- 1Department of Radiation Oncology, Massachusetts General Hospital, Boston MA, United States
- 2Harvard Medical School, Boston MA, United States
It is well known that radiation therapy causes lymphopenia in patients and that this is correlated with a negative outcome. The mechanism is not well understood because radiation can have both immunostimulatory and immunosuppressive effects. How tumor dose conformation, dose fractionation, and selective lymph node irradiation in radiation therapy does affect lymphopenia and immune response is an active area of research. In addition, understanding the impact of radiation on the immune system is important for the design and interpretation of clinical trials combining radiation with immune checkpoint inhibitors, both in terms of radiation dose and treatment schedules. Although only a few percent of the total lymphocyte population are circulating, it has been speculated that their increased radiosensitivity may contribute to, or even be the primary cause of, lymphopenia. This review summarizes published data on lymphocyte radiosensitivity based on human, small animal, and in vitro studies. The data indicate differences in radiosensitivity among lymphocyte subpopulations that affect their relative contribution and thus the dynamics of the immune response. In general, B cells appear to be more radiosensitive than T cells and NK cells appear to be the most resistant. However, the reported dose-response data suggest that in the context of lymphopenia in patients, aspects other than cell death must also be considered. Not only absolute lymphocyte counts, but also lymphocyte diversity and activity are likely to be affected by radiation. Taken together, the reviewed data suggest that it is unlikely that radiation-induced cell death in lymphocytes is the sole factor in radiation-induced lymphopenia.
1 Introduction
Radiation-induced lymphopenia (RIL) has long been observed in radiation therapy patients (1–3) and develops in up to ~70% of patients undergoing external beam radiation therapy (4–8). High-grade RIL has been shown to correlate with poor overall survival, disease recurrence, and metastasis rates (9). A correlation between lymphopenia and dose to circulating lymphocytes has been demonstrated (e.g., (6, 8, 10–13). Therefore, it has been speculated that lymphopenia is caused by an increased radiosensitivity of circulating lymphocytes (7) and the large volume of blood irradiated during radiotherapy.
Treatment delivery techniques differ in the distribution of the low dose bath outside of the planned treatment volume and in the duration of treatment in a fraction (10, 14, 15) resulting in different dose distributions experienced by circulating lymphocytes (6, 16–19). In a study of esophageal cancer, 35% of patients had grade 4 RIL when treated with concurrent chemotherapy and either intensity-modulated photon (IMRT) or proton therapy, which was correlated with overall survival (20). Due to the lower integral dose, patients treated with protons had 70% less grade 4 RIL compared to IMRT. However, this was not confirmed in a study of 150 oropharyngeal cancer patients (21) and in locally advanced non-small cell lung cancer (NSCLC) treated with either IMRT or proton therapy (15). Dose to lymphocytes is also influenced by patient specific factors such as baseline levels of absolute counts and lymphocyte subpopulations, which are known to differ between patient groups (22), as well as fractionation and dose rate (18, 23–27). Consequently, lymphocyte sparing radiation therapy has been proposed (8, 28). Smaller target volumes and hypo-fractionated regimens may be associated with higher post-treatment lymphocyte counts. For example, during a 30-fraction treatment with 2 Gy/fraction to a target volume of 8 cm in diameter, 95% of the circulating blood receives doses greater than 0.5 Gy, with a mean dose to the circulating blood greater than 2 Gy (8). Larger field sizes increased chromosomal aberrations in circulating lymphocytes in a prospective series of lung cancer patients treated with carbon-ion therapy (29) and were associated with lower post-treatment lymphocyte counts in lung cancer treated with protons (15). There have been several other studies of field size effects on lymphopenia in solid tumors (5, 30, 31).
Although the amount of circulating blood plays a role, considering that only a few percent of the total lymphocyte population is circulating, compared to those residing in organs or lymph nodes, is not clear whether RIL is simply caused by radiation-induced depletion of circulating lymphocytes. Radiation has deleterious effects not only on circulating lymphocytes but also on tumor-infiltrating lymphocytes and lymphocytes residing in structures such as the bone marrow (32), spleen (33), and lymph nodes (34). Lymphopenia has been shown to correlate strongly with dose to the spleen (33, 35–37). The capillaries in the spleen are permeable, resulting in high transit times for lymphocytes in the spleen, which in turn results in significant dose to lymphocytes in treatments involving the spleen. By assessing chromosome aberrations in lymphocytes in breast cancer patients, it has been shown that the number of lymph nodes in the field plays a significant role (34). A correlation with bone marrow dose has also been shown by several investigators (32, 38–41), but not by Saito et al. (36). Lymphopenia has also been associated with lymph node irradiation in prostate radiation therapy (42) and breast radiation therapy (43). Consequently, dose constraints to lymphoid organs have been proposed to mitigate lymphopenia (44). Reduced total counts as well as counts in lymphocyte sub-populations were reported for colorectal cancer patients (45) and liver SBRT patients (46).
The interaction of radiation with the immune system is complex (47). Radiation therapy can have both immune-stimulatory (18, 48–52) and immune-suppressive (5) effects. Radiation can promote the release of damage-associated molecular patterns (DAMPs) and tumor antigens via immunogenic cell death, activate the production of type I interferon (IFN) and IFN-stimulated genes via DNA damage that is sensed via the cGAS/STING pathway, and activate antigen-presenting cells, including dendritic cells (DCs) and macrophages (53). Antigen-presenting cells travel through lymphatic vessels to the draining lymph nodes (for instance) where they present antigens to naïve lymphocytes initiating their differentiation into effector and memory cells. Activated lymphocytes returnvia the blood to the tumor site where they recognize tumor antigens and carry out various effector functions. Radiation can also suppress the immune response via IFN-mediated upregulation of immune checkpoint molecules (e.g., PD-L1) (54) and by inducing immune-suppressive populations including myeloid-derived suppressor cells. Additionally, radiation can also directly kill immune cells and thereby modulate the immune response.
While some patients respond favorably to immunotherapy, many develop progressive disease (55). This has led to interest in combining immunotherapy with radiation (56–62). Synergistic combinations of radiation and immunotherapy have shown promise (62–66) as they help to overcome the immunosuppressive tumor microenvironment and thus enhance the therapeutic effect of radiation (67, 68). The optimal sequencing of radiation with immunotherapy (18, 69–72) as well as the best radiation modality for combination therapies (6, 16, 17, 73–76) are being studied extensively. It has even been suggested that low dose whole-body irradiation may improve outcome after subsequent treatment regimens due to radiation induced antigen release (52). Furthermore, pre-clinical data suggest that nodal irradiation may attenuate the combinatorial efficacy of immunotherapy-radiation combination regimens (77). There are numerous clinical trials combining radiation with immunotherapy (78).
While radiation-induced cell death is not the only key parameter when optimizing radiation treatments in this context, it certainly has a profound impact. Section 2 summarizes the published methods for estimating the dose delivered to circulating lymphocytes during radiation therapy. In section 3, studies assessing the radiation sensitivity of lymphocytes are reviewed.
2 Estimating the dose to the blood and to circulating lymphocytes in radiation therapy
Under the assumption that the dose to circulating blood is a surrogate for the dose to circulating lymphocytes, several efforts have been made to estimate the blood dose from radiation exposure. To estimate the cumulative blood dose from whole-body irradiation, Molloy et al. developed a blood perfusion model in which the circulation was modeled in a sinusoidal motion between the upper and lower body without regard to individual organs (79). The blood volume was divided into discrete voxels and a statistical dispersion was introduced to reflect the inhomogeneous blood flow in the body. The treatment beam was simulated assuming a time-dependent dose cloud depending on the field size and machine motion.
Yovino et al. (7) calculated the dose to circulating blood for a high-grade glioma patient as a function of dose rate and photon treatment technique. The model uses the three-dimensional dose distributions in the brain and calculates the dose to the blood passing through the radiation field by assuming that 16% of the cardiac output enters the brain with a total blood volume of 5 l and a blood flow velocity of 10 mm/sec. The model includes several simplifications, such as uniformly distributed blood flow without whole-body blood flow dynamics. Another assumption is that blood does not re-enter the treatment field during the duration of a single beam and/or segment. Between beams and between treatment fractions, the cumulative dose was calculated by convolution of the blood dose histograms. The simulations predicted that a single fraction of radiation would deliver 0.5 Gy to 5% of the circulating cells. After 30 fractions, 99% of the circulating blood had received ≥0.5 Gy. Target volume and field size were the most important parameters. This model was also used by Wild et al. (8) who came to similar conclusions.
Basler et al. (80) used dose-volume histograms for liver treatments to estimate the dose to circulating lymphocytes in VMAT. A mean hepatic blood flow velocity of 10 mm/s with a total body blood volume of 5 l was considered. Cardiac output was set at 5 l/min with a circulation time of 60 s for the total blood volume. The model assumes that regional hepatic blood flow is comparable in the different liver segments. Full blood mixing in between fields or fractions was considered and the probability of re-entering a specific liver segment and treatment field was calculated based on the cardiac output and relative volumes of the liver segments. The results show that the dose to the circulating lymphocytes was mainly influenced by the beam-on time and the target volume.
Jin et al. (81) used a similar approach as Yovino et al. to calculate the dose to the blood using a blood flow network consisting of the lungs, heart, large vessels, and body mass. The blood dose and blood volume contributing to each of these compartments during a single fraction were estimated and converted to an equivalent uniform dose, with the total effective blood dose being the sum of the contributions from all irradiated organs. The model was applied to lung treatments, taking into account mean lung dose, mean heart dose, and the integral dose. Blood dose was correlated with radiation-induced lymphopenia. This model was subsequently applied in other studies that demonstrated a correlation between blood dose and lymphopenia in non-small cell lung cancer (82), esophageal cancer (83, 84), and breast cancer (11), especially when the blood dose was above 4 Gy (84).
The dose to the blood was also estimated to analyze the transcriptional response of genes over time in blood samples after irradiation in vivo (85, 86). Considering that most of the blood is irradiated during a 2-min treatment time, the authors determined the mean blood dose as a function of the mean dose to the irradiated volume, the irradiated blood volume, and the body blood volume.
Shin et al. developed a compartmental model that considers blood flow throughout the human body based on compartments defined by the ICRP (International Commission on Radiological Protection) (23). The algorithm assumes a dynamic model describing the spatio-temporal distribution of blood particles (BPs) in organs throughout the body using a discrete-time Markov process. Blood transit times were modeled using ICRP reference mean transit time distributions assuming a Weibull distribution. This was then convolved with the time-dependent radiation field delivery. The simulations revealed different dose levels to the circulating blood for brain irradiation compared to liver irradiation even for similar field sizes due to the different blood flow characteristics of the two organs. The authors also showed that the blood dose-volume histogram is highly sensitive to changes in the treatment time, indicating that dynamic modeling of blood flow and radiation delivery is necessary to evaluate dose to the circulating blood.
To add another level of complexity and accuracy, blood dose algorithms have been developed that explicitly consider venous and arterial vascular trees to account for inhomogeneous organ dose distributions and blood flow dynamics. Hammi et al. (87) developed an intracranial blood flow model based on the major cerebral vasculature extracted from patient MRI data and extended with a network of generic brain vessels. The brain model contains more than 1000 vascular pathways. To determine the dose to the circulating blood, Monte Carlo simulations track the propagation of each individual blood particle through the brain and the time-dependent radiation field delivery. The mean dose to the blood pool was estimated after fractions of proton and photon therapy and showed that the fraction of blood volume receiving any dose after the first fraction was significantly lower for proton therapy. Higher dose rates effectively reduced the fraction of blood irradiated to low doses but increased the amount of blood receiving high doses. The model was also applied by Qian et al. (13), who showed that the treatment dose to the whole body, bone, and large blood vessels as well as the modeled dose to circulating lymphocytes were correlated with lymphopenia.
The internal vasculature of the adult liver, including hepatic arterial, hepatic venous, and hepatic portal venous vessel trees, was created within individual lobes of the ICRP adult female and male livers by Correa-Alfonso et al. (88). For each iteration of the algorithm, pressure, blood flow, and vessel radii within each tree were updated as each new vessel was created and connected to a viable bifurcation site. Liver models were created with virtual vasculature of ~6000 non-intersecting straight cylinders representing the circulations of the vascular tree. To combine the vascular trees with a dynamic dose delivery model, the trees were translated into centerlines that can be deformed to account for patient specific organ contours and for BPs entering the liver. An explicit simulation was implemented to track BPs along different vascular pathways through the liver (24). The dosimetric impact of treatment modality, delivery time, and fractionation on circulating blood cells was quantified showing that doses are highly sensitive to the beam-on time and demonstrating the trade-off between low dose to a large fraction of blood cells and high dose to a small fraction of blood cells. It was concluded that proton treatments are not necessarily advantageous in terms of dose to the blood even though they are associated with a lower integral dose because of the importance of the beam-on time. Similar vascular tree models have been developed for the brain (89) and lung (90). Such organ-specific vasculature models can be combined with a Markov chain approach to link them to whole body blood flow based on reference values for cardiac output and organ blood volumes (23, 24).
These blood dose models have been used to demonstrate how the dose to the patient’s circulating blood depends not only on hemodynamic data but also on treatment modality, beam delivery parameters such as field size, treatment time, fractionation, and dose. While they have been able to show trends in RIL, their main weakness is that the results from blood dose simulations do not necessarily translate directly to doses to circulating lymphocytes, which may have different transition and flow parameters than the blood. Unfortunately, these are more complex and not as well-known (91, 92).
Jin et al. (93) developed a lymphocyte trafficking model that is an extension of an algorithm discussed previously (21). The framework considers 5 compartments of the immune system, i.e. the circulating blood, the bone marrow, specific lymphatic organs such as spleen, lymph nodes/vessels, and other lymphatic tissues in non-lymphatic organs such as gut, lung, liver and skin. Circulating and noncirculating lymphocytes are considered separately. The model also incorporates lymphocyte radiosensitivity and reproductivity. The authors assume that lymphocytes in the blood circulate at a higher rate than the blood. Clinical beam delivery times were not taken into account as the irradiation time was assumed to be equal to the blood circulation time, and all organs were treated as homogeneous.
To study the interaction between immunotherapy and radiotherapy, Friedrich et al. introduced a biophysical model of lymphocyte trafficking that takes into account primary and distal tumor masses, immune cell kinetics targeting tumor cells, and immune cell replenishment after radiation (94). Model parameters were derived from mouse data. The model suggests that the immune response is stronger when checkpoint inhibitors are administered at the time of radiation or shortly thereafter. It predicts that there is a window for radiotherapy that optimally balances radiogenic immune response and depletion of the immune cell pool.
In order to understand the impact of high-dose rate irradiation on the dose to the circulating blood and lymphocytes an algorithmic model was developed by Cucinotta and Smirnova (95). The model also incorporates a one-target-one-hit model of radiation-induced damage as a basis to consider the response of blood lymphocytes to the radiation exposure. It considers time-dependent dose delivery, radiosensitivity and concentration of lymphocytes, as well as blood flow characteristics through the blood circulatory system including the total blood volume and heart rate. The model confirms that the level of surviving blood lymphocytes increases as the dose rate increases.
3 Radiosensitivity of lymphocytes
Monocytes and macrophages isolated from peripheral blood cells are highly radioresistant (96, 97). Monocytes do not express proteins required for non-homologous end-joining and are impaired in base excision repair, which is likely to limit repair especially at higher doses (98). When monocytes proliferate into macrophages and dendritic cells, proteins are upregulated that make these cells repair competent. Dendritic cells are thought to be highly resistant to radiation-induced apoptosis (99). However, the irradiation of dendritic cells may impair their ability to stimulate T cells (100).
Peripheral blood lymphocytes are primed to undergo apoptosis (101). While most mammalian cells are radioresistant at rest and radiosensitive during proliferation, the opposite is true for circulating lymphocytes. Even a small amount of DNA damage appears to be sufficient to activate a DNA damage response and apoptosis (102). Damage to peripheral lymphocytes (e.g., chromosome aberrations) has been used as bio-dosimeters to predict late radiation toxicity in radiation therapy patients (103).
The literature discussed in the following sections is not always consistent in terms of notation. Naïve T cells can be categorized into helper Th cells (CD3+, CD4+) and cytotoxic Tcyt cells (CD3+, CD8+) with regulatory Treg cells (CD4+ CD25+, Foxp3+) as a subset of Th cells. Categorization can also be done into naïve, effector Teff (CD25+), and memory T cells (effector memory TEM (CD45RO+, CD25-, CCR7-) and central memory TCM (CD45RO+, CD25+, CCR7+)). Naïve B cells (CD27-) and B cells (CD19+, CD20+) can also play an immune-suppressive role, for example by blocking the Tcyt cell response. Naïve NK cells (CD16-) can become effector, regulatory, and memory NK cells (CD16+, CD56+, CD3-). NKT cells are a subset of T cells that express both CD3+ and CD56+.
3.1 Lymphocyte radiosensitivity studies in humans
The results of in vivo radiosensitivity studies in patients with qualitative or quantitative information are summarized in Tables 1A, B with the latter showing estimated alpha values [Gy-1] for a linear dose-response curve (exp(-αD)). A rather comprehensive study of lymphocyte radiosensitivity was performed by Trowell et al. already in 1952 (110). After whole-body irradiation, lymphocytes were counted in lymph nodes. In addition, lymph nodes and blood samples were irradiated in vitro. In 1975, Heier et al. (1) analyzed early and late T cell and B cell counts in patients with seminoma testis. B cells seemed to be more radiosensitive. They also concluded that B cells recovered more rapidly than T cells after the irradiation of the iliac and paraaortic lymph nodes and that irradiation of the thymus did not alter lymphocyte recovery.

Table 1B Estimated alpha values (in Gy-1) for a linear dose-response curve exp(-aD) based on lymphocyte depletion in patients (fits performed using LMfit in python).
Lymphocyte radiosensitivity in vivo was evaluated by Clave et al. (105) based on whole-body irradiation of patients prior to bone marrow transplantation. Lymphocyte subpopulations were counted after irradiation at 2 Gy/fraction. B cells were the most sensitive, followed by T cells (CD4+, CD8+) and NK cells. CD34+ progenitor cells appeared to be highly radioresistant. Note that the easurements include circulating lymphocytes while also irradiating lymphatic vessels. A similar study by Girinsky et al. found no statistically significant difference in radiosensitivity between T cells and B cells (111). Lymphocyte depletion and recovery for different subpopulations has also been studied for low dose whole body irradiation from the Chernobyl accident and in atomic bomb survivors (112).
B cells were the most sensitive and NK cells the least sensitive lymphocyte fraction in cancer patients receiving pelvic radiation therapy (106). No significant differences between Th cells and Tcyt cells were reported. The counts of the lymphocyte subpopulation as a function of total body dose can be translated into alpha values in a linear dose-response curve. Belka et al. (107) evaluated lymphocyte subpopulations after radiation therapy and found that B cells and T cells seemed to be most affected. Recovery of CD8+ cells was significantly faster than that of CD4+ cells, and naïve cells were generally more sensitive than memory cells. Lymphocytes were still unable to respond adequately to antigen stimulation even after recovery of the population.
A comprehensive assessment of circulating immune cell populations in response to stereotactic body radiation therapy in patients with liver cancer was performed by Gustafson et al. (46). They found a severe decrease (~50%) in T cells in liver SBRT patients, even in the absence of bone marrow or nodes in the field, with CD4+ cells being most affected, while CD8+ cells showed no significant differences compared to pre-treatment levels. More specifically, within the CD4+ compartment, Treg cells were not affected. SBRT did not appear to affect mature NK cells (CD16+) but did affect pre-cursor cells (CD16-). McGee et al. analyzed the blood of 31 patients after stereotactic ablation radiation therapy (113). They showed that the effect of radiation on T cells and NK cells depends on the treatment site. Therapy of parenchymal sites induced a systemic immune response (i.e., a decrease in NK cells and an increase in memory CD4+ and CD8+ T cells). This was not seen in non-parenchymal sites (bone and brain).
Zhao et al. (108) analyzed lymphocyte subpopulations after SBRT of early-stage lung cancer. The number and relative percentage of CD4+ T cells were significantly decreased, whereas the number of CD8+ T cells was less affected as their relative percentage was almost unchanged. This decreasing ratio of CD4+/CD8+ T cells was also observed by Yang et al. (104) in head and neck cancer patients. The change in the ratio could not be explained by the small difference in radiosensitivities of CD4+ T cells and CD8+ T cells but was presumably caused by radiation-induced priming and mobilization of CD8+ T cells compensating for the loss of CD8+ T cells. Lymphocyte subpopulations in patients after radiation therapy have also been studied in other sites, such as in prostate cancer (42, 114–116) and in breast cancer (117, 118), demonstrating radiosensitivity of B cells in particular.
Heylmann et al. (97) analyzed T cells and monocytes after treatment in leukemia patients receiving whole body irradiation (6 times 2 Gy). Monocytes showed high radioresistance, and the difference in the lower response between T cells and NK cells was not statistically significant. Three patients who received 12 Gy in 3 days (2 times 2 Gy per day) were analyzed. Analysis of γH2AX foci indicated efficient elimination of damaged B cells during treatment. In NK cells (CD56+), DNA damage accumulated in the surviving NK after repeated irradiation. Whether these cells later undergo apoptotic death or survive in the presence of DNA damage was unclear.
Assuming an exponential dose response relationship, the alpha value of circulating lymphocytes has also been deduced indirectly in patients. A lymphocyte trafficking model was fitted to 51 patients with abdominal cancer treated with radiotherapy (93). The patient specific α values had a median of 0.40 Gy-1 (range 0.08 – 2.0 Gy-1). Similarly, for hepatocellular carcinoma the dose to circulating lymphocytes was estimated using a dynamic blood circulation model (23) and combined with the observed lymphocyte depletion in patients, empirically accounting for both cell death and lymphocyte replenishment. The in vivo derived patient-specific α had a median value of 0.58 Gy-1 (range 0.28 - 1.23 Gy-1) (109).
Schaue et al. (119) isolated lymphocytes from colorectal and prostate cancer patients before, during, and one week after chemoradiation therapy. In most patients, they found an increase of Treg cells as well as CD8+ cells after radiation which was more pronounced in colorectal patients. A relative resistance of Treg could have negative consequences in radiation therapy of their tumor protective role as compared to the immune stimulatory role of more radiosensitive Tcyt cells and Th cells. However, radiation can also reduce protein expression and reduce functionality of Treg cells (120).
3.2 Lymphocyte radiosensitivity studies in mice
Results from preclinical studies of in vivo radiosensitivity with qualitative or quantitative information are summarized in Tables 2A, B and include fitting of exponential dose-response curves where possible (Figure 1).
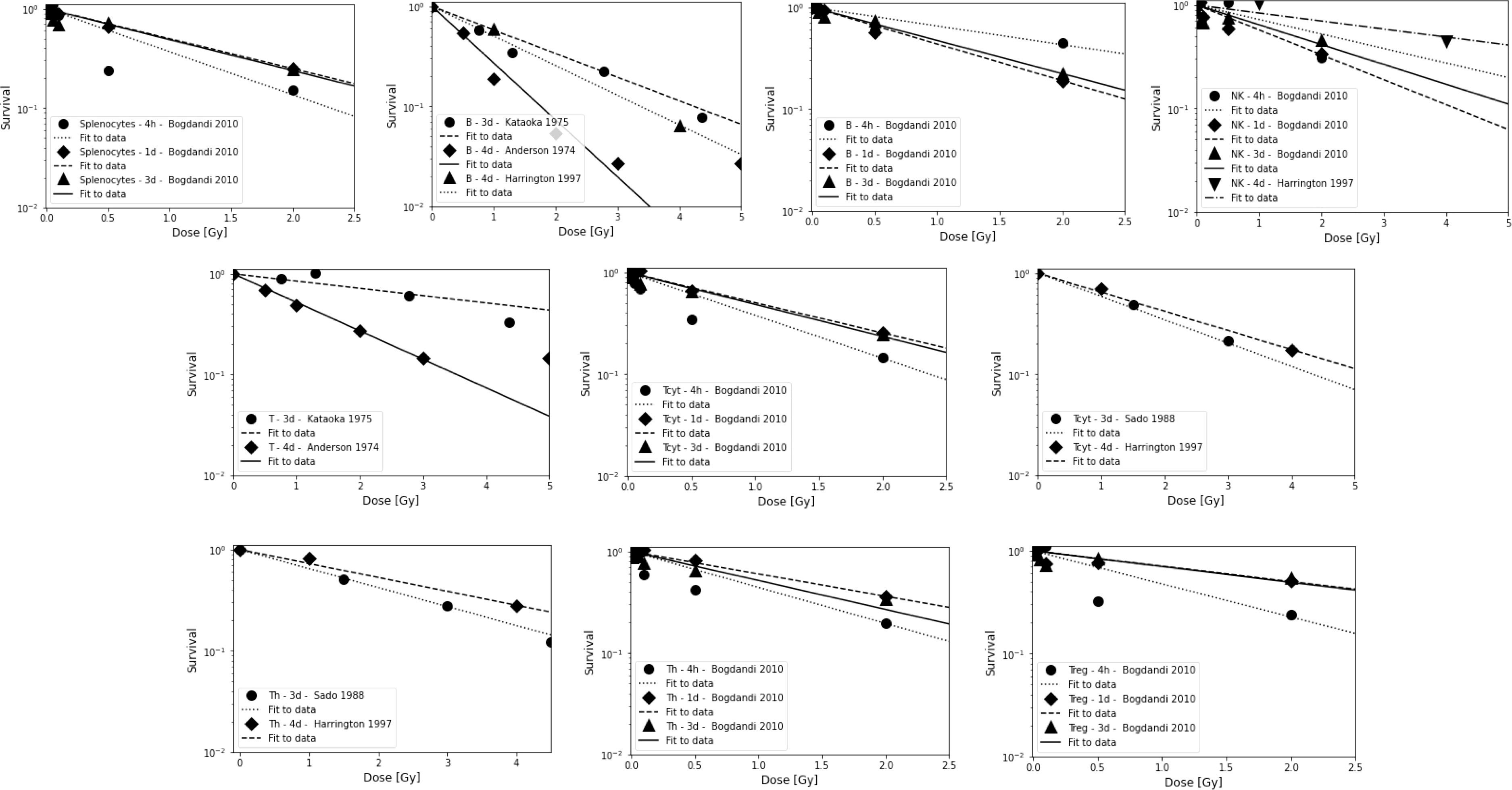
Figure 1 Radiosensitivity of lymphocyte sub-populations in mice for studies shown in Table 2B. First row: Splenocytes, B cells, and NK cells. Second row: Combined T cells and Tcyt cells. Third row: Th cells and Treg cells. Data points are shown up to 5 Gy but alpha value fits were only done for data points ≤3 Gy because lymphocytes will not receive more than the prescription dose in a single fraction in radiation therapy, and because the majority of the dose-response data show a more shallow slope and a saturation at higher doses. The data points were extracted from the published figures (using plotdigitizer (plotdigitizer.com)). Experimental error bars are not shown but are included in the fits (performed using LMfit in python).
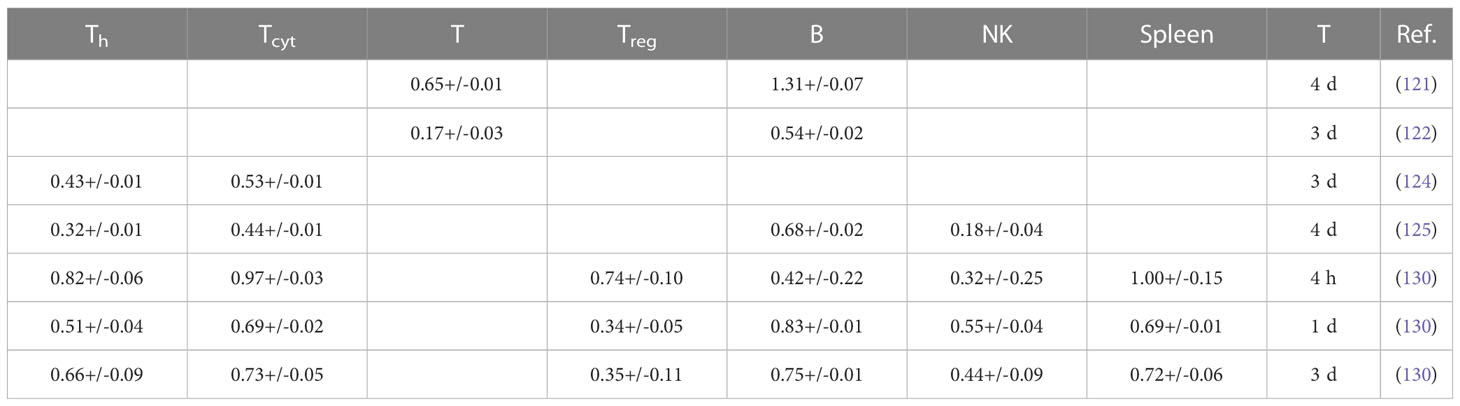
Table 2B Estimated alpha values (in Gy-1) for a linear dose-response curve exp(-aD) based on lymphocyte depletion in mice (fits were performed using LMfit in python and include only data points ≤ 3 Gy).
Anderson et al. (121) investigated the effect of radiation on lymphocyte migration. Activated thoracic duct lymphocytes from CBA inbred mice were used as a surrogate for T cells (80-85% T cells, 15-20% B cells) and those from athymic (nude, nu/nu) mice were used as a surrogate for B cells (97%). B cells were highly radiosensitive compared to T cells and activated T cells were more radioresistant than their resting counterparts. In another study, T cells were shown to be more radioresistant than B cells in the spleen of C3Hf mice (122). After whole-body irradiation, T and B cells were analyzed after 3 days. At low doses (0.47 Gy), the number of T and B cells in the spleen was significantly higher compared to unirradiated control mice.
That B cells are more radiosensitive than T cells was also observed after whole-body irradiation with doses of 0.5-15 Gy (123). Mice were sacrificed and thymus, spleen, mesenteric lymph nodes, femur, tibia and fibula were removed, and peripheral blood was analyzed after 6 days. The authors also studied recovery of T and B cell populations after 6 Gy and showed that cells in the thymus and spleen recovered more rapidly than those in the lymph nodes and in the bone marrow. Hochman et al. (140) reported the relative resistance of NK cells in the spleen of (C57BL/6 x C3H/He)F mice and the temporary cessation of progenitor activity. Sado et al. (124) showed that cells from C3H mice were more radioresistant compared to BALB/c, C57BL/6, and B10.BR mice. After whole-body irradiation, T cells were analyzed in the spleen after 3 days. CD8+ T cells were slightly more radiosensitive than CD4+ T cells.
Harrington et al. (125) irradiated C57Bl/6 mice with doses of 0-7 Gy (whole-body) and analyzed splenic T cells (CD4+ and CD8+), B cells, and NK cells after 1, 4, and 7 days after irradiation. They observed a 7-fold enrichment of NK cells and a 3-fold enrichment of T CD4+ cells, while the proportion of CD8+ cells was unchanged and B cells decreased. While radiation may reduce the total number of lymphocytes, the spleen may be enriched when comparing subpopulations. B cells were most sensitive to radiation, followed by CD8+, CD4+, and NK cells. In a study by Chambers et al. (126) on lymphocyte subpopulations after whole-body irradiation of mice, the total number of peripheral lymphocytes decreased as a function of dose and the lymphocyte distribution changed. Relative to the total number of lymphocytes, CD8+ increased slightly on day 1 and then decreased, while CD4+ increased 2-fold on day 4 after 7 Gy. The relative contribution of NK cells increased 9-fold at day 4 at 7 Gy, while the relative number of B cells decreased at all dose levels, e.g., by half at 1 Gy. This indicated radioresistance of NK cells relative to CD4+, CD8+, and B cells.
Mice were exposed to a whole-body dose of 3 Gy of protons and 60Co X-rays by Kajioka et al. (127) and acute effects on the immune system were assessed. Overall, B cells were the most sensitive cell population, while T cells were moderately sensitive and NK cells were the most resistant cell population. Within the T cell population, Th cells were more resistant than Tcyt cells. This was also true for the splenic lymphocyte population. B cells had the most rapid recovery and recovered completely in the spleen but not in circulating lymphocytes. Grayson et al. (128) found that naive T cells were more sensitive than their memory counterparts (CD8+) after whole body irradiation of mice at 2-6 Gy. Lymphocytes were isolated from the spleen, lymph nodes, bone marrow, and peripheral blood. In a dose-dependent manner, memory CD8+ T cells were enriched in the spleen, increasing from 20% of the total CD8+ population in untreated mice to 76% after 6 Gy. Garg et al. (129) analyzed immune cell populations in the intestinal mucosa after whole body irradiation of mice and found that B cells were more sensitive compared to T cells.
In another study of apoptosis in mouse spleen cells, animals were sacrificed 4 h, 1, 3 or 7 days after irradiation (130). The authors analyzed Th, Tcyt, Treg, NK, B, and CD8+CD44+ memory T cells. Low dose radiation decreased apoptosis compared to the control. In terms of apoptosis at 4h, CD8+ and B cells were more resistant to low doses but were very sensitive to 2 Gy, while NK cells and Treg were much more resistant to higher doses. B cells were the most sensitive, followed by Tcyt, Th, Treg, and NK cells. Analysis of subpopulations after 7 days showed that Tcyt cells started to regenerate earlier than Th cells.
In a series of investigations, Qu et al. compared the radiosensitivity of CD4+CD25high Foxp3+ Treg cells and CD4+CD25- T cells in mice after 2 Gy (131) and 5 Gy (132) whole-body irradiation. In vivo depletion showed an increased sensitivity of CD8+ compared to CD4+, while the level of CD4+CD25high Treg increased. For both spleen and lymph nodes, the radiosensitivity of CD8+ was higher than CD4+, followed by Treg cells. In the thymus, the levels of CD4+CD8+ decreased. However, the newly developed Treg cells in the thymus were less sensitive to radiation than other thymocytes. The function of Treg cells was impaired after 5 Gy radiation but not after 2 Gy, suggesting a threshold effect.
Assessing lymphocyte populations after low-dose total body irradiation in mice, Liu et al. found significant decrease in the Treg cell population (52), but an increase in memory T cells (CD4+/CD8+). Despite increased radiosensitivity, Tcyt cells were activated in mice after fractionated low-dose exposure (0.2 Gy), which was not previously observed for Th cells (141). Spleen cells were analyzed after whole body irradiation of mice with 10 Gy in another report (133). Analysis was performed at 24, 48, 72, and 120 h. CD4+ T cells were significantly more resistant than CD8+ T cells, and CD44high T cells, including NKT cells and memory T cells, were significantly more resistant than CD44low (naive) T cells. Furthermore, the effect of radiation on naturally occurring Treg cells was investigated in a mouse model (134). The number of Treg cells increased after irradiation as they appeared to be more radioresistant compared to other lymphocytes. Their functional integrity was also unaffected. However, this observation could also be caused by radiation-induced Treg cell activation.
Balogh et al. (135) irradiated C57Bl/6 mice with 2 Gy (whole body) and analyzed changes in lymphocyte fractions isolated from the spleen. Treg cells were less prone to apoptosis than other lymphocytes after in vivo irradiation. The results showed a greater decrease in CD4+ numbers compared to Treg cells that were not only less susceptible to radiation-induced apoptosis but also recovered faster than CD4+Foxp3- cells. However, irradiated Treg cells were functionally compromised with a reduced suppressive capacity (~2.5 fold). In addition, radiation increased the proliferation rate of surviving CD4+ cells. In a study by Pugh et al. (136), mice were irradiated in vivo at doses up to 4 Gy and splenocytes as well as peripheral lymphocytes were analyzed at 3, 12, 17, and 24 h. CD8 TEM cells were more resistant and naive T cells more sensitive. CD8 TCM cells were significantly more resistant in vivo than in vitro. The authors hypothesize that this may be due to the genome-wide chromatin structure that governs early DSB binding and survival. Chromatin remodeling occurs during the differentiation of naive T cells to memory T cells.
In T cell recovery after low-dose whole body irradiation of female C57BL/6 mice, CD4+ T cell reconstitution was delayed more than that of CD8+ T cells (142). Venkatesulu et al. showed lymphopenia after heart (2 Gy per day for 5 days) and spleen (1 Gy per day for 5 days) irradiation of female BALB/c mice in vivo (137). B cells were most sensitive in both cohorts. For cardiac irradiation this was followed by CD8+, while CD4+ depletion was moderate in comparison. For splenic irradiation there was no significant difference between CD8+ and CD4+. Radio-resistance of splenic lymphocytes compared to circulating lymphocytes has also been shown in a C57BL/6J mouse model after studying partial body irradiation with and without lymph node involvement (139). The authors also investigated the effect of different field sizes using a small animal image-guided irradiation device.
A study by Arina et al. (138) in which mice were irradiated with a whole-body dose of 8 Gy showed a dose-dependent loss of circulating CD8+ T lymphocytes, but not of tumor-infiltrating CD8+ T cells after 24 h. The authors also quantified the sensitivity of parenchymal CD8+ in various organs. Within certain solid organs there was a higher radio-resistance compared to T cells in circulation and in lymphoid organs. Lymph nodes and spleen had the most radiosensitive CD8+ T cells, while CD8+ T cells in the intestine were the most radioresistant. They hypothesized that the higher radioresistance of parenchymal CD8+ T cells from non-lymphoid compared to lymphoid solid organs is due to the presence of tissue resident memory cells. In tissues harboring the most radioresistant CD8+ T cells (intraepithelial and tumor), not only cells with the standard memory T cells but all CD8+ T cells were similarly radioresistant. In contrast, memory T cells in the liver were more radiosensitive than other T cells.
3.3 In vitro lymphocyte radiosensitivity studies
Results from in vitro radiosensitivity studies with qualitative or quantitative information are summarized in Tables 3A, B and include fitting exponential dose response curves where possible (Figure 2).
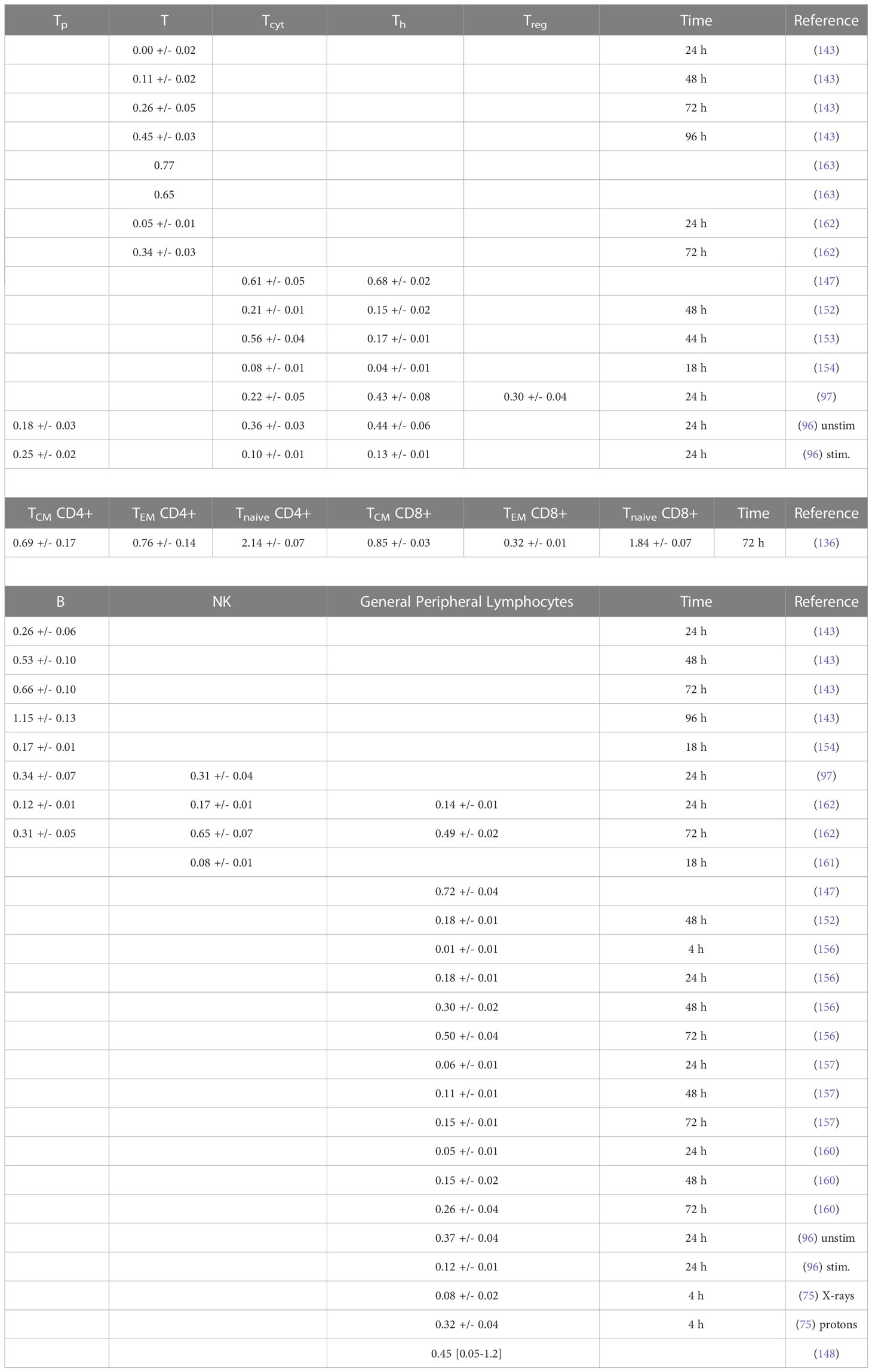
Table 3B Estimated alpha values (in Gy-1) for a linear dose-response curve exp(-aD) based on in vitro measurements (fits were performed using LMfit in python and include only data points ≤ 3 Gy).
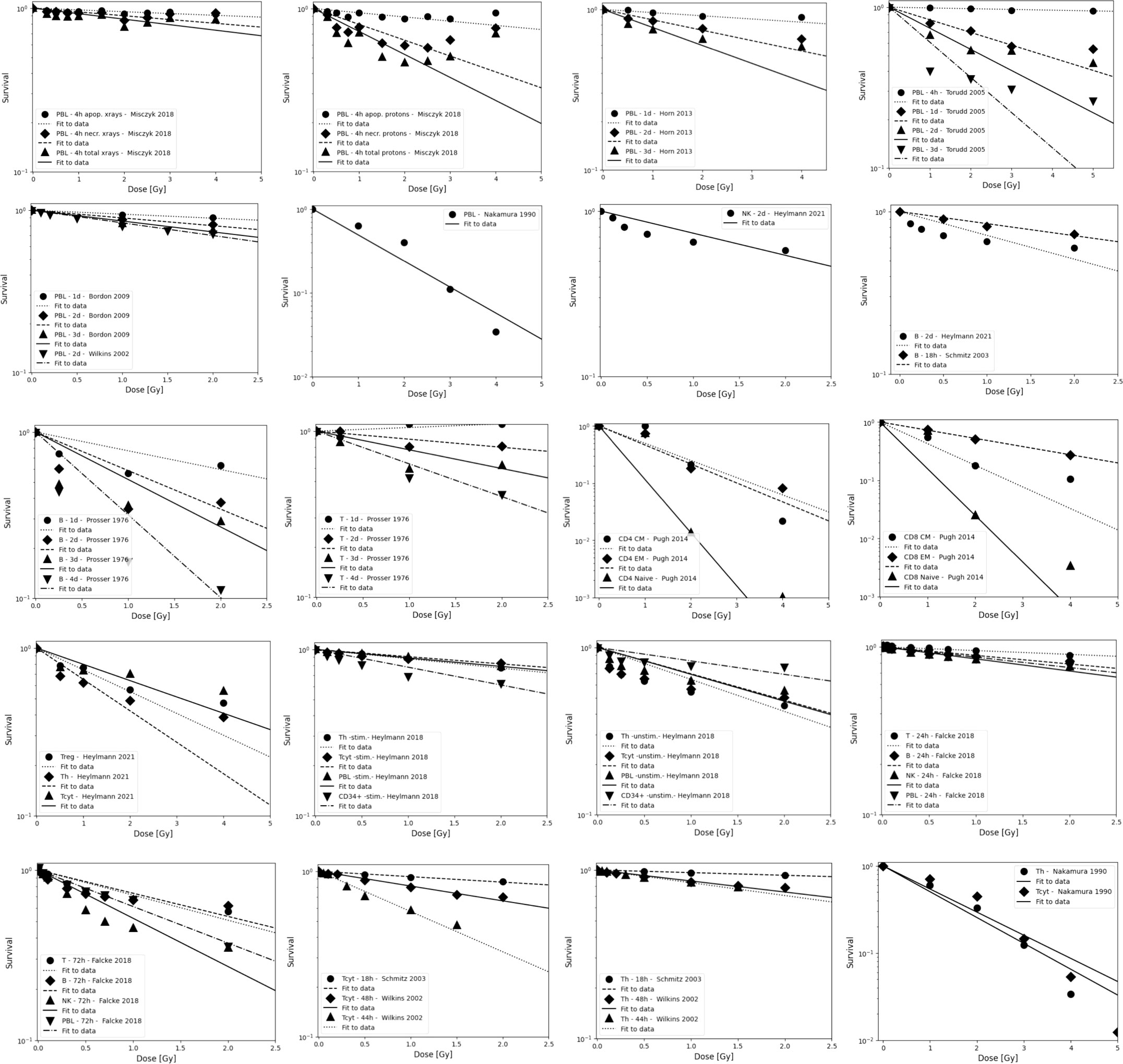
Figure 2 Radiosensitivity of peripheral lymphocytes, NK cells, T cells, and B cells. Data are grouped to illustrate both, differences between experiments as well as differences between subpopulations. Data points are shown up to 5 Gy but alpha values fits include only data points ≤ 3 Gy because lymphocytes will not receive more than the prescription dose in a single fraction in radiation therapy, and because the majority of the dose-response data show a more shallow slope and a saturation at higher doses. The data points were extracted from the published figures (using plotdigitizer (plotdigitizer.com)). Experimental error bars are not shown but are included in the fits (performed using LMfit in python).
The survival of unstimulated T and B cells from a healthy donor was evaluated in 1-day intervals up to 4 days after irradiation with doses up to 10 Gy by Prosser et al. (143). They observed a higher radiosensitivity of B cells compared to T cells. Cole et al. measured T cell survival in blood from 9 donors and T cell lines (163). The in vitro survival of human peripheral blood lymphocytes and thymocytes (T cell progenitors) was also measured after 4 days in a study by Kwan and Norman in healthy volunteers (144). B cells appeared to be slightly more radiosensitive than T cells. The authors concluded that there are subpopulations of T and B cells with different radiosensitivities, resulting in a biphasic survival curve for T cells. Brovall et al. (164) studied NK cell activity in the peripheral blood of healthy adults. While activity was lost at 30 Gy, it was enhanced at lower doses (5 to 20 Gy, depending on the donor). This suggests that radiation affects the cytotoxic function of NK cells before death or apoptosis is observed. Zarcone et al. (145) investigated the effect of radiation on different NK cell activities. The cytotoxic functions of NK and T cells showed identical sensitivity to radiation. Similarly, Rana et al. (146) investigated cytotoxic activities of NK cells as a function of dose up to 30 Gy and showed that CD16+ were the most radiosensitive.
Nakamura et al. (147) investigated the radiosensitivity of proliferating Th and Tcyt lymphocytes in vitro using a colony formation assay. This particular study is often cited when discussing lymphopenia and its relationship to lymphocyte radiosensitivity. Strikingly, the measured cell survival curves follow a linear-quadratic dose-response fitted by the linear quadratic model (PBL: α=0.29+/-0.01; β=0.14+/-0.01; CD4+ α=0.32+/-0.01; β=0.13+/-0.01; CD8+ α=0.19+/-0.03; β=0.14+/-0.01), whereas in the majority of in vitro studies reviewed, the response curves show either an exponential or upward-sloping curve and a decrease in response (saturation) at higher doses.
Using blood from cancer patients and healthy individuals, Geara et al. analyzed the radiosensitivity of peripheral T lymphocytes in vitro and demonstrated a significant variation among individuals (148). Patient-specific α values were fitted with a median of 0.45 Gy-1 (range 0.05 - 1.20 Gy-1). Seki et al. (149) showed that CD8+ T cells were more susceptible to interphase death than CD4+ T cells and NK cells were the most radiosensitive. Philippe et al. (150) assessed apoptosis after 24 h in vitro. B cells showed more apoptotic cells than T cells. Among T cells, Th cells were the most sensitive, followed by Tcyt cells. NK cells were the most resistant. Spontaneous apoptosis in immune subsets of in vitro cultured cells correlated with differences in radiation induced apoptosis. Radojcic and Crompton used peripheral lymphocytes from three donors to assess the age dependence of CD4+ and CD8+ cell apoptosis at 2 and 9 Gy and suggested that radiosensitivity may be higher in younger individuals (151). CD8+ were more sensitive than CD4+.
Wilkins et al. studied the apoptotic response in lymphocytes using blood from healthy volunteers. One study focused only on CD8+ and CD4+ cells (152). CD8+ T cells were more sensitive to radiation-induced apoptosis than CD4+ at doses up to 2 Gy at 48 h. The authors state that the relative amounts of CD4+ and CD8+ in the combined culture likely influenced the observed apoptosis due to changes in the production of specific cytokines in the cell culture. A second study examined B cells, NK cells, and CD4+ and CD8+ T-cells at 44 h and 68 h after exposure to up to 1.5 Gy (153). Although B cells showed the highest radiation-induced apoptotic response at 1 Gy, CD8+ T-cells appeared to be the most sensitive based on their low spontaneous apoptotic fraction. At 48 h, the radiation-induced apoptosis of the cell subpopulations decreased in the order of NK cells, CD8+ T cells, B cells and CD4+ T cells, although the differences were not significant. Again, lymphocytes in isolation appeared to be more responsive to radiation than those cultured in the presence of other lymphocytes.
In a study of spontaneous and radiation-induced apoptosis of human lymphocytes in vitro, lymphocytes from females were less radiosensitive compared to those from males and radiosensitivity seemed to increase with age (154). Tcyt cells were more sensitive than Th cells. Hayashi et al. (155) investigated radiation-induced apoptosis of stem/progenitor cells in human umbilical cord blood. The CD34+/CD38− stem cell population was more sensitive to radiation-induced apoptosis, compared to more differentiated CD34+/CD38+ and CD34−/CD38+ cells. Human lymphocytes were irradiated in vitro with doses up to 15 Gy by Torudd et al. (156). Apoptosis was assessed at 4, 24, 48, and 72 hours. There was very little effect at the early time point at 4 hours. In the context of establishing a predictor of patient’s response based on individual lymphocyte radiosensitivity, Bordon et al. (157) evaluated how radiation induced apoptosis correlates with late toxicity and patient’s radiosensitivity in cervical cancer. Radiation-induced apoptosis was analyzed at 24, 48, and 72 hours.
Milyavsky et al. (158) reported that human hematopoietic stem cells (CD34+) exhibited delayed DNA double-strand break rejoining, persistent γH2AX foci, and increased apoptosis after irradiation compared to progenitor cells. Cao et al. (159) compared the radiosensitivity of Treg cells (CD4+CD25+) and effector T cells (CD4+CD25-) in vitro using lymphocytes from healthy individuals and hepatocellular carcinoma patients. In the range of 0-2 Gy, Treg cells were more radiosensitive than effector T cells, the opposite trend compared to a previous in vivo study (135). Treg cell functionality was moderately affected in Cao et al. (165) using in vitro cultured and in vitro irradiated Treg cells, showing a dose-dependent reduction in Treg cell proliferation as well as an alteration in phenotype.
In another study of peripheral lymphocytes from healthy donors, radiation-induced apoptosis in vitro was not apparent until 24 h after exposure when data were analyzed at 24, 48, and 72 h (160). Radiosensitivity was highest for B cells, followed by Tcyt cells, and Th cells, but the trend was reversed for B cells and Tcyt cells after 48h and 4 Gy. Hietanen et al. (161) applied single and fractionated doses to enriched NK cell populations. Cell survival was reported from 2 to 72 h and for doses up to 80 Gy. The response based on the reported α values was very similar for CD16+ and general CD56+ cells at 18 h at doses up to 40 Gy.
A review of the radiosensitivity of human and murine peripheral blood lymphocytes concluded that stem cells, Th cells, Tcyt cells, monocytes, neutrophils and, to a high degree, B cells exhibit a radiosensitive phenotype, whereas Treg cells, macrophages, dendritic cells and NK cells appear to be more radioresistant (166). The same authors studied stimulated (proliferating) and unstimulated (non-proliferating) peripheral lymphocytes in the blood from healthy volunteers (96). Unstimulated peripheral lymphocytes contained mainly T cells arrested in G0/G1. Upon stimulation of the CD3 T-cell receptor and the CD28 co-receptor with anti-CD3 and anti-CD28, respectively, the cells begin to proliferate. Lymphocytes were shown to be highly radiosensitive but stimulation induced radioresistance in several T cell subsets, with the exception of CD34+ cells which did not become radioresistant when stimulated to proliferate. There was no difference in repair between stimulated and unstimulated cells, i.e., the difference in radiosensitivity was likely caused by the induced DNA damage. The investigators found that most of the cells underwent apoptosis with only a small fraction of necrosis, with data collected between 6 and 48 hours after irradiation. It was concluded that T cells and B cells are highly sensitive and undergo apoptosis at doses as low as 0.125 Gy with no apparent threshold and a saturation of ~50% at about 1-2 Gy. Sensitivity was highest for non-proliferating T cells followed by B cells, and NK cells. However, while non-proliferating T and B cells were sensitive, they had a high repair capacity, which was also the case for CD34+. There was no significant difference in radiosensitivity between the non-proliferating T cell subcategories. The same authors then measured the in vitro dose response of blood cells from healthy volunteers (97). The analysis included unstimulated T cells (Treg, Th, Tcyt) purified with magnetic beads as well as unstimulated B cells, and NK cells obtained from peripheral blood. Doses ranging from 0.5 to 8 Gy were administered. Th cells were the most sensitive (30% apoptosis level at 0.5 Gy, while Treg, NK and B cells showed values around 20–25%). The authors point out that absolute numbers may be associated with uncertainties because there may be early apoptotic events that have been fragmented, or late apoptotic events that have not yet materialized at the time of the assay.
Apoptotic cells may lose membrane integrity and become secondary necrotic cells that retain immune activation properties. Falcke et al. (162) studied lymphocyte cell death by apoptosis, primary necrosis, and secondary necrosis (late apoptotic). They found that B cells and NK cells died mainly by apoptosis (secondary necrosis), whereas T cells showed significant primary and secondary necrotic cells. NK cells were the most sensitive to radiation, followed by B cells and T cells. The researchers also analyzed cell viability. In an in vitro study of human peripheral lymphocytes, necrosis was more frequent than apoptosis, especially with proton irradiation (75). This may indicate a mechanistic difference in lymphocyte damage when comparing photon and proton radiation (75, 167). The accumulation of radiation-induced repair protein foci differed after proton versus X-ray irradiation (168). Annexin V labeling was performed 1 h and 4 h after irradiation with doses of 0-4 Gy (75). Alpha values for peripheral lymphocytes differed between X-rays and protons as well as between apoptosis and necrosis (apoptosis X-rays: α=0.02+/-0.01 Gy-1; apoptosis protons: α=0.03+/-0.01 Gy-1; necrosis X-rays: α=0.04+/-0.01 Gy-1; necrosis protons: α=0.15+/-0.03 Gy-1).
Using blood from healthy volunteers Beauford et al. (120) found that Th cells were more radiosensitive than Treg cells. Although Treg cells appeared to be more resistant, radiation caused a decreased Foxp3 expression as well as decreased expression of CD25 and CTLA-4, resulting in a reduced ability to suppress CD8+ T cell proliferation. Vandevoorde et al. (169) compared the dose response of CD34+ cells and umbilical cord T cells from newborns and adults. Naïve and memory T cells were analyzed in vitro 0.5 h after irradiation with low doses (100-200 mGy). Newborn peripheral T lymphocytes were significantly more radiosensitive than adult peripheral T lymphocytes. This may be due to immunophenotypic changes of T lymphocytes with age.
De Kruyff et al. (170) analyzed the functional behavior of lymph node T cells in mouse cell cultures as a function of dose. Specifically, the authors evaluated the helper activity of CD4+ T cells in terms of their ability to induce immunoglobin synthesis (IgG, IgM, and IgE synthesis) in B cells. The capacity for IgG synthesis was not affected, while that for IgE (which depends on IL-4 and IL-5) was significantly reduced. Thus, IL-4 in Th cells appears to be sensitive to radiation, causing T cell functions to show large variations in radiosensitivity. Pugh et al. (136) measured the radiosensitivity of naïve lymphocytes, effective memory cells (CD8, TEM), and central memory cells (TCM) from mice in vitro. There was no significant difference in radiosensitivity between T cell subsets. However, CD8 TEM cells were more radioresistant and showed less interphase death than TCM cells or naïve T cells. CD4 T cells were more radioresistant than CD8 T cells. This pattern was extended to both CD4 naïve T cells and TCM cell subsets. It was unclear whether the enhanced radioresistance of Treg cells could fully account for the enhanced radioresistance of any specific CD4 subset.
Qu et al. compared the radiosensitivity of CD4+CD25high Foxp3+ Treg cells and CD4+CD25- T cells in vitro showing higher sensitivity for CD4+CD25- T cells than for CD4+CD25high Treg cells at 2 Gy (131) and 5 Gy (132), respectively. They reported that more dead cells were observed in the Teff cell population than in the Treg cell pool, which correlated with a higher levels of anti-apoptotic protein expression in Treg cells. They also found that the Teff cell suppressive capacity of the in vitro irradiated Treg cells was only moderately affected by radiation. The evaluation was performed 2 weeks after irradiation.
4 Summary and discussion
The radiosensitivity of lymphocytes has been evaluated in a variety of ways, including studies in humans, pre-clinical studies, and in vitro clonogenic cell survival assays. The available data published in the open literature have been reviewed in this work. Interpretation of experimental data is often difficult. For example, in vitro measurements must be corrected for spontaneous apoptosis, which is particularly relevant for B cells. In addition, data from in vivo studies have to take into account that a part of the lympho-hematopoietic system has been irradiated, allowing for lymphocyte redistribution from non-irradiated areas. Expression changes may also occur.
Studies based on lymphocyte depletion in patients consistently suggest that B cells are the most radiosensitive, followed by T cells and NK cells, with helper T cells (CD4+) being more radiosensitive than cytotoxic T cells (CD8+). The preclinical studies support this difference between B and T cells. Preclinical studies also suggest that circulating lymphocytes appear to be more radiosensitive than non-circulating lymphocytes and tumor infiltrating T lymphocytes. In addition, parenchymal T cells from non-lymphoid solid organs appear to be more radioresistant than those from lymphoid solid organs. The obtained average dose-response alpha values derived from lymphocyte depletion in mice are ~0.8 Gy-1, ~0.6 Gy-1, and ~0.4 Gy-1, for B cells, T cells, and NK cells, respectively (for doses up to 3 Gy, after 4 hours to 4 days). For splenocytes, an average value of ~0.8 Gy-1 was extracted. There is some indication that naïve lymphocytes, which make up more than 50% of the lymphocyte population (depending on age, health status, and other factors) are more radiosensitive.
The reported in vitro data are less consistent than the in vivo results, but generally show the same ranking of radiosensitivity (B > T(CD8+) > T(CD4+) > NK) with response differences that are smaller than in vivo, i.e., average alpha values of ~0.4 Gy-1, ~0.3 Gy-1, and ~0.3 Gy-1 for B cells, T cells, and NK cells, respectively (for doses up to 3 Gy, after 4 hours to 4 days). One report shows significantly higher radiosensitivity for memory T cells. The fitted alpha values depend on the chosen dose range as most measured cell survival curves show a decreasing slope with increasing dose, i.e., a saturation typically starting already between 0.5 and 2 Gy. There is also a strong time dependence. Although radiation-induced apoptosis is measurable early after exposure, it continues to increase up to and beyond 48 hours, resulting in steeper dose-response curves. Many clinical studies on radiation-induced lymphopenia point out the importance of the dose to the circulating lymphocytes and refer to the high radiosensitivity of lymphocytes, often citing a single study (147). This widely cited study reports higher radiosensitivity than other studies and appears to be the only one showing a linear-quadratic dose response curve.
To assess the dose-response of lymphocytes in vivo for lymphopenia studies in patients, it is necessary to estimate the dose to lymphocytes. This work also reviews methods to estimate dose to the blood. While various models have been proposed to estimate the dose to the blood (based on reasonably well-known organ transit times of the blood), the dose to circulating lymphocytes is related but not identical to the blood dose. In addition to the recirculation of lymphocytes between blood and secondary lymphoid tissues, several factors cause lymphocyte transit times to be, on average, to be much longer than blood transit times. Lymphocytes often attach and detach from endothelial cells and radiation may cause upregulation of adhesion molecules that alter leucocyte adhesion to endothelial cells (171), which may increase mean transit times and thus dose to lymphocytes. In addition, they may have to deform to squeeze through capillaries because their size is much larger (~6 μm) than, for example, platelets (172, 173). In particular, pulmonary capillaries are thought to be slightly smaller than the diameter of lymphocytes (173). In the liver, a relatively low average velocity of T cells has been reported because they might be crawling on the endothelial wall of the sinusoids instead of flowing with the blood. This could reduce the average velocity to ~6-7 μm/min. Thus, CD8 T cells can super-diffuse in the liver for almost 20 minutes (172). It is therefore likely that the dose to the blood, while a potential surrogate for the dose to lymphocytes, does not accurately predict the correct dose experienced by circulating lymphocytes. Research efforts are underway to explicitly model lymphocyte trafficking rather than relying on the use of blood dose as a surrogate for dose to circulating lymphocytes (91, 92, 174).
While lymphocyte radiosensitivity is likely to play an important role in lymphopenia, radiation-induced effects such as cell survival or cell motility on lymphocytes are not necessarily robust predictors of immune suppression. Radiation also affects lymphocyte infiltration into tumors and tumor sensitization, increases antigen release, and other mechanisms (175). Zhao et al. (176) investigated lymphopenia in SBRT for early-stage lung cancer patients and concluded that lymphocyte radiosensitivity alone cannot explain lymphopenia without considering lymphocyte recovery times. The number of circulating lymphocytes might also decrease due to inflammation caused by low-dose baths in secondary irradiated organs during radiation therapy. In contrast to naïve adaptive lymphocytes which frequently migrate between secondary lymphoid organs, tissue-resident lymphocytes generally do not recirculate through the blood (177), but are also irradiated. The circulatory behavior of lymphocytes and their lymph node transit times also differs among lymphocytes subpopulations (178, 179), e.g., CD4+ seem to recirculate more rapidly compared to CD8+. In addition, radiation likely affects lymphocyte migration, for instance, through radiation-induced changes in sphingosine-1-phosphate (180). Lymphocyte depletion is also likely related to indirect mechanisms, such as radiation-induced expression of TNF-α which has a cytotoxic effect on lymphocytes (107, 181, 182). In addition, lymphocyte function appears to be affected at lower doses than cell survival in both in vitro and clinical studies (183–185).
5 Conclusions
The reported data suggest differences in radiation sensitivity among lymphocytes subpopulations, which may affect their relative contribution and thus the dynamics of the immune response. The data reviewed here show low dose (< 3Gy) radiosensitivity of lymphocytes in the same order of magnitude as normal fibroblasts (186). In general, B cells appear to be more radiosensitive than T cells, and NK cells appear to be the most resistant. Patient variability is likely to be of the same order of magnitude as the differences between subpopulations. Because tumor-infiltrating lymphocytes appear to be quite radioresistant, differences in radiosensitivity between circulating lymphocytes and lymphocytes in lymphoid organs may have implications for lymphopenia and thus for considerations of dose prescription and dose scheduling in radiation therapy as well as for fractionation and scheduling of therapies involving both radiation and immune checkpoint inhibitors. An important aspect is also the influence of radiation dose distribution, delivery time and beam arrangement, which has been discussed in the context of highly conformal radiotherapy and its positive effect on lymphopenia (114, 187).
It remains an open question whether the observed effects of radiation on lymphocyte counts in patients are indeed mainly due to the radiation sensitivity of circulating lymphocytes. To answer this question, it is necessary to consider not only the dose to different lymphocyte compartments in the field (e.g., the lymphatic system), but also radiation effects on lymphocyte trafficking and residence times (91, 92). Certainly, data on cell death don’t fully capture radiation-induced effects on lymphocyte functionality (135). This review outlines areas where additional research is needed to mechanistically explain radiation induced lymphopenia in patients and its correlation with treatment outcome.
Author contributions
The author confirms being the sole contributor of this work and has approved it for publication.
Funding
This work was supported by the National Institute of Health/National Cancer Institute: P01 CA261669 and R01 CA248901.
Acknowledgments
The author wishes to thank Ryan J Park, MD PhD, Massachusetts General Hospital, for reviewing general statements about lymphocytes and the immune system.
Conflict of interest
The author declares that the research was conducted in the absence of any commercial or financial relationships that could be construed as a potential conflict of interest.
Publisher’s note
All claims expressed in this article are solely those of the authors and do not necessarily represent those of their affiliated organizations, or those of the publisher, the editors and the reviewers. Any product that may be evaluated in this article, or claim that may be made by its manufacturer, is not guaranteed or endorsed by the publisher.
References
1. Heier HE, Christensen I, Froland SS, Engeset A. Early and late effects of irradiation for seminoma testis on the number of blood lymphocytes and their B and T subpopulations. Lymphology (1975) 8:69–74.
2. Weeke E. The development of lymphopenia in uremic patients undergoing extracorporeal irradiation of the blood with portable beta units. Radiat Res (1973) 56:554–9. doi: 10.2307/3573724
3. MacLennan IC, Kay HE. Analysis of treatment in childhood leukemia. IV. The critical association between dose fractionation and immunosuppression induced by cranial irradiation. Cancer (1978) 41:108–11. doi: 10.1002/1097-0142(197801)41:1<108::AID-CNCR2820410116>3.0.CO;2-Z
4. Grossman SA, Ellsworth S, Campian J, Wild AT, Herman JM, Laheru D, et al. Survival in patients with severe lymphopenia following treatment with radiation and chemotherapy for newly diagnosed solid tumors. J Natl Compr Cancer Network JNCCN (2015) 13:1225–31. doi: 10.6004/jnccn.2015.0151
5. Ellsworth SG. Field size effects on the risk and severity of treatment-induced lymphopenia in patients undergoing radiation therapy for solid tumors. Adv Radiat Oncol (2018) 3:512–9. doi: 10.1016/j.adro.2018.08.014
6. Fang P, Shiraishi Y, Verma V, Jiang W, Song J, Hobbs BP, et al. Lymphocyte-Sparing effect of proton therapy in patients with esophageal cancer treated with definitive chemoradiation. Int J Part Ther (2018) 4:23–32. doi: 10.14338/IJPT-17-00033.1
7. Yovino S, Kleinberg L, Grossman SA, Narayanan M, Ford E. The etiology of treatment-related lymphopenia in patients with malignant gliomas: modeling radiation dose to circulating lymphocytes explains clinical observations and suggests methods of modifying the impact of radiation on immune cells. Cancer Invest (2013) 31:140–4. doi: 10.3109/07357907.2012.762780
8. Wild AT, Herman JM, Dholakia AS, Moningi S, Lu Y, Rosati LM, et al. Lymphocyte-Sparing effect of stereotactic body radiation therapy in patients with unresectable pancreatic cancer. Int Jornal Radiat Oncol Biol Phys (2016) 94:571–9. doi: 10.1016/j.ijrobp.2015.11.026
9. Damen PJJ, Kroese TE, van Hillegersberg R, Schuit E, Peters M, Verhoeff JJC, et al. The influence of severe radiation-induced lymphopenia on overall survival in solid tumors: A systematic review and meta-analysis. Int J Radiat Oncol Biol Phys (2021) 111:936–48. doi: 10.1016/j.ijrobp.2021.07.1695
10. Shiraishi Y, Fang P, Xu C, Song J, Krishnan S, Koay EJ, et al. Severe lymphopenia during neoadjuvant chemoradiation for esophageal cancer: A propensity matched analysis of the relative risk of proton versus photon-based radiation therapy. Radiother Oncol (2018) 128:154–60. doi: 10.1016/j.radonc.2017.11.028
11. Chen F, Jin JY, Hui TSK, Jing H, Zhang H, Nong Y, et al. Radiation induced lymphopenia is associated with the effective dose to the circulating immune cells in breast cancer. Front Oncol (2022) 12:768956. doi: 10.3389/fonc.2022.768956
12. Monti S, Xu T, Liao Z, Mohan R, Cella L, Palma G. On the interplay between dosiomics and genomics in radiation-induced lymphopenia of lung cancer patients. Radiother Oncol (2022) 167:219–25. doi: 10.1016/j.radonc.2021.12.038
13. Qian JM, Akama-Garren E, Shin J, Gunasti L, Bang A, Pike LRG, et al. Dosimetric modeling of lymphopenia in patients with metastatic cancer receiving palliative radiation and PD-1 immune checkpoint inhibitors. Adv Radiat Oncol (2022) 7:100880. doi: 10.1016/j.adro.2021.100880
14. Correia D, Terribilini D, Zepter S, Pica A, Bizzocchi N, Volken W, et al. Whole-ventricular irradiation for intracranial germ cell tumors: Dosimetric comparison of pencil beam scanned protons, intensity-modulated radiotherapy and volumetric-modulated arc therapy. Clin Transl Radiat Oncol (2019) 15:53–61. doi: 10.1016/j.ctro.2019.01.002
15. Tang C, Liao Z, Gomez D, Levy L, Zhuang Y, Gebremichael RA, et al. Lymphopenia association with gross tumor volume and lung V5 and its effects on non-small cell lung cancer patient outcomes. Int J Radiat Oncol Biol Phys (2014) 89:1084–91. doi: 10.1016/j.ijrobp.2014.04.025
16. Helm A, Ebner DK, Tinganelli W, Simoniello P, Bisio A, Marchesano V, et al. Combining heavy-ion therapy with immunotherapy: An update on recent developments. Int J Particle Ther (2018) 5:84–93. doi: 10.14338/IJPT-18-00024.1
17. Gameiro SR, Malamas AS, Bernstein MB, Tsang KY, Vassantachart A, Sahoo N, et al. Tumor cells surviving exposure to proton or photon radiation share a common immunogenic modulation signature, rendering them more sensitive to T cell-mediated killing. Int J Radiat Oncol Biol Phys (2016) 95:120–30. doi: 10.1016/j.ijrobp.2016.02.022
18. Ko EC, Benjamin KT, Formenti SC. Generating antitumor immunity by targeted radiation therapy: Role of dose and fractionation. Adv Radiat Oncol (2018) 3:486–93. doi: 10.1016/j.adro.2018.08.021
19. Routman DM, Garant A, Lester SC, Day CN, Harmsen WS, Sanheuza CT, et al. A comparison of grade 4 lymphopenia with proton versus photon radiation therapy for esophageal cancer. Adv Radiat Oncol (2019) 4:63–9. doi: 10.1016/j.adro.2018.09.004
20. Davuluri R, Jiang W, Fang P, Xu C, Komaki R, Gomez DR, et al. Lymphocyte nadir and esophageal cancer survival outcomes after chemoradiation therapy. Int J Radiat Oncol Biol Phys (2017) 99:128–35. doi: 10.1016/j.ijrobp.2017.05.037
21. Jensen GL, Blanchard P, Gunn GB, Garden AS, David Fuller C, Sturgis EM, et al. Prognostic impact of leukocyte counts before and during radiotherapy for oropharyngeal cancer. Clin Transl Radiat Oncol (2017) 7:28–35. doi: 10.1016/j.ctro.2017.09.008
22. Abdullah M, Chai PS, Chong MY, Tohit ER, Ramasamy R, Pei CP, et al. Gender effect on in vitro lymphocyte subset levels of healthy individuals. Cell Immunol (2012) 272:214–9. doi: 10.1016/j.cellimm.2011.10.009
23. Shin J, Xing S, McCullum L, Hammi A, Pursley J, Correa CA, et al. HEDOS-a computational tool to assess radiation dose to circulating blood cells during external beam radiotherapy based on whole-body blood flow simulations. Phys Med Biol (2021) 66:164001. doi: 10.1088/1361-6560/ac16ea
24. Xing S, Shin J, Pursley J, Correa-Alfonso CM, Depauw N, Domal S, et al. A dynamic blood flow model to compute absorbed dose to circulating blood and lymphocytes in liver external beam radiotherapy. Phys Med Biol (2022) 67:045010. doi: 10.1088/1361-6560/ac4da4
25. Plowman PN. The effects of conventionally fractionated, extended portal radiotherapy on the human peripheral blood count. Int J Radiat Oncol Biol Phys (1983) 9:829–39. doi: 10.1016/0360-3016(83)90008-1
26. Crocenzi T, Cottam B, Newell P, Wolf RF, Hansen PD, Hammill C, et al. A hypofractionated radiation regimen avoids the lymphopenia associated with neoadjuvant chemoradiation therapy of borderline resectable and locally advanced pancreatic adenocarcinoma. J Immunother Cancer (2016) 4:45. doi: 10.1186/s40425-016-0149-6
27. Dewan MZ, Galloway AE, Kawashima N, Dewyngaert JK, Babb JS, Formenti SC, et al. Fractionated but not single-dose radiotherapy induces an immune-mediated abscopal effect when combined with anti-CTLA-4 antibody. Clin Cancer Res (2009) 15:5379–88. doi: 10.1158/1078-0432.CCR-09-0265
28. Lambin P, Lieverse RIY, Eckert F, Marcus D, Oberije C, van der Wiel AMA, et al. Lymphocyte-sparing radiotherapy: The rationale for protecting lymphocyte-rich organs when combining radiotherapy with immunotherapy. Semin Radiat Oncol (2020) 30:187–93. doi: 10.1016/j.semradonc.2019.12.003
29. Lee R, Yamada S, Yamamoto N, Miyamoto T, Ando K, Durante M, et al. Chromosomal aberrations in lymphocytes of lung cancer patients treated with carbon ions. J Radiat Res (2004) 45:195–9. doi: 10.1269/jrr.45.195
30. Rudra S, Hui C, Rao YJ, Samson P, Lin AJ, Chang X, et al. Effect of radiation treatment volume reduction on lymphopenia in patients receiving chemoradiotherapy for glioblastoma. Int J Radiat Oncol Biol Phys (2018) 101:217–25. doi: 10.1016/j.ijrobp.2018.01.069
31. Yang L, Xu Z, Ma L, Liu Q, Chang ATY, Wang Q, et al. Early onset of severe lymphopenia during definitive radiotherapy correlates with mean body dose and predicts poor survival in cervical cancer. Cancer biomark (2022) 34:149–59. doi: 10.3233/CBM-210292
32. Zhang BZ, Li Y, Xu LM, Chai YL, Qu C, Cao YJ, et al. The relationship between the radiation dose of pelvic-bone marrow and lymphocytic toxicity in concurrent chemoradiotherapy for cervical cancer. Radiat Oncol (2023) 18:12. doi: 10.1186/s13014-023-02205-8
33. Liu J, Zhao Q, Deng W, Lu J, Xu X, Wang R, et al. Radiation-related lymphopenia is associated with spleen irradiation dose during radiotherapy in patients with hepatocellular carcinoma. Radiat Oncol (2017) 12:90. doi: 10.1186/s13014-017-0824-x
34. d'Alesio V, Pacelli R, Durante M, Canale Cama G, Cella L, Gialanella G, et al. Lymph nodes in the irradiated field influence the yield of radiation-induced chromosomal aberrations in lymphocytes from breast cancer patients. Int J Radiat Oncol Biol Phys (2003) 57:732–8. doi: 10.1016/S0360-3016(03)00664-3
35. Chadha AS, Liu G, Chen HC, Das P, Minsky BD, Mahmood U, et al. Does unintentional splenic radiation predict outcomes after pancreatic cancer radiation therapy? Int J Radiat Oncol Biol Phys (2017) 97:323–32. doi: 10.1016/j.ijrobp.2016.10.046
36. Saito T, Toya R, Yoshida N, Shono T, Matsuyama T, Ninomura S, et al. Spleen dose-volume parameters as a predictor of treatment-related lymphopenia during definitive chemoradiotherapy for esophageal cancer. In Vivo (2018) 32:1519–25. doi: 10.21873/invivo.11409
37. Yalamanchali A, Zhang H, Huang KC, Mohan R, Lin SH, Zhu C, et al. Patient-specific lymphocyte loss kinetics as biomarker of spleen dose in patients undergoing radiation therapy for upper abdominal malignancies. Adv Radiat Oncol (2021) 6:100545. doi: 10.1016/j.adro.2020.08.002
38. Xu H, You G, Zhang M, Song T, Zhang H, Yang J, et al. Association of pre-surgery to pre-radiotherapy lymphocyte counts ratio with disease-free survival in rectal cancer patients receiving neoadjuvant concurrent chemoradiotherapy. World J Surg Oncol (2019) 17:199. doi: 10.1186/s12957-019-1747-9
39. Anderson JL, Newman NB, Anderson C, Sherry AD, Yock AD, Osmundson EC. Mean cardiopulmonary dose and vertebral marrow dose differentially predict lineage-specific leukopenia kinetics during radiotherapy for esophageal cancer. Radiother Oncol (2020) 152:169–76. doi: 10.1016/j.radonc.2019.12.008
40. Qing H, Chuanshu C, Weijian Z, Shaobin Z, Jing L. Low-dose range of pelvic irradiation leads to acute hematological toxicity in early-stage cervical cancer with intermediate risk factors by postoperative intensity-modulated radiotherapy. Eur J gynaecological Oncol (2019) 40:437–42. doi: 10.12892/ejgo4654.2019
41. Sini C, Fiorino C, Perna L, Noris Chiorda B, Deantoni CL, Bianchi M, et al. Dose-volume effects for pelvic bone marrow in predicting hematological toxicity in prostate cancer radiotherapy with pelvic node irradiation. Radiother Oncol (2016) 118:79–84. doi: 10.1016/j.radonc.2015.11.020
42. Eckert F, Schaedle P, Zips D, Schmid-Horch B, Rammensee HG, Gani C, et al. Impact of curative radiotherapy on the immune status of patients with localized prostate cancer. Oncoimmunology (2018) 7:e1496881. doi: 10.1080/2162402X.2018.1496881
43. Standish LJ, Torkelson C, Hamill FA, Yim D, Hill-Force A, Fitzpatrick A, et al. Immune defects in breast cancer patients after radiotherapy. J Soc Integr Oncol (2008) 6:110–21.
44. Venkatesulu B, Giridhar P, Pujari L, Chou B, Lee JH, Block AM, et al. Lymphocyte sparing normal tissue effects in the clinic (LymphoTEC): A systematic review of dose constraint considerations to mitigate radiation-related lymphopenia in the era of immunotherapy. Radiother Oncol (2022) 177:81–94. doi: 10.1016/j.radonc.2022.10.019
45. Waidhauser J, Nerlinger P, Arndt TT, Schiele S, Sommer F, Wolf S, et al. Alterations of circulating lymphocyte subsets in patients with colorectal carcinoma. Cancer Immunol Immunother (2022) 71:1937–47. doi: 10.1007/s00262-021-03127-8
46. Gustafson MP, Bornschlegl S, Park SS, Gastineau DA, Roberts LR, Dietz AB, et al. Comprehensive assessment of circulating immune cell populations in response to stereotactic body radiation therapy in patients with liver cancer. Adv Radiat Oncol (2017) 2:540–7. doi: 10.1016/j.adro.2017.08.003
47. Kaur P, Asea A. Radiation-induced effects and the immune system in cancer. Front Oncol (2012) 2:191. doi: 10.3389/fonc.2012.00191
48. Ahmed MM, Hodge JW, Guha C, Bernhard EJ, Vikram B, Coleman CN. Harnessing the potential of radiation-induced immune modulation for cancer therapy. Cancer Immunol Res (2013) 1:280–4. doi: 10.1158/2326-6066.CIR-13-0141
49. Demaria S, Ng B, Devitt ML, Babb JS, Kawashima N, Liebes L, et al. Ionizing radiation inhibition of distant untreated tumors (abscopal effect) is immune mediated. Int J Radiat Oncol Biol Phys (2004) 58:862–70. doi: 10.1016/j.ijrobp.2003.09.012
50. Rubner Y, Wunderlich R, Ruhle PF, Kulzer L, Werthmoller N, Frey B, et al. How does ionizing irradiation contribute to the induction of anti-tumor immunity? Front Oncol (2012) 2:75. doi: 10.3389/fonc.2012.00075
51. Park B, Yee C, Lee KM. The effect of radiation on the immune response to cancers. Int J Mol Sci (2014) 15:927–43. doi: 10.3390/ijms15010927
52. Liu R, Xiong S, Zhang L, Chu Y. Enhancement of antitumor immunity by low-dose total body irradiationis associated with selectively decreasing the proportion and number of T regulatory cells. Cell Mol Immunol (2010) 7:157–62. doi: 10.1038/cmi.2009.117
53. Storozynsky Q, Hitt MM. The impact of radiation-induced DNA damage on cGAS-STING-mediated immune responses to cancer. Int J Mol Sci (2020) 21:8877. doi: 10.3390/ijms21228877
54. Tuomela K, Mukherjee D, Ambrose AR, Harikrishnan A, Mole H, Hurlstone A, et al. Radiotherapy transiently reduces the sensitivity of cancer cells to lymphocyte cytotoxicity. Proc Natl Acad Sci USA (2022) 119:e2111900119. doi: 10.1073/pnas.2111900119
55. Abdel-Rahman O. Nonconventional patterns of benefit of solid tumors treated with PD-(L)1 inhibitors: a systematic review. Immunotherapy (2017) 9:995–1004. doi: 10.2217/imt-2017-0074
56. Kalbasi A, June CH, Haas N, Vapiwala N. Radiation and immunotherapy: a synergistic combination. J Clin Invest (2013) 123:2756–63. doi: 10.1172/JCI69219
57. Salama AK, Postow MA, Salama JK. Irradiation and immunotherapy: From concept to the clinic. Cancer (2016) 122:1659–71. doi: 10.1002/cncr.29889
58. Seyedin SN, Schoenhals JE, Lee DA, Cortez MA, Wang X, Niknam S, et al. Strategies for combining immunotherapy with radiation for anticancer therapy. Immunotherapy (2015) 7:967–80. doi: 10.2217/imt.15.65
59. Durante M, Reppingen N, Held KD. Immunologically augmented cancer treatment using modern radiotherapy. Trends Mol Med (2013) 19:565–82. doi: 10.1016/j.molmed.2013.05.007
60. Levy A, Chargari C, Cheminant M, Simon N, Bourgier C, Deutsch E. Radiation therapy and immunotherapy: implications for a combined cancer treatment. Crit Rev oncology/hematol (2013) 85:278–87. doi: 10.1016/j.critrevonc.2012.09.001
61. Wang Y, Deng W, Li N, Sharma A, Jiang W, Lin SH. Combining immunotherapy and radiotherapy for cancer treatment: Current challenges and future directions. Frotiers Pharmacol (2018) 9:185. doi: 10.3389/fphar.2018.00185
62. Vatner RE, Cooper BT, Vanpouille-Box C, Demaria S, Formenti SC. Combinations of immunotherapy and radiation in cancer therapy. Front Oncol (2014) 4:325. doi: 10.3389/fonc.2014.00325
63. Barker CA, Postow MA. Combinations of radiation therapy and immunotherapy for melanoma: a review of clinical outcomes. Int J Radiat Oncol Biol Phys (2014) 88:986–97. doi: 10.1016/j.ijrobp.2013.08.035
64. Tang C, Wang X, Soh H, Seyedin S, Cortez MA, Krishnan S, et al. Combining radiation and immunotherapy: a new systemic therapy for solid tumors? Cancer Immunol Res (2014) 2:831–8. doi: 10.1158/2326-6066.CIR-14-0069
65. Patel MA, Kim JE, Ruzevick J, Li G, Lim M. The future of glioblastoma therapy: synergism of standard of care and immunotherapy. Cancers (2014) 6:1953–85. doi: 10.3390/cancers6041953
66. Matsumura S, Wang B, Kawashima N, Braunstein S, Badura M, Cameron TO, et al. Radiation-induced CXCL16 release by breast cancer cells attracts effector T cells. J Immunol (2008) 181:3099–107. doi: 10.4049/jimmunol.181.5.3099
67. Demaria S, Pilones KA, Vanpouille-Box C, Golden EB, Formenti SC. The optimal partnership of radiation and immunotherapy: from preclinical studies to clinical translation. Radiat Res (2014) 182:170–81. doi: 10.1667/RR13500.1
68. Bhattacharyya T, Purushothaman K, Puthiyottil SS, Bhattacharjee A, Muttah G. Immunological interactions in radiotherapy-opening a new window of opportunity. Ann Transl Med (2016) 4:51. doi: 10.3978/j.issn.2305-5839.2015.10.44
69. Young KH, Baird JR, Savage T, Cottam B, Friedman D, Bambina S, et al. Optimizing timing of immunotherapy improves control of tumors by hypofractionated radiation therapy. PloS One (2016) 11:e0157164. doi: 10.1371/journal.pone.0157164
70. Verma V, Cushman TR, Tang C, Welsh JW. Toxicity of radiation and immunotherapy combinations. Adv Radiat Oncol (2018) 3:506–11. doi: 10.1016/j.adro.2018.08.003
71. Gunderson AJ, Young KH. Exploring optimal sequencing of radiation and immunotherapy combinations. Adv Radiat Oncol (2018) 3:494–505. doi: 10.1016/j.adro.2018.07.005
72. Pike LRG, Bang A, Mahal BA, Taylor A, Krishnan M, Spektor A, et al. The impact of radiation therapy on lymphocyte count and survival in metastatic cancer patients receiving PD-1 immune checkpoint inhibitors. Int J Radiat Oncol Biol Phys (2019) 103:142–51. doi: 10.1016/j.ijrobp.2018.09.010
73. Lee HJ Jr., Zeng J, Rengan R. Proton beam therapy and immunotherapy: an emerging partnership for immune activation in non-small cell lung cancer. Transl Lung Cancer Res (2018) 7:180–8. doi: 10.21037/tlcr.2018.03.28
74. Tsuboi K. Advantages and limitations in the use of combination therapies with charged particle radiation therapy. Int J Particle Ther (2018) 5:122–32. doi: 10.14338/IJPT-18-00019.1
75. Miszczyk J, Rawojc K, Panek A, Borkowska A, Prasanna PGS, Ahmed MM, et al. Do protons and X-rays induce cell-killing in human peripheral blood lymphocytes by different mechanisms? Clin Transl Radiat Oncol (2018) 9:23–9. doi: 10.1016/j.ctro.2018.01.004
76. Ebner DK, Tinganelli W, Helm A, Bisio A, Yamada S, Kamada T, et al. The immunoregulatory potential of particle radiation in cancer therapy. Front Immunol (2017) 8:99. doi: 10.3389/fimmu.2017.00099
77. Marciscano AE, Ghasemzadeh A, Nirschl TR, Theodros D, Kochel CM, Francica BJ, et al. Elective nodal irradiation attenuates the combinatorial efficacy of stereotactic radiation therapy and immunotherapy. Clin Cancer Res (2018) 24:5058–71. doi: 10.1158/1078-0432.CCR-17-3427
78. Crittenden M, Kohrt H, Levy R, Jones J, Camphausen K, Dicker A, et al. Current clinical trials testing combinations of immunotherapy and radiation. Semin Radiat Oncol (2015) 25:54–64. doi: 10.1016/j.semradonc.2014.07.003
79. Molloy JA. Statistical analysis of dose heterogeneity in circulating blood: implications for sequential methods of total body irradiation. Med Phys (2010) 37:5568–78. doi: 10.1118/1.3495816
80. Basler L, Andratschke N, Ehrbar S, Guckenberger M, Tanadini-Lang S. Modelling the immunosuppressive effect of liver SBRT by simulating the dose to circulating lymphocytes: an in-silico planning study. Radiat Oncol (2018) 13:10. doi: 10.1186/s13014-018-0952-y
81. Jin JY, Hu C, Xiao Y, Zhang H, Paulus R, Ellsworth SG, et al. Higher radiation dose to the immune cells correlates with worse tumor control and overall survival in patients with stage III NSCLC: A secondary analysis of RTOG0617. Cancers (2021) 13:6193. doi: 10.3390/cancers13246193
82. Ladbury CJ, Rusthoven CG, Camidge DR, Kavanagh BD, Nath SK. Impact of radiation dose to the host immune system on tumor control and survival for stage III non-small cell lung cancer treated with definitive radiation therapy. Int J Radiat Oncol Biol Phys (2019) 105:346–55. doi: 10.1016/j.ijrobp.2019.05.064
83. So TH, Chan SK, Chan WL, Choi H, Chiang CL, Lee V, et al. Lymphopenia and radiation dose to circulating lymphocytes with neoadjuvant chemoradiation in esophageal squamous cell carcinoma. Adv Radiat Oncol (2020) 5:880–8. doi: 10.1016/j.adro.2020.03.021
84. Xu C, Jin JY, Zhang M, Liu A, Wang J, Mohan R, et al. The impact of the effective dose to immune cells on lymphopenia and survival of esophageal cancer after chemoradiotherapy. Radiother Oncol (2020) 146:180–6. doi: 10.1016/j.radonc.2020.02.015
85. Cruz-Garcia L, Nasser F, O'Brien G, Grepl J, Vinnikov V, Starenkiy V, et al. Transcriptional dynamics of DNA damage responsive genes in circulating leukocytes during radiotherapy. Cancers (2022) 14:2649. doi: 10.3390/cancers14112649
86. O'Brien G, Cruz-Garcia L, Majewski M, Grepl J, Abend M, Port M, et al. FDXR is a biomarker of radiation exposure in vivo. Sci Rep (2018) 8:684. doi: 10.1038/s41598-017-19043-w
87. Hammi A, Paganetti H, Grassberger C. 4D blood flow model for dose calculation to circulating blood and lymphocytes. Phys Med Biol (2020) 65:055008. doi: 10.1088/1361-6560/ab6c41
88. Correa-Alfonso CM, Withrow JD, Domal SJ, Xing S, Shin J, Grassberger C, et al. A mesh-based model of liver vasculature: implications for improved radiation dosimetry to liver parenchyma for radiopharmaceuticals. EJNMMI Phys (2022) 9:28. doi: 10.1186/s40658-022-00456-0
89. Correa-Alfonso CM, Withrow JD, Domal SJ, President B, Dawson R, McCullum L, et al. Intra-brain vascular models within the ICRP mesh-type adult reference phantoms for applications to internal dosimetry. Phys Med Biol (2023) 68:105001. doi: 10.1088/1361-6560/acc926
90. Withrow JD, Pathak SP, Correa-Alfonso CM, Dawson RJ, Domal SJ, Beekman C, et al. Heart and lung vascular models within the adult mesh-type reference computational phantoms for applications to internal dosimetry. . Med Phys (2023) 50:e533.
91. Ganusov VV, Auerbach J. Mathematical modeling reveals kinetics of lymphocyte recirculation in the whole organism. PloS Comput Biol (2014) 10:e1003586. doi: 10.1371/journal.pcbi.1003586
92. McDaniel MM, Ganusov VV. Estimating residence times of lymphocytes in ovine lymph nodes. Front Immunol (2019) 10:1492. doi: 10.3389/fimmu.2019.01492
93. Jin J-Y, Mereniuk T, Yalamanchali A, Wang W, Machtay M, Spring, Kong F-M, et al. A framework for modeling radiation induced lymphopenia in radiotherapy. Radiother Oncol (2020) 144:105–13. doi: 10.1016/j.radonc.2019.11.014
94. Friedrich T, Scholz M, Durante M. A predictive biophysical model of the combined action of radiation therapy and immunotherapy of cancer. Int J Radiat Oncol Biol Phys (2022) 113:872–84. doi: 10.1016/j.ijrobp.2022.03.030
95. Cucinotta FA, Smirnova OA. Effects of flash radiotherapy on blood lymphocytes in humans and small laboratory animals. Radiat Res (2023) 199:240–51. doi: 10.1667/RADE-22-00093.1
96. Heylmann D, Badura J, Becker H, Fahrer J, Kaina B. Sensitivity of CD3/CD28-stimulated versus non-stimulated lymphocytes to ionizing radiation and genotoxic anticancer drugs: key role of ATM in the differential radiation response. Cell Death Dis (2018) 9:1053. doi: 10.1038/s41419-018-1095-7
97. Heylmann D, Ponath V, Kindler T, Kaina B. Comparison of DNA repair and radiosensitivity of different blood cell populations. Sci Rep (2021) 11:2478. doi: 10.1038/s41598-021-81058-1
98. Bauer M, Goldstein M, Christmann M, Becker H, Heylmann D, Kaina B. Human monocytes are severely impaired in base and DNA double-strand break repair that renders them vulnerable to oxidative stress. Proc Natl Acad Sci U.S.A. (2011) 108:21105–10. doi: 10.1073/pnas.1111919109
99. Konig L, Hommertgen A, Orschiedt L, Horner-Rieber J, Brons S, Huber PE, et al. Influence of photon, proton and carbon ion irradiation on differentiation, maturation and functionality of dendritic cells. Front Biosci (Schol Ed) (2022) 14:2. doi: 10.31083/j.fbs1401002
100. Merrick A, Errington F, Milward K, O'Donnell D, Harrington K, Bateman A, et al. Immunosuppressive effects of radiation on human dendritic cells: reduced IL-12 production on activation and impairment of naive T-cell priming. Br J Cancer (2005) 92:1450–8. doi: 10.1038/sj.bjc.6602518
101. Rathmell JC, Thompson CB. Pathways of apoptosis in lymphocyte development, homeostasis, and disease. Cell (2002) 109 Suppl:S97–107. doi: 10.1016/S0092-8674(02)00704-3
102. Belka C, Heinrich V, Marini P, Faltin H, Schulze-Osthoff K, Bamberg M, et al. Ionizing radiation and the activation of caspase-8 in highly apoptosis-sensitive lymphoma cells. Int J Radiat Biol (1999) 75:1257–64. doi: 10.1080/095530099139412
103. Vinnikov V, Hande MP, Wilkins R, Wojcik A, Zubizarreta E, Belyakov O. Prediction of the acute or late radiation toxicity effects in radiotherapy patients using ex vivo induced biodosimetric markers: A review. J Pers Med (2020) 10:285. doi: 10.3390/jpm10040285
104. Yang SJ, Rafla S, Youssef E, Selim H, Salloum N, Chuang JY. Changes in T-cell subsets after radiation therapy. Radiology (1988) 168:537–40. doi: 10.1148/radiology.168.2.3260678
105. Clave E, Socie G, Cosset JM, Chaillet MP, Tartour E, Girinsky T, et al. Multicolor flow cytometry analysis of blood cell subsets in patients given total body irradiation before bone marrow transplantation. Int J Radiat Oncol Biol Phys (1995) 33:881–6. doi: 10.1016/0360-3016(95)00213-6
106. Louagie H, Van Eijkeren M, Philippe J, Thierens H, de Ridder L. Changes in peripheral blood lymphocyte subsets in patients undergoing radiotherapy. Int J Radiat Biol (1999) 75:767–71. doi: 10.1080/095530099140113
107. Belka C, Ottinger H, Kreuzfelder E, Weinmann M, Lindemann M, Lepple-Wienhues A, et al. Impact of localized radiotherapy on blood immune cells counts and function in humans. Radiother Oncol (1999) 50:199–204. doi: 10.1016/S0167-8140(98)00130-3
108. Zhao Q, Li T, Chen G, Zeng Z, He J. Prognosis and risk factors of radiation-induced lymphopenia in early-stage lung cancer treated with stereotactic body radiation therapy. Front Oncol (2019) 9:1488. doi: 10.3389/fonc.2019.01488
109. McCullum L, Shin J, Xing S, Beekman C, Schuemann J, Hong T, et al. Predicting severity of radiation induced lymphopenia in individual proton therapy patients for varying dose rate and fractionation using dynamic 4D blood flow simulations. Int Jornal Radiat Oncol Biol Phys press (2023) 116:1226–33. doi: 10.1016/j.ijrobp.2023.01.054
110. Trowell OA. The sensitivity of lymphocytes to ionising radiation. J Pathol Bacteriol (1952) 64:687–704. doi: 10.1002/path.1700640403
111. Girinsky T, Socie G, Cosset JM, Malaise EP. Blood lymphocyte subsets after the first fraction in patients given hyperfractionated total body irradiation for bone marrow transplantation. Br J Cancer (1991) 63:646–7. doi: 10.1038/bjc.1991.148
112. Akiyama M. Late effects of radiation on the human immune system: an overview of immune response among the atomic-bomb survivors. Int J Radiat Biol (1995) 68:497–508. doi: 10.1080/09553009514551491
113. McGee HM, Daly ME, Azghadi S, Stewart SL, Oesterich L, Schlom J, et al. Stereotactic ablative radiation therapy induces systemic differences in peripheral blood immunophenotype dependent on irradiated site. Int J Radiat Oncol Biol Phys (2018) 101:1259–70. doi: 10.1016/j.ijrobp.2018.04.038
114. D'Auria F, Statuto T, Rago L, Montagna A, Castaldo G, Schiro I, et al. Modulation of peripheral immune cell subpopulations after rapidArc/moderate hypofractionated radiotherapy for localized prostate cancer: findings and comparison with 3D conformal/conventional fractionation treatment. Front Oncol (2022) 12:829812. doi: 10.3389/fonc.2022.829812
115. Yang ZR, Zhao N, Meng J, Shi ZL, Li BX, Wu XW, et al. Peripheral lymphocyte subset variation predicts prostate cancer carbon ion radiotherapy outcomes. Oncotarget (2016) 7:26422–35. doi: 10.18632/oncotarget.8389
116. Sage EK, Schmid TE, Geinitz H, Gehrmann M, Sedelmayr M, Duma MN, et al. Effects of definitive and salvage radiotherapy on the distribution of lymphocyte subpopulations in prostate cancer patients. Strahlenther Onkol (2017) 193:648–55. doi: 10.1007/s00066-017-1144-7
117. Sage EK, Schmid TE, Sedelmayr M, Gehrmann M, Geinitz H, Duma MN, et al. Comparative analysis of the effects of radiotherapy versus radiotherapy after adjuvant chemotherapy on the composition of lymphocyte subpopulations in breast cancer patients. Radiother Oncol (2016) 118:176–80. doi: 10.1016/j.radonc.2015.11.016
118. Yuan C, Wang Q. Comparative analysis of the effect of different radiotherapy regimes on lymphocyte and its subpopulations in breast cancer patients. Clin Trans Oncol (2018) 20:1219–25. doi: 10.1007/s12094-018-1851-2
119. Schaue D, Comin-Anduix B, Ribas A, Zhang L, Goodglick L, Sayre JW, et al. T-cell responses to survivin in cancer patients undergoing radiation therapy. Clin Cancer Res (2008) 14:4883–90. doi: 10.1158/1078-0432.CCR-07-4462
120. Beauford SS, Kumari A, Garnett-Benson C. Ionizing radiation modulates the phenotype and function of human CD4+ induced regulatory T cells. BMC Immunol (2020) 21:18. doi: 10.1186/s12865-020-00349-w
121. Anderson RE, Sprent J, Miller JF. Radiosensitivity of T and B lymphocytes. I. Effect of irradiation on cell migration. Eur J Immunol (1974) 4:199–203. doi: 10.1002/eji.1830040309
122. Kataoka Y, Sado T. The radiosensitivity of T and B lymphocytes in mice. Immunology (1975) 29:121–30.
123. Durum SK, Gengozian N. The comparative radiosensitivity of T and B lymphocytes. Int J Radiat Biol Relat Stud Phys Chem Med (1978) 34:1–15. doi: 10.1080/09553007814550581
124. Sado T, Kamisaku H, Ikarashi Y, Kubo E. Immediate and long-term effects of radiation on the immune system of specific-pathogen-free mice. Int J Radiat Biol Relat Stud Phys Chem Med (1988) 53:177–87. doi: 10.1080/09553008814550531
125. Harrington NP, Chambers KA, Ross WM, Filion LG. Radiation damage and immune suppression in splenic mononuclear cell populations. Clin Exp Immunol (1997) 107:417–24. doi: 10.1111/j.1365-2249.1997.272-ce1158.x
126. Chambers KA, Harrington NP, Ross WM, Filion LG. Relative alterations in blood mononuclear cell populations reflect radiation injury in mice. Cytometry (1998) 31:45–52. doi: 10.1002/(SICI)1097-0320(19980101)31:1<45::AID-CYTO6>3.0.CO;2-I
127. Kajioka EH, A. ML, Li J, Mao XW, Moyers MF, Nelson GA, et al. Acute effects of whole-ody proton irradiation on the immune system of the mouse. Radiat Res (2000) 153:587–94. doi: 10.1667/0033-7587(2000)153[0587:AEOWBP]2.0.CO;2
128. Grayson JM, Harrington LE, Lanier JG, Wherry EJ, Ahmed R. Differential sensitivity of naive and memory CD8+ T cells to apoptosis in vivo. J Immunol (2002) 169:3760–70. doi: 10.4049/jimmunol.169.7.3760
129. Garg S, Boerma M, Wang J, Fu Q, Loose DS, Kumar KS, et al. Influence of sublethal total-body irradiation on immune cell populations in the intestinal mucosa. Radiat Res (2010) 173:469–78. doi: 10.1667/RR1742.1
130. Bogdandi EN, Balogh A, Felgyinszki N, Szatmari T, Persa E, Hildebrandt G, et al. Effects of low-dose radiation on the immune system of mice after total-body irradiation. Radiat Res (2010) 174:480–9. doi: 10.1667/RR2160.1
131. Qu Y, Zhang B, Liu S, Zhang A, Wu T, Zhao Y. 2-Gy whole-body irradiation significantly alters the balance of CD4+ CD25- T effector cells and CD4+ CD25+ Foxp3+ T regulatory cells in mice. Cell Mol Immunol (2010) 7:419–27. doi: 10.1038/cmi.2010.45
132. Qu Y, Jin S, Zhang A, Zhang B, Shi X, Wang J, et al. Gamma-ray resistance of regulatory CD4+CD25+Foxp3+ T cells in mice. Radiat Res (2010) 173:148–57. doi: 10.1667/RR0978.1
133. Yao Z, Jones J, Kohrt H, Strober S. Selective resistance of CD44hi T cells to p53-dependent cell death results in persistence of immunologic memory after total body irradiation. J Immunol (2011) 187:4100–8. doi: 10.4049/jimmunol.1101141
134. Kachikwu EL, Iwamoto KS, Liao YP, DeMarco JJ, Agazaryan N, Economou JS, et al. Radiation enhances regulatory T cell representation. Int J Radiat Oncol Biol Phys (2011) 81:1128–35. doi: 10.1016/j.ijrobp.2010.09.034
135. Balogh A, Persa E, Bogdandi EN, Benedek A, Hegyesi H, Safrany G, et al. The effect of ionizing radiation on the homeostasis and functional integrity of murine splenic regulatory T cells. Inflammation Res (2013) 62:201–12. doi: 10.1007/s00011-012-0567-y
136. Pugh JL, Sukhina AS, Seed TM, Manley NR, Sempowski GD, van den Brink MR, et al. Histone deacetylation critically determines T cell subset radiosensitivity. J Immunol (2014) 193:1451–8. doi: 10.4049/jimmunol.1400434
137. Venkatesulu BP, Sharma A, Pollard-Larkin JM, Sadagopan R, Symons J, Neri S, et al. Ultra high dose rate (35 Gy/sec) radiation does not spare the normal tissue in cardiac and splenic models of lymphopenia and gastrointestinal syndrome. Sci Rep (2019) 9:17180. doi: 10.1038/s41598-019-53562-y
138. Arina A, Beckett M, Fernandez C, Zheng W, Pitroda S, Chmura SJ, et al. Tumor-reprogrammed resident T cells resist radiation to control tumors. Nat Commun (2019) 10:3959. doi: 10.1038/s41467-019-11906-2
139. Telarovic I, Yong CSM, Guckenberger M, Unkelbach J, Pruschy M. Radiation-induced lymphopenia does not impact treatment efficacy in a mouse tumor model. Neoplasia (2022) 31:100812. doi: 10.1016/j.neo.2022.100812
140. Hochman PS, Cudkowicz G, Dausset J. Decline of natural killer cell activity in sublethally irradiated mice. J Natl Cancer Inst (1978) 61:265–8. doi: 10.1093/jnci/61.1.265
141. Pandey R, Shankar BS, Sharma D, Sainis KB. Low dose radiation induced immunomodulation: effect on macrophages and CD8+ T cells. Int J Radiat Biol (2005) 81:801–12. doi: 10.1080/09553000500531886
142. Zheng X, Guo Y, Wang L, Zhang H, Wang S, Wang L, et al. Recovery profiles of T-cell subsets following low-dose total body irradiation and improvement with cinnamon. Int J Radiat Oncol Biol Phys (2015) 93:1118–26. doi: 10.1016/j.ijrobp.2015.08.034
143. Prosser JS. Survival of human T and B lymphocytes after X-irradiation. Int J Radiat Biol Relat Stud Phys Chem Med (1976) 30:459–65. doi: 10.1080/09553007614551271
144. Kwan DK, Norman A. Radiosensitivity of human lymphocytes and thymocytes. Radiat Res (1977) 69:143–51. doi: 10.2307/3574524
145. Zarcone D, Tilden AB, Lane VG, Grossi CE. Radiation sensitivity of resting and activated nonspecific cytotoxic cells of T lineage and NK lineage. Blood (1989) 73:1615–21. doi: 10.1182/blood.V73.6.1615.1615
146. Rana R, Vitale M, Mazzotti G, Manzoli L, Papa S. Radiosensitivity of human natural killer cells: binding and cytotoxic activities of natural killer cell subsets. Radiat Res (1990) 124:96–102. doi: 10.2307/3577701
147. Nakamura N, Kusunoki Y, Akiyama M. Radiosensitivity of CD4 or CD8 positive human T-lymphocytes by an in vitro colony formation assay. Radiat Res (1990) 123:224–7. doi: 10.2307/3577549
148. Geara FB, Peters LJ, Ang KK, Wike JL, Sivon SS, Guttenberger R, et al. Intrinsic radiosensitivity of normal human fibroblasts and lymphocytes after high- and low-dose-rate irradiation. Cancer Res (1992) 52:6348–52.
149. Seki H, Iwai K, Kanegane H, Konno A, Ohta K, Ohta K, et al. Differential protective action of cytokines on radiation-induced apoptosis of peripheral lymphocyte subpopulations. Cell Immunol (1995) 163:30–6. doi: 10.1006/cimm.1995.1095
150. Philippe J, Louagie H, Thierens H, Vral A, Cornelissen M, De Ridder L. Quantification of apoptosis in lymphocyte subsets and effect of apoptosis on apparent expression of membrane antigens. Cytometry (1997) 29:242–9. doi: 10.1002/(SICI)1097-0320(19971101)29:3<242::AID-CYTO7>3.0.CO;2-D
151. Radojcic M, Crompton NEA. Age dependence of T-lymphocyte apoptosis induced by high-energy proton exposure. Radiat Environ Biophys (2001) 40:131–5. doi: 10.1007/s004110100093
152. Wilkins RC, Kutzner BC, Truong M, McLean JR. The effect of the ratio of CD4+ to CD8+ T-cells on radiation-induced apoptosis in human lymphocyte subpopulations. Int J Radiat Biol (2002) 78:681–8. doi: 10.1080/09553000210144475
153. Wilkins RC, Wilkinson D, Maharaj HP, Bellier PV, Cybulski MB, McLean JR. Differential apoptotic response to ionizing radiation in subpopulations of human white blood cells. Mutat Res (2002) 513:27–36. doi: 10.1016/S1383-5718(01)00290-X
154. Schmitz A, Bayer J, Dechamps N, Thomas G. Intrinsic susceptibility to radiation-induced apoptosis of human lymphocyte subpopulations. Int J Radiat Oncol Biol Phys (2003) 57:769–78. doi: 10.1016/S0360-3016(03)00637-0
155. Hayashi T, Hayashi I, Shinohara T, Morishita Y, Nagamura H, Kusunoki Y, et al. Radiation-induced apoptosis of stem/progenitor cells in human umbilical cord blood is associated with alterations in reactive oxygen and intracellular pH. Mutat Res (2004) 556:83–91. doi: 10.1016/j.mrfmmm.2004.07.002
156. Torudd J, Protopopova M, Sarimov R, Nygren J, Eriksson S, Markova E, et al. Dose-response for radiation-induced apoptosis, residual 53BP1 foci and DNA-loop relaxation in human lymphocytes. Int J Radiat Biol (2005) 81:125–38. doi: 10.1080/09553000500077211
157. Bordon E, Henriquez Hernandez LA, Lara PC, Pinar B, Fontes F, Rodriguez Gallego C, et al. Prediction of clinical toxicity in localized cervical carcinoma by radio-induced apoptosis study in peripheral blood lymphocytes (PBLs). Radiat Oncol (2009) 4:58. doi: 10.1186/1748-717X-4-58
158. Milyavsky M, Gan OI, Trottier M, Komosa M, Tabach O, Notta F, et al. A distinctive DNA damage response in human hematopoietic stem cells reveals an apoptosis-independent role for p53 in self-renewal. Cell Stem Cell (2010) 7:186–97. doi: 10.1016/j.stem.2010.05.016
159. Cao M, Cabrera R, Xu Y, Liu C, Nelson D. Different radiosensitivity of CD4(+)CD25(+) regulatory T cells and effector T cells to low dose gamma irradiation in vitro. Int J Radiat Biol (2011) 87:71–80. doi: 10.3109/09553002.2010.518208
160. Horn S, Barnard S, Brady D, Prise KM, Rothkamm K. Combined analysis of gamma-H2AX/53BP1 foci and caspase activation in lymphocyte subsets detects recent and more remote radiation exposures. Radiat Res (2013) 180:603–9. doi: 10.1667/RR13342.1
161. Hietanen T, Pitkanen M, Kapanen M, Kellokumpu-Lehtinen PL. Effects of single and fractionated irradiation on natural killer cell populations: Radiobiological characteristics of viability and cytotoxicity in vitro. Anticancer Res (2015) 35:5193–200.
162. Falcke SE, Ruhle PF, Deloch L, Fietkau R, Frey B, Gaipl US. Clinically relevant radiation exposure differentially impacts forms of cell death in human cells of the innate and adaptive immune system. Int J Mol Sci (2018) 19:3574. doi: 10.3390/ijms19113574
163. Cole J, Arlett CF, Green MH, Harcourt SA, Priestley A, Henderson L, et al. Comparative human cellular radiosensitivity: II. The survival following gamma-irradiation of unstimulated (G0) T-lymphocytes, T-lymphocyte lines, lymphoblastoid cell lines and fibroblasts from normal donors, from ataxia-telangiectasia patients and from ataxia-telangiectasia heterozygotes. Int J Radiat Biol (1988) 54:929–43. doi: 10.1080/09553008814552331
164. Brovall C, Schacter B. Radiation sensitivity of human natural killer cell activity: control by X-linked genes. J Immunol (1981) 126:2236–9. doi: 10.4049/jimmunol.126.6.2236
165. Cao M, Cabrera R, Xu Y, Liu C, Nelson D. Gamma irradiation alters the phenotype and function of CD4+CD25+ regulatory T cells. Cell Biol Int (2009) 33:565–71. doi: 10.1016/j.cellbi.2009.02.007
166. Heylmann D, Rodel F, Kindler T, Kaina B. Radiation sensitivity of human and murine peripheral blood lymphocytes, stem and progenitor cells. Biochim Biophys Acta (2014) 1846:121–9. doi: 10.1016/j.bbcan.2014.04.009
167. Miszczyk J, Rawojc K, Panek A, Swakon J, Prasanna PG, Rydygier M. Response of human lymphocytes to proton radiation of 60 MeV compared to 250 kV X-rays by the cytokinesis-block micronucleus assay. Radiother Oncol (2015) 115:128–34. doi: 10.1016/j.radonc.2015.03.003
168. Panek A, Miszczyk J. ATM and RAD51 repair pathways in human lymphocytes irradiated with 70 MeV therapeutic proton beam. Radiat Res (2022) 197:396–402. doi: 10.1667/RADE-21-00109.1
169. Vandevoorde C, Vral A, Vandekerckhove B, Philippe J, Thierens H. Radiation sensitivity of human CD34(+) cells versus peripheral blood T lymphocytes of newborns and adults: DNA repair and mutagenic effects. Radiat Res (2016) 185:580–90. doi: 10.1667/RR14109.1
170. DeKruyff RH, Fang Y, Umetsu DT. IL-4-based helper activity of CD4+ T cells is radiation sensitive. Cell Immunol (1995) 160:248–56. doi: 10.1016/0008-8749(95)80035-H
171. Eckert D, Rapp F, Tsedeke AT, Molendowska J, Lehn R, Langhans M, et al. ROS- and radiation source-dependent modulation of leukocyte adhesion to primary microvascular endothelial cells. Cells (2021) 11:72. doi: 10.3390/cells11010072
172. Rajakaruna H, O'Connor JH, Cockburn IA, Ganusov VV. Liver environment-imposed constraints diversify movement strategies of liver-localized CD8 T cells. J Immunol (2022) 208:1292–304. doi: 10.4049/jimmunol.2100842
173. Downey GP, Doherty DE, Schwab B 3rd, Elson EL, Henson PM, Worthen GS. Retention of leukocytes in capillaries: role of cell size and deformability. J Appl Physiol (1985) (1990) 69:1767–78. doi: 10.1152/jappl.1990.69.5.1767
174. Pham TN, Coupey J, Candeias SM, Ivanova V, Valable S, Thariat J, et al. Beyond lymphopenia, unraveling radiation-induced leucocyte subpopulation kinetics and mechanisms through modeling approaches. J Exp Clin Cancer Res CR (2023) 42:50. doi: 10.1186/s13046-023-02621-4
175. Spiotto M, Fu YX, Weichselbaum RR. The intersection of radiotherapy and immunotherapy: mechanisms and clinical implications. Sci Immunol (2016) 1:1226–33. doi: 10.1126/sciimmunol.aag1266
176. Zhao Q, Li T, Du S, He J, Zeng Z. Shortened radiation time promotes recovery from radiation-induced lymphopenia in early-stage non-small cell lung cancer patients treated with stereotactic body radiation therapy. Technol Cancer Res Treat (2022) 21:15330338221112287. doi: 10.1177/15330338221112287
177. Chou C, Li MO. Tissue-resident lymphocytes across innate and adaptive lineages. Front Immunol (2018) 9:2104. doi: 10.3389/fimmu.2018.02104
178. Tong AA, Forestell B, Murphy DV, Nair A, Allen F, Myers J, et al. Regulatory T cells differ from conventional CD4(+) T cells in their recirculatory behavior and lymph node transit times. Immunol Cell Biol (2019) 97:787–98. doi: 10.1111/imcb.12276
179. Mandl JN, Liou R, Klauschen F, Vrisekoop N, Monteiro JP, Yates AJ, et al. Quantification of lymph node transit times reveals differences in antigen surveillance strategies of naive CD4+ and CD8+ T cells. Proc Natl Acad Sci U.S.A. (2012) 109:18036–41. doi: 10.1073/pnas.1211717109
180. Tse BCY, Ireland RA, Lee JY, Marsh-Wakefield F, Kok LF, Don AS, et al. Exposure to systemic immunosuppressive ultraviolet radiation alters T cell recirculation through sphingosine-1-phosphate. J Immunol (2021) 207:2278–87. doi: 10.4049/jimmunol.2001261
181. Galdiero M, Cipollaro de l'Ero G, Folgore A, Cappello M, Giobbe A, Sasso FS. Effects of irradiation doses on alterations in cytokine release by monocytes and lymphocytes. J Med (1994) 25:23–40.
182. Krivenko SI, Dryk SI, Komarovskaya ME, Karkanitsa LV. Ionizing radiation increases TNF/cachectin production by human peripheral blood mononuclear cells in vitro. Int J Hematol (1992) 55:127–30.
183. Yamazaki H, Yoshioka Y, Inoue T, Tanaka E, Nishikubo M, Sato T, et al. Changes in natural killer cell activity by external radiotherapy and/or brachytherapy. Oncol Rep (2002) 9:359–63. doi: 10.3892/or.9.2.359
184. McGinnes K, Florence J, Penny R. The effect of radiotherapy on the natural killer (NK)-cell activity of cancer patients. J Clin Immunol (1987) 7:210–7. doi: 10.1007/BF00915726
185. Mozaffari F, Lindemalm C, Choudhury A, Granstam-Bjorneklett H, Helander I, Lekander M, et al. NK-cell and T-cell functions in patients with breast cancer: effects of surgery and adjuvant chemo- and radiotherapy. Br J Cancer (2007) 97:105–11. doi: 10.1038/sj.bjc.6603840
186. Marchese MJ, Zaider M, Hall EJ. Potentially lethal damage repair in human cells. Radiother Oncol (1987) 9:57–65. doi: 10.1016/S0167-8140(87)80219-0
187. Kim N, Noh JM, Lee W, Park B, Park H, Park JY, et al. Proton beam therapy reduces the risk of severe radiation-induced lymphopenia during chemoradiotherapy for locally advanced non-small cell lung cancer: A comparative analysis of proton versus photon therapy. Radiother Oncol (2021) 156:166–73. doi: 10.1016/j.radonc.2020.12.019
Keywords: lymphopenia, lymphocytes, radiotherapy, radiosensitivity, blood dose
Citation: Paganetti H (2023) A review on lymphocyte radiosensitivity and its impact on radiotherapy. Front. Oncol. 13:1201500. doi: 10.3389/fonc.2023.1201500
Received: 06 April 2023; Accepted: 17 July 2023;
Published: 03 August 2023.
Edited by:
Timothy James Kinsella, Brown University, United StatesReviewed by:
Franz Rödel, University Hospital Frankfurt, GermanyGianluca Ferini, Rem Radiotherapy, Italy
Copyright © 2023 Paganetti. This is an open-access article distributed under the terms of the Creative Commons Attribution License (CC BY). The use, distribution or reproduction in other forums is permitted, provided the original author(s) and the copyright owner(s) are credited and that the original publication in this journal is cited, in accordance with accepted academic practice. No use, distribution or reproduction is permitted which does not comply with these terms.
*Correspondence: Harald Paganetti, hpaganetti@mgh.harvard.edu