Dopamine activity on the perceptual salience for recognition memory
- 1División de Neurociencias, Instituto de Fisiología Celular, Universidad Nacional Autónoma de México, Circuito Exterior, Ciudad Universitaria, Mexico, Mexico
- 2Departamento de Ciencias de la Salud, División de Ciencias Biológicas y de la Salud, Universidad Autónoma Metropolitana, Unidad Lerma, Estado de México, Mexico
To survive, animals must recognize relevant stimuli and distinguish them from inconspicuous information. Usually, the properties of the stimuli, such as intensity, duration, frequency, and novelty, among others, determine the salience of the stimulus. However, previously learned experiences also facilitate the perception and processing of information to establish their salience. Here, we propose “perceptual salience” to define how memory mediates the integration of inconspicuous stimuli into a relevant memory trace without apparently altering the recognition of the physical attributes or valence, enabling the detection of stimuli changes in future encounters. The sense of familiarity is essential for successful recognition memory; in general, familiarization allows the transition of labeling a stimulus from the novel (salient) to the familiar (non-salient). The novel object recognition (NOR) and object location recognition (OLRM) memory paradigms represent experimental models of recognition memory that allow us to study the neurobiological mechanisms involved in episodic memory. The catecholaminergic system has been of vital interest due to its role in several aspects of recognition memory. This review will discuss the evidence that indicates changes in dopaminergic activity during exposure to novel objects or places, promoting the consolidation and persistence of memory. We will discuss the relationship between dopaminergic activity and perceptual salience of stimuli enabling learning and consolidation processes necessary for the novel-familiar transition. Finally, we will describe the effect of dopaminergic deregulation observed in some pathologies and its impact on recognition memory.
Introduction
Organisms are continuously exposed to several stimuli and events in their environment across their lifespans. Nevertheless, individuals must efficiently and effectively guide their behavior according to the perceived relevant stimuli. The continuous processing of incoming information demands considerable cognitive effort. Therefore, selecting, filtering, and processing information is essential to preserve proper cognitive function. In this regard, the relevant information is processed with less cognitive interference, characterized by the competition of information in eliciting cognitive processes (Grachev et al., 2001) compared to neutral or inconspicuous stimuli. Selecting relevant information from the environment is easily achieved when these stimuli are intrinsically salient due to their physical properties. Thus, salience refers to the phenomenon by which a stimulus highlights or is set apart from the environment (Uddin, 2015). Generally, a salient stimulus attracts attentional resources bottom-up to facilitate information processing (Santangelo, 2015), where the stimulation drives cognitive processes that contrasts with the surroundings. Bottom-up processing states that the stimuli’s physical attributes originate from sensory information facilitating salience and perception (Riener, 2019). In this regard, perceptual processing determines the attention and cognition required for a proper behavioral reaction to the stimuli in the environment (Goldstein and Cacciamani, 2021). However, there is evidence suggesting that the physical properties of the stimuli are not the only factors that drive information processing. Top-down processing, also called knowledge-based processing, refers to the “internal” factors of the observer acquired by previous experiences (Awh et al., 2012). Top-down processing is driven by cognition, starting with memory and expectations that affect salience and perception (Riener, 2019). Therefore, the brain hierarchizes salient information according to the physical stimuli properties and the organism’s experience, facilitating perception of the stimuli.
Current evidence indicates that perception and memory interact to direct and control driving attentional and cognitive processes actions (for a review, Heurley and Ferrier, 2015). A salient stimulus is more prone to be integrated into memory traces than non-salient stimuli. Even though many stimuli appear to be non-salient due to their intrinsic low-intensity properties; they can become salient based on their meaning, consequences, or relationship with other stimuli in the environment (Santangelo, 2015). Therefore, previous experiences modulate stimuli recognition by comparing them with stored information and adjusting salience processing (Riener, 2019). Here, we propose the term perceptual salience referring to how memory modulates the integration of inconspicuous stimuli into a relevant memory without enhancing the initial sensory perception.
Memory is a fundamental adaptative mechanism that promotes organisms’ survival by identifying relevant environmental changes. Across their lifespan, animals experience several episodes associated with important information about food, shelter, and danger, among others. Thus, individuals need to recall those specific events and appropriately modify their behavior to survive. The encoding, integration, and retrieval of experienced information is defined as memory (Squire, 2009). Overall, memory formation requires acquiring information by learning events during exposure to stimuli. Then, memories are integrated into long-lasting traces through chemical and structural modification via protein synthesis (McGaugh, 2000; Bisaz et al., 2014). When necessary, internal and external cues promote selection, reactivation, and assessment of information, modulating the behavioral outcomes, a process called memory retrieval (Ben-Yakov et al., 2015; Frankland et al., 2019). During memory retrieval, memories undergo a consolidation-like process called reconsolidation, where memory updating may occur (Nader et al., 2000; Sara, 2000; Lee et al., 2017; Rodriguez-Ortiz and Bermúdez-Rattoni, 2017). Memory engages distinct neural circuits or systems accordingly to the type of information, inducing cellular and molecular changes that support memory maintenance (Nadel and Hardt, 2011).
According to the awareness during retrieval, memory systems have been classified into declarative and non-declarative. Non-declarative memories are characterized by integrating information associated with habits, motor learning, and associative learning. The most common example is the information accessed without conscious recall. Conversely, declarative memories are recalled consciously and are related to facts (semantic memory) and events (episodic memory; Squire, 2009; Nadel and Hardt, 2011). Specifically, episodic memory integrates “where,” “what,” and “when” an event happened into a spatiotemporal context (Tulving, 2002). Therefore, an essential aspect of episodic memory is the judgment of whether a recent experience, including subject, location, or event, has been previously experienced or encountered. Episodic memory integrates information related to environmental changes, facilitating the identification of different information modalities, including faces, places, sounds, objects, or changes in the context. The integrated information allows the discrimination of novel events from familiar ones. Thus, recognition memory involves familiarization by acquiring, consolidating, retrieving, and updating experienced events in a space-time frame (Squire and Zola, 1996; Tulving, 2002; Balderas et al., 2015; Morici et al., 2015).
In general, recognition memory incorporates two differential processes: Recollection and Familiarity (Brown and Aggleton, 2001; Merkow et al., 2015). Familiarity is the ability to judge whether a particular stimulus or event has already been experienced (Mandler, 1980). In contrast, recollection retrieves the stimuli or events’ characteristics (qualitative dimension; Evans and Wilding, 2012). Thus, exposure to novelty (salient stimulus) triggers a maximum behavioral response that is progressively reduced during subsequent presentations (familiar, non-salient stimulus). The novelty transitions to familiarity are gradual shifts caused by learning (Henson and Gagnepain, 2010) and neuronal plasticity changes (Lisman et al., 2011). Recollection of contextual events and their behavioral responses occur by activating several brain regions (Kafkas and Montaldi, 2014), like the entorhinal cortex (Knierim, 2015), hippocampus (Barker and Warburton, 2011), and the prefrontal cortex (Akirav and Maroun, 2006). In comparison, the items’ familiarity variations rely on parahippocampal (perirhinal, entorhinal, and postrhinal; Brown and Aggleton, 2001; Yonelinas, 2002; Evans and Wilding, 2012; Merkow et al., 2015) and insular (Bermudez-Rattoni et al., 2005; Balderas et al., 2008) cortices of the brain. Within these structures, changes in the neurotransmitters involved in the transition from novelty to familiarity include elevation in acetylcholine, noradrenaline, and dopamine which gradually diminish after the consecutive exposure to the stimulus (Miranda et al., 2000; Osorio-Gómez et al., 2016, 2017; Rodríguez-García and Miranda, 2016). The activation of the same neurotransmission systems occurs in different areas of the brain depending on memory recollection of events or stimuli recognition. Even though many neurotransmitters are involved in recognition memory, the catecholaminergic system is of particular interest due to its modulatory effect on synaptic plasticity and memory processes that might impact perceptual salience (Jay, 2003; Lisman et al., 2011; Takeuchi et al., 2016; Yang et al., 2017).
Finally, we will review how cognitive impairments are directly related to dopaminergic dysfunctions, impacting recognition memory in pathologies such as Alzheimer’s disease (Guzmán-Ramos et al., 2012; Moreno-Castilla et al., 2017), schizophrenia (Brisch et al., 2014), and Parkinson’s disease (Aarsland, 2016). Abnormal functioning in several brain regions has been related to recognition memory detriments observed during spontaneous exploration tasks in the animal model used to study these processes.
Spontaneous Object Exploration
Spontaneous novel object recognition tasks are widely used to assess long-term recognition memory’s neurobiological mechanisms. Novel object recognition (NOR) and object location recognition memory (OLRM) are the most common behavioral paradigms employed to determine the processes of acquisition, consolidation, retrieval, and updating of recognition memory (see Figure 1). NOR and OLRM are simple tasks based on the rodents’ innate preference to explore novel stimuli than familiar ones (Ennaceur and Delacour, 1988; Chan et al., 2018). Both paradigms consist of at least three sessions: a handling and habituation period to an empty open field. Then, a sample phase (acquisition session), where animals explore novel objects for first-time. Finally, a test session (retrieval) where animals discriminate and identify a novel object in the case of NOR or the displaced one in the case of OLRM (Ameen-Ali et al., 2015; Chan et al., 2018). In addition, novel information can be integrated during retrieval and updating recognition memory (Balderas et al., 2015; Kwapis et al., 2020; Wright et al., 2020; see Figure 1). These tasks allow us to assess the ability of animals to recognize environmental changes caused by exposure to a novel object or a novel spatial configuration and help determine novel/familiar discrimination and recollection processes.
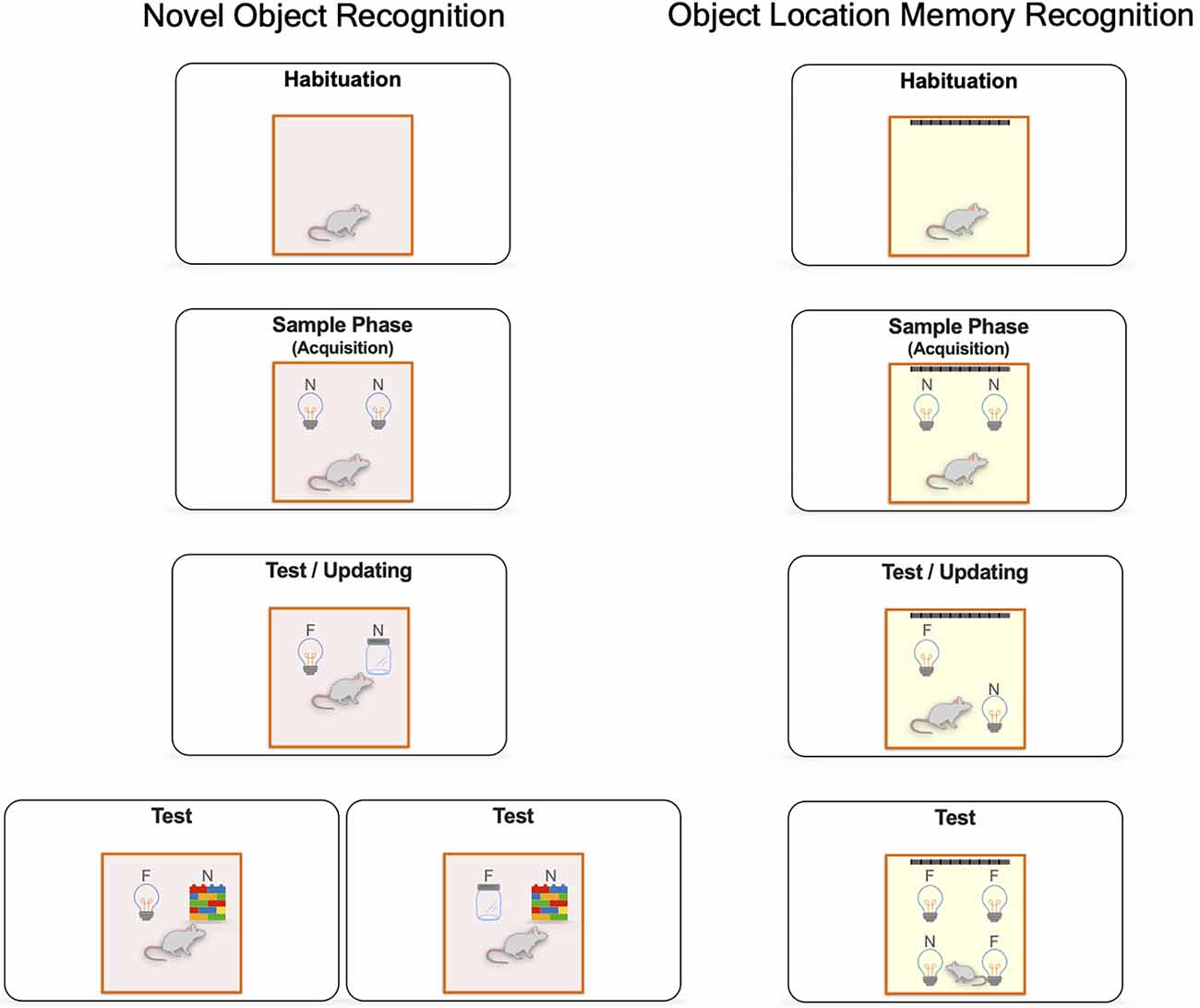
Figure 1. General procedures for spontaneous object exploration. Novel object recognition (NOR) and object location recognition memory (OLRM) paradigms include different sessions: a handling and habituation period to an empty open field to reduce stress and promote the exploratory activity; a sample phase, where the animal is given a period to freely explore two identical objects. During the test session, short-term or long-term assessment of recognition memory is determined by the length of the retention interval, in this session the animal freely explores a different novel object (jar) in the case of NOR or the displacement of an object to a novel location in the case of OLRM. Finally, recognition memory updating is evaluated if a new third object is presented (wall of brick toys) in a NOR task or when a new displaced position is presented in an OLRM task. In general, animals that remember the familiar object of the familiar spatial configuration will spend more time exploring the novel object or the novel spatial configuration Novel (N) and Familiar (F).
Several experimental approaches have evaluated the differential participation of several brain structures in the consolidation of recognition memory. Regarding OLRM, the hippocampus is a crucial brain structure in spatial-dependent tasks. Hippocampal lesions impair OLRM (Save et al., 1992; Mumby et al., 2002; Barker and Warburton, 2011). Particularly, CA3 lesions (Lee et al., 2005; Hunsaker et al., 2008) or its pharmacological inactivation (Barbosa et al., 2012) hinder spatial novelty discrimination. In addition, pharmacological inactivation of the CA1 portion impairs OLRM (Assini et al., 2009). Thus, the dorsal CA1 and CA3 portions are related to acquiring, consolidating, and retrieving contextual information (Brown and Aggleton, 2001; Barker and Warburton, 2011; Moreno-Castilla et al., 2017). Concerning NOR, this task depends on the insular cortex (Bermudez-Rattoni et al., 2005; Balderas et al., 2008), the perirhinal cortex (Warburton et al., 2003; Winters et al., 2004; Winters and Bussey, 2005; Balderas et al., 2013b), and the ventromedial prefrontal cortex (Akirav and Maroun, 2006). However, the involvement of the hippocampus in NOR has been controversial (Mumby, 2001; Balderas et al., 2008; Barker and Warburton, 2011; Haettig et al., 2011). Evidence suggests that the functional integrity of the hippocampal activity is required in NOR consolidation (Rossato et al., 2007; Myskiw et al., 2008; Cohen et al., 2013; Furini et al., 2014). For many years it was assumed that hippocampal activity was necessary only when recalling objects in a particular context (Barker and Warburton, 2011). Anisomycin administration into the dorsal hippocampus immediately after the sample phase impairs long-term but not short-term object-in-context recognition memory (Balderas et al., 2008). The object-in-context task is a spontaneous exploration paradigm in which animals spend more time exploring familiar objects within a novel context (salient information) than in a familiar one (non-salient information). However, conflicting results might be explained by differences in experimental approaches (lesions vs. temporal inactivation). The use of a particular behavioral protocol and not merely by the type of information (what vs. where; for review, please see Cohen and Stackman, 2015).
Catecholaminergic system involvement in NOR and OLMR
The catecholaminergic system is strongly related to cognitive processes and plays a vital role in the modulation of recognition memory (Yang et al., 2017; Titulaer et al., 2021). Exposure to novel stimuli or contextual information induces an elevation in catecholamines and disruption in catecholaminergic activity hinders recognition memory (Guzmán-Ramos et al., 2012; Moreno-Castilla et al., 2017). Dopaminergic and noradrenergic systems regulate neuronal plasticity events related to memory formation and consolidation. So, any alteration in the catecholaminergic system elevates the probability of cognitive impairments, including recognition memory. The main catecholaminergic inputs arise from the locus coeruleus (LC) and the ventral tegmental area (VTA), innervating several brain structures through the mesolimbic and mesocortical pathways (Ungless, 2004; Bromberg-Martin et al., 2010; Takeuchi et al., 2016).
Regarding norepinephrine, the LC supplies projections into several parts of the brain, including the medial temporal lobe (Pudovkina et al., 2001; Aston-Jones and Cohen, 2005; Morilak et al., 2005; Kempadoo et al., 2016). The noradrenergic system plays a critical role in memory consolidation processes by modulating plasticity-related events (McGaugh, 2000, 2013; McGaugh, 2015; Barsegyan et al., 2014). Norepinephrine activates α (α1 and α2) and β (β1, β2, and β3) receptors. Stimulation of α1 receptors increases Ca2+ and diacylglycerol intracellular levels, promoting the activation of phospholipase C and the subsequent activation of protein kinase C; this signaling pathway modulates neuronal changes necessary for memory establishment (Perez, 2020). Furthermore, the activation of β receptors promotes adenylate cyclase activity that increases cAMP levels. This augmentation results in the activation of protein kinase A, which ultimately promotes gene expression and memory consolidation (O’Dell et al., 2015).
LC activity increases its firing response after presenting novel objects or contexts (Sara et al., 1994; Vankov et al., 1995; Pudovkina et al., 2001; Wagatsuma et al., 2018). Recent evidence indicates that the LC projects dopaminergic and noradrenergic terminals into the dorsal hippocampus (Kempadoo et al., 2016), enhancing spatial memory consolidation through D1-like receptor activity (Takeuchi et al., 2016). Moreover, the catecholaminergic denervation through the administration of 6-OHDA into the hippocampus interferes with OLRM formation (Moreno-Castilla et al., 2017). A similar lesion in the shell subregion of the nucleus accumbens impairs object location memory (Nelson et al., 2010). Pharmacological activation of the noradrenergic receptors through the systemic administration of epinephrine improves recognition and memory consolidation (Dornelles et al., 2007). Similarly, activation of β receptors within the basolateral amygdala enhances object-in-context recognition memory (Barsegyan et al., 2014), NOR (Chen et al., 2022), and OLRM consolidation (Roozendaal et al., 2007; Song et al., 2021). The noradrenergic system presumably promotes memory consolidation due to arousal effects; since the basolateral amygdala modulates the dorsal hippocampus and insular and prelimbic cortices through the noradrenergic system (Barsegyan et al., 2019; Chen et al., 2022). Likewise, norepinephrine administration into the hippocampus immediately after the sample session promotes NOR persistence (Mello-Carpes et al., 2016). Thus, exposure to novel objects or contextual information enhances memory consolidation through LC modulation, noradrenaline release within the amygdala, and the corelease of norepinephrine and dopamine in the hippocampus. In addition, changes in norepinephrine levels would be associated with relevant stimuli detection, regulating arousal, improving cortical function, and enhancing subsequent cognitive functions such as attention and motivation (Aston-Jones and Cohen, 2005).
The other catecholaminergic neurotransmitter is dopamine, produced in midbrain neurons within the substantia nigra and the VTA (Baik, 2020). Dopamine release from the substantia nigra is mainly involved in controlling motor function and goal-directed behaviors (Grillner et al., 2008). However, dopamine release from the VTA and the LC terminals into the nucleus accumbens, prefrontal cortex, and medial temporal lobe (hippocampus, perirhinal, insular, parahippocampal, and entorhinal cortices) is involved in the formation and maintenance of declarative memories such as recognition memory (de Lima et al., 2011; Kempadoo et al., 2016; Takeuchi et al., 2016; Moreno-Castilla et al., 2017). In general, dopamine is a neuromodulator that modifies functional connectivity during synaptic plasticity (Jay, 2003; Lisman et al., 2011; Otani et al., 2015; Yang et al., 2017). These modifications occur after the activation of metabotropic receptors and the later induction of signaling pathways cascades, promoting the enhancement of neuronal plasticity. Mainly, dopamine activates D1-like (D1 and D5) and D2-like (D2, D3, and D4) receptors; activation of D1 receptors triggers Gs protein inducing an augmentation of cAMP and the subsequent activation of protein kinase A (Undieh, 2010), regulating conductance of NMDA receptors via phosphorylation of NR1 and NR2 subunits (Chen et al., 2004; Murphy et al., 2014). Moreover, activation of D1 receptors promotes AMPA (Mangiavacchi and Wolf, 2004; Rozas et al., 2015) and NMDA (Gao and Wolf, 2008; Li et al., 2010) receptors externalization. In the case of D2-like receptors, their activation inhibits adenylate cyclase through Gi proteins, suppressing neurotransmitter release from terminals (Neve et al., 2004).
Dopamine plays an essential role in recognition memory since the dopaminergic neuronal activity is modified by novel and salient stimuli (Ljungberg et al., 1992; Ungless, 2004). The VTA is a dopaminergic nucleus that displays changes in electrical activity associated with the presentation of novel stimuli (Ljungberg et al., 1992; Schultz, 1998; Düzel et al., 2009), increasing dopaminergic levels within the nucleus accumbens (Legault and Wise, 2001; Leonibus et al., 2006), striatum (Ihalainen et al., 1999), the dorsal and ventral hippocampus (Ihalainen et al., 1999; Mello-Carpes et al., 2016; Moreno-Castilla et al., 2017; Hernández-Ramírez et al., 2021; Titulaer et al., 2021), the prefrontal cortex (Feenstra and Botterblom, 1996; Ihalainen et al., 1999; Feenstra et al., 2000), and the insular cortex (Guzmán-Ramos et al., 2010, 2012; Osorio-Gómez et al., 2021). Therefore, the dopaminergic system has been related to novelty detection that triggers recognition memory establishment (Rossato et al., 2013; Otani et al., 2015; Moreno-Castilla et al., 2016; Yang et al., 2017). Object and location recognition memory depends on catecholaminergic activity. Exploring novel objects induces dopamine release into the insular cortex, but CA1 hippocampal dopamine remains unaltered during the sample phase (Guzmán-Ramos et al., 2012). Significantly, hippocampal catecholaminergic denervation by 6-OHDA administration impedes OLRM but spares NOR (Moreno-Castilla et al., 2017), indicating that the hippocampal dopaminergic activity is not involved in NOR formation or retrieval. Hence, the fine modulation of the dopaminergic system is required to establish recognition memory appropriately.
The inactivation of VTA (Rossato et al., 2013) or the denervation of the mesolimbic-cortical dopaminergic terminals (Stephen Fink and Smith, 1980) hinders NOR consolidation. While an excess of dopaminergic levels, caused by knocking out the expression of the dopamine transporter, impedes NOR formation (Chang et al., 2020). Similarly, systemic administration of methamphetamine, an enhancer of catecholamines release (Belcher et al., 2008; Camarasa et al., 2010), or the blockade of D2 receptors or D4 receptors, impairs NOR establishment (Besheer et al., 1999; Woolley et al., 2003; Watson et al., 2012; Miyauchi et al., 2017). Moreover, the systemic activation of D1-like receptors hinders OLRM and NOR retrieval (Hotte et al., 2005; Pezze et al., 2015), while the inactivation of D3 (Watson et al., 2012) enhances novel recognition retrieval. Likewise, memory persistence and memory retrieval in NOR are heightened after the systemic administration of a D1/D5 receptor agonist (Hotte et al., 2005, 2006; de Lima et al., 2011; see Table 1). Regarding the hippocampus, administering a D1 antagonist after the sample phase into the dentate gyrus impairs object recognition (Yang et al., 2017). However, administering a D1/D5 receptor antagonist into the dorsal hippocampus before or after the sample phase spares long-term NOR memory (Balderas et al., 2013a; Rossato et al., 2013). Conversely, the administration of a D1/D5 receptor antagonist into the perirhinal cortex before the sample phase spares short-term but prevents the consolidation of NOR (Balderas et al., 2013a). Moreover, the intracerebroventricular administration of D1-like receptors antagonist impairs spatial novel configuration learning (Lemon and Manahan-Vaughan, 2006), whereas blockade of D1 receptors within the prefrontal cortex or amygdala (Nagai et al., 2007; Rossato et al., 2013) impairs NOR consolidation (see Table 2).
All these results suggest that catecholaminergic activity, particularly dopaminergic modulation, is responsible for enhancing the consolidation of recognition memory. Exposure to novel stimuli induces dopamine release in several brain structures, facilitating memory establishment through the synthesis, tagging, and capture of proteins associated with synaptic plasticity generated by learning signals (Frey and Morris, 1998). In the absence of a neuromodulator, modified synapses return to the baseline level after learning, reducing the probability of consolidating memories (Takeuchi et al., 2016; Duszkiewicz et al., 2019). Therefore, it has been suggested that dopamine modulates learning signals that facilitate the consolidation of events (Montague et al., 2004) involved in the process of familiarity consolidation. Thus, dopamine might modulate perceptual salience consolidation signals, enabling recognition memory.
Dopamine and Perceptual Salience
As previously reviewed, dopaminergic signaling is widely associated with neuronal plasticity enhancement. In general, dopamine activates D1-like and D2-like receptors triggering a cascade of events that lead to cellular modifications and the induction of protein synthesis necessary for memory consolidation. Therefore, dopaminergic activity is related to the modulatory effect of object and location recognition memory establishment. Although dopaminergic activity contributes significantly to memory processes, it remains to elucidate the precise functional role of dopamine and its specific contribution to perceptual salience processing necessary for recognition memory evaluated through NOR and OLRM tasks. Novelty-related dopaminergic activity within several brain structures is involved in recognition memory. Dopamine release has been related to motivated behaviors and predicting and coding rewarding events (Schultz et al., 1993; Schultz, 1998). The evidence shows that VTA dopaminergic neurons increase their firing rate to signal reward (Berridge and Robinson, 1998; Nomoto et al., 2010; Fiorillo, 2013). Nevertheless, evidence exhibits that exposure to aversive stimuli also increases the electrical activity within VTA (Brischoux et al., 2009; Bromberg-Martin et al., 2010). Whereas unexpected stimuli prediction errors also modulate dopaminergic activity (Schultz, 1998). Therefore, novelty, the intrinsic value of the stimulus (valence), and unforeseen modifications in predicted events induce changes in dopaminergic response, probably on behalf of salience (Horvitz, 2000).
Consequently, exposure to novel stimuli triggers dopamine release facilitating memory consolidation (Balderas et al., 2013a; Osorio-Gómez et al., 2021). Notably, salient visual stimulation induces a short-latency electrical response in the substantia nigra (Comoli et al., 2003). This phasic dopaminergic activity is strongly related to salience (Bromberg-Martin et al., 2010; Barto et al., 2013; Cho et al., 2017). Intrinsically salient stimuli compete for attention, in which new and relevant visual stimuli drive attentional processes (Yantis and Hillstrom, 1994). Novelty-induced salience influences memories, attention, and motivation through dopaminergic activity (Puglisi-Allegra and Ventura, 2012). Salient stimuli prioritize the consolidation of the relevant over neutral information (Alger et al., 2019). Interestingly, it has been suggested that the brain is organized to promote the interaction of functional networks, including the salience network (Tsai et al., 2020). Evidence indicates that the insular cortex is a crucial node of the salience network (Uddin, 2015), integrating exteroceptive and interoceptive information (Seeley et al., 2007). The insular cortex might be involved in salience because of the multiple inputs arising from the amygdala, VTA, the dorsomedial nucleus of the thalamus, and the prefrontal cortex (Bermudez-Rattoni, 2014; Uddin, 2015; Gil-Lievana et al., 2020, 2022; Chen et al., 2022).
As mentioned, the physical properties of the stimulus drive cognitive processes contrasting it with the surroundings and attracting attentional resources facilitating information processing. However, the physical properties of the stimuli are not the only components that can direct information processing. Previous experiences modulate top-down processing, by which “internal” factors and cognition influence perception (Awh et al., 2012). Thus, information is hierarchized within the brain according to the physical properties of the stimulus or to the previously learned information related to that stimulus, facilitating the perception of the stimuli in future events. Consequently, perceptual salience requires integrating information into meaningful-related memories without changing the initial detection of the stimuli (see Figure 2).
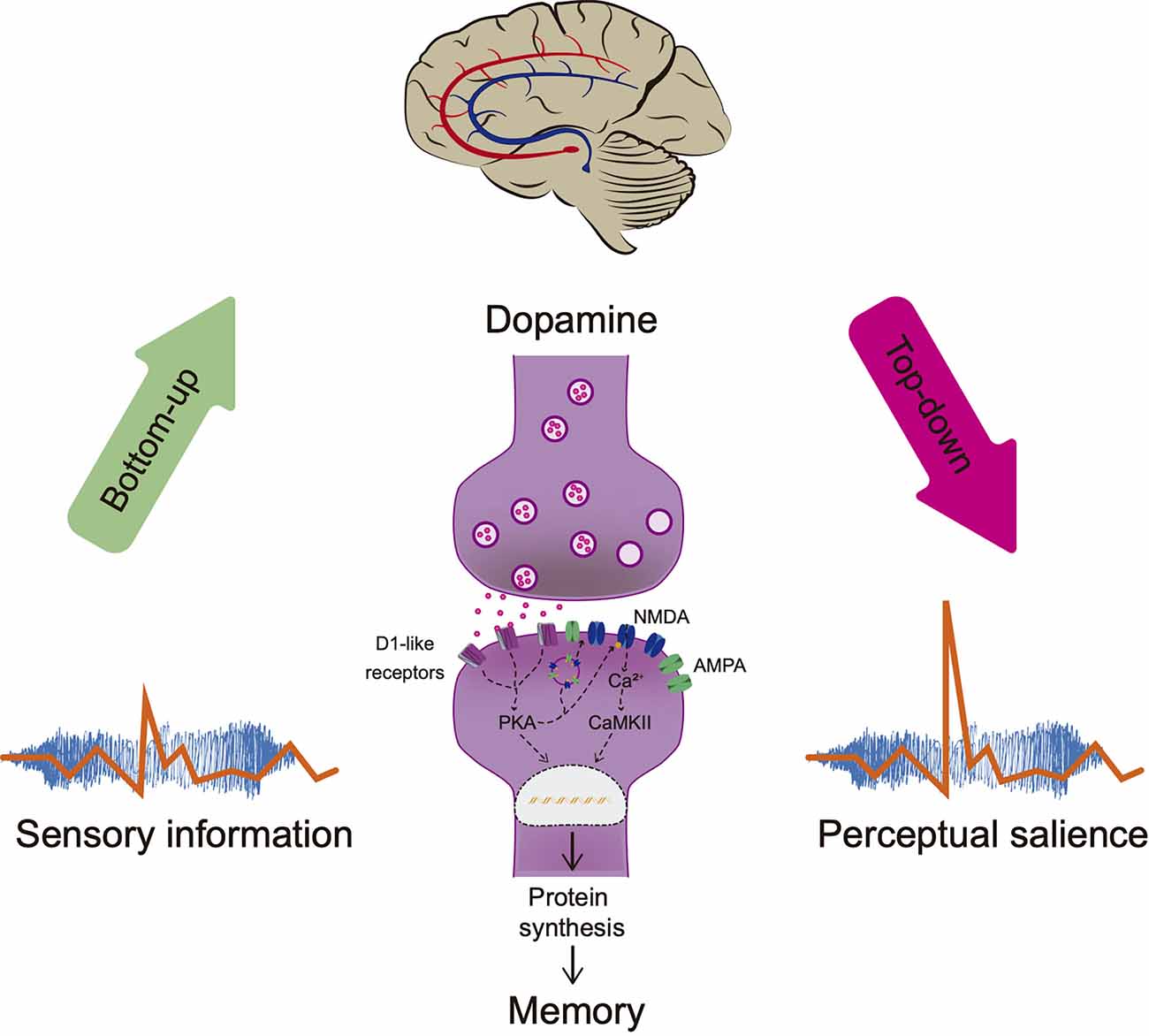
Figure 2. Perceptual salience. Sensorial stimulation attracts attentional resources bottom-up facilitating information processing. Brain hierarchizes salient information according to the physical stimuli properties and the organism’s experience (meaning, consequences, or relationship with other stimuli in the environment). Dopamine regulates neuronal plasticity through activation of D1-like receptors enhancing memory consolidation, improving NMDA’s conductance, inducing protein synthesis, and AMPA/NMDA receptors externalization. Memory modulates top-down processing without altering the initial sensory perception necessary for recognition by comparing the stimuli with the stored information and adjusting salience processing, a term referred to as perceptual salience. PKA, Protein Kinase A; Ca2+, calcium; CaMKII, calcium calmodulin kinase.
The hippocampus is involved in detecting salient spatial stimuli; hippocampal formation increases its activity when new contextual information is presented. This novelty signal is transferred to the VTA and contributes to the activation pattern observed in the VTA during novelty seeking (Lisman and Grace, 2005). For instance, the temporal inactivation of the VTA impairs NOR consolidation (Rossato et al., 2013), indicating that the dopaminergic system is essential for object recognition consolidation. This information suggests that the hippocampus and the dopaminergic inputs from the VTA and the LC form a functional loop that detects novelty and compares this information to previously integrated memories. Thus, dopaminergic activity might control the entry of crucial adaptative information and promotes subsequent integration into long-term memory through modification of synaptic plasticity (Lisman and Grace, 2005; Lisman et al., 2011).
There are illustrative examples of perceptual salience modulation via dopaminergic regulation. Optogenetic stimulation of the dopaminergic neurons within the VTA enhances behavioral response (taste neophobia) to the low-intensity stimulus facilitating taste recognition memory consolidation (Gil-Lievana et al., 2022). This effect was probably to bottom-up salience enhancement since augmented behavioral responses were observed during stimulation (acquisition). However, optogenetic stimulation of dopaminergic terminals from the VTA to the insular cortex does not modify the behavioral response (lack of neophobia) to the subthreshold stimuli while facilitating taste recognition memory performance during retrieval. It is essential to mention that optogenetic activation of VTA neurons or terminals does not alter the valence of the stimulus (Gil-Lievana et al., 2022), suggesting an increased effect over memory consolidation despite the low salient stimulus. Consequently, dopaminergic stimulation within the insular cortex enhances the perceptual salience for low-intensity stimulus. Similar results are observed after the optogenetic stimulation of LC-dopaminergic inputs into the hippocampus, which evokes dopamine release and enhances OLRM consolidation (Kempadoo et al., 2016).
Additionally, perceptual salience enhancement requires activation of the D1-like receptor. The blockade of cortical D1 receptors impairs long-term recognition memory keeping the short-term recognition memory intact. Conversely, administration of a D1 receptors agonist into the perirhinal cortex (SKF38393) before a subthreshold stimulation in a NOR protocol does not increase exploration times during acquisition. However, it induces NOR consolidation enhancement causing a clear novel object discrimination during the test (Balderas et al., 2013a). Although the blockade of D1-like receptors within the dorsal hippocampus spares NOR consolidation (Balderas et al., 2013a; Rossato et al., 2013). Recent studies report that the administration of dopamine or a D1 receptor agonist into the dorsal hippocampus enhances NOR persistence (Vargas et al., 2020; Lima et al., 2022). These results suggest that the memory trace formation expressed in short-term memory is not dopamine-dependent, but its activity enables long-term and persistent storage (Balderas et al., 2013a; Moreno-Castilla et al., 2017; Vargas et al., 2020). In contrast, the blockade of D1-like receptor activity within the insular cortex impedes perceptual salience enhancement (Gil-Lievana et al., 2022). Hence, dopaminergic stimulation promotes the consolidation and persistence of stimuli that under normal conditions are not possible considering their subthreshold properties. In this regard, it has been suggested that dopaminergic responses are not related to signaling the stimuli’s intensity but rather to the perceived intensity (de Lafuente and Romo, 2011). Although, these authors concluded that midbrain dopamine neurons code the subjective perception of the event (Romo and Rossi-Pool, 2020). We suggest that dopaminergic activity within the hippocampus, perirhinal and insular cortices facilitate the consolidation of information into long-term and persistent memories enabling the perceptual salience to guide behavior efficiently and effectively in future encounters. Considering that dopamine modulates synaptic plasticity, dopaminergic signaling promotes the consolidation of inconspicuous stimulus into a relevant memory without altering the initial sensory perception.
Impact of Catecholaminergic Alterations in Recognition Memory
Dysregulation of dopaminergic signaling hinders declarative memories such as recognition memory (Guzmán-Ramos et al., 2012; Moreno-Castilla et al., 2017; Hernández-Ramírez et al., 2021). In some pathological brain conditions, such as schizophrenia, Alzheimer’s, and Parkinson’s diseases, the alteration in the catecholaminergic system leads to cognitive impairments. Schizophrenia is a mental disorder characterized by psychotic events that alter beliefs, perceptions, and emotions (Kapur, 2003). It has been demonstrated that over-activation of the dopaminergic pathways is associated with schizophrenia (Brisch et al., 2014; Winton-Brown et al., 2014). Consequently, dysregulated dopamine transmission leads to a stimulus-independent release of dopamine, generating an aberrant assignment of salience to external objects, exaggerating the perception (Kapur, 2003). Thus, aberrant perception salience involves attentional resources during irrelevant stimuli coding and drives cognitive processes inappropriately (Roiser et al., 2013). This distorted perception results from excess dopamine signaling within several brain areas, such as the ventral striatum, the prefrontal cortex. and the hippocampus (please see Kapur, 2003; Roiser et al., 2013). Noticeably, dopaminergic alterations in functional connectivity have been reported in the salience network, including dysfunctional connectivity in the anterior insular and anterior cingulate cortices, which correlates with excessive salience attributable to internal experiences (Rössler et al., 2020). In animal studies, systemic administration of methamphetamine hinders NOR due to dopaminergic system over-activation (Belcher et al., 2008; Herring et al., 2008; Camarasa et al., 2010; Razavi et al., 2020; Khodamoradi et al., 2022). Additionally, the dopamine transporter knockout mouse mimics specific symptoms observed in schizophrenia due to the increased dopaminergic activity; this mouse model also exhibits memory impairments, including NOR alterations (Wong et al., 2012). Most antipsychotic pharmacological treatments involve dopaminergic regulation. These drugs alleviate schizophrenia symptoms and ameliorate NOR deficits, the systemic administration of a D4 receptor agonist (Miyauchi et al., 2017), a D3 (Sun et al., 2016; Gou et al., 2017), and a D2 antagonist (McIntosh et al., 2013) improves the recognition for novel objects in animal models of schizophrenia mental disorder.
Alzheimer’s disease is a progressive neurodegenerative disorder distinguished by the accumulation of amyloid β oligomers, plaques, and neurofibrillary tangles. Recent studies have suggested that the catecholaminergic system is affected during the first stages of the pathology. The neurodegeneration initiates within the LC (Braak and Del Tredici, 2015) and the VTA (Serra et al., 2018), propagating to the medial temporal lobe and cortical regions (Flores et al., 2022; Guzmán-Ramos et al., 2022), causing cognitive impairments. Our group reported that the accumulation of β-amyloid in a transgenic mouse model of Alzheimer’s disease induces catecholaminergic neuronal loss (Moreno-Castilla et al., 2016). Importantly, in the same mouse model, animals exhibit NOR and OLRM impairments; these effects are attributable to a failure in dopamine release (Guzmán-Ramos et al., 2012; Moreno-Castilla et al., 2017). Moreover, stimulation of the dopaminergic system through the systemic administration of dopamine precursor levodopa (Ambrée et al., 2009) or a dopamine reuptake blocker (Guzmán-Ramos et al., 2012) attenuates NOR impairment observed in Alzheimer’s disease mice models. Although memory deficits are strongly related to Alzheimer’s disease, some patients exhibit sensorial alterations, including visual, olfactory, somatosensory, and auditory impairments (Mapstone et al., 2006; Daulatzai, 2016), probably due to early catecholaminergic alterations (Rey et al., 2012).
Parkinson’s disease is another progressive neurodegenerative disorder related to the dysfunction of the catecholaminergic system. This pathology is characterized by several motor symptoms caused by aggressive dopaminergic cell loss in the substantia nigra (Lotharius and Brundin, 2002). Furthermore, Parkinson’s disease patients also exhibit cognitive detriments (Aarsland, 2016). Administration of 1-methyl-4-phenyl-1,2,3,6-tetrahydropyridine, a selective dopaminergic cell neurotoxin, into the substantia nigra is widely used as an animal model for Parkinson’s disease; rats treated with this neurotoxin display degeneration of nigrostriatal dopaminergic neurons and NOR impairment (Sy et al., 2010; Chang and Wang, 2021). The administration of 6-OHDA into the striatum generates NOR (Chao et al., 2013; Masini et al., 2018) and OLRM deficits (Xie and Prasad, 2020; Barón-Quiroz et al., 2021). Moreover, the chronic treatment of reserpine, a monoamine-depleting agent, is also employed as a pharmacological model of Parkinson’s disease in rodents; this animal model likewise shows long-term NOR memory impairment (Ikram and Haleem, 2019). A transgenic mice model of Parkinson’s disease (MitoPark) resembles progressive neurodegeneration and death of dopaminergic neurons, loss of motor function, and deficits in NOR tasks (Li et al., 2013). This evidence shows that NOR and OLRM memory decline accompanies dopaminergic dysfunction in Parkinson’s disease models. Although these Parkinson’s disease models are suitable for emulating motor alterations, it is essential to mention that cognitive deficits in NOR and OLRM tasks appear before motor symptoms. Recognition memory impairments observed in Parkinson’s disease could also be explained due to alterations in perception, visual hallucinations being the most frequently observed in Parkinson’s patients (for a review, Russo et al., 2019); probably caused by alterations in the dopaminergic system.
Therefore, deficits in NOR and OLRM task performance exhibited in many animal models of brain disorders result from dopaminergic dysregulation. Catecholaminergic alterations reported as hypoactivation or hyperactivation disrupt the finely tuned dopaminergic system, probably negatively impacting the salience circuits involved in recognition memory. Thus, Alzheimer’s, Parkinson’s, schizophrenia, and other brain disorders related to altered catecholaminergic signaling might alter the integration of information required for perceptual salience and the subsequent cognitive processes such as memory, attention, and motivation.
Conclusions
Relevant information is more efficiently processed in comparison to non-relevant stimuli. Selection of relevant information from the environment is accomplished since intense physical properties are intrinsically salient. However, salience could also be modulated by “internal” factors according to the observer’s experience. Hence, perceptual processing occurs according to the intense physical properties of the stimulus or the previously learned information. Former perceptual processing requires a close interaction between perception and memory, considering that integrated memories modulate perception and salience processing. Here, we propose the term perceptual salience to explain how memory mediates the integration of inconspicuous stimuli into a relevant memory trace, facilitating salience detection in future encounters without apparently altering the recognition of the physical attributes or valence of the stimuli.
Memory is a fundamental cognitive function that integrates information allowing recognition of familiar (non-salient) events from novel (salient) ones. In general, recognition memory involves acquiring, consolidating, retrieving, and updating two differential processes: recollection and familiarity. Recollection recovers the characteristics of the stimulus within a context, whereas familiarity integrates whether a stimulus is new or has already been experienced. Several brain regions integrate new information learning; the hippocampus, prefrontal cortex, parahippocampal (perirhinal, entorhinal, and postrhinal), and insular cortices are widely involved in recognition memory. Moreover, the catecholaminergic system modulates cognitive functions, including recognition memory. The main catecholaminergic inputs arise from the LC and the VTA, which innervate several brain structures related to recognition memory. Novel object and object location recognition memory are the most common behavioral paradigms employed to determine recognition memory’s acquisition, consolidation, retrieval, and updating due to the natural tendency of rodents to explore novel stimuli. Exposure to novel stimuli or spatial configuration induces a dopamine release, modulating dopaminergic receptors that strengthen learning signals, and facilitating the transition of novelty to familiarity. Mainly, dopaminergic activity within the perirhinal and insular cortices and the hippocampus mediates the consolidation process of perceptual salience. Dopamine regulates plasticity-related events that enhance memory consolidation and persistence, regardless of the initial sensory perception, improving perceptual salience during recognition memory. Importantly, brain disorders caused by neurodegenerative diseases such as Alzheimer’s or Parkinson’s, metabolic disorders, or schizophrenia alter recognition memory due to dopaminergic dysfunction, probably related to the distortion in perceptual salience and the subsequent cognition processes.
Author Contributions
The authors confirm contribution to the manuscript as follows: DO-G, KG-R, and FB-R conceptualized, reviewed, and edited the manuscript; preparation of draft manuscript by DO-G. All authors contributed to the article and approved the submitted version.
Funding
This work was supported by Departamento de Ciencias de la Salud to KG-R, DGAPA-PAPIIT IN212919 to FB-R, and DGAPA-PAPIIT IA202922 to DO-G.
Conflict of Interest
The authors declare that the research was conducted in the absence of any commercial or financial relationships that could be construed as a potential conflict of interest.
Publisher’s Note
All claims expressed in this article are solely those of the authors and do not necessarily represent those of their affiliated organizations, or those of the publisher, the editors and the reviewers. Any product that may be evaluated in this article, or claim that may be made by its manufacturer, is not guaranteed or endorsed by the publisher.
Acknowledgments
We thank Dr. Luis Rodriguez-Duran for his technical assistance.
References
Aarsland, D. (2016). Cognitive impairment in Parkinson’s disease and dementia with Lewy bodies. Parkinsonism Relat. Disord. 22, S144–S148. doi: 10.1016/j.parkreldis.2015.09.034
Akirav, I., and Maroun, M. (2006). Ventromedial prefrontal cortex is obligatory for consolidation and reconsolidation of object recognition memory. Cereb. Cortex 16, 1759–1765. doi: 10.1093/cercor/bhj114
Alger, S. E., Chen, S., and Payne, J. D. (2019). Do different salience cues compete for dominance in memory over a daytime nap? Neurobiol. Learn. Mem. 160, 48–57. doi: 10.1016/j.nlm.2018.06.005
Ambrée, O., Richter, H., Sachser, N., Lewejohann, L., Dere, E., de Souza Silva, M. A., et al. (2009). Levodopa ameliorates learning and memory deficits in a murine model of Alzheimer’s disease. Neurobiol. Aging 30, 1192–1204. doi: 10.1016/j.neurobiolaging.2007.11.010
Ameen-Ali, K. E., Easton, A., and Eacott, M. J. (2015). Moving beyond standard procedures to assess spontaneous recognition memory. Neurosci. Biobehav. Rev. 53, 37–51. doi: 10.1016/j.neubiorev.2015.03.013
Assini, F. L., Duzzioni, M., and Takahashi, R. N. (2009). Object location memory in mice: pharmacological validation and further evidence of hippocampal CA1 participation. Behav. Brain Res. 204, 206–211. doi: 10.1016/j.bbr.2009.06.005
Aston-Jones, G., and Cohen, J. D. (2005). An integrative theory of locus coeruleus-norepinephrine function: adaptive gain and optimal performance. Annu. Rev. Neurosci. 28, 403–450. doi: 10.1146/annurev.neuro.28.061604.135709
Awh, E., Belopolsky, A. V., and Theeuwes, J. (2012). Top-down versus bottom-up attentional control: a failed theoretical dichotomy. Trends Cogn. Sci. 16, 437–443. doi: 10.1016/j.tics.2012.06.010
Baik, J.-H. (2020). Stress and the dopaminergic reward system. Exp. Mol. Med. 52, 1879–1890. doi: 10.1038/s12276-020-00532-4
Balderas, I., Moreno-Castilla, P., and Bermudez-Rattoni, F. (2013a). Dopamine D1 receptor activity modulates object recognition memory consolidation in the perirhinal cortex but not in the hippocampus. Hippocampus 23, 873–878. doi: 10.1002/hipo.22143
Balderas, I., Rodriguez-Ortiz, C. J., and Bermudez-Rattoni, F. (2013b). Retrieval and reconsolidation of object recognition memory are independent processes in the perirhinal cortex. Neuroscience 253, 398–405. doi: 10.1016/j.neuroscience.2013.09.001
Balderas, I., Rodriguez-Ortiz, C. J., and Bermudez-Rattoni, F. (2015). Consolidation and reconsolidation of object recognition memory. Behav. Brain Res. 285, 213–222. doi: 10.1016/j.bbr.2014.08.049
Balderas, I., Rodriguez-Ortiz, C. J., Salgado-Tonda, P., Chavez-Hurtado, J., McGaugh, J. L., Bermudez-Rattoni, F., et al. (2008). The consolidation of object and context recognition memory involve different regions of the temporal lobe. Learn. Mem. 15, 618–624. doi: 10.1101/lm.1028008
Barbosa, F. F., de Oliveira Pontes, I. M., Ribeiro, S., Ribeiro, A. M., and Silva, R. H. (2012). Differential roles of the dorsal hippocampal regions in the acquisition of spatial and temporal aspects of episodic-like memory. Behav. Brain Res. 232, 269–277. doi: 10.1016/j.bbr.2012.04.022
Barker, G. R. I., and Warburton, E. C. (2011). When is the hippocampus involved in recognition memory? J. Neurosci. 31, 10721–10731. doi: 10.1523/JNEUROSCI.6413-10.2011
Barón-Quiroz, K., García-Ramirez, M., and Chuc-Meza, E. (2021). Dopaminergic denervation of the globus pallidus produces short-memory impairment in rats. Physiol. Behav. 240:113535. doi: 10.1016/j.physbeh.2021.113535
Barsegyan, A., McGaugh, J. L., and Roozendaal, B. (2014). Noradrenergic activation of the basolateral amygdala modulates the consolidation of object-in-context recognition memory. Front. Behav. Neurosci. 8:160. doi: 10.3389/fnbeh.2014.00160
Barsegyan, A., Mirone, G., Ronzoni, G., Guo, C., Song, Q., van Kuppeveld, D., et al. (2019). Glucocorticoid enhancement of recognition memory via basolateral amygdala-driven facilitation of prelimbic cortex interactions. Proc. Natl. Acad. Sci. U S A 116, 7077–7082. doi: 10.1073/pnas.1901513116
Barto, A., Mirolli, M., and Baldassarre, G. (2013). Novelty or Surprise? Front. Psychol. 4:907. doi: 10.3389/fpsyg.2013.00907
Belcher, A. M., Feinstein, E. M., O’Dell, S. J., and Marshall, J. F. (2008). Methamphetamine influences on recognition memory: comparison of escalating and single-day dosing regimens. Neuropsychopharmacology 33, 1453–1463. doi: 10.1038/sj.npp.1301510
Ben-Yakov, A., Dudai, Y., and Mayford, M. R. (2015). Memory retrieval in mice and men. Cold Spring Harb. Perspect. Biol. 7:a021790. doi: 10.1101/cshperspect.a021790
Bermudez-Rattoni, F. (2014). The forgotten insular cortex: its role on recognition memory formation. Neurobiol. Learn. Mem. 109, 207–216. doi: 10.1016/j.nlm.2014.01.001
Bermudez-Rattoni, F., Okuda, S., Roozendaal, B., and McGaugh, J. L. (2005). Insular cortex is involved in consolidation of object recognition memory. Learn. Mem. 12, 447–449. doi: 10.1101/lm.97605
Berridge, K. C., and Robinson, T. E. (1998). What is the role of dopamine in reward: hedonic impact, reward learning, or incentive salience? Brain Res. Rev. 28, 309–369. doi: 10.1016/s0165-0173(98)00019-8
Besheer, J., Jensen, H. C., and Bevins, R. A. (1999). Dopamine antagonism in a novel-object recognition and a novel-object place conditioning preparation with rats. Behav. Brain Res. 103, 35–44. doi: 10.1016/s0166-4328(99)00021-2
Bisaz, R., Travaglia, A., and Alberini, C. M. (2014). The neurobiological bases of memory formation: from physiological conditions to psychopathology. Psychopathology 47, 347–356. doi: 10.1159/000363702
Braak, H., and Del Tredici, K. (2015). The preclinical phase of the pathological process underlying sporadic Alzheimer’s disease. Brain J. Neurol. 138, 2814–2833. doi: 10.1093/brain/awv236
Brisch, R., Saniotis, A., Wolf, R., Bielau, H., Bernstein, H.-G., Steiner, J., et al. (2014). The role of dopamine in schizophrenia from a neurobiological and evolutionary perspective: old fashioned, but still in vogue. Front. Psychiatry 5:47. doi: 10.3389/fpsyt.2014.00047
Brischoux, F., Chakraborty, S., Brierley, D. I., and Ungless, M. A. (2009). Phasic excitation of dopamine neurons in ventral VTA by noxious stimuli. Proc. Natl. Acad. Sci. U S A 106, 4894–4899. doi: 10.1073/pnas.0811507106
Bromberg-Martin, E. S., Matsumoto, M., and Hikosaka, O. (2010). Dopamine in motivational control: rewarding, aversive and alerting. Neuron 68, 815–834. doi: 10.1016/j.neuron.2010.11.022
Brown, M. W., and Aggleton, J. P. (2001). Recognition memory: what are the roles of the perirhinal cortex and hippocampus? Nat. Rev. Neurosci. 2, 51–61. doi: 10.1038/35049064
Camarasa, J., Rodrigo, T., Pubill, D., and Escubedo, E. (2010). Memantine is a useful drug to prevent the spatial and non-spatial memory deficits induced by methamphetamine in rats. Pharmacol. Res. 62, 450–456. doi: 10.1016/j.phrs.2010.05.004
Chan, M., Eacott, M. J., Sanderson, D. J., Wang, J., Sun, M., Easton, A., et al. (2018). Continual trials spontaneous recognition tasks in mice: reducing animal numbers and improving our understanding of the mechanisms underlying memory. Front. Behav. Neurosci. 12:214. doi: 10.3389/fnbeh.2018.00214
Chang, E., and Wang, J. (2021). Brain-derived neurotrophic factor attenuates cognitive impairment and motor deficits in a mouse model of Parkinson’s disease. Brain Behav. 11:e2251. doi: 10.1002/brb3.2251
Chang, P.-K., Chu, J., Tsai, Y.-T., Lai, Y.-H., and Chen, J.-C. (2020). Dopamine D3 receptor and GSK3β signaling mediate deficits in novel object recognition memory within dopamine transporter knockdown mice. J. Biomed. Sci. 27:16. doi: 10.1186/s12929-019-0613-y
Chao, O. Y.-H., Pum, M. E., and Huston, J. P. (2013). The interaction between the dopaminergic forebrain projections and the medial prefrontal cortex is critical for memory of objects: implications for Parkinson’s disease. Exp. Neurol. 247, 373–382. doi: 10.1016/j.expneurol.2013.01.003
Chen, G., Greengard, P., and Yan, Z. (2004). Potentiation of NMDA receptor currents by dopamine D1 receptors in prefrontal cortex. Proc. Natl. Acad. Sci. U S A 101, 2596–2600. doi: 10.1073/pnas.0308618100
Chen, Y.-F., Song, Q., Colucci, P., Maltese, F., Siller-Pérez, C., Prins, K., et al. (2022). Basolateral amygdala activation enhances object recognition memory by inhibiting anterior insular cortex activity. Proc. Natl. Acad. Sci. U S A 119:e2203680119. doi: 10.1073/pnas.2203680119
Cho, J. R., Treweek, J. B., Robinson, J. E., Xiao, C., Bremner, L. R., Greenbaum, A., et al. (2017). Dorsal raphe dopamine neurons modulate arousal and promote wakefulness by salient stimuli. Neuron 94, 1205–1219.e8. doi: 10.1016/j.neuron.2017.05.020
Cohen, S. J., Munchow, A. H., Rios, L. M., Zhang, G., Asgeirsdóttir, H. N., Stackman, R. W., et al. (2013). The rodent hippocampus is essential for nonspatial object memory. Curr. Biol. 23, 1685–1690. doi: 10.1016/j.cub.2013.07.002
Cohen, S. J., Stackman R. W., Jr. (2015). Assessing rodent hippocampal involvement in the novel object recognition task. A review. Behav. Brain Res. 285, 105–117. doi: 10.1016/j.bbr.2014.08.002
Comoli, E., Coizet, V., Boyes, J., Bolam, J. P., Canteras, N. S., Quirk, R. H., et al. (2003). A direct projection from superior colliculus to substantia nigra for detecting salient visual events. Nat. Neurosci. 6, 974–980. doi: 10.1038/nn1113
Daulatzai, M. A. (2016). Dysfunctional sensory modalities, locus coeruleus and basal forebrain: early determinants that promote neuropathogenesis of cognitive and memory decline and Alzheimer’s disease. Neurotox. Res. 30, 295–337. doi: 10.1007/s12640-016-9643-3
de Lafuente, V., and Romo, R. (2011). Dopamine neurons code subjective sensory experience and uncertainty of perceptual decisions. Proc. Natl. Acad. Sci. U S A 108, 19767–19771. doi: 10.1073/pnas.1117636108
de Lima, M. N. M., Presti-Torres, J., Dornelles, A., Scalco, F. S., Roesler, R., Garcia, V. A., et al. (2011). Modulatory influence of dopamine receptors on consolidation of object recognition memory. Neurobiol. Learn. Mem. 95, 305–310. doi: 10.1016/j.nlm.2010.12.007
Dornelles, A., de Lima, M. N. M., Grazziotin, M., Presti-Torres, J., Garcia, V. A., Scalco, F. S., et al. (2007). Adrenergic enhancement of consolidation of object recognition memory. Neurobiol. Learn. Mem. 88, 137–142. doi: 10.1016/j.nlm.2007.01.005
Duszkiewicz, A. J., McNamara, C. G., Takeuchi, T., and Genzel, L. (2019). Novelty and dopaminergic modulation of memory persistence: a tale of two systems. Trends Neurosci. 42, 102–114. doi: 10.1016/j.tins.2018.10.002
Düzel, E., Bunzeck, N., Guitart-Masip, M., Wittmann, B., Schott, B. H., Tobler, P. N., et al. (2009). Functional imaging of the human dopaminergic midbrain. Trends Neurosci. 32, 321–328. doi: 10.1016/j.tins.2009.02.005
Ennaceur, A., and Delacour, J. (1988). A new one-trial test for neurobiological studies of memory in rats. 1: behavioral data. Behav. Brain Res. 31, 47–59. doi: 10.1016/0166-4328(88)90157-x
Evans, L. H., and Wilding, E. L. (2012). Recollection and familiarity make independent contributions to memory judgments. J. Neurosci. 32, 7253–7257. doi: 10.1523/JNEUROSCI.6396-11.2012
Feenstra, M. G. P., and Botterblom, M. H. A. (1996). Rapid sampling of extracellular dopamine in the rat prefrontal cortex during food consumption, handling and exposure to novelty. Brain Res. 742, 17–24. doi: 10.1016/s0006-8993(96)00945-6
Feenstra, M. G. P., Botterblom, M. H. A., and Mastenbroek, S. (2000). Dopamine and noradrenaline efflux in the prefrontal cortex in the light and dark period: effects of novelty and handling and comparison to the nucleus accumbens. Neuroscience 100, 741–748. doi: 10.1016/s0306-4522(00)00319-5
Fiorillo, C. D. (2013). Two dimensions of value: dopamine neurons represent reward but not aversiveness. Science 341, 546–549. doi: 10.1126/science.1238699
Flores, R. d., Das, S. R., Xie, L., Wisse, L. E. M., Lyu, X., Shah, P., et al. (2022). Medial temporal lobe networks in Alzheimer’s disease: structural and molecular vulnerabilities. J. Neurosci. 42, 2131–2141. doi: 10.1523/JNEUROSCI.0949-21.2021
Frankland, P. W., Josselyn, S. A., and Köhler, S. (2019). The neurobiological foundation of memory retrieval. Nat. Neurosci. 22, 1576–1585. doi: 10.1038/s41593-019-0493-1
Frey, U., and Morris, R. G. (1998). Synaptic tagging: implications for late maintenance of hippocampal long-term potentiation. Trends Neurosci. 21, 181–188. doi: 10.1016/s0166-2236(97)01189-2
Furini, C. R. G., Myskiw, J. C., Schmidt, B. E., Marcondes, L. A., and Izquierdo, I. (2014). D1 and D5 dopamine receptors participate on the consolidation of two different memories. Behav. Brain Res. 271, 212–217. doi: 10.1016/j.bbr.2014.06.027
Gao, C., and Wolf, M. E. (2008). Dopamine receptors regulate NMDA receptor surface expression in prefrontal cortex neurons. J. Neurochem. 106, 2489–2501. doi: 10.1111/j.1471-4159.2008.05597.x
Gil-Lievana, E., Balderas, I., Moreno-Castilla, P., Luis-Islas, J., McDevitt, R. A., Tecuapetla, F., et al. (2020). Glutamatergic basolateral amygdala to anterior insular cortex circuitry maintains rewarding contextual memory. Commun. Biol. 3, 1–11. doi: 10.1038/s42003-020-0862-z
Gil-Lievana, E., Ramírez-Mejía, G., Urrego-Morales, O., Luis-Islas, J., Gutierrez, R., Bermúdez-Rattoni, F., et al. (2022). Photostimulation of ventral tegmental area-insular cortex dopaminergic inputs enhances the salience to consolidate aversive taste recognition memory via D1-like receptors. Front. Cell. Neurosci. 16:823220. doi: 10.3389/fncel.2022.823220
Goldstein, E. B., and Cacciamani, L. (2021). Sensation and Perception, 11th edition. (Boston, MA: Cengage Learning.). Available online at: doi: https://web.s.ebscohost.com/ehost/pdfviewer/pdfviewer?vid=0&sid=47ff1d62-f9b0-4904-b653-302523ccfc6e%40redis
Gou, H.-Y., Sun, X., Li, F., Wang, Z.-Y., Wu, N., Su, R.-B., et al. (2017). The antipsychotic-like effects in rodents of YQA31 involve dopamine D3 and 5-HT1A receptor. Pharmacol. Rep. 69, 1125–1130. doi: 10.1016/j.pharep.2017.05.007
Grachev, I. D., Kumar, R., Ramachandran, T. S., and Szeverenyi, N. M. (2001). Cognitive interference is associated with neuronal marker N-acetyl aspartate in the anterior cingulate cortex: an in vivo 1H-MRS study of the Stroop Color-Word task. Mol. Psychiatry 6, 529–539. doi: 10.1038/sj.mp.4000940
Grillner, S., Wallén, P., Saitoh, K., Kozlov, A., and Robertson, B. (2008). Neural bases of goal-directed locomotion in vertebrates—an overview. Brain Res. Rev. 57, 2–12. doi: 10.1016/j.brainresrev.2007.06.027
Guzmán-Ramos, K., Moreno-Castilla, P., Castro-Cruz, M., McGaugh, J. L., Martínez-Coria, H., LaFerla, F. M., et al. (2012). Restoration of dopamine release deficits during object recognition memory acquisition attenuates cognitive impairment in a triple transgenic mice model of Alzheimer’s disease. Learn. Mem. 19, 453–460. doi: 10.1101/lm.026070.112
Guzmán-Ramos, K., Osorio-Gómez, D., and Bermúdez-Rattoni, F. (2022). Cognitive impairment in alzheimer’s and metabolic diseases: a catecholaminergic hypothesis. Neuroscience 306, 00271–00278. doi: 10.1016/j.neuroscience.2022.05.031
Guzmán-Ramos, K., Osorio-Gómez, D., Moreno-Castilla, P., and Bermúdez-Rattoni, F. (2010). Off-line concomitant release of dopamine and glutamate involvement in taste memory consolidation. J. Neurochem. 114, 226–236. doi: 10.1111/j.1471-4159.2010.06758.x
Haettig, J., Stefanko, D. P., Multani, M. L., Figueroa, D. X., McQuown, S. C., Wood, M. A., et al. (2011). HDAC inhibition modulates hippocampus-dependent long-term memory for object location in a CBP-dependent manner. Learn. Mem. 18, 71–79. doi: 10.1101/lm.1986911
Henson, R. N., and Gagnepain, P. (2010). Predictive, interactive multiple memory systems. Hippocampus. 20, 1315–1326. doi: 10.1002/hipo.20857
Hernández-Ramírez, S., Osorio-Gómez, D., Escobar, M. L., Rodríguez-Durán, L., Velasco, M., Bermúdez-Rattoni, F., et al. (2021). Catecholaminergic stimulation restores high-sucrose diet-induced hippocampal dysfunction. Psychoneuroendocrinology 127:105178. doi: 10.1016/j.psyneuen.2021.105178
Herring, N. R., Schaefer, T. L., Gudelsky, G. A., Vorhees, C. V., and Williams, M. T. (2008). Effect of +-methamphetamine on path integration learning, novel object recognition and neurotoxicity in rats. Psychopharmacology (Berl) 199, 637–650. doi: 10.1007/s00213-008-1183-y
Heurley, L. P., and Ferrier, L. P. (2015). What are memory-perception interactions for? Implications for action. Front. Psychol. 5:1553. doi: 10.3389/fpsyg.2014.01553
Horvitz, J. C. (2000). Mesolimbocortical and nigrostriatal dopamine responses to salient non-reward events. Neuroscience 96, 651–656. doi: 10.1016/s0306-4522(00)00019-1
Hotte, M., Naudon, L., and Jay, T. M. (2005). Modulation of recognition and temporal order memory retrieval by dopamine D1 receptor in rats. Neurobiol. Learn. Mem. 84, 85–92. doi: 10.1016/j.nlm.2005.04.002
Hotte, M., Thuault, S., Lachaise, F., Dineley, K. T., Hemmings, H. C., Nairn, A. C., et al. (2006). D1 receptor modulation of memory retrieval performance is associated with changes in pCREB and pDARPP-32 in rat prefrontal cortex. Behav. Brain Res. 171, 127–133. doi: 10.1016/j.bbr.2006.03.026
Hunsaker, M. R., Fieldsted, P. M., Rosenberg, J. S., and Kesner, R. P. (2008). Dissociating the roles of dorsal and ventral CA1 for the temporal processing of spatial locations, visual objects and odors. Behav. Neurosci. 122, 643–650. doi: 10.1037/0735-7044.122.3.643
Ihalainen, J. A., Riekkinen, P., and Feenstra, M. G. (1999). Comparison of dopamine and noradrenaline release in mouse prefrontal cortex, striatum and hippocampus using microdialysis. Neurosci. Lett. 277, 71–74. doi: 10.1016/s0304-3940(99)00840-x
Ikram, H., and Haleem, D. J. (2019). Repeated treatment with a low dose of reserpine as a progressive model of Parkinson’s dementia. Pak. J. Pharm. Sci. 32, 555–562. Available online at: doi: https://web.s.ebscohost.com/ehost/pdfviewer/pdfviewer?vid=0&sid=47ff1d62-f9b0-4904-b653-302523ccfc6e%40redis
Jay, T. M. (2003). Dopamine: a potential substrate for synaptic plasticity and memory mechanisms. Prog. Neurobiol. 69, 375–390. doi: 10.1016/s0301-0082(03)00085-6
Kafkas, A., and Montaldi, D. (2014). Two separate, but interacting, neural systems for familiarity and novelty detection: a dual-route mechanism. Hippocampus 24, 516–527. doi: 10.1002/hipo.22241
Kapur, S. (2003). Psychosis as a state of aberrant salience: a framework linking biology, phenomenology and pharmacology in schizophrenia. Am. J. Psychiatry 160, 13–23. doi: 10.1176/appi.ajp.160.1.13
Kempadoo, K. A., Mosharov, E. V., Choi, S. J., Sulzer, D., and Kandel, E. R. (2016). Dopamine release from the locus coeruleus to the dorsal hippocampus promotes spatial learning and memory. Proc. Natl. Acad. Sci. U S A 113, 14835–14840. doi: 10.1073/pnas.1616515114
Khodamoradi, M., Tirgar, F., Ghazvini, H., Rafaiee, R., Tamijani, S. M. S., Karimi, N., et al. (2022). Role of the cannabinoid CB1 receptor in methamphetamine-induced social and recognition memory impairment. Neurosci. Lett. 779:136634. doi: 10.1016/j.neulet.2022.136634
Kwapis, J. L., Alaghband, Y., Keiser, A. A., Dong, T. N., Michael, C. M., Rhee, D., et al. (2020). Aging mice show impaired memory updating in the novel OUL updating paradigm. Neuropsychopharmacology 45, 337–346. doi: 10.1038/s41386-019-0438-0
Lee, I., Hunsaker, M. R., and Kesner, R. P. (2005). The role of hippocampal subregions in detecting spatial novelty. Behav. Neurosci. 119, 145–153. doi: 10.1037/0735-7044.119.1.145
Lee, J. L. C., Nader, K., and Schiller, D. (2017). An update on memory reconsolidation updating. Trends Cogn. Sci. 21, 531–545. doi: 10.1016/j.tics.2017.04.006
Legault, M., and Wise, R. A. (2001). Novelty-evoked elevations of nucleus accumbens dopamine: dependence on impulse flow from the ventral subiculum and glutamatergic neurotransmission in the ventral tegmental area. Eur. J. Neurosci. 13, 819–828. doi: 10.1046/j.0953-816x.2000.01448.x
Lemon, N., and Manahan-Vaughan, D. (2006). Dopamine D1/D5 receptors gate the acquisition of novel information through hippocampal long-term potentiation and long-term depression. J. Neurosci. 26, 7723–7729. doi: 10.1523/JNEUROSCI.1454-06.2006
Leonibus, E. D., Verheij, M. M. M., Mele, A., and Cools, A. (2006). Distinct kinds of novelty processing differentially increase extracellular dopamine in different brain regions. Eur. J. Neurosci. 23, 1332–1340. doi: 10.1111/j.1460-9568.2006.04658.x
Li, X., Redus, L., Chen, C., Martinez, P. A., Strong, R., Li, S., et al. (2013). Cognitive dysfunction precedes the onset of motor symptoms in the mitopark mouse model of Parkinson’s disease. PLoS One 8:e71341. doi: 10.1371/journal.pone.0071341
Li, Y.-C., Liu, G., Hu, J.-L., Gao, W.-J., and Huang, Y.-Q. (2010). Dopamine D1 receptor-mediated enhancement of NMDA receptor trafficking requires rapid PKC-dependent synaptic insertion in the prefrontal neurons. J. Neurochem. 114, 62–73. doi: 10.1111/j.1471-4159.2010.06720.x
Lima, K. R., da Rosa, A. C. d. S., Picua, S. S., de Silva, S. S., Soares, N. M., Mello-Carpes, P. B., et al. (2022). Novelty promotes recognition memory persistence by D1 dopamine receptor and protein kinase A signalling in rat hippocampus. Eur. J. Neurosci. 55, 78–90. doi: 10.1111/ejn.15568
Lisman, J. E., and Grace, A. A. (2005). The hippocampal-VTA loop: controlling the entry of information into long-term memory. Neuron 46, 703–713. doi: 10.1016/j.neuron.2005.05.002
Lisman, J., Grace, A. A., and Duzel, E. (2011). A neoHebbian framework for episodic memory; role of dopamine-dependent late LTP. Trends Neurosci. 34, 536–547. doi: 10.1016/j.tins.2011.07.006
Ljungberg, T., Apicella, P., and Schultz, W. (1992). Responses of monkey dopamine neurons during learning of behavioral reactions. J. Neurophysiol. 67, 145–163. doi: 10.1152/jn.1992.67.1.145
Lotharius, J., and Brundin, P. (2002). Pathogenesis of Parkinson’s disease: dopamine, vesicles and α-synuclein. Nat. Rev. Neurosci. 3, 932–942. doi: 10.1038/nrn983
Mandler, G. (1980). Recognizing: the judgment of previous occurrence. Psychol. Rev. 87, 252–271. doi: 10.1037/0033-295X.87.3.252
Mangiavacchi, S., and Wolf, M. E. (2004). D1 dopamine receptor stimulation increases the rate of AMPA receptor insertion onto the surface of cultured nucleus accumbens neurons through a pathway dependent on protein kinase A. J. Neurochem. 88, 1261–1271. doi: 10.1046/j.1471-4159.2003.02248.x
Mapstone, M., Logan, D., and Duffy, C. J. (2006). Cue integration for the perception and control of self-movement in ageing and Alzheimer’s disease. Brain 129, 2931–2944. doi: 10.1093/brain/awl201
Masini, D., Bonito-Oliva, A., Bertho, M., and Fisone, G. (2018). Inhibition of mTORC1 signaling reverts cognitive and affective deficits in a mouse model of Parkinson’s disease. Front. Neurol. 9:208. doi: 10.3389/fneur.2018.00208
McGaugh, J. L. (2000). Memory–a century of consolidation. Science 287, 248–251. doi: 10.1126/science.287.5451.248
McGaugh, J. L. (2013). Making lasting memories: remembering the significant. Proc. Natl. Acad. Sci. U S A 110, 10402–10407. doi: 10.1073/pnas.1301209110
McGaugh, J. L. (2015). Consolidating memories. Annu. Rev. Psychol. 66, 1–24. doi: 10.1146/annurev-psych-010814-014954
McIntosh, A. L., Ballard, T. M., Steward, L. J., Moran, P. M., and Fone, K. C. F. (2013). The atypical antipsychotic risperidone reverses the recognition memory deficits induced by post-weaning social isolation in rats. Psychopharmacology (Berl.) 228, 31–42. doi: 10.1007/s00213-013-3011-2
Mello-Carpes, P. B., da Silva de Vargas, L., Gayer, M. C., Roehrs, R., and Izquierdo, I. (2016). Hippocampal noradrenergic activation is necessary for object recognition memory consolidation and can promote BDNF increase and memory persistence. Neurobiol. Learn. Mem. 127, 84–92. doi: 10.1016/j.nlm.2015.11.014
Merkow, M. B., Burke, J. F., and Kahana, M. J. (2015). The human hippocampus contributes to both the recollection and familiarity components of recognition memory. Proc. Natl. Acad. Sci. U S A 112, 14378–14383. doi: 10.1073/pnas.1513145112
Miranda, M. I., Ramírez-Lugo, L., and Bermúdez-Rattoni, F. (2000). Cortical cholinergic activity is related to the novelty of the stimulus. Brain Res. 882, 230–235. doi: 10.1016/s0926-6410(00)00050-1
Miyauchi, M., Neugebauer, N. M., and Meltzer, H. Y. (2017). Dopamine D4 receptor stimulation contributes to novel object recognition: relevance to cognitive impairment in schizophrenia. J. Psychopharmacol. 31, 442–452. doi: 10.1177/0269881117693746
Montague, P. R., Hyman, S. E., and Cohen, J. D. (2004). Computational roles for dopamine in behavioural control. Nature 431, 760–767. doi: 10.1038/nature03015
Moreno-Castilla, P., Pérez-Ortega, R., Violante-Soria, V., Balderas, I., and Bermúdez-Rattoni, F. (2017). Hippocampal release of dopamine and norepinephrine encodes novel contextual information. Hippocampus 27, 547–557. doi: 10.1002/hipo.22711
Moreno-Castilla, P., Rodriguez-Duran, L. F., Guzman-Ramos, K., Barcenas-Femat, A., Escobar, M. L., and Bermudez-Rattoni, F. (2016). Dopaminergic neurotransmission dysfunction induced by amyloid-β transforms cortical long-term potentiation into long-term depression and produces memory impairment. Neurobiol. Aging 41, 187–199. doi: 10.1016/j.neurobiolaging.2016.02.021
Morici, J. F., Bekinschtein, P., and Weisstaub, N. V. (2015). Medial prefrontal cortex role in recognition memory in rodents. Behav. Brain Res. 292, 241–251. doi: 10.1016/j.bbr.2015.06.030
Morilak, D. A., Barrera, G., Echevarria, D. J., Garcia, A. S., Hernandez, A., Ma, S., et al. (2005). Role of brain norepinephrine in the behavioral response to stress. Prog. Neuropsychopharmacol. Biol. Psychiatry 29, 1214–1224. doi: 10.1016/j.pnpbp.2005.08.007
Mumby, D. G. (2001). Perspectives on object-recognition memory following hippocampal damage: lessons from studies in rats. Behav. Brain Res. 127, 159–181. doi: 10.1016/s0166-4328(01)00367-9
Mumby, D. G., Gaskin, S., Glenn, M. J., Schramek, T. E., and Lehmann, H. (2002). Hippocampal damage and exploratory preferences in rats: memory for objects, places and contexts. Learn. Mem. 9, 49–57. doi: 10.1101/lm.41302
Murphy, J. A., Stein, I. S., Lau, C. G., Peixoto, R. T., Aman, T. K., Kaneko, N., et al. (2014). Phosphorylation of Ser1166 on GluN2B by PKA is critical to synaptic NMDA receptor function and Ca2+ signaling in spines. J. Neurosci. 34, 869–879. doi: 10.1523/JNEUROSCI.4538-13.2014
Myskiw, J. C., Rossato, J. I., Bevilaqua, L. R. M., Medina, J. H., Izquierdo, I., and Cammarota, M. (2008). On the participation of mTOR in recognition memory. Neurobiol. Learn. Mem. 89, 338–351. doi: 10.1016/j.nlm.2007.10.002
Nadel, L., and Hardt, O. (2011). Update on memory systems and processes. Neuropsychopharmacology 36, 251–273. doi: 10.1038/npp.2010.169
Nader, K., Schafe, G. E., and Le Doux, J. E. (2000). Fear memories require protein synthesis in the amygdala for reconsolidation after retrieval. Nature 406, 722–726. doi: 10.1038/35021052
Nagai, T., Takuma, K., Kamei, H., Ito, Y., Nakamichi, N., Ibi, D., et al. (2007). Dopamine D1 receptors regulate protein synthesis-dependent long-term recognition memory via extracellular signal-regulated kinase 1/2 in the prefrontal cortex. Learn. Mem. 14, 117–125. doi: 10.1101/lm.461407
Nelson, A. J. D., Thur, K. E., Marsden, C. A., and Cassaday, H. J. (2010). Dissociable roles of dopamine within the core and medial shell of the nucleus accumbens in memory for objects and place. Behav. Neurosci. 124, 789–799. doi: 10.1037/a0021114
Neve, K. A., Seamans, J. K., and Trantham-Davidson, H. (2004). Dopamine receptor signaling. J. Recept. Signal Transduct. Res. 24, 165–205. doi: 10.1081/rrs-200029981
Nomoto, K., Schultz, W., Watanabe, T., and Sakagami, M. (2010). Temporally extended dopamine responses to perceptually demanding reward-predictive stimuli. J. Neurosci. 30, 10692–10702. doi: 10.1523/JNEUROSCI.4828-09.2010
O’Dell, T. J., Connor, S. A., Guglietta, R., and Nguyen, P. V. (2015). β-Adrenergic receptor signaling and modulation of long-term potentiation in the mammalian hippocampus. Learn. Mem. 22, 461–471. doi: 10.1016/j.pestbp.2022.105151
Osorio-Gómez, D., Bermúdez-Rattoni, F., and Guzmán-Ramos, K. R. (2021). Cortical neurochemical signaling of gustatory stimuli and their visceral consequences during the acquisition and consolidation of taste aversion memory. Neurobiol. Learn. Mem. 181:107437. doi: 10.1016/j.nlm.2021.107437
Osorio-Gómez, D., Guzmán-Ramos, K., and Bermúdez-Rattoni, F. (2016). Differential involvement of glutamatergic and catecholaminergic activity within the amygdala during taste aversion retrieval on memory expression and updating. Behav. Brain Res. 307, 120–125. doi: 10.1016/j.bbr.2016.03.038
Osorio-Gómez, D., Guzmán-Ramos, K., and Bermúdez-Rattoni, F. (2017). Memory trace reactivation and behavioral response during retrieval are differentially modulated by amygdalar glutamate receptors activity: interaction between amygdala and insular cortex. Learn. Mem. 24, 14–23. doi: 10.1101/lm.042895.116
Otani, S., Bai, J., and Blot, K. (2015). Dopaminergic modulation of synaptic plasticity in rat prefrontal neurons. Neurosci. Bull. 31, 183–190. doi: 10.1007/s12264-014-1507-3
Perez, D. M. (2020). α1-adrenergic receptors in neurotransmission, synaptic plasticity and cognition. Front. Pharmacol. 11:581098. doi: 10.3389/fphar.2020.581098
Pezze, M. A., Marshall, H. J., Fone, K. C. F., and Cassaday, H. J. (2015). Dopamine D1 receptor stimulation modulates the formation and retrieval of novel object recognition memory: role of the prelimbic cortex. Eur. Neuropsychopharmacol. 25, 2145–2156. doi: 10.1016/j.euroneuro.2015.07.018
Pudovkina, O. L., Kawahara, Y., de Vries, J., and Westerink, B. H. C. (2001). The release of noradrenaline in the locus coeruleus and prefrontal cortex studied with dual-probe microdialysis. Brain Res. 906, 38–45. doi: 10.1016/s0006-8993(01)02553-7
Puglisi-Allegra, S., and Ventura, R. (2012). Prefrontal/accumbal catecholamine system processes emotionally driven attribution of motivational salience. Rev. Neurosci. 23, 509–526. doi: 10.1515/revneuro-2012-0076
Razavi, Y., Shabani, R., Mehdizadeh, M., and Haghparast, A. (2020). Neuroprotective effect of chronic administration of cannabidiol during the abstinence period on methamphetamine-induced impairment of recognition memory in the rats. Behav. Pharmacol. 31, 385–396. doi: 10.1097/FBP.0000000000000544
Rey, N. L., Jardanhazi-Kurutz, D., Terwel, D., Kummer, M. P., Jourdan, F., Didier, A., et al. (2012). Locus coeruleus degeneration exacerbates olfactory deficits in APP/PS1 transgenic mice. Neurobiol. Aging 33, 426.e1–426.e11. doi: 10.1016/j.neurobiolaging.2010.10.009
Riener, C. (2019). New approaches and debates on top-down perceptual processing. Teach. Psychol. 46, 267–272. doi: 10.1177/0098628319853943
Rodríguez-García, G., and Miranda, M. I. (2016). Opposing roles of cholinergic and GABAergic activity in the insular cortex and nucleus basalis magnocellularis during novel recognition and familiar taste memory retrieval. J. Neurosci. 36, 1879–1889. doi: 10.1523/JNEUROSCI.2340-15.2016
Rodriguez-Ortiz, C. J., and Bermúdez-Rattoni, F. (2017). Determinants to trigger memory reconsolidation: the role of retrieval and updating information. Neurobiol. Learn. Mem. 142, 4–12. doi: 10.1016/j.nlm.2016.12.005
Roiser, J. P., Howes, O. D., Chaddock, C. A., Joyce, E. M., and McGuire, P. (2013). Neural and behavioral correlates of aberrant salience in individuals at risk for psychosis. Schizophr. Bull. 39, 1328–1336. doi: 10.1093/schbul/sbs147
Romo, R., and Rossi-Pool, R. (2020). Turning touch into perception. Neuron 105, 16–33. doi: 10.1016/j.neuron.2019.11.033
Roozendaal, B., Barsegyan, A., and Lee, S. (2007). “Adrenal stress hormones, amygdala activation and memory for emotionally arousing experiences,” in Progress in Brain Research, Stress Hormones and Post Traumatic Stress Disorder Basic Studies and Clinical Perspectives, Eds E. R. De Kloet, M. S. Oitzl and E. Vermetten (Amsterdam, Netherlands: Elsevier) 79–97.
Rossato, J. I., Bevilaqua, L. R. M., Myskiw, J. C., Medina, J. H., Izquierdo, I., and Cammarota, M. (2007). On the role of hippocampal protein synthesis in the consolidation and reconsolidation of object recognition memory. Learn. Mem. 14, 36–46. doi: 10.1101/lm.422607
Rossato, J. I., Radiske, A., Kohler, C. A., Gonzalez, C., Bevilaqua, L. R., Medina, J. H., et al. (2013). Consolidation of object recognition memory requires simultaneous activation of dopamine D1/D5 receptors in the amygdala and medial prefrontal cortex but not in the hippocampus. Neurobiol. Learn. Mem. 106, 66–70. doi: 10.1016/j.nlm.2013.07.012
Rössler, J., Rössler, W., Seifritz, E., Unterrassner, L., Wyss, T., Haker, H., et al. (2020). Dopamine-induced dysconnectivity between salience network and auditory cortex in subjects with psychotic-like experiences: a randomized double-blind placebo-controlled study. Schizophr. Bull. 46, 732–740. doi: 10.1093/schbul/sbz110
Rozas, C., Carvallo, C., Contreras, D., Carreño, M., Ugarte, G., Delgado, R., et al. (2015). Methylphenidate amplifies long-term potentiation in rat hippocampus CA1 area involving the insertion of AMPA receptors by activation of β-adrenergic and D1/D5 receptors. Neuropharmacology 99, 15–27. doi: 10.1016/j.neuropharm.2015.07.003
Russo, M., Carrarini, C., Dono, F., Rispoli, M. G., Di Pietro, M., Di Stefano, V., et al. (2019). The pharmacology of visual hallucinations in synucleinopathies. Front. Pharmacol. 10:1379. doi: 10.3389/fphar.2019.01379
Santangelo, V. (2015). Forced to remember: when memory is biased by salient information. Behav. Brain Res. 283, 1–10. doi: 10.1016/j.bbr.2015.01.013
Sara, S. J. (2000). Retrieval and Reconsolidation: toward a Neurobiology of Remembering. Learn. Mem. 7, 73–84. doi: 10.1101/lm.7.2.73
Sara, S. J., Vankov, A., and Hervé, A. (1994). Locus coeruleus-evoked responses in behaving rats: a clue to the role of noradrenaline in memory. Brain Res. Bull. 35, 457–465. doi: 10.1016/0361-9230(94)90159-7
Save, E., Poucet, B., Foreman, N., and Buhot, M. C. (1992). Object exploration and reactions to spatial and nonspatial changes in hooded rats following damage to parietal cortex or hippocampal formation. Behav. Neurosci. 106, 447–456.
Schultz, W. (1998). Predictive reward signal of dopamine neurons. J. Neurophysiol. 80, 1–27. doi: 10.1152/jn.1998.80.1.1
Schultz, W., Apicella, P., and Ljungberg, T. (1993). Responses of monkey dopamine neurons to reward and conditioned stimuli during successive steps of learning a delayed response task. J. Neurosci. 13, 900–913. doi: 10.1523/JNEUROSCI.13-03-00900.1993
Seeley, W. W., Menon, V., Schatzberg, A. F., Keller, J., Glover, G. H., Kenna, H., et al. (2007). Dissociable intrinsic connectivity networks for salience processing and executive control. J. Neurosci. 27, 2349–2356. doi: 10.1523/JNEUROSCI.5587-06.2007
Serra, L., D’Amelio, M., Di Domenico, C., Dipasquale, O., Marra, C., Mercuri, N. B., et al. (2018). In vivo mapping of brainstem nuclei functional connectivity disruption in Alzheimer’s disease. Neurobiol. Aging 72, 72–82. doi: 10.1016/j.neurobiolaging.2018.08.012
Song, Q., Bolsius, Y. G., Ronzoni, G., Henckens, M. J. A. G., and Roozendaal, B. (2021). Noradrenergic enhancement of object recognition and object location memory in mice. Stress 24, 181–188. doi: 10.1080/10253890.2020.1747427
Squire, L. R. (2009). The legacy of patient H. M. for neuroscience. Neuron 61, 6–9. doi: 10.1016/j.neuron.2008.12.023
Squire, L. R., and Zola, S. M. (1996). Structure and function of declarative and nondeclarative memory systems. Proc. Natl. Acad. Sci. U S A 93, 13515–13522. doi: 10.1073/pnas.93.24.13515
Stephen Fink, J., and Smith, G. P. (1980). Mesolimbicocortical dopamine terminal fields are necessary for normal locomotor and investigatory exploration in rats. Brain Res. 199, 359–384. doi: 10.1016/0006-8993(80)90695-2
Sun, X., Gou, H., Li, F., Lu, G., Song, R., Yang, R., et al. (2016). Y-QA31, a novel dopamine D3 receptor antagonist, exhibits antipsychotic-like properties in preclinical animal models of schizophrenia. Acta Pharmacol. Sin. 37, 322–333. doi: 10.1038/aps.2015.105
Sy, H.-N., Wu, S.-L., Wang, W.-F., Chen, C.-H., Huang, Y.-T., Liou, Y.-M., et al. (2010). MPTP-induced dopaminergic degeneration and deficits in object recognition in rats are accompanied by neuroinflammation in the hippocampus. Pharmacol. Biochem. Behav. 95, 158–165. doi: 10.1016/j.pbb.2009.12.020
Takeuchi, T., Duszkiewicz, A. J., Sonneborn, A., Spooner, P. A., Yamasaki, M., Watanabe, M., et al. (2016). Locus coeruleus and dopaminergic consolidation of everyday memory. Nature 537, 357–362. doi: 10.1038/nature19325
Titulaer, J., Björkholm, C., Feltmann, K., Malmlöf, T., Mishra, D., Bengtsson Gonzales, C., et al. (2021). The importance of ventral hippocampal dopamine and norepinephrine in recognition memory. Front. Behav. Neurosci. 15:667244. doi: 10.3389/fnbeh.2021.667244
Tsai, P.-J., Keeley, R. J., Carmack, S. A., Vendruscolo, J. C. M., Lu, H., Gu, H., et al. (2020). Converging structural and functional evidence for a rat salience network. Biol. Psychiatry 88, 867–878. doi: 10.1016/j.biopsych.2020.06.023
Tulving, E. (2002). Episodic memory: from mind to brain. Annu. Rev. Psychol. 53, 1–25. doi: 10.1146/annurev.psych.53.100901.135114
Uddin, L. Q. (2015). Salience processing and insular cortical function and dysfunction. Nat. Rev. Neurosci. 16, 55–61. doi: 10.1038/nrn3857
Undieh, A. S. (2010). Pharmacology of signaling induced by dopamine D1-like receptor activation. Pharmacol. Ther. 128, 37–60. doi: 10.1016/j.pharmthera.2010.05.003
Ungless, M. A. (2004). Dopamine: the salient issue. Trends Neurosci. 27, 702–706. doi: 10.1016/j.tins.2004.10.001
Vankov, A., Hervé-Minvielle, A., and Sara, S. J. (1995). Response to novelty and its rapid habituation in locus coeruleus neurons of the freely exploring rat. Eur. J. Neurosci. 7, 1180–1187. doi: 10.1111/j.1460-9568.1995.tb01108.x
Vargas, L. S., Ramires Lima, K., Piaia Ramborger, B., Roehrs, R., Izquierdo, I., and Mello-Carpes, P. B. (2020). Catecholaminergic hippocampal activation is necessary for object recognition memory persistence induced by one-single physical exercise session. Behav. Brain Res. 379:112356. doi: 10.1016/j.bbr.2019.112356
Wagatsuma, A., Okuyama, T., Sun, C., Smith, L. M., Abe, K., and Tonegawa, S. (2018). Locus coeruleus input to hippocampal CA3 drives single-trial learning of a novel context. Proc. Natl. Acad. Sci. U S A 115, E310–E316. doi: 10.1073/pnas.1714082115
Warburton, E. C., Koder, T., Cho, K., Massey, P. V., Duguid, G., Barker, G. R. I., et al. (2003). Cholinergic neurotransmission is essential for perirhinal cortical plasticity and recognition memory. Neuron 38, 987–996. doi: 10.1016/s0896-6273(03)00358-1
Watson, D. J., Loiseau, F., Ingallinesi, M., Millan, M. J., Marsden, C. A., and Fone, K. C. (2012). Selective blockade of dopamine D3 receptors enhances while D2 receptor antagonism impairs social novelty discrimination and novel object recognition in rats: a key role for the prefrontal cortex. Neuropsychopharmacology 37, 770–786. doi: 10.1038/npp.2011.254
Winters, B. D., and Bussey, T. J. (2005). Removal of cholinergic input to perirhinal cortex disrupts object recognition but not spatial working memory in the rat. Eur. J. Neurosci. 21, 2263–2270. doi: 10.1111/j.1460-9568.2005.04055.x
Winters, B. D., Forwood, S. E., Cowell, R. A., Saksida, L. M., and Bussey, T. J. (2004). Double dissociation between the effects of peri-postrhinal cortex and hippocampal lesions on tests of object recognition and spatial memory: heterogeneity of function within the temporal lobe. J. Neurosci. 24, 5901–5908. doi: 10.1523/JNEUROSCI.1346-04.2004
Winton-Brown, T. T., Fusar-Poli, P., Ungless, M. A., and Howes, O. D. (2014). Dopaminergic basis of salience dysregulation in psychosis. Trends Neurosci. 37, 85–94. doi: 10.1016/j.tins.2013.11.003
Wong, P., Chang, C. C. R., Marx, C. E., Caron, M. G., Wetsel, W. C., and Zhang, X. (2012). Pregnenolone rescues schizophrenia-like behavior in dopamine transporter knockout mice. PLoS One 7:e51455. doi: 10.1371/journal.pone.0051455
Woolley, M. L., Marsden, C. A., Sleight, A. J., and Fone, K. C. F. (2003). Reversal of a cholinergic-induced deficit in a rodent model of recognition memory by the selective 5-HT6 receptor antagonist, Ro 04–6790. Psychopharmacology (Berl.) 170, 358–367. doi: 10.1007/s00213-003-1552-5
Wright, D. S., Bodinayake, K. K., and Kwapis, J. L. (2020). Investigating memory updating in mice using the objects in updated locations task. Curr. Protoc. Neurosci. 91:e87. doi: 10.1002/cpns.87
Xie, C., and Prasad, A. A. (2020). Probiotics treatment improves hippocampal dependent cognition in a rodent model of Parkinson’s disease. Microorganisms 8:1661. doi: 10.3390/microorganisms8111661
Yang, K., Broussard, J. I., Levine, A. T., Jenson, D., Arenkiel, B. R., and Dani, J. A. (2017). Dopamine receptor activity participates in hippocampal synaptic plasticity associated with novel object recognition. Eur. J. Neurosci. 45, 138–146. doi: 10.1111/ejn.13406
Yantis, S., and Hillstrom, A. P. (1994). Stimulus-driven attentional capture: evidence from equiluminant visual objects. J. Exp. Psychol. Hum. Percept. Perform. 20, 95–107. doi: 10.1037//0096-1523.20.1.95
Keywords: novelty, catecholamines, saliency, contextual, perception
Citation: Osorio-Gómez D, Guzman-Ramos KR and Bermudez-Rattoni F (2022) Dopamine activity on the perceptual salience for recognition memory. Front. Behav. Neurosci. 16:963739. doi: 10.3389/fnbeh.2022.963739.
Received: 07 June 2022; Accepted: 29 June 2022;
Published: 25 July 2022.
Edited by:
Flávio F. Barbosa, Federal University of Para’i-ba, BrazilReviewed by:
Janine Inez Rossato, Federal University of Rio Grande do Norte, BrazilRyuichi Nakajima, Nagoya University, Japan
Copyright © 2022 Osorio-Gómez, Guzmán-Ramos and Bermúdez-Rattoni. This is an open-access article distributed under the terms of the Creative Commons Attribution License (CC BY). The use, distribution or reproduction in other forums is permitted, provided the original author(s) and the copyright owner(s) are credited and that the original publication in this journal is cited, in accordance with accepted academic practice. No use, distribution or reproduction is permitted which does not comply with these terms.
*Correspondence: Federico Bermúdez-Rattoni, fbermude@ifc.unam.mx