- 1Cell Technology Laboratory, Institute of Fundamental Medicine, Bashkir State Medical University, Ufa, Russia
- 2Additive Technology Laboratory, Institute of Fundamental Medicine, Bashkir State Medical University, Ufa, Russia
- 3Laboratory of Immunology, Institute of Urology and Clinical Oncology, Bashkir State Medical University, Ufa, Russia
- 4Laboratory of Mathematical modeling, Institute of Fundamental Medicine, Bashkir State Medical University, Ufa, Russia
- 5Institute of Urology and Clinical Oncology, Department of Urology, Bashkir State Medical University, Ufa, Russia
- 6Laboratory for Translational Cellular and Molecular Biomedicine, Tomsk State University, Tomsk, Russia
- 7Institute of Transfusion Medicine and Immunology, Mannheim Institute of Innate Immunosciences (MI3), Medical Faculty Mannheim, Heidelberg University, Mannheim, Germany
- 8German Red Cross Blood Service Baden-Württemberg—Hessen, Mannheim, Germany
The increasing use of medical implants in various areas of medicine, particularly in orthopedic surgery, oncology, cardiology and dentistry, displayed the limitations in long-term integration of available biomaterials. The effective functioning and successful integration of implants requires not only technical excellence of materials but also consideration of the dynamics of biomaterial interaction with the immune system throughout the entire duration of implant use. The acute as well as long-term decisions about the efficiency of implant integration are done by local resident tissue macrophages and monocyte-derived macrophages that start to be recruited during tissue damage, when implant is installed, and are continuously recruited during the healing phase. Our review summarized the knowledge about the currently used macrophages-based in vitro cells system that include murine and human cells lines and primary ex vivo differentiated macrophages. We provided the information about most frequently examined biomarkers for acute inflammation, chronic inflammation, foreign body response and fibrosis, indicating the benefits and limitations of the model systems. Particular attention is given to the scavenging function of macrophages that controls dynamic composition of peri-implant microenvironment and ensures timely clearance of microorganisms, cytokines, metabolites, extracellular matrix components, dying cells as well as implant debris. We outline the perspective for the application of 3D systems for modelling implant interaction with the immune system in human tissue-specific microenvironment avoiding animal experimentation.
1 Introduction
Implants is essential line for therapy in reconstructive and regenerative medicine. Biomaterials for implants construction are constantly under development with the aim to adjust them to the tissue-specific and disease specific conditions, to enhance their biocompatibility, support healing and healthy long-term integration. The physical, chemical and bioactive characteristics of biomaterials have significant impact on the spectrum and scale of tissue responses during acute inflammatory phase that accompany initial installation of the implant. Jamieson et al. found that Al2O3 or ZrO2 ceramic particles can induce IL-1β, IL-8, CCL2, CCL3, CCL4 (C-C motif) ligand)) production in monocyte-like THP1 cells, and toll-like receptor 4 (TLR4) was found to be a principal receptor for this effect (1). The effect of different metal particles of Co-Cr-Mo alloy on macrophages has been shown to induce increased macrophage activity and production of M1-type inflammatory cytokine IL-1β (2). Lei Sun’s et al. investigated role of magnesium in modulating the behavior of macrophages (3). They exposed THP-1 cells to the various concentrations of magnesium and examined the changes in their phenotype. Expression of pro-inflammatory cytokines (tumor necrosis factor alfa (TNF-α) and IL-1 β) was significantly downregulated by magnesium in a time-dependent manner. Magnesium ions were also able to shift THP-1 cells towards M2 phenotype, characterized by enhanced secretion of anti-inflammatory cytokine IL-10 (3).
This influence continues into the resolution of inflammation/healing phase. Moreover, these characteristics play a crucial role in the long-term period, ensuring that an ideal implant does not induce specific tissue reactions and is fully integrated into the local tissue microenvironment. Physical and chemical characteristics of the implant are measurable, and precise methodology is well-established. At the level of the interaction with the biologicals systems, most frequently toxicity of dividing cells and effects of implants on the osteo-integration are examined, while the reaction of immune system on the implant materials are frequently underscored or even neglected. Each tissue in our body is equipped with the natural defense mechanism against foreign substances, where key cells in the foreign body reaction are resident tissue macrophages, that are actively control healthy tissue homeostasis and turnover (4–6).
The properties of biomaterials can promote spectrum immune responses, causing increased inflammation, impaired healing, promotion of fibrotic encapsulation, and tissue destruction, which becomes a cause of implant complications such as peri-implant inflammatory response and implant instability. Various cells such as monocytes/macrophages, dendritic cells (DCs), and neutrophils are involved in biomaterial-induced tissue remodeling that can results in scar formation or loss of function, as well as in the development of chronic inflammatory response, non-healing wounds, fibrosis, and implant failure (7, 8).
Macrophages and neutrophils perform both phagocytic and signaling functions, especially in the initial inflammatory phase of biomaterial implantation. These cell types ultimately determine the outcome of implants in the form of chronic inflammatory response, fibrosis or integration. Other cell types such as DCs, mastocytes, natural killer cells, and intrinsic lymphoid cells may also play an immunomodulatory role in the context of biomaterial implantation (9).
Macrophages are essential innate immune cells that are present in all adult tissues, and dynamically control tissue homeostasis and healthy tissue turn-over (10). In response to trauma and pathogen attack, resident tissue macrophages provide first line defense against the danger, and signal to other innate and adaptive immune cells to be recruited to the site of tissue damage from blood circulation. During the acute inflammatory phase macrophages will primarily secrete the anti-bacterial agents (reactive oxygen species (ROS), lysosomal enzymes) and inflammatory cytokines to amplify the reaction of other immune cells (11, 12). Macrophages have the ability to sense the microenvironmental signals once pathogen attack is eliminated, and to switch on the program of the resolution of inflammation followed by the health phase. At this stage the major activity of macrophages will include release of anti-inflammatory cytokines, extracellular matrix components, induction of somatic cell proliferation and vascularization, and clearance of the debris using scavenging receptors (13). Macrophages have an intrinsic ability to complete the healing phase and to restore the dynamic tissue homeostasis. This ability of macrophages to orchestrate the defense and healing processes in multiple tissues is based on their plasticity in response to changing content of the stimuli (14, 15). However, such fine-tuning can be disturbed by metabolic alterations and by foreign bodies, including implanted biomaterials (5, 16–18). Efficiency of macrophages action depends not only on the resident tissue macrophages, but also on the programming of their pre-coursers circulating monocytes, that are continuously produced by bone marrow and are massively recruited to the damaged tissue already within first hour of the traumatic injury or surgery (19–21). Inflammatory programming of monocytes can be caused by pre-existing infections, exposure to pollution, metabolic disorders or therapeutic interventions. Such inflammatory programs can be detected on transcriptional and epigenetic levels, and will interfere with efficient implant integration.
In this review, focus on the essential steps and processes in implant interaction with resident tissue macrophages, present the state-of-the art in the in vitro or ex vivo modelling of implant/macrophage interactions, and highlight the perspective of developing 3D models to assess macrophage reaction on newly developed biomaterials with high immunocompatibility score.
2 Implant materials and inflammation
2.1 Acute inflammation and the foreign body response
Implantation is always associated with surgical injury and biomaterial implantation will induce a classic pathophysiological acute inflammatory response. In addition, due to their size, shape, surface morphology and chemical properties, biomaterial implants are recognized by the immune system (including bot resident and newly attracted immune cells) as foreign bodies and induce a foreign body reaction (FBR), with the clinical manifestations of reactions depending on the type of implant, its location and individual health status of a patient (22).
In the early stages of implantation, the interaction between blood and biomaterial initiates with protein adsorption on the biomaterial surface and the formation of a temporary matrix, incorporating fibrin (22). This transient matrix provides structural and biochemical components for wound healing and responses to foreign bodies. The chemoattractive properties of chemokines, such as transforming growth factor-beta (TGF-β), platelet-derived growth factor (PDGF), CXCL4, leukotriene B4 (LTB4) and IL-1, attract macrophages to the implantation site (23). Degranulation of mast cells and histamine release also contribute to this process. Macrophage assembly around the implant leads to further recruitment of macrophages, which produce various cytokines, including PDGF, TNF-α, IL-6, G-CSF, and GM-CSF, intensifying inflammatory reactions and foreign body responses. Chemokines like CCL2, CCL4, CCL13, and CCL22 can additionally attract supplementary macrophages to the implantation site. Macrophages arriving at the implantation site may adhere and participate in subsequent FBR and wound healing, transitioning to subsequent phases of inflammation (24). Implants can also induce an inflammatory response through the activation of receptors expressed in both immune and non-immune cells (25). These receptors recognize endogenous signals activated during cell injury. These receptors include Toll-like receptor (TLR), C-type lectin receptor (CLR), retinoic acid inducible gene (RIG)-I-like receptor (RLR) and NOD-like receptor (NLR), IL-1 receptor (IL-1R), IL-6 receptor (IL-6R) and TNF receptor (TNFR). Signaling through these receptors activates an intracellular signaling cascade that leads to nuclear translocation of transcription factors such as activator protein-1 (AP-1) and NF-κB or interferon regulatory factor 3 (IRF3). Stimuli activate immune cells such as macrophages and induce the production of inflammatory cytokines such as IL-1β, IL-6, TNF-α as well as inflammatory proteins and enzymes (25).
The immediate post-implantation response is characterized by precipitation of circulating proteins such as albumin, fibrinogen, fibronectin, gamma globulins, platelet aggregation, activation of the complement system and development of a provisional matrix (26–28). The predominant component of the provisional matrix is fibrin. It has been found that traumatic injury induced by biomaterials installation drives polymerization of fibrin, a major component of blood cloths, on the implant surfaces. This process can lead to the inflammatory response, as the immune system recognizes the opsonized surface the implant as dangerous object (29). In a study of early reactions to a foreign body (FB) in mice, it was found that fibrinogen deficient mice prevented a normal inflammatory reaction until the implant was coated with this protein (26).
Types of immune cells that are involved in the recognition and responses to implants are summarized in Table 1. By investigation of the biomaterials interaction with immune cells, most commonly pro-inflammatory and anti-inflammatory cytokines are taken under consideration, while several essential functions of innate immunity that include clearance of bacteria, tissue debris and apoptotic cells, and necrotic cells do not attract the necessary attention (Figure 1).
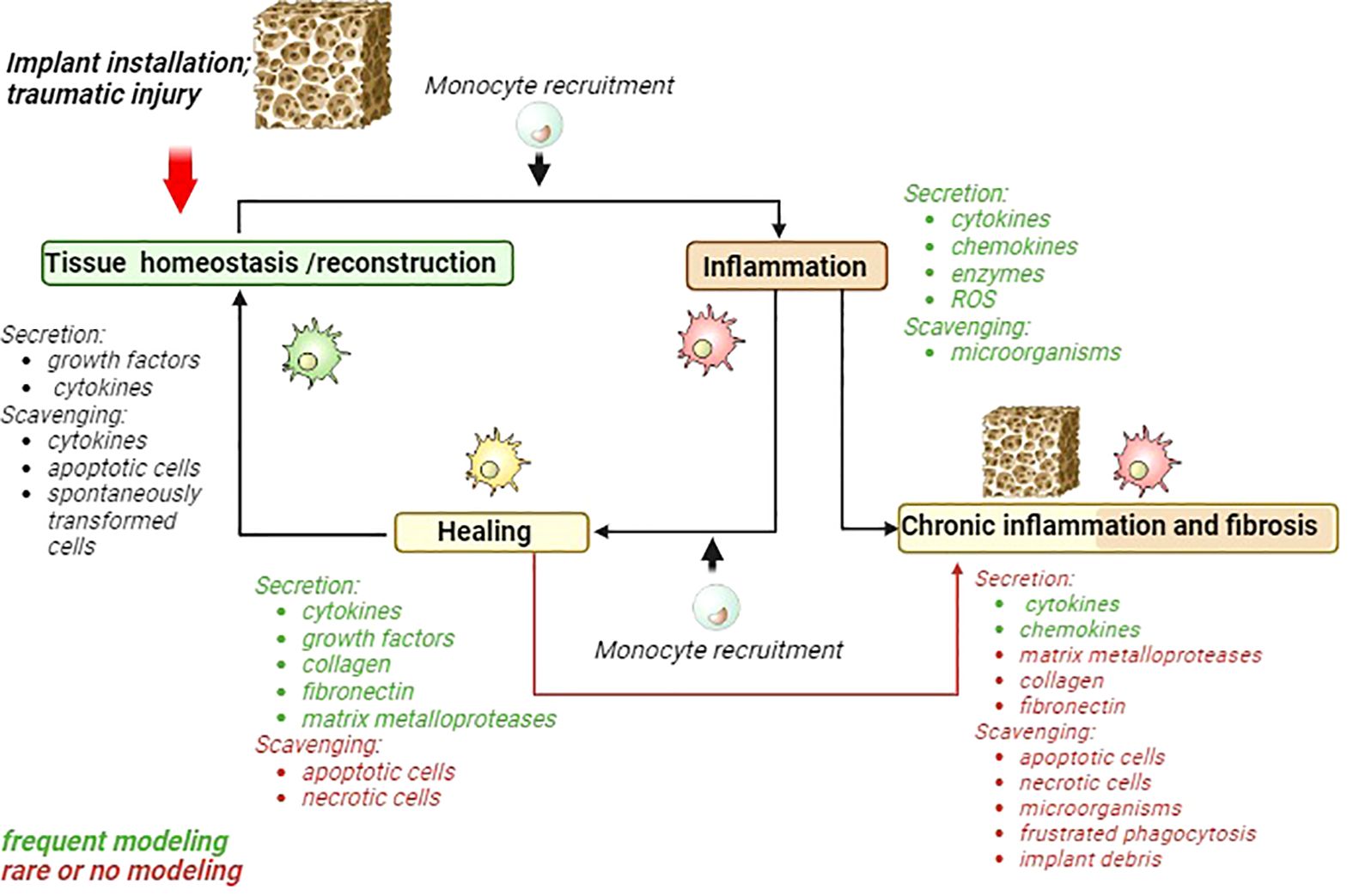
Figure 1 Schematic presentation of acute inflammatory cycle and deviations towards chronic inflammation and fibrosis. Major macrophages activities are listed for each phase. Activities frequently modeled in 2D models are marked green. Activities that are rarely modeled or almost ignored are marked in red. MMP, matrix metalloproteinases; ROS, reactive oxygen species.
In our review we focused on macrophages, key cells that orchestrate inflammation, healing and FBR. Implantation induces recruitment of circulating monocytes and responses of resident tissue macrophages in all tissue types. Upon recognition of a foreign substance, macrophages migrate and adhere to the implant surface (47). The interaction of macrophages with the substrate is mediated by cellular receptors for integrin proteins such as CR3, avß3, a5ß1. Acute inflammatory stage, where macrophages produce TNF-α, IL-1β, IL-6 is followed by resolution and clearance stage and matrix reconstruction stages, that can also lead to tissue fibrotization (5, 48, 49).
In attempt to engulf a foreign body, macrophages can attach to the surface of implant and fuse to form multinucleated cells foreign body giant cells (FBGCs). FBGCs function as macrophages display the ability to phagocytose, to generate oxygen radicals and nitrogen, to produce cytokines and growth factors. High concentration of growth factors, such as TGF-β, around the biomaterial contributes to the transformation of fibroblasts to myofibroblasts (50). In addition, macrophages can promote osteogenesis in the early and middle stages without enhancing matrix mineralization by induction of the expression of BMP-2, RUNX2 (51, 52).
Taken together, macrophages contribute to the necessary inflammatory response, but prolonged pro-inflammatory activation of macrophages leads to the detrimental in case of implant FBR granuloma formation and fibrosis, resulting in chronic inflammation and failure of biomaterial integration.
2.2 Healing phase and frustrated phagocytosis
Macrophages at healing stage are essential for the resolution of inflammation, abrogation of unnecessary immune infiltrate accumulations, scavenging of cell and matrix debris, support of somatic cell proliferation and extracellular matrix reconstruction (13). Macrophage plastic transition from M1 to anti-inflammatory M2 state is pre-requisite for the start of tissue healing, which is needed for the integration of implants (53). M1 and M2 definitions, previously used to describe subpopulations of macrophages, are now mostly applied to indicate the vectors of macrophage polarization toward acute inflammatory rarefactions (M1) or towards healing; anti-inflammatory, homeostatic or tolerogenic phenotypes, that are needed for effective tissue regeneration, but are detrimental in case of cancer (54). M2 macrophages express elevated levels of diverse, tissue specific scavenger receptors, including CD68, CD163, CD206, MARCO, CD36 (55). The common functional feature of SR is internalization molecules, molecular complexes and larger particles and targeting them for lysosomal degradation (56). Major pathway for the SR-mediated internalization include endocytosis and phagocytosis, that in healthy adult tissue are essential for the dynamic tissue homeostasis and clearance of apoptotic bodies, components of extracellular matrix and metabolic ligands. The need for the scavenging function is significantly increased during the phase of resolution of inflammation and healing when amount of apoptotic bodies and unwanted molecules is increased, and macrophage scavenging function will provide the necessary clearance and reconstitution of healthy tissue composition. However, the tolerogenic scavenging activity of macrophage can be converted to the detrimental low grade inflammatory activity by the interaction with implant materials, since scavenger receptor can cooperate with other types of receptors (for example TLRs) and can drive inflammatory signal transduction and cytokine release (57–59). Thus outcome of different SR activities will be the decision of monocytes and macrophage to guard homeostatic balance, or to create chronic inflammatory microenvironment that frequently is further complicated by fibrosis (56). Scavenger receptor on macrophages play an essential role in clearing and removing not only of cellular debris, apoptotic cells, but also fibrin, and growth factors, including epithelial growth factor (EGF) and GDF-15 (55, 60–62). To carry out this analysis, an in vitro endocytosis or phagocytosis tests can be used. In vivo and in vitro tests for phagocytosis are essential for understanding the functionality of the immune system, evaluating the effectiveness of potential therapeutic agents, and studying diseases associated with immune dysfunction (63). The choice between in vivo and in vitro testing depends on specific research objectives and the complexity of the immune response under investigation.
In vivo phagocytosis tests are conducted within a living organism, typically in an animal model (64). The selection of the model depends on specific research requirements. In vivo phagocytosis tests are valuable for comprehending the overall immune response in an organism, simulating a more intricate physiological environment (64, 65). Thus, the study of phagocytosis in vivo was carried out by injecting S. cerevisiae labelled with Congo red into the coelomic cavity of A. altiparanae, then the phagocytic index was measured in fish blood. In addition, phagocytosed and non-phagocytosed yeasts were detected by optical microscopy analysis due to Congo red labelling (66).
In vitro phagocytosis tests can be performed in controlled laboratory conditions outside a living organism (67). In vitro phagocytosis tests are useful for dissecting specific cellular mechanisms involved in phagocytosis and provide a controlled environment for studying interactions between phagocytes and foreign particles (68, 69). Thus in vitro tests allowed us to analyze the phagocytosis of human monocytes cultured with plain latex beads or FITC-labelled Escherichia coli in classically or alternatively activated macrophages (70). The research of phagocytosis function using donor-derived human monocytes allowed us to evaluate the interaction between phosphatidylserine and stabilin-1, and to determine the function of stabilin-1 on alternatively activated macrophages (71). An in vitro study by Onyishi et al. investigated the role of TLR4 in phagocytosis, where they found that loss of TLR4 function increased phagocytosis of unopsonised cryptococci by murine and human macrophages (72).
These tests play a crucial role in advancing our understanding of immune responses and are instrumental in the development and assessment of therapeutic interventions for immune-related disorders. The selection of the testing approach depends on the specific nuances of the research goals and the desired level of experimental control.
However, scavenging function is almost neglected by testing effects of biomaterials with immune system (Figure 1).
Biomaterials can influence the adhesion and migration of monocytes and macrophages, which affects inflammation and regeneration processes. Tests on cell cultures have shown that the composition of the extracellular matrix and adhesion geometry can influence the shape and function of macrophages (73). In vitro tests of mesoporous silica with D(L)-mannose modified surfaces showed that the number of macrophages that attached to the modified surface was about four times higher than to the unmodified surface (74). Tests on the macrophage cell line J774A.1 for pure titanium with polished or grained surfaces showed increased adhesion for rough surfaces (75). Therefore, selection of biomaterials for the implants or developing of new biomaterials requires consideration of their effects on the adhesion of monocytes and resident tissue macrophages.
Majority of studies that model macrophages interaction with biomaterials assess cytokine production of gene expression and secreted levels. During the healing phase macrophage secreted number of cytokines, including IL-1Ra, IL-10, VEGF, PDGF, and TGF-β, which facilitate cell proliferation, osteochondral differentiation, and angiogenesis (76). Frequently applied methods include ELISA, PCR and flow cytometry to quantify production of cytokine and growth factors. Other mediator needed for healing phase include collagen, fibronectin and matrix metalloproteases (MMPs) (Figure 1).
Proinflammatory activation of macrophages directly contacting with the implant surface results in frustrated phagocytosis, leading to local inflammation. This process is often observed in the context of medical implants, where macrophages attempt to engulf the implanted material, but fail due to the size of the implant (5). The presence of frustrated macrophages producing ROS and degradative enzymes may lead to chronic inflammation, fibrosis, and implant instability, which are undesirable for tissue repair and integration (40).
State-of-the art approaches, such as spatial transcriptomics, enable not only identifying immune cell types that migrate to the site of injury but also determinate their spatial distribution in relation to the injury site and to each other. Foster et al.’s study on wound healing in the Rainbow mouse model, with implanted stents, analyzed postoperative days (POD) 2, 7, and 14, with the undamaged skin serving as the control. Using the 10× Genomics Visium method, the study demonstrated the highest levels of activated macrophage markers, such as Msr1, in the wound’s central region at POD 7 (77). The study conducted by Theocharidis et al. investigated wound and peri-wound tissue in patients with both healing and non-healing diabetic foot ulcers. The authors employed spatial single-cell transcriptomic analysis using NanoString’s GeoMx Digital Spatial Profiling platform to reveal that patients with healing wounds exhibited an increased polarization of M1 macrophages, naive and central memory T-cells, while patients with non-healing ulcerative defects had higher levels of M2 macrophages and NK-cells (78). Evident expression of Notch2 around the implanted biomaterial was detected. Selective inhibitors of Notch signaling pathways effectively decreased M1-like macrophages and stimulated M2-like macrophages, through the support of the scaffold (79). Gong et al. investigated the spatial transcriptomics of glial scar formation in spinal cord injury in mice and identified four possible phases of scar formation: macrophage infiltration, proliferation and differentiation of scar-resident cells, scar appearance and the stable scar (80). The primary cell types identified in the scar were microglia, macrophages, astrocytes, oligodendrocytes, fibroblasts, and endothelial cells. Heterogeneity was observed within the macrophage population, and subsequently three subpopulations were identified. The first subpopulation, making up 45.3% of all macrophages, showed high expression of lysozyme (Lyz2), a specific marker for macrophages, as well as thyrotropin-releasing hormone. The second subpopulation, comprising 51.8% of macrophages, exhibited elevated levels of platelet factor 4 (PF4). The third subpopulation comprised 2.9% of the macrophages and exhibited moderate levels of Lyz2 and low levels of PF4. Interestingly, macrophages belonging to subpopulation 2 consistently resided in the central region of the injury site, while those from subpopulation 1 surrounded them. Macrophages from subpopulation 3 were exclusively observed at the periphery of the injury site. The quantity of cells in subpopulation 2 remarkably lowered, while the quantity of cells in subpopulation 3 significantly increased. Of particular importance was the observation that cells from subpopulation 3 mixed with cells from subpopulation 1, creating a circular band of cells. The expression of the macrophage marker PF4 was significantly increased after 3 and 7 days and returned to baseline levels at the intermediate stage (80). Thus, macrophages have a dynamic changes of their activity during the healing process, and such dynamics has to be taken under consideration by modeling of biomaterials/macrophage interactions.
2.3 Chronic inflammation and fibrosis
Many type of cells are involved in various inflammation phases, however macrophages play key regulatory roles at all stages of inflammation initiation and resolution. In chronic inflammation, the interaction between collagen, fibrin, and macrophages plays a crucial role in the pathological process. Collagen and fibrin are key components of the extracellular matrix (ECM)and thrombotic clotting, respectively. M1 macrophages are able to start and sustain inflammatory responses, secreting pro-inflammatory cytokines, activating endothelial cells, and inducing the recruitment of other immune cells into the inflamed tissue; on the other hand, M2 macrophages promote the resolution of inflammation, phagocytose apoptotic cells, drive collagen deposition, coordinate tissue integrity, and release anti-inflammatory mediators (81).
The study on the impact of ECM modification using carboxyethylpyrrole (CEP) on the adhesive properties of M1-polarized macrophages, especially during chronic inflammation, has revealed mechanisms in the context of inflammatory reactions (82). In vitro experiments using BSA revealed that CEP can modify 10-20 lysines within a single protein molecule. Importantly, this modification involves the substitution of positively charged lysines with pyrroles, exposing negatively charged carboxyl groups. Recent findings from the research indicate that the carboxyl group within the CEP structure plays a specific role in binding to integrins αDb2 and αMb2 (82). It was found that CEP modification of ECM proteins, such as collagen IV and laminin, enhances their adhesive properties to M1 macrophages, particularly through integrin αDβ2. This contributes to the retention of M1 macrophages at the site of inflammation and may be associated with detrimental processes during chronic inflammation, autoimmunity, and other pathological conditions (82).
The impact of tissue density changes on macrophage activation and functions remains poorly understood. In the study conducted by Sapudom et al., THP-1 monocytic cells were incorporated into three-dimensional collagen matrices with varying fibril density and differentiated into macrophages using PMA (83). Subsequent activation (MLPS/IFNγ and MIL-4/IL-13) induced differences in cytokine secretion profiles, favoring IL-1β and TNFα in MLPS/IFNγ and IL-6 in MIL-4/IL-13. Notably, cytokine secretion increased with higher fibril density (40). It was found that M1LPS/IFNγ enhanced monocyte tissue infiltration, while MIL-4/IL-13 supported fibroblast differentiation into myofibroblasts via TGF-β1, depending on fibril density, indicative of an M2a-like phenotype (83).
In study Hsieh et al. have observed that fibrin and its precursor, fibrinogen, elicit distinctive functions in macrophages (84). When macrophages were cultured on fibrin gels created by combining fibrinogen with thrombin, it stimulated the secretion of the anti-inflammatory cytokine, IL-10. In contrast, exposing macrophages to soluble fibrinogen led to a significant increase in the production of the inflammatory cytokine, TNF-α. Importantly, when macrophages were cultured on fibrin gels in the presence of soluble fibrinogen, they maintained their anti-inflammatory characteristics. Additionally, adhesion to fibrin matrices inhibited TNF-α production in response to stimuli such as LPS and IFNγ, well-known for promoting inflammatory macrophage polarization (84). These findings reveal that fibrin plays a protective role in macrophage function, preventing their inflammatory activation triggered by various factors, including fibrinogen, LPS, and IFN-γ. This study suggests that the presentation of fibrinogen could serve as a critical regulator of macrophage behavior, offering a valuable immunomodulatory strategy for tissue healing and regeneration.
In the study Rudnic et al. focused on systemic sclerosis (SSc), an autoimmune disease characterized by excessive ECM production and multiorgan fibrosis (85). The role of monocytes and macrophages in SSc fibrogenesis was unclear. Immunohistochemistry found CD14+ monocytes in collagen-rich areas, along with alpha-SMA+ fibroblasts, CD68+, and man-nose receptor+ macrophages in SSc patients’ hearts and lungs. CD14+ monocyte transcriptomics revealed dysregulation in cytoskeleton, ECM, FN1 gene, and TGF-β signaling. Single-cell RNA sequencing showed activated profibrotic signature in CD14+ pulmonary macrophages from SSc patients with lung disease. Profibrotic cytokine exposure increased type I collagen, fibronectin, αSMA in CD14+ monocytes. Co-culture with dermal fibroblasts amplified profibrotic markers. TGF-β pathway inhibitors reduced fibronectin and collagen secretion. The study provided evidence for CD14+ monocytes/macrophages as ECM producers (85).
The cytokine and chemokines parameters are often examined in the of chronic inflammation phase (Figure 1). The collagen, fibronectin, MMPs and scavenging function apoptotic, necrotic cells, microorganisms, frustrated phagocytosis and implant debris are underscored in their significance (Figure 1).
Acute and chronic inflammation can lead to fibrosis, with macrophages playing a crucial role in fibrotic processes through the release of mediators such as TGF-β1 and PDGF. These mediators contribute to fibroblast migration, proliferation, and collagen synthesis, thereby promoting fibrosis (86, 87). Macrophages also play a vital role in the production and regulation of matrix MMPs, enzymes responsible for degrading various components of the ECM. Matrix metalloproteinases are produced by immune cells, fibroblasts, endothelial cells, osteoclasts (88). In our manuscript, we focused on macrophages MMPs, which play a role in ECM remodeling by degrading ECM and promoting angiogenesis and tissue remodeling.
The production of MMPs is activated in response to pathogens, TNF-α and other inflammatory mediators, with different MMPs playing different roles in the inflammatory response (89). In particular, MMP-9 has been implicated in tissue damage and biomaterial degradation (90). Reiss et al. established a murine wound model to investigate the effect of MMP-9 on chronic wound healing and demonstrated a delayed healing process in the presence of MMP-9 (91). MMP-12, also known as macrophage elastase, is a zinc-dependent protein that is critical for tissue remodeling (13). Madala et al. found that MMP-12 deficiency leads to increased expression of other ECM-degrading MMPs such as MMP-2, MMP-9 and MMP-13. This upregulation of MMP expression may limit the degradation of ECM components, thereby reducing the development of fibrosis (92). Stawski et al. utilized MMP12KO mice to assess the contribution of MMP-12 to vascular injury and fibrosis in the angiotensin II (Ang II) model (93). They observed that MMP-12 deficiency inhibited Ang II-induced production of TGF-β1, a profibrotic mediator, in the skin and perivascular regions of the heart. In Ang II-infused MMP12KO mice, TGF-β1-positive cells were significantly decreased in the perivascular regions of the heart but not in the interstitium (93).
Chitinases, including Chitinase 1 (CHIT1), chitinase 3-like-1 (CHI3L1) are implicated in the regulation of fibrosis, a pathological condition characterized by the excessive accumulation of ECM proteins leading to scarring and organ damage (94, 95). Lee et al. demonstrated that CHIT1 is required for the development of pulmonary fibrosis, and TGF-β1 plays a critical role in this process (94). They used wild type and CHIT1 null mutant mice, and demonstrated that TGF-β1 stimulated the production of ECM proteins such as fibronectin and type 1 collagen in a CHIT1 dependent manner. Furthermore, the fibrotic responses were exaggerated in mice lungs in which both CHIT1 and TGF-β1 were expressed simultaneously compared to mice in which each factors were expressed individually (94). CHI3L1 has been implicated in fibrosis and inflammation, particularly in diseases characterized by tissue remodeling. Research suggests that CHI3L1 plays an important role in fibroproliferative responses, with increased expression observed in alveolar macrophages and bronchiolar epithelial cells adjacent to fibrotic lesions (95).
As evident from the presented information, chitinases play a role in fibrosis. However, for a deeper understanding of their impact, further research is necessary. Continuing the investigation of chitinase functions in the context of fibrosis is crucial to uncover their potential roles and contribute to our overall comprehension of these processes.
3 In vitro test systems for analysis of interaction between implant materials and macrophages
The purpose of using cell system models for the analysis of implant materials is to evaluate the complex interactions between cells and implant in controlled laboratory conditions. Various cell types can be used for this purpose, including murine cell lines such as RAW 264.7 and J774A.1, primary bone marrow-derived murine macrophages (BMDM), human macrophage cell lines (THP-1, U937) and human primary monocyte-derived macrophages. It is crucial to emphasize that every cell system model has inherent limitations, and selection of cell models depends on the medical implant application and the type of tissue that the material will interact with.
3.1 Murine cells lines
The use of murine cell lines, such as RAW 264.7 and J774A.1 (Table 2), is an important tool for analyzing the effects of materials intended for implantation. These types of cell lines are widely employed to study immune responses and inflammation during the testing of implant with different compositions and modifications (96).
These cells enable controlled laboratory experiments to assess parameters such as morphology, size, viability, cytokine levels, and gene expression. Researchers found that changes in the surface topography of the materials influences macrophage behavior and the production of inflammatory cytokines (98).
The RAW 264.7 cell line is a murine macrophage lineage derived from a tumor in a male mouse exposed to the Abelson leukemia virus. Using this cell line, Ali K. Refai et al. demonstrated that the topography of titanium surfaces significantly influences macrophage activation and their secretion of pro-inflammatory cytokines and chemokines (96). Macrophages attached to rough surfaces (acid etching and SLA) without stimulation increased the secretion of TNF-α. For macrophages stimulated with LPS, the roughest surface (SLA) led to higher levels of IL-1β, IL-6, and TNF-α at 24 and 48 hours compared to all other surfaces (96). This suggests that surface topography can modulate the expression of anti-inflammatory cytokines and chemokines by macrophages over time.
In the study by Sun et al., RAW 264.7 macrophages were cultured on layers of TiO2 nanotubes, and their morphology, adhesion, viability, and expression of BMP-2 and TGF-β1 were assessed in vitro (97). The study showed that macrophages grown on larger nanotube layers (120 nm) had elongated morphology and weak adhesion to the nanotube layers compared to control disks after four hours of incubation. Interestingly, macrophages remained viable on smaller nanotube layers (30 and 70 nm) even after 24 hours of incubation. Another significant finding was that increasing the nanotube diameter led to enhanced BMP-2 mRNA expression and increased BMP-2 protein secretion (97). This confirms that the TiO2 nanotube surface can influence BMP-2 expression in macrophages, potentially contributing to bone formation during regeneration.
Yizhou Zhu et al. demonstrated that the topography of TiO2 surfaces resembling honeycombs can influence macrophage polarization, a process in which macrophages transition between different phenotypes (98). Researchers created four scales of TiO2 structures resembling honeycombs on titanium substrates to study the cellular behavior of RAW 264.7 macrophages and their immunomodulation on osteogenesis. They found that reduced-scale TiO2 structures significantly activated the anti-inflammatory macrophage phenotype (M2). This was evidenced by the 90 nm diameter sample inducing the highest expression of CD206, IL-4, IL4-10, and releasing the greatest amount of BMP-2. The study suggests that by manipulating the surface topography of biomaterials, macrophage polarization can be controlled, enhancing implant osseointegration (98).
Meng et al. identified that ZnO nanoparticles can reduce inflammatory osteolysis by regulating the MEK-ERK-COX-2 signaling pathway (99). They found that ZnO nanoparticles inhibit MEK and ERK activation, leading to a reduction in COX-2 production. This decrease of COX-2 production results in reduced inflammation and bone resorption (99).
By modulating the inflammatory pathway, it is possible to reduce inflammation and alleviate symptoms of implant failure. Deng Z. et al. found that TiPs and CoPs can induce an inflammatory response during aseptic loosening through SIRT1-deacetylated NF-κB, causing its activation and subsequent inflammatory response (100). Mao et al. investigated the influence of bortezomib on inflammation modulation (101). RAW 264.7 cells grown with titanium particles and bortezomib showed increased expression of several inflammatory cytokines and enzymes, such as TNF-α, IL-1β, IL-6, MCP1, iNOS, and COX-2. In contrast, bortezomib treatment significantly reduced the expression of these inflammatory molecules in RAW 264.7 cells and induced IL-10 expression (101). These data suggest that bortezomib may inhibit inflammation induced by titanium particles in these cells.
J774A.1 cells is a cell line derived from the ascites of an adult female mouse with reticulum cell sarcoma. This cell line is not extensively utilized for investigating the immunological response to materials. The impact of titanium surface topography on the polarization, production of inflammatory cytokines, and nitric oxide in J774A.1 macrophages was elucidated by Tan et al. (102). Their study revealed that the topography of titanium surfaces can directly influence macrophage polarization, subsequently affecting the production of inflammatory cytokines and nitric oxide. Takebe et al. demonstrated that titanium surface topography has the potential to alter the morphology of J774A.1 macrophages, and these changes in cell shape could potentially impact the behavior and function of the cells (75). Additionally, titanium surface topography was found to modulate the expression of BMP-2 in J774A.1 macrophages, a protein crucial in bone remodeling. In contrast, Jakobsen et al. investigated the effects of hydroxyapatite (HA) coatings on the secretion of TGF-β and BMP-2 in murine J774A.1 macrophages (103). J774A.1 cells were exposed to TiAlV coating with or without HA, and the secretion of TGF-β and BMP-2 was monitored over time. The HA coatings did not significantly enhanced the secretion of TGF-β and BMP-2 in macrophages. However, these coatings did induce a pro-inflammatory cytokine response (103).
In conclusion, murine macrophage cell lines remain widely used for biomaterial testing. However, there are inherent limitations associated with these cells. For instance, RAW 264.7 cells exhibit genetic instability, potentially leading to alterations in cellular phenotype and function, thereby introducing variability that may impact experimental outcomes (104). Furthermore, the temporal utility of cell lines is constrained by their finite capacity for cell division. Additionally, the behavior of murine macrophages in biomaterial and inflammatory models may not fully align, posing challenges in the interpretation of experimental results. The murine origin of these cells imposes limitations in terms of data representation and interpretation within the broader context of biological systems.
3.2 Murine primary cells
BMDM provide a powerful tool for analyzing implant materials and enable the assessment of both pro-inflammatory and anti-inflammatory responses. The use of BMDM allows for the modulation of inflammation and implant-associated infections (Table 3). Using this cell model, Pearl et al. hypothesized that implant wear debris particles may act as pathogen-associated molecular patterns (PAMPs) or damage-associated molecular patterns (DAMPs), activating macrophages through TLR signaling (105). This activation leads to the secretion of TNF-α. For example, inhibiting the MyD88 protein, which plays a role in the TLR signaling pathway, reduces TNF-α production in response to polymethylmethacrylate (PMMA) particle-induced inflammation in BMDM (105).
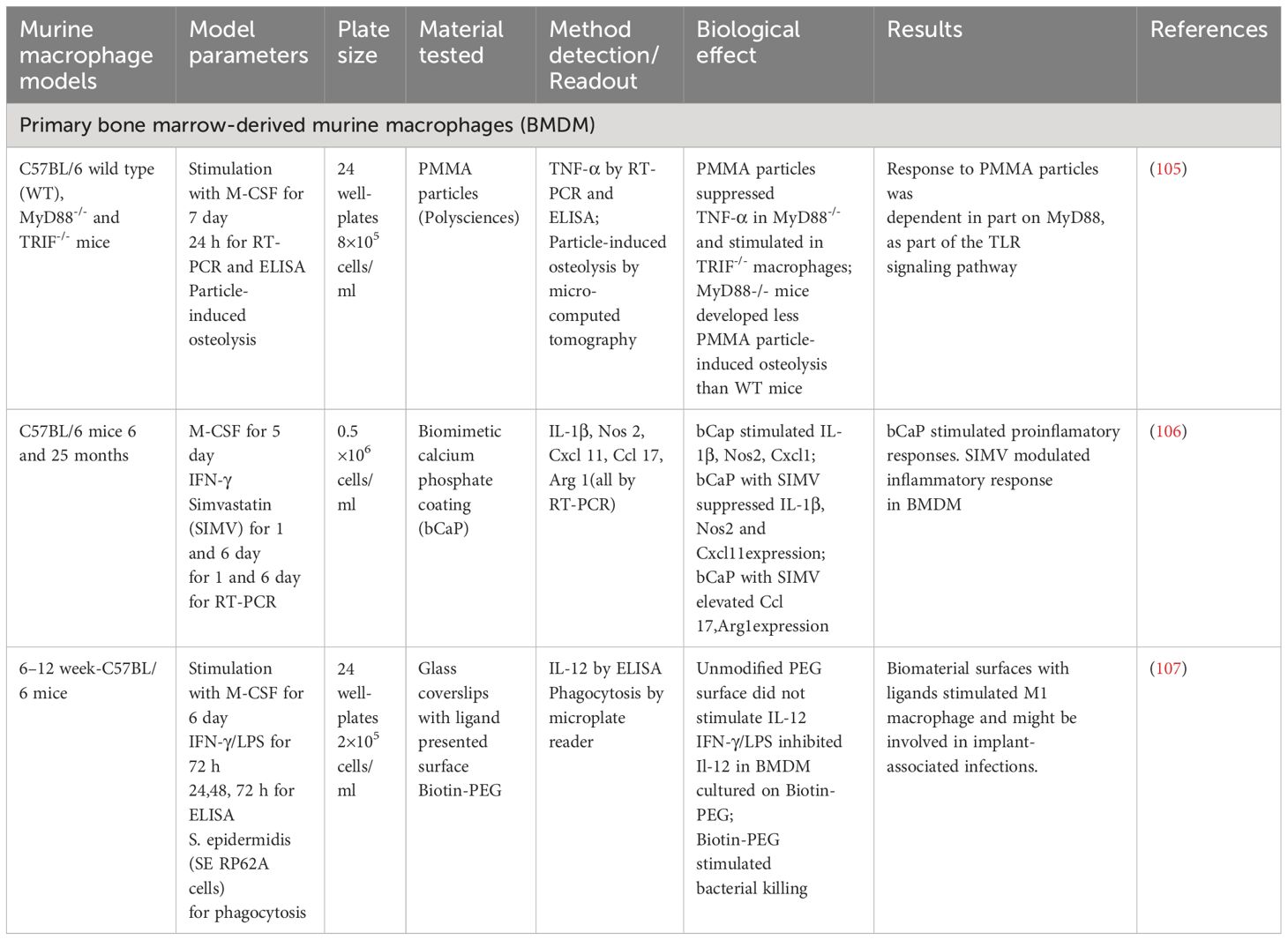
Table 3 Test systems for analysis of implant materials with Primary bone marrow-derived murine macrophages.
Alhamdi et al. explored a novel approach to control macrophage activation, especially in the context of bone healing in older adults (106). The researchers developed a biomimetic calcium phosphate coating (bCaP) that physically and temporally separated a pro-inflammatory stimulus such as IFNγ and a reparative stimulus like simvastatin (SIMV). The bCaP coating stimulated the expression of anti-inflammatory genes (IL-1β, Nos 2, Cxcl 11) in BMDM and reduced the expression of Ccl17 and Arg1. Conversely, the bCaP coating in the presence of SIMV stimulated the expression of Ccl17 and Arg1, as anti-inflammatory markers of macrophages, and reduced the expression of IL-1β, Nos 2, Cxcl 11 in BMDM. The study provided promising evidence that SIMV could be used to control macrophage activation, potentially improving bone healing (106). However, further research is needed to fully understand the involved mechanisms and explore the potential clinical applications of this approach.
Park et al. modulated a murine in vitro system to investigate the role of M1 macrophages in interactions with Staphylococcus epidermidis-associated implant infections (107). In this study, a biotin-PEG-based substrate was used. The unmodified PEG surface did not stimulate IL-12 production in BMDM. However, biotin-PEG promoted a decrease in IL-12 secretion in BMDM and stimulated bacterial killing (107).
The C57BL/6 mouse strain is one of the most widely used for material testing utilizing BMDM. However, some published data suggested that C57BL/6 mice exhibited high level of innate and adaptive immune responses (108, 109). It may exhibit different behavioral and physiological responses and lead to inaccurate results and misleading conclusion.
3.3 Human cell lines
Among human cell lines, the monocyte cell line derived from peripheral blood (THP-1) is frequently used as a macrophage model in studies investigating the healing processes of implants (Table 4). This ensures biological relevance. Additionally, the use of the THP-1 cell line provides a relatively straightforward and standardized method, ensuring reproducibility. Cell lines can be induced into macrophages using Phorbol-12-myristate-13-acetate (PMA), and both stimulated and non-stimulated cell lines are utilized. Another commonly employed cell line is the U937 monocyte cell line derived from human bone marrow (Table 4), which can also be induced into macrophages. Both cell lines serve as models for studying immune processes and inflammation in the context of tissue healing.
THP-1 and U937 cells are often used in similar experiments but may exhibit some differences in responses to various stimulants, attributed to their distinct origins and histories.
Employing various methods (ELISA, RT-PCR, XTT, flow cytometry, etc.), these cell models allow for the examination of cell survival in immune peri-implant tissues, their adhesion and migration capabilities (Figure 1). Most commonly, the models focus on the production of inflammatory cytokines. In the following study soluble cobalt, chromium, titanium, and molybdenum were administered to PMA-stimulated THP-1 macrophages, which were previously primed with LPS (110–112, 114). Studies have demonstrated a significant increase in pro-inflammatory cytokines and GM-CSF induced by titanium (Ti) ions, indicating an inflammatory response. Interestingly, the levels of other cytokines, specifically IL-1α, IL-2, IL-4, IL-12, IL-17α, and TNF-α, remained largely unaltered. Furthermore, titanium ions were discovered to stimulate inflammasome activation in human macrophages, revealing findings regarding their immunomodulatory potential (110–112, 114).
In study PMA-stimulated THP-1 macrophages were grown on titanium discs that had been coated with hydroxyapatite (Ti-HA) and β-tricalcium phosphate (Ti-β-TCP) (113). Both coatings led to a significant increase in cytokine secretion of TNF-α and TGF-β, as well as expression of marker genes for M1 and M2 macrophages. It is worth noting that Ti-HA coating caused an earlier polarization of M1 macrophages and showed greater M2 phenotype potential compared to Ti-β-TCP. In another study THP-1 macrophages stimulated with PMA were exposed to magnesium particles. The findings indicated that magnesium particles decreased the production of IL-1β, TNF-α, IL-10, and CCR7, while improving the expression of CD206 and CCR7. This suggests that magnesium particles have the capability to transform macrophages to an M2 type (3).
In U937 cells, which were cultured on porous cellulose nanofibril (CNF) substrates, the expression of several pro-inflammatory cytokines was significantly increased, while there was no significant change in anti-inflammatory cytokines (116). On the fourth day of in vitro culture, CNF scaffolds demonstrated significantly increased expression of anti-inflammatory IL-1Ra and IL-10 genes. It is noteworthy that the production of inflammatory cytokines IL-1β, IL-6, MCP-1, MIP-1α, CXCL-1, and M-CSF was significantly lower in CNF scaffolds, indicating an early and weak inflammatory response (116). U937 cells, exposed to PDBu and Vitamin D3, were cultivated on samples of hydroxyapatite (HA) and tricalcium phosphate (TCP) (117). Cell viability was consistent across all samples, and multinucleated cells that tested positive for TRAP were present on both HA and TCP surfaces, with no significant distinction.
However, it is essential to note that such models may not fully replicate the complex interactions within the organism, and results may be context-dependent. Therefore, they should be complemented and validated with data obtained from more sophisticated research systems. Such as three-dimensional (3D) cell models are an alternative to two-dimensional (2D) cell culture models that have the potential to be more physiologically relevant. Commonly used models include the generation of spheroids and organoids, bio scaffolds based on synthetic (polyacrylamide or polyethylene glycol (PEG) or natural polymers (gelatin, collagen) (118–120). 3D bioprinting techniques are outstanding for scaffold fabrication due to their ability to create porous structures with interconnected cells and growth factors for in vitro and in vivo evaluation as preclinical assessments (121). Organs-on-a-chip (OOCs) technology has been increasingly used to study the immune system, providing a more realistic in vitro environment compared to traditional 2D cultures. OOCs technology is used in the study of bone marrow, spleen, can also modulate inflammation (122–124).
These studies collectively emphasize the diverse nature of macrophages responses to a broad range of stimuli. Understanding this plasticity is crucial in uncovering the complexities of macrophage function in different physiological and pathological settings, highlighting the significant role of cellular models in immunomodulatory research.
3.4 Human primary macrophages cells lines
To understand the regulation of immune and inflammatory responses with implants, an important model for researchers is monocyte-derived macrophages differentiated on biomaterial surfaces.
In contrast to the aforementioned cell lines, human primary macrophages more accurately reflect the physiological characteristics of human tissues, as they are directly derived from the human organism. This ensures a more reliable reproduction of real conditions in tissues. Additionally, primary macrophages maintain the heterogeneity inherent in the human body. Therefore, investigating implant materials on patient-derived cell lines may enable the prediction of the inflammatory response to the implant within the patient’s body (125). However, acquiring human primary macrophages is a labor-intensive process, and differences between donors persist. When utilizing human primary macrophages, it is also possible to trace immune reactions to implant installation (Table 5). The investigation into implant using monocyte-derived macrophages M0, M1, and M2, differentiated on PAR/HA and PAR/HA+CAT surfaces, illustrated that these substances decrease TNF-α production and CD206 level. This suggests the implant have the potential to restrict inflammatory processes (126). However, the use of these materials also resulted in an increase in the level of IL6 in some donors, revealing the complexities in regulating immune responses (126).
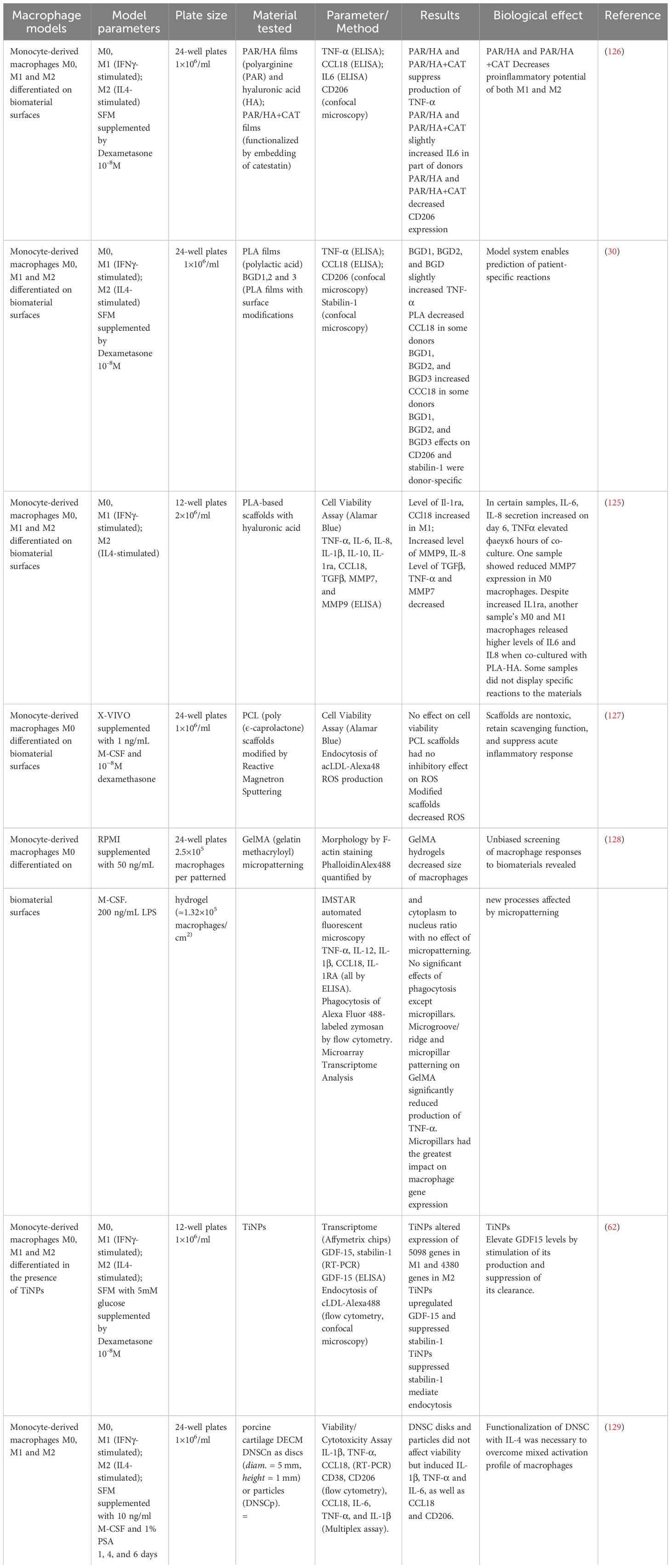
Table 5 Test systems for analysis of interaction of implant materials with human primary macrophages.
In the research conducted on polycaprolactone (PLA) implant, which analyzed several modifications of PLA films (BGD1, BGD2, and BGD3), disparate effects on M0 macrophages were observed (30). These adjustments raised TNF-α levels while also having donor-specific impacts on CCL18 (30).
For the investigation of PCL implant, M0 macrophages were subjected to micro-patterned PCL hydrogels (127). The findings suggest PCL scaffolds do not present toxicity and are capable of reducing ROS levels. This can be highly significant in curbing inflammatory responses (127).
The effects of micro-patterning on GelMA hydrogels were investigated in a study. The study found that micro-patterning had an impact on both macrophage size and TNF-α level (128). Furthermore, the researchers identified novel processes that could potentially be influenced by micro-patterning, highlighting the significance of this methodology in medical tissue engineering and implantology (128).
These studies indicate the potential of biomaterials and their modifications for regulating immune and inflammatory responses. This may provide a foundation for developing more effective and personalized strategies in medical tissue engineering and implantology.
4 Current 3D model for biomaterial testing
As of today, it is acknowledged that cell cultures in 2D models may not always faithfully represent the physiological complexity of tissues, which are structurally intricate, cellularly heterogeneous, and dynamically changing over time within the human body (130). Contemporary 3D cell culture models are gaining popularity due to their ability to achieve a higher degree of cell differentiation and tissue organization compared to 2D culture systems.
Various techniques exist for creating 3D cell cultures, such as spheroid culture, biopolymer scaffolds, microfluidics, and organs-on-chip, aiming to replicate and mimic in vivo systems (Table 6). Several studies have investigated the influence of 3D scaffolds made from different materials on the polarization of macrophages and immune responses to implants (Table 6). Almeida et al. focused on the effects of 3D-printed Polylactic Acid (PLA) and chitosan-based scaffolds on human monocyte/macrophage responses (131). PLA-based and chitosan scaffolds increased TNF-α secretion. Despite PLA-based scaffolds inducing higher production of interleukins IL-6, IL-12/23, and IL-10, chitosan scaffolds with larger porous structures and wider angles influenced cellular responses and pro-inflammatory cytokine secretion.
Barker et al. developed a 3D oral mucosal model by combining human oral fibroblasts, OKF6/TERT-2 keratinocytes, and THP-1 cells (132). Implants (TiZr-SLA, TiZr-M, ZrO2-M, PEEK-M) were inserted into the tissue-engineered oral mucosa following a 4 mm punch biopsy. Inflammation was simulated by adding LPS from E. coli and TNF-α to the culture medium. Histological data showed that the inflamed oral mucosa model closely mimicked the in vivo situation, with a 3D dimensional structure comprising the connective tissue collagen layer containing fibroblasts and monocytes and a distinct epithelial layer with multi-layered stratified oral keratinocytes (132).
Barthes et al. presented a study addressing potential adverse effects of 3D-printed silicone implants, such as tracheal defect repair due to immune reactions (134). The study focused on controlling the implant/host interface using immunomodulatory coatings. The researchers designed a new cytokine cocktail composed of interleukin-10 and prostaglandin-E2, aiming to decrease adverse immune reactions and promote tissue integration by fixing macrophages into an M2 pro-healing phenotype for an extended period. The study concluded that the ability of this new immunomodulatory hydrogel to control inflammation levels once applied to a 3D-printed silicone implant has been demonstrated (134).
Li et al. described a 3D model for foreign body response and bone regeneration (30). 3D-printed scaffolds were prepared using a combination of polycaprolactone (PCL), polyethylene glycol (PEG), and hydroxyapatite, focusing on various pore sizes of the scaffolds (P200, P400, P600). This 3D model demonstrated that P600 pore size significantly reduced the foreign body response and induced a more M2 macrophage phenotype, vascular ingrowth, and new bone formation (137).
Wei X. et al. also established a 3D model for bone regeneration using Polyetheretherketone (PEEK) (138). PEEK scaffolds were manufactured via fused deposition modeling, and a polydopamine (PDA) coating chelated with magnesium ions was applied to the surface. In vitro results showed that the activated surface promoted cell proliferation and adhesion, contributing to osteoblast differentiation and mineralization. The released magnesium ions also promoted angiogenesis (138).
These findings suggest that the design of 3D-printed scaffolds, including material selection and control of pore size and coating, can be optimized to enhance the effectiveness of tissue engineering and regenerative medicine. Recently, microphysiological systems, known as OOCs technology, enable the study of physiological processes in the human body using modern models for diseases of the lung, liver, kidney, gut, brain, bone marrow, kidneys, tumor-on-chip, and drug testing (139, 140). Sharifi F. et al. created an in vitro microfluidic platform to reproduce the dynamic effects of human primary monocytes and THP-1 cell line on Ti microbeads occurring in foreign body responses (133). They proposed that this platform would be a valuable tool for studying the immune foreign bodies response.
Organoid technology is a rapidly advancing field, providing a platform for the study of cancer behavior, drug discovery and testing, disease modeling, and host-microbiome interaction (131). Bone organoids represent a novel concept in tissue engineering. Iordachescu A. et al. constructed trabecular bone organoids using primary female osteoblastic and osteoclastic cells, seeded onto femoral head micro-trabeculae (141). These cells recapitulate relevant phenotypes and functions. Once the organoids are constructed, they are inserted into a simulated microgravity bioreactor to model a pathological state of reduced mechanical stimulation. In these constructs, osteoclastic bone resorption sites can be detected, which differ in morphology in the simulated microgravity group compared to static controls. In conclusion, bone organoids represent a promising approach for studying bone regeneration and repair.
3D bioprinting is a rapidly evolving field with the potential to revolutionize biomaterial testing and tissue engineering. It involves the layer-by-layer deposition of biological materials to create complex structures that mimic natural tissues or organs (142). This technology can be employed for material testing in various ways. In the context of bone modeling, 3D bioprinting can be used to fabricate scaffolds for bone regeneration. These scaffolds can be composed of hydrogel materials, which are ideal for this purpose, due to their controllable biological and biophysical properties (143). Hydrogels can support the attachment, proliferation, migration, and differentiation of cells, crucial for the regeneration of bone tissue.
Briefly, the exploration of 3D cell culture models, with techniques like spheroid culture, biopolymer scaffolds, microfluidics, and organs-on-chip, has shown their potential for more physiologically relevant platforms compared to traditional 2D models. Optimizing 3D-printed scaffolds through material selection, pore size control, and coating is critical for improving tissue engineering efficacy. Advanced microphysiological systems, particularly OOCs offer innovative approaches for studying various organs and tissues. Bone organoids and 3D bioprinting applications in bone modeling demonstrate these technologies’ versatility in advancing understanding of bone regeneration (Figure 2).
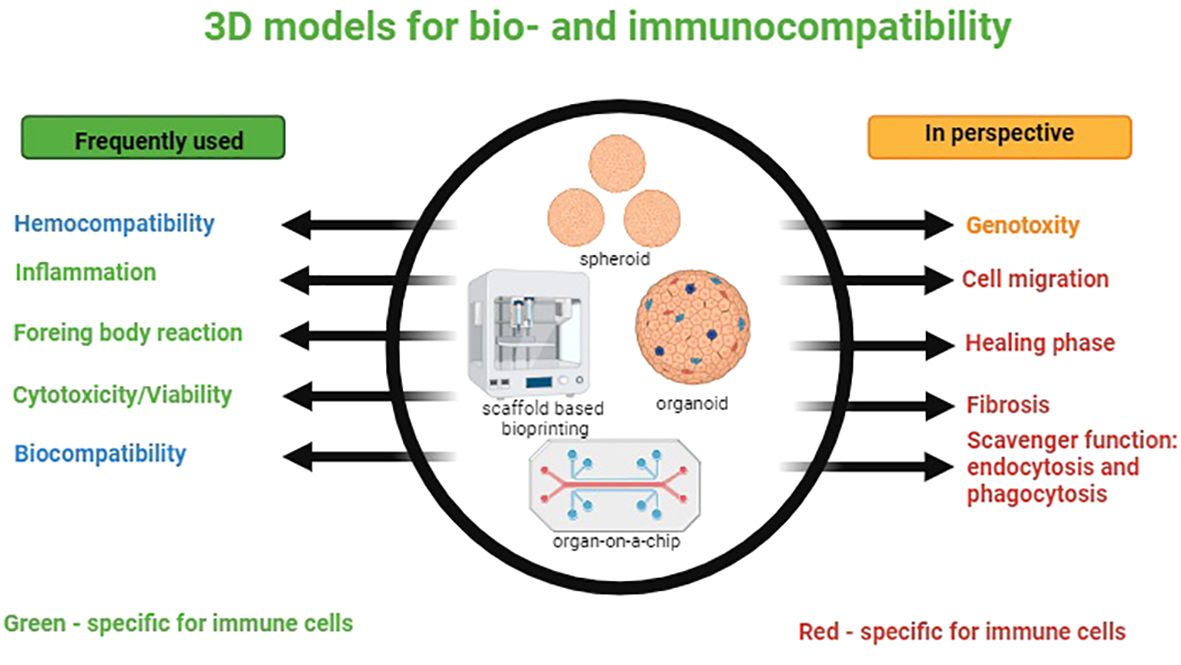
Figure 2 3D models for bio- and immunocompatibility. On the left-side processes frequently simulated in vitro models are listed; immune-specific processes are marked in green. On the right-side processes that have to be modeled in future are listed. Immune-specific processes are marked in red.
In summary, the state of the art in in vitro and ex vivo modelling of macrophage interaction with biomaterials is primarily focused on the mechanic interactions and acute inflammatory reactions, and such modeling allows to detects first line detrimental reactions of macrophage to biomaterials. However, successful implant integration requires the escape from the chronic inflammatory scenario and also suppression al the tissue –destructive activities of macrophages that constitute a part of foreign body response. Modelling of such reaction avoiding animal experimentation is challenge for the scientific community. However, a lot of information can be obtained already in 2D primary macrophage based models, where new biomarkers predicting efficiency of healing and long-term integration of the implant can be discovered. Upgrading the models to 3D conditions will allow further approximation to the in vivo events.
Author contributions
SP: Writing – original draft, Writing – review & editing. GR: Visualization, Writing – original draft, Writing – review & editing. AB: Project administration, Writing – review & editing. SC: Writing – review & editing. IA: Project administration, Writing – review & editing. VP: Project administration, Writing – review & editing. JK: Conceptualization, Supervision, Writing – original draft, Writing – review & editing, Funding acquisition.
Funding
The author(s) declare financial support was received for the research, authorship, and/or publication of this article. The research is funded by the government of the Republic of Bashkortostan within the grant for state support of scientific research conducted under the guidance of leading scientists. Part of this work was supported by the Bashkir State Medical University Development Programme (Priority-2030).The research is funded by the government of the Republic of Bashkortostan within the grant for state support of scientific research conducted under the guidance of leading scientists. Cell viability on all samples was equal.
Conflict of interest
The authors declare that the research was conducted in the absence of any commercial or financial relationships that could be construed as a potential conflict of interest.
The author(s) declared that they were an editorial board member of Frontiers, at the time of submission. This had no impact on the peer review process and the final decision.
Publisher’s note
All claims expressed in this article are solely those of the authors and do not necessarily represent those of their affiliated organizations, or those of the publisher, the editors and the reviewers. Any product that may be evaluated in this article, or claim that may be made by its manufacturer, is not guaranteed or endorsed by the publisher.
Glossary
References
1. Jamieson S, Mawdesley A, Deehan D, Kirby J, Holland J, Tyson-Capper A. Inflammatory responses to metal oxide ceramic nanopowders. Sci Rep. (2021) 11:10531. doi: 10.1038/s41598-021-89329-7
2. Caicedo MS, Samelko L, McAllister K, Jacobs JJ, Hallab NJ. Increasing both CoCrMo-alloy particle size and surface irregularity induces increased macrophage inflammasome activation in vitro potentially through lysosomal destabilization mechanisms. J Orthopaedic Res. (2013) 31:1633–42. doi: 10.1002/jor.22411
3. Sun L, Li X, Xu M, Yang F, Wang W, Niu X. In vitro immunomodulation of magnesium on monocytic cell toward anti-inflammatory macrophages. Regenerative Biomaterials. (2020) 7:391–401. doi: 10.1093/rb/rbaa010
4. Gordon S, Plüddemann A. The mononuclear phagocytic system. Generation Diversity Front Immunol. (2019) 10:1893. doi: 10.3389/fimmu.2019.01893
5. Kzhyshkowska J, Gudima A, Riabov V, Dollinger C, Lavalle P, Vrana NE. Macrophage responses to implants: prospects for personalized medicine. J Leukocyte Biol. (2015) 98:953–62. doi: 10.1189/jlb.5VMR0415-166R
6. Zhu F, Wang S, Zhu X, Pang C, Cui P, Yang F, et al. Potential effects of biomaterials on macrophage function and their signalling pathways. Biomater Sci. (2023) 11:6977–7002. doi: 10.1039/D3BM01213A
7. Batool F, Özçelik H, Stutz C, Gegout P-Y, Benkirane-Jessel N, Petit C, et al. Modulation of immune-inflammatory responses through surface modifications of biomaterials to promote bone healing and regeneration. J Tissue Eng. (2021) 12:20417314211041428. doi: 10.1177/20417314211041428
8. Yanez M, Blanchette J, Jabbarzadeh E. Modulation of inflammatory response to implanted biomaterials using natural compounds. Curr Pharm Des. (2017) 23:6347–57. doi: 10.2174/1381612823666170510124348
9. Abaricia JO, Farzad N, Heath TJ, Simmons J, Morandini L, Olivares-Navarrete R. Control of innate immune response by biomaterial surface topography, energy, and stiffness. Acta Biomater. (2021) 133:58–73. doi: 10.1016/j.actbio.2021.04.021
10. Gordon S, Plüddemann A. Tissue macrophages: heterogeneity and functions. BMC Biol. (2017) 15:53. doi: 10.1186/s12915-017-0392-4
11. Murray PJ. Macrophage polarization. Annu Rev Physiol. (2017) 79:541–66. doi: 10.1146/annurev-physiol-022516-034339
12. Rendra E, Riabov V, Mossel DM, Sevastyanova T, Harmsen MC, Kzhyshkowska J. Reactive oxygen species (ROS) in macrophage activation and function in diabetes. Immunobiology. (2019) 224:242–53. doi: 10.1016/j.imbio.2018.11.010
13. Wynn TA, Vannella KM. Macrophages in tissue repair, regeneration, and fibrosis. Immunity. (2016) 44:450–62. doi: 10.1016/j.immuni.2016.02.015
14. Gratchev A, Kzhyshkowska J, Köthe K, Muller-Molinet I, Kannookadan S, Utikal J, et al. Mφ1 and Mφ2 can be re-polarized by Th2 or Th1 cytokines, respectively, and respond to exogenous danger signals. Immunobiology. (2006) 211:473–86. doi: 10.1016/j.imbio.2006.05.017
15. Chen C, Liu T, Tang Y, Luo G, Liang G, He W. Epigenetic regulation of macrophage polarization in wound healing. Burns Trauma. (2023) 11:tkac057. doi: 10.1093/burnst/tkac057
16. Pålsson-McDermott EM, O’Neill LAJ. Targeting immunometabolism as an anti-inflammatory strategy. Cell Res. (2020) 30:300–14. doi: 10.1038/s41422-020-0291-z
17. Kim A, Downer MA, Berry CE, Valencia C, Fazilat AZ, Griffin M. Investigating immunomodulatory biomaterials for preventing the foreign body response. Bioengineering. (2023) 10:1411. doi: 10.3390/bioengineering10121411
18. Rafikova G, Piatnitskaia S, Shapovalova E, Chugunov S, Kireev V, Ialiukhova D, et al. Interaction of ceramic implant materials with immune system. IJMS. (2023) 24:4200. doi: 10.3390/ijms24044200
19. Ogle ME, Segar CE, Sridhar S, Botchwey EA. Monocytes and macrophages in tissue repair: Implications for immunoregenerative biomaterial design. Exp Biol Med (Maywood). (2016) 241:1084–97. doi: 10.1177/1535370216650293
20. Mack M. Inflammation and fibrosis. Matrix Biol. (2018) 68-69:106–21. doi: 10.1016/j.matbio.2017.11.010
21. Halade GV, Lee DH. Inflammation and resolution signaling in cardiac repair and heart failure. EBioMedicine. (2022) 79:103992. doi: 10.1016/j.ebiom.2022.103992
22. Carnicer-Lombarte A, Chen S-T, Malliaras GG, Barone DG. Foreign body reaction to implanted biomaterials and its impact in nerve neuroprosthetics. Front Bioeng Biotechnol. (2021) 9:622524. doi: 10.3389/fbioe.2021.622524
23. Broughton G, Janis JE, Attinger CE. The basic science of wound healing. Plast Reconstructive Surg. (2006) 117:12S–34S. doi: 10.1097/01.prs.0000225430.42531.c2
24. Anderson JM, Rodriguez A, Chang DT. Foreign body reaction to biomaterials. Semin Immunol. (2008) 20:86–100. doi: 10.1016/j.smim.2007.11.004
25. Chen L, Deng H, Cui H, Fang J, Zuo Z, Deng J, et al. Inflammatory responses and inflammation-associated diseases in organs. Oncotarget. (2017) 9:7204–18. doi: 10.18632/oncotarget.23208
26. Barker TH, Engler AJ. The provisional matrix: setting the stage for tissue repair outcomes. Matrix Biol. (2017) 60–61:1–4. doi: 10.1016/j.matbio.2017.04.003
27. Ekdahl KN, Lambris JD, Elwing H, Ricklin D, Nilsson PH, Teramura Y, et al. Innate immunity activation on biomaterial surfaces: a mechanistic model and coping strategies. Adv Drug Delivery Rev. (2011) 63:1042–50. doi: 10.1016/j.addr.2011.06.012
28. Nilsson B, Ekdahl KN, Mollnes TE, Lambris JD. The role of complement in biomaterial-induced inflammation. Mol Immunol. (2007) 44:82–94. doi: 10.1016/j.molimm.2006.06.020
29. Tang Y, Wang J, Lian Y, Fan C, Zhang P, Wu Y, et al. Linking long non-coding RNAs and SWI/SNF complexes to chromatin remodeling in cancer. Mol Cancer. (2017) 16:42. doi: 10.1186/s12943-017-0612-0
30. Stankevich KS, Gudima A, Filimonov VD, Klüter H, Mamontova EM, Tverdokhlebov SI, et al. Surface modification of biomaterials based on high-molecular polylactic acid and their effect on inflammatory reactions of primary human monocyte-derived macrophages: Perspective for personalized therapy. Materials Sci Engineering: C. (2015) 51:117–26. doi: 10.1016/j.msec.2015.02.047
31. Saleh LS, Bryant SJ. In vitro and in vivo models for assessing the host response to biomaterials. Drug Discovery Today Dis Models. (2017) 24:13–21. doi: 10.1016/j.ddmod.2018.04.002
32. Hachim D, LoPresti ST, Yates CC, Brown BN. Shifts in macrophage phenotype at the biomaterial interface via IL-4 eluting coatings are associated with improved implant integration. Biomaterials. (2017) 112:95–107. doi: 10.1016/j.biomaterials.2016.10.019
33. Zhang L, Cao Z, Bai T, Carr L, Ella-Menye J-R, Irvin C, et al. Zwitterionic hydrogels implanted in mice resist the foreign-body reaction. Nat Biotechnol. (2013) 31:553–6. doi: 10.1038/nbt.2580
34. Landgraeber S, Jäger M, Jacobs JJ, Hallab NJ. The pathology of orthopedic implant failure is mediated by innate immune system cytokines. Mediators Inflammation. (2014) 2014:1–9. doi: 10.1155/2014/185150
35. Baht GS, Vi L, Alman BA. The role of the immune cells in fracture healing. Curr Osteoporos Rep. (2018) 16:138–45. doi: 10.1007/s11914-018-0423-2
36. Song Z, Cheng Y, Chen M, Xie X. Macrophage polarization in bone implant repair: A review. Tissue Cell. (2023) 82:102112. doi: 10.1016/j.tice.2023.102112
37. Cong Y, Wang Y, Yuan T, Zhang Z, Ge J, Meng Q, et al. Macrophages in aseptic loosening: Characteristics, functions, and mechanisms. Front Immunol. (2023) 14:1122057. doi: 10.3389/fimmu.2023.1122057
38. Wynn TA. Cellular and molecular mechanisms of fibrosis. J Pathol. (2008) 214:199–210. doi: 10.1002/path.2277
39. Noskovicova N, Hinz B, Pakshir P. Implant fibrosis and the underappreciated role of myofibroblasts in the foreign body reaction. Cells. (2021) 10:1794. doi: 10.3390/cells10071794
40. Selders GS, Fetz AE, Radic MZ, Bowlin GL. An overview of the role of neutrophils in innate immunity, inflammation and host-biomaterial integration. Regener Biomater. (2017) 4:55–68. doi: 10.1093/rb/rbw041
41. Branzk N, Lubojemska A, Hardison SE, Wang Q, Gutierrez MG, Brown GD, et al. Neutrophils sense microbe size and selectively release neutrophil extracellular traps in response to large pathogens. Nat Immunol. (2014) 15:1017–25. doi: 10.1038/ni.2987
42. Ardi VC, Kupriyanova TA, Deryugina EI, Quigley JP. Human neutrophils uniquely release TIMP-free MMP-9 to provide a potent catalytic stimulator of angiogenesis. Proc Natl Acad Sci USA. (2007) 104:20262–7. doi: 10.1073/pnas.0706438104
43. Tang L, Jennings TA, Eaton JW. Mast cells mediate acute inflammatory responses to implanted biomaterials. Proc Natl Acad Sci USA. (1998) 95:8841–6. doi: 10.1073/pnas.95.15.8841
44. Rezzani R, Rodella L, Tartaglia GM, Paganelli C, Sapelli P, Bianchi R. Mast cells and the inflammatory response to different implanted biomaterials. Arch Histol Cytol. (2004) 67:211–7. doi: 10.1679/aohc.67.211
45. Zhang B, Su Y, Zhou J, Zheng Y, Zhu D. Toward a better regeneration through implant-mediated immunomodulation: harnessing the immune responses. Advanced Sci. (2021) 8:2100446. doi: 10.1002/advs.202100446
46. Abel AM, Yang C, Thakar MS, Malarkannan S. Natural killer cells: development, maturation, and clinical utilization. Front Immunol. (2018) 9:1869. doi: 10.3389/fimmu.2018.01869
47. Sheikh Z, Brooks PJ, Barzilay O, Fine N, Glogauer M. Macrophages, foreign body giant cells and their response to implantable biomaterials. Materials (Basel). (2015) 8:5671–701. doi: 10.3390/ma8095269
48. Riabov V, Salazar F, Htwe SS, Gudima A, Schmuttermaier C, Barthes J, et al. Generation of anti-inflammatory macrophages for implants and regenerative medicine using self-standing release systems with a phenotype-fixing cytokine cocktail formulation. Acta Biomaterialia. (2017) 53:389–98. doi: 10.1016/j.actbio.2017.01.071
49. Higgins DM, Basaraba RJ, Hohnbaum AC, Lee EJ, Grainger DW, Gonzalez-Juarrero M. Localized immunosuppressive environment in the foreign body response to implanted biomaterials. Am J Pathol. (2009) 175:161–70. doi: 10.2353/ajpath.2009.080962
50. Martin KE, García AJ. Macrophage phenotypes in tissue repair and the foreign body response: Implications for biomaterial-based regenerative medicine strategies. Acta Biomater. (2021) 133:4–16. doi: 10.1016/j.actbio.2021.03.038
51. Ekström K, Omar O, Granéli C, Wang X, Vazirisani F, Thomsen P. Monocyte exosomes stimulate the osteogenic gene expression of mesenchymal stem cells. PloS One. (2013) 8:e75227. doi: 10.1371/journal.pone.0075227
52. Omar OM, Granéli C, Ekström K, Karlsson C, Johansson A, Lausmaa J, et al. The stimulation of an osteogenic response by classical monocyte activation. Biomaterials. (2011) 32:8190–204. doi: 10.1016/j.biomaterials.2011.07.055
53. Sridharan R, Cameron AR, Kelly DJ, Kearney CJ, O’Brien FJ. Biomaterial based modulation of macrophage polarization: a review and suggested design principles. Materials Today. (2015) 18:313–25. doi: 10.1016/j.mattod.2015.01.019
54. Larionova I, Kazakova E, Patysheva M, Kzhyshkowska J. Transcriptional, epigenetic and metabolic programming of tumor-associated macrophages. Cancers. (2020) 12:1411. doi: 10.3390/cancers12061411
55. Kazakova E, Iamshchikov P, Larionova I, Kzhyshkowska J. Macrophage scavenger receptors: Tumor support and tumor inhibition. Front Oncol. (2023) 12:1096897. doi: 10.3389/fonc.2022.1096897
56. Kzhyshkowska J, Neyen C, Gordon S. Role of macrophage scavenger receptors in atherosclerosis. Immunobiology. (2012) 217:492–502. doi: 10.1016/j.imbio.2012.02.015
57. Linares-Alcántara E, Mendlovic F. Scavenger receptor A1 signaling pathways affecting macrophage functions in innate and adaptive immunity. Immunol Invest. (2022) 51:1725–55. doi: 10.1080/08820139.2021.2020812
58. Kissick HT, Dunn LK, Ghosh S, Nechama M, Kobzik L, Arredouani MS. The scavenger receptor MARCO modulates TLR-induced responses in dendritic cells. PloS One. (2014) 9:e104148. doi: 10.1371/journal.pone.0104148
59. Murshid A, Borges TJ, Lang BJ, Calderwood SK. The scavenger receptor SREC-I cooperates with toll-like receptors to trigger inflammatory innate immune responses. Front Immunol. (2016) 7:226. doi: 10.3389/fimmu.2016.00226
60. Larionova I, Kiselev A, Kazakova E, Liu T, Patysheva M, Iamshchikov P, et al. Tumor-associated macrophages respond to chemotherapy by detrimental transcriptional reprogramming and suppressing stabilin-1 mediated clearance of EGF. Front Immunol. (2023) 14:1000497. doi: 10.3389/fimmu.2023.1000497
61. Schledzewski K, Géraud C, Arnold B, Wang S, Gröne H-J, Kempf T, et al. Deficiency of liver sinusoidal scavenger receptors stabilin-1 and -2 in mice causes glomerulofibrotic nephropathy via impaired hepatic clearance of noxious blood factors. J Clin Invest. (2011) 121:703–14. doi: 10.1172/JCI44740
62. Silva-Bermudez LS, Sevastyanova TN, Schmuttermaier C, de la Torre C, Schumacher L, Klüter H, et al. Titanium nanoparticles enhance production and suppress stabilin-1-mediated clearance of GDF-15 in human primary macrophages. Front Immunol. (2021) 12:760577. doi: 10.3389/fimmu.2021.760577
63. Adolfsson K, Abariute L, Dabkowska AP, Schneider M, Häcker U, Prinz CN. Direct comparison between in vivo and in vitro microsized particle phagocytosis assays in Drosophila melanogaster. Toxicol Vitro. (2018) 46:213–8. doi: 10.1016/j.tiv.2017.10.014
64. Xu C, Wu H, Liu Y, Li F, Manne RK, Lin H-K. Protocol for detecting macrophage-mediated cancer cell phagocytosis in vitro and in vivo. STAR Protoc. (2023) 4. doi: 10.1016/j.xpro.2022.101940
65. Alidori S, Bowman RL, Yarilin D, Romin Y, Barlas A, Mulvey JJ, et al. Deconvoluting hepatic processing of carbon nanotubes. Nat Commun. (2016) 7:12343. doi: 10.1038/ncomms12343
66. Levy-Pereira N, Yasui GS, Evangelista MM, Nascimento NF, Santos MP, Siqueira-Silva DH, et al. In vivo phagocytosis and hematology in Astyanax altiparanae, a potential model for surrogate technology. Braz J Biol. (2020) 80:336–44. doi: 10.1590/1519-6984.205893
67. Hult A, Toss F, Malm C, Oldenborg PA. In vitro phagocytosis of liquid-stored red blood cells requires serum and can be inhibited with fucoidan and dextran sulphate. Vox Sang. (2020) 115:647–54. doi: 10.1111/vox.12922
68. Yamada C, Beron-Pelusso C, Algazzaz N, Heidari A, Luz D, Rawas-Qalaji M, et al. Age-dependent effect between MARCO and TLR4 on PMMA particle phagocytosis by macrophages. J Cell Mol Med. (2019) 23:5827–31. doi: 10.1111/jcmm.14494
69. Zhang J, Qu C, Li T, Cui W, Wang X, Du J. Phagocytosis mediated by scavenger receptor class BI promotes macrophage transition during skeletal muscle regeneration. J Biol Chem. (2019) 294:15672–85. doi: 10.1074/jbc.RA119.008795
70. Gratchev A, Kzhyshkowska J, Utikal J, Goerdt S. Interleukin-4 and dexamethasone counterregulate extracellular matrix remodelling and phagocytosis in type-2 macrophages. Scandinavian J Immunol. (2005) 61:10–7. doi: 10.1111/j.0300-9475.2005.01524.x
71. Park S-Y, Jung M-Y, Lee S-J, Kang K-B, Gratchev A, Riabov V, et al. Stabilin-1 mediates phosphatidylserine-dependent clearance of cell corpses in alternatively activated macrophages. J Cell Sci. (2009) 122:3365–73. doi: 10.1242/jcs.049569
72. Onyishi CU, Desanti GE, Wilkinson AL, Lara-Reyna S, Frickel E-M, Fejer G, et al. Toll-like receptor 4 and macrophage scavenger receptor 1 crosstalk regulates phagocytosis of a fungal pathogen. Nat Commun. (2023) 14:4895. doi: 10.1038/s41467-023-40635-w
73. Luu TU, Liu WF. Regulation of macrophages by extracellular matrix composition and adhesion geometry. Regener Eng Transl Med. (2018) 4:238–46. doi: 10.1007/s40883-018-0065-z
74. Self-assembled monolayers of enantiomerically functionalized periodic mesoporous organosilicas and the effect of surface chirality on cell adhesion behaviour - RSC Advances . RSC Publishing. Available online at: https://pubs.rsc.org/en/content/articlelanding/2015/ra/c4ra11451e/unauth (Accessed February 2, 2024).
75. Takebe J, Champagne CM, Offenbacher S, Ishibashi K, Cooper LF. Titanium surface topography alters cell shape and modulates bone morphogenetic protein 2 expression in the J774A.1 macrophage cell line. J Biomed Materials Res. (2003) 64A:207–16. doi: 10.1002/jbm.a.10275
76. Lu Y, Wang L, Zhang Y. The promising roles of macrophages in geriatric hip fracture. Front Cell Dev Biol. (2022) 10:962990. doi: 10.3389/fcell.2022.962990
77. Foster DS, Januszyk M, Yost KE, Chinta MS, Gulati GS, Nguyen AT, et al. Integrated spatial multiomics reveals fibroblast fate during tissue repair. Proc Natl Acad Sci USA. (2021) 118:e2110025118. doi: 10.1073/pnas.2110025118
78. Theocharidis G, Thomas BE, Sarkar D, Mumme HL, Pilcher WJR, Dwivedi B, et al. Single cell transcriptomic landscape of diabetic foot ulcers. Nat Commun. (2022) 13:181. doi: 10.1038/s41467-021-27801-8
79. Yang Y, Chu C, Liu L, Wang C, Hu C, Rung S, et al. Tracing immune cells around biomaterials with spatial anchors during large-scale wound regeneration. Nat Commun. (2023) 14:5995. doi: 10.1038/s41467-023-41608-9
80. Gong L, Gu Y, Han X, Luan C, Liu C, Wang X, et al. Spatiotemporal dynamics of the molecular expression pattern and intercellular interactions in the glial scar response to spinal cord injury. Neurosci Bull. (2023) 39:213–44. doi: 10.1007/s12264-022-00897-8
81. Viola A, Munari F, Sánchez-Rodríguez R, Scolaro T, Castegna A. The metabolic signature of macrophage responses. Front Immunol. (2019) 10:1462. doi: 10.3389/fimmu.2019.01462
82. Casteel JL, Keever KR, Ardell CL, Williams DL, Gao D, Podrez EA, et al. Modification of extracellular matrix by the product of DHA oxidation switches macrophage adhesion patterns and promotes retention of macrophages during chronic inflammation. Front Immunol. (2022) 13:867082. doi: 10.3389/fimmu.2022.867082
83. Sapudom J, Mohamed WKE, Garcia-Sabaté A, Alatoom A, Karaman S, Mahtani N, et al. Collagen fibril density modulates macrophage activation and cellular functions during tissue repair. Bioengineering. (2020) 7:33. doi: 10.3390/bioengineering7020033
84. Hsieh JY, Smith TD, Meli VS, Tran TN, Botvinick EL, Liu WF. Differential regulation of macrophage inflammatory activation by fibrin and fibrinogen. Acta Biomaterialia. (2017) 47:14–24. doi: 10.1016/j.actbio.2016.09.024
85. Rudnik M, Hukara A, Kocherova I, Jordan S, Schniering J, Milleret V, et al. Elevated fibronectin levels in profibrotic CD14+ Monocytes and CD14+ Macrophages in systemic sclerosis. Front Immunol. (2021) 12:642891. doi: 10.3389/fimmu.2021.642891
86. Rockey DC, Bell PD, Hill JA. Fibrosis–a common pathway to organ injury and failure. N Engl J Med. (2015) 372:1138–49. doi: 10.1056/NEJMra1300575
87. Wynn TA, Barron L. Macrophages: master regulators of inflammation and fibrosis. Semin Liver Dis. (2010) 30:245–57. doi: 10.1055/s-0030-1255354
88. Luchian I, Goriuc A, Sandu D, Covasa M. The role of matrix metalloproteinases (MMP-8, MMP-9, MMP-13) in periodontal and peri-implant pathological processes. Int J Mol Sci. (2022) 23:1806. doi: 10.3390/ijms23031806
89. Unger J, Boeynaems JM, Van Herle A, Van Sande J, Rocmans P, Mockel J. In vitro nonbutanol-extractable iodine release in dog thyroid. Endocrinology. (1979) 105:225–31. doi: 10.1210/endo-105-1-225
90. Yang Y-N, Bauer D, Wasmuth S, Steuhl K-P, Heiligenhaus A. Matrix metalloproteinases (MMP-2 and 9) and tissue inhibitors of matrix metalloproteinases (TIMP-1 and 2) during the course of experimental necrotizing herpetic keratitis. Exp Eye Res. (2003) 77:227–37. doi: 10.1016/S0014-4835(03)00112-X
91. Reiss MJ, Han Y-P, Garcia E, Goldberg M, Yu H, Garner WL. Matrix metalloproteinase-9 delays wound healing in a murine wound model. Surgery. (2010) 147:295–302. doi: 10.1016/j.surg.2009.10.016
92. Madala SK, Pesce JT, Ramalingam TR, Wilson MS, Minnicozzi S, Cheever AW, et al. Matrix metalloproteinase 12-deficiency augments extracellular matrix degrading metalloproteinases and attenuates IL-13-dependent fibrosis. J Immunol. (2010) 184:3955–63. doi: 10.4049/jimmunol.0903008
93. Stawski L, Haines P, Fine A, Rudnicka L, Trojanowska M. MMP-12 deficiency attenuates angiotensin II-induced vascular injury, M2 macrophage accumulation, and skin and heart fibrosis. PloS One. (2014) 9:e109763. doi: 10.1371/journal.pone.0109763
94. Lee C-M, He C-H, Park JW, Lee JH, Kamle S, Ma B, et al. Correction: Chitinase 1 regulates pulmonary fibrosis by modulating TGF-β/SMAD7 pathway via TGFBRAP1 and FOXO3. Life Sci Alliance. (2023) 6:e202302065. doi: 10.26508/lsa.202302065
95. Lee S-Y, Lee C-M, Ma B, Kamle S, Elias JA, Zhou Y, et al. Targeting chitinase 1 and chitinase 3-like 1 as novel therapeutic strategy of pulmonary fibrosis. Front Pharmacol. (2022) 13:826471. doi: 10.3389/fphar.2022.826471
96. Refai AK, Textor M, Brunette DM, Waterfield JD. Effect of titanium surface topography on macrophage activation and secretion of proinflammatory cytokines and chemokines. J Biomed Materials Res. (2004) 70A:194–205. doi: 10.1002/jbm.a.30075
97. Sun SJ, Yu WQ, Zhang YL, Jiang XQ, Zhang FQ. Effects of TiO2 nanotube layers on RAW 264.7 macrophage behaviour and bone morphogenetic protein-2 expression. Cell Prolif. (2013) 46:685–94. doi: 10.1111/cpr.12072
98. Zhu Y, Liang H, Liu X, Wu J, Yang C, Wong TM, et al. Regulation of macrophage polarization through surface topography design to facilitate implant-to-bone osteointegration. Sci Adv. (2021) 7:eabf6654. doi: 10.1126/sciadv.abf6654
99. Meng X, Zhang W, Lyu Z, Long T, Wang Y. ZnO nanoparticles attenuate polymer-wear-particle induced inflammatory osteolysis by regulating the MEK-ERK-COX-2 axis. J Orthopaedic Translation. (2022) 34:1–10. doi: 10.1016/j.jot.2022.04.001
100. Deng Z, Jin J, Wang Z, Wang Y, Gao Q, Zhao J. The metal nanoparticle-induced inflammatory response is regulated by SIRT1 through NF-κB deacetylation in aseptic loosening. IJN. (2017) 12:3617–36. doi: 10.2147/IJN.S124661
101. Mao X, Pan X, Peng X, Cheng T, Zhang X. Inhibition of titanium particle-induced inflammation by the proteasome inhibitor bortezomib in murine macrophage-like RAW 264.7 cells. Inflammation. (2012) 35:1411–8. doi: 10.1007/s10753-012-9454-5
102. Tan KS, Qian L, Rosado R, Flood PM, Cooper LF. The role of titanium surface topography on J774A.1 macrophage inflammatory cytokines and nitric oxide production. Biomaterials. (2006) 27:5170–7. doi: 10.1016/j.biomaterials.2006.05.002
103. Jakobsen SS, Larsen A, Stoltenberg M, Bruun JM, Soballe K. Hydroxyapatite Coatings Did not Increase TGF-β and BMP-2 Secretion in Murine J774A.1 Macrophages, but Induced a Pro-inflammatory Cytokine Response. J Biomaterials Science Polymer Edition. (2009) 20:455–65. doi: 10.1163/156856209X416476
104. Taciak B, Białasek M, Braniewska A, Sas Z, Sawicka P, Kiraga Ł, et al. Evaluation of phenotypic and functional stability of RAW 264.7 cell line through serial passages. PloS One. (2018) 13:e0198943. doi: 10.1371/journal.pone.0198943
105. Pearl JI, Ma T, Irani AR, Huang Z, Robinson WH, Smith RL, et al. Role of the Toll-like receptor pathway in the recognition of orthopedic implant wear-debris particles. Biomaterials. (2011) 32:5535–42. doi: 10.1016/j.biomaterials.2011.04.046
106. Alhamdi JR, Peng T, Al-Naggar IM, Hawley KL, Spiller KL, Kuhn LT. Controlled M1-to-M2 transition of aged macrophages by calcium phosphate coatings. Biomaterials. (2019) 196:90–9. doi: 10.1016/j.biomaterials.2018.07.012
107. Park KR, Bryers JD. Effect of macrophage classical (M1) activation on implant-adherent macrophage interactions with Staphylococcus epidermidis : A murine in vitro model system. J Biomed Materials Res. (2012) 100A:2045–53. doi: 10.1002/jbm.a.34087
108. Mills CD, Kincaid K, Alt JM, Heilman MJ, Hill AM. M-1/M-2 macrophages and the Th1/Th2 paradigm. J Immunol. (2000) 164:6166–73. doi: 10.4049/jimmunol.164.12.6166
109. Song HK, Hwang DY. Use of C57BL/6N mice on the variety of immunological researches. Lab Anim Res. (2017) 33:119–23. doi: 10.5625/lar.2017.33.2.119
110. Bylski D, Wedemeyer C, Xu J, Sterner T, Löer F, Von Knoch M. Alumina ceramic particles, in comparison with titanium particles, hardly affect the expression of RANK-, TNF-α-, and OPG-mRNA in the THP-1 human monocytic cell line. J Biomed Materials Res. (2009) 89A:707–16. doi: 10.1002/jbm.a.31956
111. Schwarz F, Langer M, Hagena T, Hartig B, Sader R, Becker J. Cytotoxicity and proinflammatory effects of titanium and zirconia particles. Int J Implant Dent. (2019) 5:25. doi: 10.1186/s40729-019-0178-2
112. Pettersson M, Kelk P, Belibasakis GN, Bylund D, Molin Thorén M, Johansson A. Titanium ions form particles that activate and execute interleukin-1β release from lipopolysaccharide-primed macrophages. J Periodontal Res. (2017) 52:21–32. doi: 10.1111/jre.12364
113. Fernandes KR, Zhang Y, Magri AMP, Renno ACM, Van Den Beucken JJJP. Biomaterial property effects on platelets and macrophages: an in vitro study. ACS Biomater Sci Eng. (2017) 3:3318–27. doi: 10.1021/acsbiomaterials.7b00679
114. Vallés G, González-Melendi P, Saldaña L, Rodriguez M, Munuera L, Vilaboa N. Rutile and titanium particles differentially affect the production of osteoblastic local factors. J Biomed Materials Res. (2008) 84A:324–36. doi: 10.1002/jbm.a.31315
115. Yagil-Kelmer E, Kazmier P, Rahaman MN, Bal BS, Tessman RK, Estes DM. Comparison of the response of primary human blood monocytes and the U937 human monocytic cell line to two different sizes of alumina ceramic particles. J Orthopaedic Res. (2004) 22:832–8. doi: 10.1016/j.orthres.2003.10.022
116. Rashad A, Suliman S, Mustafa M, Pedersen TØ, Campodoni E, Sandri M, et al. Inflammatory responses and tissue reactions to wood-Based nanocellulose scaffolds. Materials Sci Engineering: C. (2019) 97:208–21. doi: 10.1016/j.msec.2018.11.068
117. Detsch R, Mayr H, Ziegler G. Formation of osteoclast-like cells on HA and TCP ceramics. Acta Biomaterialia. (2008) 4:139–48. doi: 10.1016/j.actbio.2007.03.014
118. Gunti S, Hoke ATK, Vu KP, London NR. Organoid and spheroid tumor models: techniques and applications. Cancers (Basel). (2021) 13:874. doi: 10.3390/cancers13040874
119. Tang X-Y, Wu S, Wang D, Chu C, Hong Y, Tao M, et al. Human organoids in basic research and clinical applications. Sig Transduct Target Ther. (2022) 7:168. doi: 10.1038/s41392-022-01024-9
120. Fang JY, Yang Z, Han B. Switch of macrophage fusion competency by 3D matrices. Sci Rep. (2020) 10:10348. doi: 10.1038/s41598-020-67056-9
121. Chen EP, Toksoy Z, Davis BA, Geibel JP. 3D Bioprinting of Vascularized Tissues for in vitro and in vivo Applications. Front Bioeng Biotechnol. (2021) 9:664188. doi: 10.3389/fbioe.2021.664188
122. Sieber S, Wirth L, Cavak N, Koenigsmark M, Marx U, Lauster R, et al. Bone marrow-on-a-chip: Long-term culture of human haematopoietic stem cells in a three-dimensional microfluidic environment. J Tissue Eng Regener Med. (2018) 12:479–89. doi: 10.1002/term.2507
123. Rigat-Brugarolas LG, Elizalde-Torrent A, Bernabeu M, De Niz M, Martin-Jaular L, Fernandez-Becerra C, et al. A functional microengineered model of the human splenon-on-a-chip. Lab Chip. (2014) 14:1715–24. doi: 10.1039/C3LC51449H
124. Irimia D, Wang X. Inflammation-on-a-chip: probing the immune system ex vivo. Trends Biotechnol. (2018) 36:923–37. doi: 10.1016/j.tibtech.2018.03.011
125. Kudryavtseva V, Stankevich K, Gudima A, Kibler E, Zhukov Y, Bolbasov E, et al. Atmospheric pressure plasma assisted immobilization of hyaluronic acid on tissue engineering PLA-based scaffolds and its effect on primary human macrophages. Materials Design. (2017) 127:261–71. doi: 10.1016/j.matdes.2017.04.079
126. Özçelik H, Vrana NE, Gudima A, Riabov V, Gratchev A, Haikel Y, et al. Harnessing the multifunctionality in nature: A bioactive agent release system with self-antimicrobial and immunomodulatory properties. Adv Healthcare Materials. (2015) 4:2026–36. doi: 10.1002/adhm.201500546
127. Stankevich KS, Kudryavtseva VL, Bolbasov EN, Shesterikov EV, Larionova IV, Shapovalova YG, et al. Modification of PCL scaffolds by reactive magnetron sputtering: A possibility for modulating macrophage responses. ACS Biomater Sci Eng. (2020) 6:3967–74. doi: 10.1021/acsbiomaterials.0c00440
128. Singh S, Awuah D, Rostam HM, Emes RD, Kandola NK, Onion D, et al. Unbiased analysis of the impact of micropatterned biomaterials on macrophage behavior provides insights beyond predefined polarization states. ACS Biomater Sci Eng. (2017) 3:969–78. doi: 10.1021/acsbiomaterials.7b00104
129. Gvaramia D, Kern J, Jakob Y, Tritschler H, Brenner RE, Breiter R, et al. Modulation of the inflammatory response to decellularized collagen matrix for cartilage regeneration. J Biomed Materials Res. (2022) 110:1021–35. doi: 10.1002/jbm.a.37349
130. Duval K, Grover H, Han L-H, Mou Y, Pegoraro AF, Fredberg J, et al. Modeling physiological events in 2D vs. 3D cell culture. Physiology. (2017) 32:266–77. doi: 10.1152/physiol.00036.2016
131. Almeida CR, Serra T, Oliveira MI, Planell JA, Barbosa MA, Navarro M. Impact of 3-D printed PLA- and chitosan-based scaffolds on human monocyte/macrophage responses: unraveling the effect of 3-D structures on inflammation. Acta Biomater. (2014) 10:613–22. doi: 10.1016/j.actbio.2013.10.035
132. Barker E, AlQobaly L, Shaikh Z, Franklin K, Moharamzadeh K. Implant soft-tissue attachment using 3D oral mucosal models-A pilot study. Dent J (Basel). (2020) 8:72. doi: 10.3390/dj8030072
133. Sharifi F, Htwe SS, Righi M, Liu H, Pietralunga A, Yesil-Celiktas O, et al. A foreign body response-on-a-chip platform. Adv Healthcare Materials. (2019) 8:1801425. doi: 10.1002/adhm.201801425
134. Barthes J, Lagarrigue P, Riabov V, Lutzweiler G, Kirsch J, Muller C, et al. Biofunctionalization of 3D-printed silicone implants with immunomodulatory hydrogels for controlling the innate immune response: An in vivo model of tracheal defect repair. Biomaterials. (2021) 268:120549. doi: 10.1016/j.biomaterials.2020.120549
135. Li W, Dai F, Zhang S, Xu F, Xu Z, Liao S, et al. Pore size of 3D-printed polycaprolactone/polyethylene glycol/hydroxyapatite scaffolds affects bone regeneration by modulating macrophage polarization and the foreign body response. ACS Appl Mater Interfaces. (2022) 14:20693–707. doi: 10.1021/acsami.2c02001
136. Mestres G, Carter S-SD, Hailer NP, Diez-Escudero A. A practical guide for evaluating the osteoimmunomodulatory properties of biomaterials. Acta Biomaterialia. (2021) 130:115–37. doi: 10.1016/j.actbio.2021.05.038
137. Cohen AS, Connors LH. The pathogenesis and biochemistry of amyloidosis. J Pathol. (1987) 151:1–10. doi: 10.1002/path.1711510102
138. Wei X, Zhou W, Tang Z, Wu H, Liu Y, Dong H, et al. Magnesium surface-activated 3D printed porous PEEK scaffolds for in vivo osseointegration by promoting angiogenesis and osteogenesis. Bioact Mater. (2023) 20:16–28. doi: 10.1016/j.bioactmat.2022.05.011
139. Danku AE, Dulf E-H, Braicu C, Jurj A, Berindan-Neagoe I. Organ-on-A-chip: A survey of technical results and problems. Front Bioeng Biotechnol. (2022) 10:840674. doi: 10.3389/fbioe.2022.840674
140. Morsink M, Willemen N, Leijten J, Bansal R, Shin S. Immune organs and immune cells on a chip: an overview of biomedical applications. Micromachines. (2020) 11:849. doi: 10.3390/mi11090849
141. Iordachescu A, Hughes EAB, Joseph S, Hill EJ, Grover LM, Metcalfe AD. Trabecular bone organoids: a micron-scale ‘humanised’ prototype designed to study the effects of microgravity and degeneration. NPJ Microgravity. (2021) 7:17. doi: 10.1038/s41526-021-00146-8
142. Sachdev A, Acharya S, Gadodia T, Shukla S, Harshita J, Akre C, et al. A review on techniques and biomaterials used in 3D bioprinting. Cureus. (2022) 14(8):e28463. doi: 10.7759/cureus.28463
Keywords: implants, inflammation, cytokine, healing, fibrosis, monocyte
Citation: Piatnitskaia S, Rafikova G, Bilyalov A, Chugunov S, Akhatov I, Pavlov V and Kzhyshkowska J (2024) Modelling of macrophage responses to biomaterials in vitro: state-of-the-art and the need for the improvement. Front. Immunol. 15:1349461. doi: 10.3389/fimmu.2024.1349461
Received: 04 December 2023; Accepted: 21 February 2024;
Published: 26 March 2024.
Edited by:
Yasser M. El-Sherbiny, Nottingham Trent University, United KingdomReviewed by:
Daniela Pereira Vasconcelos, Universidade do Porto, PortugalKui Xu, Anhui University of Chinese Medicine, China
Chengcheng Yin, China Medical University, China
Copyright © 2024 Piatnitskaia, Rafikova, Bilyalov, Chugunov, Akhatov, Pavlov and Kzhyshkowska. This is an open-access article distributed under the terms of the Creative Commons Attribution License (CC BY). The use, distribution or reproduction in other forums is permitted, provided the original author(s) and the copyright owner(s) are credited and that the original publication in this journal is cited, in accordance with accepted academic practice. No use, distribution or reproduction is permitted which does not comply with these terms.
*Correspondence: Julia Kzhyshkowska, Julia.kzhyshkowska@medma.uni-heidelberg.de
†These authors have contributed equally to this work