Microvascular dysfunction following cardiopulmonary bypass plays a central role in postoperative organ dysfunction
- Cardiothoracic Surgery Research Laboratory, Department of Cardiothoracic Surgery, Rhode Island Hospital, Lifespan, Providence, RI, United States
Despite significant advances in surgical technique and strategies for tissue/organ protection, cardiac surgery involving cardiopulmonary bypass is a profound stressor on the human body and is associated with numerous intraoperative and postoperative collateral effects across different tissues and organ systems. Of note, cardiopulmonary bypass has been shown to induce significant alterations in microvascular reactivity. This involves altered myogenic tone, altered microvascular responsiveness to many endogenous vasoactive agonists, and generalized endothelial dysfunction across multiple vascular beds. This review begins with a survey of in vitro studies that examine the cellular mechanisms of microvascular dysfunction following cardiac surgery involving cardiopulmonary bypass, with a focus on endothelial activation, weakened barrier integrity, altered cell surface receptor expression, and changes in the balance between vasoconstrictive and vasodilatory mediators. Microvascular dysfunction in turn influences postoperative organ dysfunction in complex, poorly understood ways. Hence the second part of this review will highlight in vivo studies examining the effects of cardiac surgery on critical organ systems, notably the heart, brain, renal system, and skin/peripheral tissue vasculature. Clinical implications and possible areas for intervention will be discussed throughout the review.
1. Introduction
Open-heart operations are among the most frequently performed operations in the United States. Indeed, prior to the COVID-19 pandemic, over 350,000 coronary artery bypass grafting (CABG) operations were performed annually in the US (1). For the past several decades, most open-heart operations, including CABGs, have been performed using cardioplegia and cardiopulmonary bypass (CPB), which arrests cardiac contractility to facilitate surgery while maintaining systemic organ perfusion. Although techniques for cardiac surgery, cardioplegia, and cardiopulmonary bypass have evolved significantly over the years, the associated stress response and inflammation remain major drawbacks.
Many studies in animal models and patients have shown that cardiac operations, especially when involving cardioplegia and cardiopulmonary bypass, induce extensive vascular dysfunction (2). This dysfunction affects large- to medium-sized vessels and the microcirculation, the terminal vascular network of the systemic circulation consisting of a vast array of microvessels < 200 um in diameter. Microvessels can be further subdivided into arterioles, capillaries, and venules, all of which have important roles in maintaining organ function. Arterioles are the primary site of resistance within the vascular network. Thin-walled capillaries are the primary sites of nutrient and gas exchange in the vascular beds that supply every organ system. Finally, venules complete the process of nutrient and gas exchange and begin the process of transporting nutrient-poor, deoxygenated blood from peripheral organ systems back to the heart. Acting in concert, each element of the microcirculation plays a central role in regulating tissue and organ perfusion (3).
Microvascular dysfunction after cardiac surgery is characterized by altered myogenic and vasomotor tone as well as generalized endothelial dysfunction that, in combination, clinically manifest as systemic hypotension and organ damage (2, 4). For example, intraoperative and postoperative inflammation in the microcirculation triggers leukocyte activation, initiating the coagulation cascade in venules. Alternatively, activation of the coagulation cascade in capillaries restricts available surface area for diffusion, resulting in impaired nutrient and gas exchange. Patients may require vasopressors, aggressive intravenous electrolyte repletion, or further escalation in care postoperatively to overcome these clinical consequences.
Understanding the pathogenesis and clinical impact of micro- and macrovascular dysfunction following cardiac surgery is imperative for optimizing surgical technique and postoperative management to reduce morbidity and mortality. This review will begin with a survey of in vitro studies that examine the cellular mechanisms of microvascular dysfunction during and after cardiac surgery, paying particular attention to alterations in vascular responsiveness to vasoactive mediators, endothelial activation, and the consequences of inflammation with respect to endothelial injury and barrier integrity. The next section will discuss in vivo studies that examine the effects of ensuing microvascular dysfunction on critical organ systems, chiefly the kidneys, brain, lungs, and skin/peripheral tissue vasculature.
2. Mechanisms of microvascular dysfunction following cardiac surgery: In vitro studies
2.1. Dysregulated responses to vasoactive agonists
Figure 1 provides a framework for conceptualizing microvascular dysfunction following cardiac surgery. Extensive research has been done to characterize changes in microvascular responsiveness to vasoactive agonists following cardiac surgery. These agents include neuromodulators, hormones, and endothelium-derived factors that regulate vasomotor tone. Dysfunctional vasomotor tone manifests differently in different vascular beds, triggering reduced vascular resistance in some (e.g., skeletal muscle and peripheral circulation) and increased propensity for vasospasm in others (cardiac, pulmonary, mesenteric, and cerebral vascular circulation) (3). Aberrant vasomotor control in vessel beds supplying major organs contributes to impaired organ perfusion after cardiac surgery, which in turn promotes organ damage.
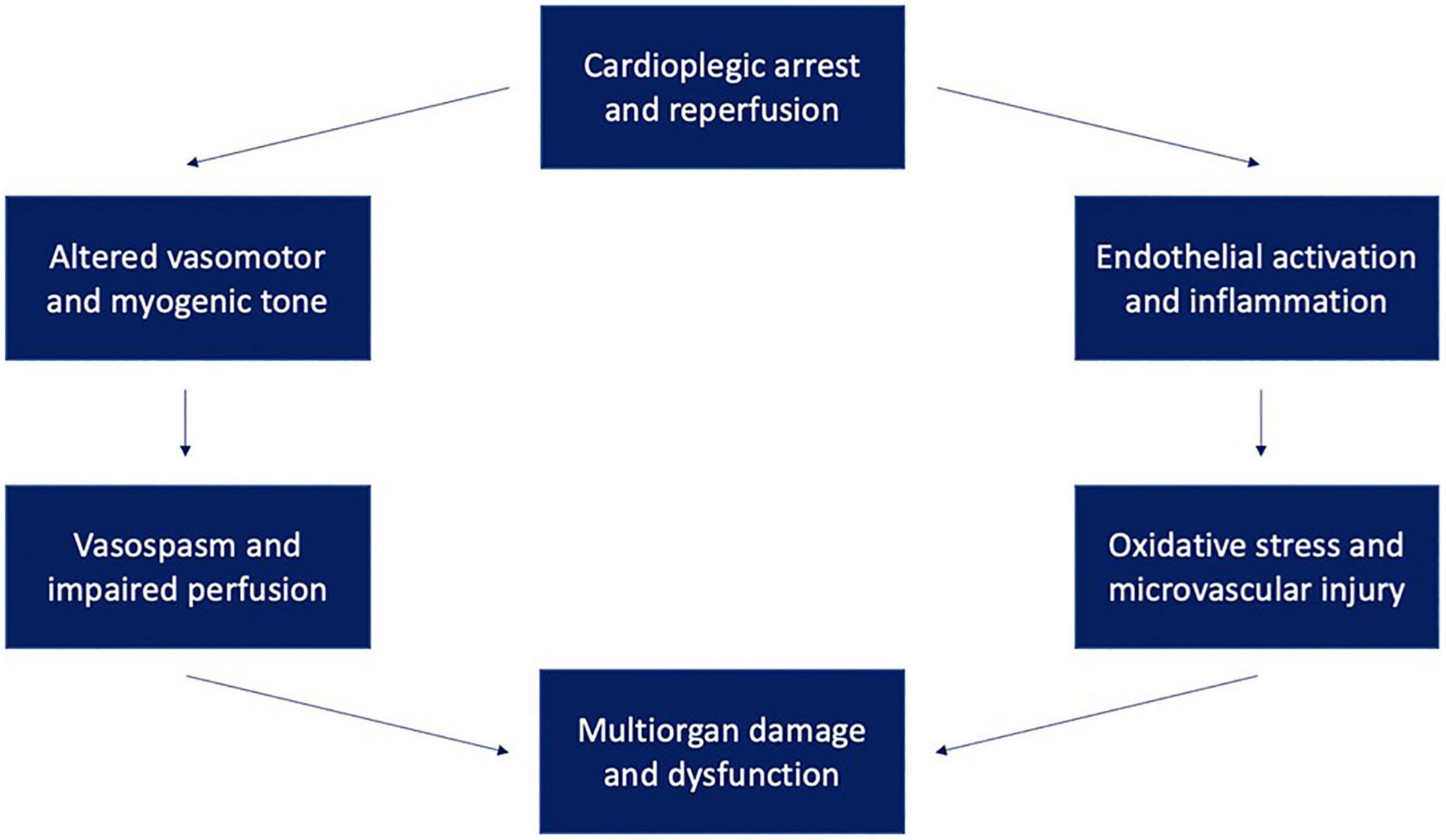
Figure 1. Ischemia-reperfusion injury following cardioplegic arrest may lead to multisystem dysfunction through multiple mechanisms. These mechanisms include endothelial activation and inflammation leading to oxidative stress and altered vasomotor and myogenic tone leading to vasospasm and organ malperfusion.
2.1.1. Neuromodulator systems
Serotonin is a critical mediator of vasomotor tone, and functions predominantly as a vasoconstrictor through the action of the 5HT2A receptor (5). However, serotonin may also act as a vasodilator in certain vascular beds, such as human skeletal muscle arterioles, through action of 5HT2B and 5HT7 receptors (5). Variable changes in serotonergic activity after CPB in different vascular beds may contribute to differing degrees of postoperative organ injury across different systems. For example, studies of human peripheral microvessels dissected from harvested skeletal muscle tissue before and after cardiac surgery involving CPB showed decreased in vitro vasomotor responses to serotonin after CPB, with no changes in receptor expression (6). These findings were replicated in the coronary microcirculation through vessels harvested from atrial tissue of patients undergoing cardiac surgery with CPB, with one key exception: in coronary vessels, authors found increased 5HT1B receptor mRNA and protein expression after surgery (7). Furthermore, atrial coronary arterioles from patients undergoing CABG showed increased serotonin-induced microvascular contraction which correlated with increased cyclooxygenase 2 (COX2) protein expression; COX2 inhibition blocked serotonin-induced human coronary microvascular contraction following acute myocardial ischemia (8, 9). Turning to the pulmonary system, swine pulmonary microvessels showed increased contractile responses to serotonin following CPB (10).
Cardiac surgery affects microvascular responsiveness to norepinephrine. Much like serotonin, norepinephrine may have vasodilatory or vasoconstrictive effects depending on the specific receptor involved. Norepinephrine is an important vasoconstrictor in many vascular beds, primarily through action of the alpha-1 adrenergic receptor (11). Meanwhile, norepinephrine may act as a vasodilator through action of beta-adrenergic receptors, in locations such as the myocardial microvasculature (beta-1 receptors) and skeletal muscle vasculature (beta-2 receptors). Changes in adrenergic activity following CPB have predominantly been studied thus far in the coronary and peripheral microcirculations. In the coronary circulation, in vitro studies of human coronary arterioles harvested before and after CPB show diminished contractile responses to the alpha-1 agonist phenylephrine, with no differences in alpha-1A or alpha-1B protein expression in harvested tissue (12). In the peripheral circulation, in vitro studies of skeletal muscle microvessels from patients before and after CPB showed diminished responsiveness to the beta-adrenergic receptor agonist isoproterenol after surgery (13). No significant changes in total protein expression of any subset of beta-adrenergic receptors were detected (13).
Cardiac surgery may affect activity of neuropeptide Y, another vasoactive neuromodulator. Neuropeptide Y receptor is expressed in human myocardial tissue, primarily in the right atrium (14, 15). Neuropeptide Y signaling has largely been studied in the coronary microcirculation, while further research will be required to elucidate any CPB-driven changes in other vascular beds. In coronary and atrial circulations, neuropeptide Y promotes coronary arteriolar vasoconstriction through action of Y1, Y2, and Y5 receptors (16). Y1 receptors may also mediate action of neuropeptide Y in the peripheral vasculature (17). Results from several studies suggest gender variations in distribution of atrial neuropeptide Y receptor following CPB. One study found that mRNA levels of neuropeptide Y1 and Y5 receptors were significantly lower after cardiac surgery in males, while neuropeptide Y, Y1 receptor, and Y5 receptor levels were significantly higher after cardiac surgery in females (18). These differences were not reflected in intercostal muscle arterioles of patients undergoing CPB, as no significant differences were found in arterial microvascular responsiveness to neuropeptide Y agonists before and after surgery (19).
Finally, cardiac surgery has been shown to influence microvascular responses to the neuromodulator vasopressin. Vasopressin stimulates vascular smooth muscle constriction through V1 receptors and is often used in clinical practice to treat postoperative hypotension and vasoplegia (20, 21). As was the case with norepinephrine, cell-and molecular level analyses of CPB-driven changes in vasopressin activity and responsiveness have largely been studied in the coronary and peripheral microcirculations. Coronary arterioles harvested from right atrial tissue of patients undergoing CPB exhibit significantly increased contractility postoperatively (12). Furthermore, postoperative V1A receptor protein levels were significantly increased compared with preoperative values (12). In contrast, human skeletal muscle arterioles showed decreased vasopressin responsiveness after CPB, suggesting that activation of particular biochemical pathways may be organ-specific (12). Additional work is required to investigate whether these changes are also present in other microcirculations perfusing other critical organs, such as the renal or pulmonary microcirculations.
Altogether, cardiac surgery significantly alters microvascular responses to key neuromodulators, including serotonin, norepinephrine, neuropeptide Y, and vasopressin.
2.1.2. Endothelium-derived agonists
Akin to neuromodulators, a variety of endothelium-derived vasomodulators exhibit altered activity and/or microvascular responsiveness after cardiac surgery, which may also contribute to impaired organ perfusion and organ damage across various key systems. One major endothelial agent is thromboxane A2, a product of endothelial cell membrane arachidonic acid metabolism (22). Thromboxane A2 causes vasoconstriction through its action on vascular smooth muscle thromboxane A2 receptors (22). Thromboxane A2 has been extensively studied in the human coronary microcirculation, where coronary arterioles from patients undergoing cardiac surgery harvested before and after cardioplegic ischemia-reperfusion exhibited attenuated contractile responses to thromboxane A2 analog U-46619 (23). No significant differences were found in the expression of thromboxane A2 receptors before and after cardioplegic ischemia-reperfusion (23). It remains an open question to what extent similar changes may occur in other organ beds.
Endothelin-1 is a potent vasoconstrictor, exerting its action through ETA receptors on vascular smooth muscle cells, and has been studied in coronary and peripheral microcirculations (24). Studies of human skeletal muscle arterioles dissected from tissue harvested from patients before and after cardiac surgery involving cardiopulmonary bypass show that in vitro contractile responses to endothelin-1 are significantly decreased after bypass, while total protein levels of ETA and ETB do not change (25). Similar results were found in human coronary arterioles harvested from patients before and after cardiac surgery involving CPB (26). Curiously, some studies have shown that plasma endothelin-1 content increases up to 85% postoperatively, suggesting that decreased microvascular responsiveness to endothelin-1 may be a function of downstream effectors. Finally, given the known role of endothelin-1 in pulmonary microvascular remodeling in pulmonary vascular disease, investigating pulmonary microvascular endothelin-1 responsiveness following CPB may provide very interesting results.
The vascular endothelium also produces many vasodilatory substances, such as nitric oxide, prostacyclin, and endothelium-derived hyperpolarizing factor (EDHF), all of which may be affected by cardiac surgery. Nitric oxide is also known as endothelial-derived relaxing factor (EDRF) and is a byproduct of arginine metabolism by nitric oxide synthase, an enzyme constitutively expressed in endothelial cells. Nitric oxide, in turn, triggers vasodilation through a guanylyl cyclase-protein kinase G signal transduction pathway (27). Three nitric oxide synthase isoforms have been identified: endothelial nitric oxide synthase (eNOS), inducible nitric oxide synthase (iNOS), and neuronal nitric oxide synthase (nNOS). Of these, eNOS and iNOS are of particular interest in microvascular dysfunction following CPB, although the specific effects of CPB on each remain a matter of debate. One in vitro study by Toprak et al. of internal mammary arteries taken from patients undergoing CABG with CPB found significantly increased eNOS immunoreactivity postoperatively, with no significant changes in iNOS (28). Meanwhile, a rat model of CPB studied by Hayashi et al. observed increased iNOS activity following CPB (29). Other in vitro studies in a variety of animal models, including dogs, pigs, and mice, have shown that eNOS is decreased in coronary and mesenteric microcirculations following cardiac surgery involving CPB (30, 31). Mayers et al. found increased plasma nitric oxide levels in a dog model of cardiopulmonary bypass, along with increased calcium-independent nitric oxide synthase activity (32).
In addition, increased free radical generation due to the systemic pro-inflammatory state induced by CPB may also affect nitric oxide levels (33). Nitric oxide can react with free radicals in several different ways. One way that nitric oxide can react with free radicals is through a process called “scavenging.” In this process, the nitric oxide molecule donates an electron to the free radical, effectively neutralizing it and preventing it from causing damage to cells or tissues. Nitric oxide can also react with certain types of free radicals to form other reactive nitrogen species, such as peroxynitrite or dinitrogen trioxide, which can have a variety of effects on cells and tissues depending on the specific circumstances.
Endothelium-derived hyperpolarizing factor (EDHF), another important vasodilator, plays a central role in an endothelium-dependent, calcium-sensitive signaling pathway that ultimately results in vascular smooth muscle hyperpolarization and vasodilation (34, 35). The ion channels responsible for driving vasodilation in the EDHF pathway are small conductance calcium-activated potassium channels, also known as SK channels (34–37). Extensive research has shown that cardiac surgery induces changes in EDHF pathways across the microvasculature. For example, human coronary microvessels harvested following CPB showed decreased vascular smooth muscle relaxation in response to SK channel activation when compared to vessels harvested before surgery, despite no changes in SK channel gene/protein expression (38, 39). In vitro studies of human skeletal muscle arterioles mirrored these results: SK channel activity is reduced following CPB independent of alterations in SK channel polypeptide levels (37).
Hence cardiac surgery has significant effects on microvascular responses to endothelium derived agonists, including vasoconstrictors such as endothelin-1 and thromboxane A2, and vasodilators such as nitric oxide, prostacyclin, and EDHF.
2.2. Inflammation and endothelial activation
2.2.1. Overview
Cardiac surgery provokes a strong systemic inflammatory response that has numerous consequences for the microvasculature. Initiating factors for inflammation include exposure of blood to the foreign material of the CPB circuit and ischemia-reperfusion injury, both of which result in increased expression of inflammatory cytokines and generation of reactive oxygen species (40). Studies have demonstrated activation of the complement system and coagulation cascades as blood passes through the CPB circuit. For example, plasma C3a levels were more than five times higher at the end of CPB than the start; this same study also found significant neutrophilia following bypass (41). In addition, plasma thrombin levels have been shown to be significantly increased following CPB (42). Likewise, a meta-analysis of coagulation parameters in patients following cardiac surgery showed that prothrombin time and activated partial thromboplastin time both increased during CPB by up to 33.3 and 17.9%. However, considerable variability was present between different studies (43).
2.2.2. Complement
Complement factors induce synthesis of pro-inflammatory cytokines that promote endothelial activation and chemokine secretion (44). Rossaint et al. investigated the effects of CPB on the leukocyte recruitment cascade, using blood samples from patients undergoing cardiac surgery (45). They found that CPB surprisingly interfered with leukocyte E-selectin-/P-selectin-endothelial ICAM1 interactions and slow rolling (45). However, CPB enhanced neutrophil Mac-1-dependent transmigration across the endothelium into tissue, the final stage of the leukocyte recruitment cascade (45). Chemokine-induced leukocyte migration was significantly enhanced as well, which explains the overall increased leukocyte recruitment during and after CPB (45). Use of leukocyte-reduced blood during cardioplegic ischemia-reperfusion in porcine models appears to promote improved postoperative myocardial perfusion and coronary and cerebral vascular function, suggesting a role for leukocyte-mediated microvascular injury following cardiac surgery (46).
The terminal complement components C5b-9, which form the membrane attack complex, can cause direct damage to the vascular endothelium, as demonstrated in a porcine model of CPB (47). The CPB pump generates shear forces that cause mechanical damage to erythrocyte cell membranes (48), thereby increasing vulnerability to the membrane attack complex. Tofukuji et al. also showed that treatment with a C5a monoclonal antibody reduced endothelial dysfunction as evidenced by endothelium-dependent relaxation responses to ADP and substance P in mesenteric arterioles (47). Other studies have found similar results in other vascular beds, such as swine pulmonary arteries (49). Hence complement activation and membrane attack complex formation are important mediators of inflammatory microvascular damage.
2.2.3. Arachidonic acid metabolism
Arachidonic acid metabolism, another significant inflammatory pathway, is also affected by cardiac surgery. Two critical enzymes that mediate key steps of arachidonic acid metabolism are cyclooxygenase (COX) 1 and 2. COX1 is a housekeeping enzyme, constitutively expressed in most cells. In contrast, COX2 is inducible, with expression increasing in response to endothelial activation and exposure to inflammatory cytokines (50). COX activity also provides a link between inflammation and modulation of vasomotor tone as two key vasoactive mediators, thromboxane A2 and prostacyclin, are both byproducts of arachidonic acid metabolism (thromboxane A2 was discussed extensively earlier).
In vitro studies show that inflammation and endothelial stress upregulate COX2 expression in human vascular endothelial cells. This correlates with increased PGI2 and decreased thromboxane A2 production, altering the balance of vasodilation (mediated by PGI2) versus vasoconstriction (mediated by thromboxane A2) in the microvasculature and increasing proclivity for vasospasm (51–53). Looking specifically at cardiac surgery, CPB increases COX2 expression throughout the microvasculature in a manner consistent with inflammation-induced endothelial injury and activation during ischemia-reperfusion (54). Furthermore, experiments in coronary microvessels harvested from patients before and after CPB showed enhanced post-bypass bradykinin-induced relaxation responses, along with increased COX2 protein expression (55). Other studies have shown that inhibiting COX2 following CPB reduces vasoconstriction, not vasodilation, suggesting that the net effect of increased COX2 activity may be increased vasoconstriction (56). Ultimately, what is clear is that arachidonic acid metabolism, and COX enzyme action in particular, play an important role in inflammatory endothelial injury in the microvasculature during cardiac surgery.
2.2.4. Ischemia-reperfusion and free radicals
Both inflammation and ischemia-reperfusion during cardiac surgery in combination lead to generation of free radicals, which when derived from oxygen, are called reactive oxygen species (ROS) (57). First, increased neutrophil activation as blood passes through the extracorporeal CPB circuit enhances NADPH phagosome oxidase activity, leading to increased production of superoxide (58, 59). CPB also increases neutrophil elastase production (60). Next, cardioplegic ischemia-reperfusion disrupts normal tissue blood flow and oxygenation, temporarily interrupting aerobic metabolism in microvascular endothelial cells and perfused tissue. Certain organs, like peripheral vasculature and their end-organs are more resistant to temporary changes in tissue oxygenation than others—including vasculature supplying cardiac or brain tissue, which exhibit functional impairment within minutes of ischemia. Even though cardioprotective refinements over the past several decades have improved outcomes significantly, ischemia-reperfusion injury remains a persistent adverse effect of CPB (61).
Ischemia during cardiac surgery triggers a cascade of events that result in increased oxidative stress (62; Figure 2). First, oxygen depletion disrupts oxidative phosphorylation, the cornerstone of aerobic metabolism, and forces cells to rely exclusively on anaerobic glycolysis. Anaerobic metabolism is considerably less efficient at generating ATP than aerobic metabolism, and results in increased lactic acid generation, thus lowering intracellular pH and creating a suboptimal environment for the function of many intracellular enzymes. Low intracellular ATP interferes with the ability of the Na+-K+ ATPase to transport Na+ and K+ across the cell membrane, ultimately disrupting resting cell membrane potential. With prolonged exposure to ischemia, intracellular calcium levels rise in ischemic cells, leading to activation of phospholipases, nucleases, and proteases that begin degrading organelles, cytoskeletal protein, and cellular DNA. One of the organelles particularly sensitive to calcium is the mitochondrion, as calcium promotes opening of mitochondrial permeability transition pores (MPTPs) that allow pro-apoptotic oxidative mitochondrial enzymes, such as cytochrome c, to leak into the cytosol and increase oxidative stress.
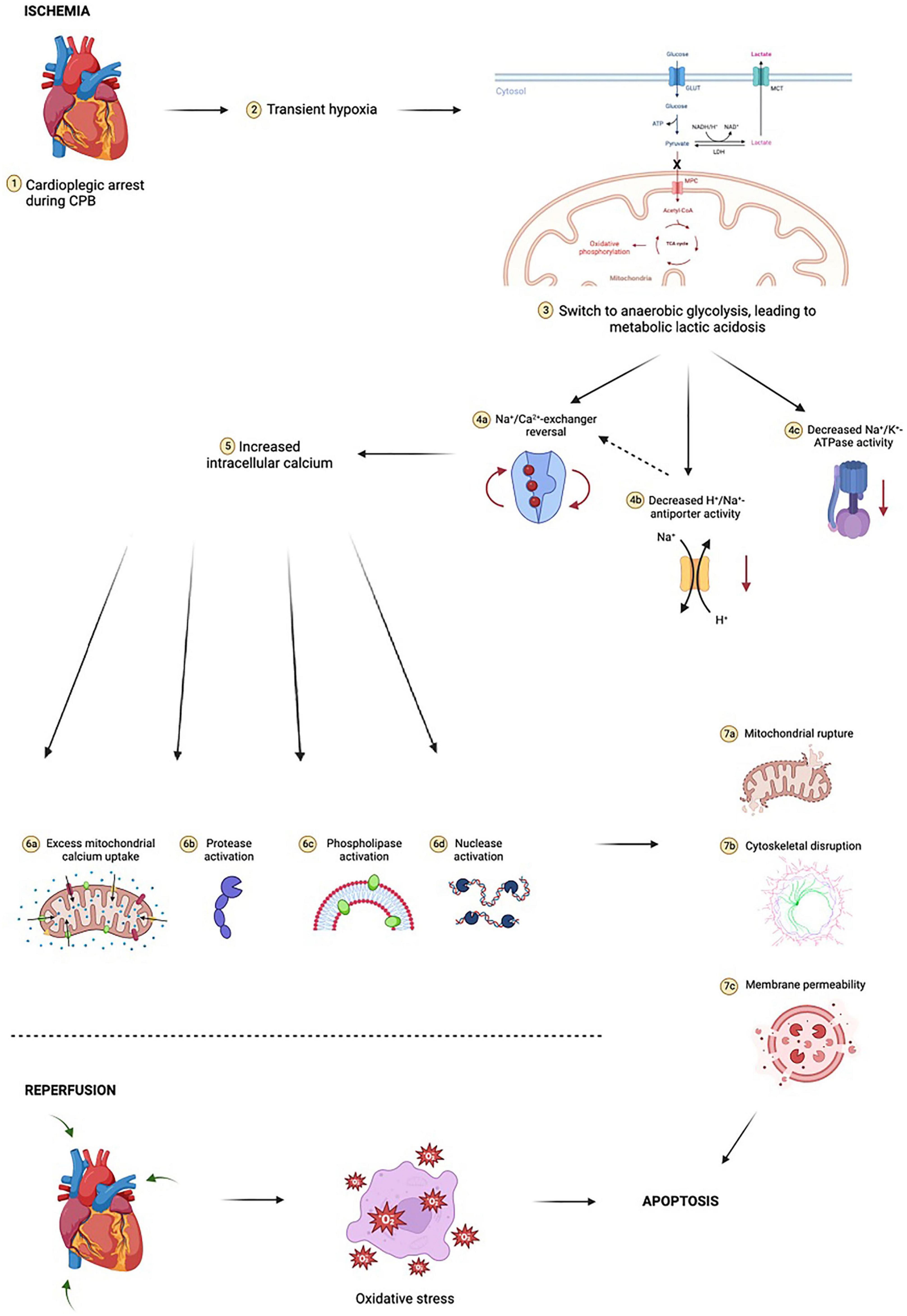
Figure 2. Ischemia-reperfusion injury is complex and multifactorial. At the cellular level, initial transient hypoxia due to cardiopulmonary bypass (CPB) leads to a switch from mitochondrial oxidative phosphorylation to anaerobic glycolysis, with a marked reduction in ATP yield. In poorly oxygenated tissues, pyruvate (the end-product of glycolysis) is shunted away from mitochondria and instead undergoes conversion to lactate by cytosolic lactate dehydrogenase (LDH). The resulting lactic acidosis in combination with high extracellular potassium and hypothermia due to cold cardioplegia inhibits the activity of Na+/K+-ATPase, an enzyme critical for maintaining physiologic resting membrane potential of cardiomyocytes close to the Nerst equilibrium potential of potassium (approximately –85 to –90 millivolts). Metabolic acidosis dually increases H+/Na+-antiporter activity, resulting in a small but notable increase in intracellular sodium, further exacerbating the sodium “window current” phenomenon during hyperkalemic cardioplegia. These electrochemical events drive the new membrane potential closer to –50 millivolts. The Na+/Ca2+-exchanger begins to operate in reverse, moving three Na+ ions out of the cardiomyocyte for every Ca2+ ion in. Voltage-gated slow calcium channels also begin to activate, leading to further calcium influx. This myocardial calcium loading in turn activates proteases, nucleases, and phospholipases, resulting in phospholipid membrane degradation, organelle destruction, accumulation of catabolic byproducts, compromised ultrastructural integrity of sarcolemma membrane integral to calcium homeostasis, and accelerated depletion of intracellular ATP stores. Prolonged exposure to ischemia increases mitochondrial calcium uptake through reversal of mitochondrial Na+/Ca2+-exchanger in a manner akin to reversal of cardiomyocyte Na+/Ca2+-exchanger. This leads to critical and irreversible damage to mitochondria. Following reperfusion, mitochondria have reduced capacity for neutralizing reactive oxygen species (ROS) and contribute to sustained oxidative stress by way of ROS production. This mitochondrial oxidative stress occurs in both cardiomyocytes as well as in the microvasculature, compounding the effects. Cytosolic cytochrome c is implicated in activating caspases involved in intrinsic apoptotic pathways. Reperfusion results in additional cardiac injury independent of ischemic insult which manifests as dysrhythmia, myocardial stunning, and myocardial necrosis. Notably, concurrent oxidative stress within the microvasculature results in microvascular dysfunction, further compounding cardiac injury. Figure created using BioRender.com.
Reperfusion after ischemia initiates yet another biochemical cascade that results in ROS generation (Figure 2). Abrupt restoration of blood flow to hypoxic tissue and reentry of oxygen into vascular endothelial cells stimulate pathways involving enzymes such as NADPH oxidase, xanthine oxidase, and nitric oxide synthase. These pathways generate primary ROS (e.g., superoxide anions) and secondary ROS (such as hydroxyl radicals, peroxynitrite, and hypochlorite) (63). Notably, red blood cell destruction due to membrane attack complex formation also results in leakage of free hemoglobin into the blood, which in the presence of hydrogen peroxide, may act as a peroxidase.
Peroxynitrite disrupts nucleic acids, proteins, and lipids. Cell and mitochondrial membrane damage due to ROS promotes apoptosis, which in the microvasculature results in extensive endothelial dysfunction and cell death (64). ROS may also further activate proapoptotic and proinflammatory pathways, such as MAPK and NFkB signaling, through nitrosylation, carbonylation, disulfide bond formation, and glutathionylation (63). An in vitro model of cardioplegic hypoxia-reoxygenation using mouse small coronary arteries and endothelial cells showed that application of the mitochondria-targeted antioxidant, Mito-Tempo, protected vascular relaxation responses after cardioplegic hypoxia-reoxygenation (65). Overall, Mito-Tempo reduced mitochondrial ROS overload, and also protected endothelial SK channel currents, suggesting a link between ROS and SK channel dysfunction after cardiac surgery. Further research in humans will be needed to verify the translational potential of mitochondrial ROS inhibition as a tool for mitigating coronary microvascular dysfunction after CPB. Altogether, ischemia-reperfusion injury, and free radicals/ROS generated in the process, serves as a critical mechanism of microvascular damage following cardiac surgery.
3. Postoperative vascular dysfunction and organ damage: In vivo studies
3.1. Vascular dysfunction in renal injury
3.1.1. Overview
Acute kidney injury (AKI) remains a common and well-established postoperative complication of cardiac surgery, in particular cardiac surgery involving CPB. AKI is defined as an abrupt decline in kidney function due to renal impairment (66). There are many causes of AKI, which may fall under one of three broad categories: pre-renal, intrinsic renal, and acute post-renal disease. Two of these categories, pre- and post-renal, reflect the consequences of extrarenal processes, while intrinsic renal disease represents pathology associated with glomeruli, interstitial vessels, or renal tubules. Pre-renal causes of AKI are linked to decreased renal perfusion, which may arise from a variety of factors including intravascular volume depletion, medication effect, sepsis, heart failure, or other causes of shock (67). Intrinsic renal causes of AKI include acute tubular necrosis due to infection, drugs, or toxins; acute interstitial nephritis, and glomerulonephritis. Postrenal causes of AKI center on urinary tract obstruction. AKI may result from a combination of causes in these three categories.
The diagnosis of AKI largely involves interpretation of serum creatinine, often in the context of a decline in urine output (68, 69). According to the Kidney Disease Improving Global Outcomes classification (KDIGO), AKI can be categorized from stage I (less severe) up to stage III (most severe) depending on serum creatinine, glomerular filtration rate (GFR), and/or need for renal replacement therapy (69).
The renal system is exquisitely vulnerable to microvascular dysfunction and subsequent hypoxemia, which drives AKI and subsequent macrohemodynamic pathology. First, the kidneys consume nearly 25% of total cardiac output, an amount necessary for sustaining glomerular filtration (70). Renal oxygen consumption per gram of tissue is also extraordinarily high despite relatively poor renal oxygen extraction; only the heart itself consumes more (2.7 mmol/kg/min for the kidneys vs. 4.3 mmol/kg/min for heart) (70). The most significant contributor to high renal oxygen demand is tubular transport (e.g., oxygen costs of ion reabsorption along the tubular network).
Renal microvascular oxygen supply and consumption are regulated by a variety of factors, chief among which are nitric oxide, angiotensin II, and HIF-1alpha (71–73). Nitric oxide-induced vasodilation augments renal blood flow and oxygen delivery, and decreased nitric oxide levels may contribute to high renal oxygen demand in the setting of chronic kidney disease (71). Angiotensin II induces renal vasoconstriction, reducing renal oxygen delivery and increasing renal oxygen demand; pathologic/hyperactive angiotensin II is also thought ot play a role in ischemic renal disorders such as hypertension and chronic kidney disease (72). HIF-1alpha can activate inducible nitric oxide synthase and VEGF to promote renal oxygen delivery and angiogenesis (73).
The incidence of AKI following cardiac surgery has been reported to be as low as 3.4% and as high as 40% (74–76). Indeed, longer duration of CPB is related to increased likelihood of postoperative AKI and acute renal failure requiring renal replacement therapy (77–80). Many factors have been shown to contribute to this, including hypotension and hemodynamic disturbances, inflammation, and nephrotoxins; Figure 3 provides an overview of factors influencing AKI after cardiac surgery.
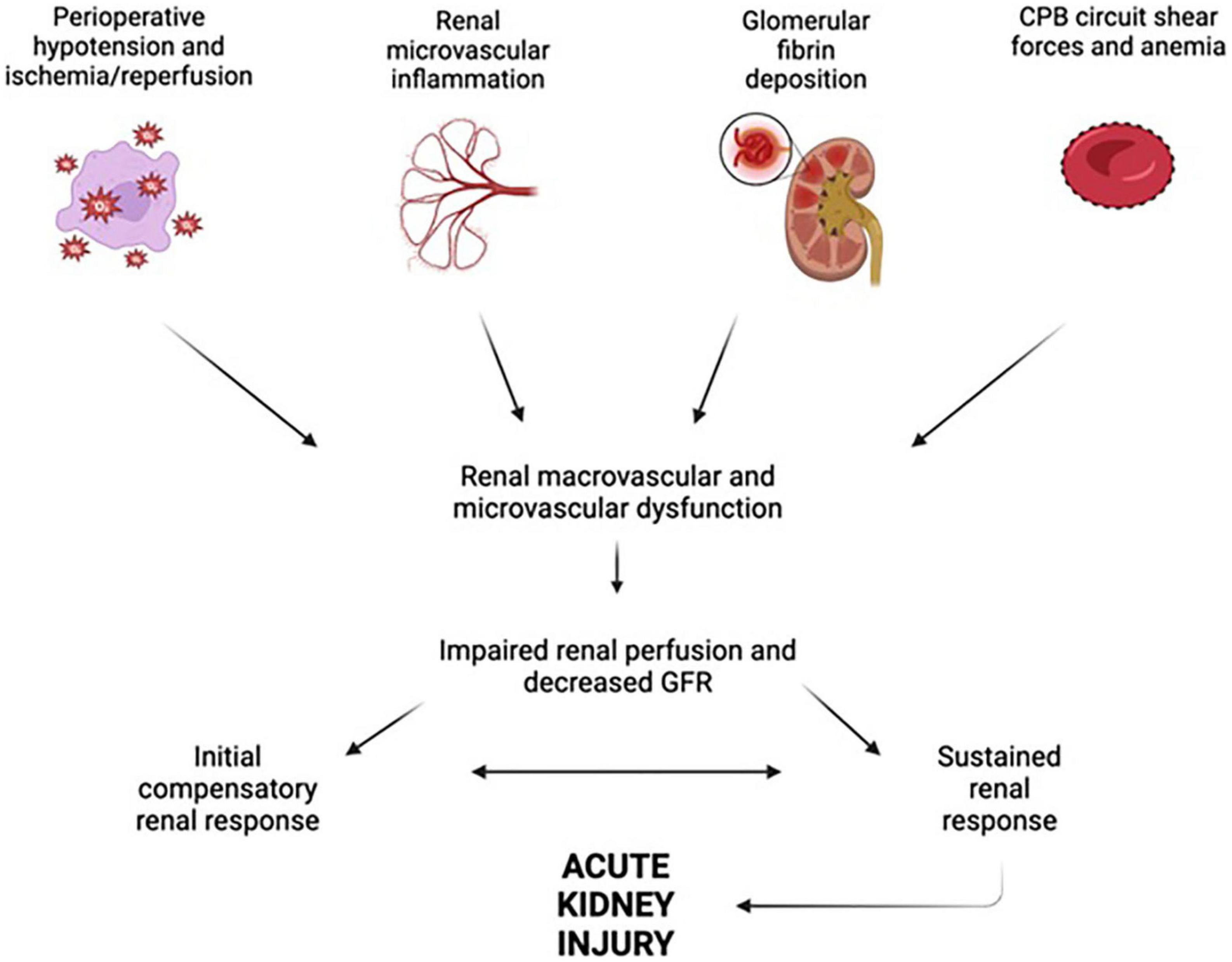
Figure 3. Factors leading to renal dysfunction during and after cardiopulmonary bypass. Perioperative hypotension, renal microvascular inflammation, glomerular fibrin deposition, and cardiopulmonary bypass (CPB) circuit shear forces all act in concert to impair renal perfusion and glomerular filtration rate (GFR), ultimately leading to acute kidney injury. Figure created using BioRender.com.
3.1.2. Perioperative hypotension and AKI after CPB
Of these factors, the most well-studied is hemodynamic instability due to sustained perioperative hypotension (81). In one retrospective study examining AKI after cardiac surgery, cardiogenic hypotension was determined to be a major factor underlying AKI in 46.3% of patients (82). Recovery from anesthesia is one potential cause of postoperative hypotension (83). However, a more significant factor driving sustained hypotension after CPB is likely vasoplegia, with a pathophysiology very similar to that of sepsis-induced vasodilatory shock (84). Indeed, hallmarks of vasoplegia include normal or augmented cardiac output in the setting of low systemic vascular resistance, leading to organ hypoperfusion (84, 85).
Potential causes of vasoplegia include altered microvascular responsiveness to vasomodulators such as serotonin, endothelin-1, vasopressin, EDHF, and thromboxane A-2, all of which were discussed extensively earlier. Inflammation and pathologic endothelial activation as blood is exposed to the CPB circuit may also contribute to abnormal postoperative microvascular myogenic tone and vasoplegia. Duration of postoperative vasoplegia and extent of microvascular recovery can be widely variable between patients; however, a large portion of patients require at least temporary period of vasopressor support to maintain blood pressure in the immediate postoperative period (83, 85).
Another retrospective study determined that duration of intraoperative hypotension was significantly associated with development of stroke, AKI, and postoperative mortality (p = 0.032) (86). There is no universally accepted definition of intraoperative hypotension. Most blood pressure value thresholds have traditionally been chosen based on what is required to maintain adequate cerebral perfusion (86). However, the lower limits of cerebral autoregulation may vary wildly, anywhere between 40 to 160 mmHg; this implies a wide range of flexibility in determining MAP during cardiac surgery involving CPB (86). Causes of intraoperative hypotension during cardiac surgery include decreased venous return from cardiac manipulation, arrhythmias, and decreased systemic vascular resistance (incipient vasoplegia, which is magnified in the postoperative period (87). However, the effect of intraoperative hypotension and reduced renal perfusion pressure during cardiac surgery on AKI may not be as clear-cut as initially thought. For example, a recent randomized controlled trial compared incidence of postoperative AKI in 90 patients who underwent cardiac surgery involving CPB, with the patients divided into a high arterial pressure group (defined in this study as MAP during CPB greater than 60 mmHg) and a control group. Total postoperative incidence of AKI was 41%, and the investigators found no significant differences between the control and high MAP groups (88). Similar findings were reported in a multicenter retrospective cohort study conducted by Kotani et al., who examined the impact of mean perfusion pressure deficit, defined as difference between MAP and central venous pressure (CVP), on AKI in over 700 adult patients admitted to 14 ICUs after elective cardiac surgery (89). Overall, no clear association between relative hypotension and AKI progression post-cardiac surgery was appreciated (89).
3.1.3. Microvascular inflammation and AKI after CPB
Another potential contributor to acute kidney injury after cardiac surgery is the systemic pro-inflammatory state induced by CPB during on-pump surgery. Zhang et al. examined associations between two key inflammatory cytokines, IL-6 and IL-10, and AKI in a six-center study of adult cardiac surgery patients (90). IL-6 and IL-10 levels increased after cardiac surgery, and higher postoperative levels of IL-6 and IL-10 were significantly associated with higher risk of AKI in a dose-dependent manner (90). A separate multicenter study of adult cardiac surgery patients conducted by Moledina et al. found that cardiac surgery led to significant increases in Th1- and Th2-dependent cytokines, most notably interferon-gamma, IL-4, and IL-13 levels (91). Patients with higher levels of IL-4, IL-13, and interferon-gamma had higher odds of postoperative AKI and mortality (91).
Intraoperative inflammation has been linked to exposure of blood to the CPB extracorporeal circuit, ischemia-reperfusion injury, and oxidative damage (92). Ischemia-reperfusion in particular triggers production of ROS through various mechanisms involving mitochondrial damage, endothelial activation, and plasma membrane breakdown (93, 94). ROS upregulate many pro-inflammatory transcription factors such as NFkB, which (1) promote expression of inflammatory cytokines, chemokines, and inflammasome formation, and (2) regulate activation and differentiation of T cells and innate immune cells (95). Immune cells, activated by cytokines, demarginate out of systemic circulation and extravasate into different organ systems including the renal parenchyma, stimulating parenchymal inflammation, tubulo-interstitial fibrosis, and AKI (96). It is important to note here that a similar cascade of microvascular inflammation is a likely contributor to postoperative organ damage in other organ systems, as will be discussed in the following sections.
3.1.4. CPB shear forces, hemoglobin, and AKI
Cardiopulmonary bypass (CPB) also exposes blood to a variety of shear forces in the extracorporeal circuit (pump, suction catheters, filters) that lyse erythrocytes and result in free hemoglobin spilling into the systemic circulation (97, 98). Circulating haptoglobin binds a portion of free hemoglobin, but haptoglobin stores may become quickly depleted in the setting of large amounts of free hemoglobin. Remaining free hemoglobin is ultimately filtered by the kidneys.
There are several ways in which hemoglobin is nephrotoxic. First, the kidneys sequester a large amount of free iron, and hemoglobin promotes intrarenal conversion of ferrous hemoglobin to ferric hemoglobin and accumulation of hemoglobin-cross linked products, which cause precipitation of Tamms Horsfall proteins in the collecting system, leading to intrinsic acute tubular injury (99). In addition, hemoglobin facilitates free radical production in the kidneys, along with enhanced expression of heat shock proteins (100). Free radicals stimulate lipid peroxidation and catalyze formation of deleterious ROS. In a case control study comparing 10 cardiac surgery patients with AKI to 10 risk-matched controls, investigators found that patients who developed AKI after cardiac surgery had twice as much plasma-free hemoglobin than controls (101). Curiously, the iron chelating agent deferoxamine was shown to decrease lipid peroxidation during CPB (102). Although some studies have demonstrated promising initial results for the use of iron chelation as a therapeutic tool to prevent AKI, specific studies examining whether intraoperative iron chelation may be protective against cardiac surgery-induced AKI are required to provide greater clarity (103).
Anemia due to CPB-related hemodilution may itself influence postoperative AKI given the exquisite sensitivity of the kidney to adequate tissue oxygenation (104). Preoperative anemia has been associated with increased incidence of AKI post-CABG with CPB (105). Moreover, a critical perioperative hemoglobin nadir of < 8.05 g/dL has been associated with AKI, acute myocardial infarction, and stroke following CPB (106). Meanwhile, Cao et al. report a critical hemoglobin threshold of > 9 g/dL with respect to incidence of AKI following CPB; although curiously, the impact of intraoperative RBC transfusions on AKI to maintain hemoglobin levels above 9 g/dL was of unclear effectiveness (104).
3.1.5. Pre-existing microvascular dysfunction and kidney injury after CPB
Pre-existing microvascular dysfunction in cardiac surgery patients may influence the likelihood of renal injury due to microvascular dysfunction during and after CPB. For example, anywhere between 30−−40% of patients undergoing cardiac surgery carry a preoperative diagnosis of DM (107). Poorly controlled diabetes/uncontrolled hyperglycemia has numerous deleterious consequences on the microvasculature, including accelerated atherosclerosis, increased capillary basement membrane thickening and extracellular matrix proliferation, and inflammation and oxidative stress resulting in endothelial injury due to advanced glycated end products (108, 109). In the renal system, a combination of diabetic micro and macroangiopathy along with direct hyperglycemic/inflammatory damage to the renal parenchyma produces characteristic histopathological changes including intimal hyalinosis of afferent and efferent arterioles leading to glomerular hyperfiltration, and ultimately diffuse diabetic glomerulosclerosis (110).
Elevated preoperative hemoglobin A1c and fasting blood glucose levels prior to CABG surgery have been linked with increased frequency of postoperative morbidity and mortality, including postoperative wound infection, bleeding, arrhythmias, and atelectasis (111). Studies specifically examining the relationship between preoperative hemoglobin A1c and postoperative renal morbidity reveal a higher incidence of postoperative AKI in CABG patients with hemoglobin A1c greater than 5.9% (112). Likewise, Wang et al. found that independent of baseline renal or cardiac function, patients with DM were more likely to have AKI after CABG (113). Furthermore, diabetic patients on insulin had increased odds ratio for AKI than diabetic patients on oral glycemic agents (113).
Another important pre-existing condition widely prevalent among cardiac surgery patients is hypertension (114), an unsurprising observation given the strong connection between hypertension and cardiovascular disease writ large. Uncontrolled hypertension induces microvascular dysfunction through abnormal vasomotor tone (enhanced vasoconstrictive and impaired vasodilatory responses) and pathological vascular remodeling (115). Increased vascular stiffness results from hypercontractility of vascular smooth muscle cells, intimal hyperplasia, advential fibrosis, and eventually hypertensive arteriolosclerosis (116).
Hypertensive vasculopathy ultimately affects every vascular bed in the body; however, particularly deleterious effects have been observed in the renal and cerebral microvasculature. In the kidneys, hypertensive arteriolosclerosis compromises blood flow to the kidneys, resulting in tubular atrophy, interstitial fibrosis, glomerular ischemia, and ultimately glomerulosclerosis (117). Despite these well-known renovascular consequences, few studies have directly examined links between preoperative hypertension and AKI following cardiac surgery, although plenty of studies have examined postoperative hypertension following cardiopulmonary bypass (118, 119). One review by Weir et al. found that overall, preexisting systolic hypertension appears to correlate well with postoperative AKI (120). However, further work is required to fully characterize the cellular and molecular-level changes underpinning these observations.
3.1.6. Techniques of perfusion and kidney injury after CPB
Finally, the form of perfusion used during CPB may also influence postoperative kidney injury. For example, use of pulsatile flow during CPB has been increasing with advancements in extracorporeal circuit technology (121). In theory, pulsatile flow more closely approximates physiological blood flow of the human heart and delivers increased mechanical energy to the vascular endothelium (122). The resulting endothelial shear stress is thought to augment release of vasodilatory substances, decrease systemic vascular resistance, and improve organ perfusion (122). With respect to the kidneys, renal tissue perfusion was substantially higher during pulsatile perfusion vs. non-pulsatile in a porcine model of CPB (123).
Milano et al. found that patients undergoing pulsatile perfusion CPB during aortic valve replacement had no significant differences in pre vs. postoperative creatine clearance, while a statistically significant difference was observed in patients undergoing normal perfusion (122). Similar results were found by Natarajan et al. (124). Mohammadzadeh et al. found more extensive dysregulation in BUN and creatinine levels in patients undergoing non-pulsatile CPB (125). In addition, a meta-analysis conducted by Sievert and Sistino of 298 articles found that patients undergoing pulsatile perfusion had significantly higher creatinine clearance postoperatively in the ICU, although there were no significant differences between groups in terms of mean postoperative creatinine levels or BUN (126). Nonetheless, in their conclusion, the authors report that the potential benefit of pulsatile perfusion during CPB with respect to renal preservation should strongly be considered (126).
Overall, AKI is a common postoperative insult following cardiac surgery, and is driven by a variety of forces that harm the renal microvasculature including intraoperative hypotension, renal inflammation and glomerular fibrin deposition, and hemoglobin nephrotoxicity due to red blood cell shearing during cardiopulmonary bypass.
3.2. Vascular dysfunction and CNS injury
3.2.1. Overview
A variety of neurological deficits may occur following cardiac surgery involving cardioplegia/CPB. Early iterations categorized such deficits into two broad groups: Type 1 and Type 2 deficits. Type 1 deficits refer to major focal complications including stroke, coma, and hypoxic encephalopathy, while type 2 deficits refer to generalized deteriorations in intellectual function, including confusion, agitation, disorientation, and memory deficits (127–129). The most recent update of the American College of Cardiology/American Heart Association guidelines for CABG operations in 2011 present three overarching types of postoperative neurological deficits: stroke, coma, and general neurocognitive deficits (130). Like the old type 2 grouping, general neurocognitive deficits include features such as postoperative deteriorations in memory and intellectual function without definitive evidence of focal neurologic injury. When comparing a patient’s baseline and postoperative neurocognitive status, the presence of the symptoms on a neurocognitive assessment battery may indicate neurocognitive decline.
In a multicenter prospective study, Roach et al. found that adverse cerebral outcomes (including focal injury, stupor, coma, memory deficits, seizures, and general deterioration in intellectual function) occurred in 6.1% of patients (127). Patients with adverse neurologic outcomes exhibited increased mortality, longer hospitalization, and higher rate of discharge to long-term care facilities (127). El Bardissi et al. analyzed data from the Society of Thoracic Surgeons adult cardiac surgery database between 2000 and 2009, finding an average incidence of post-CABG stroke of 1.3% (131). Moreover, in a prospective MRI study of 127 CABG patients, Nah et al. found that 27.6% showed new cerebral infarcts postoperatively, although most were clinically silent. Clinically evident (symptomatic) stroke was seen in 3.1% of patients (132).
Turning to neurocognitive decline, different studies report varying incidence rates following cardiac surgery at different time periods. For example, in one study of over 200 CABG patients, about 53% of patients experienced cognitive decline (defined as a drop of one standard deviation in test scores measuring any one of four cognitive domains postoperatively vs. preoperatively) at discharge, 36% at 6 weeks, 24% at 6 months, and 42% at 5 years (133). This same study also found that cognitive function at discharge was a good predictor of long-term cognitive function (133). Other studies report incidence rates of postoperative neurocognitive decline may vary anywhere from 10 to 40% 1 to 3 months after cardiac surgery (134).
3.2.2. Emboli during CPB and cerebral vascular dysfunction
Much like renal injury after cardiac surgery, the pathophysiology of brain injury after cardiac surgery is multifaceted and implicates micro- and macrovascular complications (Figure 4). First, thromboembolic events obstructing the cerebral vasculature play a significant role in post-cardiac surgery stroke and encephalopathy (135). A study of 388 post-CABG stroke patients in New England between 1992 and 2000 showed that embolic strokes accounted for 62.1% of strokes, with the remainder being attributed to other etiologies such as hypoperfusion, hemorrhage, and lacunar infarcts (136). Sources of emboli are many. Regarding macroemoboli, intraoperative ultrasonography of the ascending aorta performed in 500 cardiac surgery patients showed that 68 (13.6%) had significant atheromatous disease of ascending aorta, putting them at high risk for atheroembolism (137). Alternatively, pre or intraoperative atrial fibrillation may also potentiate development of macroemboli due to atrial blood pooling, as suggested by results of another study analyzing data from 3,008 patients who underwent CABG or valve surgery between 2008 and 2012 (138). In this study, the incidence of postoperative stroke in patients with new-onset atrial fibrillation and in patients with preoperative and postoperative atrial fibrillation was significantly higher than in patients with no atrial fibrillation (138).
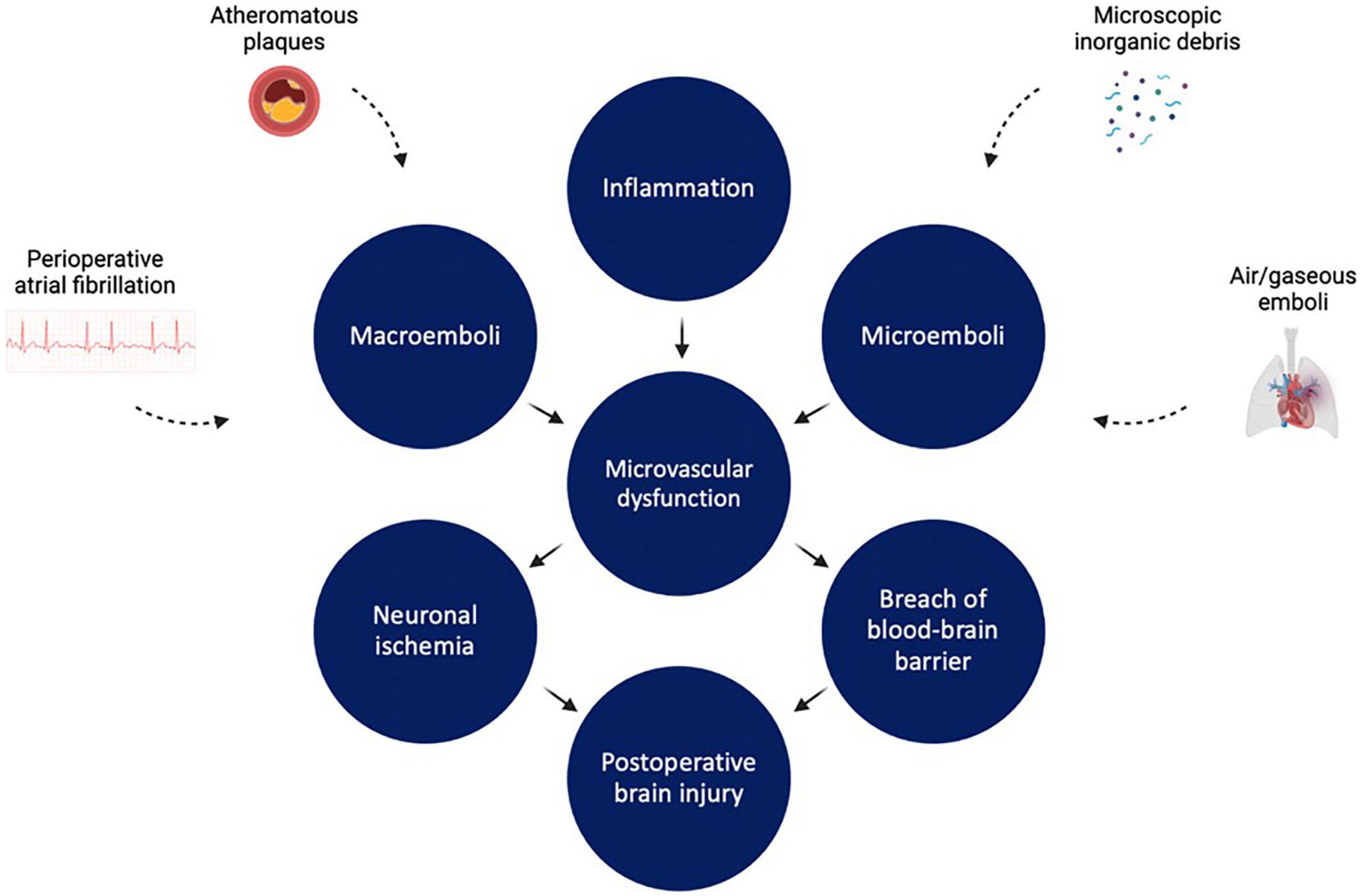
Figure 4. Factors leading to cerebrovascular dysfunction during and after cardiopulmonary bypass. Inflammation, microemboli, and macroemboli all lead to microvascular dysfunction. This in turn leads to neuronal ischemia and disruption of blood brain barrier integrity, resulting in brain injury. Figure created using BioRender.com.
In contrast to macroemboli, microemboli are generally particulate (e.g., microscopic epicardial fat globules, microscopic inorganic debris) or gaseous (e.g., air emboli). These often enter the CPB circuit during venous cannulation or during perfusionist interventions (e.g., when air is injected into the venous side of the CPB circuit), and can cause significant harm to the vasculature. Gas bubbles in particular can cause significant damage to the vascular beds of all organ systems, inducing inflammation, coagulation, platelet activation, and complement activation (139). In the cerebral vasculature, small gas bubbles less than 15 mm in diameter (a common size for gas microemboli in patients undergoing CPB) may disrupt the blood-brain barrier (140). This exposes the brain to a variety of systemic toxins, alters regulation of cerebral blood flow, and exacerbates a pro-inflammatory microenvironment in the central nervous system (141, 142). Of note, increased duration of CPB has been linked with increased incidence of postoperative cerebral microemboli (143).
Gas microemboli have been implicated in global neurocognitive dysfunction following cardiac surgery (144, 145). Transcranial doppler ultrasonography of 100 patients undergoing cardiopulmonary bypass showed that patients with increased brain microembolic burden had more significant neuropsychological deficits after surgery (146). Borger et al. administered a battery of neuropsychologic tests pre- and postoperatively to 83 patients who underwent elective CABG, and found that patients exposed to more than 10 perfusionist interventions, which correlated with increased burden of gaseous microemboli, exhibited lower mean scores on 9 of 10 neuropsychological tests; Results from Rey Auditory Verbal Learning, Digit Span, and Visual Span achieved statistical significance (144). Finally, an analysis of 102 CABG patients showed a strong inverse correlation between the intensity of intraoperative gaseous microembolic signals (monitored by non-invasive, real-time ultrasound) and performance on postoperative neurocognitive tests (147). Curiously, no definitive link has been found linking duration of CPB and postoperative cognitive impairment (148).
Given these findings, the question arises whether intraoperative interventions to reduce microemboli may mitigate cerebral microvascular damage and improve postoperative neuropsychological function. In a porcine model of CPB, intraoperative hypobaric oxygenation to reduce dissolved gases in the blood and CPB circuit resulted in significant reduction in cerebrovascular gaseous microemboli burden; subsequent histopathologic analysis of brain tissue suggested decreased microvascular injury (149). Gerriets et al. studied whether an intra-aortic filter designed to reduce solid microemboli or a dynamic bubble trap designed to reduce gaseous microemboli affect cognitive functioning 3 months after CABG (150). Transcranial doppler sonography was used to detect signs of microemboli during surgery. Final analysis showed that the dynamic bubble trap group exhibited better executive functioning and short-term memory compared with controls, while there was no difference in cognitive test results between the intra-aortic filter group and controls (150). Total microemboli were lower in the dynamic bubble trap group compared to all other groups (150).
3.2.3. Impact of cerebral hypoperfusion and pre-existing microvascular dysfunction
Beyond macro- and microemboli, cerebral hypoperfusion is another potential mechanism of cerebrovascular injury after cardiac surgery. Autoregulation of vascular tone, influenced by soluble mediators, is critical for maintenance of adequate cerebral blood flow. PaCO2 is one such mediator. During cardiac surgery involving cardioplegia and CPB, alpha-stat pH management cools the blood passing through the CPB circuit, resulting in heightened blood CO2 solubility and increased pH (151). For this reason, CO2 is often added to the CPB circuit in order to normalize PaCO2—this in turn facilitates cerebral vasodilation and over time, exhausts autoregulation (135). Cerebral hypoperfusion may have an even greater impact on brain injury during off-pump cardiac surgery, where cerebral venous hypertension may occur secondary to traction on the superior vena cava as the heart is displaced to achieve better access to the coronary arteries (152).
Moreover, in a single photon emission computed tomography (SPECT) imaging study of 82 patients referred for CABG, 75% of scans demonstrated regions of abnormal cerebral perfusion, associated with old age, tobacco use, and diabetes (153). Pre-existing conditions that lead to altered cerebral perfusion are prevalent in patients undergoing cardiac operations, putting these patients at greater risk of cerebrovascular injury in the setting of intraoperative cerebral hypoperfusion during CPB.
For example, as discussed earlier, many patients undergoing cardiac surgery carry a preoperative diagnosis of hypertension, which itself is a well-established cause of extensive micro and macrovascular disease across all vascular beds. Hypertension is associated with pathological cerebrovascular remodeling, resulting in impaired resting cerebral blood flow and higher resting cerebrovascular resistance (154). This also results in impaired cerebral autoregulation, placing the brain at increased vulnerability to ischemia from neurovascular disease. Hypertension may also cause breakdown of the blood-brain barrier through a variety of mechanisms involving inflammation and oxidative stress due to shear forces and endothelial activation (155).
Furthermore, hypertensive small-vessel disease itself is a major cause of deep brain lacunar infarcts, alongside cerebral amyloid angiopathy. Hence unsurprisingly, hypertension is considered critical risk factor for perioperative stroke in patients undergoing cardiac surgery (156). Likewise, hypertensive microvascular disease may be connected to increased likelihood of general neurocognitive impairment after cardiac surgery (134). However, more work will be required to elucidate mechanisms behind any potential connections.
In addition, diabetes has been linked to cerebrovascular disease, largely due to accelerated atherosclerosis, increased edema, and neovascularization in the setting of endothelial damage, oxidative stress, and aberrant inflammatory activation (157) (see previous section for general discussion on diabetic microvascular disease). Similar to hypertension, extensive atherosclerosis in the setting of DM contributes to ischemic cerebrovascular disease and vascular cognitive impairment, increasing risk for cerebral infarction and transient ischemic attacks (157). Indeed, diabetic patients are 2–6 times more susceptible than non-diabetic patients to stroke events (158). Multiple studies have demonstrated that both type 1 and type 2 DM are associated with increased long term risk of stroke following CABG, although much like hypertension, the specific mechanisms underpinning this observation in the setting of cardiac surgery require elucidation (159, 160).
A study of 15 patients at high risk for postoperative stroke who underwent CABG and found that an intraoperative drop in MAP compared to baseline MAP portended a decrease in Mini-Mental Status Examination score postoperatively (161). A separate study examining 98 patients with clinical stroke after cardiac surgery who underwent diffusion-weighted MRI showed that bilateral watershed infarcts were present in 48% of MRIs (162). Further analysis showed that patients with decrease in MAP of at least 10 mmHg intraoperatively were 4.1 times more likely to have experienced bilateral watershed infarcts, as watershed regions in the brain are particularly vulnerable to ischemic stroke in the setting of cerebral hypoperfusion and microvascular dysfunction (162).
3.2.4. Techniques of perfusion and brain injury after CPB
Much like with the renal system, pulsatile perfusion may have a benefit in terms of reduced postoperative brain injury. A systematic review conducted by Ji and Undar found that pulsatile flow significantly improved intraoperative and postoperative blood flow to brain, heart, liver, and pancreas in pediatric and adult patients undergoing cardiac surgery (163). Furthermore, in a pediatric population of patients undergoing CPB to repair congenital heart defects, patients undergoing pulsatile perfusion had lower decreases in regional cerebral oxygen saturation from baseline compared to patients undergoing non-pulsatile perfusion (164). In contrast, non-pulsatile perfusion during CPB has been associated with increased cerebrovascular carbon dioxide reactivity and more profound decreases in cerebral blood flow during periods of hypocapnia (165).
With respect to specific neurocognitive outcomes, one prospective study showed a significantly reduced rate of mild cognitive impairment in patients undergoing pulsatile perfusion during CABG (166). However, a different prospective study of CABG patients by Ozturk et al. found no significant difference in postoperative MMSE scores between pulsatile vs. non-pulsatile perfusion groups (167). Overall, there is no clear consensus regarding whether pulsatile perfusion truly impacts postoperative cognitive decline following CPB, and the matter remains one of extensive debate (168, 169). In addition, there is a surprising lack of research examining whether pulsatile perfusion affects incidence of postoperative ischemic stroke, which is another vital area of investigation.
3.2.5. Role of temperature and brain injury after CPB
Temperature of CPB, chiefly degree of hypothermia, may also have an impact on postoperative CNS injury. A variety of studies have examined the impact of CPB temperature on cerebral oxygenation. One single center trial by Lenkin et al. showed that cerebral tissue oxygen delivery and consumption were significantly higher with normothermic (36.6 degrees Celsius) vs. hypothermic (32 degrees Celsius) CPB (170). However, Kadoi et al. found that regional cerebral oxygenation state decreased with normothermic (> 35 degrees Celsius) vs. hypothermic (30 degrees Celsius) CPB (171). In addition, both normothermic (37 degrees Celsius) and hypothermic (37 degrees Celsius) CPB have been associated with similar degrees of postoperative cerebral edema based on T1-weighted and FLAIR MRI imaging (172).
Unfortunately, the extent to which normothermic vs. hypothermic CPB protects or promotes postoperative brain injury is unclear. A porcine model of deep hypothermic CPB (cooling to 18 degrees Celsius) demonstrated significantly increased cerebral mitochondrial dysfunction compared to pigs undergoing normothermic CPB (173). Likewise, hypothermic circulatory arrest was associated with significantly increased blood-brain-barrier dysfunction compared to normothermic CPB (174). Along this line, some studies, such as that by Grimm et al. found that patients undergoing mild hypothermic (32 degrees Celsius) CPB had significantly worse neurocognitive functioning 1 week after surgery and 4 months after surgery compared to patients undergoing normothermic (37 degrees Celsius) CPB (175). However, by 4 months after surgery, no significant difference could be found (175). Meanwhile, other studies and systematic reviews have reported insufficient evidence to declare any significant differences (176, 177).
3.2.6. Intraoperative NIRS and cerebral injury following CPB
Near-infrared spectroscopy (NIRS) has been investigated as a non-invasive real time tool to monitor oxygenation at the level of the microcirculation in the CNS (178, 179). One single-center prospective study of patients undergoing CPB found that NIRS-directed intraoperative interventions (e.g., increasing MAP, increasing inspired oxygen, correcting bypass cannula) aimed at maintain cerebral saturations at or above baseline resulted in significant improvement in 6 months general neurocognitive function postoperatively, although only length of ICU stay was statistically significant (shorter) in the NIRS group (180). Another single-center prospective trial examining NIRS-directed intraoperative interventions (including fluid challenge, deepening anesthesia, and administration of vasoactive medications) during episodes of cerebral oxygen desaturation (< 60% for > 60 consecutive seconds) during CPB found that 6 months postoperative group mean memory change scores were significantly improved in the intervention group, although overall perioperative outcomes did not differ between intervention and control groups (181). Likewise, a retrospective study evaluating association of intraoperative cerebral oxygen monitoring during cardiopulmonary bypass on postoperative complications found that cerebral oximetry monitoring during cardiothoracic surgery was associated with lower incidence of stroke and overall mortality rate (182).
Curiously, results from systematic reviews examining impact of NIRS-directed intraoperative interventions on neurologic outcomes following cardiopulmonary bypass have been mixed. One review by Serraino and Murphy of 10 randomized trials found no clear evidence to support a significant clinical benefit for cerebral NIRS-based algorithms in cardiac surgery (183). Similarly, a systematic review by Zheng et al. found that while intraoperative reductions in regional cerebral oxygen saturation (rScO2) may be able to identify CPB cannula malposition, only low-level evidence linked low rScO2 with postoperative neurological complications such as stroke or postoperative cognitive decline (184).
Finally, as with the renal system, hemodilution and subsequent anemia during CPB may influence degree of impaired cerebral oxygenation and brain injury. Hemodilution during CPB worsened cerebral infarct size after middle cerebral artery occlusion in rats randomized to CPB with extensive hemodilution (hemoglobin 6 g/dL) vs. controls (hemoglobin 11 g/dL) (185). Moreover, a pig model of CPB investigating the impact of total blood prime resulting in high hematocrit (33%) vs. crystalloid prime resulting in low hematocrit (14%) revealed significantly decreased cerebral oxyhemoglobin and total hemoglobin with low hematocrit by NIRS assessment (186). Addition of hemoglobin-based oxygen carrier attenuated CPB = induced cerebral damage, measured through biomarkers such as neuron-specific enolase, in cerebrospinal fluid and serum of a dog model of CPB (187). Finally, a study evaluating patients undergoing mitral valve replacement under CPB determined a hemoglobin threshold of 9.2–9.4 g/dL for avoiding significant EEG band shifts during CPB (188). Such studies may help influence development of guidelines for maintaining intraoperative hematocrit/hemoglobin levels, for which there are currently no universally accepted standards.
In summary, brain injury following cardiac surgery can manifest in a variety of ways, some hyperacute, such as ischemic/thromboembolic stroke, and others more subtle, such as postoperative neurocognitive decline. Vascular injury due to cerebral hypoperfusion, inflammation, microemboli and macroemboli are all important contributors to postoperative brain injury.
3.3. Vascular dysfunction and the pulmonary system
3.3.1. Overview
A variety of pulmonary complications may occur after cardiac surgery (Figure 5). One observational analysis of 517 patients undergoing cardiac surgery involving CPB reports a postoperative pulmonary complication incidence of 6.2%, including atelectasis respiratory failure, pneumonia, and acute respiratory distress syndrome (ARDS) (189). Retrospective analysis found that major risk factors for postoperative pulmonary complications included advanced age (> 60 years), prolonged bypass time, and preoperative pulmonary hypertension (189). Similarly, a prospective study examining 179 cardiac surgery patients found a cumulative pulmonary complication incidence of 15.08%; more specifically, in 7.82% of CABG patients, 2.23% of valve replacement patients, and in 5.05% of patients with correction of congenital heart disease (190).
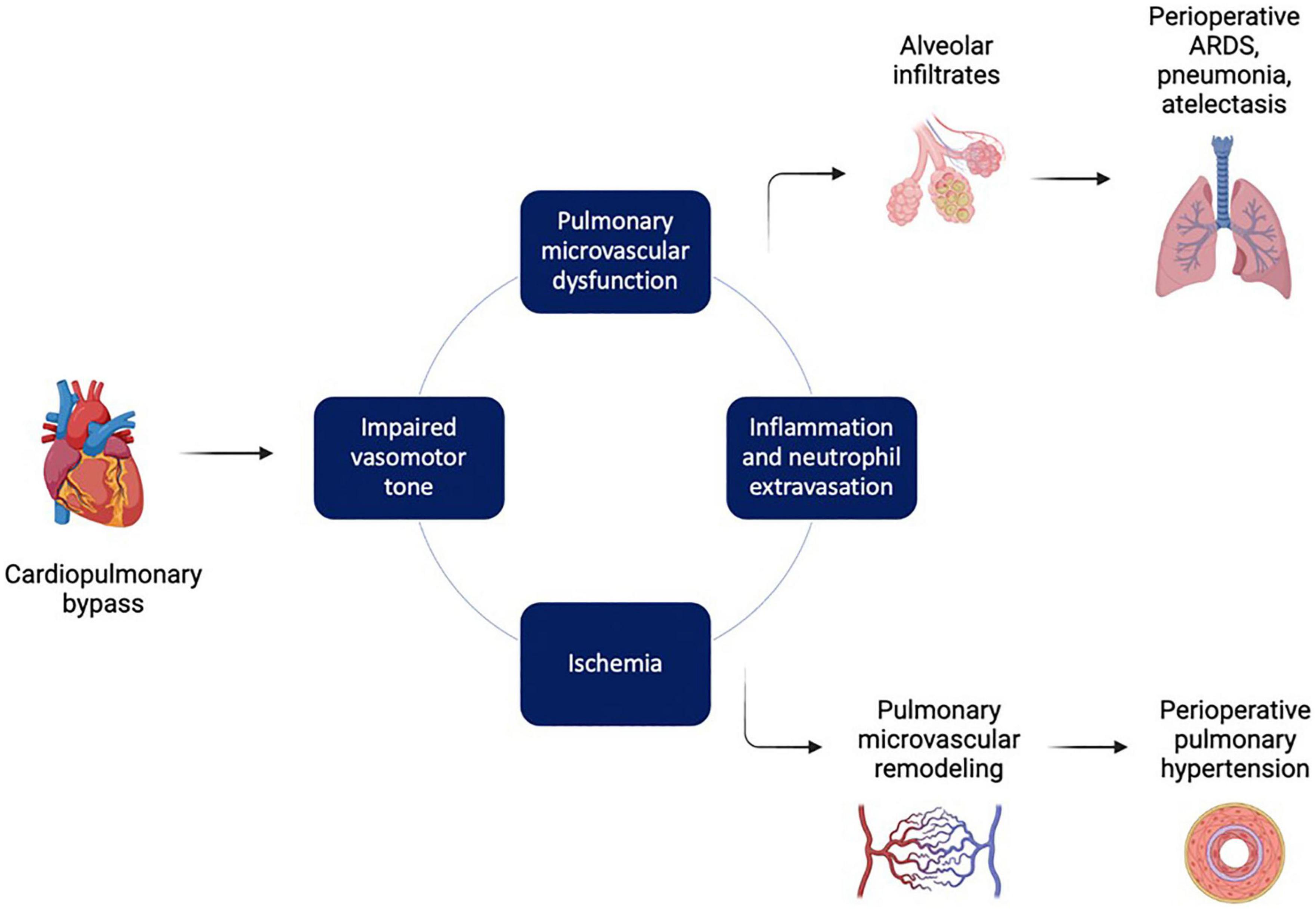
Figure 5. Factors leading to pulmonary injury during and after cardiopulmonary bypass. Cardiopulmonary bypass (CPB) triggers a pathological series of impaired vasomotor tone, ischemia, and inflammation that drives pulmonary microvascular dysfunction. This in turn can lead to disruption of the alveolar-capillary membranes, pulmonary edema, acute respiratory distress syndrome (ARDS), and atelectasis. In addition, pathologic pulmonary microvascular remodeling can lead to postoperative pulmonary hypertension. Figure created using BioRender.com.
3.3.2. Causes of pulmonary microvascular dysfunction following CPB
Pulmonary vascular dysfunction may contribute to lung injury following cardiac surgery. Microvascular inflammation and ischemia-reperfusion are major contributors to postoperative ARDS, a highly fatal postoperative pulmonary complication. A retrospective study of 457 patients undergoing valvular surgery showed that 8.1% of patients developed postoperative ARDS, with a mortality rate of 29.7% (191). Furthermore, more severe stages of ARDS were associated with reduced postoperative survival (191).
Pro-inflammatory cytokines, chemokines, and complement activated by the systemic inflammatory response to cardiac surgery activate endothelial cells in the pulmonary vasculature (192). As discussed earlier, activated endothelial cells upregulate adhesion molecules on their luminal surface, facilitating leukocyte rolling, adhesion, and transmigration across the pulmonary endothelium (192). Increased endothelial barrier permeability after cardiac surgery, in part due to inflammation-induced endothelial injury, further potentiates extravasation of vascular luminal contents (193, 194). This process results in activated neutrophils and macrophages entering the lung parenchyma and secreting ROS and matrix metalloproteinases that cause alveolar damage and further promote influx of proteinaceous fluid into damaged alveoli (195).
As with the renal and cerebral microcirculations, ischemia-reperfusion also contributes to microvascular injury in the pulmonary microcirculation. During CPB, the lungs are solely supplied by the bronchial circulation, with paradoxical pulmonary vasoconstriction leading to decreased bronchial arterial flow and relative pulmonary ischemia (196, 197). A porcine model of CPB showed that bronchial artery ligation prior to initiation of CPB resulted in markedly increased signs of lung injury compared to no bronchial artery ligation (198). Decreased bronchial artery blood flow during and after CPB resulted in increased pulmonary edema and significant intrapulmonary shunting that in turn led to severe arterial hypoxemia (198). Finally, it is also plausible that increased inflammatory activity in pulmonary capillaries restricts area available for adequate gas exchange and further disrupts integrity of the alveolar-capillary barrier, contributing to postoperative pulmonary edema, atelectasis, and ARDS.
Cessation of pulmonary arterial clamping in CPB allows for reperfusion, which then triggers reperfusion injury driven by increased oxidative stress in response to sudden oxygen overload after a period of prolonged ischemia (196). Furthermore, the particularly small diameter of pulmonary capillaries may lead to activated neutrophils and platelets impeding red blood cell transit, obstructing the microcirculation, and resulting in persistent vascular insufficiency even after reperfusion (196). Inflammation and ischemia-reperfusion work in combination to cause microvascular dysfunction, disrupt endothelial and alveolar epithelial barriers, and facilitate postoperative ARDS, along with other pulmonary complications such as atelectasis and pleural effusions.
3.3.3. Pulmonary microvascular dysfunction and postoperative pulmonary hypertension
Microvascular dysfunction in the pulmonary system may also contribute to postoperative pulmonary hypertension. The Sixth World Symposium in Pulmonary Hypertension defined pulmonary hypertension as a mean pulmonary arterial pressure (mPAP) greater than 20 mmHg (199). Currently, there are five defined categories of pulmonary hypertension: pulmonary arterial hypertension (an idiopathic hypertensive vasculopathy of the pulmonary arteries), pulmonary hypertension due to left-sided heart disease, pulmonary hypertension due to lung disease or hypoxia, pulmonary hypertension due to pulmonary artery obstruction, and pulmonary hypertension of unknown etiology (199). Over time, prolonged pulmonary hypertension promotes pulmonary vascular remodeling that leads to further elevations in pulmonary vascular pressures, ultimately resulting in right heart failure and significant morbidity and mortality (200).
Pulmonary hypertension after cardiac surgery may result from several complex mechanisms acting in concert, including preoperative structural cardiac dysfunction, altered vasomotor tone, inflammation, and ischemia-reperfusion injury (201). First, preoperative left ventricular systolic or diastolic dysfunction, along with valvular disease, may predispose patients to increased risk of pulmonary hypertension after cardiac surgery involving cardiopulmonary bypass (201). Next, altered vasomotor tone in the pulmonary microcirculation following CPB in animal models has been well-characterized in several studies. Impaired cholinergic and beta-adrenergic responses, impaired endothelium-dependent vasodilation, increased endothelin-1 responsiveness, and impaired nitric-oxide-induced vasodilation together result in increased pulmonary vasoconstriction, decreased myogenic tone, and increased pulmonary vascular resistance (3, 56, 202–208). Of note, administration of protamine during cardiac surgery can also cause significant pulmonary vasoconstriction through a mechanism involving reduced nitric oxide release in the pulmonary vasculature (209, 210).
Dora et al. studied isolated human pulmonary intralobular arteries through lung biopsies from patients undergoing CABG surgery and found significantly altered pulmonary arterial contractile tone and endothelium-dependent vasodilation postoperatively (211). Furthermore, live cell viability analysis found that while 90% of harvested vascular endothelial cells had intact cell membranes preoperatively, only 58% had intact cell membranes postoperatively (211). Inflammation and ischemia during cardiac surgery may also contribute to pulmonary hypertension through pulmonary vascular remodeling driven by ROS products, such as isoprostanes, and dysregulated growth factor activity (e.g., PDGF-induced pulmonary arterial smooth muscle proliferation) (212–214).
Little to no literature exists specifically investigating the incidence of pulmonary hypertension after cardiac surgery in humans. Results of a swine model showed that CPB resulted in pulmonary hypertension and increased pulmonary vascular resistance (215). Similar results were found in other studies using porcine models (204, 208). Moreover, hypothermic CPB significantly augmented pulmonary vascular resistance and vasoconstriction responses to endothelial agonists in a lamb model (216). Further research examining postoperative pulmonary hypertension in humans are required to better characterize this complication.
Several studies have investigated potential therapeutic targets for postoperative pulmonary hypertension. One study demonstrated that inhaled nitric oxide administered to patients during CPB resulted in lower postoperative mPAPs (217). Administration of inhaled nitric oxide postoperatively after weaning from bypass has also been shown to reduce mPAPs and pulmonary vascular resistance (218, 219). Iloprost leads to decreased mPAPs and pulmonary vascular resistance after weaning from CPB in adults and children (220, 221). Other vasodilatory drugs that have shown promise include inhaled prostacyclin (219, 222), and the phosphodiesterase inhibitors, sildenafil (223–225) and milrinone (226, 227), particularly after mitral valve operations.
Of note, longer duration of CPB has been linked with increased incidence of postoperative lung injury; however, the mechanisms are unclear. One possibility may be extended restriction of bronchial artery blood circulation, which is substantially reduced during CPB and has been associated with pathologic metabolic and ultrastructural changes in lung tissue (228). Alternatively prolonged CPB time may promote a prolonged intraoperative inflammatory response in the pulmonary system and more extensive coagulation cascade activation in the pulmonary microcirculation, restricting nutrient and gas exchange and disrupting the integrity of the alveolar-capillary interface (228, 229).
3.3.4. Impact of pre-existing pulmonary microvascular dysfunction
As in the renal and brain systems, pre-existing comorbidities may influence extent of postoperative microvascular dysfunction following CPB in the pulmonary system. For example, recent studies suggest a link between both type 1 and type 2 DM and pulmonary arterial disease (230). Indeed, patients with type 2 DM appear to have a higher prevalence of pulmonary hypertension, which is independent of hypertension, coronary artery disease, or heart failure (230). Furthermore, streptozotocin-induced type 1 DM rats exhibited extensive pulmonary endothelial dysfunction associated with increased superoxide production and enhanced pulmonary vascular responsiveness to serotonin (230, 231).
Moreover, an analysis of over 200 patients with pulmonary hypertension showed that patients with diabetes trended toward reduced responses to inducible nitric oxide, a finding consistent with animal models of DM that showed reduced pulmonary vascular tone and responsiveness to vasodilators (232). Unfortunately, few studies have investigated the impact of pre-existing DM on postoperative lung injury following cardiac surgery. One study by Lauruschkat et al. found that patients with undiagnosed and insulin-treated DM have higher risk of postoperative pulmonary complications than non-diabetic patients; however, the specific mechanisms behind this finding are unclear (233).
3.3.5. Techniques of perfusion and pulmonary microvascular injury
Finally, several studies have examined the impact of pulsatile vs. non-pulsatile CPB on postoperative lung injury following CPB. A study of patients with chronic obstructive pulmonary disease (COPD) undergoing cardiac surgery showed that pulmonary vascular resistance at the time of aortic cross clamping was significantly lower in the pulsatile vs. non-pulsatile group, along with duration of postoperative mechanical ventilation (234). Next, in a porcine model of CPB, pulsatile perfusion was associated with a reduced pulmonary inflammatory response, evidence by lower levels of NF-kappa-beta, IL-1, IL-6, and TNF-alpha (235). Moreover, a meta-analysis of 8 studies involving 474 patients receiving pulsatile perfusion vs. 496 patients receiving non-pulsatile perfusion during CPB found that patients receiving pulsatile perfusion had significantly lower incidences of postoperative ARDS and intubation time (236).
Santini et al. report that pulsatile pulmonary perfusion during CPB in CABG patients resulted in better preserved alveolar-arterial oxygen gradients, lung compliances, and overall pulmonary hemodynamics (237). The authors also found that postoperative bronchoalveolar lavage specimens from patients undergoing pulsatile perfusion demonstrated lower absolute white counts and lower percentage of neutrophils (237). Donodov et al. found a similar benefit of pulsatile perfusion with respect to pulmonary protection during cardiac surgery; however, Engels et al. found no significant differences in postoperative ventilation time between pulsatile vs. non-pulsatile groups (238, 239).
Altogether, pulmonary microvascular injury during cardiac surgery due to inflammation, altered vasomotor tone, and ischemia-reperfusion can lead to several adverse effects in the lungs including postoperative ARDS, atelectasis, and (if extensive pulmonary microvascular remodeling occurs), postoperative pulmonary hypertension.
3.4. Vascular dysfunction: Impact on peripheral circulations
While most research has focused on examining vascular damage following cardiac surgery in major organ systems such as lungs, brain, and kidneys, a smaller body of studies has considered the impact of cardiac surgery on certain peripheral microcirculations, such as skin and mucous membranes. It is important to consider the peripheral circulation due to its critical role in transporting blood throughout the body, storing blood, promoting exchange between blood and tissue, and regulating cardiac output (240). One such study assessed endothelium-dependent microvascular reactivity in the skin of the foreheads of children and infants undergoing correction for cyanotic and acyanotic congenital heart disease, using single point laser Doppler perfusion monitoring and local thermal hyperemia to examine blood flow (241). Vasodilation induced by local thermal hyperemia was significantly attenuated during CPB relative to preoperative responses, and these responses largely normalized after cessation of bypass (241).
An earlier study using laser Doppler monitoring of cutaneous vessels before, during, and after CABG in adults had shown similar findings of reduced postoperative cutaneous blood flow, which improved by postoperative day 6 (242). Finally, several studies have demonstrated reactive hyperemia in cutaneous microvessels following CPB, such as a study by Gruber et al. examining forearm blood flow, and a study by Pezawas et al. that used skin surface temperature gradients as a proxy for microvascular tone (243, 244).
Hence cardiac surgery can have a significant impact on peripheral microvascular reactivity in the postoperative period.
4. Conclusion
A variety of mechanisms contribute to microvascular dysfunction following cardiac surgery. Cardiac surgery significantly disrupts the balance between vasodilators and vasoconstrictors in the microcirculation through altered responsiveness to endothelium-derived and neuromodulatory vasoactive mediators. This leads to altered vasomotor and myogenic tone, and increased propensity for vasospasm that disrupts normal tissue perfusion. Additionally, CPB induces a systemic pro-inflammatory state characterized by endothelial activation, activation of coagulation and complement pathways, arachidonic acid metabolism, and generalized endothelial injury throughout the microvasculature. Microvascular dysfunction in turn leads to organ damage in several key systems. Hypoperfusion, ischemia-reperfusion, and microvascular inflammation are common themes underpinning postoperative renal, brain, and pulmonary injury. Altogether, understanding the effects of cardiac surgery on microvascular function and organ damage will prove crucial for future innovators working to improve surgical technique and develop new methods of organ protection to reduce postoperative morbidity and mortality.
Author contributions
JF contributed to conceptualization, design, and revision of the manuscript. SK and DB contributed to drafting, design, and revision of the manuscript. SS contributed to design and revision of the manuscript. FS contributed to revision of the manuscript. All authors contributed to the article and approved the submitted version.
Funding
This research project was supported in part by NIH R01-HL46716 and RO1HL128831 to FS; T32 GM065085 to DB; F32HL160063 to SS; 1R01HL127072-01A1, 1R01 HL136347-01, and R01HL136347-04S1 to JF.
Conflict of interest
The authors declare that the research was conducted in the absence of any commercial or financial relationships that could be construed as a potential conflict of interest.
Publisher’s note
All claims expressed in this article are solely those of the authors and do not necessarily represent those of their affiliated organizations, or those of the publisher, the editors and the reviewers. Any product that may be evaluated in this article, or claim that may be made by its manufacturer, is not guaranteed or endorsed by the publisher.
References
1. Bakaeen F, Chu D, Kelly R, Holman W, Jessen M, Ward H. Perioperative outcomes after on- and off-pump coronary artery bypass grafting. Tex Heart Inst J. (2014) 41:144–51. doi: 10.14503/THIJ-13-3372
2. Feng J, Sellke F. Microvascular dysfunction in patients with diabetes after cardioplegic arrest and cardiopulmonary bypass. Curr Opin Cardiol. (2016) 31:618–24. doi: 10.1097/HCO.0000000000000340
3. Ruel M, Khan T, Voisine P, Bianchi C, Sellke F. Vasomotor dysfunction after cardiac surgery. Eur J Cardiothorac Surg. (2004) 26:1002–14. doi: 10.1016/j.ejcts.2004.07.040
4. Feng J, Kant S, Sellke F. Microvascular dysfunction following cardioplegic arrest and cardiopulmonary bypass. Vessel Plus. (2021) 5:30. doi: 10.20517/2574-1209.2021.57
5. Watts S, Morrison S, Davis R, Barman S. Serotonin and blood pressure regulation. Pharmacol Rev. (2012) 64:359–88. doi: 10.1124/pr.111.004697
6. Sabe S, Feng J, Liu Y, Scrimgeour L, Ehsan A, Sellke F. Decreased contractile response of peripheral arterioles to serotonin after CPB in patients with diabetes. Surgery. (2018) 164:288–93. doi: 10.1016/j.surg.2018.03.007
7. Robich M, Araujo E, Feng J, Osipov R, Clements R, Bianchi C, et al. Altered coronary microvascular serotonin receptor expression after coronary artery bypass grafting with cardiopulmonary bypass. J Thorac Cardiovasc Surg. (2010) 139:1033–40. doi: 10.1016/j.jtcvs.2009.05.032
8. Métais C, Li J, Simons M, Sellke F. Serotonin-induced coronary contraction increases after blood cardioplegia-reperfusion: role of COX-2 expression. Circulation. (1999) 100(19 Suppl.):II328–34. doi: 10.1161/01.cir.100.suppl_2.ii-328
9. Métais C, Bianchi C, Li J, Li J, Simons M, Sellke F. Serotonin-induced human coronary microvascular contraction during acute myocardial ischemia is blocked by COX-2 inhibition. Basic Res Cardiol. (2001) 96:59–67. doi: 10.1007/s003950170078
10. Sato K, Li J, Metais C, Bianchi C, Sellke F. Increased pulmonary vascular contraction to serotonin after cardiopulmonary bypass: role of cyclooxygenase. J Surg Res. (2000) 90:138–43. doi: 10.1006/jsre.2000.5869
11. van Brummelen P, Jie K, van Zwieten P. Alpha-adrenergic receptors in human blood vessels. Br J Clin Pharmacol. (1986) 21(Suppl. 1):33S–9S. doi: 10.1111/j.1365-2125.1986.tb02851.x
12. Sellke N, Kuczmarski A, Lawandy I, Cole V, Ehsan A, Singh A, et al. Enhanced coronary arteriolar contraction to vasopressin in patients with diabetes after cardiac surgery. J Thorac Cardiovasc Surg. (2018) 156:2098–107. doi: 10.1016/j.jtcvs.2018.05.090
13. Ziegler O, Anderson K, Liu YH, Ehsan A, Sodha NR, Feng J, et al. Skeletal muscle microvasculature response to beta-adrenergic stimuli diminished with cardiac surgery. Surgery. (2020) 167:493–98. doi: 10.1016/j.surg.2019.07.018
14. Ejaz A, LoGerfo F, Khabbaz K, Pradhan L. Expression of neuropeptide Y, substance P, and their receptors in the right atrium of diabetic patients. Clin Transl Sci. (2011) 4:346–50. doi: 10.1111/j.1752-8062.2011.00318.x
15. Matyal R, Mahmood F, Robich M, Glazer H, Khabbaz K, Hess P, et al. Chronic type II diabetes mellitus leads to changes in neuropeptide Y receptor expression and distribution in human myocardial tissue. Eur J Pharmacol. (2011) 665:19–28. doi: 10.1016/j.ejphar.2011.04.039
16. McDermott B, Bell D. NPY and cardiac diseases. Curr Top Med Chem. (2007) 7:1692–703. doi: 10.2174/156802607782340939
17. Tan C, Green P, Tapoulal N, Lewandowski A, Leeson P, Herring N. The role of neuropeptide Y in cardiovascular health and disease. Front Physiol. (2018) 9:1281. doi: 10.3389/fphys.2018.01281
18. Meng F, Han J, Wang J, Zhang H, Xu C, Meng X. The gender-specific expression of neuropeptide Y and neuropeptide Y receptors in human atrial tissue during cardiopulmonary bypass surgery. J Thorac Dis. (2018) 10:6563–8. doi: 10.21037/jtd.2018.11.126
19. Mirman B, Ikeda I, Zhang Z, Liu Y, Yu L, Ehsan A, et al. Effects of neuropeptide Y on the microvasculature of human skeletal muscle. Surgery. (2020) 168:155–9. doi: 10.1016/j.surg.2020.04.020
20. Masetti P, Murphy S, Kouchoukos N. Vasopressin therapy for vasoplegic syndrome following cardiopulmonary bypass. J Card Surg. (2002) 17:485–9. doi: 10.1046/j.1540-8191.2002.01002.x
21. Holmes C, Landry D, Granton J. Science review: vasopressin and the cardiovascular system part 1–receptor physiology. Crit Care. (2003) 7:427–34. doi: 10.1186/cc2337
22. Nakahata N. Thromboxane A2: physiology/pathophysiology, cellular signal transduction and pharmacology. Pharmacol Ther. (2008) 118:18–35. doi: 10.1016/j.pharmthera.2008.01.001
23. Feng J, Liu Y, Singh A, Dobrilovic N, Feng W, Chu L, et al. Impaired contractile response of human peripheral arterioles to thromboxane A-2 after cardiopulmonary bypass. Surgery. (2011) 150:263–71. doi: 10.1016/j.surg.2011.06.004
24. Kowalczyk A, Kleniewska P, Kolodziejczyk M, Skibska B, Goraca A. The role of endothelin-1 and endothelin receptor antagonists in inflammatory response and sepsis. Arch Immunol Ther Exp. (2015) 63:41–52. doi: 10.1007/s00005-014-0310-1
25. Feng J, Liu Y, Chu L, Clements R, Khabbaz K, Robich M, et al. Thromboxane-induced contractile response of human coronary arterioles is diminished after cardioplegic arrest. Ann Thorac Surg. (2011) 92:829–36. doi: 10.1016/j.athoracsur.2011.04.049
26. Feng J, Chu L, Robich M, Clements R, Khabbaz K, Hagberg R, et al. Effects of cardiopulmonary bypass on endothelin-1-induced contraction and signaling in human skeletal muscle microcirculation. Circulation. (2010) 122(11 Suppl.):S150–5. doi: 10.1161/CIRCULATIONAHA.109.928226
27. Chen K, Pittman R, Popel A. Nitric oxide in the vasculature: where does it come from and where does it go? A quantitative perspective. Antioxid Redox Signal. (2008) 10:1185–98. doi: 10.1089/ars.2007.1959
28. Toprak V, Sirin B, Tok D, Ozbilgin K, Saribülbül O. The effect of cardiopulmonary bypass on the expression of inducible nitric oxide synthase, endothelial nitric oxide synthase, and vascular endothelial growth factor in the internal mammary artery. J Cardiothorac Vasc Anesth. (2006) 20:63–7. doi: 10.1053/j.jvca.2005.02.002
29. Hayashi Y, Sawa Y, Fukuyama N, Nakazawa H, Matsuda H. Inducible nitric oxide production is an adaptation to cardiopulmonary bypass-induced inflammatory response. Ann Thorac Surg. (2001) 72:149–55. doi: 10.1016/s0003-497502637-6
30. Andrási T, Soós P, Bakos G, Stumpf N, Blazovics A, Hagl S, et al. L-arginine protects the mesenteric vascular circulation against cardiopulmonary bypass-induced vascular dysfunction. Surgery. (2003) 134:72–9. doi: 10.1067/msy.2003.208
31. He G, Yang C. Hyperkalemia alters endothelium-dependent relaxation through non-nitric oxide and noncyclooxygenase pathway: a mechanism for coronary dysfunction due to cardioplegia. Ann Thorac Surg. (1996) 61:1394–9. doi: 10.1016/0003-497500086-0
32. Mayers I, Salas E, Hurst T, Johnson D, Radomski M. Increased nitric oxide synthase activity after canine cardiopulmonary bypass is suppressed by s-nitrosoglutathione. J Thorac Cardiovasc Surg. (1999) 117:1009–16. doi: 10.1016/S0022-522370383-1
33. Kukreja R, Hess M. The oxygen free radical system: from equations through membrane-protein interactions to cardiovascular injury and protection. Cardiovasc Res. (1992) 26:641–55. doi: 10.1093/cvr/26.7.641
34. Liu Y, Kabakov A, Xie A, Shi G, Singh A, Sodha N, et al. Metabolic regulation of endothelial SK channels and human coronary microvascular function. Int J Cardiol. (2020) 312:1–9. doi: 10.1016/j.ijcard.2020.03.028
35. Liu Y, Xie A, Singh A, Ehsan A, Choudhary G, Dudley S, et al. Inactivation of endothelial small/intermediate conductance of calcium-activated potassium channels contributes to coronary arteriolar dysfunction in diabetic patients. J Am Heart Assoc. (2015) 4:e002062. doi: 10.1161/JAHA.115.002062
36. Si H, Heyken W, Wölfle S, Tysiac M, Schubert R, Grgic I, et al. Impaired endothelium-derived hyperpolarizing factor-mediated dilations and increased blood pressure in mice deficient of the intermediate-conductance Ca2+-activated K+ channel. Circ Res. (2006) 99:537–44. doi: 10.1161/01.RES.0000238377.08219.0c
37. Liu Y, Sellke E, Feng J, Clements R, Sodha N, Khabbaz K, et al. Calcium-activated potassium channels contribute to human skeletal muscle microvascular endothelial dysfunction related to cardiopulmonary bypass. Surgery. (2008) 144:239–44. doi: 10.1016/j.surg.2008.03.032
38. Feng J, Liu Y, Clements R, Sodha N, Khabbaz K, Senthilnathan V, et al. Calcium-activated potassium channels contribute to human coronary microvascular dysfunction after cardioplegic arrest. Circulation. (2008) 118(14 Suppl.):S46–51. doi: 10.1161/CIRCULATIONAHA.107.755827
39. Liu Y, Cole V, Lawandy I, Ehsan A, Sellke F, Feng J. Decreased coronary arteriolar response to KCa channel opener after cardioplegic arrest in diabetic patients. Mol Cell Biochem. (2018) 445:187–94. doi: 10.1007/s11010-017-3264-x
40. Laffey J, Boylan J, Cheng D. The systemic inflammatory response to cardiac surgery: implications for the anesthesiologist. Anesthesiology. (2002) 97:215–52. doi: 10.1097/00000542-200207000-00030
41. Chenoweth D, Cooper S, Hugli T, Stewart R, Blackstone E, Kirklin J. Complement activation during cardiopulmonary bypass: evidence for generation of C3a and C5a anaphylatoxins. N Engl J Med. (1981) 304:497–503. doi: 10.1056/NEJM198102263040901
42. Koster A, Fischer T, Praus M, Haberzettl H, Kuebler W, Hetzer R, et al. Hemostatic activation and inflammatory response during cardiopulmonary bypass: impact of heparin management. Anesthesiology. (2002) 97:837–41. doi: 10.1097/00000542-200210000-00014
43. Höfer J, Fries D, Solomon C, Velik-Salchner C, Ausserer J. A snapshot of coagulopathy after cardiopulmonary bypass. Clin Appl Thromb Hemost. (2016) 22:505–11. doi: 10.1177/1076029616651146
44. Pinckard R, Olson M, Giclas P, Terry R, Boyer J, O’Rourke R. Consumption of classical complement components by heart subcellular membranes in vitro and in patients after acute myocardial infarction. J Clin Invest. (1975) 56:740–50. doi: 10.1172/JCI108145
45. Rossaint J, Berger C, Van Aken H, Scheld H, Zahn P, Rukosujew A, et al. Cardiopulmonary bypass during cardiac surgery modulates systemic inflammation by affecting different steps of the leukocyte recruitment cascade. PLoS One. (2012) 7:e45738. doi: 10.1371/journal.pone.0045738
46. Kawata H, Sawatari K, Mayer J. Evidence for the role of neutrophils in reperfusion injury after cold cardioplegic ischemia in neonatal lambs. J Thorac Cardiovasc Surg. (1992) 103:908–17; discussion 917–8.
47. Tofukuji M, Stahl G, Metais C, Tomita M, Agah A, Bianchi C, et al. Mesenteric dysfunction after cardiopulmonary bypass: role of complement C5a. Ann Thorac Surg. (2000) 69:799–807. doi: 10.1016/s0003-497501408-3
48. Morariu A, Gu Y, Huet R, Siemons W, Rakhorst G, Oeveren W. Red blood cell aggregation during cardiopulmonary bypass: a pathogenic cofactor in endothelial cell activation? Eur J Cardiothorac Surg. (2004) 26:939–46. doi: 10.1016/j.ejcts.2004.06.010
49. Park K, Tofukuji M, Metais C, Comunale M, Dai H, Simons M, et al. Attenuation of endothelium-dependent dilation of pig pulmonary arterioles after cardiopulmonary bypass is prevented by monoclonal antibody to complement C5a. Anesth Analg. (1999) 89:42–8. doi: 10.1097/00000539-199907000-00008
50. Crofford L. COX-1 and COX-2 tissue expression: implications and predictions. J Rheumatol Suppl. (1997) 49:15–9.
51. Caughey G, Cleland L, Penglis P, Gamble J, James M. Roles of cyclooxygenase (COX)-1 and COX-2 in prostanoid production by human endothelial cells: selective up-regulation of prostacyclin synthesis by COX-2. J Immunol. (2001) 167:2831–8. doi: 10.4049/jimmunol.167.5.2831
52. Matsumoto H, Naraba H, Murakami M, Kudo I, Yamaki K, Ueno A, et al. Concordant induction of prostaglandin E2 synthase with cyclooxygenase-2 leads to preferred production of prostaglandin E2 over thromboxane and prostaglandin D2 in lipopolysaccharide-stimulated rat peritoneal macrophages. Biochem Biophys Res Commun. (1997) 230:110–4. doi: 10.1006/bbrc.1996.5894
53. Ricciotti E, Yu Y, Grosser T, Fitzgerald G. COX-2, the dominant source of prostacyclin. Proc Natl Acad Sci U.S.A. (2013) 110:E183. doi: 10.1073/pnas.1219073110
54. Sodha N, Clements R, Sellke F. Vascular changes after cardiac surgery: role of NOS, COX, kinases, and growth factors. Front Biosci. (2009) 14:689–98. doi: 10.2741/3273
55. Feng J, Anderson K, Singh A, Ehsan A, Mitchell H, Liu Y, et al. Diabetes upregulation of cyclooxygenase 2 contributes to altered coronary reactivity after cardiac surgery. Ann Thorac Surg. (2017) 104:568–76. doi: 10.1016/j.athoracsur.2016.11.025
56. Sellke F. Vascular changes after cardiopulmonary bypass and ischemic cardiac arrest: roles of nitric oxide synthase and cyclooxygenase. Braz J Med Biol Res. (1999) 32:1345–52. doi: 10.1590/s0100-879x1999001100004
57. Gupta R, Patel A, Shah N, Chaudhary A, Jha U, Yadav U, et al. Oxidative stress and antioxidants in disease and cancer: a review. Asian Pac J Cancer Prev. (2014) 15:4405–9. doi: 10.7314/apjcp.2014.15.11.4405
58. Kawahito K, Kobayashi E, Ohmori M, Harada K, Kitoh Y, Fujimura A, et al. Enhanced responsiveness of circulatory neutrophils after cardiopulmonary bypass: increased aggregability and superoxide producing capacity. Artif Organs. (2000) 24:37–42. doi: 10.1046/j.1525-1594.2000.06381.x
59. Winterbourn C, Kettle A, Hampton M. Reactive oxygen species and neutrophil function. Annu Rev Biochem. (2016) 85:765–92. doi: 10.1146/annurev-biochem-060815-014442
60. Haga Y, Hatori N, Yoshizu H, Okuda E, Uriuda Y, Tanaka S. Granulocyte superoxide anion and elastase release during cardiopulmonary bypass. Artif Organs. (1993) 17:837–42. doi: 10.1111/j.1525-1594.1993.tb00391.x
61. Zakkar M, Guida G, Suleiman M, Angelini G. Cardiopulmonary bypass and oxidative stress. Oxid Med Cell Longev. (2015) 2015:189863. doi: 10.1155/2015/189863
62. Suleiman M, Zacharowski K, Angelini G. Inflammatory response and cardioprotection during open-heart surgery: the importance of anaesthetics. Br J Pharmacol. (2008) 153:21–33. doi: 10.1038/sj.bjp.0707526
63. Ikhlas M, Atherton N. Vascular Reperfusion Injury. (2022). Available online at: https://www.ncbi.nlm.nih.gov/books/NBK562210/ (accessed Aug 30, 2021).
64. Inauen W, Suzuki M, Granger D. Mechanisms of cellular injury: potential sources of oxygen free radicals in ischemia/reperfusion. Microcirc Endothelium Lymphatics. (1989) 5:143–55.
65. Song Y, Xing H, He Y, Zhang Z, Shi G, Wu S, et al. Inhibition of mitochondrial reactive oxygen species improves coronary endothelial function after cardioplegic hypoxia/reoxygenation. J Thorac Cardiovasc Surg. (2022) 164:e207–26. doi: 10.1016/j.jtcvs.2021.06.029
66. Makris K, Spanou L. Acute kidney injury: definition, pathophysiology and clinical phenotypes. Clin Biochem Rev. (2016) 37:85–98.
67. Manzoor H, Bhatt H. Prerenal Kidney Failure. (2022). Available online at: https://www.ncbi.nlm.nih.gov/books/NBK560678/ (accessed Aug 4, 2021).
68. Bellomo R, Ronco C, Kellum J, Mehta R, Palevsky P. Acute renal failure – Definition, outcome measures, animal models, fluid therapy and information technology needs: the Second International Consensus Conference of the Acute Dialysis Quality Initiative (ADQI) group. Crit Care. (2004) 8:R204–12. doi: 10.1186/cc2872
69. Ostermann M, Joannidis M. Acute kidney injury 2016: diagnosis and diagnostic workup. Crit Care. (2016) 20:299. doi: 10.1186/s13054-016-1478-z
70. Singh P, Ricksten S, Bragadottir G, Redfors B, Nordquist L. Renal oxygenation and haemodynamics in acute kidney injury and chronic kidney disease. Clin Exp Pharmacol Physiol. (2013) 40:138–47. doi: 10.1111/1440-1681.12036
71. Laycock S, Vogel T, Forfia P, Tuzman J, Xu X, Ochoa M, et al. Role of nitric oxide in the control of renal oxygen consumption and the regulation of chemical work in the kidney. Circ Res. (1998) 82:1263–71. doi: 10.1161/01.res.82.12.1263
72. Deng A, Tang T, Singh P, Wang C, Satriano J, Thomson S, et al. Regulation of oxygen utilization by angiotensin II in chronic kidney disease. Kidney Int. (2009) 75:197–204. doi: 10.1038/ki.2008.481
73. Gunaratnam L, Bonventre J. HIF in kidney disease and development. J Am Soc Nephrol. (2009) 20:1877–87. doi: 10.1681/ASN.2008070804
74. Bove T, Calabrò M, Landoni G, Aletti G, Marino G, Crescenzi G, et al. The incidence and risk of acute renal failure after cardiac surgery. J Cardiothorac Vasc Anesth. (2004) 18:442–5. doi: 10.1053/j.jvca.2004.05.021
75. Vandenberghe W, Gevaert S, Kellum J, Bagshaw S, Peperstraete H, Herck I, et al. Acute kidney injury in cardiorenal syndrome type 1 patients: a systematic review and meta-analysis. Cardiorenal Med. (2016) 6:116–28. doi: 10.1159/000442300
76. Machado M, Nakazone M, Maia L. Prognostic value of acute kidney injury after cardiac surgery according to kidney disease: improving global outcomes definition and staging (KDIGO) criteria. PLoS One. (2014) 9:e98028. doi: 10.1371/journal.pone.0098028
77. Kumar A, Suneja M, Bayman E, Weide G, Tarasi M. Association between postoperative acute kidney injury and duration of cardiopulmonary bypass: a meta-analysis. J Cardiothorac Vasc Anesth. (2012) 26:64–9. doi: 10.1053/j.jvca.2011.07.007
78. Lee T, Lee C, Chen J, Fan P, Tu Y, Yen C, et al. Assessment of cardiopulmonary bypass duration improves novel biomarker detection for predicting postoperative acute kidney injury after cardiovascular surgery. J Clin Med. (2021) 10:2741. doi: 10.3390/jcm10132741
79. Axtell A, Fiedler A, Melnitchouk S, D’Alessandro D, Villavicencio M, Jassar A, et al. Correlation of cardiopulmonary bypass duration with acute renal failure after cardiac surgery. J Thorac Cardiovasc Surg. (2019) 19:30286–87. doi: 10.1016/j.jtcvs.2019.01.072
80. Mohamed Ali MA-E, Khalek Akl DA-E, Salama MS. Relation between prolonged cardiopulmonary bypass time in cardiac surgery in adult patients and post-operative acute kidney injury. QJM Int J Med. (2021) 114:hcab086.070. doi: 10.1093/qjmed/hcab086.070
81. Hudson C, Hudson J, Swaminathan M, Shaw A, Stafford-Smith M, Patel U. Emerging concepts in acute kidney injury following cardiac surgery. Semin Cardiothorac Vasc Anesth. (2008) 12:320–30. doi: 10.1177/1089253208328582
82. Han G, Zhang J, Lu S, Li Y. Etiological and prognostic factors in patients with acute renal failure after cardiac surgery. Chin J Blood Purific. (2005) 4:302–5.
83. Cengic S, Zuberi M, Bansal V, Ratzlaff R, Rodrigues E, Festic E. Hypotension after intensive care unit drop-off in adult cardiac surgery patients. World J Crit Care Med. (2020) 9:20–30. doi: 10.5492/wjccm.v9.i2.20
84. Busse L, Barker N, Petersen C. Vasoplegic syndrome following cardiothoracic surgery-review of pathophysiology and update of treatment options. Crit Care. (2020) 24:36. doi: 10.1186/s13054-020-2743-8
85. Tremblay J, Laramée P, Lamarche Y, Denault A, Beaubien-Souligny W, Frenette A, et al. Potential risks in using midodrine for persistent hypotension after cardiac surgery: a comparative cohort study. Ann Intensive Care. (2020) 10:121. doi: 10.1186/s13613-020-00737-w
86. de la Hoz M, Rangasamy V, Bastos A, Xu X, Novack V, Saugel B, et al. Intraoperative hypotension and acute kidney injury, stroke, and mortality during and outside cardiopulmonary bypass: a retrospective observational cohort study. Anesthesiology. (2022) 136:927–39. doi: 10.1097/ALN.0000000000004175
87. Sun L, Chung A, Farkouh M, van Diepen S, Weinberger J, Bourke M, et al. Defining an intraoperative hypotension threshold in association with stroke in cardiac surgery. Anesthesiology. (2018) 129:440–7. doi: 10.1097/ALN.0000000000002298
88. Kandler K, Nilsson J, Oturai P, Jensen M, Møller C, Clemmesen J, et al. Higher arterial pressure during cardiopulmonary bypass may not reduce the risk of acute kidney injury. J Cardiothorac Surg. (2019) 14:107. doi: 10.1186/s13019-019-0929-4
89. Kotani Y, Yoshida T, Kumasawa J, Kamei J, Taguchi A, Kido K, et al. The impact of relative hypotension on acute kidney injury progression after cardiac surgery: a multicenter retrospective cohort study. Ann Intensive Care. (2021) 11:178. doi: 10.1186/s13613-021-00969-4
90. Zhang W, Garg A, Coca S, Devereaux P, Eikelboom J, Kavsak P, et al. Plasma IL-6 and IL-10 concentrations predict AKI and long-term mortality in adults after cardiac surgery. J Am Soc Nephrol. (2015) 26:3123–32. doi: 10.1681/ASN.2014080764
91. Moledina D, Mansour S, Jia Y, Obeid W, Thiessen-Philbrook H, Koyner J, et al. Association of T cell-derived inflammatory cytokines with acute kidney injury and mortality after cardiac surgery. Kidney Int Rep. (2019) 4:1689–97. doi: 10.1016/j.ekir.2019.09.003
92. O’Neal J, Shaw A, Billings F. Acute kidney injury following cardiac surgery: current understanding and future directions. Crit Care. (2016) 20:187. doi: 10.1186/s13054-016-1352-z
93. Stoner J, Clanton T, Aune S, Angelos M. O2 delivery and redox state are determinants of compartment-specific reactive O2 species in myocardial reperfusion. Am J Physiol Heart Circ Physiol. (2007) 292:H109–16. doi: 10.1152/ajpheart.00925.2006
94. Granger D, Kvietys P. Reperfusion injury and reactive oxygen species: the evolution of a concept. Redox Biol. (2015) 6:524–51. doi: 10.1016/j.redox.2015.08.020
95. Liu T, Zhang L, Joo D, Sun S. NF-κB signaling in inflammation. Signal Transduct Target Ther. (2017) 2:17023. doi: 10.1038/sigtrans.2017.23
96. Edelstein C, Schrier R. Pathophysiology of ischemic acute renal injury. 8th ed. In: R Schrier editor. Diseases of the Kidney and Urinary Tract. Philadelphia, PA: Lippincott Williams and Wilkins (2007). p. 930–61.
97. Billings F, Yu C, Byrne J, Petracek M, Pretorius M. Heme oxygenase-1 and acute kidney injury following cardiac surgery. Cardiorenal Med. (2014) 4:12–21. doi: 10.1159/000357871
98. Moat N, Evans T, Quinlan G, Gutteridge J. Chelatable iron and copper can be released from extracorporeally circulated blood during cardiopulmonary bypass. FEBS Lett. (1993) 328:103–6. doi: 10.1016/0014-579380974-y
99. Deuel J, Schaer C, Boretti F, Opitz L, Garcia-Rubio I, Baek J, et al. Hemoglobinuria-related acute kidney injury is driven by intrarenal oxidative reactions triggering a heme toxicity response. Cell Death Dis. (2016) 7:e2064. doi: 10.1038/cddis.2015.392
100. Haase M, Haase-Fielitz A, Bagshaw S, Ronco C, Bellomo R. Cardiopulmonary bypass-associated acute kidney injury: a pigment nephropathy? Contrib Nephrol. (2007) 156:340–53. doi: 10.1159/000102125
101. Billings F, Ball S, Roberts L, Pretorius M. Postoperative acute kidney injury is associated with hemoglobinemia and an enhanced oxidative stress response. Free Radic Biol Med. (2011) 50:1480–7. doi: 10.1016/j.freeradbiomed.2011.02.011
102. Menasché P, Antebi H, Alcindor L, Teiger E, Perez G, Giudicelli Y, et al. Iron chelation by deferoxamine inhibits lipid peroxidation during cardiopulmonary bypass in humans. Circulation. (1990) 82(5 Suppl.):IV390–6.
103. Sharma S, Leaf D. Iron chelation as a potential therapeutic strategy for AKI prevention. J Am Soc Nephrol. (2019) 30:2060–71. doi: 10.1681/ASN.2019060595
104. Cao L, Ru W, Hu C, Shen Y. Interaction of hemoglobin, transfusion, and acute kidney injury in patients undergoing cardiopulmonary bypass: a group-based trajectory analysis. Ren Fail. (2022) 44:1368–75. doi: 10.1080/0886022X.2022.2108840
105. De Santo L, Romano G, Della Corte A, de Simone V, Grimaldi F, Cotrufo M, et al. Preoperative anemia in patients undergoing coronary artery bypass grafting predicts acute kidney injury. J Thorac Cardiovasc Surg. (2009) 138:965–70. doi: 10.1016/j.jtcvs.2009.05.013
106. Soh S, Shim J, Song J, Kang B, Kwak Y. Perioperative nadir hemoglobin concentration and outcome in off-pump coronary artery bypass surgery – A retrospective review. Circ J. (2020) 85:37–43. doi: 10.1253/circj.CJ-20-0694
107. Holt R, Barnard-Kelly K, Dritsakis G, Thorne K, Cohen L, Dixon E, et al. Developing an intervention to optimise the outcome of cardiac surgery in people with diabetes: the OCTOPuS pilot study. Pilot Feasibility Stud. (2021) 7:157. doi: 10.1186/s40814-021-00887-z
108. Chawla A, Chawla R, Jaggi S. Microvasular and macrovascular complications in diabetes mellitus: distinct or continuum? Indian J Endocrinol Metab. (2016) 20:546–51. doi: 10.4103/2230-8210.183480
109. Vithian K, Hurel S. Microvascular complications: pathophysiology and management. Clin Med. (2010) 10:505–9. doi: 10.7861/clinmedicine.10-5-505
110. Toth-Manikowski S, Atta M. Diabetic kidney disease: pathophysiology and therapeutic targets. J Diabetes Res. (2015) 2015:697010. doi: 10.1155/2015/697010
111. Faritous Z, Ardeshiri M, Yazdanian F, Jalali A, Totonchi Z, Azarfarin R. Hyperglycemia or high hemoglobin A1C: which one is more associated with morbidity and mortality after coronary artery bypass graft surgery? Ann Thorac Cardiovasc Surg. (2014) 20:223–8. doi: 10.5761/atcs.oa.13.02282
112. Gumus F, Polat A, Sinikoglu S, Yektas A, Erkalp K, Alagol A. Use of a lower cut-off value for HbA1c to predict postoperative renal complication risk in patients undergoing coronary artery bypass grafting. J Cardiothorac Vasc Anesth. (2013) 27:1167–73. doi: 10.1053/j.jvca.2013.02.030
113. Wang R, Zhang H, Zhu Y, Chen W, Chen X. The impact of diabetes mellitus on acute kidney injury after coronary artery bypass grafting. J Cardiothorac Surg. (2020) 15:289. doi: 10.1186/s13019-020-01312-x
114. Aronow W. Management of hypertension in patients undergoing surgery. Ann Transl Med. (2017) 5:227. doi: 10.21037/atm.2017.03.54
115. Serné E, de Jongh R, Eringa E, IJzerman R, Stehouwer C. Microvascular dysfunction: a potential pathophysiological role in the metabolic syndrome. Hypertension. (2007) 50:204–11. doi: 10.1161/HYPERTENSIONAHA.107.089680
116. Guzik TJ, Touyz RM. Vascular pathophysiology of hypertension. Oxford Med. (2017) 1:291–308. doi: 10.1093/med/9780198755777.003.0019
117. Bidani A, Griffin K. Pathophysiology of hypertensive renal damage: implications for therapy. Hypertension. (2004) 44:595–601. doi: 10.1161/01.HYP.0000145180.38707.84
118. Zappitelli M, Parikh C, Kaufman J, Go A, Kimmel P, Hsu C, et al. Acute kidney injury and risk of CKD and hypertension after pediatric cardiac surgery. Clin J Am Soc Nephrol. (2020) 15:1403–12. doi: 10.2215/CJN.00150120
119. Aronson S, Fontes M, Miao Y, Mangano D. Risk index for perioperative renal dysfunction/failure: critical dependence on pulse pressure hypertension. Circulation. (2007) 115:733–42. doi: 10.1161/CIRCULATIONAHA.106.623538
120. Weir M, Aronson S, Avery E, Pollack C. Acute kidney injury following cardiac surgery: role of perioperative blood pressure control. Am J Nephrol. (2011) 33:438–52. doi: 10.1159/000327601
121. Tan Z, Besser M, Anderson S, Newey C, Iles R, Dunning J, et al. Pulsatile versus nonpulsatile flow during cardiopulmonary bypass: extent of hemolysis and clinical significance. ASAIO J. (2020) 66:1025–30. doi: 10.1097/MAT.0000000000001154
122. Milano A, Dodonov M, Van Oeveren W, Onorati F, Gu Y, Tessari M, et al. Pulsatile cardiopulmonary bypass and renal function in elderly patients undergoing aortic valve surgery†. Eur J Cardiothorac Surg. (2015) 47:291–8; discussion 298. doi: 10.1093/ejcts/ezu136
123. Kim H, Son H, Fang Y, Park S, Hwang C, Sun K. The effects of pulsatile flow upon renal tissue perfusion during cardiopulmonary bypass: a comparative study of pulsatile and nonpulsatile flow. ASAIO J. (2005) 51:30–6. doi: 10.1097/01.mat.0000150324.02040.b4
124. Natarajan K, Jeeva G, Sophia I, Ramachandran V, Vishwanathan B, Ninan B. Is pulsatile perfusion has significant effects on renal function in patients undergoing cardiac surgery? An observational study. J Cardiothorac Vasc Anesth. (2016) 30:20–21. doi: 10.1053/j.jvca.2016.03.064
125. Mohammadzadeh A, Jafari N, Hasanpour M, Sahandifar S, Ghafari M, Alaei V. Effects of pulsatile perfusion during cardiopulmonary bypass on biochemical markers and kidney function in patients undergoing cardiac surgeries. Am J Cardiovasc Dis. (2013) 3:158–62.
126. Sievert A, Sistino J. A meta-analysis of renal benefits to pulsatile perfusion in cardiac surgery. J Extra Corpor Technol. (2012) 44:10–4.
127. Roach G, Kanchuger M, Mangano C, Newman M, Nussmeier N, Wolman R, et al. Adverse cerebral outcomes after coronary bypass surgery. Multicenter Study of Perioperative Ischemia Research Group and the Ischemia Research and Education Foundation Investigators. N Engl J Med. (1996) 335:1857–63. doi: 10.1056/NEJM199612193352501
128. Eagle K, Guyton R, Davidoff R, Ewy G, Fonger J, Gardner T, et al. ACC/AHA guidelines for coronary artery bypass graft surgery: executive summary and recommendations : a report of the American College of Cardiology/American Heart Association Task Force on Practice Guidelines (Committee to revise the 1991 guidelines for coronary artery bypass graft surgery). Circulation. (1999) 100:1464–80. doi: 10.1161/01.cir.100.13.1464
129. Savageau J, Stanton B, Jenkins C, Frater R. Neuropsychological dysfunction following elective cardiac operation. II. A six-month reassessment. J Thorac Cardiovasc Surg. (1982) 84:595–600.
130. Hillis L, Smith P, Anderson J, Bittl J, Bridges C, Byrne J, et al. 2011 ACCF/AHA guideline for coronary artery bypass graft surgery. A report of the American College of Cardiology Foundation/American Heart Association Task Force on Practice Guidelines. Developed in collaboration with the American Association for Thoracic Surgery, Society of Cardiovascular Anesthesiologists, and Society of Thoracic Surgeons. J Am Coll Cardiol. (2011) 58:e123–210. doi: 10.1016/j.jacc.2011.08.009
131. ElBardissi A, Aranki S, Sheng S, O’Brien S, Greenberg C, Gammie J. Trends in isolated coronary artery bypass grafting: an analysis of the Society of Thoracic Surgeons adult cardiac surgery database. J Thorac Cardiovasc Surg. (2012) 143:273–81. doi: 10.1016/j.jtcvs.2011.10.029
132. Nah H, Lee J, Chung C, Choo S, Kwon S, Kim J, et al. New brain infarcts on magnetic resonance imaging after coronary artery bypass graft surgery: lesion patterns, mechanism, and predictors. Ann Neurol. (2014) 76:347–55. doi: 10.1002/ana.24238
133. Newman M, Kirchner J, Phillips-Bute B, Gaver V, Grocott H, Jones R, et al. Longitudinal assessment of neurocognitive function after coronary-artery bypass surgery. N Engl J Med. (2001) 344:395–402. doi: 10.1056/NEJM200102083440601
134. Berger M, Terrando N, Smith S, Browndyke J, Newman M, Mathew J. Neurocognitive function after cardiac surgery: from phenotypes to mechanisms. Anesthesiology. (2018) 129:829–51. doi: 10.1097/ALN.0000000000002194
135. Hogue C, Gottesman R, Stearns J. Mechanisms of cerebral injury from cardiac surgery. Crit Care Clin. (2008) 24:83–98, viii–ix. doi: 10.1016/j.ccc.2007.09.004
136. Likosky D, Marrin C, Caplan L, Baribeau Y, Morton J, Weintraub R, et al. Determination of etiologic mechanisms of strokes secondary to coronary artery bypass graft surgery. Stroke. (2003) 34:2830–4. doi: 10.1161/01.STR.0000098650.12386.B3
137. Wareing T, Davila-Roman V, Barzilai B, Murphy S, Kouchoukos N. Management of the severely atherosclerotic ascending aorta during cardiac operations. A strategy for detection and treatment. J Thorac Cardiovasc Surg. (1992) 103:453–62.
138. Wang K, Liu W, Chew S, Ti L, Shen L. New-onset atrial fibrillation after cardiac surgery is a significant risk factor for long-term stroke: an eight-year prospective cohort study. J Cardiothorac Vasc Anesth. (2021) 35:3559–64. doi: 10.1053/j.jvca.2021.07.003
139. Mitchell S, Merry A. Perspective on cerebral microemboli in cardiac surgery: significant problem or much ado about nothing? J Extra Corpor Technol. (2015) 47:10–5.
140. Jabur G, Willcox T, Zahidani S, Sidhu K, Mitchell S. Reduced embolic load during clinical cardiopulmonary bypass using a 20 micron arterial filter. Perfusion. (2014) 29:219–25. doi: 10.1177/0267659113504445
141. Chryssanthou C, Springer M, Lipschitz S. Blood-brain and blood-lung barrier alteration by dysbaric exposure. Undersea Biomed Res. (1977) 4:117–29.
142. Hills B, James P. Microbubble damage to the blood-brain barrier: relevance to decompression sickness. Undersea Biomed Res. (1991) 18:111–6.
143. Brown W, Moody D, Challa V, Stump D, Hammon J. Longer duration of cardiopulmonary bypass is associated with greater numbers of cerebral microemboli. Stroke. (2000) 31:707–13. doi: 10.1161/01.str.31.3.707
144. Borger M, Peniston C, Weisel R, Vasiliou M, Green R, Feindel C. Neuropsychologic impairment after coronary bypass surgery: effect of gaseous microemboli during perfusionist interventions. J Thorac Cardiovasc Surg. (2001) 121:743–9. doi: 10.1067/mtc.2001.112526
145. Taylor R, Borger M, Weisel R, Fedorko L, Feindel C. Cerebral microemboli during cardiopulmonary bypass: increased emboli during perfusionist interventions. Ann Thorac Surg. (1999) 68:89–93. doi: 10.1016/s0003-497500475-0
146. Pugsley W, Klinger L, Paschalis C, Treasure T, Harrison M, Newman S. The impact of microemboli during cardiopulmonary bypass on neuropsychological functioning. Stroke. (1994) 25:1393–9. doi: 10.1161/01.str.25.7.1393
147. Doganci S, Gunaydin S, Kocak O, Yilmaz S, Demirkilic U. Impact of the intensity of microemboli on neurocognitive outcome following cardiopulmonary bypass. Perfusion. (2013) 28:256–62. doi: 10.1177/0267659112470693
148. Soenarto R, Mansjoer A, Amir N, Aprianti M, Perdana A. Cardiopulmonary bypass alone does not cause postoperative cognitive dysfunction following open heart surgery. Anesth Pain Med. (2018) 8:e83610. doi: 10.5812/aapm.83610
149. Gipson K, Rosinski D, Schonberger R, Kubera C, Mathew E, Nichols F, et al. Elimination of gaseous microemboli from cardiopulmonary bypass using hypobaric oxygenation. Ann Thorac Surg. (2014) 97:879–86. doi: 10.1016/j.athoracsur.2013.08.074
150. Gerriets T, Schwarz N, Sammer G, Baehr J, Stolz E, Kaps M, et al. Protecting the brain from gaseous and solid micro-emboli during coronary artery bypass grafting: a randomized controlled trial. Eur Heart J. (2010) 31:360–8. doi: 10.1093/eurheartj/ehp178
151. Murkin J, Farrar J, Tweed W, McKenzie F, Guiraudon G. Cerebral autoregulation and flow/metabolism coupling during cardiopulmonary bypass: the influence of PaCO2. Anesth Analg. (1987) 66:825–32.
152. Fong H, Sands L, Leung J. The role of postoperative analgesia in delirium and cognitive decline in elderly patients: a systematic review. Anesth Analg. (2006) 102:1255–66. doi: 10.1213/01.ane.0000198602.29716.53
153. Moraca R, Lin E, Holmes J, Fordyce D, Campbell W, Ditkoff M, et al. Impaired baseline regional cerebral perfusion in patients referred for coronary artery bypass. J Thorac Cardiovasc Surg. (2006) 131:540–6. doi: 10.1016/j.jtcvs.2005.10.046
154. Neumann S, Burchell A, Rodrigues J, Lawton C, Burden D, Underhill M, et al. Cerebral blood flow response to simulated hypovolemia in essential hypertension: a magnetic resonance imaging study. Hypertension. (2019) 74:1391–8. doi: 10.1161/HYPERTENSIONAHA.119.13229
155. Pires P, Dams Ramos C, Matin N, Dorrance A. The effects of hypertension on the cerebral circulation. Am J Physiol Heart Circ Physiol. (2013) 304:H1598–614. doi: 10.1152/ajpheart.00490.2012
156. Gaudino M, Benesch C, Bakaeen F, DeAnda A, Fremes S, Glance L, et al. Considerations for reduction of risk of perioperative stroke in adult patients undergoing cardiac and thoracic aortic operations: a scientific statement from the American Heart Association. Circulation. (2020) 142:e193–209. doi: 10.1161/CIR.0000000000000885
157. Zhou H, Zhang X, Lu J. Progress on diabetic cerebrovascular diseases. Bosn J Basic Med Sci. (2014) 14:185–90. doi: 10.17305/bjbms.2014.4.203
158. Ergul A, Kelly-Cobbs A, Abdalla M, Fagan S. Cerebrovascular complications of diabetes: focus on stroke. Endocr Metab Immune Disord Drug Targets. (2012) 12:148–58. doi: 10.2174/187153012800493477
159. Nyström T, Holzmann M, Sartipy U. Long-term risk of stroke in patients with type 1 and type 2 diabetes following coronary artery bypass grafting. J Am Heart Assoc. (2015) 4:e002411. doi: 10.1161/JAHA.115.002411
160. Bucerius J, Gummert J, Borger M, Walther T, Doll N, Onnasch J, et al. Stroke after cardiac surgery: a risk factor analysis of 16,184 consecutive adult patients. Ann Thorac Surg. (2003) 75:472–8. doi: 10.1016/s0003-497504370-9
161. Gottesman R, Hillis A, Grega M, Borowicz L, Selnes O, Baumgartner W, et al. Early postoperative cognitive dysfunction and blood pressure during coronary artery bypass graft operation. Arch Neurol. (2007) 64:1111–4. doi: 10.1001/archneur.64.8.noc70028
162. Gottesman R, Sherman P, Grega M, Yousem D, Borowicz L, Selnes O, et al. Watershed strokes after cardiac surgery: diagnosis, etiology, and outcome. Stroke. (2006) 37:2306–11. doi: 10.1161/01.STR.0000236024.68020.3a
163. Ji B, Undar A. An evaluation of the benefits of pulsatile versus nonpulsatile perfusion during cardiopulmonary bypass procedures in pediatric and adult cardiac patients. ASAIO J. (2006) 52:357–61. doi: 10.1097/01.mat.0000225266.80021.9b
164. Su X, Guan Y, Barnes M, Clark J, Myers J, Undar A. Improved cerebral oxygen saturation and blood flow pulsatility with pulsatile perfusion during pediatric cardiopulmonary bypass. Pediatr Res. (2011) 70:181–5. doi: 10.1203/PDR.0b013e3182226b75
165. Veraar C, Rinösl H, Kühn K, Skhirtladze-Dworschak K, Felli A, Mouhieddine M, et al. Non-pulsatile blood flow is associated with enhanced cerebrovascular carbon dioxide reactivity and an attenuated relationship between cerebral blood flow and regional brain oxygenation. Crit Care. (2019) 23:426. doi: 10.1186/s13054-019-2671-7
166. Aykut K, Albayrak G, Guzeloglu M, Hazan E, Tufekci M, Erdoğan I. Pulsatile versus non-pulsatile flow to reduce cognitive decline after coronary artery bypass surgery: a randomized prospective clinical trial. J Cardiovasc Dis Res. (2013) 4:127–9. doi: 10.1016/j.jcdr.2013.05.005
167. Öztürk S, Saçar M, Baltalarlı A, Öztürk İ. Effect of the type of cardiopulmonary bypass pump flow on postoperative cognitive function in patients undergoing isolated coronary artery surgery. Anatol J Cardiol. (2016) 16:875–80. doi: 10.14744/AnatolJCardiol.2015.6572
168. Yuan S, Lin H. Postoperative cognitive dysfunction after coronary artery bypass grafting. Braz J Cardiovasc Surg. (2019) 34:76–84. doi: 10.21470/1678-9741-2018-0165
169. Tan AM, Amoako D. Postoperative cognitive dysfunction after cardiac surgery. Contin Educ Anaesth Crit Care Pain. (2013) 13:218–23. doi: 10.1093/bjaceaccp/mkt022
170. Lenkin A, Zaharov V, Lenkin P, Smetkin A, Bjertnaes L, Kirov M. Normothermic cardiopulmonary bypass increases cerebral tissue oxygenation during combined valve surgery: a single-centre, randomized trial. Interact Cardiovasc Thorac Surg. (2013) 16:595–601. doi: 10.1093/icvts/ivt016
171. Kadoi Y, Kawahara F, Saito S, Morita T, Kunimoto F, Goto F, et al. Effects of hypothermic and normothermic cardiopulmonary bypass on brain oxygenation. Ann Thorac Surg. (1999) 68:34–9. doi: 10.1016/s0003-497500306-9
172. Harris D, Oatridge A, Dob D, Smith P, Taylor K, Bydder G. Cerebral swelling after normothermic cardiopulmonary bypass. Anesthesiology. (1998) 88:340–5. doi: 10.1097/00000542-199802000-00011
173. Mavroudis C, Karlsson M, Ko T, Hefti M, Gentile J, Morgan R, et al. Cerebral mitochondrial dysfunction associated with deep hypothermic circulatory arrest in neonatal swine. Eur J Cardiothorac Surg. (2018) 54:162–8. doi: 10.1093/ejcts/ezx467
174. Cavaglia M, Seshadri S, Marchand J, Ochocki C, Mee R, Bokesch P. Increased transcription factor expression and permeability of the blood brain barrier associated with cardiopulmonary bypass in lambs. Ann Thorac Surg. (2004) 78:1418–25. doi: 10.1016/j.athoracsur.2004.04.036
175. Grimm M, Czerny M, Baumer H, Kilo J, Madl C, Kramer L, et al. Normothermic cardiopulmonary bypass is beneficial for cognitive brain function after coronary artery bypass grafting–a prospective randomized trial. Eur J Cardiothorac Surg. (2000) 18:270–5. doi: 10.1016/s1010-794000510-8
176. McLean R, Wong B. Normothermic versus hypothermic cardiopulmonary bypass: central nervous system outcomes. J Cardiothorac Vasc Anesth. (1996) 10:45–52; quiz 52–3. doi: 10.1016/s1053-077080178-9
177. Rees K, Beranek-Stanley M, Burke M, Ebrahim S. Hypothermia to reduce neurological damage following coronary artery bypass surgery. Cochrane Database Syst Rev. (2001) 2001:CD002138. doi: 10.1002/14651858.CD002138
178. Nenna A, Barbato R, Greco S, Pugliese G, Lusini M, Covino E, et al. Near-infrared spectroscopy in adult cardiac surgery: between conflicting results and unexpected uses. J Geriatr Cardiol. (2017) 14:659–61. doi: 10.11909/j.issn.1671-5411.2017.11.001
179. Chan M, Chung T, Glassford N, Bellomo R. Near-infrared spectroscopy in adult cardiac surgery patients: a systematic review and meta-analysis. J Cardiothorac Vasc Anesth. (2017) 31:1155–65. doi: 10.1053/j.jvca.2017.02.187
180. Bennett S, Smith N, Bennett M. Cerebral oximetry in adult cardiac surgery to reduce the incidence of neurological impairment and hospital length-of-stay: a prospective, randomized, controlled trial. J Intensive Care Soc. (2022) 23:109–16. doi: 10.1177/1751143720977280
181. Uysal S, Lin H, Trinh M, Park C, Reich D. Optimizing cerebral oxygenation in cardiac surgery: a randomized controlled trial examining neurocognitive and perioperative outcomes. J Thorac Cardiovasc Surg. (2020) 159:943–53.e3. doi: 10.1016/j.jtcvs.2019.03.036
182. Juliana N, Abu Yazit N, Kadiman S, Muhammad Hafidz K, Azmani S, Mohd Fahmi Teng N, et al. Intraoperative cerebral oximetry in open heart surgeries reduced postoperative complications: a retrospective study. PLoS One. (2021) 16:e0251157. doi: 10.1371/journal.pone.0251157
183. Serraino G, Murphy G. Effects of cerebral near-infrared spectroscopy on the outcome of patients undergoing cardiac surgery: a systematic review of randomised trials. BMJ Open. (2017) 7:e016613. doi: 10.1136/bmjopen-2017-016613
184. Zheng F, Sheinberg R, Yee M, Ono M, Zheng Y, Hogue C. Cerebral near-infrared spectroscopy monitoring and neurologic outcomes in adult cardiac surgery patients: a systematic review. Anesth Analg. (2013) 116:663–76. doi: 10.1213/ANE.0b013e318277a255
185. Homi H, Yang H, Pearlstein R, Grocott H. Hemodilution during cardiopulmonary bypass increases cerebral infarct volume after middle cerebral artery occlusion in rats. Anesth Analg. (2004) 99:974–81. doi: 10.1213/01.ANE.0000131504.90754.D0
186. Miura T, Sakamoto T, Kobayashi M, Shin’oka T, Kurosawa H. Hemodilutional anemia impairs neurologic outcome after cardiopulmonary bypass in a piglet model. J Thorac Cardiovasc Surg. (2007) 133:29–36. doi: 10.1016/j.jtcvs.2006.08.048
187. Li Q, Li S, Yang Q, Li T, Liu J, Yang C. Hemoglobin-based oxygen carrier attenuates cerebral damage by improving tissue oxygen preload in a dog model of cardiopulmonary bypass. Artif Cells Nanomed Biotechnol. (2015) 43:87–92. doi: 10.3109/21691401.2014.916716
188. Del Felice A, Tessari M, Formaggio E, Menon T, Petrilli G, Gamba G, et al. Hemoglobin concentration affects electroencephalogram during cardiopulmonary bypass: an indication for neuro-protective values. Artif Organs. (2016) 40:169–75. doi: 10.1111/aor.12533
189. Naveed A, Azam H, Murtaza H, Ahmad R, Baig M. Incidence and risk factors of pulmonary complications after cardiopulmonary bypass. Pak J Med Sci. (2017) 33:993–6. doi: 10.12669/pjms.334.12846
190. Al-Qubati F, Damag A, Noman T. Incidence and outcome of pulmonary complications after open cardiac surgery, Thowra Hospital, Cardiac center, Sana’a, Yemen. Egypt J Chest Dis Tuberc. (2013) 62:775–80.
191. Chen S, Chang C, Chu P, Chen T, Wu V, Huang Y, et al. Risk factor analysis of postoperative acute respiratory distress syndrome in valvular heart surgery. J Crit Care. (2016) 31:139–43. doi: 10.1016/j.jcrc.2015.11.002
192. Grommes J, Soehnlein O. Contribution of neutrophils to acute lung injury. Mol Med. (2011) 17:293–307. doi: 10.2119/molmed.2010.00138
193. Hilbert T, Duerr G, Hamiko M, Frede S, Rogers L, Baumgarten G, et al. Endothelial permeability following coronary artery bypass grafting: an observational study on the possible role of angiopoietin imbalance. Crit Care. (2016) 20:51. doi: 10.1186/s13054-016-1238-0
194. Koning N, Overmars M, van den Brom C, van Bezu J, Simon L, Vonk A, et al. Endothelial hyperpermeability after cardiac surgery with cardiopulmonary bypass as assessed using an in vitro bioassay for endothelial barrier function. Br J Anaesth. (2016) 116:223–32. doi: 10.1093/bja/aev411
195. Young R. Prevention of lung injury in cardiac surgery: a review. J Extra Corpor Technol. (2014) 46:130–41.
196. Tanner T, Colvin M. Pulmonary complications of cardiac surgery. Lung. (2020) 198:889–96. doi: 10.1007/s00408-020-00405-7
197. Schlensak C, Doenst T, Preusser S, Wunderlich M, Kleinschmidt M, Beyersdorf F. Bronchial artery perfusion during cardiopulmonary bypass does not prevent ischemia of the lung in piglets: assessment of bronchial artery blood flow with fluorescent microspheres. Eur J Cardiothorac Surg. (2001) 19:326–31; disciussion 331–2. doi: 10.1016/s1010-794000581-4
198. Dodd-o J, Welsh L, Salazar J, Walinsky P, Peck E, Shake J, et al. Effect of bronchial artery blood flow on cardiopulmonary bypass-induced lung injury. Am J Physiol Heart Circ Physiol. (2004) 286:H693–700. doi: 10.1152/ajpheart.00888.2003
199. Simonneau G, Montani D, Celermajer D, Denton C, Gatzoulis M, Krowka M, et al. Haemodynamic definitions and updated clinical classification of pulmonary hypertension. Eur Respir J. (2019) 53:1801913. doi: 10.1183/13993003.01913-2018
200. Diaz-Rodriguez N, Nyhan S, Kolb T, Steppan J. How we would treat our own pulmonary hypertension if we needed to undergo cardiac surgery. J Cardiothorac Vasc Anesth. (2022) 36:1540–8. doi: 10.1053/j.jvca.2021.09.030
201. Denault A, Deschamps A, Tardif J, Lambert J, Perrault L. Pulmonary hypertension in cardiac surgery. Curr Cardiol Rev. (2010) 6:1–14. doi: 10.2174/157340310790231671
202. Friedman M, Wang S, Stahl G, Johnson R, Sellke F. Altered beta-adrenergic and cholinergic pulmonary vascular responses after total cardiopulmonary bypass. J Appl Physiol. (1995) 79:1998–2006. doi: 10.1152/jappl.1995.79.6.1998
203. Ng C, Wan S, Yim A, Arifi A. Pulmonary dysfunction after cardiac surgery. Chest. (2002) 121:1269–77. doi: 10.1378/chest.121.4.1269
204. Serraf A, Sellak H, Hervé P, Bonnet N, Robotin M, Detruit H, et al. Vascular endothelium viability and function after total cardiopulmonary bypass in neonatal piglets. Am J Respir Crit Care Med. (1999) 159:544–51. doi: 10.1164/ajrccm.159.2.9803024
205. Komai H, Adatia I, Elliott M, de Leval M, Haworth S. Increased plasma levels of endothelin-1 after cardiopulmonary bypass in patients with pulmonary hypertension and congenital heart disease. J Thorac Cardiovasc Surg. (1993) 106:473–8.
206. Bond B, Dorman B, Clair M, Walker C, Pinosky M, Reeves S, et al. Endothelin-1 during and after cardiopulmonary bypass: association to graft sensitivity and postoperative recovery. J Thorac Cardiovasc Surg. (2001) 122:358–64. doi: 10.1067/mtc.2001.114936
207. Knothe C, Boldt J, Zickmann B, Ballesteros M, Dapper F, Hempelmann G. Endothelin plasma levels in old and young patients during open heart surgery: correlations to cardiopulmonary and endocrinology parameters. J Cardiovasc Pharmacol. (1992) 20:664–70. doi: 10.1097/00005344-199210000-00022
208. Kirshbom P, Jacobs M, Tsui S, DiBernardo L, Schwinn D, Ungerleider R, et al. Effects of cardiopulmonary bypass and circulatory arrest on endothelium-dependent vasodilation in the lung. J Thorac Cardiovasc Surg. (1996) 111:1248–56. doi: 10.1016/s0022-522370228-3
209. Ocal A, Kiriş I, Erdinç M, Peker O, Yavuz T, Ibrişim E. Efficiency of prostacyclin in the treatment of protamine-mediated right ventricular failure and acute pulmonary hypertension. Tohoku J Exp Med. (2005) 207:51–8. doi: 10.1620/tjem.207.51
210. Viaro F, Dalio M, Evora P. Catastrophic cardiovascular adverse reactions to protamine are nitric oxide/cyclic guanosine monophosphate dependent and endothelium mediated: should methylene blue be the treatment of choice? Chest. (2002) 122:1061–6. doi: 10.1378/chest.122.3.1061
211. Dora K, Stanley C, Al Jaaly E, Fiorentino F, Ascione R, Reeves B, et al. Isolated human pulmonary artery structure and function pre- and post-cardiopulmonary bypass surgery. J Am Heart Assoc. (2016) 5:e002822. doi: 10.1161/JAHA.115.002822
212. Crosswhite P, Sun Z. Nitric oxide, oxidative stress and inflammation in pulmonary arterial hypertension. J Hypertens. (2010) 28:201–12. doi: 10.1097/HJH.0b013e328332bcdb
213. Janssen L. Isoprostanes and lung vascular pathology. Am J Respir Cell Mol Biol. (2008) 39:383–9. doi: 10.1165/rcmb.2008-0109TR
214. Yi S, Kantores C, Belcastro R, Cabacungan J, Tanswell A, Jankov R. 8-Isoprostane-induced endothelin-1 production by infant rat pulmonary artery smooth muscle cells is mediated by Rho-kinase. Free Radic Biol Med. (2006) 41:942–9. doi: 10.1016/j.freeradbiomed.2006.05.035
215. Carteaux J, Roux S, Siaghy M, Schjöth B, Dolofon P, Bechamps Y, et al. Acute pulmonary hypertension after cardiopulmonary bypass in pig: the role of endogenous endothelin. Eur J Cardiothorac Surg. (1999) 15:346–52. doi: 10.1016/s1010-794000017-2
216. Reddy V, Hendricks-Munoz K, Rajasinghe H, Petrossian E, Hanley F, Fineman J. Post-cardiopulmonary bypass pulmonary hypertension in lambs with increased pulmonary blood flow. A role for endothelin 1. Circulation. (1997) 95:1054–61. doi: 10.1161/01.cir.95.4.1054
217. Beck J, Mongero L, Kroslowitz R, Choudhri A, Chen J, DeRose J, et al. Inhaled nitric oxide improves hemodynamics in patients with acute pulmonary hypertension after high-risk cardiac surgery. Perfusion. (1999) 14:37–42. doi: 10.1177/026765919901400106
218. Fullerton D, Jones S, Jaggers J, Piedalue F, Grover F, McIntyre R. Effective control of pulmonary vascular resistance with inhaled nitric oxide after cardiac operation. J Thorac Cardiovasc Surg. (1996) 111:753–62; discussion 762–3. doi: 10.1016/s0022-522370335-5
219. Fattouch K, Sbraga F, Sampognaro R, Bianco G, Gucciardo M, Lavalle C, et al. Treatment of pulmonary hypertension in patients undergoing cardiac surgery with cardiopulmonary bypass: a randomized, prospective, double-blind study. J Cardiovasc Med. (2006) 7:119–23. doi: 10.2459/01.JCM.0000203850.97890.fe
220. Winterhalter M, Simon A, Fischer S, Rahe-Meyer N, Chamtzidou N, Hecker H, et al. Comparison of inhaled iloprost and nitric oxide in patients with pulmonary hypertension during weaning from cardiopulmonary bypass in cardiac surgery: a prospective randomized trial. J Cardiothorac Vasc Anesth. (2008) 22:406–13. doi: 10.1053/j.jvca.2007.10.015
221. Kirbas A, Yalcin Y, Tanrikulu N, Gürer O, Isik O. Comparison of inhaled nitric oxide and aerosolized iloprost in pulmonary hypertension in children with congenital heart surgery. Cardiol J. (2012) 19:387–94. doi: 10.5603/cj.2012.0070
222. Lowson S, Doctor A, Walsh B, Doorley P. Inhaled prostacyclin for the treatment of pulmonary hypertension after cardiac surgery. Crit Care Med. (2002) 30:2762–4. doi: 10.1097/00003246-200212000-00023
223. Ram E, Sternik L, Klempfner R, Eldar M, Goldenberg I, Peled Y, et al. Sildenafil for pulmonary hypertension in the early postoperative period after mitral valve surgery. J Cardiothorac Vasc Anesth. (2019) 33:1648–56. doi: 10.1053/j.jvca.2018.12.023
224. Villanueva D, Agustin R, Llanes E. Pre-operative sildenafil for patients with pulmonary hypertension undergoing mitral valve surgery: a systematic review and meta-analysis. Cardiol Res. (2019) 10:369–77. doi: 10.14740/cr962
225. Bentlin M, Saito A, De Luca A, Bossolan G, Bonatto R, Martins A, et al. Sildenafil for pulmonary hypertension treatment after cardiac surgery. J Pediatr. (2005) 81:175–8.
226. Wang H, Gong M, Zhou B, Dai A. Comparison of inhaled and intravenous milrinone in patients with pulmonary hypertension undergoing mitral valve surgery. Adv Ther. (2009) 26:462–8. doi: 10.1007/s12325-009-0019-4
227. Eskandr A, Metwally A, Abu Elkassem M, Sadik S, Elmiligy A, Mourad M, et al. Dobutamine and nitroglycerin versus milrinone for perioperative management of pulmonary hypertension in mitral valve surgery. A randomized controlled study. J Cardiothorac Vasc Anesth. (2018) 32:2540–6. doi: 10.1053/j.jvca.2018.04.032
228. Schlensak C, Beyersdorf F. Lung injury during CPB: pathomechanisms and clinical relevance. Interact Cardiovasc Thorac Surg. (2005) 4:381–2. doi: 10.1510/icvts.2005.117853
229. Apostolakis E, Koletsis E, Baikoussis N, Siminelakis S, Papadopoulos G. Strategies to prevent intraoperative lung injury during cardiopulmonary bypass. J Cardiothorac Surg. (2010) 5:1. doi: 10.1186/1749-8090-5-1
230. Lopez-Lopez J, Moral-Sanz J, Frazziano G, Gomez-Villalobos M, Flores-Hernandez J, Monjaraz E, et al. Diabetes induces pulmonary artery endothelial dysfunction by NADPH oxidase induction. Am J Physiol Lung Cell Mol Physiol. (2008) 295:L727–32. doi: 10.1152/ajplung.90354.2008
231. Moral-Sanz J, Lopez-Lopez J, Menendez C, Moreno E, Barreira B, Morales-Cano D, et al. Different patterns of pulmonary vascular disease induced by type 1 diabetes and moderate hypoxia in rats. Exp Physiol. (2012) 97:676–86. doi: 10.1113/expphysiol.2011.062257
232. Abernethy A, Stackhouse K, Hart S, Devendra G, Bashore T, Dweik R, et al. Impact of diabetes in patients with pulmonary hypertension. Pulm Circ. (2015) 5:117–23. doi: 10.1086/679705
233. Lauruschkat A, Arnrich B, Albert A, Walter J, Amann B, Rosendahl U, et al. Diabetes mellitus as a risk factor for pulmonary complications after coronary bypass surgery. J Thorac Cardiovasc Surg. (2008) 135:1047–53. doi: 10.1016/j.jtcvs.2007.07.066
234. Tarcan O, Ozatik M, Kale A, Akgül A, Kocakulak M, Balci M, et al. Comparison of pulsatile and non-pulsatile cardiopulmonary bypass in patients with chronic obstructive pulmonary disease. Med Sci Monit. (2004) 10:CR294–9.
235. Siepe M, Goebel U, Mecklenburg A, Doenst T, Benk C, Stein P, et al. Pulsatile pulmonary perfusion during cardiopulmonary bypass reduces the pulmonary inflammatory response. Ann Thorac Surg. (2008) 86:115–22. doi: 10.1016/j.athoracsur.2008.03.062
236. Lim C, Nam M, Lee J, Kim H, Kim J, Shin H, et al. A meta-analysis of pulmonary function with pulsatile perfusion in cardiac surgery. Artif Organs. (2015) 39:110–7. doi: 10.1111/aor.12312
237. Santini F, Onorati F, Telesca M, Patelli F, Berton G, Franchi G, et al. Pulsatile pulmonary perfusion with oxygenated blood ameliorates pulmonary hemodynamic and respiratory indices in low-risk coronary artery bypass patients. Eur J Cardiothorac Surg. (2011) 40:794–803. doi: 10.1016/j.ejcts.2011.01.065
238. Engels G, Dodonov M, Rakhorst G, van Oeveren W, Milano A, Gu Y, et al. The effect of pulsatile cardiopulmonary bypass on lung function in elderly patients. Int J Artif Organs. (2014) 37:679–87. doi: 10.5301/ijao.5000352
239. Dodonov M, Onorati F, Luciani G, Francica A, Tessari M, Menon T, et al. Efficacy of pulsatile flow perfusion in adult cardiac surgery: hemodynamic energy and vascular reactivity. J Clin Med. (2021) 10:5934. doi: 10.3390/jcm10245934
240. Prasad K. Peripheral circulation and its control mechanism. In: JB Chang editor. Textbook of Angiology. New York, NY: Springer (2000). doi: 10.1007/978-1-4612-1190-7_3
241. Ugenti V, Romano A, Tibirica E. Microvascular endothelial dysfunction during cardiopulmonary bypass in surgery for correction of cyanotic and acyanotic congenital heart disease. Microvasc Res. (2018) 120:55–8. doi: 10.1016/j.mvr.2018.06.004
242. al-Khaja N, Belboul A, Bergman P, Roberts D, William-Olsson G. Cutaneous microcirculation and blood rheology following cardiopulmonary bypass. Laser Doppler flowmetric and blood cell rheologic studies. Scand J Thorac Cardiovasc Surg. (1988) 22:149–53. doi: 10.3109/14017438809105948
243. Gruber E, Schwarz B, Germann R, Breuss M, Bonatti J, Hasibeder W. Reactive hyperemia in skin after cardiopulmonary bypass. J Cardiothorac Vasc Anesth. (2000) 14:161–5. doi: 10.1016/s1053-077090011-9
Keywords: microvasculature, cardiac surgery, vasomotor tone, ischemia-reperfusion, organ damage
Citation: Kant S, Banerjee D, Sabe SA, Sellke F and Feng J (2023) Microvascular dysfunction following cardiopulmonary bypass plays a central role in postoperative organ dysfunction. Front. Med. 10:1110532. doi: 10.3389/fmed.2023.1110532
Received: 28 November 2022; Accepted: 30 January 2023;
Published: 14 February 2023.
Edited by:
Charissa van den Brom, Amsterdam University Medical Center, NetherlandsReviewed by:
Ugur Aksu, Istanbul University, TürkiyeChristopher G. Ellis, Western University, Canada
Copyright © 2023 Kant, Banerjee, Sabe, Sellke and Feng. This is an open-access article distributed under the terms of the Creative Commons Attribution License (CC BY). The use, distribution or reproduction in other forums is permitted, provided the original author(s) and the copyright owner(s) are credited and that the original publication in this journal is cited, in accordance with accepted academic practice. No use, distribution or reproduction is permitted which does not comply with these terms.
*Correspondence: Jun Feng, jfeng@lifespan.org