Grazing and Recovery of Kelp Gametophytes Under Ocean Warming
- 1National Marine Science Centre, Faculty of Science and Engineering, Southern Cross University, Coffs Harbour, NSW, Australia
- 2New South Wales (NSW) Department of Primary Industries, National Marine Science Centre, Coffs Harbour, NSW, Australia
Kelp forests are economically important ecosystems that support a wealth of biodiversity but are declining globally. They are often replaced by biologically depauperate alternate stable states dominated by turfing algae. Hysteresis maintains algal turfs by inhibiting kelp recruitment, preventing the reestablishment of kelp forests. The mechanisms inhibiting kelp recruitment remain poorly understood as microscopic stages of kelp are difficult to study in situ. A potential mechanism contributing to the suppression of recruitment may be turf-facilitated grazing of kelp gametophytes, the haploid free-living reproductive life stage. Here we assess the resilience of kelp gametophytes to grazing pressure from a gastropod micrograzer commonly present in turf under current and future ocean warming scenarios. Gametophyte coverage and abundance were significantly reduced following grazing under all temperatures, however there was no significant effect of temperature on grazing rates. Once grazing pressure was removed, gametophyte abundance recovered to control levels, but the total coverage and length of gametophytes continued to decline in all treatments. Gametophytes were found to survive micrograzer ingestion and continued to grow in aggregations in the gastropod’s mucus trail and faeces, even producing sporophytes. Gametophyte survival post-ingestion may positively contribute to dispersal and sporophyte recruitment, however the lack of gametophyte recovery at elevated temperatures may counteract this effect under future ocean warming. Taken together, this study demonstrates complex interactions that take place in the turf micro-habitat of kelp gametophytes and highlights biotic factors influencing transitions between kelp forests and algal turfs.
Introduction
Kelp forests are crucial habitats that are globally recognized as biodiversity hotspots in temperate coastal systems (Steneck et al., 2002). The global decline of kelp forests challenges the conservation of temperate coastal marine environments and threatens the social and economic values associated with kelp ecosystems (Bennett et al., 2016; Krumhansl et al., 2016; Wernberg et al., 2019b). Stressors that cause the collapse of canopy forming kelps often have complex synergistic effects that can be difficult to untangle (Filbee-Dexter et al., 2016; Krumhansl et al., 2016; Provost et al., 2017). Understanding drivers of kelp loss, including the interaction between biotic and abiotic stressors, is a priority to inform future marine conservation and management (Harley et al., 2012; Wood et al., 2019, in press; Veenhof et al., 2022).
Kelp loss is often followed by the establishment of alternative biotic communities, such as turfing algae, which is concurrent with the loss of kelp-associated macrofauna (Filbee-Dexter and Scheibling, 2014; Filbee-Dexter et al., 2016). Turfing algae are defined as short (5 mm – 50 mm) filamentous algae from various taxonomic groups (Connell et al., 2014). Algal turfs can rapidly establish as an alternate stable state, and strong hysteresis often allows them to persist even after the original drivers of kelp loss are removed (Petraitis and Dudgeon, 2004; Burek et al., 2018; Layton et al., 2019; Zarco-Perello et al., 2021). Turfing algae have poor structural diversity, resulting in loss of species that depend on structurally diverse kelp forests (Harley et al., 2012; Coleman and Kennelly, 2019; Harvey et al., 2021; Pessarrodona et al., 2021) and causing a loss of ecological function, economic value (Filbee-Dexter and Wernberg, 2018; Pessarrodona et al., 2021) and net productivity (Copertino et al., 2005; Miller et al., 2009) of coastal marine systems.
A critical step in reversing phase shifts from kelp forests to algal turfs is understanding the mechanisms by which the return to kelp forests is inhibited by turf. Similar to the complexity of initial stressors, the feedback mechanisms responsible for the persistence of alternative stable states can be complex (Petraitis and Dudgeon, 2004). For example, abiotic factors including the chemical environment (Layton et al., 2019), availability of suitable attachment space (Burek et al., 2018) and rates of sedimentation (Alestra et al., 2014) can alter the micro-environment in favour of turfs. Additionally, biotic factors may prevent kelp re-establishment through supressing recruitment of juvenile kelps (O’Brien and Scheibling, 2018; Layton et al., 2019; Zarco-Perello et al., 2021). Kelp recruitment takes place after zoospores settle onto the substratum and germinate into microscopic haploid gametophytes, which grow into male and females that sexually reproduce, fertilise and recruit to sporophytes. (Schiel and Foster, 2006). However, little is known about the progression of gametophytes through these stages in field settings, particularly the ecological processes that influence gametophytes (Veenhof et al., 2022). Thus, the mechanisms involved in the suppression of kelp recruitment by turfing algae remain poorly understood.
Algal turfs are home to diverse grazer communities, including micrograzers such as gastropods (Kelaher et al., 2001; Sala and Graham, 2002). Micrograzers (<2.5 mm) inhabiting algal turfs have been suggested as a potential factor contributing to the strong inhibitory effect turfs have on kelp recruitment (Dayton et al., 1984), yet there remains a paucity of experiential evidence for evaluating this hypothesis. While the impact of grazers on adult plants (Ling et al., 2015; Vergés et al., 2016) and juvenile sporophytes (Sala and Dayton, 2011; Franco et al., 2017) can be severe, there remains a critical knowledge gap surrounding the effect of grazers on kelp gametophytes (Veenhof et al., 2022). Common grazers in both kelp forests and turfing algae include invertebrates such as gastropods (Kelaher, 2002; Christie et al., 2007), which can change ecological interactions within both systems (Underwood, 1980; Vadas et al., 1992; Falkenberg et al., 2014; Miranda et al., 2019). Of the few studies that exist on gastropod grazing of gametophytes, it has been shown that grazer-gametophyte interactions are species-specific (Martinez and Santelices, 1998; Zacher et al., 2016) and can be influenced by interspecific competition between grazers (Henriquez et al., 2011). Gametophytes can also be grazed by echinoids (Santelices et al., 1983; Dean et al., 1984; Leonard, 1994) and can survive ingestion by these grazers (Santelices et al., 1983). However, it is not known what effect grazer ingestion and survival has on gametophyte performance, and if there is any impact on key developmental stages such as gametogenesis or recruitment. Abiotic factors, such as temperature and sedimentation, interact with gastropod grazing to change gametophyte persistence and recruitment success (Zacher et al., 2016). As elevated temperatures can facilitate the expansion of turfing algae (Filbee-Dexter et al., 2016; Feehan et al., 2019; Zarco-Perello et al., 2021), interactions between temperature and grazing of turf-associated herbivores should be considered.
Climate change induced warming is altering ecological interactions in kelp forests (Johnson et al., 2011; Vergés et al., 2014; Wernberg et al., 2016; Qiu et al., 2019; Krause-Jensen et al., 2020). Macro-grazers such as fish and larger invertebrates have been found to maintain turf expansion under elevated temperatures (Falkenberg et al., 2014; Zarco-Perello et al., 2021). Rising temperature can increase the metabolic rates of grazers (O’Connor, 2009; Leung et al., 2021), change the palatability of seaweeds (Poore et al., 2013; Poore et al., 2016; Hargrave et al., 2017) and enhance consumption of sporophytes (Provost et al., 2017; Miranda et al., 2019). Separately, temperature can have strong effects on kelp gametophyte survival and recruitment (Mohring et al., 2014; Muth et al., 2019; Liesner et al., 2020). For example, Zacher et al. (2016) demonstrated that the interaction between biotic (grazing) and abiotic (sedimentation and temperature) factors can have unpredictable and complex effects on gametophyte persistence, where negative consequences of one factor could be counteracted by others. It is, therefore, possible that increasing temperature may increase grazing of gametophytes while interacting with the direct effects of temperature on gametophyte growth and survival.
Here, we assessed the effect of grazing by a common turf associated gastropod micrograzer (Anachis atkinsoni) under ambient and future temperature scenarios on gametophytes of the dominant canopy forming kelp occurring in Australia, Ecklonia radiata. Experimental temperatures were chosen to align with approximate average winter (20°C) and summer ocean temperatures (23°C), as well as the average summer maxima (26°C) currently experienced at the warm edge of the distribution of E. radiata off eastern Australia. Projected future ocean warming of ~2°C throughout the Australian coastal ocean by 2100 indicates that the upper experimental temperature applied here is likely to become more prevalent throughout the distribution of E. radiata (IPCC, 2014). Specifically, we tested 1. the effects of grazing, temperature and their interaction on the abundance, coverage, length, surface area and recovery of E. radiata gametophytes, and 2. how ingestion of gametophytes by grazers influenced survival and recruitment into the sporophyte stage. Taken together, these experiments contribute to our emerging understanding of the ecological interactions that occur when turfing algae replace kelp forests and the effects of ocean warming on the grazing of kelp gametophytes.
Methods
Study Species
Ecklonia radiata is the dominant habitat forming kelp supporting temperate reef ecosystems in Australia (Wernberg et al., 2019a). E. radiata is a foundation species on Australia’s Great Southern Reef, which is a renowned biodiversity hotspot (Bennett et al., 2016) that is currently declining (Wernberg et al., 2013; Vergés et al., 2016). Fertile E. radiata plants (n = 5) were collected from Muttonbird Island (30°18’S 153°08’E) on 11th of November 2020 and returned to the laboratory in a moist calico bag for processing. Fronds were cleaned of epiphytes by gently scraping with a knife and steeped in an iodine/povidone Betadine Antiseptic (Faulding Pharmaceuticals) solution (2% in 1 µm filtered, UV-sterilised seawater (FSW)) for 5 seconds to prevent contamination of cultures (Alsuwaiyan et al., 2019). After drying at 20°C for one hour, visibly fertile tissue was submerged in 2L FSW at 20°C and gently stirred. The number of spores released was counted using a compound microscope and a hemocytometer counting chamber, and the final density was 21 250 spores mL-1 of seawater. Of this spore solution, 20 mL was used to inoculate experimental dishes for experiment 1. For experiment 2, stock cultures of E. radiata gametophytes of mixed origin from the Solitary Islands (Coffs Harbour region, NSW, Australia), which were stored at 20°C in permanent culture and produced in a similar manner, were used.
Micrograzers present in turf surrounding patches of E. radiata were collected from Charlesworth Bay (30°16’S 153°08’E) on 22nd of January 2021 for experiment 1, and on 12th of June 2021 for experiment 2. The gastropod Anachis atkinsoni was selected as the grazer in the experiments as this species commonly occurs in turf habitats along Australia’s east coast. This marine gastropod is endemic to Australia and occurs widely within tropical and temperate systems (Wilson, 1994). For both experiments, individual A. atkinsoni all of similar size were assigned to different temperature treatments (20°C, 23°C and 26°C) and were acclimatized from the collection temperature over a period of 7 days by adjusting temperatures each day until treatment levels were achieved. While acclimatizing, gastropods were fed ad libitum Sargassum spp.
Experiment 1: Grazing and Recovery
A full factorial design was used to test the effect of the presence or absence of micro grazers on gametophytes under three temperatures, resulting in a total of six treatments. Five independent replicates were available for each treatment at each planned sampling time (n = 5), resulting in a total of 150 experimental replicates. To establish experimental gametophyte cultures, petri dishes (n = 150) containing one 20x20 mm coverslip as a surface for settlement were inoculated with 20 mL of the spore solution. Spores were left to settle overnight at 20 °C, after which the media was refreshed with FSW and quarter strength F growth media (AlgaBoostTM 2000×, AusAqua Pty Ltd., Wallaroo, SA, Australia) and Petri dishes were assigned to a treatment temperature of 20°C, 23°C or 26°C (n = 50 dishes per temperature). The 20°C treatment was established via the ambient room temperature, which was controlled by a thermostat, while the 23°C and 26°C treatments were established using replicate heating mats (Lerway 21W Heating Incubator Mat) (n = 3 mats per temperature treatment), which were slowly increased to desired temperatures over a 7-day period. Gametophytes were cultured in the experimental treatments for two months before grazers were added. In this time they germinated, and the gametophytes had grown considerably (average length over all treatments 146 µm). Temperatures were checked daily to ensure stability among treatments (20.4 ± 0.07, 23.2 ± 0.03 and 25.8 ± 0.04, respectively; mean ± SE). Light levels were also measured among experimental replicates daily (36.5 ± 0.8 µmol/photons/second;, mean ± SE) to ensure consistency among treatments and to confirm light levels were within the optimal range (~15-42 µmol/photons/second) for growth of E. radiata gametophytes (Novaczek, 1984a; Mabin et al., 2013). Heating mats and the ambient temperature treatment were interspersed along laboratory benches so there was no systematic bias in light levels or any other factor. Throughout the duration of the experiment, cultures were refreshed every week with FSW and quarter strength F growth media (AlgaBoost™ 2000×, AusAqua Pty Ltd., Wallaroo, SA, Australia).
Gametophytes were sampled before grazing exposure to establish a baseline with which to compare recovery after grazing. This was done by taking three random photographs of coverslips (n = 5) from each treatment at x200 magnification using a MIchrome 20 Color Microscope camera mounted on a stereo microscope (Olympus BX53). Total coverage [total surface area (µm2) of gametophytes per field of view (FOV=3.2mm2)], mean individual length [averaged over 10 individuals (µm)], mean individual surface area [averaged over 10 individuals (µm2)] and abundance (number of individuals per FOV) of gametophytes was quantified using ImageJ 1.53e imaging software and averaged over three photos per replicate. Temperature acclimated snails were then assigned at random to half the gametophyte cultures (n = 1 per dish) and allowed to graze for 48 h after which they were removed, and their length (2.25 mm ± 0.06) and survival were recorded using an MIchrome 20 Color Microscope camera mounted on a dissecting microscope and ImageJ 1.53e imaging software. This grazing time period was sufficient to detect an effect of grazing without risk of all gametophytes being grazed, as determined by a prior pilot study. Immediately after the removal of grazers (i.e day 1 post-grazing) sampling of gametophytes was done using the aforementioned photographic method. To track post-grazing recovery of gametophytes, cultures were maintained for 32 days and sampled 7, 18 and 32 days after grazers were removed. Individual replicates were available for each sampling time, so dishes were never re-sampled. The cultures sampled at day 32 were affected by fouling so were not included in the final analysis of gametophyte recovery post-grazing.
We also tested whether grazing rates of A. atkinsoni differed between temperature treatments independent of gametophyte growth. A. atkinsoni were collected near Charlesworth Bay (n = 30) on 9th of August 2021 and assigned to different temperature treatments (19°C, 23°C and 26°C, n = 10 per temperature). A. atkinsoni were allowed to graze on plates of dental wax (Thompson et al., 1997) for 3 days following acclimatization. Temperature measurements were taken daily to ensure temperature stability (19.2 ± 0.1, 23.2 ± 0.1, 26.0 ± 0.2; mean ± SE). After removal of A. atkinsoni, the total grazed surface area (µm2) of wax plates was measured using ImageJ 1.53e imaging software.
Experiment 2: Survival After Ingestion
To investigate if gametophytes survive ingestion by A. atkinsoni, we assessed regrowth of gametophytes from grazer faecal matter and undigested but highly aggregated gametophytes in grazer mucus. A. atkinsoni were collected near Charlesworth Bay (n = 12) on 12th of June 2021 and acclimated to 19°C for 48 hours in aerated holding tanks. Gametophytes taken from stock cultures kept at 19°C were blended using a hand-held stick blender to small (approximately 5-10 cells) equal fragments and left to settle in 50mL glass beakers (n = 4) with 40 mL FSW and containing 3 coverslips for 48 hours. A. atkinsoni were added to the beakers and left to graze for 5 days, which allowed sufficient faeces to appear. Both faecal pellets (ingested gametophytes, n = 24) and undigested but highly aggregated gametophytes in mucus of A. atkinsoni (n = 12) were taken from the beakers and cultured in separate multi-well plates at 19°C (19.0 ± 0.2 °C and 19.6 ± 0.4 µmol/photons/second, respectively; mean ± SE).
Sampling of faecal matter and clumped gametophytes was performed at 7, 14 and 21 days after subculturing. Individual replicates for each sampling time were available, so that at every timepoint 8 dishes with faecal pellets and 4 dishes with clumped gametophytes were examined. For every piece of faecal matter, it was noted if visible gametophytes and/or sporophytes were present and whether they were dead (unpigmented) or alive (pigmented). The clumped gametophytes were photographed at 3 random fields at x200 magnification using a MIchrome 20 Color Microscope camera mounted on a stereo microscope (Olympus BX53). Total coverage [total surface area of gametophytes per field of view (µm2)] of gametophytes was measured using ImageJ 1.53e imaging software.
Statistical Analysis
Presumably due to the small volume of culture media in dishes, approximately 50% of grazers died just before the grazing period (48H) had concluded. However, we made sure that grazing did occur in each dish and photos that did not contain faeces, a grazing trail or evidence that the grazer did not graze prior to removal or death were removed from the dataset to represent the effects of grazing as accurately as possible. This resulted the loss of 31 experimental replicates.
Two-factor analyses of variance (ANOVA) were used to test for an effect of temperature and grazing on the total coverage, average individual length, average individual surface area and abundance of gametophytes at the conclusion of the grazing period. A single-factor ANOVA was used to test for an effect of temperature on the size of A. atkinsoni grazing marks on wax plates. A three-factor ANOVA was used to test for an effect of grazing, temperature and time post-grazing on the recovery of gametophytes. Individual experimental replicates were used at every sampling time, allowing the inclusion of ‘time’ as an independent explanatory variable. Response variables analysed to assess gametophyte recovery were total coverage, average individual length, average individual surface area and abundance of gametophytes quantified from day 1 to day 18. Baseline data were used to compare gametophyte recovery. A single-factor ANOVA was used to test for an effect of time on the coverage of clumped gametophytes following A. atkinsoni grazing. All ANOVAs utilised type III sums of squares as appropriate for unbalanced data (Shaw and Mitchell-Olds, 1993; Queen et al., 2002), which resulted from grazer mortalities.
The assumption of homogeneity of variance was assessed using a Levene’s test. Normality of data was assessed visually using QQ-plots and tested using a Shapiro-Wilk test. Gametophyte abundance, coverage, length and surface area were log-transformation prior to analysis on data from experiment 1 in order to not violate the assumption of normality. Significance was set at alpha level 0.05 and pair-wise comparisons were undertaken when significant fixed effects were detected. Data were analysed and plotted using the software R (R Core Team, 2020).
Results
Effects of Grazing and Temperature
The presence of grazers significantly reduced the total coverage of gametophytes (µm2) after the 48-hour grazing period (F1,23 = 5.97, p=0.02), but there was no effect of temperature on the cover of gametophytes (F2,23 = 1.96, p=0.16) and no interaction between grazers and temperature during this 48-hour period (F2,23 = 1.32, p=0.29, Figures 1A; Table S1). Similarly, the presence of grazers significantly reduced the abundance of gametophytes (F1,23 = 7.1, p=0.01) after a 48-hour grazing period but there was no effect of temperature on abundance (F2,23 = 0.5, p=0.61) nor an interaction between grazing and temperature (F2,23 = 0.10, p=0.91; Figures 1B; Table S1). There was no effect of grazing or temperature on mean individual length or mean individual surface area of gametophytes (Table S1). Temperature did not have a significant effect on the average surface area of A. atkinsoni grazing marks in dental wax (F2,27 = 0.24, p=0.79).
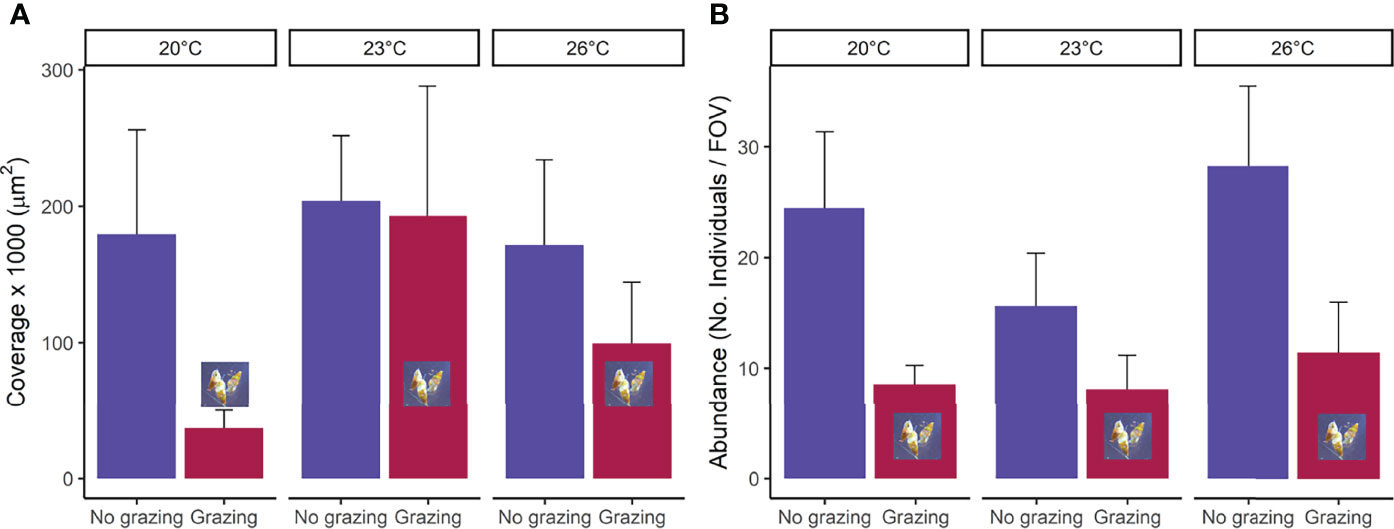
Figure 1 (A) mean total coverage per field of view (FOV=3.2mm2) and (B) abundance (No. of individuals per FOV) of Ecklonia radiata gametophytes in the presence (grazed) and absence (non-grazed) of Anachis atkinsoni at three temperatures (20°C, 23°C, 26°C) directly after removal of the grazer (48H). Error bars represent SE, n = 5.
Recovery After Grazing
The presence of grazers significantly reduced the total coverage of gametophytes (F1,71 = 13.74, p<0.01, Figure 2 and Tables 1, S2). The total coverage of gametophytes was significantly reduced at 18 days relative to day 1 and day 7 (F2.71 = 4.66, p=0.01, pair-wise test among time points; Figure 2 and Tables 1, S2). Moreover, total gametophyte coverage was also significantly lower at 26°C than at 23°C, while no difference was found in coverage between 20°C and the other temperature treatments (F2,71 = 3.56, p=0.03, pair-wise test among temperatures; Figure 2 and Tables 1, S2). While there were no interactions (F4,71 = 0.51, p=0.73) between these factors (Figure 2 and Tables 1, S2), gametophyte coverage decreased considerably over time in the grazed treatments at 23°C and 26°C. There was a final decrease in coverage of 73% and 83% for the grazed treatments, respectively, compared to 42% and 60% in non-grazed treatments (Fig 2.). Additionally, after 18 days only the non-grazed treatment at 20°C saw gametophyte coverage recover to control conditions (Figure 2).
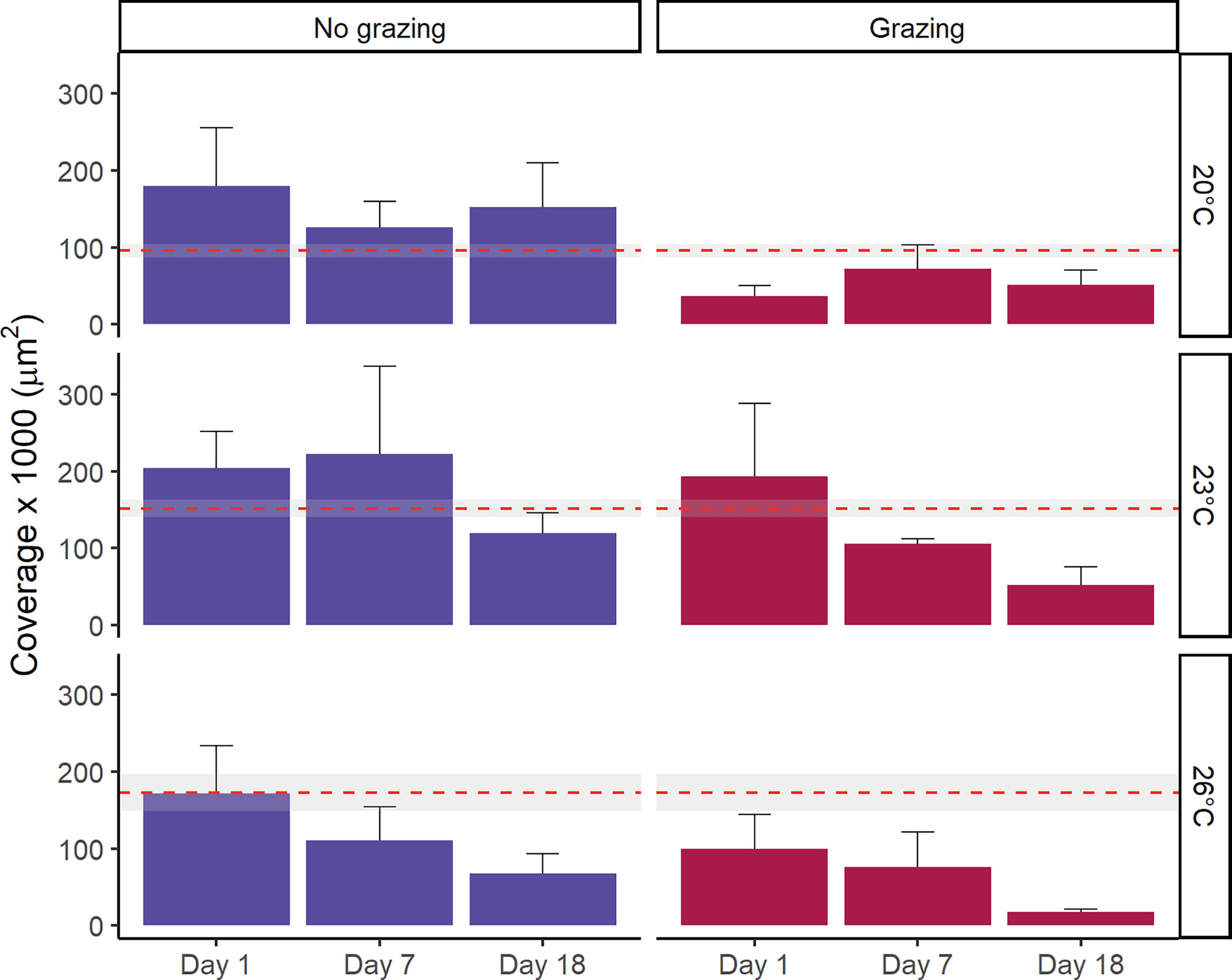
Figure 2 Mean total coverage of Ecklonia radiata gametophytes (µm2) at three temperatures (20 °C, 23°C and 26°C) tracked over an 18-day period after exposure to grazing. Error bars represent SE (n = 5). The red dashed line represents the mean coverage of each temperature treatment prior to grazer exposure (i.e. baseline coverage data), with the grey area denoting the 95% confidence interval.
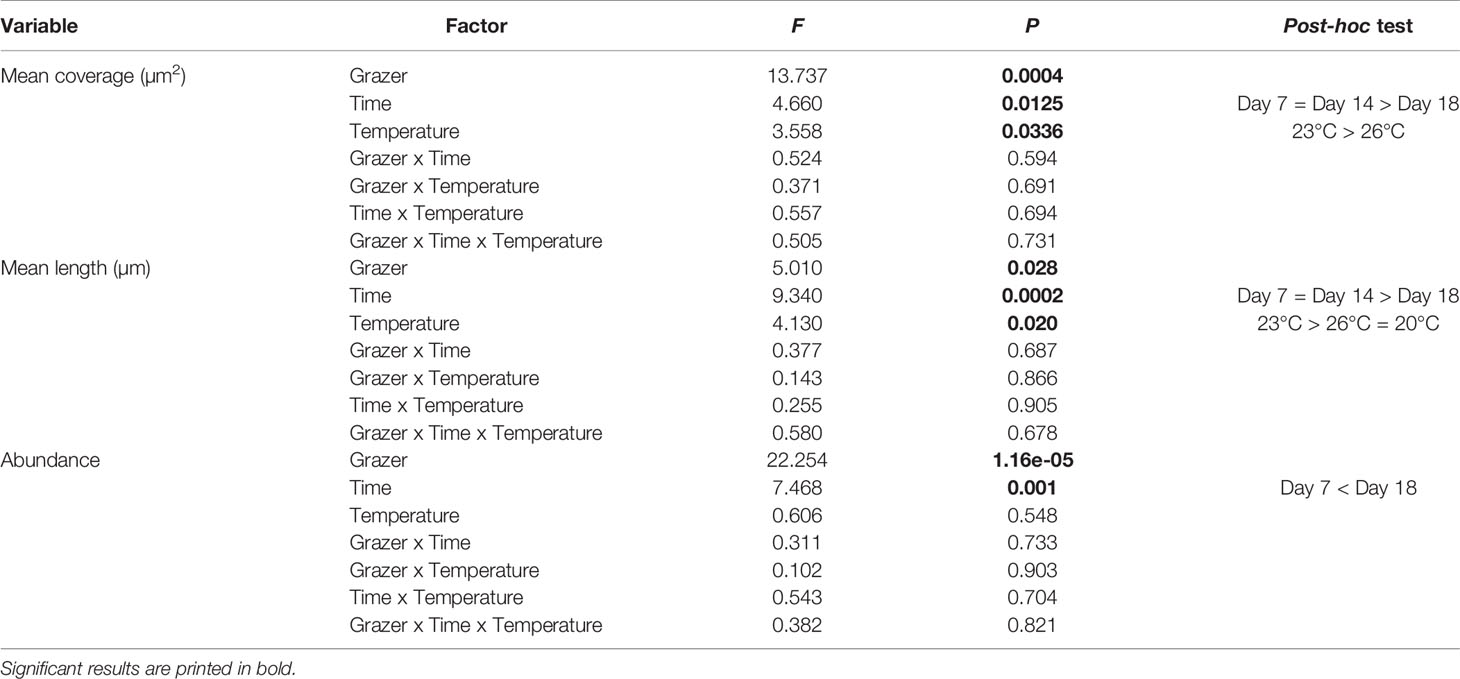
Table 1 Summary table of 3-factor ANOVA of total mean coverage of gametophytes (µm2), mean length (µm) and abundance with grazing, time, and temperature as fixed factors.
Exposure to grazers significantly reduced the mean individual length of gametophytes (F1,71 = 5.01, p=0.03, Figure 3 and Tables 1, S2). The mean length of gametophytes was significantly smaller at 18 days, while they were similar size at day 1 and day 7 (F2,71 = 9.34, p<0.01, post-hoc test among time; Figure 3 and Tables 1, S2). The mean length was also lower at 26°C and 20°C than 23°C (F2,71 = 4.13, p=0.02, pair-wise test among temperature; Figure 3 and Tables 1, S2). While there were no significant interactions between these factors on gametophyte length (F4,71 = 0.58, p=0.68), the decrease in length appeared steeper in grazed compared to non-grazed treatments. This was particularly apparent at 23°C and 26°C, which were associated with declines in gametophyte length of 51% and 70% in the grazed treatments, respectively (Figure 3), compared to 26% and 44% in non-grazed treatments. Additionally, both the grazed and non-grazed treatments did not recover to control levels (Figure 3), with length decreasing in all treatments during the post-grazing period.
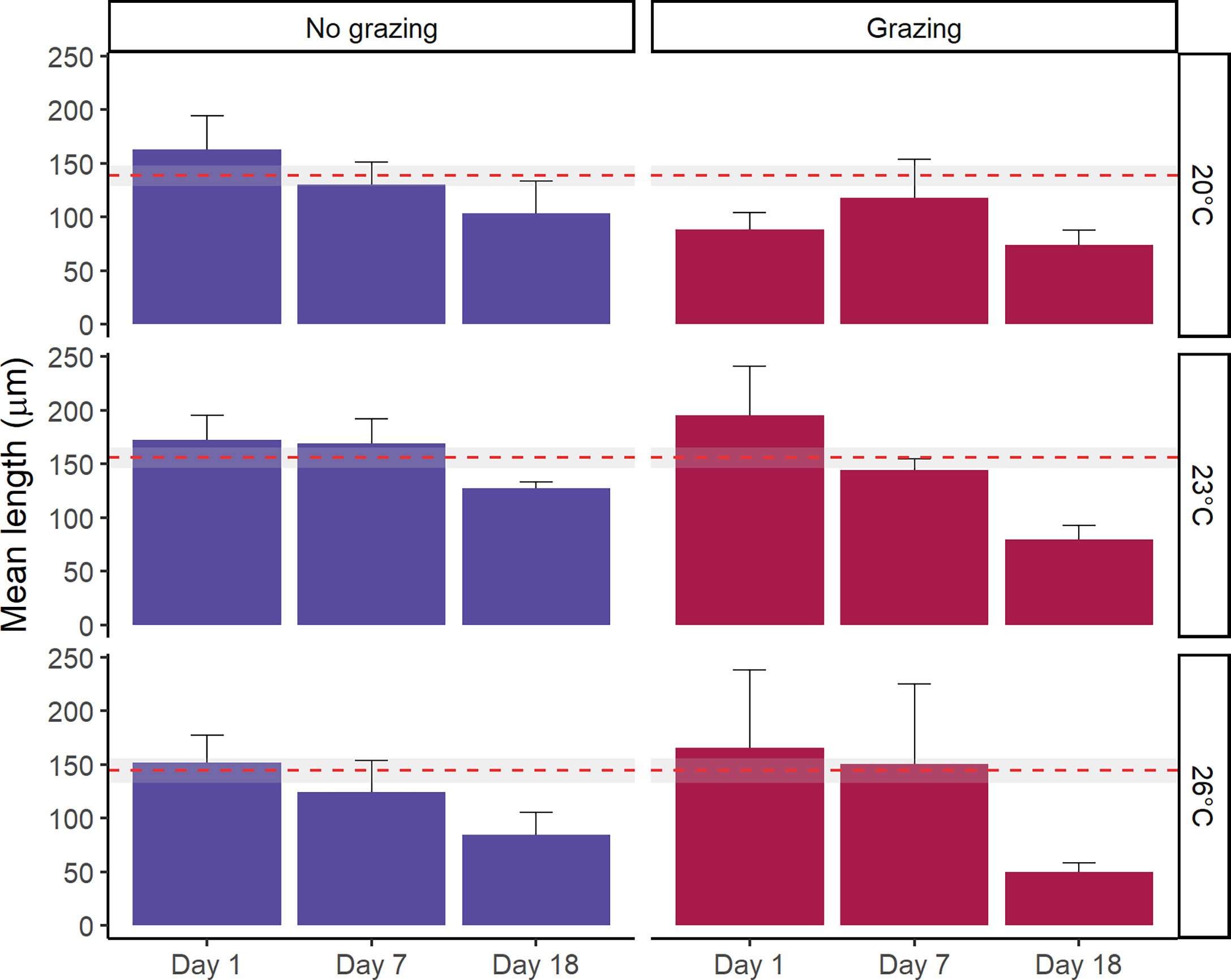
Figure 3 Mean length of Ecklonia radiata gametophytes (µm) at three temperatures (20°C, 23°C and 26°C) tracked over an 18-day period after exposure to grazing. Error bars represent SE, n = 5. The red dashed line represents the mean length of each temperature treatment prior to grazer exposure (i.e. baseline length data), with the grey area denoting the 95% confidence interval.
The presence of grazers significantly reduced the abundance of gametophytes (F1,71 = 22.25, p<0.01). Moreover, the mean abundance of gametophytes at 18 days was significantly greater than abundance at 7 days (F2,71 = 7.47, p<0.01, pair-wise test among time; Figure 4 and Table 1, S2). However, temperature did not have a significant effect on gametophyte abundance (F2,71 = 0.61, p=0.55) and there were no interactions (F2,71 = 0.38, p=0.82) among factors (Figure 4 and Table 1, S2).
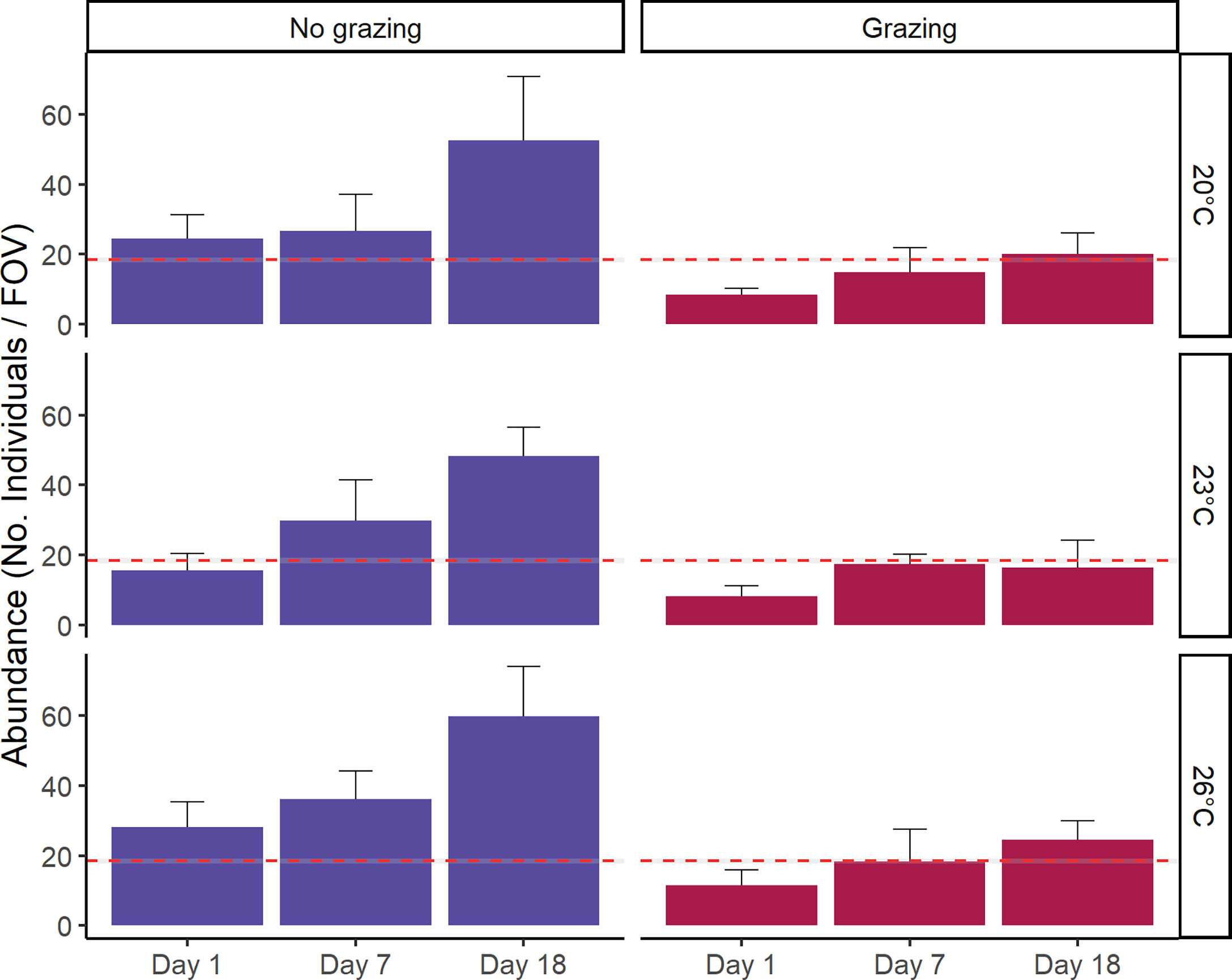
Figure 4 Mean abundance [No. of individuals per field of view (FOV) (3.2mm2)] after grazing tracked over an 18-day period after grazing. Separate bar plots are shown for grazer treatment. Error bars represent SE, n = 5. The red dashed line represents the mean abundance prior to grazer exposure (baseline data), with the grey area denoting the 95% confidence interval.
Gametophyte abundance increased by just over 50% from day 1 to day 18. The abundance of gametophytes recovered to be greater than control levels in both grazed and non-grazed treatments (Figure 4). As no extra spores were added to the dishes, the increased abundance can either come from the growth of gametophyte fragments too small to notice in earlier counts or the fragmentation by grazers.
Survival After Ingestion
Live (pigmented) gametophytes were repeatedly found in A. atkinsoni faecal matter, with the proportion of total faecal matter containing live gametophytes increasing during 21 days of culturing (Figure 5). Throughout the 21-day culturing period, dead (unpigmented) gametophytes were present in 50% of faecal matter. However, live gametophyte appeared in 13% of faecal matter on day 7, which increased to 40% at subsequent dates. Live gametophytes were identified as full filaments (Figure 6B) as well as spores (Figure 6C) in faecal pellets of A. atkinsoni.
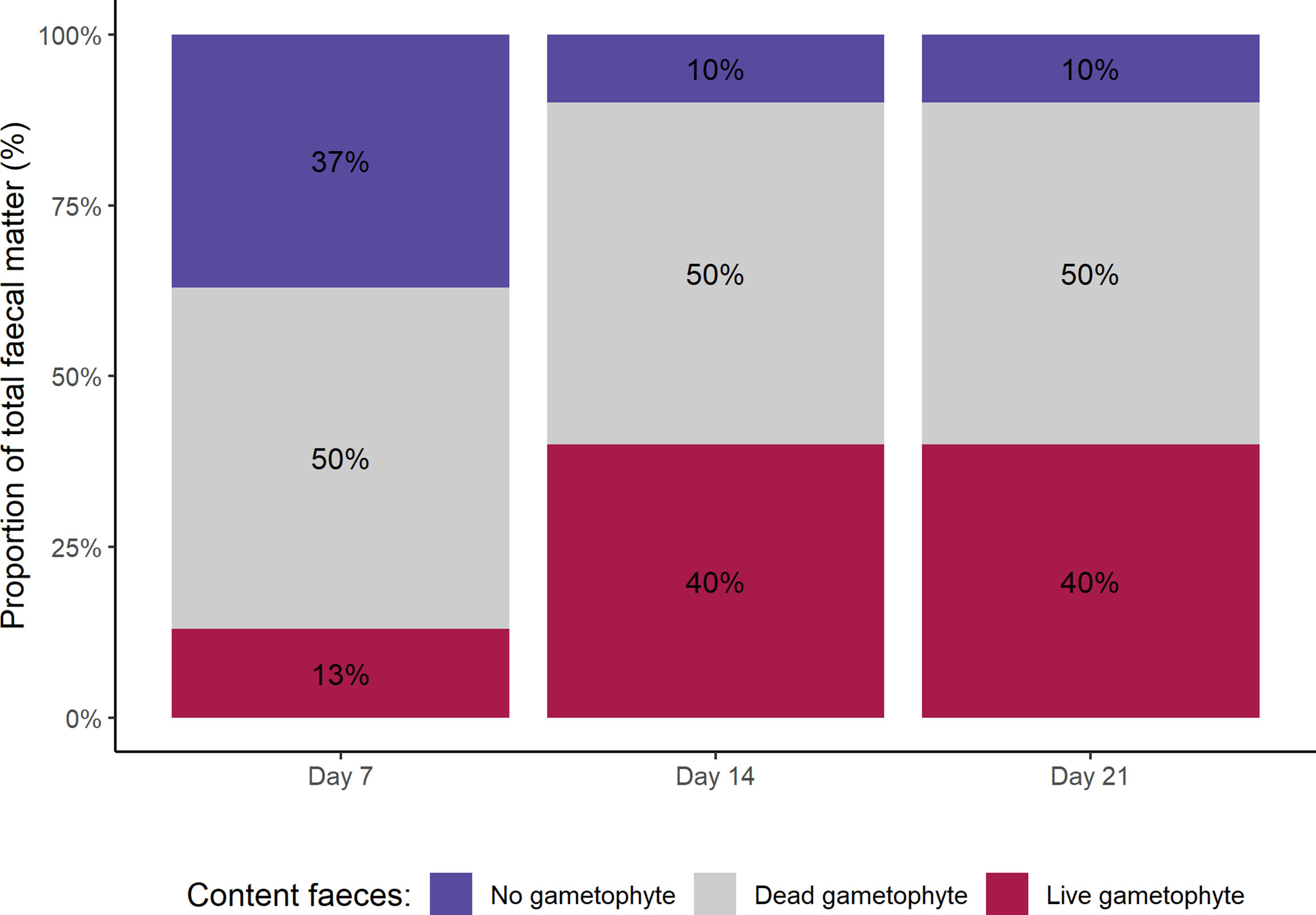
Figure 5 Content of A. atkinsoni faecal matter after 7, 14 and 21 days of culturing faecal pellets. Separate faecal pellets (n = 10) were examined at each time point and were categorised as containing no gametophyte, a dead gametophyte (i.e. no pigmentation) or a live gametophyte (i.e. pigments present).
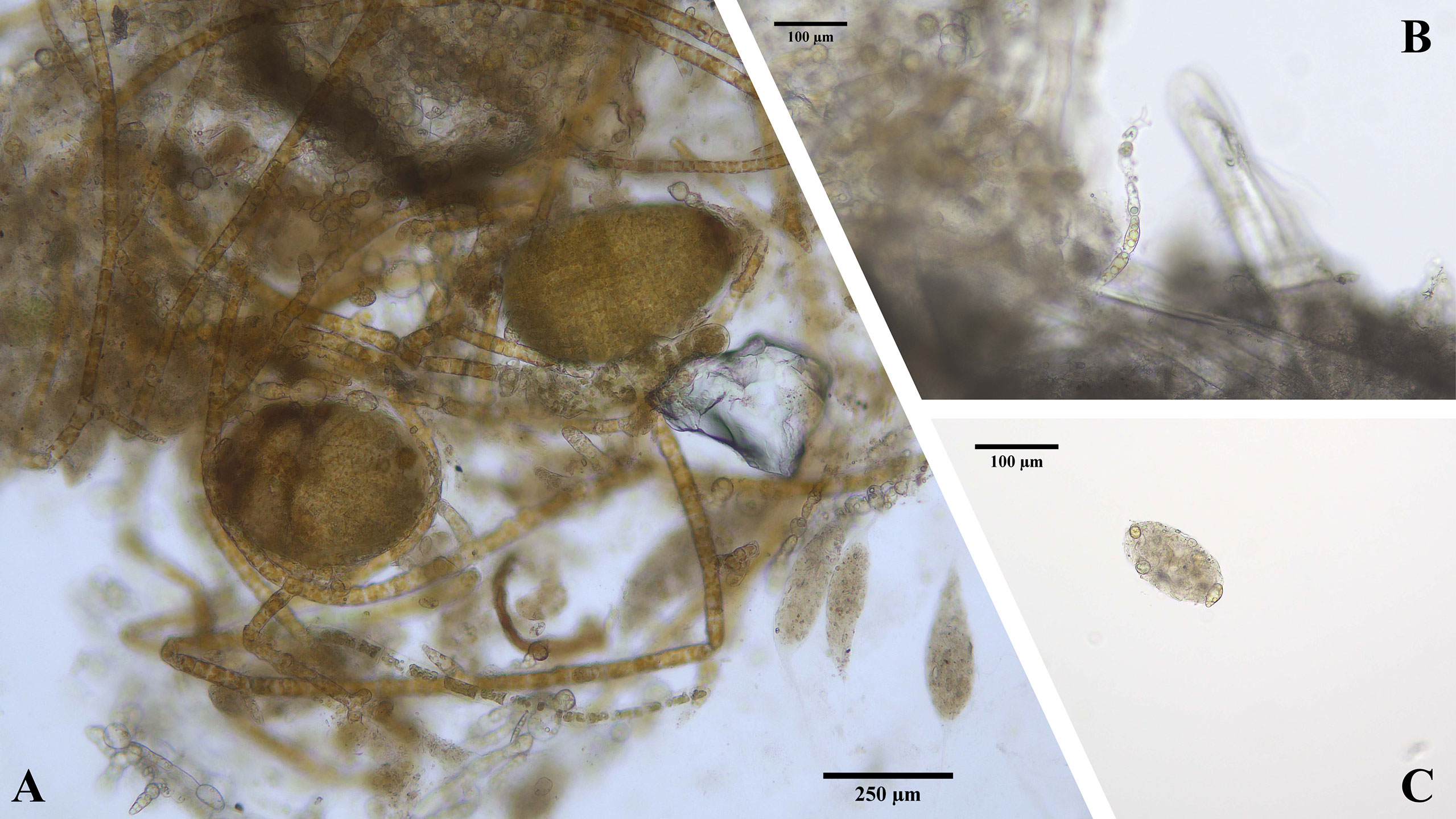
Figure 6 Different stages of E. radiata microscopic stages in faecal matter of the herbivore A. atkinsoni. (A) Juvenile sporophytes growing in aggregated clumps of gametophytes and faecal matter. (B) Filament of gametophyte growing out of faecal matter. (C) E. radiata spores in faecal pellet.
No significant difference was found in the coverage of clumped undigested gametophytes over time (F2,9 = 0.99, p=0.41), signifying gametophytes remained aggregated in A. atkinsoni mucus over a 3-week period post-grazing. In one replicate of clumped gametophytes, juvenile sporophytes appeared after 21 days, while these never appeared in experimental treatments in experiment 1 (Figure 6A).
Discussion
The replacement of kelp forests by turf seascapes is a pressing threat to kelp persistence and recovery (Filbee-Dexter and Wernberg, 2018). Turf cover is thought to be maintained through supressed recruitment of kelp microscopic stages (O’Brien and Scheibling, 2018) which could be mediated through grazing pressure within turfs. We investigated the resilience of kelp gametophytes to grazing and ingestion by a turf-associated grazer under current and future ocean warming scenarios. We found that a common turf-associated micrograzer reduced E. radiata gametophyte cover, length, and abundance. Similarly, higher temperatures negatively impacted gametophytes. However, there was no interactive effect of temperature on grazing. Interestingly, E. radiata gametophytes were found to be resilient to ingestion and may benefit from aggregation that occurs within faeces and mucus trails of gastropods as the only sporophytes seen in these experiments occurred in faecal pellets. Together, these results suggest complex interactions of grazing within algal turfs.
Impacts of Micrograzers on Gametophytes
Turfing algal expansion is thought to be facilitated by suppressed recruitment of kelp microscopic stages (O’Brien and Scheibling, 2018), and micrograzers present in turfs may contribute to this continued inhibition by consuming kelp gametophytes (Dayton et al., 1984). We found that A. atkinsoni, a turf associated grazer, significantly reduced both the abundance and coverage of E. radiata gametophytes in laboratory experiments after 48H. Presumably in natural settings when grazers continually graze gametophytes within turf, this impact may be much larger. These results provide novel evidence that a gastropod micrograzer (<2.5 mm) commonly present in turfing algae can impact the microscopic stages of a kelp gametophyte and could inhibit kelp recruitment and transitions from turf to kelp forest. Studies on such trophic interactions of gametophytes are sparse (Veenhof et al., 2022), but those that exist also suggest that gastropods do graze on microscopic stages of kelp. For example, the limpet Margarites helicinus was found to graze gametophytes of kelps Alaria escuelenta, Laminaria digitata and Saccharina latissima, which could result in reduced sporophyte recruitment (Zacher et al., 2016). Similarly, Macrocystis pyrifera gametophytes were grazed by two species of gastropod, reducing their abundance (Henriquez et al., 2011). While studies on micrograzers are not common, meso-grazers have been shown to impact macro algae (Hay et al., 1987). For instance, selective grazing of meso-grazers can decrease growth rates and biomass in kelps (Poore et al., 2014; Gutow et al., 2020) and facilitate spore release in red seaweed (Buschmann and Santelices, 1987). In addition, juvenile stages of meso- and other larger grazers such as sea urchins and gastropods could fulfil the same ecological role, but this remains untested.
Trophic interactions between algae and grazers can be impacted by increases in temperature consistent with projected levels of ocean warming (Provost et al., 2017; Christie et al., 2019; Miranda et al., 2019). Increases in temperature have been found to both enhance grazing rates (e.g. Miranda et al., 2019) and inhibit grazing (e.g. Provost et al., 2017), and population models show that herbivore abundances can be unaffected by increased temperatures (O’Connor et al., 2011). Our temperature treatments did not influence grazing by A. atkinsoni as indicated by no difference in grazing on dental wax and no interactions between temperature and grazing on gametophyte abundance and coverage. This is most likely due to the fact that experimental temperatures fell within the range of temperatures A. atkinsoni naturally experiences throughout its wide distribution, which spans tropical to temperate habitats (Wilson, 1994). Given the suite of micrograzers present in turfing algae, increasing temperatures may differentially affect grazing rates among different species present, specifically those with narrower distributions. To understand how ocean warming and grazing rates may influence kelp gametophyte persistence within turfs, a larger range or even entire communities of micrograzers need to be explored.
Interactions with abiotic factors other than temperature can also change the outcomes of ecological interactions and may be at play within turfs. For example, the clearing of sediment by grazers has been found to enhance gametophyte survival (Zacher et al., 2016), which may indicate that micrograzers can counteract negatives effects of accumulated sediment in turfs on gametophyte recruitment. Conversely, the physical structure of turfing algae may shelter gametophytes from grazers (Harris et al., 1984; Camus, 1994) and counteract negative impacts of micro-grazing on survival of gametophytes. These interactions can thus change the impact of micrograzers alone on gametophyte survival in turfs. While these factors were not taken into account in this study, the finding that grazers present in turf can reduce gametophyte cover and abundance demonstrates a biological mechanism that may contribute to the strong hysteresis observed when turf cover is maintained over kelp forests.
Post-Grazing Recovery and Temperature
The ability of gametophytes to recover post-grazing differed depending on which variable was measured. Abundance increased steadily post-grazing, while coverage and length decreased over time. While abundance was lower overall in grazed compared to non-grazed treatments, it increased over time to recover to eventually exceed initial abundances at the start of the experiment. This could signify the continual germination of spores that were too small to record at earlier sampling times. These spores could have germinated and grown larger over time and were noted as small gametophytes at later sampling points, also accounting for the reduction in the length of gametophytes over time. Alternatively, grazing may have fragmented gametophytes creating a higher abundance of smaller individuals. Given that an increase in abundance was seen in both grazed and non-grazed treatments the former hypothesis is more likely. In natural systems, filamentous gametophytes may persist on the benthos for 3 to 7 months, as a bank of microscopic growth forms that can recruit into adults when conditions are suitable (Carney et al., 2013; Akita et al., 2019; Schoenrock et al., 2020; Veenhof et al. 2022, in press). In culture, spores and gametophytes can remain dormant in a vegetative state for decades (Veenhof et al., 2022; Barrento et al., 2016, in press). While it is not known how long such seed banks can persist, it is possible that there exists a bank of diverse ages and sizes of spores and gametophytes that could withstand periodic grazing pressure.
While there was no significant effect of temperature on post-grazing recovery, we did detect an overall temperature effect on gametophyte length and coverage, which is consistent with the major influence temperature has on general gametophyte growth and survival (Veenhof et al., 2022; tom Dieck, 1993, in press). Specifically, gametophyte cultures at 23°C had greater length and coverage than cultures at 20°C and 26°C, irrespective of grazing. Ocean temperatures of approximately 26°C are representative of the upper thermal limits for populations of E. radiata, and growth is optimal between 20 and 23°C for Western Australian E. radiata populations (Novaczek, 1984b; Mohring et al., 2013; Mohring et al., 2014; Alsuwaiyan et al., in review). In situ temperature has been found to correlate with temperature tolerance of E. radiata gametophytes (Mohring et al., 2014) and our results are consistent with this result. The E. radiata cultured here is from sporophytes inhabiting the warmest edge at the tropical-temperature transition zone off eastern Australia where average annual temperatures are 23°C. As ocean warming is projected to continue, however, E. radiata populations will come under considerable temperature stress especially at the warm, equatorward edge of their distribution (Martínez et al., 2018; Davis et al., 2021), where temperatures often exceed 26°C.
Survival of Ingested and Grazed Gametophytes May Enhance Sporophyte Recruitment
Our results reveal that E. radiata gametophytes can survive consumption by the common turf grazer A. atkinsoni. This is consistent with previous studies on other kelp species. For instance, spores of the kelp Lessonia nigrescens were found to survive ingestion by the urchin Tetrapygus niger (Santelices et al., 1983) and could grow following cultivation of faecal pellets. Similarly, microscopic stages of algae were found in the gut content of several molluscs and were able to grow from fragmented pieces and spores in faecal pellets (Santelices and Correa, 1985). In culture, gametophytes that are shredded have the ability to regrow quickly and become sexually mature and this is a commonly used culture technique. Passing through the digestive track of grazers might thus increase biomass through shredding and regrowth, which in turn can increase abundance. In addition, the aggregation of gametophytes in the mucus trails of A. atkinsoni appeared to benefit stage transitions. Aggregated gametophytes in mucus appeared to be healthy and even produced the only juvenile sporophytes observed in this study. How this may translate into field settings is unknown but an increased density of Lessonia berteroana juvenile sporophytes was seen in patches where herbivores were present (Oróstica et al., 2014). Additionally, Henriquez et al. (2011) found recruitment pulses of M. pyrifera after annual die-back of adult canopy despite a high presence of limpets, and Zacher et al. (2016) found an increased number of microscopic sporophytes in some of their experimentally grazed treatments. While both these studies hypothesized that cleared substrate by grazers give the gametophytes a chance to recruit, we suggest that the ingestion and aggregation by gastropod grazing might also contribute to the observed recruitment pulses. Fertilization in seaweeds and kelps can only take place if the male and female gametophytes are in close enough proximity (Reed, 1990) which can be influenced by water motion, substrate rugosity and other environmental factors (Amsler et al., 1992). We hypothesize that some of these barriers to fertilization could be overcome by aggregation of gametophytes in mucus trails of gastropod grazers. While the densities of spores, gametophytes and adult plants necessary for recruitment has been researched extensively (e.g. Reed et al., 1991; Graham, 2003; Layton et al., 2020), these models do not consider the possibility of aggregation via grazing. The relative contribution of grazers to kelp recruitment is not known but based on our results could prove an integral component in recruitment models. For instance, if gametophyte density is low due to turf expansion or limited spore settlement and germination in turfs, micrograzers such as A. atkinsoni could increase the chances of successful recruitment through shredding and dispersal, allowing gametophytes to increase their biomass, as well as increasing chances of fertilization by aggregating gametophytes in their mucus trail. In this way, micrograzers may serve a similar ecological role to pollinators in land plants. Certainly, both large and small herbivores can influence the patterns of recruitment post-disturbance (Oróstica et al., 2014), indicating they can shape recovery of kelp forests.
In summary, micrograzers can have both positive (dispersal, increased fertilization, and sporophyte recruitment) and negative (reduced coverage and abundance after grazing) impacts on gametophytes highlighting the complexity of trophic interactions in turf-kelp dynamics. Negative consequences of grazing were expected as herbivory by grazers such as fish and larger gastropods is known to maintain turf cover, particularly at species range edges (Bennett et al., 2015; Zarco-Perello et al., 2021). Moreover, the lack of recovery in gametophyte coverage post-grazing is consistent with global increases in turf cover (Filbee-Dexter and Wernberg, 2018; Feehan et al., 2019; Pessarrodona et al., 2021). Higher temperatures consistent with future warming also decreased gametophyte coverage, but had no impact on grazing rates, suggesting that E. radiata gametophytes may be increasingly challenged under climate change. However, the survival of gametophytes and possible enhanced recruitment of sporophytes following grazer ingestion may indicate beneficial effects of grazing. This highlights the complexity of ecological interactions that take place on a microscale. Gastropod micrograzers are both high in abundance and biomass and thus likely to influence kelps through grazing, and while their influence is poorly understood (Barnes, 2019) they may represent a potential underexplored driver of change in kelp-turf dynamics.
Data Availability Statement
The raw data supporting the conclusions of this article will be made available by the authors, without undue reservation.
Author Contributions
RV, SD, and MC designed the study. RV performed the experimental work. RV and CC analysed the data. The writing of the manuscript was led by RV, and all authors contributed to reviewing and revising the final manuscript.
Funding
This work contributes to the NSW Primary Industries Climate Change Research Strategy, funded by the NSW Climate Change Fund. Funding was also provided by Australian Research Council grant DP190100058 awarded to MC. Funding was also provided by the Southern Cross University Higher Degree Research Scholarship. This work is supported by the Holsworth Wildlife Research Endowment, awarded to RV.
Conflict of Interest
The authors declare that the research was conducted in the absence of any commercial or financial relationships that could be construed as a potential conflict of interest.
Publisher’s Note
All claims expressed in this article are solely those of the authors and do not necessarily represent those of their affiliated organizations, or those of the publisher, the editors and the reviewers. Any product that may be evaluated in this article, or claim that may be made by its manufacturer, is not guaranteed or endorsed by the publisher.
Acknowledgments
The authors would like to thank Dr. M.J. Nimbs for his help with identifying A. atkinsoni and M. Tan for fieldwork support.
Supplementary Material
The Supplementary Material for this article can be found online at: https://www.frontiersin.org/articles/10.3389/fmars.2022.866136/full#supplementary-material
References
Akita S., Takano Y., Nagai S., Kuwahara H., Kajihara R., Tanabe A. S., et al. (2019). Rapid Detection of Macroalgal Seed Bank on Cobbles: Application of DNA Metabarcoding Using Next-Generation Sequencing. J. Appl. Phycol 31 (4), 2743–2753. doi: 10.1007/s10811-018-1730-9
Alestra T., Tait L. W., Schiel D. R. (2014). Effects of Algal Turfs and Sediment Accumulation on Replenishment and Primary Productivity of Fucoid Assemblages. Mar Ecol. Prog. Ser. 511, 59–70. doi: 10.3354/meps10932
Alsuwaiyan N. A., Mohring M. B., Cambridge M., Coleman M. A., Kendrick G. A., Wernberg T. (2019). A Review of Protocols for the Experimental Release of Kelp (Laminariales) Zoospores. Ecol. Evol. 9 (14), 8387–8398. doi: 10.1002/ece3.5389
Amsler C. D., Reed D. C., Neushul M. (1992). The Microclimate Inhabited by Macroalgal Propagules. Br. Phycol J. 27 (3), 253–270. doi: 10.1080/00071619200650251
Barnes R. S. K. (2019). Spatial Structure of a Multi-Species Guild: The Dominant Biofilm-Grazing Microgastropods of Seagrass. Hydrobiologia 827 (1), 293–307. doi: 10.1007/s10750-018-3781-y
Barrento S., Camus C., Sousa-Pinto I., Buschmann A. H. (2016). Germplasm Banking of the Giant Kelp: Our Biological Insurance in a Changing Environment. Algal Res. 13, 134–140. doi: 10.1016/j.algal.2015.11.024
Bennett S., Wernberg T., Connell S. D., Hobday A. J., Johnson C. R., Poloczanska E. S. (2016). The ‘Great Southern Reef’: Social, Ecological and Economic Value of Australia’s Neglected Kelp Forests. Mar Freshwater Res. 67 (1), 47–56. doi: 10.1071/MF15232
Bennett S., Wernberg T., Harvey E. S., Santana-Garcon J., Saunders B. J. (2015). Tropical Herbivores Provide Resilience to a Climate-Mediated Phase Shift on Temperate Reefs. Ecol. Lett. 18 (7), 714–723. doi: 10.1111/ele.12450
Burek K. E., O’Brien J. M., Scheibling R. E. (2018). Wasted Effort: Recruitment and Persistence of Kelp on Algal Turf. Mar Ecol. Prog. Ser. 600, 3–19. doi: 10.3354/meps12677
Buschmann A., Santelices B. (1987). Micrograzers and Spore Release in Iridaea Laminarioides Bory (Rhodophyta: Gigartinales). J. Exp. Mar Biol. Ecol. 108 (2), 171–179. doi: 10.1016/S0022-0981(87)80021-7
Camus P. A. (1994). Recruitment of the Intertidal Kelp Lessonia Nigrescens Bory in Northern Chile: Successional Constraints and Opportunities. J. Exp. Mar Biol. Ecol. 184 (2), 171–181. doi: 10.1016/0022-0981(94)90003-5
Carney L. T., Bohonak A. J., Edwards M. S., Alberto F. (2013). Genetic and Experimental Evidence for a Mixed-Age, Mixed-Origin Bank of Kelp Microscopic Stages in Southern California. Ecology 94 (9), 1955–1965. doi: 10.1890/13-0250.1
Christie H., Gundersen H., Rinde E., Filbee-Dexter K., Norderhaug K. M., Pedersen T., et al. (2019). Can Multitrophic Interactions and Ocean Warming Influence Large-Scale Kelp Recovery? Ecol. Evol. 9 (5), 2847–2862. doi: 10.1002/ece3.4963
Christie H., Jørgensen N. M., Norderhaug K. M. (2007). Bushy or Smooth, High or Low; Importance of Habitat Architecture and Vertical Position for Distribution of Fauna on Kelp. J. Sea Res. 58 (3), 198–208. doi: 10.1016/j.seares.2007.03.006
Coleman M. A., Kennelly S. J. (2019). Microscopic Assemblages in Kelp Forests and Urchin Barrens. Aquat. Bot. 154, 66–71. doi: 10.1016/j.aquabot.2019.01.005
Connell S. D., Foster M. S., Airoldi L. (2014). What are Algal Turfs? Towards a Better Description of Turfs. Mar Ecol. Prog. Ser. 495, 299–307. doi: 10.3354/meps10513
Copertino M., Connell S. D., Cheshire A. (2005). The Prevalence and Production of Turf-Forming Algae on a Temperate Subtidal Coast. Phycologia 44 (3), 241–248. doi: 10.2216/0031-8884(2005)44[241:Tpapot]2.0.Co;2
Davis T. R., Champion C., Coleman M. A. (2021). Climate Refugia for Kelp Within an Ocean Warming Hotspot Revealed by Stacked Species Distribution Modelling. Mar Environ. Res. 166, 105267. doi: 10.1016/j.marenvres.2021.105267
Dayton P. K., Currie V., Gerrodette T., Keller B. D., Rosenthal R., Tresca D. V. (1984). Patch Dynamics and Stability of Some California Kelp Communities. Ecol. Monogr. 54 (3), 253–289. doi: 10.2307/1942498
Dean T. A., Schroeter S. C., Dixon J. D. (1984). Effects of Grazing by Two Species of Sea Urchins (Strongylocentrotus Franciscanus and Lytechinus Anamesus) on Recruitment and Survival of Two Species of Kelp (Macrocystis Pyrifera and Pterygophora Californica). Mar Biol. 78 (3), 301–313. doi: 10.1007/BF00393016
Falkenberg L. J., Connell S. D., Russell B. D. (2014). Herbivory Mediates the Expansion of an Algal Habitat Under Nutrient and CO2 Enrichment. Mar Ecol. Prog. Ser. 497, 87–92. doi: 10.3354/meps10557
Feehan C. J., Grace S. P., Narvaez C. A. (2019). Ecological Feedbacks Stabilize a Turf-Dominated Ecosystem at the Southern Extent of Kelp Forests in the Northwest Atlantic. Sci. Rep. 9 (1), 7078. doi: 10.1038/s41598-019-43536-5
Filbee-Dexter K., Feehan C. J., Scheibling R. E. (2016). Large-Scale Degradation of a Kelp Ecosystem in an Ocean Warming Hotspot. Mar Ecol. Prog. Ser. 543, 141–152. doi: 10.3354/meps11554
Filbee-Dexter K., Scheibling R. E. (2014). Sea Urchin Barrens as Alternative Stable States of Collapsed Kelp Ecosystems. Mar Ecol. Prog. Ser. 495, 1–25. doi: 10.3354/meps10573
Filbee-Dexter K., Wernberg T. (2018). Rise of Turfs: A New Battlefront for Globally Declining Kelp Forests. BioScience 68 (2), 64–76. doi: 10.1093/biosci/bix147
Franco J. N., Wernberg T., Bertocci I., Jacinto D., Maranhão P., Pereira T., et al. (2017). Modulation of Different Kelp Life Stages by Herbivory: Compensatory Growth Versus Population Decimation. Mar Biol. 164 (8), 164. doi: 10.1007/s00227-017-3196-8
Graham M. H. (2003). Coupling Propagule Output to Supply at the Edge and Interior of a Giant Kelp Forest. Ecology 84 (5), 1250–1264. doi: 10.1890/0012-9658(2003)084[1250:Cpotsa]2.0.Co;2
Gutow L., Poore A. G. B., Díaz Poblete M. A., Villalobos V., Thiel M. (2020). Small Burrowing Amphipods Cause Major Damage in a Large Kelp. Proc. R. Soc. B: Biol. Sci. 287 (1926), 20200330. doi: 10.1098/rspb.2020.0330
Hargrave M. S., Foggo A., Pessarrodona A., Smale D. A. (2017). The Effects of Warming on the Ecophysiology of Two Co-Existing Kelp Species With Contrasting Distributions. Oecologia 183 (2), 531–543. doi: 10.1007/s00442-016-3776-1
Harley C. D. G., Anderson K. M., Demes K. W., Jorve J. P., Kordas R. L., Coyle T. A., et al. (2012). Effects of Climate Change on Global Seaweed Communities. J. Phycol 48 (5), 1064–1078. doi: 10.1111/j.1529-8817.2012.01224.x
Harris L. G., Ebeling A. W., Laur D. R., Rowley R. J. (1984). Community Recovery After Storm Damage: A Case of Facilitation in Primary Succession. Science 224 (4655), 1336–1338. doi: 10.1126/science.224.4655.1336
Harvey B. P., Kon K., Agostini S., Wada S., Hall-Spencer J. M. (2021). Ocean Acidification Locks Algal Communities in a Species-Poor Early Successional Stage. Global Change Biol. 27 (10), 2174–2187. doi: 10.1111/gcb.15455
Hay M. E., Duffy J. E., Pfister C. A., Fenical W. (1987). Chemical Defense Against Different Marine Herbivores: Are Amphipods Insect Equivalents? Ecology 68 (6), 1567–1580. doi: 10.2307/1939849
Henriquez L. A., Buschmann A. H., Maldonado M. A., Graham M. H., Hernandez-Gonzalez M. C., Pereda S. V., et al. (2011). Grazing on Giant Kelp Microscopic Phases and the Recruitment Success of Annual Populations of Macrocystis Pyrifera (Laminariales, Phaeophyta) in Southern Chile. J. Phycol 47 (2), 252–258. doi: 10.1111/j.1529-8817.2010.00955.x
IPCC (2014). Climate Change 2014: Synthesis Report. Contribution of Working Groups I, II and III to the Fifth Assessment Report of the Intergovernmental Panel on Climate Change (Geneva, Switzerland: IPCC).
Johnson C. R., Banks S. C., Barrett N. S., Cazassus F., Dunstan P. K., Edgar G. J., et al. (2011). Climate Change Cascades: Shifts in Oceanography, Species’ Ranges and Subtidal Marine Community Dynamics in Eastern Tasmania. J. Exp. Mar Biol. Ecol. 400 (1), 17–32. doi: 10.1016/j.jembe.2011.02.032
Kelaher P. B. (2002). Influence of Physical Characteristics of Coralline Turf on Associated Macrofaunal Assemblages. Mar Ecol. Prog. Ser. 232, 141–148. doi: 10.3354/meps232141
Kelaher B. P., Chapman M. G., Underwood A. J. (2001). Spatial Patterns of Diverse Macrofaunal Assemblages in Coralline Turf and Their Association With Environmental Variables. J. Mar Biol. Assoc. United Kingdom 81, 291–307. doi: 10.1017/S0025315401004842
Krause-Jensen D., Archambault P., Assis J., Bartsch I., Bischof K., Filbee-Dexter K., et al. (2020). Imprint of Climate Change on Pan-Arctic Marine Vegetation. Front. Mar Sci. 7 (1129). doi: 10.3389/fmars.2020.617324
Krumhansl K. A., Okamoto D. K., Rassweiler A., Novak M., Bolton J. J., Cavanaugh K. C., et al. (2016). Global Patterns of Kelp Forest Change Over the Past Half-Century. Proc. Natl. Acad. Sci. 113 (48), 13785–13790. doi: 10.1073/pnas.1606102113
Layton C., Cameron M. J., Shelamoff V., Fernandez P. A., Britton D., Hurd C. L., et al. (2019). Chemical Microenvironments Within Macroalgal Assemblages: Implications for the Inhibition of Kelp Recruitment by Turf Algae. Limnol Oceanogr 64 (4), 1600–1613. doi: 10.1002/lno.11138
Layton C., Cameron M. J., Tatsumi M., Shelamoff V., Wright J. T., Johnson C. R. (2020). Habitat Fragmentation Causes Collapse of Kelp Recruitment. Mar Ecol. Prog. Ser. 648, 111–123. doi: 10.3354/meps13422
Leonard G. H. (1994). Effect of the Bat Star Asterina Miniata (Brandt) on Recruitment of the Giant Kelp Macrocystis Pyrifera C. Agardh. J. Exp. Mar Biol. Ecol. 179 (1), 81–98. doi: 10.1016/0022-0981(94)90018-3
Leung J. Y. S., Russell B. D., Coleman M. A., Kelaher B. P., Connell S. D. (2021). Long-Term Thermal Acclimation Drives Adaptive Physiological Adjustments of a Marine Gastropod to Reduce Sensitivity to Climate Change. Sci. Total Environ. 771, 145208. doi: 10.1016/j.scitotenv.2021.145208
Liesner D., Shama L. N., Diehl N., Valentin K., Bartsch I. (2020). Thermal Plasticity of the Kelp Laminaria Digitata (Phaeophyceae) Across Life Cycle Stages Reveals the Importance of Cold Seasons for Marine Forests. Front. Mar Sci. 7, 456. doi: 10.3389/fmars.2020.00456
Ling S. D., Scheibling R. E., Rassweiler A., Johnson C. R., Shears N., Connell S. D., et al. (2015). Global Regime Shift Dynamics of Catastrophic Sea Urchin Overgrazing. Philos. Trans. R. Soc. B-Biol Sci. 370 (1659), 10. doi: 10.1098/rstb.2013.0269
Mabin C. J., Gribben P. E., Fischer A., Wright J. T. (2013). Variation in the Morphology, Reproduction and Development of the Habitat-Forming Kelp Ecklonia Radiata With Changing Temperature and Nutrients. Mar Ecol. Prog. Ser. 483, 117–131. doi: 10.3354/meps10261
Martínez B., Radford B., Thomsen M. S., Connell S. D., Carreño F., Bradshaw C. J., et al. (2018). Distribution Models Predict Large Contractions of Habitat-Forming Seaweeds in Response to Ocean Warming. Diversity Distrib 24 (10), 1350–1366. doi: 10.1111/ddi.12767
Martinez E. A., Santelices B. (1998). Selective Mortality on Haploid and Diploid Microscopic Stages of Lessonia Nigrescens Bory (Phaeophyta, Laminariales). J. Exp. Mar Biol. Ecol. 229 (2), 219–239. doi: 10.1016/S0022-0981(98)00063-X
Miller R. J., Reed D. C., Brzezinski M. A. (2009). Community Structure and Productivity of Subtidal Turf and Foliose Algal Assemblages. Mar Ecol. Prog. Ser. 388, 1–11. doi: 10.3354/meps08131
Miranda R. J., Coleman M. A., Tagliafico A., Rangel M. S., Mamo L. T., Barros F., et al. (2019). Invasion-Mediated Effects on Marine Trophic Interactions in a Changing Climate: Positive Feedbacks Favour Kelp Persistence. Proc. R. Soc. B: Biol. Sci. 286 (1899), 20182866. doi: 10.1098/rspb.2018.2866
Mohring M. B., Kendrick G. A., Wernberg T., Rule M. J., Vanderklift M. A. (2013). Environmental Influences on Kelp Performance Across the Reproductive Period: An Ecological Trade-Off Between Gametophyte Survival and Growth? PloS One 8 (6), e65310. doi: 10.1371/journal.pone.0065310
Mohring M. B., Wernberg T., Wright J. T., Connell S. D., Russell B. D. (2014). Biogeographic Variation in Temperature Drives Performance of Kelp Gametophytes During Warming. Mar Ecol. Prog. Ser. 513, 85–96. doi: 10.3354/meps10916
Muth A. F., Graham M. H., Lane C. E., Harley C. D. G. (2019). Recruitment Tolerance to Increased Temperature Present Across Multiple Kelp Clades. Ecology 100 (3), e02594. doi: 10.1002/ecy.2594
Novaczek I. (1984a). Response of Ecklonia Radiata (Laminariales) to Light at 15°C With Reference to the Field Light Budget at Goat Island Bay, New Zealand. Mar Biol. 80 (3), 263–272. doi: 10.1007/BF00392821
Novaczek I. (1984b). Response of Gametophytes of Ecklonia Radiata (Laminariales) to Temperature in Saturating Light. Mar Biol. 82 (3), 241–245. doi: 10.1007/Bf00392405
O’Brien J. M., Scheibling R. E. (2018). Turf Wars: Competition Between Foundation and Turf-Forming Species on Temperate and Tropical Reefs and its Role in Regime Shifts. Mar Ecol. Prog. Ser. 590, 1–17. doi: 10.3354/meps12530
O’Connor M. I. (2009). Warming Strengthens an Herbivore–Plant Interaction. Ecology 90 (2), 388–398. doi: 10.1890/08-0034.1
O’Connor M. I., Gilbert B., Brown C. J. (2011). Theoretical Predictions for How Temperature Affects the Dynamics of Interacting Herbivores and Plants. Am. Nat. 178 (5), 626–638. doi: 10.1086/662171
Oróstica M. H., Aguilera M. A., Donoso G. A., Vásquez J. A., Broitman B. R. (2014). Effect of Grazing on Distribution and Recovery of Harvested Stands of Lessonia Berteroana Kelp in Northern Chile. Mar Ecol. Prog. Ser. 511, 71–82. doi: 10.3354/meps10931
Pessarrodona A., Filbee-Dexter K., Alcoverro T., Boada J., Feehan C., Fredriksen S., et al. (2021). Homogenization and Miniaturization of Habitat Structure in Temperate Marine Forests. Global Change Biol. 00, 1–14. doi: 10.1111/gcb.15759
Petraitis P. S., Dudgeon S. R. (2004). Detection of Alternative Stable States in Marine Communities. J. Exp. Mar Biol. Ecol. 300 (1), 343–371. doi: 10.1016/j.jembe.2003.12.026
Poore A. G. B., Graba-Landry A., Favret M., Sheppard Brennand H., Byrne M., Dworjanyn S. A. (2013). Direct and Indirect Effects of Ocean Acidification and Warming on a Marine Plant–Herbivore Interaction. Oecologia 173 (3), 1113–1124. doi: 10.1007/s00442-013-2683-y
Poore A. G. B., Graham S. E., Byrne M., Dworjanyn S. A. (2016). Effects of Ocean Warming and Lowered pH on Algal Growth and Palatability to a Grazing Gastropod. Mar Biol. 163 (5), 99. doi: 10.1007/s00227-016-2878-y
Poore A. G., Gutow L., Pantoja J. F., Tala F., Madariaga D. J., Thiel M. (2014). Major Consequences of Minor Damage: Impacts of Small Grazers on Fast-Growing Kelps. Oecologia 174 (3), 789–801. doi: 10.1007/s00442-013-2795-4
Provost E. J., Kelaher B. P., Dworjanyn S. A., Russell B. D., Connell S. D., Ghedini G., et al. (2017). Climate-Driven Disparities Among Ecological Interactions Threaten Kelp Forest Persistence. Global Change Biol. 23 (1), 353–361. doi: 10.1111/gcb.13414
Qiu Z., Coleman M. A., Provost E., Campbell A. H., Kelaher B. P., Dalton S. J., et al. (2019). Future Climate Change is Predicted to Affect the Microbiome and Condition of Habitat-Forming Kelp. Proc. R. Soc. B 286 (1896), 20181887. doi: 10.1098/rspb.2018.1887
Queen J. P., Quinn G. P., Keough M. J. (2002). Experimental Design and Data Analysis for Biologists (Cambridge: university press).
R Core Team. (2020). "R: A Language and Environment for Statistical Computing" (Vienna, Austria: R Foundation for Statistical Computing).
Reed D. C. (1990). The Effects of Variable Settlement and Early Competition on Patterns of Kelp Recruitment. Ecology 71 (2), 776–787. doi: 10.2307/1940329
Reed D. C., Neushul M., Ebeling A. W. (1991). Role of Settlement Density on Gametophyte Growth and Reproduction in the Kelps Pterygophora Californica and Macrocystis Pyrifera (Phaeophyceae). J. Phycol 27 (3), 361–366. doi: 10.1111/j.0022-3646.1991.00361.x
Sala E., Dayton P. K. (2011). Predicting Strong Community Impacts Using Experimental Estimates of Per Capita Interaction Strength: Benthic Herbivores and Giant Kelp Recruitment. Mar Ecol-an Evol Perspect 32 (3), 300–312. doi: 10.1111/j.1439-0485.2011.00471.x
Sala E., Graham M. H. (2002). Community-Wide Distribution of Predator-Prey Interaction Strength in Kelp Forests. Proc. Natl. Acad. Sci. United States America 99 (6), 3678–3683. doi: 10.1073/pnas.052028499
Santelices B., Correa J. (1985). Differential Survival of Macroalgae to Digestion by Intertidal Herbivore Molluscs. J. Exp. Mar Biol. Ecol. 88 (2), 183–191. doi: 10.1016/0022-0981(85)90037-1
Santelices B., Correa J., Avila M. (1983). Benthic Algal Spores Surviving Digestion by Sea Urchins. J. Exp. Mar Biol. Ecol. 70 (3), 263–269. doi: 10.1016/0022-0981(83)90093-X
Schiel D. R., Foster M. S. (2006). The Population Biology of Large Brown Seaweeds: Ecological Consequences of Multiphase Life Histories in Dynamic Coastal Environments. Annu. Rev. Ecol. Evol. Syst 37, 343–372. doi: 10.1146/annurev.ecolsys.37.091305.110251
Schoenrock K. M., McHugh T. A., Krueger-Hadfield S. A. (2020). Revisiting the ‘Bank of Microscopic Forms’ in Macroalgal-Dominated Ecosystems. J. Phycol. 14–29. doi: 10.1111/jpy.13092
Shaw R. G., Mitchell-Olds T. (1993). ANOVA for Unbalanced Data: An Overview. J. Ecol. 74 (6), 1638–1645. doi: 10.2307/1939922
Steneck R. S., Graham M. H., Bourque B. J., Corbett D., Erlandson J. M., Estes J. A., et al. (2002). Kelp Forest Ecosystems: Biodiversity, Stability, Resilience and Future. Environ. Conserv. 29 (4), 436–459. doi: 10.1017/S0376892902000322
Thompson R. C., Johnson L. E., Hawkins S. J. (1997). A Method for Spatial and Temporal Assessment of Gastropod Grazing Intensity in the Field: The Use of Radula Scrapes on Wax Surfaces. J. Exp. Mar Biol. Ecol. 218 (1), 63–76. doi: 10.1016/S0022-0981(97)00068-3
tom Dieck I. (1993). Temperature Tolerance and Survival in Darkness of Kelp Gametophytes (Laminariales, Phaeophyta) - Ecological and Biogeographical Implications. Mar Ecol. Prog. Ser. 100 (3), 253–264. doi: 10.3354/meps100253
Underwood A. J. (1980). The Effects of Grazing by Gastropods and Physical Factors on the Upper Limits of Distribution of Intertidal Macroalgae. Oecologia 46 (2), 201–213. doi: 10.1007/BF00540127
Vadas R. L., Johnson S., Norton T. A. (1992). Recruitment and Mortality of Early Post-Settlement Stages of Benthic Algae. Br. Phycol J. 27 (3), 331–351. doi: 10.1080/00071619200650291
Veenhof R. J., Champion C., Dworjanyn S. A., Wernberg T., Minne A. J. P., Layton C., et al. (2022). Kelp Gametophytes in Changing Oceans. Oceanogr. Mar. Biol. Annu. Rev. 60.
Vergés A., Doropoulos C., Malcolm H. A., Skye M., Garcia-Pizá M., Marzinelli E. M., et al. (2016). Long-Term Empirical Evidence of Ocean Warming Leading to Tropicalization of Fish Communities, Increased Herbivory, and Loss of Kelp. Proc. Natl. Acad. Sci. 113 (48), 13791–13796. doi: 10.1073/pnas.1610725113
Vergés A., Steinberg P. D., Hay M. E., Poore A. G. B., Campbell A. H., Ballesteros E., et al. (2014). The Tropicalization of Temperate Marine Ecosystems: Climate-Mediated Changes in Herbivory and Community Phase Shifts. Proc. R. Soc. B 281 (1789), 20140846. doi: 10.1098/rspb.2014.0846
Wernberg T., Bennett S., Babcock R. C., de Bettignies T., Cure K., Depczynski M., et al. (2016). Climate-Driven Regime Shift of a Temperate Marine Ecosystem. Science 353 (6295), 169–172. doi: 10.1126/science.aad8745
Wernberg T., Coleman M. A., Babcock R. C., Bell S. Y., Bolton J. J., Connell S. D., et al. (2019a). Biology and Ecology of the Globally Significant Kelp Ecklonia Radiata. Oceanogr Mar Biol: Annu. Rev. 57, 265–324. doi: 10.1201/9780429026379-6
Wernberg T., Krumhansl K., Filbee-Dexter K., Pedersen M. F. (2019b). Status and Trends for the World’s Kelp Forests World Seas: An Environmental Evaluation. Ed. Sheppard C. (London, UK: Elsevier), 57–78.
Wernberg T., Smale D. A., Tuya F., Thomsen M. S., Langlois T. J., de Bettignies T., et al. (2013). An Extreme Climatic Event Alters Marine Ecosystem Structure in a Global Biodiversity Hotspot. Nat. Climate Change 3 (1), 78–82. doi: 10.1038/nclimate1627
Wood G., Marzinelli E. M., Coleman M. A., Campbell A. H., Santini N. S., Kajlich L., et al. (2019). Restoring Subtidal Marine Macrophytes in the Anthropocene: Trajectories and Future-Proofing. Mar Freshwater Res. 70 (7), 936–951. doi: 10.1071/MF18226
Zacher K., Bernard M., Bartsch I., Wiencke C. (2016). Survival of Early Life History Stages of Arctic Kelps (Kongsfjorden, Svalbard) Under Multifactorial Global Change Scenarios. Polar Biol. 39 (11), 2009–2020. doi: 10.1007/s00300-016-1906-1
Keywords: climate change, gametophyte, grazing, kelp, turfing algae
Citation: Veenhof RJ, Dworjanyn SA, Champion C and Coleman MA (2022) Grazing and Recovery of Kelp Gametophytes Under Ocean Warming. Front. Mar. Sci. 9:866136. doi: 10.3389/fmars.2022.866136
Received: 30 January 2022; Accepted: 28 March 2022;
Published: 20 April 2022.
Edited by:
Susana Carvalho, King Abdullah University of Science and Technology, Saudi ArabiaReviewed by:
Matthew Edwards, San Diego State University, United StatesFrancisco Arenas, University of Porto, Portugal
Gustavo Martins, Universidade dos Açores, Portugal
Copyright © 2022 Veenhof, Dworjanyn, Champion and Coleman. This is an open-access article distributed under the terms of the Creative Commons Attribution License (CC BY). The use, distribution or reproduction in other forums is permitted, provided the original author(s) and the copyright owner(s) are credited and that the original publication in this journal is cited, in accordance with accepted academic practice. No use, distribution or reproduction is permitted which does not comply with these terms.
*Correspondence: Reina J. Veenhof, reinaveenhof@gmail.com