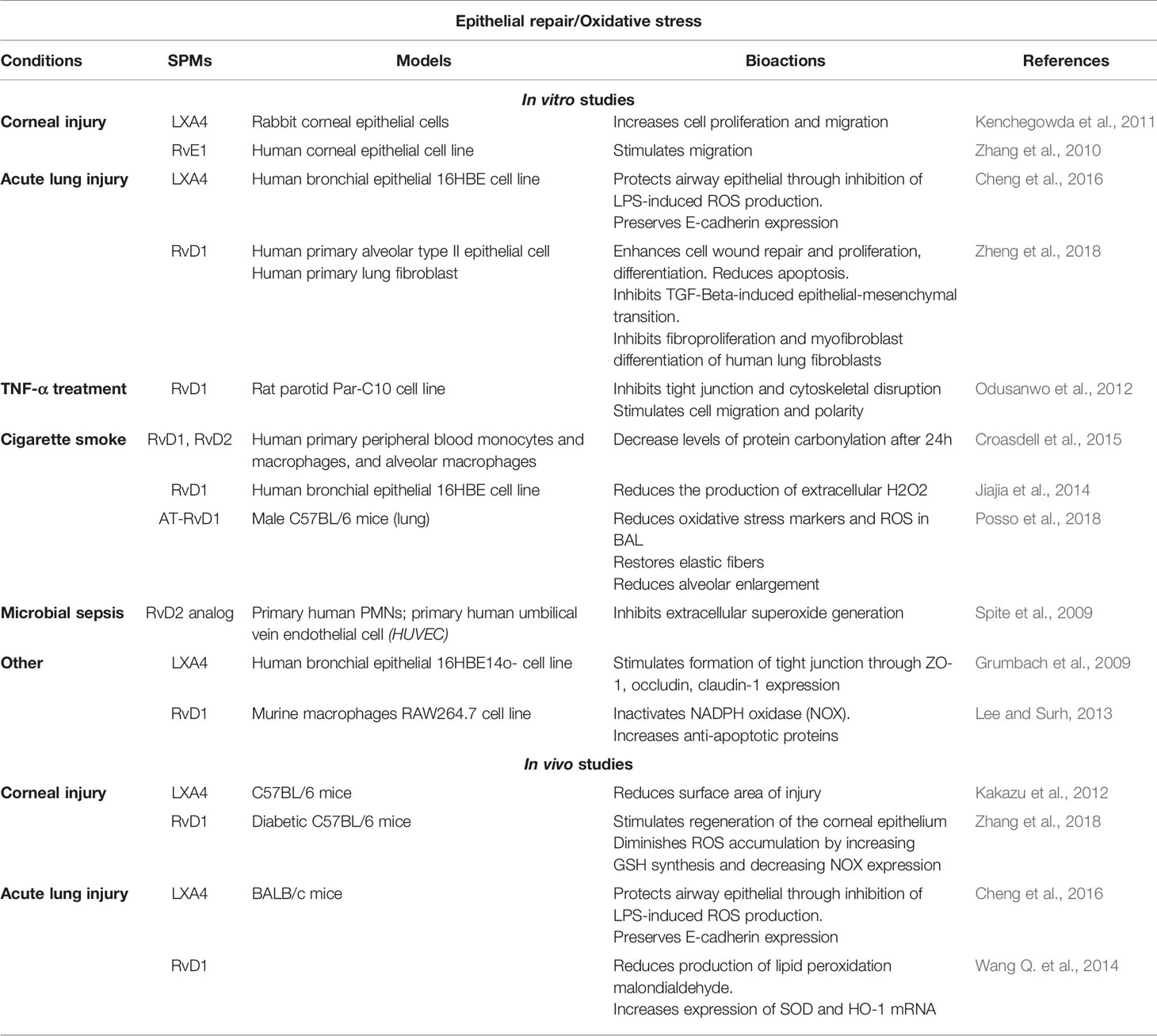
Table 4 Bioactions of SPMs on epithelial repair and oxidative stress, demonstrated in non-CF models.
Other Possible Impacts of SPMS on Altered Airway Functions in CF
Although not demonstrated in CF, the role of SPMs on airway functions altered in CF but demonstrated in other disease models can be pointed out (Tables 2–5).
Oxidative Stress
Antibiotics, despite their beneficial action on pulmonary function during acute infections, do not treat oxidative stress (Wood et al., 2002). Although not demonstrated in CF, SPMs have been found to help reduce oxidative stress by restoring oxidant/antioxidant balance through the nuclear erythroid 2-related transcription factor 2 (Nrf2) pathway, which is defective in CF (Posso et al., 2018; Zhang et al., 2018). In the murine model of cigarette smoke- and LPS-induced lung injury, or type 1-diabetes, RvD1 reduces ROS levels and increases antioxidant defense (Wang L. et al., 2014; Posso et al., 2018; Zhang et al., 2018). In corneal epithelium of type 1-diabetic mice, RvD1 increases the amount of the antioxidant GSH (Zhang et al., 2018). In vitro, following induced-efferocytosis, RvD1 inactivates NOX in murine macrophages (Lee and Surh, 2013). In human macrophages and bronchial epithelial cells undergoing cigarette-induced oxidative stress, RvD1 and RvD2 decrease protein oxidation (Croasdell et al., 2015) and decrease extracellular H2O2 production (Jiajia et al., 2014) (Table 4).
Mucus Secretion
Recent studies reveal the impact of SPMs on mucus secretion. Although not studied in CF, RvD1, LXA4, and Mar1 mediated an increase in glycoconjugate and mucin secretion in conjunctival cells (Hodges et al., 2017; Lippestad et al., 2017; Olsen et al., 2020). However, Mar1, RvD1, RvE1, and AT-RvD1 are able to block conjunctival goblet cell secretion when triggered by histamine or leukotriene (Dartt et al., 2011; Li et al., 2013; Olsen et al., 2020). In asthma, RvE1 reduced mucus score (Aoki et al., 2008) and RvD1 lowered mucous metaplasia in infected mice (Codagnone et al., 2018) (Table 2).
Regulation of MicroRNA
The microRNA signature of resolution of inflammation has recently started to be reported. Studies at basal state or during inflammation in mice and human leukocytes have revealed the capacity of resolvins (RvD1/D2, RvD1 stable analog, PD1 and RvE1) to regulate miRNAs that influence NF-κB activity and cytokine production (miR21, miR146b, miR208a, miR155), endothelial integrity (miR-126), leukotriene production (miR219), and pathogen recognition (miR21) (Recchiuti et al., 2011; Fredman et al., 2012; Codagnone et al., 2017; Codagnone et al., 2018). LXA4 also attenuates renal fibrosis by inducing let-7c (Brennan et al., 2013). Furthermore, miR-181b which is overexpressed in CF macrophages and airway epithelial cells, down-regulates the expression of the FPR2 receptor and the pro-resolution signaling pathways (Pierdomenico et al., 2015; Pierdomenico et al., 2017) (Table 5).
Adaptative Immunity
SPMs might also play a role in the mediation of the adaptive response. RvD1, RvD2, and Mar1 modulate adaptive immune responses in human peripheral blood lymphocytes. These SPMs prevent naïve CD4+ T cell differentiation into TH1 and TH17 in a mechanism mediated by the GPR32 and the FPR2 receptors (Chiurchiù et al., 2016). This role of SPMs in adaptive immunity might be of interest for CF. Indeed, some reports suggested T lymphocytes and activated eosinophils in airway mucosa in CF (Azzawi et al., 2012) and intrinsic impairment of T cell differentiation may contribute to the greater severity and more rapid progression of CF lung disease (Kushwah et al., 2014).
CF Airway Disease Treatments and Inflammation
Antibiotics
Until recently, antibiotics have been the only therapeutic agents that could be used to treat airways inflammation in CF. Antibiotics can work in synergy with the host immunity to kill bacteria with great efficiency. Antibiotics influence immune responses in various ways beyond merely eliminating the source of inflammation, depending on how they are used and how effective they are. Indeed, antibiotics that induce bacteria lysis can lead to the release of highly inflammatory molecules, pathogen-associated molecular patterns (PAMPs), such as lipopolysaccharide (LPS) and therefore augment inflammation (Raetz and Whitfield, 2002).
In contrast, some antibiotics have shown immunomodulatory properties (Ruh et al., 2017) such as macrolides which suppress inflammatory cytokines (Hoyt and Robbins, 2001; Amsden, 2005). Azithromycin has been particularly studied in CF and is routinely used for its immunomodulating effects, having shown to reduce exacerbation frequency and slightly improve lung function when taken continuously (Bush and Rubin, 2003; Southern and Barker, 2004; Olveira et al., 2017). Of interest, while PMN counts and IL-8 levels decrease in CF patients’ sputum after antibiotic therapy, LXA4 levels significantly increase and are inversely correlated with IL-8 levels (Chiron et al., 2008). However, despite its beneficial effects in CF patients, said effects are marginal, especially when taking into account the list of oral treatments patients already contend with on a daily basis, without mentioning the long half-life of this antibiotic and the potential risk of selecting resistant bacteria strains and altering microbiota.
CFTR Modulators
High-throughput screening has allowed to identify small molecules, such as lumacaftor (VX809) tezacaftor (VX661), and elexacaftor (VX-445) to modulate processing and trafficking of CFTR (CFTR correctors) and ivacaftor (VX770) to potentiate its activity as a chloride channel (CFTR potentiator) providing the first drugs to specifically target the CFTR protein defect. Associations of these molecules, the first ones being currently prescribed in routine since the mid-2010s, restore chloride transport and normalizing sweat test results (Lopes-Pacheco, 2019). Beside their main action on epithelial chloride transport and encouraging results on disease progression, their impact on inflammatory cytokine production are controversial and their long term immunomodulatory effects are still discussed (Jarosz-Griffiths et al., 2020; Volkova et al., 2020). Indeed, the corrector VX809 does not produce an effect on proinflammatory cytokines production by macrophages exposed to P. aeruginosa while stimulating their phagocytosis activity. In contrast, the potentiator VX770 reduces proinflammatory cytokines but inhibits macrophages’ phagocytosis activity (Barnaby et al., 2018). VX809 alone or in combination with VX770 does not affect pro-inflammatory cytokines production by CF airway epithelial cells. P. aeruginosa exposure however reduces VX809-stimulated F508del-CFTR chloride secretion by airway epithelial cells (Stanton et al., 2015). However, another study has shown that correcting CFTR activity by a combination of VX809 and VX770 reduces IL-8 production by CF (F508del) airway epithelial cells and enhanced epithelial repair (Ruffin et al., 2018). The effects of the latest VX-445 on inflammation have yet to be assessed. Although these small molecules have considerably changed the quality of life for many patients, not all CF genotypes can benefit from such therapy. Moreover, the clinical response within an identical genotype is variable and lung function still declines over time when bronchiectasis is established.
Novel Anti-Inflammatory Molecules
To combat inflammation, non-steroid anti-inflammatory drugs (NSAID) have been studied; especially Ibuprofen, which seems to slow lung disease progression (Lands and Stanojevic, 2013) but at the cost of long term usage at high doses and the entailing risks.
Taking another approach and directly targeting biosynthesis of the inflammatory eicosanoid LTB4, acebilustat is a new anti-inflammatory oral drug that inhibits LTA4H. As LTB4 is one of the inflammatory eicosanoids that initiate and amplify PMNs’ recruitment, suppressing its effect could diminish the pulmonary consequences of overactive PMNs. In a phase I study, acebilustat has decreased PMN inflammation biomarkers levels in sputum and was well tolerated with only mild to moderate adverse events reported (Elborn et al., 2017). However, a phase II randomized control trial (RCT) did not meet its primary endpoint and no further trials with acebilustat are planned (Elborn et al., 2018). In addition, these anti-inflammatory molecules clearly have a different mechanism of action than immuno-resolvents.
Lenabasum, formerly called JBT-101, is another oral drug under investigation for autoimmune diseases and CF. A first phase II trial conducted with 83 patients has established the safety and tolerance of this drug in CF patients. Another phase II multi-center RCT is ongoing for CF patients with pulmonary exacerbations as the primary outcome. Lenabasum is an endocannabinoid-mimetic that selectively binds to cannabinoid receptor 2 expressed by immune cells, reducing alveolar macrophage secretion of IL-6 and TNFα in preclinical studies (Ribeiro et al., 2017). Those immunomodulatory effects can be explained by lenabasum’s potential to induce SPMs production in animal models (Zurier et al., 2009) and healthy human subjects (Motwani et al., 2018a). This last example showcases how treating chronic inflammation by mobilizing the body’s endogenous pro-resolving system rather than trying to stop one of the many triggers of an established and thriving inflammatory process may be the most inclusive and efficient way to treat inflammatory diseases in the future.
Conclusion
Nearly 30 years after the discovery of the CFTR gene, many aspects of the pathophysiology of CF remain unclear. The combination of advanced knowledge in the field of the regulation of innate immunity and the current description of CF airway disease revealed novel cellular and molecular mechanisms involved in the abnormal resolution of inflammation in CF. This review highlighted evidence for a role of SPMs to overcome the absence of functional CFTR by stimulating chloride secretion through calcium-dependent channels, while limiting unwanted persistence of inflammation, infection, and tissue damage, and accelerating the return to homeostasis by acting on multiple cells and molecular targets (Figure 4).
Even in the age of CFTR modulators, anti-inflammatory therapy remains an area of intense research in CF. The therapeutic use of SPMs might constitute a promising avenue to treat chronic inflammation and infection in all CF patients. However, the therapeutic use of SPMs is greatly limited by their rapid metabolic inactivation before they can reach the site of inflammation. An RvE1 stable analog for dry eye inflammation (NCT00799552), ocular inflammation and pain in cataract surgery (NCT02329743), and allergic conjunctivitis (NCT01639846), and a LXA4 stable analog for periodontal inflammation (NCT0234269) are under clinical trials. The use of SPMs stable analog incorporated in nanoparticles also provides a new possibility for local delivery at the site of inflammation (Van Dyke et al., 2015; Lance et al., 2017). Although promising results have been obtained in animal studies, the efficacy of this experimental data must be established in human clinical trials. Indeed, using analogs with a much longer half-life than native SPMs does raise the question of the potential adverse effects. Long-term exposure to stable SPM analogs with prolonged and enhanced activity might prevent the initiation of further inflammatory responses. Another approach would be to understand more precisely the mechanism by which CFTR dysfunction is related to an abnormal SPMs biosynthesis and potentially reveal new therapeutic targets to treat a wide range of CF mutations. Furthermore, the presentation of CF is very heterogeneous in terms of severity and response to actual treatments; therefore, exploring the correlation at the individual level between SPMs biosynthesis, CF genotype, and the severity of the airway disease could lead to new diagnostic and therapeutic tools as personalized care.
Author Contributions
MB wrote the first draft on the impact of SPMs on airway functions altered in CF. MS wrote the first draft of the abnormal lipid metabolism and SPMs biosynthesis in CF. All authors contributed to the article and approved the submitted version.
Conflict of Interest
The authors declare that the research was conducted in the absence of any commercial or financial relationships that could be construed as a potential conflict of interest.
Acknowledgments
We thank the Institut National de la Santé Et de la Recherche Médicale (INSERM), the French CF patients association (Vaincre la Mucoviscidose, RF20190502472), the Chancellerie des Universités de Paris (Legs Poix 2020), the Institut Mondor de Recherche Biomédicale (AO inter équipes 2020), the Agence Régionale de Santé Normandie which currently support the authors activity on the role of SPMs in Cystic Fibrosis.
References
Adam, D., Bilodeau, C., Sognigbé, L., Maillé, É., Ruffin, M., Brochiero, E. (2018). CFTR rescue with VX-809 and VX-770 favors the repair of primary airway epithelial cell cultures from patients with class II mutations in the presence of Pseudomonas aeruginosa exoproducts. J. Cyst. Fibros. 17, 705–714. doi: 10.1016/j.jcf.2018.03.010
Al-Alawi, M., Buchanan, P., Verriere, V., Higgins, G., McCabe, O., Costello, R. W., et al. (2014). Physiological levels of lipoxin A4 inhibit ENaC and restore airway surface liquid height in cystic fibrosis bronchial epithelium. Physiol. Rep. 2, e12093. doi: 10.14814/phy2.12093
Al-Turkmani, M. R., Freedman, S. D., Laposata, M. (2007). Fatty acid alterations and n-3 fatty acid supplementation in cystic fibrosis. Prostaglandins Leukot. Essent. Fatty Acids 77, 309–318. doi: 10.1016/j.plefa.2007.10.009
Amsden, G. W. (2005). Anti-inflammatory effects of macrolides–an underappreciated benefit in the treatment of community-acquired respiratory tract infections and chronic inflammatory pulmonary conditions? J. Antimicrob. Chemother. 55, 10–21. doi: 10.1093/jac/dkh519
Anderson, M. P., Gregory, R. J., Thompson, S., Souza, D. W., Paul, S., Mulligan, R. C., et al. (1991). Demonstration that CFTR is a chloride channel by alteration of its anion selectivity. Science 253, 202–205. doi: 10.1126/science.1712984
Antigny, F., Norez, C., Becq, F., Vandebrouck, C. (2008a). Calcium homeostasis is abnormal in cystic fibrosis airway epithelial cells but is normalized after rescue of F508del-CFTR. Cell Calcium 43, 175–183. doi: 10.1016/j.ceca.2007.05.002
Antigny, F., Norez, C., Cantereau, A., Becq, F., Vandebrouck, C. (2008b). Abnormal spatial diffusion of Ca2+ in F508del-CFTR airway epithelial cells. Respir. Res. 9, 70. doi: 10.1186/1465-9921-9-70
Antigny, F., Girardin, N., Raveau, D., Frieden, M., Becq, F., Vandebrouck, C. (2009). Dysfunction of mitochondria Ca2+ uptake in cystic fibrosis airway epithelial cells. Mitochondrion 9, 232–241. doi: 10.1016/j.mito.2009.02.003
Antigny, F., Norez, C., Dannhoffer, L., Bertrand, J., Raveau, D., Corbi, P., et al. (2011). Transient Receptor Potential Canonical Channel 6 Links Ca2+ Mishandling to Cystic Fibrosis Transmembrane Conductance Regulator Channel Dysfunction in Cystic Fibrosis. Am. J. Respir. Cell Mol. Biol. 44, 83–90. doi: 10.1165/rcmb.2009-0347OC
Aoki, H., Hisada, T., Ishizuka, T., Utsugi, M., Kawata, T., Shimizu, Y., et al. (2008). Resolvin E1 dampens airway inflammation and hyperresponsiveness in a murine model of asthma. Biochem. Biophys. Res. Commun. 367, 509–515. doi: 10.1016/j.bbrc.2008.01.012
Arita, M., Oh, S. F., Chonan, T., Hong, S., Elangovan, S., Sun, Y. P., et al. (2006). Metabolic inactivation of resolvin E1 and stabilization of its anti-inflammatory actions. J. Biol. Chem. 281, 22847–22854. doi: 10.1074/jbc.M603766200
Armstrong, D. S., Grimwood, K., Carlin, J. B., Carzino, R., Gutièrrez, J. P., Hull, J., et al. (1997). Lower airway inflammation in infants and young children with cystic fibrosis. Am. J. Respir. Crit. Care Med. 156, 1197–1204. doi: 10.1164/ajrccm.156.4.96-11058
Atlante, A., Favia, M., Bobba, A., Guerra, L., Casavola, V., Reshkin, S. J. (2016). Characterization of mitochondrial function in cells with impaired cystic fibrosis transmembrane conductance regulator (CFTR) function. J. Bioenerg. Biomembr. 48, 197–210. doi: 10.1007/s10863-016-9663-y
Azzawi, M., Johnston, P. W., Majumdar, S., Kay, A. B., Jeffery, P. K. (2012). T Lymphocytes and Activated Eosinophils in Airway Mucosa in Fatal Asthma and Cystic Fibrosis. Am. Rev. Respir. Dis. 145 (6), 1477–1482. doi: 10.1164/ajrccm/145.6.1477
Balázs, A., Mall, M. A. (2019). Mucus obstruction and inflammation in early cystic fibrosis lung disease: Emerging role of the IL-1 signaling pathway. Pediatr. Pulmonol. 54(Suppl 3), S5–S12. doi: 10.1002/ppul.24462
Balghi, H., Robert, R., Rappaz, B., Zhang, X., Wohlhuter-Haddad, A., Evagelidis, A., et al. (2011). Enhanced Ca2+ entry due to Orai1 plasma membrane insertion increases IL-8 secretion by cystic fibrosis airways. FASEB J. 25, 4274–4291. doi: 10.1096/fj.11-187682
Ballard, S. T., Trout, L., Mehta, A., Inglis, S. K. (2002). Liquid secretion inhibitors reduce mucociliary transport in glandular airways. Am. J. Physiol.-Lung Cell. Mol. Physiol. 283, L329–L335. doi: 10.1152/ajplung.00277.2001
Balode, L., Strazda, G., Jurka, N., Kopeika, U., Kislina, A., Bukovskis, M., et al. (2012). Lipoxygenase-derived arachidonic acid metabolites in chronic obstructive pulmonary disease. Medicina (Kaunas) 48 (6), 292–298.
Balough, K., McCubbin, M., Weinberger, M., Smits, W., Ahrens, R., Fick, R. (1995). The relationship between infection and inflammation in the early stages of lung disease from cystic fibrosis. Pediatr. Pulmonol 20, 63–70. doi: 10.1002/ppul.1950200203
Bang, S., Xie, Y.-K., Zhang, Z.-J., Wang, Z., Xu, Z.-Z., Ji, R.-R. (2018). GPR37 regulates macrophage phagocytosis and resolution of inflammatory pain. J. Clin. Invest. 128, 3568–3582. doi: 10.1172/JCI99888
Bangel, N., Dahlhoff, C., Sobczak, K., Weber, W.-M., Kusche-Vihrog, K. (2008). Upregulated expression of ENaC in human CF nasal epithelium. J. Cyst. Fibros. 7, 197–205. doi: 10.1016/j.jcf.2007.07.012
Barden, A. E., Mas, E., Mori, T. A. (2016). n-3 Fatty acid supplementation and proresolving mediators of inflammation. Curr. Opin. Lipidol. 27, 26–32. doi: 10.1097/MOL.0000000000000262
Barnaby, R., Koeppen, K., Nymon, A., Hampton, T. H., Berwin, B., Ashare, A., et al. (2018). Lumacaftor (VX-809) restores the ability of CF macrophages to phagocytose and kill Pseudomonas aeruginosa. Am. J. Physiol. Lung Cell. Mol. Physiol. 314, L432–L438. doi: 10.1152/ajplung.00461.2017
Barnig, C., Cernadas, M., Dutile, S., Liu, X., Perrella, M. A., Kazani, S., et al. (2013). Lipoxin A4 regulates natural killer cell and type 2 innate lymphoid cell activation in asthma. Sci. Transl. Med. 5, 174ra26. doi: 10.1126/scitranslmed.3004812
Bartlett, J. A., Ramachandran, S., Wohlford-Lenane, C. L., Barker, C. K., Pezzulo, A. A., Zabner, J., et al. (2016). Newborn Cystic Fibrosis Pigs Have a Blunted Early Response to an Inflammatory Stimulus. Am. J. Respir. Crit. Care Med. 194, 845–854. doi: 10.1164/rccm.201510-2112OC
Bartling, T. R., Drumm, M. L. (2009). Oxidative Stress Causes IL8 Promoter Hyperacetylation in Cystic Fibrosis Airway Cell Models. Am. J. Respir. Cell Mol. Biol. 40, 58–65. doi: 10.1165/rcmb.2007-0464OC
Bena, S., Brancaleone, V., Wang, J. M., Perretti, M., Flower, R. J. (2012). Annexin A1 interaction with the FPR2/ALX receptor: identification of distinct domains and downstream associated signaling. J. Biol. Chem. 287, 24690–24697. doi: 10.1074/jbc.M112.377101
Berridge, M. J., Bootman, M. D., Roderick, H. L. (2003). Calcium signalling: dynamics, homeostasis and remodelling. Nat. Rev. Mol. Cell Biol. 4, 517–529. doi: 10.1038/nrm1155
Bhura-Bandali, F. N., Suh, M., Man, S. F., Clandinin, M. T. (2000). The deltaF508 mutation in the cystic fibrosis transmembrane conductance regulator alters control of essential fatty acid utilization in epithelial cells. J. Nutr. 130, 2870–2875. doi: 10.1093/jn/130.12.2870
Birnbaum, Y., Ye, Y., Lin, Y., Freeberg, S. Y., Huang, M.-H., Perez-Polo, J. R., et al. (2007). Aspirin augments 15-epi-lipoxin A4 production by lipopolysaccharide, but blocks the pioglitazone and atorvastatin induction of 15-epi-lipoxin A4 in the rat heart. Prostaglandins Other Lipid Mediat. 83, 89–98. doi: 10.1016/j.prostaglandins.2006.10.003
Blohmke, C. J., Mayer, M. L., Tang, A. C., Hirschfeld, A. F., Fjell, C. D., Sze, M. A., et al. (2012). Atypical Activation of the Unfolded Protein Response in Cystic Fibrosis Airway Cells Contributes to p38 MAPK-Mediated Innate Immune Responses. J. Immunol. 189, 5467–5475. doi: 10.4049/jimmunol.1103661
Bobadilla, J. L., Macek, M., Fine, J. P., Farrell, P. M. (2002). Cystic fibrosis: a worldwide analysis of CFTR mutations–correlation with incidence data and application to screening. Hum. Mutat. 19, 575–606. doi: 10.1002/humu.10041
Boncoeur, E., Criq, V. S., Bonvin, E., Roque, T., Henrion-Caude, A., Gruenert, D. C., et al. (2008). Oxidative stress induces extracellular signal-regulated kinase 1/2 mitogen-activated protein kinase in cystic fibrosis lung epithelial cells: Potential mechanism for excessive IL-8 expression. Int. J. Biochem. Cell Biol. 40, 432–446. doi: 10.1016/j.biocel.2007.08.013
Bonfield, T. L., Panuska, J. R., Konstan, M. W., Hilliard, K. A., Hilliard, J. B., Ghnaim, H., et al. (1995). Inflammatory cytokines in cystic fibrosis lungs. Am. J. Respir. Crit. Care Med. 152, 2111–2118. doi: 10.1164/ajrccm.152.6.8520783
Bonnans, C., Vachier, I., Chavis, C., Godard, P., Bousquet, J., Chanez, P. (2002). Lipoxins are potential endogenous antiinflammatory mediators in asthma. Am. J. Respir. Crit. Care Med. 165, 1531–1535. doi: 10.1164/rccm.200201-053OC
Bonnans, C., Mainprice, B., Chanez, P., Bousquet, J., Urbach, V. (2003). Lipoxin A4 stimulates a cytosolic Ca2+ increase in human bronchial epithelium. J. Biol. Chem. 278, 10879–10884. doi: 10.1074/jbc.M210294200
Bonnans, C., Fukunaga, K., Levy, M. A., Levy, B. D. (2006). Lipoxin A4 Regulates Bronchial Epithelial Cell Responses to Acid Injury. Am. J. Pathol. 168, 1064–1072. doi: 10.2353/ajpath.2006.051056
Borot, F., Vieu, D.-L., Faure, G., Fritsch, J., Colas, J., Moriceau, S., et al. (2009). Eicosanoid release is increased by membrane destabilization and CFTR inhibition in Calu-3 cells. PLoS One 4, e7116. doi: 10.1371/journal.pone.0007116
Botten, N., Hodges, R. R., Li, D., Bair, J. A., Shatos, M. A., Utheim, T. P., et al. (2019). Resolvin D2 elevates cAMP to increase intracellular [Ca2+] and stimulate secretion from conjunctival goblet cells. FASEB J. 33, 8468–8478. doi: 10.1096/fj.201802467R
Boucher, R. C. (2007). Evidence for airway surface dehydration as the initiating event in CF airway disease. J. Intern. Med. 261, 5–16. doi: 10.1111/j.1365-2796.2006.01744.x
Brady, H. R., Papayianni, A., Serhan, C. N. (1995). Potential vascular roles for lipoxins in the “stop programs” of host defense and inflammation. Trends Cardiovasc. Med. 5, 186–192. doi: 10.1016/1050-1738(95)00055-E
Brennan, E. P., Nolan, K. A., Börgeson, E., Gough, O. S., McEvoy, C. M., Docherty, N. G., et al. (2013). Lipoxins Attenuate Renal Fibrosis by Inducing let-7c and Suppressing TGF β R1. J. Am. Soc Nephrol. 24, 627–637. doi: 10.1681/ASN.2012060550
Brown, R. K., Wyatt, H., Price, J. F., Kelly, F. J. (1996). Pulmonary dysfunction in cystic fibrosis is associated with oxidative stress. Eur. Respir. J. 9, 334–339. doi: 10.1183/09031936.96.09020334
Brüne, B. (2005). The Intimate Relation Between Nitric Oxide and Superoxide in Apoptosis and Cell Survival. Antioxid. Redox Signal. 7, 497–507. doi: 10.1089/ars.2005.7.497
Bruscia, E. M., Bonfield, T. L. (2016). Cystic Fibrosis Lung Immunity: The Role of the Macrophage. J. Innate Immun. 8, 550–563. doi: 10.1159/000446825
Bruscia, E. M., Zhang, P.-X., Ferreira, E., Caputo, C., Emerson, J. W., Tuck, D., et al. (2009). Macrophages Directly Contribute to the Exaggerated Inflammatory Response in Cystic Fibrosis Transmembrane Conductance Regulator–/– Mice. Am. J. Respir. Cell Mol. Biol. 40, 295–304. doi: 10.1165/rcmb.2008-0170OC
Bruscia, E. M., Zhang, P.-X., Satoh, A., Caputo, C., Medzhitov, R., Shenoy, A., et al. (2011). Abnormal trafficking and degradation of TLR4 underlie the elevated inflammatory response in cystic fibrosis. J. Immunol. Baltim. Md 1950 186, 6990–6998. doi: 10.4049/jimmunol.1100396
Buchanan, P. J., McNally, P., Harvey, B. J., Urbach, V. (2013). Lipoxin A4;-mediated KATP potassium channel activation results in cystic fibrosis airway epithelial repair. Am. J. Physiol. Lung Cell. Mol. Physiol. 305, L193–L201. doi: 10.1152/ajplung.00058.2013
Bush, A., Rubin, B. K. (2003). Macrolides as Biological Response Modifiers in Cystic Fibrosis and Bronchiectasis. Semin. Respir. Crit. Care Med. 24, 737–748. doi: 10.1055/s-2004-815669
Button, B., Cai, L.-H., Ehre, C., Kesimer, M., Hill, D. B., Sheehan, J. K., et al. (2012). Periciliary Brush Promotes the Lung Health by Separating the Mucus Layer from Airway Epithelia. Science 337, 937–941. doi: 10.1126/science.1223012
Bystrom, J., Evans, I., Newson, J., Stables, M., Toor, I., van Rooijen, N., et al. (2008). Resolution-phase macrophages possess a unique inflammatory phenotype that is controlled by cAMP. Blood 112, 4117–4127. doi: 10.1182/blood-2007-12-129767
Cabrini, G., De Togni, P. (1985). Increased cytosolic calcium in cystic fibrosis neutrophils effect on stimulus - secretion coupling. Life Sci. 36, 1561–1567. doi: 10.1016/0024-3205(85)90380-7
Causer, A. J., Shute, J. K., Cummings, M. H., Shepherd, A., II, Gruet, M., Costello, J. T., et al. (2020). Circulating biomarkers of antioxidant status and oxidative stress in people with cystic fibrosis: A systematic review and meta-analysis. Redox Biol. 32, 101436. doi: 10.1016/j.redox.2020.101436
Chao, A. C., Zifferblatt, J. B., Wagner, J. A., Dong, Y.-J., Gruenert, D. C., Gardner, P. (1994). Stimulation of chloride secretion by P1 purinoceptor agonists in cystic fibrosis phenotype airway epithelial cell line CFPEo-. Br. J. Pharmacol. 112, 169–175. doi: 10.1111/j.1476-5381.1994.tb13047.x
Chen, J., Kinter, M., Shank, S., Cotton, C., Kelley, T. J., Ziady, A. G. (2008). Dysfunction of Nrf-2 in CF Epithelia Leads to Excess Intracellular H2O2 and Inflammatory Cytokine Production. PLoS One 3, e3367. doi: 10.1371/journal.pone.0003367
Chen, J.-H., Stoltz, D. A., Karp, P. H., Ernst, S. E., Pezzulo, A. A., Moninger, T. O., et al. (2010). Loss of anion transport without increased sodium absorption characterizes newborn porcine cystic fibrosis airway epithelia. Cell 143, 911–923. doi: 10.1016/j.cell.2010.11.029
Cheng, X., He, S., Yuan, J., Miao, S., Gao, H., Zhang, J., et al. (2016). Lipoxin A4 attenuates LPS-induced mouse acute lung injury via Nrf2-mediated E-cadherin expression in airway epithelial cells. Free Radic. Biol. Med. 93, 52–66. doi: 10.1016/j.freeradbiomed.2016.01.026
Chiang, N., Serhan, C. N. (2004). Aspirin triggers formation of anti-inflammatory mediators: New mechanism for an old drug. Discov. Med. 4, 470–475. doi: 10.1038/ncb1456
Chiang, N., Serhan, C. N. (2017). Structural elucidation and physiologic functions of specialized pro-resolving mediators and their receptors. Mol. Aspects Med. 58, 114–129. doi: 10.1016/j.mam.2017.03.005
Chiang, N., Takano, T., Arita, M., Watanabe, S., Serhan, C. N. (2003). A novel rat lipoxin A4 receptor that is conserved in structure and function. Br. J. Pharmacol. 139, 89–98. doi: 10.1038/sj.bjp.0705220
Chiang, N., Serhan, C. N., Dahlén, S.-E., Drazen, J. M., Hay, D. W. P., Rovati, G. E., et al. (2006). The lipoxin receptor ALX: potent ligand-specific and stereoselective actions in vivo. Pharmacol. Rev. 58, 463–487. doi: 10.1124/pr.58.3.4
Chiang, N., Fredman, G., Bäckhed, F., Oh, S. F., Vickery, T., Schmidt, B. A., et al. (2012). Infection regulates pro-resolving mediators that lower antibiotic requirements. Nature 484, 524–528. doi: 10.1038/nature11042
Chiang, N., Shinohara, M., Dalli, J., Mirakaj, V., Kibi, M., Choi, A. M. K., et al. (2013). Inhaled Carbon Monoxide Accelerates Resolution of Inflammation via Unique Proresolving Mediator–Heme Oxygenase-1 Circuits. J. Immunol. 190, 6378–6388. doi: 10.4049/jimmunol.1202969
Chiang, N., Dalli, J., Colas, R. A., Serhan, C. N. (2015). Identification of resolvin D2 receptor mediating resolution of infections and organ protection. J. Exp. Med. 212, 1203–1217. doi: 10.1084/jem.20150225
Chiang, N., de la Rosa, X., Libreros, S., Serhan, C. N. (2017). Novel Resolvin D2 Receptor Axis in Infectious Inflammation. J. Immunol. Baltim. Md 1950 198, 842–851. doi: 10.4049/jimmunol.1601650
Chiang, N., Libreros, S., Norris, P. C., de la Rosa, X., Serhan, C. N. (2019). Maresin 1 activates LGR6 receptor promoting phagocyte immunoresolvent functions. J. Clin. Invest. 129, 5294–5311. doi: 10.1172/JCI129448
Chiron, R., Grumbach, Y. Y., Quynh, N. V., Verriere, V., Urbach, V. (2008). Lipoxin A(4) and interleukin-8 levels in cystic fibrosis sputum after antibiotherapy. J. Cyst Fibros 7, 463–468. doi: 10.1016/j.jcf.2008.04.002
Chiurchiù, V., Leuti, A., Dalli, J., Jacobsson, A., Battistini, L., Maccarrone, M., et al. (2016). Proresolving lipid mediators resolvin D1, resolvin D2, and maresin 1 are critical in modulating T cell responses. Sci. Transl. Med. 8, 353ra111–353ra111. doi: 10.1126/scitranslmed.aaf7483
Chmiel, J. F., Davis, P. B. (2003). State of the Art: Why do the lungs of patients with cystic fibrosis become infected and why can’t they clear the infection? Respir. Res. 4:8. doi: 10.1186/1465-9921-4-8
Cho, D.-Y., Hwang, P. H., Illek, B., Fischer, H. (2011). Acid and base secretion in freshly excised nasal tissue from cystic fibrosis patients with ΔF508 mutation. Int. Forum Allergy Rhinol. 1, 123–127. doi: 10.1002/alr.20028
Clària, J., Serhan, C. N. (1995). Aspirin triggers previously undescribed bioactive eicosanoids by human endothelial cell-leukocyte interactions. Proc. Natl. Acad. Sci. U. S. A. 92, 9475–9479. doi: 10.1073/pnas.92.21.9475
Coakley, R. D., Grubb, B. R., Paradiso, A. M., Gatzy, J. T., Johnson, L. G., Kreda, S. M., et al. (2003). Abnormal surface liquid pH regulation by cultured cystic fibrosis bronchial epithelium. Proc. Natl. Acad. Sci. 100, 16083–16088. doi: 10.1073/pnas.2634339100
Codagnone, M., Recchiuti, A., Lanuti, P., Pierdomenico, A. M., Cianci, E., Patruno, S., et al. (2017). Lipoxin A4 stimulates endothelial miR-126–5p expression and its transfer via microvesicles. FASEB J. 31, 1856–1866. doi: 10.1096/fj.201600952R
Codagnone, M., Cianci, E., Lamolinara, A., Mari, V. C., Nespoli, A., Isopi, E., et al. (2018). Resolvin D1 enhances the resolution of lung inflammation caused by long-term Pseudomonas aeruginosa infection. Mucosal Immunol. 11, 35–49. doi: 10.1038/mi.2017.36
Colas, R. A., Shinohara, M., Dalli, J., Chiang, N., Serhan, C. N. (2014). Identification and signature profiles for pro-resolving and inflammatory lipid mediators in human tissue. Am. J. Physiol. Cell Physiol. 307, C39–C54. doi: 10.1152/ajpcell.00024.2014
Colas, R. A., Dalli, J., Chiang, N., Vlasakov, I., Sanger, J. M., Riley, I. R., et al. (2016). Identification and Actions of the Maresin 1 Metabolome in Infectious Inflammation. J. Immunol. 197, 4444–4452. doi: 10.4049/jimmunol.1600837
Collawn, J. F., Lazrak, A., Bebok, Z., Matalon, S. (2012). The CFTR and ENaC debate: how important is ENaC in CF lung disease? Am. J. Physiol. Lung Cell. Mol. Physiol. 302, L1141–L1146. doi: 10.1152/ajplung.00036.2012
Corvol, H., Blackman, S. M., Boëlle, P.-Y., Gallins, P. J., Pace, R. G., Stonebraker, J. R., et al. (2015). Genome-wide association meta-analysis identifies five modifier loci of lung disease severity in cystic fibrosis. Nat. Commun. 6, 1–8. doi: 10.1038/ncomms9382
Coste, T. C., Armand, M., Lebacq, J., Lebecque, P., Wallemacq, P., Leal, T. (2007). An overview of monitoring and supplementation of omega 3 fatty acids in cystic fibrosis. Clin. Biochem. 40, 511–520. doi: 10.1016/j.clinbiochem.2007.01.002
Cox, R., Phillips, O., Fukumoto, J., Fukumoto, I., Tamarapu Parthasarathy, P., Mandry, M., et al. (2015). Resolvins Decrease Oxidative Stress Mediated Macrophage and Epithelial Cell Interaction through Decreased Cytokine Secretion. PLoS One 10, e0136755. doi: 10.1371/journal.pone.0136755
Croasdell, A., Thatcher, T. H., Kottmann, R. M., Colas, R. A., Dalli, J., Serhan, C. N., et al. (2015). Resolvins attenuate inflammation and promote resolution in cigarette smoke-exposed human macrophages. Am. J. Physiol.-Lung Cell. Mol. Physiol. 309, L888–L901. doi: 10.1152/ajplung.00125.2015
Csóka, B., Selmeczy, Z., Koscsó, B., Németh, Z. H., Pacher, P., Murray, P. J., et al. (2012). Adenosine promotes alternative macrophage activation via A2A and A2B receptors. FASEB J. 26, 376–386. doi: 10.1096/fj.11-190934
Cystic Fibrosis Mutation Database, Available at: http://www.genet.sickkids.on.ca/cftr/ (Accessed March 23, 2020).
Czerska, K., Sobczynska-Tomaszewska, A., Sands, D., Nowakowska, A., Bak, D., Wertheim, K., et al. (2010). Prostaglandin-endoperoxide synthase genes COX1 and COX2 - novel modifiers of disease severity in cystic fibrosis patients. J. Appl. Genet. 51, 323–330. doi: 10.1007/BF03208862
Dalli, J., Serhan, C. N. (2012). Specific lipid mediator signatures of human phagocytes: microparticles stimulate macrophage efferocytosis and pro-resolving mediators. Blood 120, e60–e72. doi: 10.1182/blood-2012-04-423525
Dalli, J., Rosignoli, G., Hayhoe, R. P., Edelman, A., Perretti, M. (2010). CFTR inhibition provokes an inflammatory response associated with an imbalance of the annexin A1 pathway. Am. J. Pathol. 177, 176–186. doi: 10.2353/ajpath.2010.091149
Dalli, J., Consalvo, A. P., Ray, V., Di Filippo, C., D’Amico, M., Mehta, N., et al. (2013a). Proresolving and Tissue-Protective Actions of Annexin A1–Based Cleavage-Resistant Peptides Are Mediated by Formyl Peptide Receptor 2/Lipoxin A4 Receptor. J. Immunol. 190, 6478–6487. doi: 10.4049/jimmunol.1203000
Dalli, J., Consalvo, A. P., Ray, V., Filippo, C. D., D’Amico, M., Mehta, N., et al. (2013b). Proresolving and Tissue-Protective Actions of Annexin A1–Based Cleavage-Resistant Peptides Are Mediated by Formyl Peptide Receptor 2/Lipoxin A4 Receptor. J. Immunol. 190, 6478–6487. doi: 10.4049/jimmunol.1203000
Dalli, J., Chiang, N., Serhan, C. N. (2014). Identification of 14-series sulfido-conjugated mediators that promote resolution of infection and organ protection. Proc. Natl. Acad. Sci. U. S. A. 111, E4753–E4761. doi: 10.1073/pnas.1415006111
Dalli, J., Kraft, B. D., Colas, R. A., Shinohara, M., Fredenburgh, L. E., Hess, D. R., et al. (2015). The Regulation of Proresolving Lipid Mediator Profiles in Baboon Pneumonia by Inhaled Carbon Monoxide. Am. J. Respir. Cell Mol. Biol. 53, 314–325. doi: 10.1165/rcmb.2014-0299OC
Dalli, J., Vlasakov, I., Riley, I. R., Rodriguez, A. R., Spur, B. W., Petasis, N. A., et al. (2016). Maresin conjugates in tissue regeneration biosynthesis enzymes in human macrophages. Proc. Natl. Acad. Sci. U. S. A. 113, 12232–12237. doi: 10.1073/pnas.1607003113
Dalli, J., Colas, R. A., Arnardottir, H., Serhan, C. N. (2017). Vagal regulation of Group 3 innate lymphoid cells and the immunoresolvent PCTR1 controls infection resolution. Immunity 46, 92–105. doi: 10.1016/j.immuni.2016.12.009
Dartt, D. A., Hodges, R. R., Li, D., Shatos, M. A., Lashkari, K., Serhan, C. N. (2011). Conjunctival Goblet Cell Secretion Stimulated by Leukotrienes Is Reduced by Resolvins D1 and E1 To Promote Resolution of Inflammation. J. Immunol. Baltim. Md 1950 186, 4455–4466. doi: 10.4049/jimmunol.1000833
Davis, P. B. (2006). Cystic fibrosis since 1938. Am. J. Respir. Crit. Care Med. 173, 475–482. doi: 10.1164/rccm.200505-840OE
Denning, G. M., Railsback, M. A., Rasmussen, G. T., Cox, C. D., Britigan, B. E. (1998). Pseudomonas pyocyanine alters calcium signaling in human airway epithelial cells. Am. J. Physiol.-Lung Cell. Mol. Physiol. 274, L893–L900. doi: 10.1152/ajplung.1998.274.6.L893
Derichs, N., Jin, B., Song, Y., Finkbeiner, W. E., Verkman, A. S. (2011). Hyperviscous airway periciliary and mucous liquid layers in cystic fibrosis measured by confocal fluorescence photobleaching. FASEB J. 25, 2325–2332. doi: 10.1096/fj.10-179549
Deriy, L. V., Gomez, E. A., Zhang, G., Beacham, D. W., Hopson, J. A., Gallan, A. J., et al. (2009). Disease-causing Mutations in the Cystic Fibrosis Transmembrane Conductance Regulator Determine the Functional Responses of Alveolar Macrophages. J. Biol. Chem. 284, 35926–35938. doi: 10.1074/jbc.M109.057372
Di, A., Brown, M. E., Deriy, L. V., Li, C., Szeto, F. L., Chen, Y., et al. (2006). CFTR regulates phagosome acidification in macrophages and alters bactericidal activity. Nat. Cell Biol. 8, 933–944.
Dif, F., Wu, Y.-Z., Burgel, P.-R., Ollero, M., Leduc, D., Aarbiou, J., et al. (2010). Critical role of cytosolic phospholipase A2{alpha} in bronchial mucus hypersecretion in CFTR-deficient mice. Eur. Respir. J. 36, 1120–1130. doi: 10.1183/09031936.00183409
Dong, J., Jiang, X., Zhang, X., Liu, K. S., Zhang, J., Chen, J., et al. (2015). Dynamically Regulated CFTR Expression and Its Functional Role in Cutaneous Wound Healing: CFTR IN CUTANEOUS WOUND HEALING. J. Cell. Physiol. 230, 2049–2058. doi: 10.1002/jcp.24931
D’Acquisto, F., Perretti, M., Flower, R. J. (2008). Annexin-A1: a pivotal regulator of the innate and adaptive immune systems. Br. J. Pharmacol. 155, 152–169. doi: 10.1038/bjp.2008.252
Eickmeier, O., Seki, H., Haworth, O., Hilberath, J. N., Gao, F., Uddin, M., et al. (2013). Aspirin-triggered resolvin D1 reduces mucosal inflammation and promotes resolution in a murine model of acute lung injury. Mucosal Immunol. 6, 256–266. doi: 10.1038/mi.2012.66
Eickmeier, O., Fussbroich, D., Mueller, K., Serve, F., Smaczny, C., Zielen, S., et al. (2017). Pro-resolving lipid mediator Resolvin D1 serves as a marker of lung disease in cystic fibrosis. PLoS One 12, e0171249. doi: 10.1371/journal.pone.0171249
Elborn, J., Horsley, A., MacGregor, G., Bilton, D., Grosswald, R., Ahuja, S., et al. (2017). Phase I Studies of Acebilustat: Biomarker Response and Safety in Patients with Cystic Fibrosis: Acebilustat Phase I Biomarkers in Patients with Cystic Fibrosis. Clin. Transl. Sci. 10, 28–34. doi: 10.1111/cts.12428
Elborn, J. S., Ahuja, S., Springman, E., Mershon, J., Grosswald, R., Rowe, S. M. (2018). EMPIRE-CF: A phase II randomized placebo-controlled trial of once-daily, oral acebilustat in adult patients with cystic fibrosis – Study design and patient demographics. Contemp. Clin. Trials 72, 86–94. doi: 10.1016/j.cct.2018.07.014
Emerson, J., Rosenfeld, M., McNamara, S., Ramsey, B., Gibson, R. L. (2002). Pseudomonas aeruginosa and other predictors of mortality and morbidity in young children with cystic fibrosis. Pediatr. Pulmonol. 34, 91–100. doi: 10.1002/ppul.10127
English, J. T., Norris, P. C., Hodges, R. R., Dartt, D. A., Serhan, C. N. (2017). Identification and Profiling of Specialized Pro-Resolving Mediators in Human Tears by Lipid Mediator Metabolomics. Prostaglandins Leukot Essent Fat. Acids 117, 17–27. doi: 10.1016/j.plefa.2017.01.004
Esposito, L. A., Melov, S., Panov, A., Cottrell, B. A., Wallace, D. C. (1999). Mitochondrial disease in mouse results in increased oxidative stress. Proc. Natl. Acad. Sci. 96, 4820–4825. doi: 10.1073/pnas.96.9.4820
Esther, C. R., Muhlebach, M. S., Ehre, C., Hill, D. B., Wolfgang, M. C., Kesimer, M., et al. (2019). Mucus accumulation in the lungs precedes structural changes and infection in children with cystic fibrosis. Sci. Transl. Med. 11 (486), eaav3488. doi: 10.1126/scitranslmed.aav3488
Farrell, P. M., Bieri, J. G., Fratantoni, J. F., Wood, R. E., di Sant’Agnese, P. A. (1977). The Occurrence and Effects of Human Vitamin E Deficiency. J. Clin. Invest. 60, 233–241. doi: 10.1172/JCI108760
Favia, M., de Bari, L., Bobba, A., Atlante, A. (2019). An Intriguing Involvement of Mitochondria in Cystic Fibrosis. J. Clin. Med. 8:1890. doi: 10.3390/jcm8111890
Feigal, R. J., Tomczyk, M. S., Shapiro, B. L. (1982). The calcium abnormality in cystic fibrosis mitochondria: Relative role of respiration and ATP hydrolysis. Life Sci. 30, 93–98. doi: 10.1016/0024-3205(82)90640-3
Fiore, S., Ryeom, S. W., Weller, P. F., Serhan, C. N. (1992). Lipoxin recognition sites. Specific binding of labeled lipoxin A4 with human neutrophils. J. Biol. Chem. 267, 16168–16176. doi: 10.1084/jem.180.1.253
Fiore, S., Maddox, J. F., Perez, H. D., Serhan, C. N. (1994). Identification of a human cDNA encoding a functional high affinity lipoxin A4 receptor. J. Exp. Med. 180, 253–260.
Fisher, J. T., Tyler, S. R., Zhang, Y., Lee, B. J., Liu, X., Sun, X., et al. (2013). Bioelectric Characterization of Epithelia from Neonatal CFTR Knockout Ferrets. Am. J. Respir. Cell Mol. Biol. 49, 837–844. doi: 10.1165/rcmb.2012-0433OC
Folco, G., Murphy, R. C. (2006). Eicosanoid transcellular biosynthesis: from cell-cell interactions to in vivo tissue responses. Pharmacol. Rev. 58, 375–388. doi: 10.1124/pr.58.3.8
Fredman, G., Li, Y., Dalli, J., Chiang, N., Serhan, C. N. (2012). Self-Limited versus Delayed Resolution of Acute Inflammation: Temporal Regulation of Pro-Resolving Mediators and MicroRNA. Sci. Rep. 2, 639. doi: 10.1038/srep00639
Fredman, G., Ozcan, L., Spolitu, S., Hellmann, J., Spite, M., Backs, J., et al. (2014). Resolvin D1 limits 5-lipoxygenase nuclear localization and leukotriene B4 synthesis by inhibiting a calcium-activated kinase pathway. Proc. Natl. Acad. Sci. U. S. A. 111, 14530–14535. doi: 10.1073/pnas.1410851111
Freedman, S. D., Blanco, P. G., Zaman, M. M., Shea, J. C., Ollero, M., Hopper, I. K., et al. (2004). Association of cystic fibrosis with abnormalities in fatty acid metabolism. N. Engl. J. Med. 350, 560–569. doi: 10.1056/NEJMoa021218
Funk, C. D. (2001). Prostaglandins and Leukotrienes: Advances in Eicosanoid Biology. Science 294, 1871–1875. doi: 10.1126/science.294.5548.1871
Gangemi, S., Luciotti, G., D’Urbano, E., Mallamace, A., Santoro, D., Bellinghieri, G., et al. (2003). Physical exercise increases urinary excretion of lipoxin A4 and related compounds. J. Appl. Physiol. Bethesda Md 1985 94, 2237–2240. doi: 10.1152/japplphysiol.01004.2002
Garnett, J. P., Hickman, E., Burrows, R., Hegyi, P., Tiszlavicz, L., Cuthbert, A. W., et al. (2011). Novel Role for Pendrin in Orchestrating Bicarbonate Secretion in Cystic Fibrosis Transmembrane Conductance Regulator (CFTR)-expressing Airway Serous Cells. J. Biol. Chem. 286, 41069–41082. doi: 10.1074/jbc.M111.266734
Garnett, J. P., Kalsi, K. K., Sobotta, M., Bearham, J., Carr, G., Powell, J., et al. (2016). Hyperglycaemia and Pseudomonas aeruginosa acidify cystic fibrosis airway surface liquid by elevating epithelial monocarboxylate transporter 2 dependent lactate-H+ secretion. Sci. Rep. 6, 37955. doi: 10.1038/srep37955
Gavins, F. N. E., Hughes, E. L., Buss, N. A. P. S., Holloway, P. M., Getting, S. J., Buckingham, J. C. (2012). Leukocyte recruitment in the brain in sepsis: involvement of the annexin 1-FPR2/ALX anti-inflammatory system. FASEB J. 26, 4977–4989. doi: 10.1096/fj.12-205971
Gentzsch, M., Dang, H., Dang, Y., Garcia-Caballero, A., Suchindran, H., Boucher, R. C., et al. (2010). The cystic fibrosis transmembrane conductance regulator impedes proteolytic stimulation of the epithelial Na+ channel. J. Biol. Chem. 285, 32227–32232. doi: 10.1074/jbc.M110.155259
Germolec, D. R., Shipkowski, K. A., Frawley, R. P., Evans, E. (2018). “Markers of Inflammation,” in Immunotoxicity Testing: Methods and Protocols Methods in Molecular Biology. Eds. DeWitt, J. C., Rockwell, C. E., Bowman, C. C. (New York, NY: Springer), 57–79. doi: 10.1007/978-1-4939-8549-4_5
Gewirtz, A. T., McCormick, B., Neish, A. S., Petasis, N. A., Gronert, K., Serhan, C. N., et al. (1998). Pathogen-induced chemokine secretion from model intestinal epithelium is inhibited by lipoxin A4 analogs. J. Clin. Invest. 101, 1860–1869. doi: 10.1172/JCI1339
Ghosh, M., Tucker, D. E., Burchett, S. A., Leslie, C. C. (2006). Properties of the Group IV phospholipase A2 family. Prog. Lipid Res. 45, 487–510. doi: 10.1016/j.plipres.2006.05.003
Gibson, R. A., Teubner, J. K., Haines, K., Cooper, D. M., Davidson, G. P. (1986). Relationships between pulmonary function and plasma fatty acid levels in cystic fibrosis patients. J. Pediatr. Gastroenterol. Nutr. 5, 408–415. doi: 10.1097/00005176-198605000-00013
Godson, C., Mitchell, S., Harvey, K., Petasis, N. A., Hogg, N., Brady, H. R. (2000). Cutting edge: lipoxins rapidly stimulate nonphlogistic phagocytosis of apoptotic neutrophils by monocyte-derived macrophages. J. Immunol. Baltim. Md 1950 164, 1663–1667. doi: 10.4049/jimmunol.164.4.1663
Gomez, C. C. S., Parazzi, P. L. F., Clinckspoor, K. J., Mauch, R. M., Pessine, F. B. T., Levy, C. E., et al. (2020). Safety, Tolerability, and Effects of Sodium Bicarbonate Inhalation in Cystic Fibrosis. Clin. Drug Investig. 40, 105–117. doi: 10.1007/s40261-019-00861-x
Gorrieri, G., Scudieri, P., Caci, E., Schiavon, M., Tomati, V., Sirci, F., et al. (2016). Goblet Cell Hyperplasia Requires High Bicarbonate Transport To Support Mucin Release. Sci. Rep. 6, 36016. doi: 10.1038/srep36016
Grabiec, A. M., Hussell, T. (2016). The role of airway macrophages in apoptotic cell clearance following acute and chronic lung inflammation. Semin. Immunopathol. 38, 409–423. doi: 10.1007/s00281-016-0555-3
Greger, R., Mall, M., Bleich, M., Ecke, D., Warth, R., Riedemann, N., et al. (1996). Regulation of epithelial ion channels by the cystic fibrosis transmembrane conductance regulator. J. Mol. Med. Berl. Ger. 74, 527–534. doi: 10.1007/bf00204979
Grumbach, Y., Quynh, N. V. T., Chiron, R., Urbach, V. (2009). LXA4 stimulates ZO-1 expression and transepithelial electrical resistance in human airway epithelial (16HBE14o-) cells. Am. J. Physiol. Lung Cell. Mol. Physiol. 296, L101–L108. doi: 10.1152/ajplung.00018.2008
Güney, E., Emiralioğlu, N., Cinel, G., Yalçın, E., Doğru, D., Kiper, N., et al. (2019). Nasal nitric oxide levels in primary ciliary dyskinesia, cystic fibrosis and healthy children. Turk. J. Pediatr. 61:20. doi: 10.24953/turkjped.2019.01.004
Hachicha, M., Pouliot, M., Petasis, N. A., Serhan, C. N. (1999). Lipoxin (LX)A4 and aspirin-triggered 15-epi-LXA4 inhibit tumor necrosis factor 1alpha-initiated neutrophil responses and trafficking: regulators of a cytokine-chemokine axis. J. Exp. Med. 189, 1923–1930. doi: 10.1084/jem.189.12.1923
Haeggström, J. Z., Funk, C. D. (2011). Lipoxygenase and leukotriene pathways: biochemistry, biology, and roles in disease. Chem. Rev. 111, 5866–5898. doi: 10.1021/cr200246d
Hanukoglu, I., Hanukoglu, A. (2016). Epithelial sodium channel (ENaC) family: Phylogeny, structure-function, tissue distribution, and associated inherited diseases. Gene 579, 95–132. doi: 10.1016/j.gene.2015.12.061
Hayhoe, R. P. G., Kamal, A. M., Solito, E., Flower, R. J., Cooper, D., Perretti, M. (2006). Annexin 1 and its bioactive peptide inhibit neutrophil-endothelium interactions under flow: indication of distinct receptor involvement. Blood 107, 2123–2130. doi: 10.1182/blood-2005-08-3099
He, R., Sang, H., Ye, R. D. (2003). Serum amyloid A induces IL-8 secretion through a G protein-coupled receptor, FPRL1/LXA4R. Blood 101, 1572–1581. doi: 10.1182/blood-2002-05-1431
Hellmann, J., Tang, Y., Kosuri, M., Bhatnagar, A., Spite, M. (2011). Resolvin D1 decreases adipose tissue macrophage accumulation and improves insulin sensitivity in obese-diabetic mice. FASEB J. 25, 2399–2407. doi: 10.1096/fj.10-178657
Henderson, A. G., Ehre, C., Button, B., Abdullah, L. H., Cai, L.-H., Leigh, M. W., et al. (2014). Cystic fibrosis airway secretions exhibit mucin hyperconcentration and increased osmotic pressure. J. Clin. Invest. 124, 3047–3060. doi: 10.1172/JCI73469
Higgins, G., Buchanan, P., Perriere, M., Al-Alawi, M., Costello, R. W., Verriere, V., et al. (2014). Activation of P2RY11 and ATP release by lipoxin A4 restores the airway surface liquid layer and epithelial repair in cystic fibrosis. Am. J. Respir. Cell Mol. Biol. 51, 178–190. doi: 10.1165/rcmb.2012-0424OC
Higgins, G., Fustero Torre, C., Tyrrell, J., McNally, P., Harvey, B. J., Urbach, V. (2016). Lipoxin A 4 prevents tight junction disruption and delays the colonization of cystic fibrosis bronchial epithelial cells by Pseudomonas aeruginosa. Am. J. Physiol.-Lung Cell. Mol. Physiol. 310, L1053–L1061. doi: 10.1152/ajplung.00368.2015
Hill, D. B., Long, R. F., Kissner, W. J., Atieh, E., Garbarine, I. C., Markovetz, M. R., et al. (2018). Pathological mucus and impaired mucus clearance in cystic fibrosis patients result from increased concentration, not altered pH. Eur. Respir. J. 52, 1801297. doi: 10.1183/13993003.01297-2018
Hodges, R. R., Li, D., Shatos, M. A., Bair, J. A., Lippestad, M., Serhan, C. N., et al. (2017). Lipoxin A4 activates ALX/FPR2 Receptor to Regulate Conjunctival Goblet Cell Secretion. Mucosal Immunol. 10, 46–57. doi: 10.1038/mi.2016.33
Hoegger, M. J., Fischer, A. J., McMenimen, J. D., Ostedgaard, L. S., Tucker, A. J., Awadalla, M. A., et al. (2014). Impaired mucus detachment disrupts mucociliary transport in a piglet model of cystic fibrosis. Science 345, 818–822. doi: 10.1126/science.1255825
Hoyt, J. C., Robbins, R. A. (2001). Macrolide antibiotics and pulmonary inflammation. FEMS Microbiol. Lett. 205, 1–7. doi: 10.1111/j.1574-6968.2001.tb10917.x
Hsiao, H.-M., Thatcher, T. H., Levy, E. P., Fulton, R. A., Owens, K. M., Phipps, R. P., et al. (2014). Resolvin D1 Attenuates Polyinosinic-Polycytidylic Acid–Induced Inflammatory Signaling in Human Airway Epithelial Cells via TAK1. J. Immunol. 193, 4980–4987. doi: 10.4049/jimmunol.1400313
Hu, X., Shen, H., Wang, Y., Zhang, L., Zhao, M. (2019). Aspirin-triggered resolvin D1 alleviates paraquat-induced acute lung injury in mice. Life Sci. 218, 38–46. doi: 10.1016/j.lfs.2018.12.028
Hubbard, V. S., Dunn, G. D., di Sant’Agnese, P. A. (1977). Abnormal fatty-acid composition of plasma-lipids in cystic fibrosis. A primary or a secondary defect? Lancet Lond. Engl. 2, 1302–1304. doi: 10.1016/s0140-6736(77)90359-2
Hudson, V. M. (2001). Rethinking cystic fibrosis pathology: the critical role of abnormal reduced glutathione (GSH) transport caused by CFTR mutation. Free Radic. Biol. Med. 30, 1440–1461. doi: 10.1016/S0891-5849(01)00530-5
Hughes, G. W., Ridley, C., Collins, R., Roseman, A., Ford, R., Thornton, D. J. (2019). The MUC5B mucin polymer is dominated by repeating structural motifs and its topology is regulated by calcium and pH. Sci. Rep. 9, 17350. doi: 10.1038/s41598-019-53768-0
Hybiske, K., Fu, Z., Schwarzer, C., Tseng, J., Do, J., Huang, N., et al. (2007). Effects of cystic fibrosis transmembrane conductance regulator and ΔF508CFTR on inflammatory response, ER stress, and Ca 2+ of airway epithelia. Am. J. Physiol.-Lung Cell. Mol. Physiol. 293, L1250–L1260. doi: 10.1152/ajplung.00231.2007
Illek, B., Yankaskas, J. R., Machen, T. E. (1997). cAMP and genistein stimulate HCO3- conductance through CFTR in human airway epithelia. Am. J. Physiol.-Lung Cell. Mol. Physiol. 272, L752–L761. doi: 10.1152/ajplung.1997.272.4.L752
Immler, R., Simon, S., II, Sperandio, M. (2018). Calcium signalling and related ion channels in neutrophil recruitment and function. Eur. J. Clin. Invest. 48, e12964. doi: 10.1111/eci.12964
Inglis, S. K., Corboz, M. R., Taylor, A. E., Ballard, S. T. (1997). Effect of anion transport inhibition on mucus secretion by airway submucosal glands. Am. J. Physiol.-Lung Cell. Mol. Physiol. 272, L372–L377. doi: 10.1152/ajplung.1997.272.2.L372
Inglis, S. K., Corboz, M. R., Ballard, S. T. (1998). Effect of anion secretion inhibitors on mucin content of airway submucosal gland ducts. Am. J. Physiol.-Lung Cell. Mol. Physiol. 274, L762–L766. doi: 10.1152/ajplung.1998.274.5.L762
Inglis, S. K., Collett, A., McAlroy, H. L., Wilson, S. M., Olver, R. E. (1999). Effect of luminal nucleotides on Cl- secretion and Na+ absorption in distal bronchi. Pflugers Arch. 438, 621–627. doi: 10.1007/s004249900096
Ismailov, I., II, Awayda, M. S., Jovov, B., Berdiev, B. K., Fuller, C. M., Dedman, J. R., et al. (1996). Regulation of epithelial sodium channels by the cystic fibrosis transmembrane conductance regulator. J. Biol. Chem. 271, 4725–4732. doi: 10.1074/jbc.271.9.4725
Isobe, Y., Arita, M., Matsueda, S., Iwamoto, R., Fujihara, T., Nakanishi, H., et al. (2012). Identification and structure determination of novel anti-inflammatory mediator resolvin E3, 17,18-dihydroxyeicosapentaenoic acid. J. Biol. Chem. 287, 10525–10534. doi: 10.1074/jbc.M112.340612
Isopi, E., Mattoscio, D., Codagnone, M., Mari, V. C., Lamolinara, A., Patruno, S., et al. (2020). Resolvin D1 Reduces Lung Infection and Inflammation Activating Resolution in Cystic Fibrosis. Front. Immunol. 11, 581. doi: 10.3389/fimmu.2020.00581
Itani, O. A., Chen, J.-H., Karp, P. H., Ernst, S., Keshavjee, S., Parekh, K., et al. (2011). Human cystic fibrosis airway epithelia have reduced Cl- conductance but not increased Na+ conductance. Proc. Natl. Acad. Sci. U. S. A. 108, 10260–10265. doi: 10.1073/pnas.1106695108
Itokazu, Y., Pagano, R. E., Schroeder, A. S., O’Grady, S. M., Limper, A. H., Marks, D. L. (2014). Reduced GM1 ganglioside in CFTR-deficient human airway cells results in decreased β 1 -integrin signaling and delayed wound repair. Am. J. Physiol.-Cell Physiol. 306, C819–C830. doi: 10.1152/ajpcell.00168.2013
Jarosz-Griffiths, H. H., Scambler, T., Wong, C. H., Lara-Reyna, S., Holbrook, J., Martinon, F., et al. (2020). Different CFTR modulator combinations downregulate inflammation differently in cystic fibrosis. eLife 9, e54556. doi: 10.7554/eLife.54556
Jeanson, L., Guerrera, I. C., Papon, J. F., Chhuon, C., Zadigue, P., Prulière-Escabasse, V., et al. (2014). Proteomic analysis of nasal epithelial cells from cystic fibrosis patients. PLoS One 9, e108671. doi: 10.1371/journal.pone.0108671
Jiajia, D., Mingke, Z., Zenglin, L., Wei, W., Tao, W., Lei, C., et al. (2014). Resolvin-D1 inhibits interleukin-8 and hydrogen peroxide production induced by cigarette smoke extract in 16HBE cells via attenuating NF-κB activation. Chin. Med. J. (Engl.) 127 (3), 511–517. doi: 10.3760/cma.j.issn.0366-6999.20131674
Jozsef, L., Zouki, C., Petasis, N. A., Serhan, C. N., Filep, J. G. (2002). Lipoxin A4 and aspirin-triggered 15-epi-lipoxin A4 inhibit peroxynitrite formation, NF- B and AP-1 activation, and IL-8 gene expression in human leukocytes. Proc. Natl. Acad. Sci. 99, 13266–13271. doi: 10.1073/pnas.202296999
Jungas, T., Motta, I., Duffieux, F., Fanen, P., Stoven, V., Ojcius, D. M. (2002). Glutathione Levels and BAX Activation during Apoptosis Due to Oxidative Stress in Cells Expressing Wild-type and Mutant Cystic Fibrosis Transmembrane Conductance Regulator. J. Biol. Chem. 277, 27912–27918. doi: 10.1074/jbc.M110288200
Kakazu, A., He, J., Kenchegowda, S., Bazan, H. E. P. (2012). Lipoxin A4 inhibits platelet-activating factor inflammatory response and stimulates corneal wound healing of injuries that compromise the stroma. Exp. Eye Res. 103, 9–16. doi: 10.1016/j.exer.2012.07.008
Karp, C. L., Flick, L. M., Park, K. W., Softic, S., Greer, T. M., Keledjian, R., et al. (2004). Defective lipoxin-mediated anti-inflammatory activity in the cystic fibrosis airway. Nat. Immunol. 5, 388–392. doi: 10.1038/ni1056
Karra, L., Haworth, O., Priluck, R., Levy, B. D., Levi-Schaffer, F. (2015). Lipoxin B4; promotes the resolution of allergic inflammation in the upper and lower airways of mice. Mucosal Immunol. 8, 852–862. doi: 10.1038/mi.2014.116
Katz, S., Schöni, M. H., Bridges, M. A. (1984). The calcium hypothesis of Cystic Fibrosis. Cell Calcium 5, 421–440. doi: 10.1016/0143-4160(84)90021-6
Keiser, N. W., Birket, S. E., Evans, I. A., Tyler, S. R., Crooke, A. K., Sun, X., et al. (2015). Defective innate immunity and hyperinflammation in newborn cystic fibrosis transmembrane conductance regulator-knockout ferret lungs. Am. J. Respir. Cell Mol. Biol. 52, 683–694. doi: 10.1165/rcmb.2014-0250OC
Kelly-Aubert, M., Trudel, S., Fritsch, J., Nguyen-Khoa, T., Baudouin-Legros, M., Moriceau, S., et al. (2011). GSH monoethyl ester rescues mitochondrial defects in cystic fibrosis models. Hum. Mol. Genet. 20, 2745–2759. doi: 10.1093/hmg/ddr173
Kenchegowda, S., Bazan, N. G., Bazan, H. E. P. (2011). EGF Stimulates Lipoxin A4 Synthesis and Modulates Repair in Corneal Epithelial Cells through ERK and p38 Activation. Investig. Opthalmol. Vis. Sci. 52, 2240. doi: 10.1167/iovs.10-6199
Kerbiriou, M., Le Drévo, M.-A., Férec, C., Trouvé, P. (2007). Coupling cystic fibrosis to endoplasmic reticulum stress: Differential role of Grp78 and ATF6. Biochim. Biophys. Acta BBA - Mol. Basis Dis. 1772, 1236–1249. doi: 10.1016/j.bbadis.2007.10.004
Kerem, B., Rommens, J. M., Buchanan, J. A., Markiewicz, D., Cox, T. K., Chakravarti, A., et al. (1989). Identification of the cystic fibrosis gene: genetic analysis. Science 245, 1073–1080. doi: 10.1126/science.2570460
Kerem, E., Corey, M., Kerem, B. S., Rommens, J., Markiewicz, D., Levison, H., et al. (1990). The relation between genotype and phenotype in cystic fibrosis–analysis of the most common mutation (delta F508). N. Engl. J. Med. 323, 1517–1522. doi: 10.1056/NEJM199011293232203
Kettle, A. J., Turner, R., Gangell, C. L., Harwood, D. T., Khalilova, I. S., Chapman, A. L., et al. (2014). Oxidation contributes to low glutathione in the airways of children with cystic fibrosis. Eur. Respir. J. 44, 122–129. doi: 10.1183/09031936.00170213
Khan, T. Z., Wagener, J. S., Bost, T., Martinez, J., Accurso, F. J., Riches, D. W. (1995). Early pulmonary inflammation in infants with cystic fibrosis. Am. J. Respir. Crit. Care Med. 151, 1075–1082. doi: 10.1164/ajrccm/151.4.1075
Kim, D., Kim, J., Burghardt, B., Best, L., Steward, M. C. (2014). Role of anion exchangers in Cl– and HCO3- secretion by the human airway epithelial cell line Calu-3. Am. J. Physiol.-Cell Physiol. 307, C208–C219. doi: 10.1152/ajpcell.00083.2014
Knorre, A., Wagner, M., Schaefer, H.-E., Colledge, W. H., Pahl, H. L. (2002). deltaF508-CFTR Causes Constitutive NF-kB Activation through an ER-Overload Response in Cystic Fibrosis Lungs. Biol. Chem. 383, 271–282. doi: 10.1515/BC.2002.029
Knowles, M. R., Boucher, R. C. (2002). Mucus clearance as a primary innate defense mechanism for mammalian airways. J. Clin. Invest. 109, 571–577. doi: 10.1172/JCI15217
König, J., Schreiber, R., Voelcker, T., Mall, M., Kunzelmann, K. (2001). The cystic fibrosis transmembrane conductance regulator (CFTR) inhibits ENaC through an increase in the intracellular Cl– concentration. EMBO Rep. 2, 1047–1051. doi: 10.1093/embo-reports/kve232
Konstan, M. W., Walenga, R. W., Hilliard, K. A., Hilliard, J. B. (1993). Leukotriene B4 markedly elevated in the epithelial lining fluid of patients with cystic fibrosis. Am. Rev. Respir. Dis. 148, 896–901. doi: 10.1164/ajrccm/148.4_Pt_1.896
Konstan, M. W., Morgan, W. J., Butler, S. M., Pasta, D. J., Craib, M. L., Silva, S. J., et al. (2007). Risk factors for rate of decline in forced expiratory volume in one second in children and adolescents with cystic fibrosis. J. Pediatr. 151134–139, 139.e1. doi: 10.1016/j.jpeds.2007.03.006
Kotas, M. E., Medzhitov, R. (2015). Homeostasis, inflammation, and disease susceptibility. Cell 160, 816–827. doi: 10.1016/j.cell.2015.02.010
Krishnamoorthy, S., Recchiuti, A., Chiang, N., Yacoubian, S., Lee, C.-H., Yang, R., et al. (2010). Resolvin D1 binds human phagocytes with evidence for proresolving receptors. Proc. Natl. Acad. Sci. U. S. A. 107, 1660–1665. doi: 10.1073/pnas.0907342107
Krishnamoorthy, N., Burkett, P. R., Dalli, J., Abdulnour, R. E., Colas, R., Ramon, S., et al. (2015). Cutting edge: maresin-1 engages regulatory T cells to limit type 2 innate lymphoid cell activation and promote resolution of lung inflammation. J. Immunol. 194, 863–867. doi: 10.4049/jimmunol.1402534
Kunzelmann, K., Kathöfer, S., Greger, R. (1995). Na+ and Cl- conductances in airway epithelial cells: increased Na+ conductance in cystic fibrosis. Pflugers Arch. 431, 1–9. doi: 10.1007/bf00374371
Kunzelmann, K., Schreiber, R., Nitschke, R., Mall, M. (2000). Control of epithelial Na+ conductance by the cystic fibrosis transmembrane conductance regulator. Pflugers Arch. 440, 193–201. doi: 10.1007/s004240000255
Kunzelmann, K. (2003). ENaC is inhibited by an increase in the intracellular Cl(-) concentration mediated through activation of Cl(-) channels. Pflugers Arch. 445, 504–512. doi: 10.1007/s00424-002-0958-y
Kuo, P. T., Huang, N. N., Bassett, D. R. (1962). The fatty acid composition of the serum chylomicrons and adipose tissue of children with cystic fibrosis of the pancreas. J. Pediatr. 60, 394–403. doi: 10.1016/s0022-3476(62)80065-1
Kushwah, R., Gagnon, S., Sweezey, N. B. (2014). T cell unresponsiveness in a pediatric cystic fibrosis patient: a case report. Allergy Asthma Clin. Immunol. 10:2. doi: 10.1186/1710-1492-10-2
Lance, K. D., Chatterjee, A., Wu, B., Mottola, G., Nuhn, H., Lee, P. P., et al. (2017). Unidirectional and Sustained Delivery of the Pro-Resolving Lipid Mediator Resolvin D1 from a Biodegradable Thin Film Device. J. Biomed. Mater. Res. A 105, 31–41. doi: 10.1002/jbm.a.35861
Lands, L. C., Stanojevic, S. (2013). Oral non-steroidal anti-inflammatory drug therapy for lung disease in cystic fibrosis. Cochrane Database Syst. Rev. 6:CD001505. doi: 10.1002/14651858.CD001505.pub3
Lara-Reyna, S., Scambler, T., Holbrook, J., Wong, C., Jarosz-Griffiths, H. H., Martinon, F., et al. (2019). Metabolic Reprograming of Cystic Fibrosis Macrophages via the IRE1α Arm of the Unfolded Protein Response Results in Exacerbated Inflammation. Front. Immunol. 10, 1789. doi: 10.3389/fimmu.2019.01789
Lazrak, A., Jurkuvenaite, A., Chen, L., Keeling, K. M., Collawn, J. F., Bedwell, D. M., et al. (2011). Enhancement of alveolar epithelial sodium channel activity with decreased cystic fibrosis transmembrane conductance regulator expression in mouse lung. Am. J. Physiol. Lung Cell. Mol. Physiol. 301, L557–L567. doi: 10.1152/ajplung.00094.2011
Lee, H. N., Surh, Y. J. (2013). Resolvin D1-mediated NOX2 inactivation rescues macrophages undertaking efferocytosis from oxidative stress-induced apoptosis. Biochem. Pharmacol. 86, 759–769. doi: 10.1016/j.bcp.2013.07.002
Lee, T. H., Horton, C. E., Kyan-Aung, U., Haskard, D., Crea, A. E., Spur, B. W. (1989). Lipoxin A4 and lipoxin B4 inhibit chemotactic responses of human neutrophils stimulated by leukotriene B4 and N-formyl-L-methionyl-L-leucyl-L-phenylalanine. Clin. Sci. Lond. Engl. 1979 77, 195–203. doi: 10.1042/cs0770195
Lee, T. H., Crea, A. E., Gant, V., Spur, B. W., Marron, B. E., Nicolaou, K. C., et al. (1990). Identification of lipoxin A4 and its relationship to the sulfidopeptide leukotrienes C4, D4, and E4 in the bronchoalveolar lavage fluids obtained from patients with selected pulmonary diseases. Am. Rev. Respir. Dis. 141, 1453–1458. doi: 10.1164/ajrccm/141.6.1453
Lee, H.-N., Kundu, J. K., Cha, Y.-N., Surh, Y.-J. (2013). Resolvin D1 stimulates efferocytosis through p50/p50-mediated suppression of tumor necrosis factor- expression. J. Cell Sci. 126, 4037–4047. doi: 10.1242/jcs.131003
Levy, B. D., Serhan, C. N. (2000). A novel polyisoprenyl phosphate signaling cascade in human neutrophils. Ann. N. Acad. Sci. 905, 69–80. doi: 10.1111/j.1749-6632.2000.tb06539.x
Levy, B. D., Fokin, V. V., Clark, J. M., Wakelam, M. J., Petasis, N. A., Serhan, C. N. (1999). Polyisoprenyl phosphate (PIPP) signaling regulates phospholipase D activity: a “stop” signaling switch for aspirin-triggered lipoxin A4. FASEB J. 13, 903–911. doi: 10.1096/fasebj.13.8.903
Levy, B. D., Clish, C. B., Schmidt, B., Gronert, K., Serhan, C. N. (2001). Lipid mediator class switching during acute inflammation: signals in resolution. Nat. Immunol. 2, 612–619. doi: 10.1038/89759
Levy, B. D., Bonnans, C., Silverman, E. S., Palmer, L. J., Marigowda, G., Israel, E., et al. (2005a). Diminished lipoxin biosynthesis in severe asthma. Am. J. Respir. Crit. Care Med. 172, 824–830. doi: 10.1164/rccm.200410-1413OC
Levy, B. D., Hickey, L., Morris, A. J., Larvie, M., Keledjian, R., Petasis, N. A., et al. (2005b). Novel polyisoprenyl phosphates block phospholipase D and human neutrophil activation in vitro and murine peritoneal inflammation in vivo. Br. J. Pharmacol. 146, 344–351. doi: 10.1038/sj.bjp.0706338
Levy, B. D., Kohli, P., Gotlinger, K., Haworth, O., Hong, S., Kazani, S., et al. (2007). Protectin D1 is generated in asthma and dampens airway inflammation and hyperresponsiveness. J. Immunol. 178, 496–502. doi: 10.4049/jimmunol.178.1.496
Ley, K., Laudanna, C., Cybulsky, M., II, Nourshargh, S. (2007). Getting to the site of inflammation: the leukocyte adhesion cascade updated. Nat. Rev. Immunol. 7, 678–689. doi: 10.1038/nri2156
Li, D., Hodges, R. R., Jiao, J., Carozza, R. B., Shatos, M. A., Chiang, N., et al. (2013). Resolvin D1 and aspirin-triggered resolvin D1 regulate histamine-stimulated conjunctival goblet cell secretion. Mucosal Immunol. 6, 1119–1130. doi: 10.1038/mi.2013.7
Liao, Z., Dong, J., Wu, W., Yang, T., Wang, T., Guo, L., et al. (2012). Resolvin D1 attenuates inflammation in lipopolysaccharide-induced acute lung injury through a process involving the PPARγ/NF-κB pathway. Respir. Res. 13:110. doi: 10.1186/1465-9921-13-110
Lim, S. H., Legere, E.-A., Snider, J., Stagljar, I. (2018). Recent Progress in CFTR Interactome Mapping and Its Importance for Cystic Fibrosis. Front. Pharmacol. 8, 997. doi: 10.3389/fphar.2017.00997
Lin, J. H., Walter, P., Yen, T. S. B. (2008). Endoplasmic Reticulum Stress in Disease Pathogenesis. Annu. Rev. Pathol. Mech. Dis. 3, 399–425. doi: 10.1146/annurev.pathmechdis.3.121806.151434
Linsdell, P., Hanrahan, J. W. (1998). Glutathione permeability of CFTR. Am. J. Physiol.-Cell Physiol. 275, C323–C326. doi: 10.1152/ajpcell.1998.275.1.C323
Linsdell, P. (2017). Architecture and functional properties of the CFTR channel pore. Cell. Mol. Life Sci. CMLS 74, 67–83. doi: 10.1007/s00018-016-2389-5
Lippestad, M., Hodges, R. R., Utheim, T. P., Serhan, C. N., Dartt, D. A. (2017). Resolvin D1 Increases Mucin Secretion in Cultured Rat Conjunctival Goblet Cells via Multiple Signaling Pathways. Investig. Opthalmol. Vis. Sci. 58, 4530. doi: 10.1167/iovs.17-21914
Lippestad, M., Hodges, R. R., Utheim, T. P., Serhan, C. N., Dartt, D. A. (2018). Signaling pathways activated by resolvin E1 to stimulate mucin secretion and increase intracellular Ca 2+ in cultured rat conjunctival goblet cells. Exp. Eye Res. 173, 64–72. doi: 10.1016/j.exer.2018.04.015
Liu, M., Chen, S., Shang, Y., Yao, S. (2018). Aspirin-triggered lipoxin A4 attenuates lipopolysaccharide-induced acute lung injury by inhibiting activation of mitogen-activated protein kinases and NF-κB in mice. Int. J. Clin. Exp. Pathol. 11, 2570–2578.
Lloyd-Still, J. D., Powers, C. A., Hoffman, D. R., Boyd-Trull, K., Lester, L. A., Benisek, D. C., et al. (2006). Bioavailability and safety of a high dose of docosahexaenoic acid triacylglycerol of algal origin in cystic fibrosis patients: a randomized, controlled study. Nutr. Burbank Los Angel. Cty. Calif 22, 36–46. doi: 10.1016/j.nut.2005.05.006
Lopes-Pacheco, M. (2019). CFTR Modulators: The Changing Face of Cystic Fibrosis in the Era of Precision Medicine. Front. Pharmacol. 10, 1662. doi: 10.3389/fphar.2019.01662
MacNee, W. (2001). Oxidative stress and lung inflammation in airways disease. Eur. J. Pharmacol. 429, 195–207. doi: 10.1016/S0014-2999(01)01320-6
Maddox, J. F., Hachicha, M., Takano, T., Petasis, N. A., Fokin, V. V., Serhan, C. N. (1997). Lipoxin A4 stable analogs are potent mimetics that stimulate human monocytes and THP-1 cells via a G-protein-linked lipoxin A4 receptor. J. Biol. Chem. 272, 6972–6978. doi: 10.1074/jbc.272.11.6972
Mall, M., Grubb, B. R., Harkema, J. R., O’Neal, W. K., Boucher, R. C. (2004). Increased airway epithelial Na+ absorption produces cystic fibrosis-like lung disease in mice. Nat. Med. 10, 487–493. doi: 10.1038/nm1028
Marino, C. R., Matovcik, L. M., Gorelick, F. S., Cohn, J. A. (1991). Localization of the cystic fibrosis transmembrane conductance regulator in pancreas. J. Clin. Invest. 88, 712–716. doi: 10.1172/JCI115358
Marson, F. A., de, L., Bertuzzo, C. S., Secolin, R., Ribeiro, A. F., Ribeiro, J. D. (2013). Genetic interaction of GSH metabolic pathway genes in cystic fibrosis. BMC Med. Genet. 14, 60. doi: 10.1186/1471-2350-14-60
Marson, F. A., de, F. A., Bertuzzo, C. S., Ribeiro, A. F., Ribeiro, J. D. (2014). Polymorphisms in the glutathione pathway modulate cystic fibrosis severity: a cross-sectional study. BMC Med. Genet. 15, 27. doi: 10.1186/1471-2350-15-27
Martínez, T. M., Llapur, C. J., Williams, T. H., Coates, C., Gunderman, R., Cohen, M. D., et al. (2005). High-resolution computed tomography imaging of airway disease in infants with cystic fibrosis. Am. J. Respir. Crit. Care Med. 172, 1133–1138. doi: 10.1164/rccm.200412-1665OC
Mas, E., Croft, K. D., Zahra, P., Barden, A., Mori, T. A. (2012). Resolvins D1, D2, and other mediators of self-limited resolution of inflammation in human blood following n-3 fatty acid supplementation. Clin. Chem. 58, 1476–1484. doi: 10.1373/clinchem.2012.190199
Matte, A., Recchiuti, A., Federti, E., Koehl, B., Mintz, T., El Nemer, W., et al. (2019). Resolution of sickle cell disease–associated inflammation and tissue damage with 17R-resolvin D1. Blood 133, 252–265. doi: 10.1182/blood-2018-07-865378
Mattoscio, D., Evangelista, V., De Cristofaro, R., Recchiuti, A., Pandolfi, A., Di Silvestre, S., et al. (2010). Cystic fibrosis transmembrane conductance regulator (CFTR) expression in human platelets: impact on mediators and mechanisms of the inflammatory response. FASEB J. 24, 3970–3980. doi: 10.1096/fj.10-159921
Maurice, N. M., Bedi, B., Yuan, Z., Goldberg, J. B., Koval, M., Hart, C. M., et al. (2019). Pseudomonas aeruginosa Induced Host Epithelial Cell Mitochondrial Dysfunction. Sci. Rep. 9, 11929. doi: 10.1038/s41598-019-47457-1
McKiernan, P. J., Greene, C. M. (2015). MicroRNA Dysregulation in Cystic Fibrosis. Mediators Inflamm. 2015:529642. doi: 10.1155/2015/529642
McShane, D., Davies, J. C., Davies, M. G., Bush, A., Geddes, D. M., Alton, E. W. F. W. (2003). Airway surface pH in subjects with cystic fibrosis. Eur. Respir. J. 21, 37–42. doi: 10.1183/09031936.03.00027603
Mekus, F., Ballmann, M., Bronsveld, I., Bijman, J., Veeze, H., Tümmler, B. (2000). Categories of deltaF508 homozygous cystic fibrosis twin and sibling pairs with distinct phenotypic characteristics. Twin Res. 3, 277–293. doi: 10.1375/136905200320565256
Miele, L., Cordella-Miele, E., Xing, M., Frizzell, R., Mukherjee, A. B. (1997). Cystic fibrosis gene mutation (deltaF508) is associated with an intrinsic abnormality in Ca2+-induced arachidonic acid release by epithelial cells. DNA Cell Biol. 16, 749–759. doi: 10.1089/dna.1997.16.749
Mills, C. D., Kincaid, K., Alt, J. M., Heilman, M. J., Hill, A. M. (2000). M-1/M-2 macrophages and the Th1/Th2 paradigm. J. Immunol. Baltim. Md 1950 164, 6166–6173. doi: 10.4049/jimmunol.164.12.6166
Mirakaj, V., Dalli, J., Granja, T., Rosenberger, P., Serhan, C. N. (2014). Vagus nerve controls resolution and pro-resolving mediators of inflammation. J. Exp. Med. 211, 1037–1048. doi: 10.1084/jem.20132103
Mitchell, S., Thomas, G., Harvey, K., Cottell, D., Reville, K., Berlasconi, G., et al. (2002). Lipoxins, aspirin-triggered epi-lipoxins, lipoxin stable analogues, and the resolution of inflammation: stimulation of macrophage phagocytosis of apoptotic neutrophils in vivo. J. Am. Soc. Nephrol. 13, 2497–2507. doi: 10.1097/01.asn.0000032417.73640.72
Montgomery, S. T., Mall, M. A., Kicic, A., Stick, S. M., AREST, C. F. (2017). Hypoxia and sterile inflammation in cystic fibrosis airways: mechanisms and potential therapies. Eur. Respir. J. 49 (1), 1600903. doi: 10.1183/13993003.00903-2016
Morris, T., Stables, M., Hobbs, A., de Souza, P., Colville-Nash, P., Warner, T., et al. (2009). Effects of low-dose aspirin on acute inflammatory responses in humans. J. Immunol. Baltim. Md 1950 183, 2089–2096. doi: 10.4049/jimmunol.0900477
Motwani, M. P., Bennett, F., Norris, P. C., Maini, A. A., George, M. J., Newson, J., et al. (2018a). Potent Anti-Inflammatory and Pro-Resolving Effects of Anabasum in a Human Model of Self-Resolving Acute Inflammation. Clin. Pharmacol. Ther. 104, 675–686. doi: 10.1002/cpt.980
Motwani, M. P., Colas, R. A., George, M. J., Flint, J. D., Dalli, J., Richard-Loendt, A., et al. (2018b). Pro-resolving mediators promote resolution in a human skin model of UV-killed Escherichia coli-driven acute inflammation. JCI Insight 3 (6), e94463. doi: 10.1172/jci.insight.94463
Muhlebach, M. S., Noah, T. L. (2002). Endotoxin activity and inflammatory markers in the airways of young patients with cystic fibrosis. Am. J. Respir. Crit. Care Med. 165, 911–915. doi: 10.1164/ajrccm.165.7.2107114
Muhlebach, M. S., Stewart, P. W., Leigh, M. W., Noah, T. L. (1999). Quantitation of inflammatory responses to bacteria in young cystic fibrosis and control patients. Am. J. Respir. Crit. Care Med. 160, 186–191. doi: 10.1164/ajrccm.160.1.9808096
Nathan, C., Ding, A. (2010). Nonresolving inflammation. Cell 140, 871–882. doi: 10.1016/j.cell.2010.02.029
Nigam, S., Fiore, S., Luscinskas, F. W., Serhan, C. N. (1990). Lipoxin A4 and lipoxin B4 stimulate the release but not the oxygenation of arachidonic acid in human neutrophils: dissociation between lipid remodeling and adhesion. J. Cell Physiol. 143, 512–523. doi: 10.1002/jcp.1041430316
Nixon, G. M., Armstrong, D. S., Carzino, R., Carlin, J. B., Olinsky, A., Robertson, C. F., et al. (2001). Clinical outcome after early Pseudomonas aeruginosa infection in cystic fibrosis. J. Pediatr. 138, 699–704. doi: 10.1067/mpd.2001.112897
Noah, T. L., Black, H. R., Cheng, P. W., Wood, R. E., Leigh, M. W. (1997). Nasal and bronchoalveolar lavage fluid cytokines in early cystic fibrosis. J. Infect. Dis. 175, 638–647. doi: 10.1093/infdis/175.3.638
Norling, L. V., Headland, S. E., Dalli, J., Arnardottir, H. H., Haworth, O., Jones, H. R., et al. (2016). Proresolving and cartilage-protective actions of resolvin D1 in inflammatory arthritis. JCI Insight 1, e85922. doi: 10.1172/jci.insight.85922
Norris, P. C., Libreros, S., Serhan, C. N. (2019). Resolution metabolomes activated by hypoxic environment. Sci. Adv. 5, eaax4895. doi: 10.1126/sciadv.aax4895
Odusanwo, O., Chinthamani, S., McCall, A., Duffey, M. E., Baker, O. J. (2012). Resolvin D1 prevents TNF-α-mediated disruption of salivary epithelial formation. Am. J. Physiol.-Cell Physiol. 302, C1331–C1345. doi: 10.1152/ajpcell.00207.2011
Oh, S. F., Dona, M., Fredman, G., Krishnamoorthy, S., Irimia, D., Serhan, C. N. (2012). Resolvin E2 formation and impact in inflammation-resolution. J. Immunol. Baltim. Md 1950 188, 4527–4534. doi: 10.4049/jimmunol.1103652
Oliver, C., Watson, H. (2016). Omega-3 fatty acids for cystic fibrosis. Cochrane Database Syst. Rev. 2016 (1), CD002201. doi: 10.1002/14651858.CD002201.pub5
Olsen, M. V., Lyngstadaas, A. V., Bair, J. A., Hodges, R. R., Utheim, T. P., Serhan, C. N., et al. (2020). Maresin 1, a specialized proresolving mediator, stimulates intracellular [Ca2+] and secretion in conjunctival goblet cells. J. Cell. Physiol. 1–14. doi: 10.1002/jcp.29846
Olveira, C., Padilla, A., Dorado, A., Contreras, V., Garcia-Fuentes, E., Rubio-Martin, E., et al. (2017). Inflammation and Oxidation Biomarkers in Patients with Cystic Fibrosis: The Influence of Azithromycin. Eurasian J. Med. 49, 118–123. doi: 10.5152/eurasianjmed.2017.17010
Orr, S. K., Colas, R. A., Dalli, J., Chiang, N., Serhan, C. N. (2015). Proresolving actions of a new resolvin D1 analog mimetic qualifies as an immunoresolvent. Am. J. Physiol. Lung Cell Mol. Physiol. 308, L904–L911. doi: 10.1152/ajplung.00370.2014
Ostedgaard, L. S., Moninger, T. O., McMenimen, J. D., Sawin, N. M., Parker, C. P., Thornell, I. M., et al. (2017). Gel-forming mucins form distinct morphologic structures in airways. Proc. Natl. Acad. Sci. U. S. A. 114, 6842–6847. doi: 10.1073/pnas.1703228114
Owens, J. M., Shroyer, K. R., Kingdom, T. T. (2008). Expression of cyclooxygenase and lipoxygenase enzymes in sinonasal mucosa of patients with cystic fibrosis. Arch. Otolaryngol. Head Neck Surg. 134, 825–831. doi: 10.1001/archotol.134.8.825
Papayianni, A., Serhan, C. N., Brady, H. R. (1996). Lipoxin A4 and B4 inhibit leukotriene-stimulated interactions of human neutrophils and endothelial cells. J. Immunol. 156, 2264–2272.
Park, H. S., Kim, S. R., Lee, Y. C. (2009). Impact of oxidative stress on lung diseases. Respirology 14, 27–38. doi: 10.1111/j.1440-1843.2008.01447.x
Perez-Vilar, J., Boucher, R. C. (2004). Reevaluating gel-forming mucins’ roles in cystic fibrosis lung disease. Free Radic. Biol. Med. 37, 1564–1577. doi: 10.1016/j.freeradbiomed.2004.07.027
Perretti, M., D’Acquisto, F. (2009). Annexin A1 and glucocorticoids as effectors of the resolution of inflammation. Nat. Rev. Immunol. 9, 62–70. doi: 10.1038/nri2470
Perretti, M., Chiang, N., La, M., Fierro, I. M., Marullo, S., Getting, S. J., et al. (2002a). Endogenous lipid- and peptide-derived anti-inflammatory pathways generated with glucocorticoid and aspirin treatment activate the lipoxin A4 receptor. Nat. Med. 8, 1296–1302. doi: 10.1038/nm786
Perretti, M., Chiang, N., La, M., Fierro, I. M., Marullo, S., Getting, S. J., et al. (2002b). Endogenous lipid- and peptide-derived anti-inflammatory pathways generated with glucocorticoid and aspirin treatment activate the lipoxin A4 receptor. Nat. Med. 8, 1296–1302. doi: 10.1038/nm786
Pezzulo, A. A., Tang, X. X., Hoegger, M. J., Abou Alaiwa, M. H., Ramachandran, S., Moninger, T. O., et al. (2012). Reduced airway surface pH impairs bacterial killing in the porcine cystic fibrosis lung. Nature 487, 109–113. doi: 10.1038/nature11130
Philippe, R., Urbach, V. (2018). Specialized Pro-Resolving Lipid Mediators in Cystic Fibrosis. Int. J. Mol. Sci. 19 (10), 2865. doi: 10.3390/ijms19102865
Philippe, R., Antigny, F., Buscaglia, P., Norez, C., Becq, F., Frieden, M., et al. (2015). SERCA and PMCA pumps contribute to the deregulation of Ca2+ homeostasis in human CF epithelial cells. Biochim. Biophys. Acta BBA - Mol. Cell Res. 1853, 892–903. doi: 10.1016/j.bbamcr.2015.01.010
Pierdomenico, A. M., Recchiuti, A., Simiele, F., Codagnone, M., Mari, V. C., Davì, G., et al. (2015). MicroRNA-181b Regulates ALX/FPR2 Receptor Expression and Proresolution Signaling in Human Macrophages. J. Biol. Chem. 290, 3592–3600. doi: 10.1074/jbc.M114.592352
Pierdomenico, A. M., Patruno, S., Codagnone, M., Simiele, F., Mari, V. C., Plebani, R., et al. (2017). microRNA-181b is increased in cystic fibrosis cells and impairs lipoxin A4 receptor-dependent mechanisms of inflammation resolution and antimicrobial defense. Sci. Rep. 7, 13519. doi: 10.1038/s41598-017-14055-y
Pillarisetti, N., Williamson, E., Linnane, B., Skoric, B., Robertson, C. F., Robinson, P., et al. (2011). Infection, inflammation, and lung function decline in infants with cystic fibrosis. Am. J. Respir. Crit. Care Med. 184, 75–81. doi: 10.1164/rccm.201011-1892OC
Pinhal-Enfield, G., Ramanathan, M., Hasko, G., Vogel, S. N., Salzman, A. L., Boons, G.-J., et al. (2003). An Angiogenic Switch in Macrophages Involving Synergy between Toll-Like Receptors 2, 4, 7, and 9 and Adenosine A2A Receptors. Am. J. Pathol. 163, 711–721. doi: 10.1016/S0002-9440(10)63698-X
Pistorius, K., Souza, P. R., De Matteis, R., Austin-Williams, S., Primdahl, K. G., Vik, A., et al. (2018). PDn-3 DPA Pathway Regulates Human Monocyte Differentiation and Macrophage Function. Cell Chem. Biol. 25, 749–760.e9. doi: 10.1016/j.chembiol.2018.04.017
Planagumà, A., Kazani, S., Marigowda, G., Haworth, O., Mariani, T. J., Israel, E., et al. (2008). Airway lipoxin A4 generation and lipoxin A4 receptor expression are decreased in severe asthma. Am. J. Respir. Crit. Care Med. 178, 574–582. doi: 10.1164/rccm.200801-061OC
Pongnimitprasert, N., Hurtado, M., Lamari, F., El Benna, J., Dupuy, C., Fay, M., et al. (2012). Implication of NADPH Oxidases in the Early Inflammation Process Generated by Cystic Fibrosis Cells. ISRN Inflamm. 2012, 1–11. doi: 10.5402/2012/481432
Porto, P. D., Cifani, N., Guarnieri, S., Di Domenico, E. G., Mariggiò, M. A., Spadaro, F., et al. (2011). Dysfunctional CFTR Alters the Bactericidal Activity of Human Macrophages against Pseudomonas aeruginosa. PLoS One 6 (5), e19970. doi: 10.1371/journal.pone.0019970
Posso, S. V., Quesnot, N., Moraes, J. A., Brito-Gitirana, L., Kennedy-Feitosa, E., Barroso, M. V., et al. (2018). AT-RVD1 repairs mouse lung after cigarette smoke-induced emphysema via downregulation of oxidative stress by NRF2/KEAP1 pathway. Int. Immunopharmacol. 56, 330–338. doi: 10.1016/j.intimp.2018.01.045
Poulsen, J. H., Fischer, H., Illek, B., Machen, T. E. (1994). Bicarbonate conductance and pH regulatory capability of cystic fibrosis transmembrane conductance regulator. Proc. Natl. Acad. Sci. 91, 5340–5344. doi: 10.1073/pnas.91.12.5340
Psychogios, N., Hau, D. D., Peng, J., Guo, A. C., Mandal, R., Bouatra, S., et al. (2011). The Human Serum Metabolome. PLoS One 6 (2), e16957. doi: 10.1371/journal.pone.0016957
Quinton, P. M. (2008). Cystic fibrosis: impaired bicarbonate secretion and mucoviscidosis. Lancet 372, 415–417. doi: 10.1016/S0140-6736(08)61162-9
Raetz, C. R. H., Whitfield, C. (2002). Lipopolysaccharide Endotoxins. Annu. Rev. Biochem. 71, 635–700. doi: 10.1146/annurev.biochem.71.110601.135414
Ranganathan, S. C., Hall, G. L., Sly, P. D., Stick, S. M., Douglas, T. A., Australian Respiratory Early Surveillance Team for Cystic Fibrosis (AREST-CF) (2017). Early Lung Disease in Infants and Preschool Children with Cystic Fibrosis. What Have We Learned and What Should We Do about It? Am. J. Respir. Crit. Care Med. 195, 1567–1575. doi: 10.1164/rccm.201606-1107CI
Recchiuti, A., Krishnamoorthy, S., Fredman, G., Chiang, N., Serhan, C. N. (2011). MicroRNAs in resolution of acute inflammation: identification of novel resolvin D1-miRNA circuits. FASEB J. 25, 544–560. doi: 10.1096/fj.10-169599
Recchiuti, A., Codagnone, M., Pierdomenico, A. M., Rossi, C., Mari, V. C., Cianci, E., et al. (2014). Immunoresolving actions of oral resolvin D1 include selective regulation of the transcription machinery in resolution-phase mouse macrophages. FASEB J. 28, 3090–3102. doi: 10.1096/fj.13-248393
Ribeiro, C. M. P., Zhang, G., Lubamba, B. A., Tepper, M. A. (2017). Anabasum Reduces Excessive Inflammatory Responses in Cystic Fibrosis Patient-Derived Lung Macrophages. In: PEDIATRIC PULMONOLOGY (NJ USA: WILEY). Vol. 52, pp. S251–S251.
Ribeiro, C. M. P., Paradiso, A. M., Carew, M. A., Shears, S. B., Boucher, R. C. (2005a). Cystic Fibrosis Airway Epithelial Ca2+i Signaling: The Mechanism For The Larger Agonist-Mediated Ca2+i Signals In Human Cystic Fibrosis Airway Epithelia. J. Biol. Chem. 280, 10202–10209. doi: 10.1074/jbc.M410617200
Ribeiro, C. M. P., Paradiso, A. M., Schwab, U., Perez-Vilar, J., Jones, L., O’Neal, W., et al. (2005b). Chronic Airway Infection/Inflammation Induces a Ca2+i-dependent Hyperinflammatory Response in Human Cystic Fibrosis Airway Epithelia. J. Biol. Chem. 280, 17798–17806. doi: 10.1074/jbc.M410618200
Ribeiro, C. M. P. (2006). The Role of Intracellular Calcium Signals in Inflammatory Responses of Polarised Cystic Fibrosis Human Airway Epithelia. Drugs R. D. 7, 17–31. doi: 10.2165/00126839-200607010-00002
Ringholz, F. C., Buchanan, P. J., Clarke, D. T., Millar, R. G., McDermott, M., Linnane, B., et al. (2014). Reduced 15-lipoxygenase 2 and lipoxin A4/leukotriene B4 ratio in children with cystic fibrosis. Eur. Respir. J. 44, 394–404. doi: 10.1183/09031936.00106013
Ringholz, F. C., Higgins, G., Hatton, A., Sassi, A., Moukachar, A., Fustero-Torre, C., et al. (2018). Resolvin D1 regulates epithelial ion transport and inflammation in cystic fibrosis airways. J. Cyst. Fibros. 17, 607–615. doi: 10.1016/j.jcf.2017.11.017
Riordan, J. R., Rommens, J. M., Kerem, B., Alon, N., Rozmahel, R., Grzelczak, Z., et al. (1989). Identification of the cystic fibrosis gene: cloning and characterization of complementary DNA. Science 245, 1066–1073. doi: 10.1126/science.2475911
Roca-Ferrer, J., Pujols, L., Gartner, S., Moreno, A., Pumarola, F., Mullol, J., et al. (2006). Upregulation of COX-1 and COX-2 in nasal polyps in cystic fibrosis. Thorax 61, 592–596. doi: 10.1136/thx.2004.039842
Rogerio, A. P., Haworth, O., Croze, R., Oh, S. F., Uddin, M., Carlo, T., et al. (2012). Resolvin D1 and aspirin-triggered resolvin D1 promote resolution of allergic airways responses. J. Immunol. 189, 1983–1991. doi: 10.4049/jimmunol.1101665
Romano, M., Maddox, J. F., Serhan, C. N. (1996). Activation of human monocytes and the acute monocytic leukemia cell line (THP-1) by lipoxins involves unique signaling pathways for lipoxin A4 versus lipoxin B4: evidence for differential Ca2+ mobilization. J. Immunol. 157, 2149–2154.
Rommens, J. M., Iannuzzi, M. C., Kerem, B., Drumm, M. L., Melmer, G., Dean, M., et al. (1989). Identification of the cystic fibrosis gene: chromosome walking and jumping. Science 245, 1059–1065. doi: 10.1126/science.2772657
Rosen, B. H., Evans, T., II, Moll, S. R., Gray, J. S., Liang, B., Sun, X., et al. (2018). Infection Is Not Required for Mucoinflammatory Lung Disease in CFTR-Knockout Ferrets. Am. J. Respir. Crit. Care Med. 197, 1308–1318. doi: 10.1164/rccm.201708-1616OC
Rottner, M., Tual-Chalot, S., Mostefai, H. A., Andriantsitohaina, R., Freyssinet, J.-M., Martínez, M. C. (2011). Increased Oxidative Stress Induces Apoptosis in Human Cystic Fibrosis Cells. PLoS One 6, e24880. doi: 10.1371/journal.pone.0024880
Roulet, M., Frascarolo, P., Rappaz, I., Pilet, M. (1997). Essential fatty acid deficiency in well nourished young cystic fibrosis patients. Eur. J. Pediatr. 156, 952–956. doi: 10.1007/s004310050750
Roum, J. H., Buhl, R., McElvaney, N. G., Borok, Z., Crystal, R. G. (1993). Systemic deficiency of glutathione in cystic fibrosis. J. Appl. Physiol. 75, 2419–2424. doi: 10.1152/jappl.1993.75.6.2419
Ruffin, M., Voland, M., Marie, S., Bonora, M., Blanchard, E., Blouquit-Laye, S., et al. (2013). Anoctamin 1 dysregulation alters bronchial epithelial repair in cystic fibrosis. Biochim. Biophys. Acta BBA - Mol. Basis Dis. 1832, 2340–2351. doi: 10.1016/j.bbadis.2013.09.012
Ruffin, M., Roussel, L., Maillé, É., Rousseau, S., Brochiero, E. (2018). Vx-809/Vx-770 treatment reduces inflammatory response to Pseudomonas aeruginosa in primary differentiated cystic fibrosis bronchial epithelial cells. Am. J. Physiol. Lung Cell. Mol. Physiol. 314, L635–L641. doi: 10.1152/ajplung.00198.2017
Ruh, C., Banjade, R., Mandadi, S., Marr, C., Sumon, Z., Crane, J. K. (2017). Immunomodulatory Effects of Antimicrobial Drugs. Immunol. Invest. 46, 847–863. doi: 10.1080/08820139.2017.1373900
Sala, A., Folco, G., Murphy, R. C. (2010). Transcellular biosynthesis of eicosanoids. Pharmacol. Rep. PR 62, 503–510. doi: 10.1016/s1734-1140(10)70306-6
Samuelsson, B., Borgeat, P., Hammarström, S., Murphy, R. C. (1980). Leukotrienes: a new group of biologically active compounds. Adv. Prostaglandin Thromboxane Res. 6, 1–18.
Samuelsson, B., Dahlén, S. E., Lindgren, J. A., Rouzer, C. A., Serhan, C. N. (1987). Leukotrienes and lipoxins: structures, biosynthesis, and biological effects. Science 237, 1171–1176. doi: 10.1126/science.2820055
Sasaki, A., Fukuda, H., Shiida, N., Tanaka, N., Furugen, A., Ogura, J., et al. (2015). Determination of ω-6 and ω-3 PUFA metabolites in human urine samples using UPLC/MS/MS. Anal. Bioanal. Chem. 407, 1625–1639. doi: 10.1007/s00216-014-8412-5
Scannell, M., Flanagan, M. B., deStefani, A., Wynne, K. J., Cagney, G., Godson, C., et al. (2007). Annexin-1 and Peptide Derivatives Are Released by Apoptotic Cells and Stimulate Phagocytosis of Apoptotic Neutrophils by Macrophages. J. Immunol. 178, 4595–4605. doi: 10.4049/jimmunol.178.7.4595
Schett, G., Neurath, M. F. (2018). Resolution of chronic inflammatory disease: universal and tissue-specific concepts. Nat. Commun. 9, 3261. doi: 10.1038/s41467-018-05800-6
Schiller, K. R., Maniak, P. J., O’Grady, S. M. (2010). Cystic fibrosis transmembrane conductance regulator is involved in airway epithelial wound repair. Am. J. Physiol.-Cell Physiol. 299, C912–C921. doi: 10.1152/ajpcell.00215.2010
Schultz, A., Puvvadi, R., Borisov, S. M., Shaw, N. C., Klimant, I., Berry, L. J., et al. (2017). Airway surface liquid pH is not acidic in children with cystic fibrosis. Nat. Commun. 8, 1409. doi: 10.1038/s41467-017-00532-5
Schwab, J. M., Chiang, N., Arita, M., Serhan, C. N. (2007). Resolvin E1 and protectin D1 activate inflammation-resolution programmes. Nature 447, 869–874. doi: 10.1038/nature05877
Schwarzer, C., Illek, B., Suh, J. H., Remington, S. J., Fischer, H., Machen, T. E. (2007). Organelle redox of CF and CFTR-corrected airway epithelia. Free Radic. Biol. Med. 43, 300–316. doi: 10.1016/j.freeradbiomed.2007.04.015
Scudieri, P., Musante, I., Caci, E., Venturini, A., Morelli, P., Walter, C., et al. (2018). Increased expression of ATP12A proton pump in cystic fibrosis airways. JCI Insight 3, e123616. doi: 10.1172/jci.insight.123616
Serhan, C. N., Levy, B. D. (2018). Resolvins in inflammation: emergence of the pro-resolving superfamily of mediators. J. Clin. Invest. 128, 2657–2669. doi: 10.1172/JCI97943
Serhan, C. N., Hamberg, M., Samuelsson, B. (1984). Trihydroxytetraenes: a novel series of compounds formed from arachidonic acid in human leukocytes. Biochem. Biophys. Res. Commun. 118, 943–949. doi: 10.1016/0006-291x(84)91486-4
Serhan, C. N., Maddox, J. F., Petasis, N. A., Akritopoulou-Zanze, I., Papayianni, A., Brady, H. R., et al. (1995). Design of lipoxin A4 stable analogs that block transmigration and adhesion of human neutrophils. Biochemistry 34, 14609–14615. doi: 10.1021/bi00044a041
Serhan, C. N., Arita, M., Hong, S., Gotlinger, K. (2004). Resolvins, docosatrienes, and neuroprotectins, novel omega-3-derived mediators, and their endogenous aspirin-triggered epimers. Lipids 39, 1125–1132. doi: 10.1007/s11745-004-1339-7
Serhan, C. N., Chiang, N., Dalli, J. (2018). New pro-resolving n-3 mediators bridge resolution of infectious inflammation to tissue regeneration. Mol. Aspects Med. 64, 1–17. doi: 10.1016/j.mam.2017.08.002
Shanthikumar, S., Neeland, M. N., Saffery, R., Ranganathan, S. (2019). Gene modifiers of cystic fibrosis lung disease: A systematic review. Pediatr. Pulmonol. 54, 1356–1366. doi: 10.1002/ppul.24366
Shei, R.-J., Peabody, J. E., Rowe, S. M. (2018). Functional Anatomic Imaging of the Airway Surface. Ann. Am. Thorac. Soc 15, S177–S183. doi: 10.1513/AnnalsATS.201806-407AW
Simonin, J., Bille, E., Crambert, G., Noel, S., Dreano, E., Edwards, A., et al. (2019). Airway surface liquid acidification initiates host defense abnormalities in Cystic Fibrosis. Sci. Rep. 9, 6516. doi: 10.1038/s41598-019-42751-4
Six, D. A., Dennis, E. A. (2000). The expanding superfamily of phospholipase A(2) enzymes: classification and characterization. Biochim. Biophys. Acta 1488, 1–19. doi: 10.1016/s1388-1981(00)00105-0
Song, Y., Salinas, D., Nielson, D. W., Verkman, A. S. (2006). Hyperacidity of secreted fluid from submucosal glands in early cystic fibrosis. Am. J. Physiol.-Cell Physiol. 290, C741–C749. doi: 10.1152/ajpcell.00379.2005
Southern, K. W., Barker, P. M. (2004). Azithromycin for cystic fibrosis. Eur. Respir. J. 24, 834–838. doi: 10.1183/09031936.04.00084304
Soyombo, O., Spur, B. W., Lee, T. H. (1994). Effects of lipoxin A4 on chemotaxis and degranulation of human eosinophils stimulated by platelet-activating factor and N-formyl-L-methionyl-L-leucyl-L-phenylalanine. Allergy 49, 230–234. doi: 10.1111/j.1398-9995.1994.tb02654.x
Spite, M., Norling, L. V., Summers, L., Yang, R., Cooper, D., Petasis, N. A., et al. (2009). Resolvin D2 is a potent regulator of leukocytes and controls microbial sepsis. Nature 461, 1287–1291. doi: 10.1038/nature08541
Stanke, F., van Barneveld, A., Hedtfeld, S., Wölfl, S., Becker, T., Tümmler, B. (2014). The CF-modifying gene EHF promotes p.Phe508del-CFTR residual function by altering protein glycosylation and trafficking in epithelial cells. Eur. J. Hum. Genet. 22, 660–666. doi: 10.1038/ejhg.2013.209
Stanton, B. A., Coutermarsh, B., Barnaby, R., Hogan, D. (2015). Pseudomonas aeruginosa Reduces VX-809 Stimulated F508del-CFTR Chloride Secretion by Airway Epithelial Cells. PLoS One 10 (5), e0127742. doi: 10.1371/journal.pone.0127742
Starosta, V., Ratjen, F., Rietschel, E., Paul, K., Griese, M. (2006). Anti-inflammatory cytokines in cystic fibrosis lung disease. Eur. Respir. J. 28, 581–587. doi: 10.1183/09031936.06.00071405
Stick, S. M., Brennan, S., Murray, C., Douglas, T., von Ungern-Sternberg, B. S., Garratt, L. W., et al. (2009). Bronchiectasis in infants and preschool children diagnosed with cystic fibrosis after newborn screening. J. Pediatr. 155, 623–628.e1. doi: 10.1016/j.jpeds.2009.05.005
Strandvik, B., Gronowitz, E., Enlund, F., Martinsson, T., Wahlström, J. (2001). Essential fatty acid deficiency in relation to genotype in patients with cystic fibrosis. J. Pediatr. 139, 650–655. doi: 10.1067/mpd.2001.118890
Stutts, M. J., Canessa, C. M., Olsen, J. C., Hamrick, M., Cohn, J. A., Rossier, B. C., et al. (1995). CFTR as a cAMP-dependent regulator of sodium channels. Science 269, 847–850. doi: 10.1126/science.7543698
Sun, Y.-P., Oh, S. F., Uddin, J., Yang, R., Gotlinger, K., Campbell, E., et al. (2007). Resolvin D1 and its aspirin-triggered 17R epimer. Stereochemical assignments, anti-inflammatory properties, and enzymatic inactivation. J. Biol. Chem. 282, 9323–9334. doi: 10.1074/jbc.M609212200
Sun, X., Olivier, A. K., Liang, B., Yi, Y., Sui, H., Evans, T., II, et al. (2014). Lung Phenotype of Juvenile and Adult Cystic Fibrosis Transmembrane Conductance Regulator–Knockout Ferrets. Am. J. Respir. Cell Mol. Biol. 50, 502–512. doi: 10.1165/rcmb.2013-0261OC
Takamiya, R., Fukunaga, K., Arita, M., Miyata, J., Seki, H., Minematsu, N., et al. (2012). Resolvin E1 maintains macrophage function under cigarette smoke-induced oxidative stress. FEBS Open Bio 2, 328–333. doi: 10.1016/j.fob.2012.10.001
Taminelli, G. L., Sotomayor, V., Valdivieso, Á.G., Teiber, M. L., Marín, M. C., Santa-Coloma, T. A. (2008). CISD1 codifies a mitochondrial protein upregulated by the CFTR channel. Biochem. Biophys. Res. Commun. 365, 856–862. doi: 10.1016/j.bbrc.2007.11.076
Tarique, A. A., Sly, P. D., Holt, P. G., Bosco, A., Ware, R. S., Logan, J., et al. (2017). CFTR-dependent defect in alternatively-activated macrophages in cystic fibrosis. J. Cyst. Fibros. 16, 475–482. doi: 10.1016/j.jcf.2017.03.011
Tarran, R., Grubb, B. R., Gatzy, J. T., Davis, C. W., Boucher, R. C. (2001). The relative roles of passive surface forces and active ion transport in the modulation of airway surface liquid volume and composition. J. Gen. Physiol. 118, 223–236. doi: 10.1085/jgp.118.2.223
Tarran, R. (2004). Regulation of Airway Surface Liquid Volume and Mucus Transport by Active Ion Transport. Proc. Am. Thorac. Soc. 1, 42–46. doi: 10.1513/pats.2306014
Teopompi, E., Risé, P., Pisi, R., Buccellati, C., Aiello, M., Pisi, G., et al. (2019). Arachidonic Acid and Docosahexaenoic Acid Metabolites in the Airways of Adults With Cystic Fibrosis: Effect of Docosahexaenoic Acid Supplementation. Front. Pharmacol. 10, 938. doi: 10.3389/fphar.2019.00938
Thompson, G. N. (1989). Relationships between essential fatty acid levels, pulmonary function and fat absorption in pre-adolescent cystic fibrosis children with good clinical scores. Eur. J. Pediatr. 148, 327–329. doi: 10.1007/bf00444126
Tirouvanziam, R., de Bentzmann, S., Hubeau, C., Hinnrasky, J., Jacquot, J., Péault, B., et al. (2000). Inflammation and infection in naive human cystic fibrosis airway grafts. Am. J. Respir. Cell Mol. Biol. 23, 121–127. doi: 10.1165/ajrcmb.23.2.4214
Tjonahen, E., Oh, S. F., Siegelman, J., Elangovan, S., Percarpio, K. B., Hong, S., et al. (2006). Resolvin E2: identification and anti-inflammatory actions: pivotal role of human 5-lipoxygenase in resolvin E series biosynthesis. Chem. Biol. 13, 1193–1202. doi: 10.1016/j.chembiol.2006.09.011
Trinh, N. T. N., Privé, A., Maillé, E., Noël, J., Brochiero, E. (2008). EGF and K+ channel activity control normal and cystic fibrosis bronchial epithelia repair. Am. J. Physiol.-Lung Cell. Mol. Physiol. 295, L866–L880. doi: 10.1152/ajplung.90224.2008
Trinh, N. T. N., Bardou, O., Privé, A., Maillé, E., Adam, D., Lingée, S., et al. (2012). Improvement of defective cystic fibrosis airway epithelial wound repair after CFTR rescue. Eur. Respir. J. 40, 1390–1400. doi: 10.1183/09031936.00221711
Tuggle, K. L., Birket, S. E., Cui, X., Hong, J., Warren, J., Reid, L., et al. (2014). Characterization of defects in ion transport and tissue development in cystic fibrosis transmembrane conductance regulator (CFTR)-knockout rats. PLoS One 9, e91253. doi: 10.1371/journal.pone.0091253
Tugores, A., Le, J., Sorokina, I., Snijders, A. J., Duyao, M., Reddy, P. S., et al. (2001). The epithelium-specific ETS protein EHF/ESE-3 is a context-dependent transcriptional repressor downstream of MAPK signaling cascades. J. Biol. Chem. 276, 20397–20406. doi: 10.1074/jbc.M010930200
Uluçkan, Ö., Wagner, E. F. (2017). Chronic systemic inflammation originating from epithelial tissues. FEBS J. 284, 505–516. doi: 10.1111/febs.13904
Underwood, B. A., Denning, C. R., Navab, M. (1972). Polyunsaturated fatty acids and tocopherol levels in patients with cystic fibrosis. Ann. N. Y. Acad. Sci. 203, 237–247. doi: 10.1111/j.1749-6632.1972.tb27879.x
Vachier, I., Bonnans, C., Chavis, C., Farce, M., Godard, P., Bousquet, J., et al. (2005). Severe asthma is associated with a loss of LX4, an endogenous anti-inflammatory compound. J. Allergy Clin. Immunol. 115, 55–60. doi: 10.1016/j.jaci.2004.09.038
Valdivieso, Á.G., Marcucci, F., Taminelli, G., Guerrico, A. G., Álvarez, S., Teiber, M. L., et al. (2007). The expression of the mitochondrial gene MT-ND4 is downregulated in cystic fibrosis. Biochem. Biophys. Res. Commun. 356, 805–809. doi: 10.1016/j.bbrc.2007.03.057
Valdivieso, A. G., Clauzure, M., Marín, M. C., Taminelli, G. L., Massip Copiz, M. M., Sánchez, F., et al. (2012). The Mitochondrial Complex I Activity Is Reduced in Cells with Impaired Cystic Fibrosis Transmembrane Conductance Regulator (CFTR) Function. PLoS One 7, e48059. doi: 10.1371/journal.pone.0048059
Van Dyke, T. E., Hasturk, H., Kantarci, A., Freire, M. O., Nguyen, D., Dalli, J., et al. (2015). Proresolving Nanomedicines Activate Bone Regeneration in Periodontitis. J. Dent. Res. 94, 148–156. doi: 10.1177/0022034514557331
Vass, G., Horvath, I. (2008). Adenosine and Adenosine Receptors in the Pathomechanism and Treatment of Respiratory Diseases. Curr. Med. Chem. 15, 917–922. doi: 10.2174/092986708783955392
Velsor, L. W., Kariya, C., Kachadourian, R., Day, B. J. (2006). Mitochondrial Oxidative Stress in the Lungs of Cystic Fibrosis Transmembrane Conductance Regulator Protein Mutant Mice. Am. J. Respir. Cell Mol. Biol. 35, 579–586. doi: 10.1165/rcmb.2005-0473OC
Verrière, V., Higgins, G., Al-Alawi, M., Costello, R. W., McNally, P., Chiron, R., et al. (2012). Lipoxin A4 Stimulates Calcium-Activated Chloride Currents and Increases Airway Surface Liquid Height in Normal and Cystic Fibrosis Airway Epithelia. PLoS One 7, e37746. doi: 10.1371/journal.pone.0037746
Viart, V., Bergougnoux, A., Bonini, J., Varilh, J., Chiron, R., Tabary, O., et al. (2015). Transcription factors and miRNAs that regulate fetal to adult CFTR expression change are new targets for cystic fibrosis. Eur. Respir. J. 45, 116–128. doi: 10.1183/09031936.00113214
Volkova, N., Moy, K., Evans, J., Campbell, D., Tian, S., Simard, C., et al. (2020). Disease progression in patients with cystic fibrosis treated with ivacaftor: Data from national US and UK registries. J. Cyst. Fibros. Off. J. Eur. Cyst. Fibros. Soc 19, 68–79. doi: 10.1016/j.jcf.2019.05.015
Von Ruecker, A. A., Bertele, R., Karsten Harms, H. (1984). Calcium Metabolism and Cystic Fibrosis: Mitochondrial Abnormalities Suggest a Modification of the Mitochondrial Membrane. Pediatr. Res. 18, 594–599. doi: 10.1203/00006450-198407000-00005
Wallace, J. L., Ianaro, A., Flannigan, K. L., Cirino, G. (2015). Gaseous mediators in resolution of inflammation. Semin. Immunol. 27, 227–233. doi: 10.1016/j.smim.2015.05.004
Wang, L., Ruixia, Y., Chengyue, Y., Qingping, W., Christelle, M., Wanli, X., et al. (2014). Effects of resolvin D1 on inflammatory responses and oxidative stress of lipopolysaccharide-induced acute lung injury in mice. Chin. Med. J. (Engl.) 127 (5), 803–809. doi: 10.3760/cma.j.issn.0366-6999.20131044
Wang, Q., Zheng, X., Cheng, Y., Zhang, Y.-L., Wen, H.-X., Tao, Z., et al. (2014). Resolvin D1 Stimulates Alveolar Fluid Clearance through Alveolar Epithelial Sodium Channel, Na,K-ATPase via ALX/cAMP/PI3K Pathway in Lipopolysaccharide-Induced Acute Lung Injury. J. Immunol. 192, 3765–3777. doi: 10.4049/jimmunol.1302421
Wang, H., Anthony, D., Yatmaz, S., Wijburg, O., Satzke, C., Levy, B., et al. (2017). Aspirin-triggered resolvin D1 reduces pneumococcal lung infection and inflammation in a viral and bacterial coinfection pneumonia model. Clin. Sci. Lond. Engl. 1979 131, 2347–2362. doi: 10.1042/CS20171006
Weber, A. J., Soong, G., Bryan, R., Saba, S., Prince, A. (2001). Activation of NF-κB in airway epithelial cells is dependent on CFTR trafficking and Cl – channel function. Am. J. Physiol.-Lung Cell. Mol. Physiol. 281, L71–L78. doi: 10.1152/ajplung.2001.281.1.L71
Weiss, G. A., Troxler, H., Klinke, G., Rogler, D., Braegger, C., Hersberger, M. (2013). High levels of anti-inflammatory and pro-resolving lipid mediators lipoxins and resolvins and declining docosahexaenoic acid levels in human milk during the first month of lactation. Lipids Health Dis. 12, 89. doi: 10.1186/1476-511X-12-89
Wheelock, C. E., Strandvik, B. (2020). Abnormal n-6 fatty acid metabolism in cystic fibrosis contributes to pulmonary symptoms. Prostaglandins Leukot. Essent. Fatty Acids 160, 102156. doi: 10.1016/j.plefa.2020.102156
Wood, L. G., Fitzgerald, D. A., Gibson, P. G., Cooper, D. M., Garg, M. L. (2002). Increased plasma fatty acid concentrations after respiratory exacerbations are associated with elevated oxidative stress in cystic fibrosis patients. Am. J. Clin. Nutr. 75, 668–675. doi: 10.1093/ajcn/75.4.668
Wright, F. A., Strug, L. J., Doshi, V. K., Commander, C. W., Blackman, S. M., Sun, L., et al. (2011). Genome-wide association and linkage identify modifier loci of lung disease severity in cystic fibrosis at 11p13 and 20q13.2. Nat. Genet. 43, 539–546. doi: 10.1038/ng.838
Xu, K.-P., Li, Y., Ljubimov, A. V., Yu, F.-S. X. (2009). High Glucose Suppresses Epidermal Growth Factor Receptor/Phosphatidylinositol 3-Kinase/Akt Signaling Pathway and Attenuates Corneal Epithelial Wound Healing. Diabetes 58, 1077–1085. doi: 10.2337/db08-0997
Yang, Y., Cheng, Y., Lian, Q.-Q., Yang, L., Qi, W., Wu, D.-R., et al. (2013). Contribution of CFTR to Alveolar Fluid Clearance by Lipoxin A 4 via PI3K/Akt Pathway in LPS-Induced Acute Lung Injury. Mediators Inflamm. 2013, 1–10. doi: 10.1155/2013/862628
Zhang, F., Yang, H., Pan, Z., Wang, Z., Wolosin, J. M., Gjorstrup, P., et al. (2010). Dependence of Resolvin-Induced Increases in Corneal Epithelial Cell Migration on EGF Receptor Transactivation. Investig. Opthalmol. Vis. Sci. 51, 5601. doi: 10.1167/iovs.09-4468
Zhang, J.-L., Zhuo, X.-J., Lin, J., Luo, L.-C., Ying, W.-Y., Xie, X., et al. (2017). Maresin1 stimulates alveolar fluid clearance through the alveolar epithelial sodium channel Na,K-ATPase via the ALX/PI3K/Nedd4-2 pathway. Lab. Invest. 97, 543–554. doi: 10.1038/labinvest.2016.150
Zhang, Z., Hu, X., Qi, X., Di, G., Zhang, Y., Wang, Q., et al. (2018). Resolvin D1 promotes corneal epithelial wound healing and restoration of mechanical sensation in diabetic mice. Mol. Vis. 24, 274.
Zheng, S., Wang, Q., D’Souza, V., Bartis, D., Dancer, R., Parekh, D., et al. (2018). ResolvinD1 stimulates epithelial wound repair and inhibits TGF-β-induced EMT whilst reducing fibroproliferation and collagen production. Lab. Invest. 98, 130–140. doi: 10.1038/labinvest.2017.114
Keywords: cystic fibrosis, CF airway inflammation, specialized pro-resolving lipid mediators, bioactive lipids, lipoxin, resolvin
Citation: Briottet M, Shum M and Urbach V (2020) The Role of Specialized Pro-Resolving Mediators in Cystic Fibrosis Airways Disease. Front. Pharmacol. 11:1290. doi: 10.3389/fphar.2020.01290
Received: 30 April 2020; Accepted: 04 August 2020;
Published: 02 September 2020.
Edited by:
Noel Gerard McElvaney, Royal College of Surgeons in Ireland, IrelandReviewed by:
Pallavi R. Devchand, University of Calgary, CanadaPatricia Machado Rodrigues Silva, Oswaldo Cruz Foundation (Fiocruz), Brazil
Copyright © 2020 Briottet, Shum and Urbach. This is an open-access article distributed under the terms of the Creative Commons Attribution License (CC BY). The use, distribution or reproduction in other forums is permitted, provided the original author(s) and the copyright owner(s) are credited and that the original publication in this journal is cited, in accordance with accepted academic practice. No use, distribution or reproduction is permitted which does not comply with these terms.
*Correspondence: Valerie Urbach, valerie.urbach@inserm.fr