First-Principles Insight Into Au-Doped MoS2 for Sensing C2H6 and C2H4
- 1Electric Power Science Research Institute of Yunnan Power Grid Co., Ltd., Kunming, China
- 2State Key Laboratory of Power Transmission Equipment and System Security and New Technology, Chongqing University, Chongqing, China
- 3College of Engineering and Technology, Southwest University, Chongqing, China
C2H6 and C2H4 gases are two typical decompositions produced by partial discharge of transformer oil. To fully evaluate the feasibility of MoS2-based materials for the detection of C2H6 and C2H4 gases, the adsorption of C2H6 and C2H4 molecules on intrinsic and Au-doped MoS2 monolayer have been studied in this paper by the First-principle of Density Functional Theory (DFT). The adsorption mechanism of MoS2-based monolayer were investigated carefully in terms of adsorption energy, adsorption distance, bandgap structure, charge transfer and density of states (DOS). The calculated results show that the adsorption structures of the C2H6 and C2H4 molecules on Au-doped MoS2 monolayer with larger adsorption energies were stable, and have shorter adsorption distance, higher charge transfer, and stronger orbital hybridization compared with the corresponding MoS2 monolayer adsorption structures. It is concluded that the doped-Au atom affects the electronic structure of MoS2 monolayer to enhance the adsorption capacity. From this aspect, the present research offers a theoretical guidance to the application of Au-doped MoS2 materials as the sensing material for C2H6 and C2H4 gases.
Introduction
The transformers are considered to be the most important equipment in the power system, which affects the running state of power transmission (Chen W. G. et al., 2013; Ma H. et al., 2015; Uddin et al., 2016). After a long period of operation, the security and reliable operation ability of power transformers will be weakened due to inevitable defects, which will bring about power accidents and cause great inconvenience to the citizens and the society (Ma G. M. et al., 2015; Nobrega et al., 2019). Currently, failures within an oil-immersed transformer will cause the oil to decompose into several characteristic gases dissolved in it, including H2, CO, CO2, C2H2, CH4, C2H6, and C2H4. Some minor faults in the transformers may lead to the spark discharge in the transformer oil, then cause the oil decompose and produces C2H6, C2H4 gases (Benounis et al., 2008; Yang et al., 2011). Therefore, dissolved gas analysis (DGA) to monitor dissolved gas in transformer oil is regarded to be the most direct method to guarantee the stable running of power transformer or to predict some fault trends (Chatterjee et al., 2013; Suryavanshi et al., 2017; Cun et al., 2019; de Lima et al., 2019).
In recent decades, metal inorganic compound semiconductor sensors have been widely used in the field of gas detection (Zhang et al., 2017c; Zhou et al., 2018c, 2019; Chao et al., 2019; Kim et al., 2019, 2020; Wang et al., 2019c, 2020). Among them, nanowires or fiber materials sensors have attracted extensive attention due to their high sensitivity, fast response and recovery, low power consumption, and other excellent properties. The high sensitivity of nanowires or fiber materials sensors can be attributed to the large number of adsorption sites provided by high specific surface area (Chen X. et al., 2013; Qin and Ye, 2016; Wang et al., 2018). Molybdenum disulfide (MoS2) belongs to hexagonal crystal system and is a typical transition metal sulfide with a lamellar structure similar to graphene, which has the characteristics of surface effect, small size effect and quantum effect, and large specific surface area (Zhang et al., 2017b; Chen et al., 2019a). In addition, some studies have proved that nanometer MoS2, with excellent performances in adsorption capacity and other aspects, which is a special source of gas storage materials or sensing materials (Wen et al., 2016; Zhang et al., 2017a; Zhang D. Z. et al., 2018; Zhang Y. J. et al., 2018; Zhou et al., 2018b).
At present, the sensitivity or selectivity of most sensors are limited to some extent. In order to promote the sensing performance of gas sensors, dopant is usually used to enhance electron mobility and chemical reactivity of sensing materials. For instance, Zhou at al. verified that Pt nanoparticles doped SnO2 nanoneedles can be an effective gas sensing material for the detection of CO, which exhibits excellent response and recovery characteristic and remarkable selectivity (Zhou et al., 2018d). Zhang et al. studied the behavior of a MoS2/Co3O4-based ammonia gas sensor. They concluded that the MoS2/Co3O4 gas sensor exhibited excellent sensitivity performance to low-concentration ammonia, which is better than the pure Co3O4 gas sensor (Zhang et al., 2017a). Zhou at al. synthesized pure and Ni doped SnO2 nanomaterials and found the Ni doped SnO2 gas sensor shows lower optimum operating temperature and superior sensitivity properties to CO compared with the pure one (Zhou et al., 2018a).
Density functional theory (DFT) is a method to calculate the atomic or electronic structure and characteristic information of a material system by first-principles (Zhao and Wu, 2018; Wang et al., 2019c). Moreover, it has been widely employed to effectively predict the sensing properties of materials or interpret sensing mechanisms. Wang et al. analyzed the influence of Ni atom on the adsorption properties of ZnO (100) surface by using DFT calculations and found the doped Ni atom, as the active site, significantly enhance the adsorbability of SO2, SOF2, SO2F2 gases (Wang et al., 2019a). Zahra et al. investigated the adsorption of H2S on transition element (such as Ni, Cu, and Zn) atom-doped graphene based on DFT and concluded that the adsorption configuration of the H2S molecule near the dopant on the doped graphene surface is most stable (Zahra, 2018). Esrafili et al. used DFT to analyze the sensing mechanism of C-doped h-BN nanosheets, and the results indicated that the sensing of NO and NO2 can be selectively conducted in the presence of CO, H2O, CO2, and NH3 gases (Esrafili and Rad, 2019). Many studies have reported the performance and mechanism of MoS2-based gas sensors. However, MoS2 based fiber-materials as the gas sensor to detect transformer oil dissolved-gases has hardly been studied theoretically and experimentally.
In this study, Au was employed as a dopant to change the structural characteristics and electronic properties of MoS2 fiber-materials, and the adsorption performance of Au-doped MoS2 to C2H6 and C2H4 gases was investigated through DFT study. The stable adsorption structures of C2H6 and C2H4 molecules on intrinsic or Au-doped MoS2 monolayer were built. Furthermore, the adsorption energies, charge transfer, adsorption distance, density of states, and bandgap have been calculated to comprehensively investigate the adsorption abilities of Au-doped MoS2 monolayer to C2H6 and C2H4 gases. The results of this research have important guiding significance for the design of high efficiency MoS2 fiber based sensors to detect fault characteristic gas in transformer oil (C2H6 and C2H4 gases).
Calculation DetaiLS
All the First-principles calculations in this study were processed in Dmol3 package of Materials Studio software based on DFT. General Gradient Approximate (GGA) of Perdew-Burke-Ernzerhof (PBE) were selected to optimize the geometric and calculate the exchange-correlation energy (Qian et al., 2019). The double numerical plus polarization (DNP) and the density functional semi-core pseudopotential (DSPP) were taken to processed the core electron. In addition, the k-point grid was generated according to the Monkhorst-Pack scheme and the sampling k point of Brillouin zone was set to 5 × 5 × 1. The self-consistent field (SCF) convergence precision was set to 1 × 10−6 Ha and the DIIS was set to 6 to shorten the convergence time of SCF. The convergence tolerance, maximum force and maximum displacement were set to 1 × 10−5 Ha, 2 × 10−3 Ha/Å, and 5 × 10−3 Ha in the geometry optimization processes. All simulations results in present study were based on a 4 × 4 × 1 MoS2 supercell with 48 atoms (including 32 S atoms and 16 Mo atoms). With regard to the periodic boundary conditions (PBC), a vacuum layer in z-direction of 20 Å, which is perpendicular to the xy-plane, was employed to prevent the interaction between the adjacent layers.
The adsorption energy (Eads) of gases (C2H6, C2H4, and C2H2) on the pristine or Au-doped MoS2 monolayer is defined in Equation (1) (Chen et al., 2019b).
where Etotal, Emonolayer, and Egas are the energy of pristine or Au-doped MoS2 monolayer adsorbed system, corresponding monolayer and gas molecule, respectively. A negative adsorption energy represents spontaneous adsorption. The amount of charge transfer (Qt) can be obtained based on the Mulliken analysis, and Qt is calculated by Equation (2) (Chen et al., 2015).
where Qa and Qb are the carried charge of the adsorbed gas and the isolated gas. A positive Qt suggests the gas accept charges from monolayer during the adsorption.
Results
Properties of Gas Molecules and MoS2 Monolayer
The optimized structures with corresponding parameters of C2H6 and C2H4 are shown in Figure 1. The bond length of C-C, C-H in C2H6 are 1.527 and 1.100 Å, and the bond angle of H-C-H is 107.4°. For C2H4 molecule, the structure is symmetric around the C-C bond (the length is 1.335 Å), the H-C-H angle is 116.6° and the length of C-H bond is similar to C2H6, which is 1.092 Å. Mulliken atomic charge analysis (Table 1) exhibits that the C atom of C2H6 molecule has negative charges of −0.868e, while the carried charges of H atoms is 0.047e. The carried charges of C and H atoms in C2H4 are −0.101e and 0.050e. It can be concluded that the electrons of C atoms are transferred from H atoms, which indicates the interaction between C atoms and H atoms.
The optimized structure of MoS2 monolayer model with the lattice parameters of 12.424, 12.584, and 18.152 Å in the a, b, and c directions, as shown in Figure 2A. Besides, the optimized bond angles of S-Mo-S and Mo-S-Mo are achieved as 81.5°, which are comparable to 81.5° in previous study (Wang et al., 2019b). The electronic structural characteristics of MoS2 monolayer were investigated, the relevant band gap structure and DOSs are shown in Figures 2B–D. It is easily to distinguish the valence band maximum (VBM) and conduction band minimum (CBM), and the Fermi level (represented by the line of zero energy) is located in the energy bandgap. As a result, the considered MoS2 monolayer with band gap of 2.058 eV is observed to be a direct band semiconductor. The total density of state in Figure 2C is composed of the conduction band, upper valence band, and lower valence band with energy values of 1.9~2.5 eV, −5.8~0 eV, and −14~−11.8 eV, respectively. According to the PDOS in Figure 2D, the conduction band is mainly dominated by 4d-orbital of Mo atom, while the 4p, 4d-orbitals of Mo atom and 3s, 3p-orbitals of S atom contribute to the valence band.
Adsorption Properties of Molecules on MoS2 Monolayer
The C2H6, C2H4, and C2H2 molecules were placed above the MoS2 monolayer, the optimized adsorption structures and DOSs are shown in Figure 3. Table 2 reports the corresponding adsorption energy (Eads), charge transfer (Qt), adsorption distance (d), and band gap (Eg). As exhibited in Figures 3C,D, the density of state of two adsorption systems change only slightly. In addition, the Eads of C2H6, C2H4, and C2H2 adsorbed MoS2 monolayer are only −0.082, −0.106, and −0.101 eV, the corresponding Qt and the change of band gap are very small, which clearly suggests that the adsorptions belong to physical adsorption with Van der Waals force. In conclusion, the C2H6, C2H4, and C2H2 gases are difficult to be stably adsorbed on pristine MoS2 monolayer at room temperature, because the material is theoretically not active enough to adsorb them.
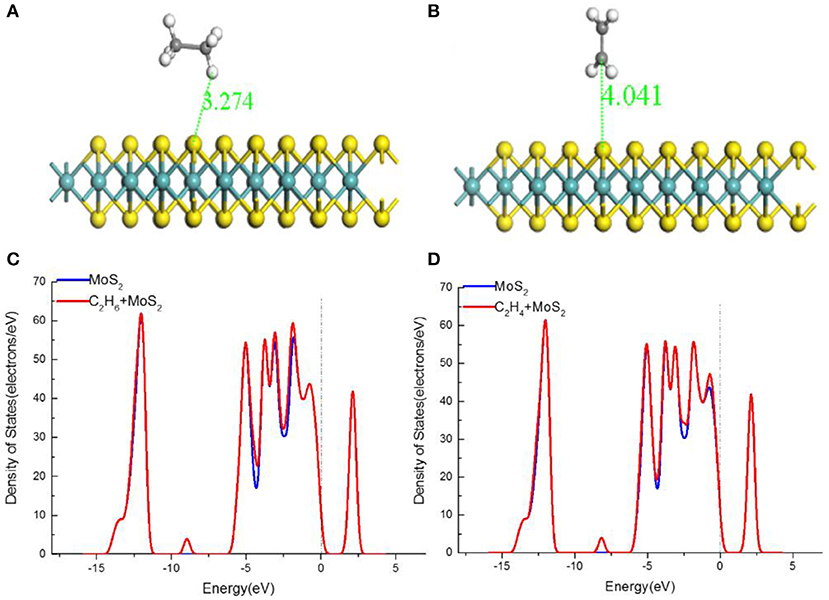
Figure 3. The structures of MoS2 monolayer of (A) C2H6-adsorbed MoS2 monolayer, (B) C2H4-adsorbed MoS2 monolayer and the TDOS of (C) C2H6-adsorbed MoS2 monolayer, (D) C2H4-adsorbed MoS2 monolayer.
Properties of Au-Doped MoS2 Monolayer
According to the previous research, it is the stable doped position that putting Au atom on the top of the Mo atom. As shown in Figure 4A, three Au-S bonds with the same length of 2.809 Å have been formed and the distance (3.749 Å) between Au and Mo atoms is too far to form bond due to the weak interaction. Compared with the undoped structure, the bond angle of Mo–S–Mo (80.8°) and S–Mo–S (81.3°) in Au doped MoS2 monolayer have slightly decreases. The carried electrons of the Au atom to MoS2 monolayer was calculated to 0.043e, suggesting the interaction between Au atom and the surface of MoS2 monolayer leads to a steady doping structure.
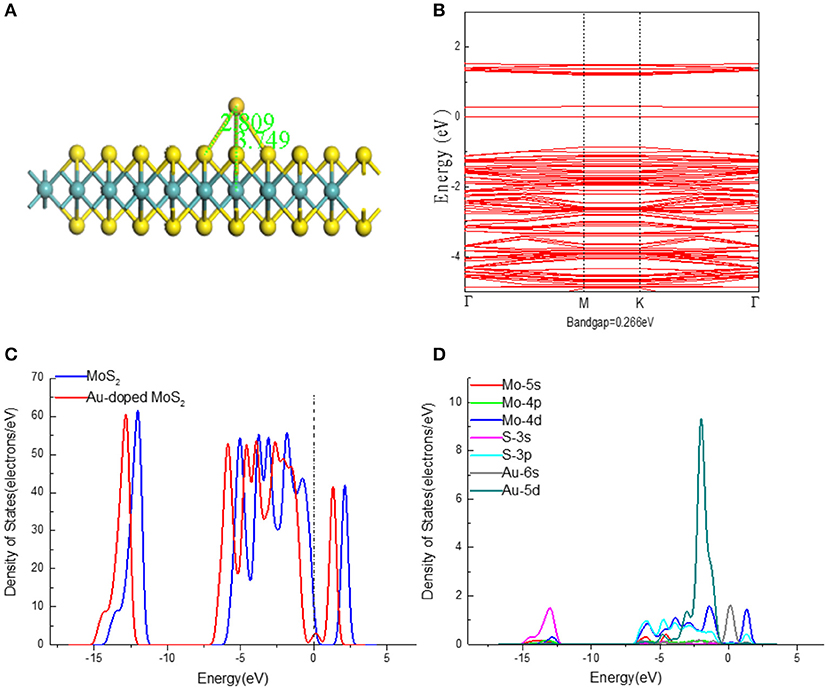
Figure 4. (A) The structures, (B) Band structures, (C) TDOS, and (D) PDOS of Au-doped MoS2 monolayer.
For the PDOS (Figure 4D) of doped system, it can be found there is large overlap area from −3.7 to 0 eV of S-3p orbital and Au-5d orbital, verifying that strong hybridization occurs between S-3p and Au-5d orbitals. It can be inferred that chemical interaction exists between the doped Au atom and MoS2 to promote the formation of the final stable doping structure. Figure 4C shows the waveform of DOS has barely changed except for the obvious left shift after the dope of Au atom, which not only indicates an increase in the amount of system electrons, but means a promotion in the metallicity. The Au-doping introduces some impurity energy levels from the Au-6s and 5d orbitals into the band gap, leading to a narrowing of the bandgap (0.266 eV), which is corresponding with Figure 4B. The smaller band gap implies the lower kinetic stability and the higher chemical activity. Therefore, Au-doping makes the transfer from valence band to conduction band of electrons become easier, thus the conductivity and reactivity are improved.
Adsorption Properties of Molecules on Au-Doped MoS2 Monolayer
To investigate the adsorption properties of Au-doped MoS2 monolayer to C2H6 molecule, a C2H6 was placed at different positions to close the Au atom, and geometric optimization was conducted. The most stable structure is shown in Figure 5A, the corresponding adsorption energy, charge transfer, adsorption distance and band gap of this adsorption configurations are listed in Table 3 and the density of states (DOS) are illustrated in Figures 5B–D.
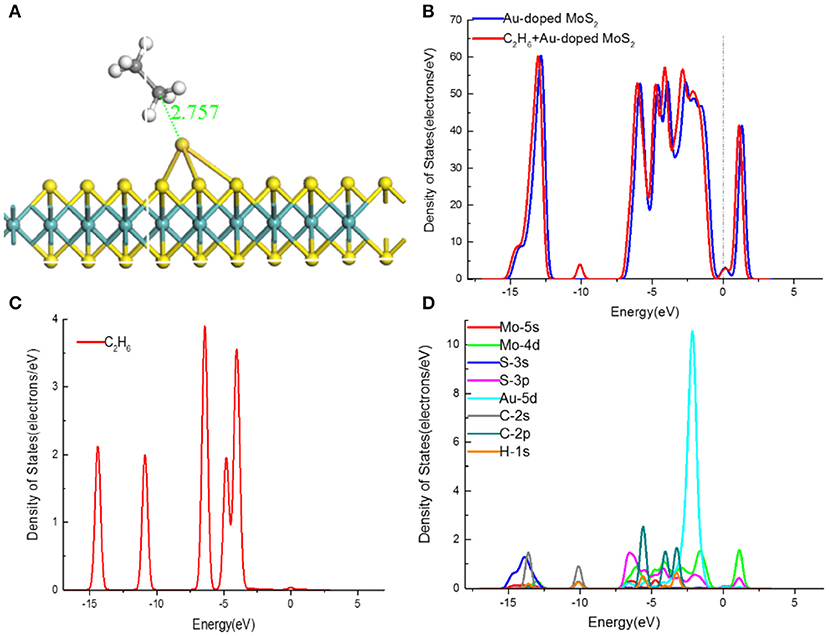
Figure 5. (A) The structures, (B) TDOS, (D) PDOS of C2H6-adsorbed MoS2 monolayer, and (C) the TDOS of C2H6 molecular.
The C2H6 molecule was trapped at the Au doping site and the distance between Au atom and C atom is 2.757 Å, which is significantly smaller than that in MoS2 monolayer adsorption system (3.274 Å). Besides, it can be found that the structure of C2H6 molecule changes little after being adsorbed by Au-doped MoS2 monolayer, while the change of the Au-S bonds is clearly observed. According to Table 3, the adsorption energy is −0.463 eV, which is greater than the intrinsic adsorption structure. Moreover, the value of transferred electrons that calculated by Mulliken analysis is 0.118e, indicating the Au–MoS2 monolayer acts as an acceptor for the electrons transferred from the C2H6 molecule. It is observed that the band gap of Au-doped MoS2 monolayer system reduce from 0.266 to 0.206 eV. Therefore, the conclusion that the C2H6 molecule was adsorbed on the Au–MoS2 monolayer by chemisorption is obtained.
To further explore the adsorption mechanism between the Au–doped MoS2 monolayer and C2H6 molecule, the total density of states (TDOS) and the projected density of states (PDOS) of the adsorption system are analyzed in detail. Adsorption is mainly affected by the outer atomic orbitals, hence the Mo-5s, Mo-4d, S-3s, S-3p, Au-5d, C-2s, and H-1s orbitals were investigated, as shown in Figure 5D. Distinctly, some changes of TDOS after C2H6 adsorption can be observed in Figure 5B, such as the increases around the Fermi level, which may be consistent with the reduction of the bandgap. It can be deduced that this phenomenon is due to the introduction of impurity energy level caused by a large amount of electrons transfer in the adsorption. In addition, a narrower bandgap suggests the adsorption structure conducts electricity better and the electrons are more easily to transfer. The TDOS has a left shift and some new peaks at −10 and −2.4 eV appear. By the comparison with Figure 5C, it can be found that the changes of TDOS are influenced by the C2H6 molecule and the slight peak near the Fermi level in the DOS of C2H6 contributes to the decrease of the bandgap, particularly. Moreover, it can be implied that some orbitals hybridization occur to the C2H6 adsorption Au–doped MoS2 monolayer system. Figure 5D concludes that the C-2p, Mo-4d and Au-5d orbitals dominate the changes of TDOS. The H-1s and Au-5d overlap around −3 eV, while the obvious overlapping region of C-2p, Mo-4d, and Au-5d orbitals at −5.5 and −2.5 eV. The PDOS overlap of the orbitals indicates hybridization happen between them. Therefore, it can be concluded by the contribution of the C-2p orbitals that during the adsorption process, the Au–doped MoS2 monolayer mainly adsorbs C2H6 molecule by capturing C atom.
Above all, the Au–doped MoS2 monolayer has a large adsorption energy, more transferred electrons, short adsorption distance and more obvious orbitals hybridization than the corresponding intrinsic adsorption system. In conclusion, the adsorption performance of MoS2 monolayer has a significant improvement after Au doped and the doped structure can effectively absord the C2H6 molecule.
For C2H4 molecule adsorption on Au-doped MoS2 monolayer, the most stable adsorption structure is presented in Figure 6A, where the molecule was adsorbed by the direction of parallel to MoS2 monolayer. The adsorption properties parameters are classified at Table 3.
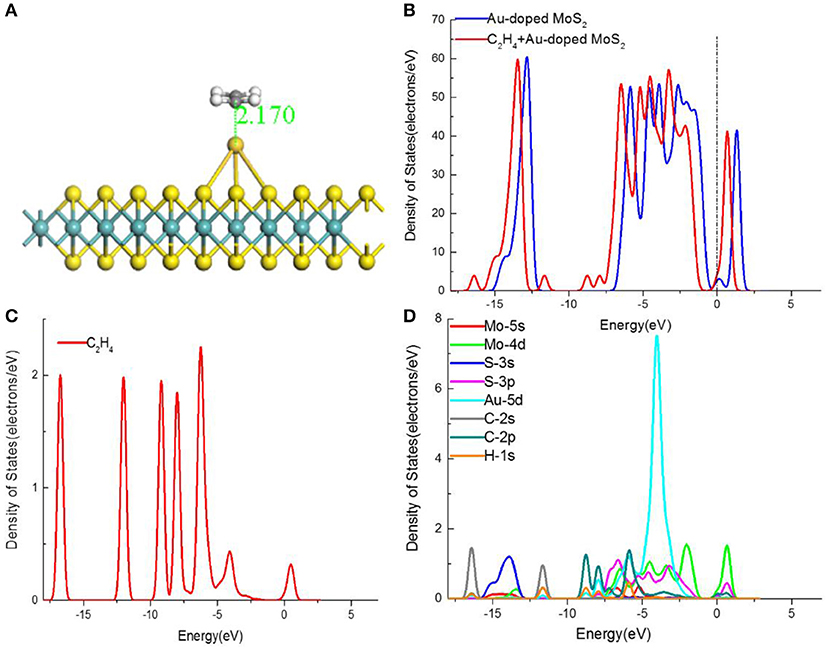
Figure 6. (A) The structures, (B) TDOS, (D) PDOS of C2H4-adsorbed MoS2 monolayer, and (C) the TDOS of C2H4 molecular.
The adsorption of C2H4 molecule is characterized by a large negative adsorption energy (−0.952 eV), suggesting the chemical adsorption happen to the C2H4 molecule and Au-doped MoS2 monolayer. By comparing with the adsorption on pristine MoS2 monolayer, the higher adsorption energy implies that the interaction is stronger. Moreover, it can be noticed that the adsorption distance between doped Au atom and C atom in C2H4 molecule is 2.170 Å, illustrating the formation of Au-C covalent bond. Additionally, the amount of transferred charges in this adsorption system is 0.309e. The reorganization of charges indicates that the Au-doped MoS2 monolayer acquires charges from C2H4 molecule. It is observed the charge transfer is much larger than the corresponding pristine MoS2 monolayer adsorption structure, which indicates the dope of Au atom make the adsorption reaction between monolayer and C2H4 stronger. The band gap is decreased to 0.122 eV compared with that of Au-doped MoS2 monolayer (0.266 eV), and the change represents the C2H4 molecule has the influence on the electronic structure of monolayer. In a conclusion, the C2H4 molecule could be detected effectively by the Au-doped MoS2 monolayer.
The DOS and PDOS of C2H4 molecule adsorbed Au–doped MoS2 monolayer were conducted to understand the effect of the C2H4 on the electronic properties of Au–doped MoS2 monolayer. Adsorption is mainly affected by the outer atomic orbitals, hence the Mo-5s, Mo-4d, S-3s, S-3p, Au-5d, C-2s, and H-1s orbitals were investigated, as shown in Figure 6D. From Figure 6A, it is observed that the DOS of adsorption system has a certain displacement compared with the Au–doped MoS2 monolayer, in which there is the apparent transition near the Fermi level. Furthermore, the bandgap decreased after the adsorption of C2H4 molecule, indicating the transfer of electrons between the conduction band and the valance band is easier. The DOS shifts to a lower region, which is consistent well with the charges accepting behavior of Au–doped MoS2 monolayer. To be specific, the electron density in the conduction band is improve due to the electron-gaining and right shift of the Fermi level is happened, which in accordance well with the left shift of DOS. In conclusion, the electrical conductivity of Au–doped MoS2 monolayer is greatly improved after adsorbing C2H4 molecule. Combined with the observation of Figures 6B,C, it can be found that the contributions of C2H4 to the DOS of system are at the energies of −17, −12, −9, −4, and 0.5 eV. The obvious overlapping peak Au-5d, C-2p, and H-1s orbitals at −6 eV displayed in Figure 6D reveals the strong hybridization among them, which elucidate the strong attraction of Au–doped MoS2 monolayer to C2H4 molecule.
The large adsorption energy, larger transferred electrons, shorter adsorption distance and stronger orbitals hybridization perhaps reveal that the stronger interaction in Au-doped adsorption system than in MoS2 monolayer adsorption structure. In consequence, the Au–doped MoS2 monolayer is sensitive enough to detect C2H4 gas and it is able to be a potential sensing material for C2H4 sensors.
Conclusion
In this paper, the adsorption mechanism of C2H6 and C2H4 gases on MoS2 based sensing materials were analyzed by the First-principles with DFT calculations. Firstly, the model of intrinsic MoS2 monolayer was optimized and its structural parameters and electronic characteristics were calculated. It is found that MoS2 monolayer with band gap of 2.058 eV is observed to be a direct band semiconductor. Then the adsorption of C2H6 and C2H4 molecules on MoS2 monolayer were investigated, the corresponding structural parameters, charge transfer, adsorption energies and density of states were calculated to obtain the adsorption capacity. The results show that the intrinsic MoS2 monolayer cannot adsorb gas effectively. The Au atom was chose as the dopant to enhance the properties of MoS2 monolayer and the bandgap of Au-doped MoS2 monolayer is decreased to 0.266 eV, which indicates a promotion in the metallicity. In final, the adsorption structures of Au-doped MoS2 monolayer to C2H6 and C2H4 molecules were modeled and the electronic structure of adsorption systems was investigated in detail. The adsorption energies to C2H6 and C2H4 are −0.463 and −0.952 eV, and the transferred charges from molecules to Au-doped MoS2 monolayer are 0.118e and 0.309e, respectively. The impressive evidences from PDOS verify the hybridization between the orbitals of molecules and Au-doped MoS2 monolayer, which implies the strong interaction between them. In addition, the increased conductivity after gases adsorption were indicated by the decreased band gap. Therefore, it is concluded that the Au doping promotes the adsorption capacity of MoS2 monolayer to C2H6 and C2H4 molecules by influencing the electronic properties. In summary, the present study demonstrates the surface reactivity to C2H6 and C2H4 gases of MoS2 based sensing materials, which is meaningful to develop the MoS2 based gas sensors for the detection of C2H6 and C2H4 gases.
Data Availability Statement
The raw data supporting the conclusions of this article will be made available by the authors, without undue reservation, to any qualified researcher.
Author Contributions
All authors listed have made a substantial, direct and intellectual contribution to the work, and approved it for publication.
Conflict of Interest
The authors declare that the research was conducted in the absence of any commercial or financial relationships that could be construed as a potential conflict of interest.
References
Benounis, M., Aka-Ngnui, T., Jaffrezic, N., and Dutasta, J. P. (2008). NIR and optical fiber sensor for gases detection produced by transformation oil degradation. Sens. Actuators A Phys. 141, 76–83. doi: 10.1016/j.sna.2007.07.036
Chao, J. F., Chen, Y. H., Xing, S. M., Zhang, D. L., and Shen, W. L. (2019). Facile fabrication of ZnO/C nanoporous fibers and ZnO hollow spheres for high performance gas sensor. Sens. Actuators B Chem. 298:126927. doi: 10.1016/j.snb.2019.126927
Chatterjee, A., Bhattacharjee, P., Roy, N. K., and Kumbhakar, P. (2013). Usage of nanotechnology based gas sensor for health assessment and maintenance of transformers by DGA method. Int. J. Electr. Power 45, 137–141. doi: 10.1016/j.ijepes.2012.08.044
Chen, D. C., Tang, J., Zhang, X. X., Li, Y., and Liu, H. J. (2019a). Detecting decompositions of sulfur hexafluoride using MoS2 monolayer as gas sensor. IEEE Sens. J. 19, 39–46. doi: 10.1109/JSEN.2018.2876637
Chen, D. C., Zhang, X. X., Tang, J., Cui, Z. L., and Cui, H. (2019b). Pristine and Cu decorated hexagonal InN monolayer, a promising candidate to detect and scavenge SF6 decompositions based on first-principle study. J. Hazard. Mater. 363, 346–357. doi: 10.1016/j.jhazmat.2018.10.006
Chen, W. G., Zhou, Q., Gao, T. Y., Su, X. P., and Wan, F. (2013). Pd-doped SnO2-based sensor detecting characteristic fault hydrocarbon gases in transformer oil. J. Nanomater. 2013, 2521–2535. doi: 10.1155/2013/127345
Chen, X., Wong, C. K. Y., Yuan, C. A., and Zhang, G. (2013). Nanowire-based gas sensors. Sens. Actuators B Chem. 177, 178–195. doi: 10.1016/j.snb.2012.10.134
Chen, Y. P., Wang, X. F., Shi, C. M., Li, L., Qin, H. W., and Hu, J. F. (2015). Sensing mechanism of SnO2 (1 1 0) surface to H2: density functional theory calculations. Sens. Actuators B Chem. 220, 279–287. doi: 10.1016/j.snb.2015.05.061
Cun, H., Chen, D. C., Zhang, Y., and Zhang, X. X. (2019). Dissolved gas analysis in transformer oil using Pd catalyst decorated MoSe2 monolayer: a first-principles theory. Sustain. Mater. Technol. 17:e00094. doi: 10.1016/j.susmat.2019.e00094
de Lima, R. A., Soares, V. H., Martins, J. F., and Fontana, E. (2019). Tailoring a spectral line detection system for applications in dissolved gas analysis. Sens. Actuators A Phys. 293, 178–188. doi: 10.1016/j.sna.2019.04.020
Esrafili, M. D., and Rad, F. A. (2019). Carbon-doped boron nitride nanosheets as highly sensitive materials for detection of toxic NO and NO2 gases: a DFT study. Vacuum 166, 127–134. doi: 10.1016/j.vacuum.2019.04.065
Kim, J. H., Mirzaei, A., Kim, H. W., and Kim, S. S. (2019). Realization of Au-decorated WS2 nanosheets as low power-consumption and selective gas sensors. Sens. Actuators B Chem. 296:126659. doi: 10.1016/j.snb.2019.126659
Kim, J. H., Mirzaei, A., Kim, H. W., and Kim, S. S. (2020). Variation of shell thickness in ZnO-SnO2 core-shell nanowires for optimizing sensing behaviors to CO, C6H6, and C7H8 gases. Sens. Actuators B Chem. 302:127150. doi: 10.1016/j.snb.2019.127150
Ma, G. M., Jiang, J., Li, C. R., Song, H. Y., Luo, Y. T., and Wang, H. B. (2015). Pd/Ag coated fiber Bragg grating sensor for hydrogen monitoring in power transformers. Rev. Sci. Instrum. 86:045003. doi: 10.1063/1.4918802
Ma, H., Saha, T. K., Ekanayake, C., and Martine, D. (2015). Smart transformer for smart grid-intelligent framework and techniques for power transformer asset management. IEEE. Trans. Smart. Grid. 2, 1026–1034. doi: 10.1109/TSG.2014.2384501
Nobrega, L. A. M. M., Xavier, G. V. R., Aquino, M. V. D., Serres, A. J. R. C. C. R., Albuquerque, C. C. R., and Costa, E. G. (2019). Design and development of a bio-inspired UHF sensor for partial discharge detection in power transformers. Sensors 19:653. doi: 10.3390/s19030653
Qian, H., Lu, W. H., Wei, X. X., Chen, W., and Deng, J. (2019). H2S and SO2 adsorption on Pt-MoS2 adsorbent for partial discharge elimination: a DFT study. Results Phys. 12, 107–112. doi: 10.1016/j.rinp.2018.11.035
Qin, Y., and Ye, Z. (2016). DFT study on interaction of NO2 with the vacancy-defected WO3 nanowires for gas-sensing. Sens. Actuators B Chem. 222, 499–507. doi: 10.1016/j.snb.2015.08.040
Suryavanshi, H., Velandy, J., and Sakthivel, M. (2017). Wavelet power ratio signature spectrum analysis for prediction of winding insulation defects in transformer and shunt reactor. IEEE Trans. Dielectr. Electr. Insul. 24, 2649–2659. doi: 10.1109/TDEI.2017.006328
Uddin, A. S. M. I., Yaqoob, U., and Chung, G. S. (2016). Dissolved hydrogen gas analysis in transformer oil using Pd catalyst decorated on ZnO nanorod array. Sens. Actuators B Chem. 226, 90–95. doi: 10.1016/j.snb.2015.11.110
Wang, J., Jiang, S. S., Liu, H. L., Wang, S. H., Pan, Q. J., Yin, Y. D., et al. (2020). P-type gas-sensing behavior of Ga2O3/Al2O3 nanocomposite with high sensitivity to NOx at room temperature. J. Alloy. Compd. 814:152284. doi: 10.1016/j.jallcom.2019.152284
Wang, J. X., Zhou, Q., Lu, Z. R., Gui, Y. G., and Zeng, W. (2019b). Adsorption of H2O molecule on TM (Au, Ag) doped-MoS2 monolayer: a first-principles study. Physica E 113, 72–78. doi: 10.1016/j.physe.2019.05.006
Wang, J. X., Zhou, Q., Lu, Z. R., Wei, Z. J., and Zeng, W. (2019c). Gas sensing performances and mechanism at atomic level of Au-MoS2 microspheres. Appl. Surf. Sci. 490, 124–136. doi: 10.1016/j.apsusc.2019.06.075
Wang, J. X., Zhou, Q., and Zeng, W. (2019a). Competitive adsorption of SF6 decompositions on Ni-doped ZnO (100) surface: computational and experimental study. Appl. Surf. Sci. 479, 185–197. doi: 10.1016/j.apsusc.2019.01.255
Wang, X. H., Wang, D. W., Yang, A. J., Koratkar, N., Chu, J. F., Lv, P. L., et al. (2018). Effects of adatom and gas molecule adsorption on the physical properties oftellurene: a first principles investigation. Phys. Chem. Chem. Phys. 20, 4058–4066. doi: 10.1039/C7CP07906K
Wen, M. Q., Xiong, T., Zang, Z. G., Wei, W., Tang, X. T., and Dong, F. (2016). Synthesis of MoS2/g-C3N4 nanocomposites with enhanced visible-light photocatalytic activity for the removal of nitric oxide (NO). Opt. Express 24, 10205–10212. doi: 10.1364/OE.24.010205
Yang, F., Jung, D., and Penner, R. M. (2011). Trace detection of dissolved hydrogen gas in oil using a palladium nanowire array. Anal. Chem. 83, 9472–9477. doi: 10.1021/ac2021745
Zahra, K. (2018). Evaluation of H2S sensing characteristics of metals–doped graphene and metals-decorated graphene: insights from DFT study. Physica E 99, 261–268. doi: 10.1016/j.physe.2018.02.022
Zhang, D. Z., Jiang, C. X., Li, P., and Sun, Y. E. (2017a). Layer-by-layer self-assembly of Co3O4 nanorod-decorated MoS2 nanosheet-based nanocomposite toward high-performance ammonia detection. ACS. Appl. Mater. Inter. 9, 6462–6471. doi: 10.1021/acsami.6b15669
Zhang, D. Z., Jiang, C. X., and Wu, J. F. (2018). Layer-by-layer assembled In2O3 nanocubes/flower-like MoS2 nanofilm for room temperature formaldehyde sensing. Sens. Actuators B Chem. 273, 176–184. doi: 10.1016/j.snb.2018.06.044
Zhang, D. Z., Sun, Y. E., Jiang, C. X., and Zhang, Y. (2017b). Room temperature hydrogen gas sensor based on palladium decorated tin oxide/molybdenum disulfide ternary hybrid via hydrothermal route. Sens. Actuators B Chem. 242, 15–24. doi: 10.1016/j.snb.2016.11.005
Zhang, D. Z., Wu, J. F., Li, P., and Cao, Y. H. (2017c). Room-temperature SO2 gas sensing properties based on metal-doped MoS2 nanoflower: an experimental and density functional theory Investigation. J. Mater. Chem. A, 5, 20666–20677. doi: 10.1039/C7TA07001B
Zhang, Y. J., Zeng, W., and Li, Y. Q. (2018). Hydrothermal synthesis and controlled growth of hierarchical 3D flower-like MoS2 nanospheres assisted with CTAB and their NO2 gas sensing properties. Appl. Surf. Sci. 455, 276–282. doi: 10.1016/j.apsusc.2018.05.224
Zhao, C. J., and Wu, H. R. (2018). A first-principles study on the interaction of biogas with noble metal (Rh, Pt, Pd) decorated nitrogen doped graphene as a gas sensor: a DFT study. Appl. Surf. Sci. 435, 1199–1212. doi: 10.1016/j.apsusc.2017.11.146
Zhou, Q., Chen, W. G., Xu, L. N., Kumarc, R., Gui, Y. G., Zhao, Z. Y., et al. (2018a). Highly sensitive carbon monoxide (CO) gas sensors based on Ni and Zn doped SnO2 nanomaterials. Ceram. Int. 44, 4392–4399. doi: 10.1016/j.ceramint.2017.12.038
Zhou, Q., Hong, C. X., Yao, Y., Hussain, S., Xu, L. N., Zhang, Q. Y., et al. (2018b). Mingsong Wang, Hierarchically MoS2 nanospheres assembled from nanosheets for superior CO gas-sensing properties. Mater. Res. Bull. 101, 132–139. doi: 10.1016/j.materresbull.2018.01.030
Zhou, Q., Umar, A., Sodki, E. M., Amine, A., Xu, L. N., Gui, Y. G., et al. (2018c). Fabrication and characterization of highly sensitive and selective sensors based on porous NiO nanodisks. Sens. Actuators B Chem. 259, 604–615. doi: 10.1016/j.snb.2017.12.050
Zhou, Q., Xu, L. N., Umar, A., Chen, W. G., and Kumar, R. (2018d). Pt nanoparticles decorated SnO2 nanoneedles for efficient CO gas sensing applications. Sens. Actuators B Chem. 256, 656–664. doi: 10.1016/j.snb.2017.09.206
Keywords: adsorption, Au-doped MoS2 monolayer, DFT calculation, dissolved gases, gas sensors
Citation: Qian G, Peng Q, Zou D, Wang S, Yan B and Zhou Q (2020) First-Principles Insight Into Au-Doped MoS2 for Sensing C2H6 and C2H4. Front. Mater. 7:22. doi: 10.3389/fmats.2020.00022
Received: 18 October 2019; Accepted: 20 January 2020;
Published: 07 February 2020.
Edited by:
Anita Lloyd Spetz, Linköping University, SwedenCopyright © 2020 Qian, Peng, Zou, Wang, Yan and Zhou. This is an open-access article distributed under the terms of the Creative Commons Attribution License (CC BY). The use, distribution or reproduction in other forums is permitted, provided the original author(s) and the copyright owner(s) are credited and that the original publication in this journal is cited, in accordance with accepted academic practice. No use, distribution or reproduction is permitted which does not comply with these terms.
*Correspondence: Qu Zhou, zhouqu@swu.edu.cn