An Experimental Framework for Selectively Breeding Corals for Assisted Evolution
- 1School of Natural and Environmental Sciences, Newcastle University, Newcastle upon Tyne, United Kingdom
- 2Omaha’s Henry Doorly Zoo and Aquarium, Omaha, NE, United States
- 3Horniman Museum and Gardens, London, United Kingdom
- 4Palau International Coral Reef Center, Koror, Palau
- 5Department of Biology, Loyola University Maryland, Baltimore, MD, United States
- 6Aquatic Research Facility, Environmental Sustainability Research Centre, University of Derby, Derby, United Kingdom
Coral cover on tropical reefs has declined during the last three decades due to the combined effects of climate change, destructive fishing, pollution, and land use change. Drastic reductions in greenhouse gas emissions combined with effective coastal management and conservation strategies are essential to slow this decline. Innovative approaches, such as selective breeding for adaptive traits combined with large-scale sexual propagation, are being developed with the aim of pre-adapting reefs to increased ocean warming. However, there are still major gaps in our understanding of the technical and methodological constraints to producing corals for such restoration interventions. Here we propose a framework for selectively breeding corals and rearing them from eggs to 2.5-year old colonies using the coral Acropora digitifera as a model species. We present methods for choosing colonies for selective crossing, enhancing early survivorship in ex situ and in situ nurseries, and outplanting and monitoring colonies on natal reefs. We used a short-term (7-day) temperature stress assay to select parental colonies based on heat tolerance of excised branches. From six parental colonies, we produced 12 distinct crosses, and compared survivorship and growth of colonies transferred to in situ nurseries or outplanted to the reef at different ages. We demonstrate that selectively breeding and rearing coral colonies is technically feasible at small scales and could be upscaled as part of restorative assisted evolution initiatives. Nonetheless, there are still challenges to overcome before selective breeding can be implemented as a viable conservation tool, especially at the post-settlement and outplanting phases. Although interdisciplinary approaches will be needed to overcome many of the challenges identified in this study, selective breeding has the potential to be a viable tool within a reef managers toolbox to support the persistence of selected reefs in the face of climate change.
Introduction
The Anthropocene, the era in which humans have become a global geophysical force, is characterized by the degradation of ecosystem structure and function, loss of biodiversity and increased rates of species extinction (Steffen et al., 2007; Ceballos et al., 2015). Unfortunately, many existing conservation practices that are based on local management are inadequate in the face of global scale stressors such as those caused by climate change (Lennon, 2015). Coral reefs are among the ecosystems most impacted by human activities and climate change (Hoegh-Guldberg et al., 2019), leading to more rapid increases in extinction risk for many coral species compared to mammals, birds, and amphibians (Bongaarts, 2019). During the last 30 years coral cover worldwide has decreased by an estimated 20% (Hoegh-Guldberg et al., 2019), and four pan-tropical coral bleaching events since 1983 have led to coral declines on hundreds of reefs (Lough et al., 2018). Catastrophic coral bleaching and mortality driven by high sea temperatures occurred throughout Australia’s Great Barrier Reef Marine Park, between 2015 and 2017, highlighting the limitations of localized management of reef fisheries and water quality (Hughes et al., 2017). The present rate of reef degradation emphasizes the urgent need to develop innovative conservation approaches that can maintain ecosystem services and ecological function despite projected sea warming owing to climate change.
As a result of anthropogenic climate change, the frequency, duration, and intensity of marine heat waves increased more than 20-fold between 1981 to 2017 (Laufkötter et al., 2020). Global mean sea surface temperature is projected to reach 1.5°C above that in pre-industrial times between 2030 and 2052, suggesting that shallow water corals have ∼10–30 years to adapt to this temperature increase (Hoegh-Guldberg et al., 2019). For many coral species this period will be too short for adaptation to happen by natural selection, given the sporadic nature of heatwaves at local scales (Bay et al., 2017). Even if warming can be limited to <1.5°C, it is highly likely that large areas will be experiencing regular mass bleaching events, threatening 70–90% of reefs by 2050 (Hoegh-Guldberg et al., 2018). In addition to tackling climate change, traditional conservation efforts will likely need to be coupled with restoration to assist recovery from disturbances (Anthony et al., 2017). Innovative solutions for actively assisting coral populations to pre-adapt to climate change via assisted evolution have been proposed to be included in management strategies for coral reefs (van Oppen et al., 2015). The goal of assisted evolution is to deliberately enhance certain traits in selected organisms, increasing their chances of surviving in the face of global change (Jones and Monaco, 2009). Such practices may involve induced acclimatization, modification of the microbial or the Symbiodiniaceae symbiont communities, and selective breeding (SB) for adaptive traits.
Selective breeding is the process by which humans choose individuals with specific heritable phenotypic traits to breed together and produce offspring. Humans have practiced SB for centuries to improve the production and taste of crops and livestock (Denison et al., 2003). More recently, such practices have been used to select for traits that might be beneficial in a changing climate such as drought resistance in plants (Hu and Xiong, 2014). SB can also be used as a conservation method for preserving populations of endangered species, however, there are only a few examples where this has been considered as a management strategy (Jones et al., 2007; Aitken and Bemmels, 2016). In marine invertebrates, SB has been used primarily in mollusk aquaculture to improve their growth (Hollenbeck and Johnston, 2018), protein content (Gjedrem et al., 2012), and disease resistance (Parker et al., 2012), highlighting that this approach can be adapted for calcifying organisms. To successfully conduct SB, adult colonies with adaptive, heritable traits (i.e., heat tolerance, growth rate, reproductive output, etc.) need to be selected as broodstock. Selecting for heat tolerance in corals is one of the approaches proposed in assisted evolution initiatives, given the importance of this trait in climate adaptation. There is evidence for heritability of heat tolerance at different life stages in some coral species under laboratory conditions (Csaszar et al., 2010; Dixon et al., 2015; Kenkel et al., 2015; Kirk et al., 2018; Quigley et al., 2020; Yetsko et al., 2020), suggesting that selective crosses between colonies with known tolerances could produce offspring with above-average heat resistance. Broodstock colonies can come from different populations exposed to contrasting temperatures profiles at a range of spatial scales (Dixon et al., 2015; Liew et al., 2020; McClanahan et al., 2020), or among individuals from within a single population where there is sufficient intrapopulation variability. While it is well established that coral populations experiencing higher mean sea surface temperatures or more variable temperatures tend to be more tolerant to heat stress (Howells et al., 2012; Thomas et al., 2018), less is known about the extent of within-population variation in heat tolerance (Bay and Palumbi, 2014; van Oppen et al., 2018). However, if sufficient variability does exist, then this approach has the advantage of reducing the likelihood of maladaptation to environmental variables other than temperature (Cotto et al., 2019) and may reduce the risk of inadvertently selecting different genetic variants or sub-species (however see Gomez-Corrales and Prada, 2020).
For assisted evolution methods to be successfully incorporated into resilience adaptation programs, they will need to be combined with techniques to restore and rehabilitate coral reefs (van Oppen et al., 2017). Some of the techniques associated with assisted evolution will rely on successful coral larval propagation (CLP) via sexual reproduction. For the purpose of this article, we define CLP as the process of producing and rearing corals from eggs through to colonies that are recruited into the population. We define a recruited colony as one that has been transplanted to the reef, has self-attached (sensu Guest et al., 2011), and contributes to the emergent properties of the population (growth, survivorship, and/or reproduction rates). CLP is an emerging method for producing large numbers of corals for reef rehabilitation and restoration, that overcomes early survivorship bottlenecks via a combination of land (ex situ) or ocean (in situ) based nurseries for rearing the early life stages. Several advances have been made to improve the practices associated with CLP in recent years. The modes of sexual reproduction for approximately half of extant species of hermatypic scleractinians have been identified (Baird et al., 2009) and the timing of spawning cataloged for >300 Indo-Pacific coral species (Baird et al., 2021). CLP has been successfully executed in different geographical regions with several species under ex situ and in situ conditions (Omori, 2019; Randall et al., 2020) with sexually propagated colonies that were outplanted to the reef reaching sexual maturity (Nakamura et al., 2011; Baria et al., 2012; Guest et al., 2014; Chamberland et al., 2016). Competent coral larvae have been seeded en masse onto natural substrates to enhance recruitment (dela Cruz and Harrison, 2017; Doropoulos et al., 2019) and substrates have been designed to settle coral larvae for nursery rearing and outplantation improving early survivorship (Guest et al., 2014; Chamberland et al., 2017). Despite advances in the practice of CLP, most research has focused on the steps during and post-spawning, with little attention given to the provenance or phenotype of the broodstock colonies (apart from laboratory based studies, e.g., Dixon et al., 2015; Liew et al., 2020; Quigley et al., 2020). To the best of our knowledge, there have been no attempts to select parents for adaptive traits, such as heat tolerance, as part of CLP for reef rehabilitation.
For SB to be successfully implemented as a coral reef management tool we need to understand the biology of the trait of interest (Figure 1A) and also know how to logistically perform CLP using an assisted evolution approach (Figure 1B). Here we present a practical framework for developing, testing and implementing SB that can be adapted for coral reef rehabilitation and assisted evolution programs. In this study we combine SB trials with CLP using Acropora digitifera as a model species to select for heat tolerance. This framework can also be applied to other propagule-producing organisms (Vanderklift et al., 2020), and other adaptive traits like growth rate, disease resistance or wound healing capability (Baums et al., 2019). The proposed framework is structured into six sections: (1) selection of parental colonies with traits of interest based on phenotypic or other functional characteristics (e.g., known genotypic markers), (2) design of crosses for SB, (3) methods for collecting gametes to perform SB with corals, (4) methods of larval rearing and settlement onto substrate units en masse to produce coral colonies, (5) rearing of coral colonies (in situ or ex situ) for later outplanting to natural reef habitats, and (6) outplant of corals to the reef and monitoring of their growth and survivorship. Testing for heritability and potential resource trade-offs are also critical steps in SB (Ortiz et al., 2013; Cunning et al., 2015), and are being carried out as part of our ongoing work, however, the results of these studies will be reported elsewhere. For this study, we assume that heat tolerance is a heritable trait and that resource trade-offs between heat tolerance and other adaptive traits can maintain populations under future climate change scenarios. Both, the heritability of the trait and potential trade-offs are determinant for SB to be successfully implemented in the field. Further research needs to be done to confirm these assumptions, but these are outside the scope of the present study.
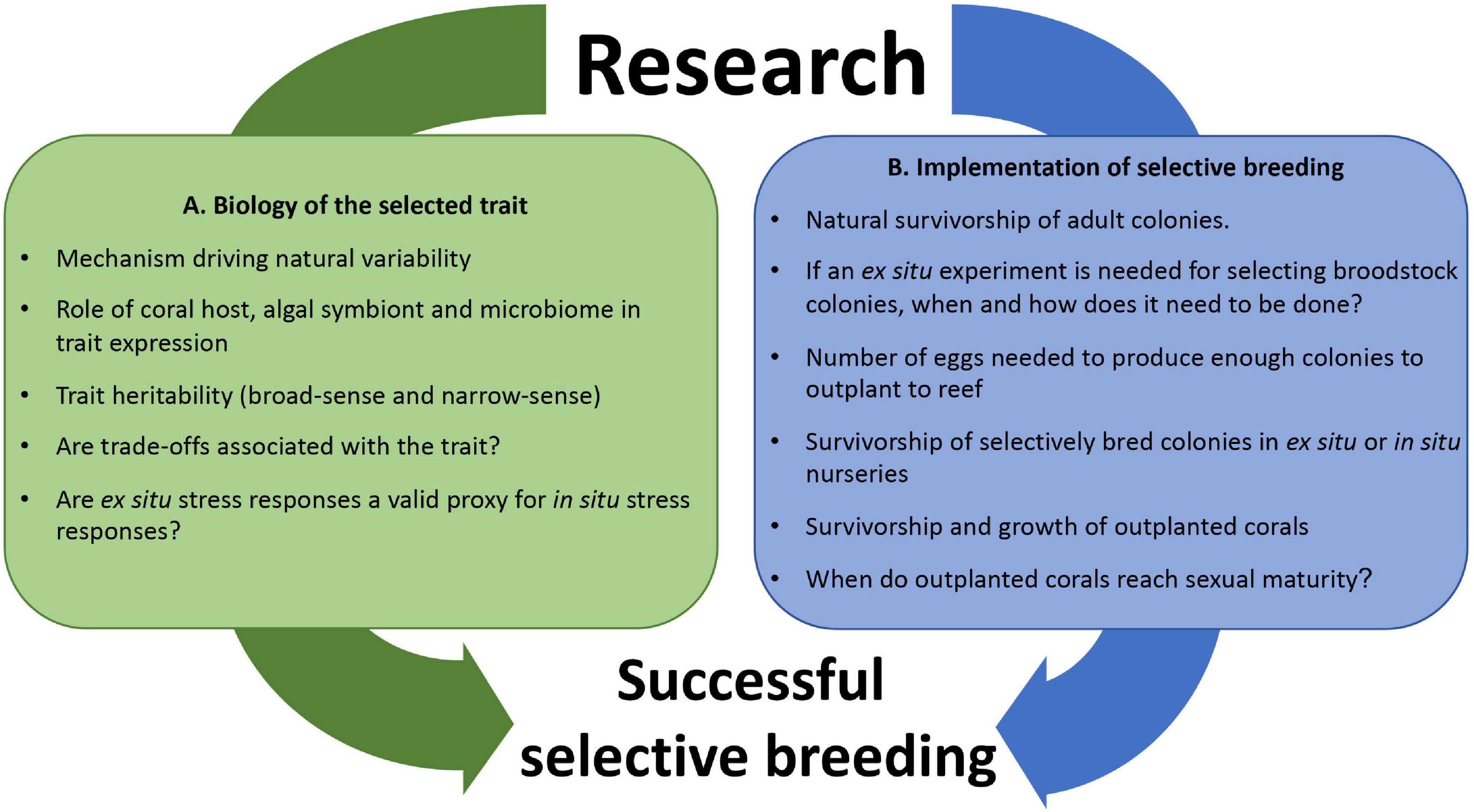
Figure 1. Illustration of research needs for coral selective breeding related to (A) biology of the selected trait, and (B) implementation in the field. It is imperative to develop both research areas simultaneously as the time left to implement these conservation initiatives, given current climate change predictions, is very short (20–30 years).
Materials and Methods
Selection of Parental Colonies for Selective Breeding
The reef-building coral A. digitifera, was used as a model for SB as it is widely distributed and abundant on shallow reefs throughout the Indo-West Pacific. Its digitate morphology facilitates fragment removal for conducting stress assays, and spawning times are established for many locations (Keith et al., 2016; Baird et al., 2021). All of the work described here was carried out at the Palau International Coral Reef Center (PICRC) in the Republic of Palau located in Western Pacific Ocean (Supplementary Material 1A). The source site for all colonies is a shallow, exposed patch-like reef (Mascherchur, N 07°17′29.3″; E 134°31′8.00″; Supplementary Material 1B), where A. digitifera is abundant at depths ranging between 0 and 4 m. In November 2017, 99 visibly healthy adult coral colonies were tagged and mapped along eleven 20-m long fixed transects. The distance between the selected colonies was at least 3 m to maximize the chance of sampling distinct genets rather than clonemates. From these 99 colonies, 34 were randomly selected to assess their performance during a short-term (7-day) temperature stress assay to select parental colonies for the broodstock.
For this short-term assay, seven ∼3 cm long fragments were excised from each colony and transported by boat in 50 L seawater tanks to PICRC (∼20 min boat travel time). The donor colonies remained on the reef to recover for approximately five months before SB work began. The 238 fragments were glued to aragonite substrata (∼20 mm diameter, Oceans Wonders LLC) with ethyl cyanoacrylate gel (Coraffix gel), labeled and mounted into plastic holders, that were attached to plexiglass racks (Supplementary Material 2A–J). To determine the relative heat tolerance of each colony, a 7-day temperature stress experiment was performed using two temperature levels: (a) ambient seawater temperature conditions (30.37 ± 0.46°C, three replicate tanks, Supplementary Material 2C,F,I), and (b) heat stress conditions (Supplementary Material 2A,B,D,E,G,H), where temperature was raised incrementally over the course of 3 days (+2°C on day 1, and +1.5°C on day 3), reaching a daily average temperature of 32.95°C (±0.37) during days 4–7 (five replicate tanks, Supplementary Material 3). Replicate fragments were randomly distributed among seven treatment tanks (24 fragments per tank), with all colonies having at least two replicates in independent stress tanks and at least one replicate in an ambient temperature tank (used as a control for handling stress). The status of each fragment was visually inspected by the same observer daily and ranked as: (1) healthy (no signs of discoloration or mortality), (2) partial mortality (less than 30% of surface area dead) or, (3) dead (more than 30% of the surface with bare skeleton and without tissue). Relative heat tolerance was determined by the end-point mortality (6 days after the first temperature increase). Colonies with all replicate stressed fragments alive (0% mortality) were considered to have relatively high heat tolerance (RHHT), whereas colonies with all stressed fragments dead (100% mortality) were classified as having relatively low heat tolerance (RLHT). Colonies that were not classified either as RHHT or RLHT were considered as unclassified. For brevity and ease of comprehension we will henceforward usually refer to RHHT colonies as “highs” and RLHT as “lows” in the main text but one should be clear that the terms are purely relative and pertain to the particular stress test conducted. Relative heat tolerance was considered as unresolved for colonies with control fragments held at ambient temperature that showed a stress response (partial mortality or death), as this might have resulted from handling stress. Relevant National and State permits were obtained for the collection of fragments (National Marine Research Permits: RE-018, RE-18-13).
Coral Spawning
In anticipation of A. digitifera spawning in Palau (Penland et al., 2003; Gouezo et al., 2020), the 34 colonies used in the temperature stress assay were surveyed to assess reproductive (gravid or non-gravid) and health (alive, partial mortality, or dead) statuses before the April full moon (April 1, 2018). Reproductive status was established by fracturing two branches per colony and checking for the presence of visible pigmented oocytes (Figure 2A; following Baird et al., 2002). Of the 12 colonies identified as lows and four colonies identified as highs (see sections “Materials and Methods” and “”Results”), five and three colonies respectively contained visible, pigmented gametes (Supplementary Material 4). Three gravid colonies were haphazardly chosen from each relative heat tolerance category and collected on March 29 for the SB crosses and transported in 50 L containers to PICRC. Colonies were maintained in an outdoor flow-through 760 L holding tank where water was mixed using three magnetic pumps (Pondmaster 1200 GPH). Four days after the full moon, setting (gamete bundles visible within polyp mouths) was observed in all colonies. We used standard coral larval rearing methods (Guest et al., 2010), with modifications to ensure that individual crosses were isolated. From sunset onward (19:00 h), colonies were checked visually for signs of bundle setting every 30 min. As soon as one colony was seen setting, all colonies were isolated in individual 80 L static tanks to prevent cross fertilization. When most bundles were released (Figure 2B), 200 ml plastic cups were used to scoop buoyant bundles from the water surface. Egg-sperm bundles were separated by transferring them onto a 100 μm mesh filter immersed in a bowl containing a small amount of UV-treated (Trop UV Sterilizer Type 6/IV – TPE, Trop-Electronic GMbH, Germany) 0.2 μm filtered sea water (FSW). Sperm remained in the bowl while eggs remained immersed in FSW within the filter. The filter was removed quickly and transferred to a new bowl with UV-treated 0.2 μm FSW, and eggs were washed five times to remove any sperm residue. Throughout this process, all bowls, filters and other utensils were rinsed with diluted bleach (1%) and FSW. All implements were labeled and used exclusively for individual colonies or crosses to avoid cross contamination. After spawning, colonies were returned to the holding tank, and a week later they were transplanted at the natal reef (Mascherchur).
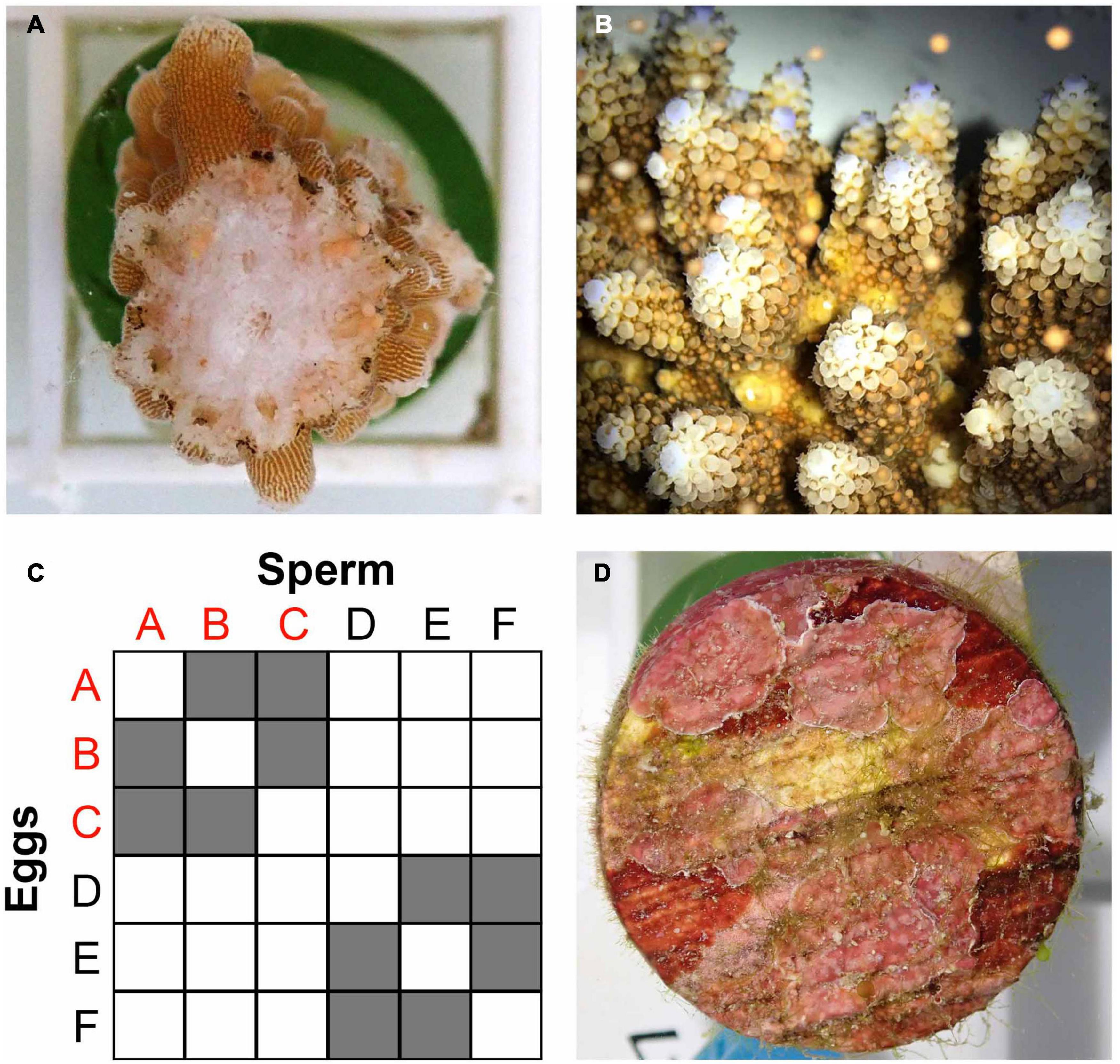
Figure 2. (A) Fragment of a gravid colony of Acropora digitifera with pigmented eggs. (B) A. digitifera bundle release. (C) Schematic representation of the 12 selective breeding crosses performed using three parental colonies of A. digitifera with relatively high heat tolerance (RHHT, colonies A, B, and C), and three colonies with relatively low heat tolerance (RLHT, colonies D, E, and F). (D) Substrate unit conditioned with CCA before been offered to larvae for settlement.
Fertilization and Selective Crosses
Separated gametes were cross fertilized to produce two types of crosses (1) high sire × high dam, and (2) low sire × low dam, and each type of cross was replicated six times using different combinations of parental colonies to produce 12 unique crosses (Figure 2C). The collection and separation of gametes, the performance of SB crosses, the washing of embryos after fertilization, and the maintenance of cultures were carried out by six researchers, two of them with expertise in ex situ coral spawning (JRG and AH). The resulting crosses were maintained in 15 L cone-shaped tanks (Pentair Vaki Scotland Ltd.) at ambient temperature, with 0.2 L/min flow-through with UV-treated 0.2 μm FSW, resulting in one turnover per hour per tank (Figure 3, Supplementary Material 5). A PVC “banjo” with a wedge shape, covered with 100 μm mesh filter was fixed to the inside of the outflows of the tanks to avoid loss of larvae. Each cross was divided between two rearing tanks, resulting in 24 larvae culture tanks. Gentle aeration was introduced 24 h after fertilization, when embryo development had progressed sufficiently, and larvae were round and motile.
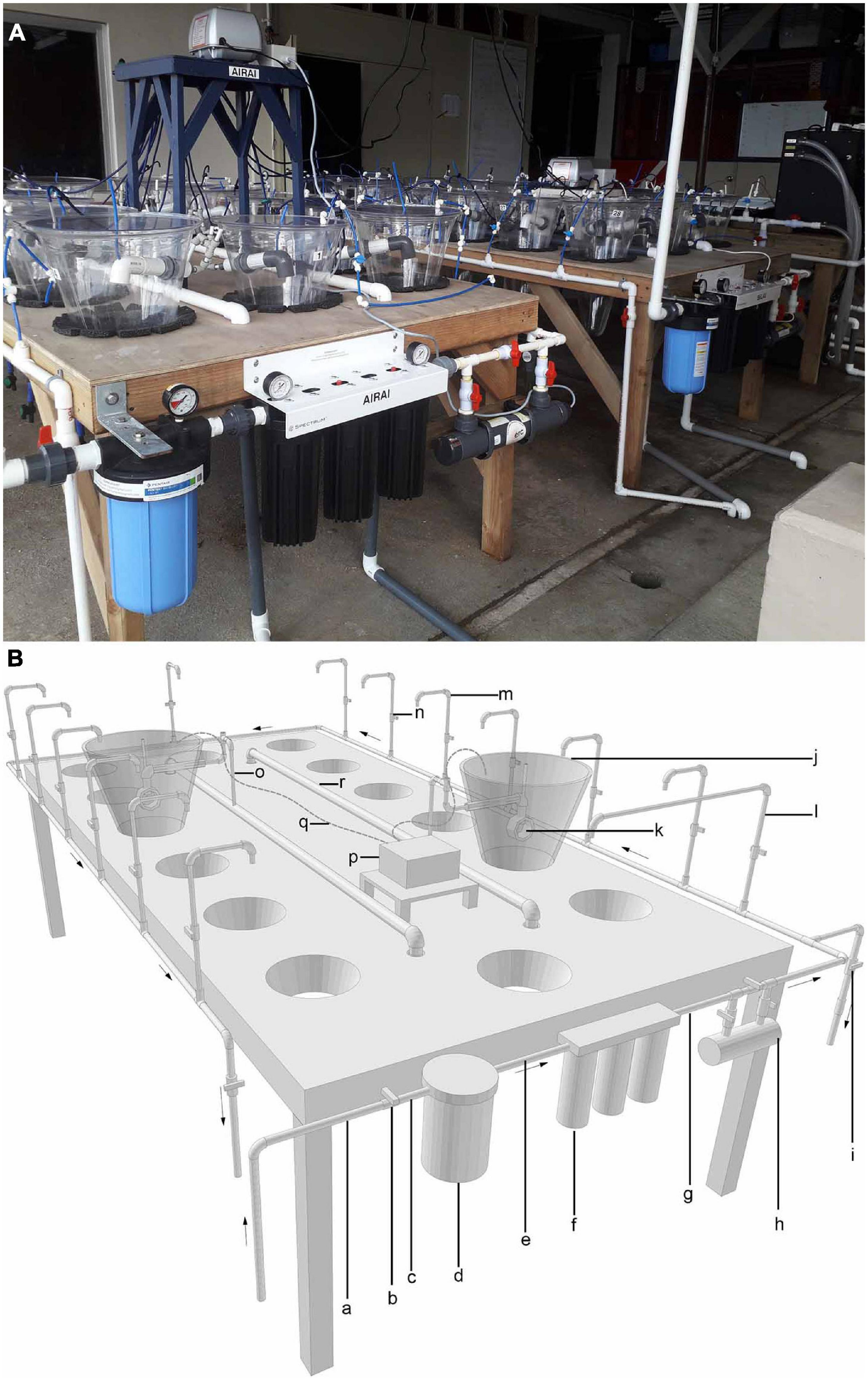
Figure 3. (A) Overview of the larval rearing system consisting of 15 L cone shaped tanks with flow-through. Sea water is filtered through four filters (50, 10, 5, and 0.2 μm) and then exposed to UV light before entering into the tanks. Each tank has inflow pipe for the filtered and UV treated sea water, an outflow pipe for waste-water, and an airline connected to an air pump. (B) Diagram showing the main components of the larval rearing system. Arrows indicate the direction of water flow. (a) Unfiltered sea water inflow, (b) polyvinyl chloride (PVC) valve 1”, (c) 1” PVC pipe, (d) first filtering station (50 μm), e) 3/4” pipe, (f) Second filtering station (10, 5, 1, or 0.2 μm), (g) 1/2” pipe, (h) Trop UV Sterilizer, (i) PVC valve 1/2”, (j) 15 L cone-shaped tanks, (k) Banjo with 100 μm mesh filter, (l) Water inflow tube 1/4”, (m) Elbow 1/4”, (n) Valve 1/4”, (o) Water outflow tube 1/2”, (p) Air pump, (q) Airline, and (r) 1” PVC waste pipe.
Larval Settlement
Circular ceramic substrates (Oceans Wonders LLC) ∼2 cm in diameter with a 1.5 cm stem (hereafter referred as substrate units “SUs”), overgrown with crustose coralline algae (CCA; Figure 2D) were offered to the larvae for settlement. The SUs had been biologically conditioned for four months (130 days) before spawning in two 300 L holding tanks with flow-through water mixed using four pumps each (Taam Rio +800 Powerhead). SUs were arranged on plastic egg crates raised from the bottom of the tank, with fragments of CCA collected at Mascherchur placed on top of the SUs. To stimulate growth of CCA over the SUs and avoid the colonization of filamentous algae, frames with shading cloth were placed over the tanks to reduce light levels to 4 μmol photons m–2 s–1. Three days after fertilization, larvae were transferred from 24 larval rearing tanks to 24 settlement tanks filled up with 10 L of 10 μm FSW and 80 conditioned SUs each. Half of the water in each tank was changed daily with new FSW. Two days after larvae were moved to the settlement tanks, SUs were transferred to flow-through nursery tanks (ex situ nurseries). Each SU was tagged with a cable tie using a color coded system to identify from which cross and replicate culture the colonies had originated (resulting in 24 color codes from 12 crosses).
Ex situ Nursery Tanks
Substrate units with settled corals were randomly distributed among four ex situ flow-through nurseries consisting of 184 L tanks (length: 128 cm, width: 85 cm, water level: 17 cm) with 50 μm FSW. Each tank was illuminated with two Aquarium lights (48” 50/50 XHO Led, Reef Brite Ltd.) at an intensity of 200 μmol photons m–2 s–1 over a 12:12 h diurnal cycle, and had two pumps (Hydor Koralia Nano Circulation Pump/Powerhead, Figures 4A,B) to create water circulation. Fragments from each parental colony were added to each tank to promote Symbiodiniaceae uptake by the coral settlers, together with fragments of CCA. To minimize growth of turf algae, eight small herbivorous juvenile rabbitfish (Siganus lineatus,∼5 cm long) and numerous small grazing snails (Cerithium sp.) were added to each tank. Fish were fed daily with fish pellets (Ocean Nutrition Formula) and nurseries were cleaned every other week by siphoning off the detritus from the bottom of the tanks.
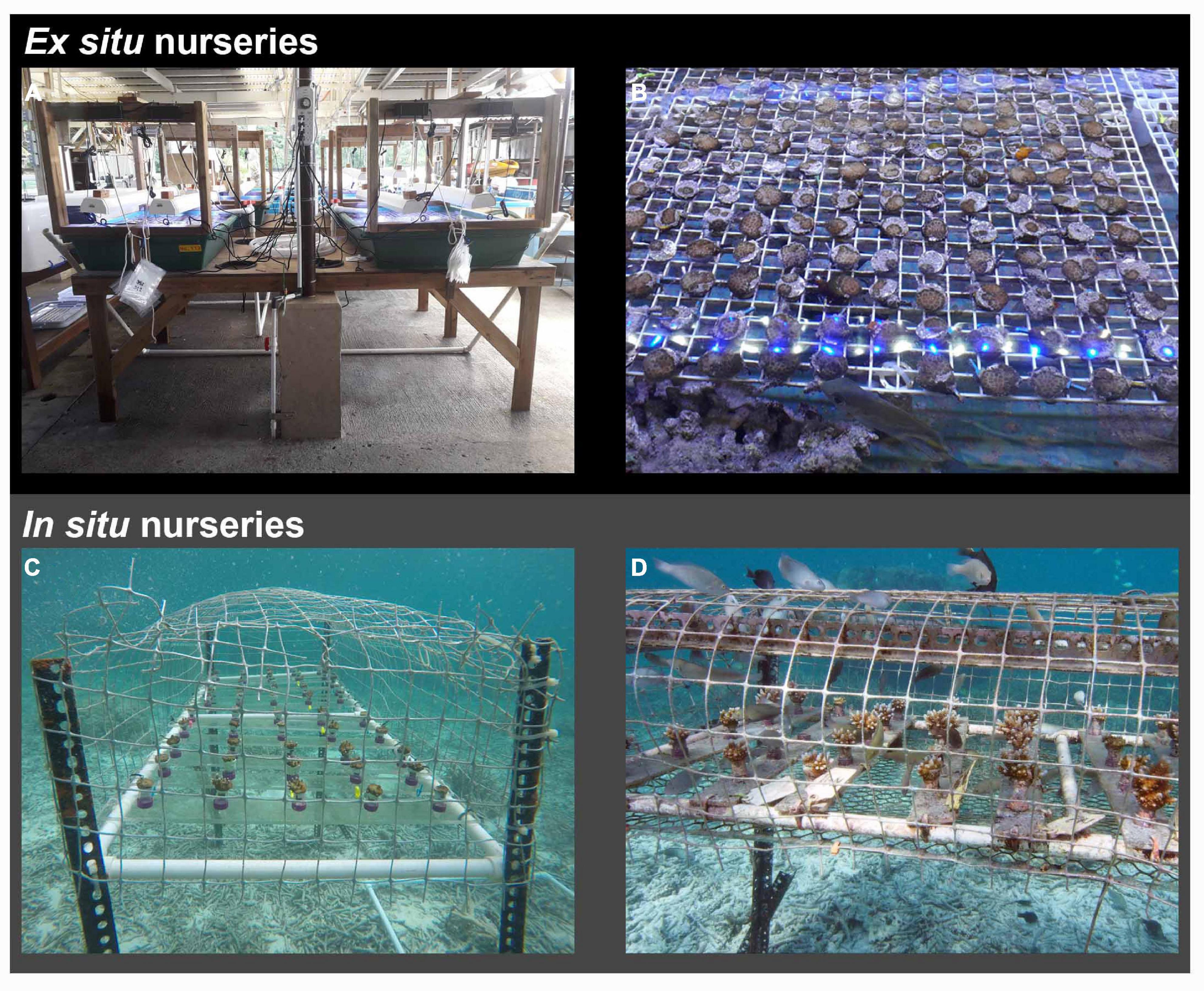
Figure 4. (A) Ex situ nursery tanks, (B) detail of ex situ nurseries with the presence of herbivores Siganus lineatus. (C) Coral colonies when transferred from the ex situ to the in situ caged nursery (13-months old). (D) Corals (17-months old) in the in situ nursery showing the presence of small herbivore fish within the cage.
Coral Outplanting to the Reef
Corals from 12 crosses were outplanted from the ex situ nursery to the natal reef of parental broodstock (Mascherchur) when colonies were 5- and 11-months old (144 and 318 days, n = 288 and 96 colonies respectively, Supplementary Material 6). The number of colonies outplanted at 11-months was limited by the workforce available at the time. To facilitate monitoring, SUs with corals were outplanted along 16 fixed 10-m transects at a depth interval of 1.5–4 m. To attach each SU to the reef substrate, 11 mm holes were drilled into bare reef substratum with a submersible cordless drill (Nemo Divers Drill) and SUs were glued with epoxy (Milliput Standard) after cleaning the reef surface area with a wire brush. Nails were hammered next to each SU to which a cable tie, color coded for each cross was attached. Two divers were required to outplant 25 corals in one 2-h dive. Colonies were monitored at 11, 17, 25, and 32-months (318, 515, 767, and 974-days old respectively) to assess their status (alive, missing, or dead) and photographed from directly above with an underwater camera (Olympus Tough TG-5), and with a ruler for scale.
In situ Nurseries
After 13-months (386-days) of ex situ rearing, the remaining colonies on 296 SUs were transferred to six in situ nurseries (N 7°18′19.80″N; E 134°30′6.70″E, Figure 1B) 2.20 km away from the natal reef. Nurseries were constructed in situ using steel slotted angle bars 40 × 40 mm (length: 135 cm, width: 60 cm), raised from the seafloor (85 cm). A plastic mesh (aperture size 5 cm) was used to cover the nursery structures (Figures 4C,D) to exclude larger corallivores (Baria et al., 2010). Corals were attached to plexiglass racks with five colonies per rack, spaced 3 cm apart. Ten racks were placed in each nursery resulting in a total of 50 colonies per nursery. Meshes and racks were cleaned monthly using a stiff plastic brush to remove algal overgrowth. When colonies were 17-months old (504 days), each colony was photographed with an underwater camera (Olympus Tough TG-5) from directly above, and with a ruler for scale.
Costs of Producing, Rearing, Outplanting, and Monitoring ∼2.5 years Old Coral Colonies Using a Selective Breeding Approach
The cost of producing ∼2.5-years old (32-months) live colonies was calculated from the total cost of materials and hours of labor needed to run the experimental setup at full capacity with 24 larval cultures, rear, outplant at the natal reef and monitor the resulting colonies. The cost of the experiment to characterize the relative heat tolerance of the parental colonies (Supplementary Material 7) was not included in this analysis, as this will vary considerably according to the trait of interest being selected for, the methodology (i.e., experiments under laboratory conditions, type of analysis, etc.) used to identify colonies of interest, and their location. Due to logistical constraints, we were not able to quantify fertilization success, larval survivorship, and initial settlement densities and survivorships, hence, values reported in the literature were used for the analysis. Details of the assumptions of the analysis, costs of consumables, equipment, and person hours are provided in the Supplementary Material 7. Cost per coral was estimated by dividing the total cost for the project by the number of SUs containing one surviving 2.5-year old coral for different ages at outplant (5 or 11-months). To compare costs of rearing in ex situ and in situ nurseries we considered the costs of consumables for their construction and their maintenance during a 11-month period, and cost per SUs was estimated by dividing the total cost of building and maintenance of the nurseries by the number of SUs. The overall efficiency for each outplantation age (5 or 11-months) was estimated by dividing the number of coral eggs used by the number of living colonies after 2.5-years. The aim of this analysis was to: (1) estimate the minimum cost of CLP using a SB framework, (2) identify the steps of the framework (coral collection, spawning to competency, settlement, rearing, outplanting, and monitoring) that incur the highest cost of the budget, and (3) evaluate the effect on efficiency and cost per coral of outplanting colonies at two ages (5 and 11-months). The total cost of this framework should not be used as a reference for SB under assisted evolution since: (1) the costs resulted from a small spatial scale experiment and are not representative of expenses of restoration initiatives at large spatial scales, (2) specific assumptions were made for its computation (Supplementary Material 7) and any change in these assumptions will change the total costs, (3) upscaling each of the steps will reduce their cost due to economies of scale, and (4) our results do not include data on the reproductive status of the recruited colonies 2.5 years post-fertilization. Before the predicted spawning event in 2020, the reproductive status of nine colonies with the biggest diameter in the in situ nursery was assessed (as described in section “Coral Spawning”), with none containing visible eggs. Additionally, recruited colonies had smaller sizes than the corals in the in situ nursery and were only just starting to develop branches (Figure 5), suggesting that they were not reproductive at this time.
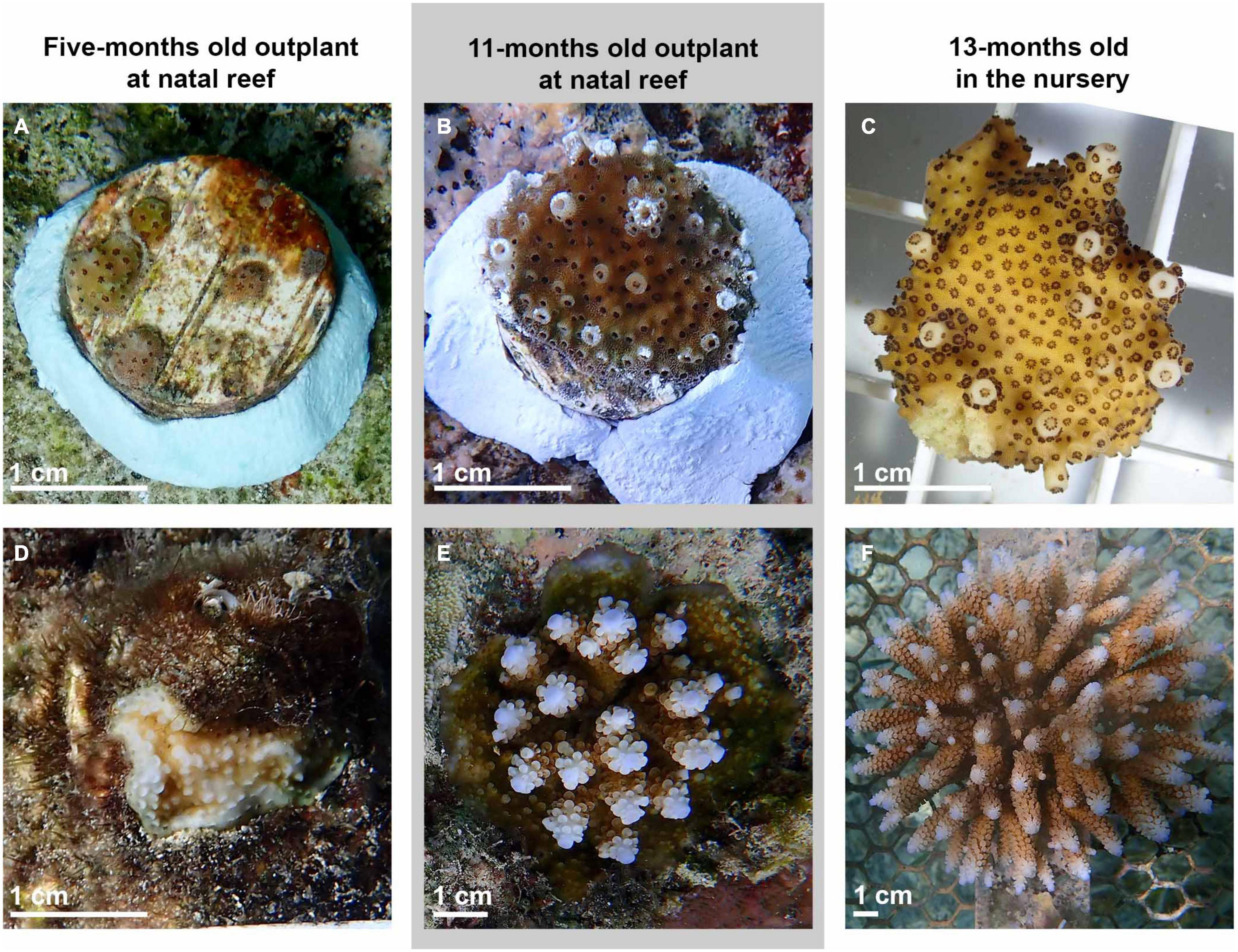
Figure 5. Images showing representative colonies to illustrate differences in size and morphology at the time of outplanting to the reef, or transfer to the in situ nursery (A: 5-month old outplant, B: 11-month old outplant, and C: 13-month old before transferring to the in situ nursery), and during the last monitoring (D: 5-month old outplant, E: 11-month old outplant, and F: in situ nursery) when colonies were 2.5 years old (52 days after the spawning event of 2020).
Settlement Density
To provide practical guidance for planning CLP, the minimum number of settlers needed to obtain SUs with at least one colony after four-months (132-days old) of nursery rearing was determined by testing the effect of settlement density on colony survivorship. This experiment was carried out during the spawning event on April 6, 2020 using three adult A. digitifera colonies collected at Mascherchur. A mass culture was produced with the gametes of the colonies following the protocol previously described for collecting bundles and rearing larvae. Four days after spawning, once larvae were competent to settle, 1.5 L static tanks were stocked at three levels of larval densities (10, 25, and 50 larvae per SU or 67, 167, and 333 larvae L–1, with n = 8 replicate tanks). Each tank contained between four and ten SUs previously conditioned with CCA for 198 days (Supplementary Material 9). Water changes were carried out twice daily over a week with UV treated 1 μm FSW. Ten days after settlement, the number of settlers on each SUs were counted using a stereomicroscope. SUs (n = 157) with live settlers (between one and 15 per SU) were then randomly distributed across four ex situ nurseries (described in section “Ex situ Nursery Tanks”). The number of live corals per SU was again recorded after 4 months using a stereomicroscope.
Data Analysis
Natural mortality of tagged colonies on the reef was estimated using yearly exponential rates of survival (Clark and Edwards, 1995). Colony survivorship was compared between the two different outplanting times to the reef (5 and 11-month old) using right censored data with the Kaplan–Meier model and the log-rank statistic (Harrington and Fleming, 1982). As it was not possible to determine the exact time of death for each coral, the date that a coral died was estimated as the middle time point between survey dates. Survivorship functions of corals were compared (a) among outplanting times at different ages (5 and 11-months old), and (b) once outplanted i.e., with respect to days out on the reef rather than age attained. Colony size, measured as planar area for corals outplanted to the reef (at an age of 5 and 11-months) or moved to the in situ nursery was estimated from scaled downward-facing images taken when corals were 17-months old using ImageJ. During image analysis, the number of developed branches per colony was recorded as an indicator of volume. The number of colonies with branches was compared using basic descriptive summary methods (percentages of branching colonies of the total alive outplants). The effect of outplanting method (three-level fixed effect) on colony size at 17-months was tested using Generalized Linear Mixed effects Models (GLMM; Brooks et al., 2017), accounting for differences in size due to cross replication (12-level random factor). Log-Gamma link was used to prevent negative fitted values of this strictly positive response variable. Among method comparisons were tested using a post hoc Tukey Test.
The effect of larval culture density (three-level fixed effect) on settlement density after 10 days was tested using GLMM, accounting for variability among settlement tanks (eight-level random effect). The relationship between settlement density (fixed effect) and number of 4-month-old colonies per SU was tested using GLMM, accounting for variability among holding tanks (four-level random effect). All model validation steps included assessing homogeneity of residuals versus fitted values, over and under dispersion and a simulation study to test the ability of the model to capture zero-inflation (Zuur and Ieno, 2017). Poisson models suitable for count data (Bates et al., 2015) were over-dispersed due to underestimation of zeros, however, negative binomial models with quadratic parameterization variance structure (Brooks et al., 2017) passed all validation routines (Supplementary Material 9). Marginal R2 values, the proportion of variance explained by fixed effects alone were computed for the final model (Lüdecke et al., 2020).
Results
Selection of Parental Colonies
Relative heat tolerance varied among colonies (n = 34), with 15% categorized as high and 32% categorized as low (Table 1). For 21% of the colonies at least one of the fragments held in the control tanks died, and therefore their relative heat tolerance was considered as unresolved (Table 1). For the remaining 32% colonies, between 25 and 66% of fragments died during the experiment and colonies were unclassified in terms of relative heat tolerance (Table 1). Natural mortality rates of tagged colonies at Mascherchur were estimated at ∼20% per year, with eight colonies recorded as dead 140 days after tagging (n = 99). Partial mortality was observed in 6% of the tagged colonies and one colony could not be relocated (Supplementary Material 4). From the surviving, visibly healthy colonies (n = 84), 38% contained pigmented eggs in March 2018, and these included three colonies classified as high and five as low (Supplementary Material 4).
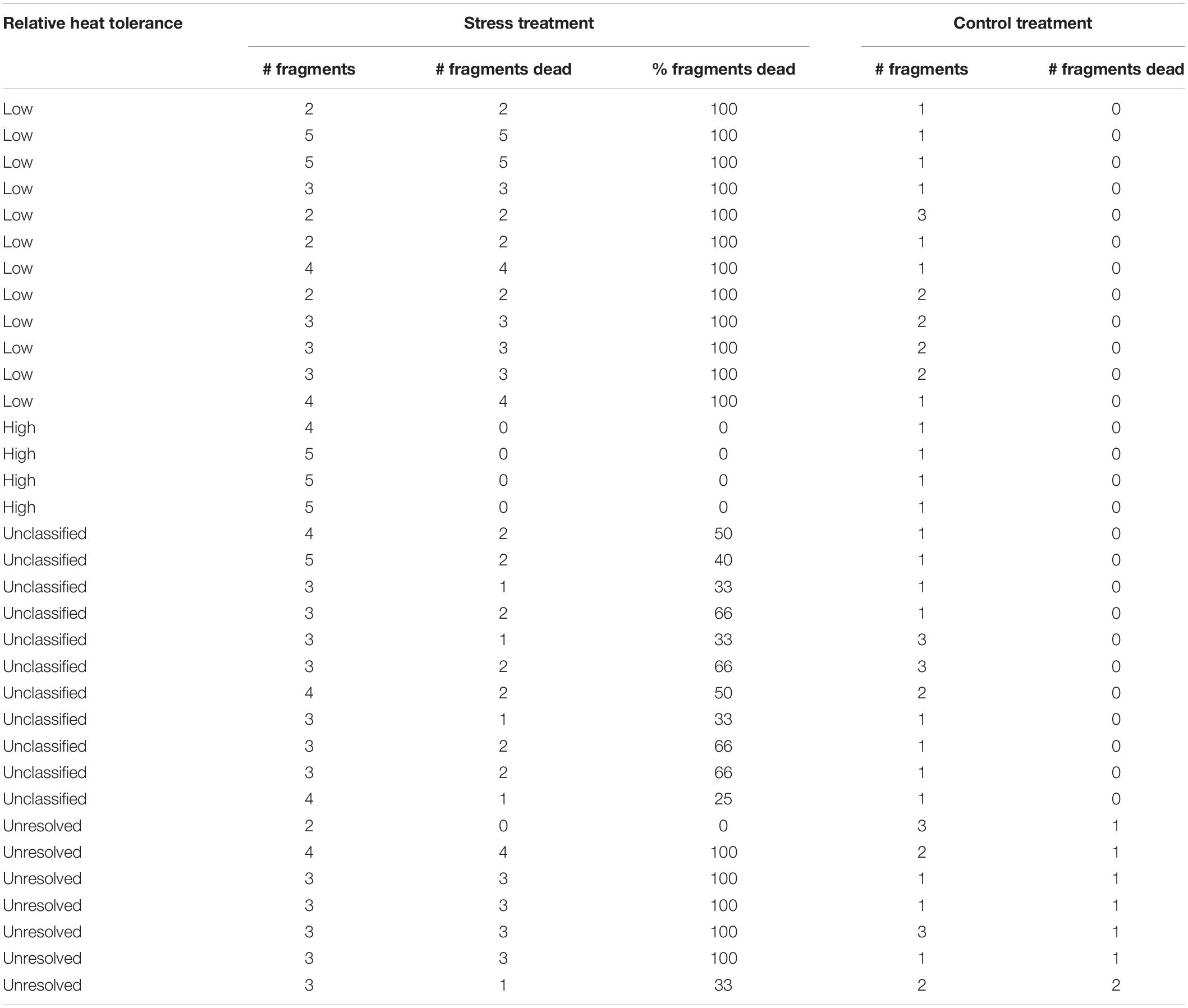
Table 1. Results of the 7-day heat stress exposure used to determine the relative heat tolerance of the colonies.
Settlement Density Experiment
In 2020, the density of the larval culture had a significant effect on settlement densities (Figure 6A). The expected mean settlement density using a larval culture of 50 larvae per SU was two- and threefold higher than that of the two other larval cultures with 25 and 10 larvae per SU respectively (GLMM, R2 = 0.28, p < 0.01; Supplementary Material 10). The density of live settlers per SU at 10 days post-settlement had a positive effect on the density of colonies four months later (Figure 6A, GLMM, R2 = 0.36, p < 0.001; Supplementary Material 11). On average, a settlement density of four settlers per SU was sufficient to obtain at least one coral per SU after four months under ex situ nursery conditions (1.3 settlers per cm2 of effective settling surface, Figure 6B).
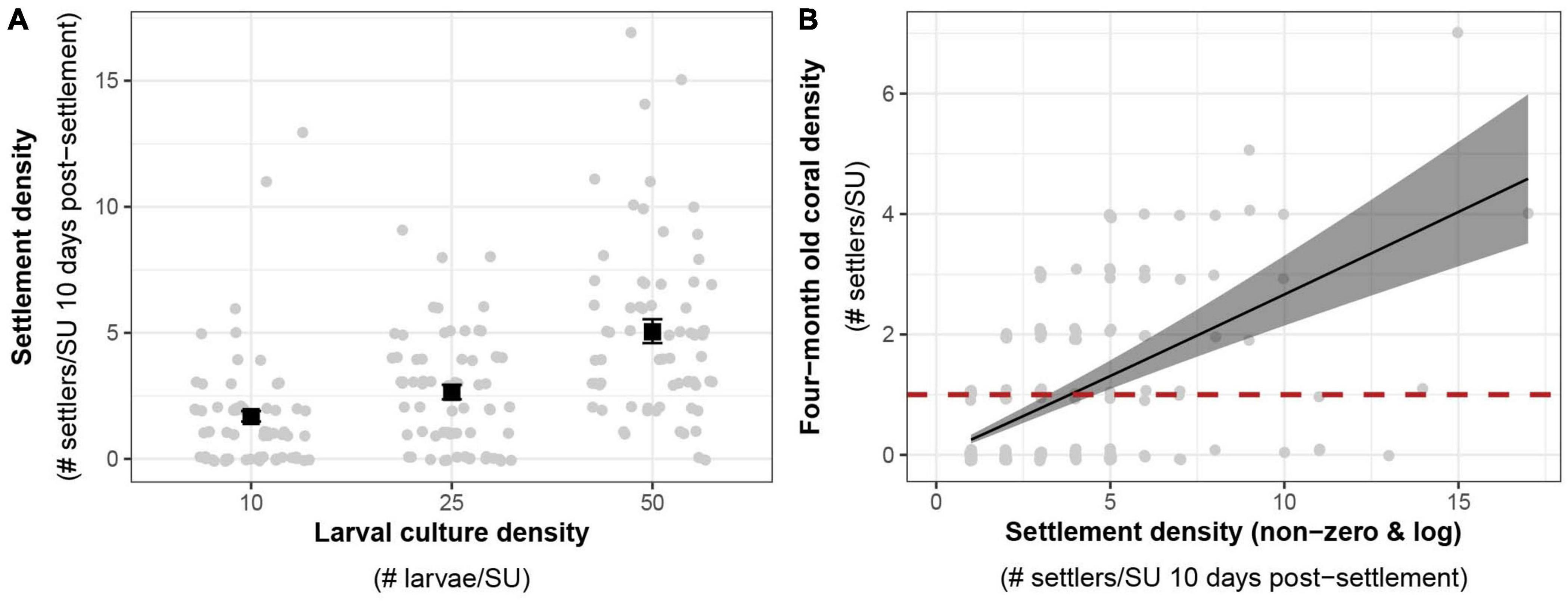
Figure 6. (A) Effects of larval density on settlement density. Average expected response values among settlement tanks (black squares) from negative binomial GLMMs are shown with standard error bars. (B) Expected relationship between settlement density and colony density after 4 months (132 days, black line and standard error) among ex situ nursery tanks derived from a negative binomial GLMM. Equation: four months coral density = exp[–1.44 + 1.02 × log(Non-zero settlement density)]. The critical target threshold of 1 coral per substrate after four months is represented with a red dashed line. Raw count data (gray circles) are visualized with a jitter to minimize overlap of points in the two graphs.
Effects of ex situ and in situ Nursery Rearing on Colony Survivorship
Colony survivorship was significantly affected by age at the time of outplanting (F1,380 = 102, p < 0.05, Figure 7A). The median survival age of corals outplanted at 11-months old was more than twice that of those outplanted at 5-months old (median survival age of 646 and 257 days respectively). The relative survival once outplanted (i.e., with respect to days out on the reef) increased more than threefold when ex situ nursery time was extended, with colonies outplanted 5-months post-fertilization surviving a median time of 104 days, whereas outplants at 11-months post-fertilization survived for a median time of 324 days post-outplant. Only ∼6% of corals that were outplanted at 5-months post-fertilization survived to 32-months old. In contrast, corals that were reared in nurseries for 11-months prior to outplanting to the reef had five times better survivorship (∼30%) to 32-months old.
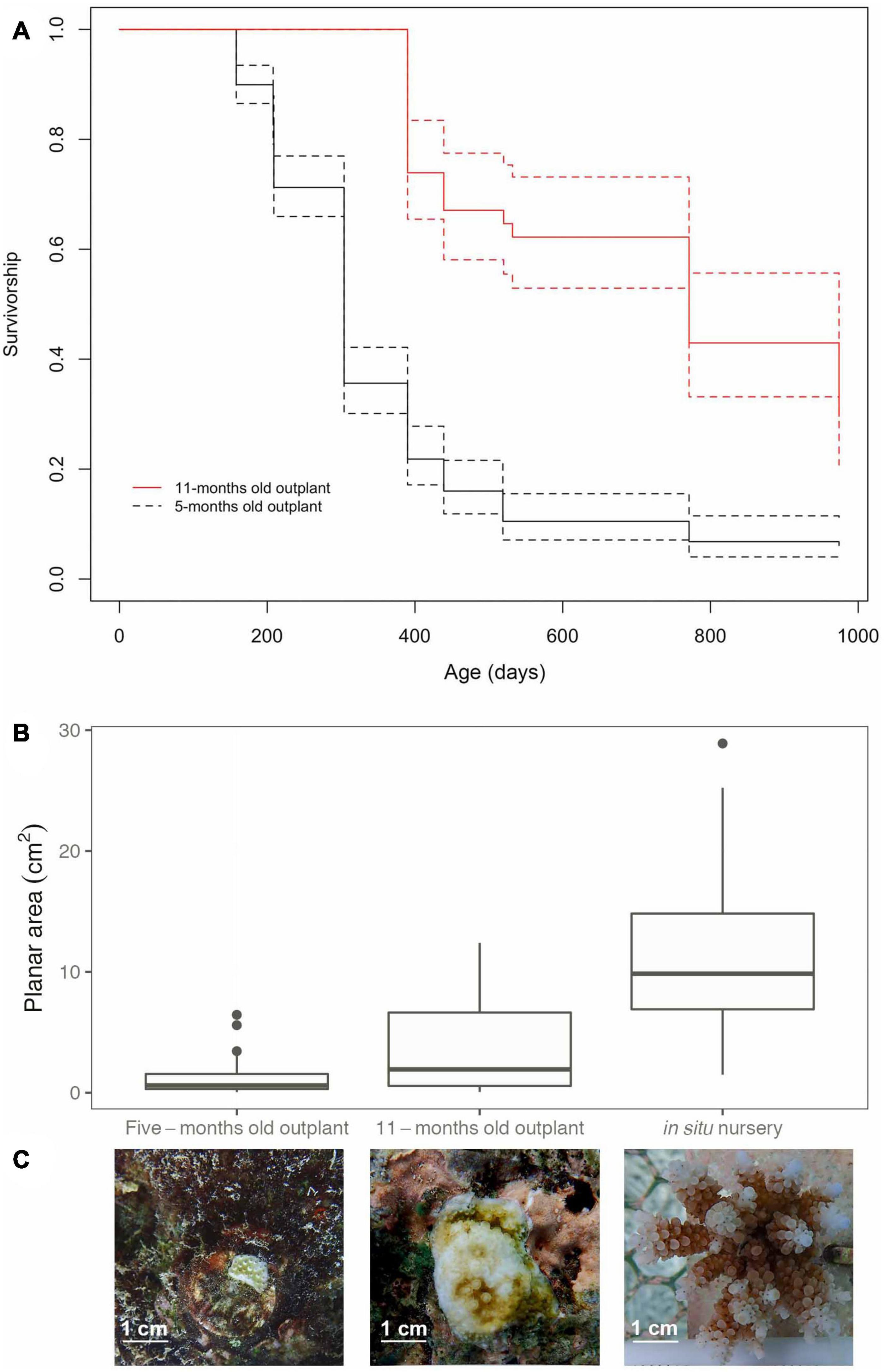
Figure 7. (A) Survivorship curves for Acropora digitifera corals outplanted to the reef when colonies were 5-months (black line) and 11-months old (red line). Dashed lines indicate 95% confidence intervals. (B) Planar area comparison between colonies from the ex situ nursery that were outplanted to the reef at 5 and 11-months old or transferred to the in situ nursery at 13-months old. (C) Images showing representative corals in the different treatments at the same age (17-months old).
At 17-months, corals at the in situ nursery started developing a 3D structure with branches present in 72% of the colonies, compared to corals outplanted to the reef where none had started branching for both 5 and 11-months outplants. The planar area of corals was greater in treatments that had longer husbandry times (i.e., were held in the in situ or ex situ nurseries for longer periods). Coral size at 17-months was strongly affected by age at time of outplanting (i.e., outplanted to the reef at 5-month, 11-months, or transferred to the in situ nursery after 13-months, Figures 7B,C). In situ nursery reared corals were significantly larger than those outplanted at 5 and 11-months (GLMM p < 0.001, Supplementary Material 12), and corals outplanted at 11-months were significantly larger than those outplanted at 5-months (GLMM Tukey Test, p < 0.001).
Cost Analysis
The total cost of producing, rearing, outplanting, and monitoring 2.5-years old corals using a SB framework was US$23,817 for colonies outplanted at 5-months old (Supplementary Material 13, 14), and US$22,500 for those outplanted at 11-months old (Table 2 and Supplementary Material 15). The total cost when outplanting corals at 11-months old was US$1,317 less than when outplanting at 5-months, because a greater number of colonies (300 extra colonies) needed to be outplanted and monitored thus incurring higher labor and consumable costs. The overall efficiency to produce 12 distinctive crosses with two replicate cultures each and rearing the resulting colonies under ex situ nursery conditions until outplant age (either 5 or 11-months old) was increased fourfold when outplanted at the later age (0.09 and 0.4, respectively). Equally, the cost of each SU with a live coral after 2.5 years was five times lower when nursery period was extended (US$227 for outplants at 5-months old compared to US$49 for 11-months old outplants). Outplanting was the activity that incurred the highest costs (35 and 32% of the total cost for the 5 and 11-months old respectively; Table 2 and Supplementary Material 15), followed by monitoring (33 and 31% of the total cost for the 5 and 11-months old respectively, Table 2 and Supplementary Material 15). Costs associated with rearing corals for 11-months in ex situ compared to in situ nurseries differed considerably, with cost per SUs being sixfold lower for the ex situ nurseries (US$0.97 and US$5.75 respectively, Supplementary Material 15–17).
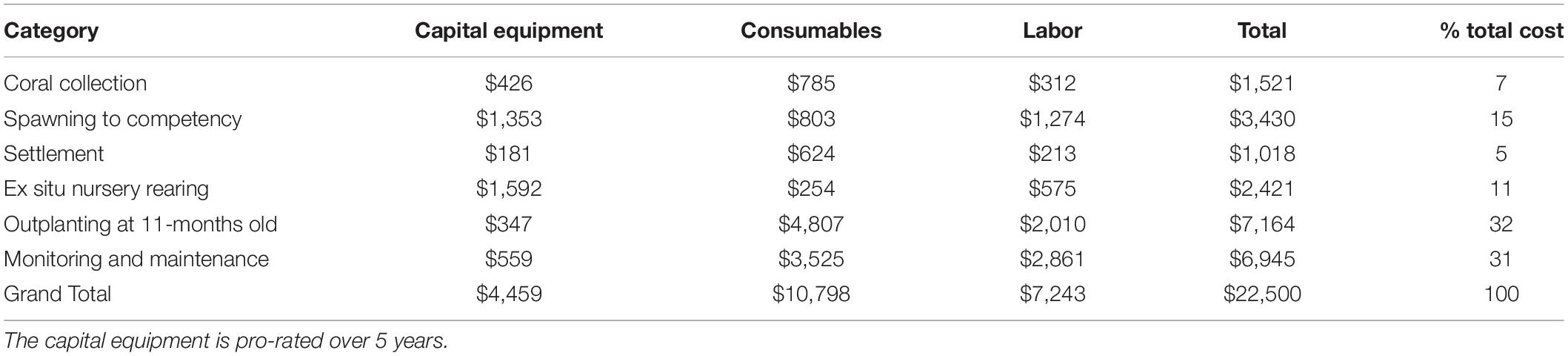
Table 2. Cost of sexually propagating Acropora digitifera corals using a selective breeding framework with corals reared under ex situ nursery tanks until 11-months old when they were outplanted to the reef.
Discussion
Research to assess the feasibility of assisting adaptation of corals in the face of ocean warming is accelerating, however frameworks for practical application of this research to conservation and management are lacking. Here we present a framework for CLP that involves prior selection of parental colonies based on intrapopulation variation in their heat tolerance (Figure 8). We established optimal settlement densities of larvae to obtain one coral per SU after four-months of ex situ rearing and demonstrate the potential of rearing corals using a combination of ex situ and in situ nurseries to optimize their growth and survivorship. We found large differences in growth between corals outplanted to the reef and those reared in in situ nurseries. We also found differences in survivorship depending on age at outplant from ex situ nurseries, emphasizing the benefits of long nursery phases on final costs per coral. Our data highlight some of the major challenges associated with combining SB with CLP, and the areas that need further research and development to improve efficiency and to reduce the high costs involved.
The selection of parental colonies was made based on intrapopulation variation in heat tolerance using a 7-day temperature stress exposure (Figure 8.1). Our results show that our study population of A. digitifera contained considerable intrapopulation variability in heat tolerance, with some colonies (∼15%) able to withstand a short-term heat stress with zero fragment mortality. In this case, there was clearly sufficient intrapopulation variability in relative heat tolerance to provide broodstock for SB initiatives. While further work is needed to determine if the selected trait is passed on to offspring, the relative heat tolerance among the selected broodstock was not related to the Symbiodiniaceae community (Supplementary Material 19). The high annual mortality rate of the tagged colonies (9.5%) and the low proportion of the population spawning in the same month (38%) meant that we had relatively few colonies to choose from for SB. Rates of annual mortality recorded here are typical for Acropora (Madin et al., 2014) and spawning synchrony can vary considerably among taxa, location, and year (Baird et al., 2009; Gouezo et al., 2020), or can shift with handling and transportation stress or while in captivity (Okubo et al., 2006; Craggs et al., 2017). These facts highlight the need to identify and test relatively large numbers of corals within populations to increase the likelihood of having sufficient numbers of gravid colonies for SB. Selecting the broodstock from within populations (rather from distinct populations) avoids risks associated with maladaptation, genetic swamping or outbreeding depression (Edmands, 2006; Baums, 2008). It also reduces logistical challenges associated with the collection and transport of colonies or gametes between populations (i.e., between geographically distant locations or countries). Conversely, a limitation of conducting SB within populations is the need to identify in advance sufficient numbers of distinct genets with the desired trait using appropriate stress trials, and to avoid breeding closely related colonies. Sufficient tagged colonies also need to survive, remain healthy, and be gravid at the predicted spawning time. An alternative to direct testing for a desired phenotype is the identification of biomarkers of stress tolerance (i.e., a specific lipid or protein constituents, immune profiles, genes, microbial or Symbiodiniaceae symbiont communities, etc.). However, as yet no biomarkers have been developed for any trait in corals and further research in this area is needed to develop these as tools. An option to overcome this hurdle is to create clonal broodstocks of colonies with desired traits either in an in situ nursery or at the parental reef. A combination of these two practices could increase the access to genets for SB, and even provide access to the same broodstock during consecutive years.
We limited our SB design to single pairwise crosses (only two parental colonies, Figure 8.2A), because the number of eggs available per colony limited the number of crosses that could be performed, while still producing sufficient colonies for outplant. To perform single pairwise crosses, broodstock needed to be collected and isolated in tanks before spawning, with all spawning and fertilization work carried out ex situ in relatively controlled laboratory conditions (Figure 8.3B). In some cases, this approach might not be possible, if for example, spawn collection has to be done in situ (Figure 8.3A), or if natal reefs are far from facilities where spawning and rearing will take place. The total number of pairwise crosses that can be achieved during a spawning event will depend on the extent of synchronous spawning among colonies and the workforce available for the collection, separation and washing of gametes during their viable time period (<2 h; Omori et al., 2001). In addition, in order to maximize the number of pairwise crosses produced requires that each cross is reared independently, and therefore the number of culture vessels can quickly escalate. This then requires increased workforce effort to manage the husbandry during the early post spawning period.
For studies where heritability of the trait has been reported with both sperm and eggs (i.e., Liew et al., 2020), then mass crosses (more than two selected parental colonies, i.e., colonies with relative high heat tolerance, Figure 8.2B) may be a better approach than carrying out many individual crosses. These can be done either by (a) pooling gametes of all colonies with the trait of interest to produce one culture that is divided into replicate larval rearing tanks, or by (b) pooling the sperm of several colonies to fertilize the eggs of a single colony. An advantage of pooling gametes is that corals can be allowed to spawn together en masse in a single tank reducing the work involved in collecting and separating bundles from many individual colonies. However, culture viability can be compromised by a modest percentage of unfertilized eggs that could originate from a single colony (Pollock et al., 2017), or the resulting larvae could be closely related if one of the genets dominates the pool and drives fertilization. Alternatively, pooling the sperm of several colonies to fertilize eggs from one colony requires collection, separation, and washing of gametes as previously described for pairwise crosses. The benefits of this procedure are that it can be replicated with eggs from all donor colonies, resulting in several distinctive crosses with potentially higher fertilization success, as the concentration of gametes can be better controlled. However, whilst logistical constraints during spawning and fertilization periods are eased through either colony or sperm pooling, a significant drawback to these approaches is that resulting cohorts produced are a mix of either half or non-related kin. Long-term post-settlement survival in these kin groups may be lower, compared to fully related offspring, due to negative allogenic interactions following developmental onset of the corals’ immune response (Puill-Stephan et al., 2012). Therefore, trade-offs occur between increased work and facilities required to produce large numbers of pairwise crosses versus simplified logistics and reduced larval rearing costs but potentially increased post settlement mortality.
Scientists have successfully conducted CLP for three decades, and great progress has been achieved in controlling steps associated with the early stages, i.e., controlling spawning times (Craggs et al., 2017), fertilizing gametes en masse, and rearing larvae to obtain coral colonies (Guest et al., 2010; Randall et al., 2020). Thus, one of the more challenging steps in CLP is to settle larvae efficiently onto SUs and enhance their survivorship and growth until outplant (Figure 8.4). Our results show an average of four corals per SU (1.3 settlers per cm2) at 10 days after settlement, resulted in at least one colony after four months of ex situ husbandry rearing. Despite coral post-settlement survivorship being influenced by settlement density and husbandry conditions (Conlan et al., 2017; Cameron and Harrison, 2020), our results provide a useful guideline for optimal settlement densities when using SUs for CLP. For SUs outplanted at different ages, similar analyses are needed to provide information on the minimum number of larvae to settle to obtain one colony per SU at the age of outplant. Furthermore, restoration initiatives will benefit from knowing the optimal number of larvae per SU that will maximize the probability of obtaining a colony that attains sexual maturity, a factor that will be dependent on the overall survivorship of the outplant. An optimal use of available competent larvae for settlement will be a defining factor of the efficiency of the effort. However, the production of larvae is one of the steps which incurs the lowest costs in the framework (Table 2 and Supplementary Material 14) so efficiency and cost-efficiency may not go hand in hand. Settling more larvae per SU than needed will waste larvae, whereas, settling too few larvae per SU lowers yield and increases costs. Evidence indicates that low larval settlement densities can compromise the production of SUs with at least one colony at the time of outplanting due to high post-settlement mortality, while high settlement densities can compromise survivorship due to density dependent effects (Doropoulos et al., 2017; Cameron and Harrison, 2020). Additionally, high larval densities promote the formation of chimeras, a factor that needs further research as their implications on colony growth and survivorship are still understudied (however see Rinkevich, 2019; Huffmyer et al., 2021).
Husbandry of corals during the early post-settlement stages is a key step for successfully conducting CLP as it has a significant impact on growth and survivorship. Techniques for rearing settlers until they can be outplanted to the reef in sufficiently large numbers remain largely experimental. Early survivorship after settlement is the primary bottleneck in CLP for improving cost-effectiveness. Our results show that extending ex situ nursery times increased both survivorship and growth rates of outplants and reduced the costs of the production of colonies. Husbandry during the early months has been proven to increase coral survivorship (Baria et al., 2010; Guest et al., 2014; Craggs et al., 2019) and growth (this study) by reducing predation and competition pressures (Doropoulos et al., 2016; Gallagher and Doropoulos, 2017). Moreover, ex situ rearing can enhance colony growth by controlling light, water and food quality, and by co-culturing corals with herbivores to limit algal overgrowth (Craggs et al., 2019) and reduce costs (Figure 8.5A, Table 2, and Supplementary Material 14, 15). However, there may also be drawbacks associated with longer nursery times such as: (1) acquisition of a Symbiodiniaceae community that differs from the one at the outplant site (Baums et al., 2019), (2) potential fitness consequences of plastic and epigenetic changes due to exposure to different environmental conditions during nursery rearing (Parkinson and Baums, 2014), and (3) development of pathogens or diseases during husbandry (Sheridan et al., 2013).
Many of the disadvantages encountered in ex situ nurseries (see above and van Woesik et al., 2021) can be avoided under in situ conditions (Figure 8.5B), especially if the location of the nursery is close to the outplant sites. However, the costs associated with in situ (Supplementary Material 18) husbandry are considerably higher than ex situ conditions (Table 2), given the logistic and practical constraints associated with working underwater. The location of the in situ nursery in a place with similar environmental conditions to the outplant site, in a shallow area with good water quality that is protected from storms, and that is easy to access can enhance the survivorship and growth of the corals and reduce costs. Local knowledge and historical environmental data will improve the chances of locating appropriate in situ nurseries sites. However, one major limitation of in situ nurseries is that they are prone to damage due to environmental stressors (i.e., temperature fluctuations and storms) and human activities (e.g., diver or anchor impacts). Moreover, enhanced growth rates at in situ nurseries can be associated with reductions in skeletal densities (Pratchett et al., 2015), which may disadvantage colony performance once outplanted to the reef.
The outplanting phase was the most expensive activity of this framework, highlighting the need for technical development (Figure 8.6) if restoration is to be scaled up. The method used for outplanting corals in this study is not suitable for larger scale restoration and should not be considered as such as it is laborious, slow, and costly and only appropriate at a scale of tens to hundreds of square meters (Guest et al., 2014). The outplanting methodology should minimize the use of tools and attachment materials (i.e., Coralclip®; Suggett et al., 2019). SUs should be designed so that they are easy to transport, can be rapidly and easy deployed, can be readily attached to the reef substrate (i.e., tetrapods; Chamberland et al., 2017) and, if possible be made of sustainable and ecologically friendly constituents (i.e., sustainable cement; de Brito and Kurda, 2021). The design of SU should enhance coral survivorship until they reach an escape size (i.e., with micro-refugia to protect colonies from grazing pressure) and improve the success of early outplant, reducing husbandry time. Interdisciplinary teams of ecologist, aquarists and engineers are key to developing novel designs of SUs that can be easily deployed in different reef environments (i.e., reef crest and reef flat), which is a critical factor for CLP and SB techniques to be adopted as a management strategy of coral reefs.
The cost analysis reveals that extending husbandry time has a major impact on the efficiency of the propagation effort and reducing the cost of 2.5-year-old colonies five times as a result of early survivorship increases. Total costs could be reduced considerably if post-outplant survivorship is increased at younger ages, thus decreasing husbandry times, hence innovation in this area is fundamental to reduce total costs. Likewise, several strategies could be adopted to reduce costs associated with monitoring, for example, (a) surveying only a representative subset of outplants, (b) developing a citizen science program which incorporates the local and tourist community during the monitoring phase (Sinclair et al., 2021), and (c) use of innovative technologies like photogrammetry to monitor large reef areas (∼250 m) while minimizing time underwater (Lechene et al., 2019). Cost breakdown of CLP is not straightforward, owing to several factors that vary between coral species, sites, facilities and countries. Yet, doing the exercise of estimating such expenses accounting for types of costs (i.e., capital equipment, consumables, and labor) provides a quantitative method to identify the steps that need further development to improve overall efficiency and reduce costs. Reef rehabilitation initiatives using CLP under a SB approach will effectively become a management strategy to promote adaptation and resilience of reefs in the Anthropocene when techniques are proven to be cost-effective.
Data Availability Statement
The original contributions presented in the study are included in the article/Supplementary Material, further inquiries can be directed to the corresponding author/s.
Author Contributions
AH, JCB, AJE, PP, ES, AT, and JRG contributed to conception and design of the study. AH and AJE organized the database. AH, AJE, LL, and ES performed the statistical analysis. AH wrote the first draft of the manuscript. AJE, LL, ES, and JRG wrote sections of the manuscript. All authors contributed to data collection, manuscript revision, read, and approved the submitted version.
Funding
The work presented here was funded by the European Research Council Horizon 2020 project CORALASSIST (Project number 725848). EB and LL received funding from Natural Environment Research Council (NERC) ONE Planet Doctoral Training Partnership (NE/S007512/1). JR received funding from the Women Divers Hall of Fame.
Conflict of Interest
The authors declare that the research was conducted in the absence of any commercial or financial relationships that could be construed as a potential conflict of interest.
Acknowledgments
We are grateful for support and advice from Omaha’s Henry Doorly Zoo and Aquarium and SECORE International. We are indebted to the staff of the Palau International Coral Reef Center for their support during the field work.
Supplementary Material
The Supplementary Material for this article can be found online at: https://www.frontiersin.org/articles/10.3389/fmars.2021.669995/full#supplementary-material
References
Aitken, S. N., and Bemmels, J. B. (2016). Time to get moving: assisted gene flow of forest trees. Evol. Appl. 9, 271–290. doi: 10.1111/eva.12293
Anthony, K., Bay, L. K., Costanza, R., Firn, J., Gunn, J., Harrison, P., et al. (2017). New interventions are needed to save coral reefs. Nat. Ecol. Evol. 1, 1420–1422. doi: 10.1038/s41559-017-0313-5
Baird, A. H., Guest, J. R., and Willis, B. L. (2009). Systematic and biogeographical patterns in the reproductive biology of scleractinian corals. Annu. Rev. Ecol. Evol. Syst. 40, 551–571.
Baird, A. H., Guest, J. R., Edwards, A. J., Bauman, A. G., Bouwmeester, J., Mera, H., et al. (2021). An indo-pacific coral spawning database. Sci. Data 8:35. doi: 10.1038/s41597-020-00793-8
Baird, A. H., Marshall, P. A., and Wolstenholme, J. (2002). “Latitudinal variation in the reproduction of acropora in the coral sea,” in Proceedings of the 9th International Coral Reef Symposium, (Bali).
Baria, M. V. B., dela Cruz, D. W., Villanueva, R. D., and Guest, J. R. (2012). Spawning of three-year-old acropora millepora corals reared from larvae in Northwestern Philippines. Bull. Mar. Sci. 88, 61–62. doi: 10.5343/bms.2011.1075
Baria, M. V. B., Guest, J. R., Edwards, A. J., Aliño, P. M., Heyward, A. J., and Gomez, E. D. (2010). Caging enhances post-settlement survival of juveniles of the scleractinian coral Acropora tenuis. Mar. Ecol. Prog. Ser. 394, 149–153.
Bates, D., Mächler, M., Bolker, B. M., and Walker, S. C. (2015). Fitting linear mixed-effects models using Lme4. J. Stat. Softw. 67. arXiv:1406.5823. doi: 10.18637/jss.v067.i01
Baums, I. B. (2008). A restoration genetics guide for coral reef conservation. Mol. Ecol. 17, 2796–2811. doi: 10.1111/j.1365-294X.2008.03787.x
Baums, I. B., Baker, A. C., Davies, S. W., Grottoli, A. G., Kenkel, C. D., Kitchen, S. A., et al. (2019). Considerations for maximizing the adaptive potential of restored coral populations in the western Atlantic. Ecol. Appl. 29:e01978.
Bay, R. A., and Palumbi, S. R. (2014). Multilocus adaptation associated with heat resistance in reef-building corals. Curr. Biol. 24, 2952–2956. doi: 10.1016/j.cub.2014.10.044
Bay, R. A., Rose, N. H., Logan, C. A., and Palumbi, S. R. (2017). Genomic models predict successful coral adaptation if future ocean warming rates are reduced. Sci. Adv. 3:e1701413. doi: 10.1126/sciadv.1701413
Bongaarts, J. (2019). IPBES, 2019. summary for policymakers of the global assessment report on biodiversity and ecosystem services of the intergovernmental science-policy platform on biodiversity and ecosystem services. Popul. Dev. Rev. 45, 680–681.
Brooks, M. E., Kristensen, K., van Benthem, K. J., Magnusson, A., Berg, C. W., Nielsen, A., et al. (2017). GlmmTMB balances speed and flexibility among packages for zero-inflated generalized linear mixed modeling. R J. 9, 378–400. doi: 10.32614/rj-2017-066
Cameron, K. A., and Harrison, P. L. (2020). Density of coral larvae can influence settlement, post-settlement colony abundance and coral cover in larval restoration. Sci. Rep. 10:5488. doi: 10.1038/s41598-020-62366-4
Ceballos, G., Ehrlich, P. R., Barnosky, A. D., García, A., Pringle, R. M., and Palmer, T. M. (2015). Accelerated modern human–induced species losses: entering the sixth mass extinction. Sci. Adv. 1:e1400253. doi: 10.1126/sciadv.1400253
Chamberland, V. F., Petersen, D., Guest, J. R., Petersen, U., Brittsan, M., and Vermeij, M. J. A. (2017). New seeding approach reduces costs and time to outplant sexually propagated corals for reef restoration. Sci. Rep. 7:18076. doi: 10.1038/s41598-017-17555-z
Chamberland, V. F., Petersen, D., Latijnhouwers, K. R. W., Snowden, S., Mueller, B., and Vermeij, M. J. A. (2016). Four-year-old caribbean acropora colonies reared from field-collected gametes are sexually mature. Bull. Mar. Sci. 92, 263–264. doi: 10.5343/bms.2015.1074
Clark, S., and Edwards, A. J. (1995). Coral transplantation as an aid to reef rehabilitation: evaluation of a case study in the Maldive Islands. Coral Reefs 14:201.
Conlan, J. A., Humphrey, C. A., Severati, A., and Francis, D. S. (2017). Influence of different feeding regimes on the survival, growth, and biochemical composition of Acropora coral recruits. PLoS One 12:e0188568. doi: 10.1371/journal.pone.0188568
Cotto, O., Sandell, L., Chevin, L. M., and Ronce, O. (2019). Maladaptive shifts in life history in a changing environment. Am. Nat. 194, 558–573. doi: 10.1086/702716
Craggs, J., Guest, J. R., Davis, M., Simmons, J., Dashti, E., and Sweet, M. (2017). Inducing broadcast coral spawning ex situ: closed system mesocosm design and husbandry protocol. Ecol. Evol. 7, 11066–11078. doi: 10.1002/ece3.3538
Craggs, J., Guest, J., Bulling, M., and Sweet, M. (2019). Ex situ co culturing of the sea urchin, Mespilia globulus and the coral Acropora millepora enhances early post-settlement survivorship. Sci. Rep. 9:12984. doi: 10.1038/s41598-019-49447-9
Csaszar, N. B. M., Ralph, P. J., Frankham, R., Berkelmans, R., and van Oppen, M. J. H. (2010). Estimating the potential for adaptation of corals to climate warming. PLoS One 5:e9751. doi: 10.1371/journal.pone.0009751
Cunning, R., Gillette, P., Capo, T., Galvez, K., and Baker, A. C. (2015). Growth tradeoffs associated with thermotolerant symbionts in the coral Pocillopora damicornis are lost in warmer oceans. Coral Reefs 34, 155–160. doi: 10.1007/s00338-014-1216-4
de Brito, J., and Kurda, R. (2021). The past and future of sustainable concrete: a critical review and new strategies on cement-based materials. J. Clean. Prod. 281:123558. doi: 10.1016/j.jclepro.2020.123558
dela Cruz, D. W. D., and Harrison, P. L. (2017). Enhanced larval supply and recruitment can replenish reef corals on degraded reefs. Sci. Rep. 7:13985. doi: 10.1038/s41598-017-14546-y
Denison, R. F., Kiers, E. T., and West, S. A. (2003). Darwinian agriculture: when can humans find solutions beyond the reach of natural selection? Q. Rev. Biol. 78, 145–168. doi: 10.1086/374951
Dixon, G. B., Davies, S. W., Aglyamova, G. V., Meyer, E., Bay, L. K., and Matz, M. V. (2015). Genomic determinants of coral heat tolerance across latitudes. Science 348, 1460–1462. doi: 10.1126/science.1261224
Doropoulos, C., Elzinga, J., ter Hofstede, R., van Koningsveld, M., and Babcock, R. C. (2019). Optimizing industrial-scale coral reef restoration: comparing harvesting wild coral spawn slicks and transplanting gravid adult colonies. Restor. Ecol. 27, 758–767. doi: 10.1111/rec.12918
Doropoulos, C., Evensen, N. R., Gomez-Lemos, L. A., and Babcock, R. C. (2017). Density-dependent coral recruitment displays divergent responses during distinct early life-history stages. R Soc. Open Sci. 4:170082. doi: 10.1098/rsos.170082
Doropoulos, C., Roff, G., Bozec, Y.-M., Zupan, M., Werminghausen, J., and Mumby, P. J. (2016). Characterizing the ecological trade-offs throughout the early ontogeny of coral recruitment. Ecol. Monogr. 86, 20–44. doi: 10.1890/15-0668.1
Edmands, S. (2006). Between a rock and a hard place: evaluating the relative risks of inbreeding and outbreeding for conservation and management. Mol. Ecol. 16, 463–475. doi: 10.1111/j.1365-294X.2006.03148.x
Gallagher, C., and Doropoulos, C. (2017). Spatial refugia mediate juvenile coral survival during coral–predator interactions. Coral Reefs. 36, 51–61. doi: 10.1007/s00338-016-1518-9
Gjedrem, T., Robinson, N., and Rye, M. (2012). The importance of selective breeding in aquaculture to meet future demands for animal protein: a review. Aquaculture 35, 117–129. doi: 10.1016/j.aquaculture.2012.04.008
Gomez-Corrales, M., and Prada, C. (2020). Cryptic lineages respond differently to coral bleaching. Mol. Ecol. 29, 4265–4273. doi: 10.1111/mec.15631
Gouezo, M., Doropoulos, C., Fabricius, K., Olsudong, D., Nestor, V., Kurihara, H., et al. (2020). Multispecific coral spawning events and extended breeding periods on an equatorial reef. Coral Reefs 39, 1107–1123. doi: 10.1007/s00338-020-01941-7
Guest, J. R., Baria, M. V., Gomez, E. D., Heyward, A. J., and Edwards, A. J. (2014). Closing the circle: is it feasible to rehabilitate reefs with sexually propagated corals? Coral Reefs 33, 45–55. doi: 10.1007/s00338-013-1114-1
Guest, J. R., Dizon, R. M., Edwards, A. J., Franco, C., and Gomez, E. D. (2011). How quickly do fragments of coral “Self-Attach” after transplantation? Restor. Ecol. 19, 234–242. doi: 10.1111/j.1526-100X.2009.00562.x
Guest, J. R., Heyward, A., Omori, M., Iwao, K., Morse, A. N. C., and Boch, C. (2010). “Rearing coral larvae for reef rehabilitation,” in Reef Rehabilitation Manual, ed. A. Edwards (St. Lucia: Coral Reef Targeted Research & Capacity Building for Management Program), 73–98.
Harrington, D. P., and Fleming, T. R. (1982). A class of rank test procedures for censored survival data. Biometrika 69, 553–566.
Hoegh-Guldberg, O., Jacob, D., Taylor, M., Bindi, M., Brown, S., Camilloni, I., et al. (2018). “Impacts of 1.5°C Global Warming on Natural and Human Systems,” in Global Warming of 1.5°C. An IPCC Special Report on the Impacts of Global Warming of 1.5°C Above Pre-Industrial Levels and Related Global Greenhouse Gas Emission Pathways, in the Context of Strengthening the Global Response to the Threat of Climate Change, Sustainable Development, and Efforts to Eradicate Poverty, eds V. Masson-Delmotte, P. Zhai, H.-O. Pörtner, D. Roberts, J. Skea, P. R. Shukla, et al. (Geneva: Intergovernmental Panel on Climate Change).
Hoegh-Guldberg, O., Jacob, D., Taylor, M., Guillen Bolanos, T., Bindi, M., Brown, S., et al. (2019). The human imperative of stabilizing global climate change at 1.5 degrees C. Science 365:eaaw6974. doi: 10.1126/science.aaw6974
Hollenbeck, C. M., and Johnston, I. A. (2018). Genomic tools and selective breeding in molluscs. Front. Genet. 9:253. doi: 10.3389/fgene.2018.00253
Howells, E. J., Beltran, V. H., Larsen, N. W., Bay, L. K., Willis, B. L., and van Oppen, M. J. H. (2012). Coral thermal tolerance shaped by local adaptation of photosymbionts. Nat. Clim. Chang. 2, 116–120.
Hu, H., and Xiong, L. (2014). Genetic engineering and breeding of drought-resistant crops. Annu. Rev. Plant Biol. 65, 715–741. doi: 10.1146/annurev-arplant-050213-040000
Huffmyer, A. S., Drury, C., Majerová, E., Lemus, J. D., and Gates, R. D. (2021). Tissue fusion and enhanced genotypic diversity support the survival of Pocillopora acuta coral recruits under thermal stress. Coral Reefs 40, 447–458. doi: 10.1007/s00338-021-02074-1
Hughes, T. P., Kerry, J. T., Álvarez-Noriega, M., Álvarez-Romero, J. G., Anderson, K. D., Baird, A. H., et al. (2017). Global warming and recurrent mass bleaching of corals. Nature 543:373. doi: 10.1038/nature21707
Jones, M. E., Jarman, P. J., Lees, C. M., Hesterman, H., Hamede, R. K., Mooney, N. J., et al. (2007). Conservation management of tasmanian devils in the context of an emerging, extinction-threatening disease: devil facial tumor disease. EcoHealth 4, 326–337. doi: 10.1007/s10393-007-0120-6
Jones, T. A., and Monaco, T. A. (2009). A role for assisted evolution in designing native plant materials for domesticated landscapes. Front. Ecol. Environ. 7:541–547. doi: 10.1890/080028
Keith, S. A., Maynard, J. A., Edwards, A. J., Guest, J. R., Bauman, A. G., van Hooidonk, R., et al. (2016). Coral mass spawning predicted by rapid seasonal rise in ocean temperature. Proc. R. Soc. B Biol. Sci. 283:20160011. doi: 10.1098/rspb.2016.0011
Kenkel, C. D., Setta, S. P., and Matz, M. V. (2015). Heritable differences in fitness-related traits among populations of the mustard hill coral, Porites astreoides. Heredity 115, 509–516. doi: 10.1038/hdy.2015.52
Kirk, N. L., Howells, E. J., Abrego, D., Burt, J. A., and Meyer, E. (2018). Genomic and transcriptomic signals of thermal tolerance in heat-tolerant corals (Platygyra daedalea) of the Arabian/Persian Gulf. Mol. Ecol. 27, 5180–5194. doi: 10.1111/mec.14934
Laufkötter, C., Zscheischler, J., and Frölicher, T. L. (2020). High-impact marine heatwaves attributable to human-induced global warming. Science 369, 1621–1625. doi: 10.1126/science.aba0690
Lechene, M. A. A., Haberstroh, A. J., Byrne, M., Figueira, W., and Ferrari, R. (2019). Optimising sampling strategies in coral reefs using large-area mosaics. Remote Sens. 11:2907. doi: 10.3390/rs11242907
Lennon, M. (2015). Nature conservation in the Anthropocene: preservation, restoration and the challenge of novel ecosystems. Plan. Theory Pract. 16, 285–290. doi: 10.1080/14649357.2015.1027047
Liew, Y. J., Howells, E. J., Wang, X., Michell, C. T., Burt, J. A., Idaghdour, Y., et al. (2020). Intergenerational epigenetic inheritance in reef-building corals. Nat. Clim. Chang. 10, 254–259. doi: 10.1038/s41558-019-0687-2
Lough, J. M., Anderson, K. D., and Hughes, T. P. (2018). Increasing thermal stress for tropical coral reefs: 1871-2017. Sci. Rep. 8:6079. doi: 10.1038/s41598-018-24530-9
Lüdecke, D., Makowski, D., Waggoner, P., and Patil, I. (2020). Performance: Assessment of Regression Models Performance. CRAN. Available online at: https://doi.org/10.5281/zenodo.3952174 (accessed January 20, 2021).
Madin, J. S., Baird, A. H., Dornelas, M., and Connolly, S. R. (2014). Mechanical vulnerability explains size-dependent mortality of reef corals. Ecol. Lett. 17, 1008–1015. doi: 10.1111/ele.12306
McClanahan, T. R., Maina, J. M., Darling, E. S., Guillaume, M. M. M., Muthiga, N. A., D’agata, S., et al. (2020). Large geographic variability in the resistance of corals to thermal stress. Glob. Ecol. Biogeogr. 29, 2229–2247. doi: 10.1111/geb.13191
Nakamura, R., Ando, W., Yamamoto, H., Kitano, M., Sato, A., Nakamura, M., et al. (2011). Corals mass-cultured from eggs and transplanted as juveniles to their native, remote coral reef. Mar. Ecol. Prog. Ser. 436, 161–168. doi: 10.3354/meps09257
Okubo, N., Motokawa, T., and Omori, M. (2006). When fragmented coral spawn? Effect of size and timing on survivorship and fecundity of fragmentation in Acropora formosa. Mar. Biol. 151, 353–363. doi: 10.1007/s00227-006-0490-2
Omori, M. (2019). Coral restoration research and technical developments: what we have learned so far. Mar. Biol. Res. 15, 377–409. doi: 10.1080/17451000.2019.1662050
Omori, M., Fukami, H., Kobinata, H., and Hatta, M. (2001). Significant drop in fertilization of Acropora corals in 1999: an after effect of coral bleaching? Limnol. Oceanogr. 46, 704–706.
Ortiz, J. C., González-Rivero, M., and Mumby, P. J. (2013). Can a thermally tolerant symbiont improve the future of Caribbean coral reefs? Glob. Chang. Biol. 19, 273–281. doi: 10.1111/gcb.12027
Parker, L. M., Ross, P. M., O’Connor, W. A., Borysko, L., Raftos, D. A., and Pörtner, H.-O. (2012). Adult exposure influences offspring response to ocean acidification in oysters. Glob. Chang. Biol. 18, 82–92. doi: 10.1111/j.1365-2486.2011.02520.x
Parkinson, J. E., and Baums, I. B. (2014). The extended phenotypes of marine symbioses: ecological and evolutionary consequences of intraspecific genetic diversity in coral-algal associations. Front. Microbiol. 5:445. doi: 10.3389/fmicb.2014.00445
Penland, L., Kloulechad, J., and Idip, D. Jr. (2003). Timing of Coral Spawning in Palau. Toward the Desirable Future of Coral Reefs in Palau and the Western Pacific, 94.
Pollock, F. J., Katz, S. M., van de Water, J. A. J. M., Davies, S. W., Hein, M., Torda, G., et al. (2017). Coral larvae for restoration and research: a large-scale method for rearing Acropora millepora larvae, inducing settlement, and establishing symbiosis. PeerJ 5:e3732. doi: 10.7717/peerj.3732
Pratchett, M. S., Anderson, K. D., Hoogenboom, M. O., Widman, E., Baird, A. H., Pandolfi, J. M., et al. (2015). Spatial, temporal and taxonomic variation in coral growth—implications for the structure and function of coral reef ecosystems. Oceanogr. Mar. Biol. 53, 215–295.
Puill-Stephan, E., Willis, B. L., Abrego, D., Raina, J. B., and Oppen, M. J. H. (2012). Allorecognition maturation in the broadcast-spawning coral Acropora millepora. Coral Reefs 31, 1019–1028. doi: 10.1007/s00338-012-0912-1
Quigley, K. M., Bay, L. K., and van Oppen, M. J. H. (2020). Genome-wide SNP analysis reveals an increase in adaptive genetic variation through selective breeding of coral. Mol. Ecol. 29, 2176–2188. doi: 10.1111/mec.15482
Randall, C. J., Negri, A. P., Quigley, K. M., Foster, T., Ricardo, G. F., Webster, N. S., et al. (2020). Sexual production of corals for reef restoration in the Anthropocene. Mar. Ecol. Prog. Ser. 635, 203–232. doi: 10.3354/meps13206
Rinkevich, B. (2019). Coral chimerism as an evolutionary rescue mechanism to mitigate global climate change impacts. Glob. Chang. Biol. 25, 1198–1206. doi: 10.1111/gcb.14576
Sheridan, C., Kramarsky-Winter, E., Sweet, M., Kushmaro, A., and Leal, M. C. (2013). Diseases in coral aquaculture: causes, implications and preventions. Aquaculture 39, 124–135. doi: 10.1016/j.aquaculture.2013.02.037
Sinclair, E. A., Sherman, C. D. H., Statton, J., Copeland, C., Matthews, A., Waycott, M., et al. (2021). Advances in approaches to seagrass restoration in Australia. Ecol. Manag. Restor. 22, 10–21. doi: 10.1111/emr.12452
Steffen, W., Crutzen, P. J., and McNeill, J. R. (2007). The anthropocene: are humans now overwhelming the great forces of nature? Ambio 36, 614–621. doi: 10.2307/25547826
Suggett, D. J., Edmondson, J., Howlett, L., and Camp, E. F. (2019). Coralclip®: a low-cost solution for rapid and targeted out-planting of coral at scale. Restor. Ecol. 28, 289–296. doi: 10.1111/rec.13070
Thomas, L., Rose, N. H., Bay, R. A., Lopez, E. H., Morikawa, M. K., Ruiz-Jones, L., et al. (2018). Mechanisms of thermal tolerance in reef-building corals across a fine-grained environmental mosaic: lessons from ofu, american samoa. Front. Mar. Sci. 4:434. doi: 10.3389/fmars.2017.00434
van Oppen, M. J., Bongaerts, P., Frade, P., Peplow, L. M., Boyd, S. E., Nim, H. T., et al. (2018). Adaptation to reef habitats through selection on the coral animal and its associated microbiome. Mol. Ecol. 27, 2956–2971.
van Oppen, M. J., Gates, R. D., Blackall, L. L., Cantin, N., Chakravarti, L. J., Chan, W. Y., et al. (2017). Shifting paradigms in restoration of the world’s coral reefs. Glob. Chang. Biol. 23, 3437–3448. doi: 10.1111/gcb.13647
van Oppen, M. J., Oliver, J. K., Putnam, H. M., and Gates, R. D. (2015). Building coral reef resilience through assisted evolution. Proc. Natl. Acad. Sci. U.S.A. 112, 2307–2313. doi: 10.1073/pnas.1422301112
van Woesik, R., Banister, R. B., Bartels, E., Gilliam, D. S., Goergen, E. A., Lustic, C., et al. (2021). Differential survival of nursery-reared Acropora cervicornis outplants along the Florida reef tract. Restor. Ecol. 29:e13302. doi: 10.1111/rec.13302
Vanderklift, M. A., Doropoulos, C., Gorman, D., Leal, I., Minne, A. J. P., Statton, J., et al. (2020). Using propagules to restore coastal marine ecosystems. Front. Mar. Sci. 7:724. doi: 10.3389/fmars.2020.00724
Yetsko, K., Ross, M., Bellantuono, A., Merselis, D., Rodriquez-Lanetty, M., and Gilg, M. R. (2020). Genetic differences in thermal tolerance among colonies of threatened coral Acropora cervicornis: potential for adaptation to increasing temperature. Mar. Ecol. Prog. Ser. 646, 45–68. doi: 10.3354/meps13407
Keywords: selective breeding, larval rearing, nursery, restorative efforts, outplanting, growth, monitoring, survivorship
Citation: Humanes A, Beauchamp EA, Bythell JC, Carl MK, Craggs JR, Edwards AJ, Golbuu Y, Lachs L, Martinez HM, Palmowski P, Paysinger F, Randle JL, van der Steeg E, Sweet M, Treumann A and Guest JR (2021) An Experimental Framework for Selectively Breeding Corals for Assisted Evolution. Front. Mar. Sci. 8:669995. doi: 10.3389/fmars.2021.669995
Received: 19 February 2021; Accepted: 04 May 2021;
Published: 28 May 2021.
Edited by:
Iliana B. Baums, Pennsylvania State University (PSU), United StatesReviewed by:
Zachary Fuller, Columbia University, United StatesCarly Kenkel, University of Southern California, Los Angeles, United States
Copyright © 2021 Humanes, Beauchamp, Bythell, Carl, Craggs, Edwards, Golbuu, Lachs, Martinez, Palmowski, Paysinger, Randle, van der Steeg, Sweet, Treumann and Guest. This is an open-access article distributed under the terms of the Creative Commons Attribution License (CC BY). The use, distribution or reproduction in other forums is permitted, provided the original author(s) and the copyright owner(s) are credited and that the original publication in this journal is cited, in accordance with accepted academic practice. No use, distribution or reproduction is permitted which does not comply with these terms.
*Correspondence: Adriana Humanes, adrihumanes@gmail.com