- 1Lung Imaging Laboratory, Division of Pulmonary, Critical Care, and Sleep Medicine, Department of Medicine, Icahn School of Medicine at Mount Sinai, New York, NY, United States
- 2Global Health and Emerging Pathogens Institute, Department of Microbiology, Icahn School of Medicine at Mount Sinai, New York, NY, United States
- 3Department of Medicine, College of Physicians and Surgeons, Columbia University Medical Center, New York, NY, United States
- 4Department of Physiology and Cellular Biophysics, College of Physicians and Surgeons, Columbia University Medical Center, New York, NY, United States
Lung infection by influenza A virus (IAV) is a major cause of global mortality from lung injury, a disease defined by widespread dysfunction of the lung’s air-blood barrier. Endocytosis of IAV virions by the alveolar epithelium – the cells that determine barrier function – is central to barrier loss mechanisms. Here, we address the current understanding of the mechanistic steps that lead to endocytosis in the alveolar epithelium, with an eye to how the unique structure of lung alveoli shapes endocytic mechanisms. We highlight where future studies of alveolar interactions with IAV virions may lead to new therapeutic approaches for IAV-induced lung injury.
1 Introduction
Lung infection by influenza A virus (IAV) is a major cause of global mortality. In the last century alone, four global IAV pandemics caused more than 50 million deaths (1, 2). In the modern era, seasonal IAV infections cause nearly half a million deaths every year (3, 4).
Death from IAV lung infection often results from lung injury (5, 6). Acute lung injury is defined by damage to lung alveoli that causes loss of alveolar barrier function, leading to airspace flooding with protein-rich edema fluid (7). Therapy for IAV-induced lung injury centers on antiviral drugs. But, antiviral drugs do not contain lung injury once it initiates (8–11) and are increasingly hindered by viral drug resistance (12, 13), creating a critical need for new approaches to therapy. The sizeable annual risk of a recurrent IAV pandemic (1, 14) makes that need not only critical, but urgent.
Endocytosis of IAV virions by alveolar cells is a central event on the path from lung infection to lung injury (15) and a target for antiviral drug development (16, 17). However, the understanding of endocytic mechanisms is largely informed by findings generated in systems that incorporate non-alveolar cells or that isolate alveolar cells from their normal microenvironment. The extent to which such systems represent IAV uptake mechanisms in alveoli in vivo may be limited, since unique aspects of alveolar structure that likely impact endocytic mechanisms are difficult to replicate in vitro.
Whereas previous reviews of IAV lung infection have focused on viral entry mechanisms derived from studies in cultured cells, here we will consider viral endocytosis in the alveolar epithelium from the standpoint of structural features of intact alveoli that likely impact endocytic mechanisms. Although alveolar macrophages also shape IAV lung pathogenesis, we will not address them in this review. By highlighting structural features of alveoli, we hope to stimulate new investigations of viral endocytosis in the alveolar epithelium that may lead to new therapeutic approaches for IAV-induced lung injury.
2 IAV virion inhalation into alveoli
Alveolar infection initiates when IAV virions are inhaled into the lung. IAV virions consist of a membrane envelope that surrounds the viral genome. The envelope contains host-derived lipids and proteins (18–20) and three viral proteins: hemagglutinin (HA), neuraminidase (NA), and the M2 ion channel (21). The viral matrix protein, M1 supports the envelope on its inner aspect. The genome consists of eight discrete, negative-sense, single-stranded RNA segments that each associate with viral nucleoprotein and an RNA-dependent RNA polymerase complex to form eight rod-like structures.
2.1 Determinants of virion inhalation into alveoli
Virions inhaled into the lungs are conducted through a series of branching airways of successively smaller diameter that transition distally from terminal bronchioles to acini, which include respiratory bronchioles, alveolar ducts, and alveoli. Alveoli are cup-like chambers that average 100-250 um in diameter in human lungs and comprise more than 95% – the vast majority – of the lung luminal surface (Figure 1) (22, 23). Despite their extensive surface area, alveoli are not the primary site of deposition for all inhaled particles. Only particles smaller than about 2 um diameter appear to be preferentially deposited in alveoli after inhalation (24). Studies of viral transmission suggest IAV virions are inhaled in liquid-containing particles as small as 1.5 um diameter (25–27) – a size that makes them likely to reach alveoli based on particle inhalation studies in human lungs (24). The possibility that inhaled IAV virions reach alveoli is supported by pathological studies of fixed lungs of rodents, primates, and humans with severe IAV infection, which identify virions, viral nucleic acids, and viral proteins associated with the alveolar epithelial type 1 (AT1) and type 2 (AT2) cells that line alveolar walls (28–31).
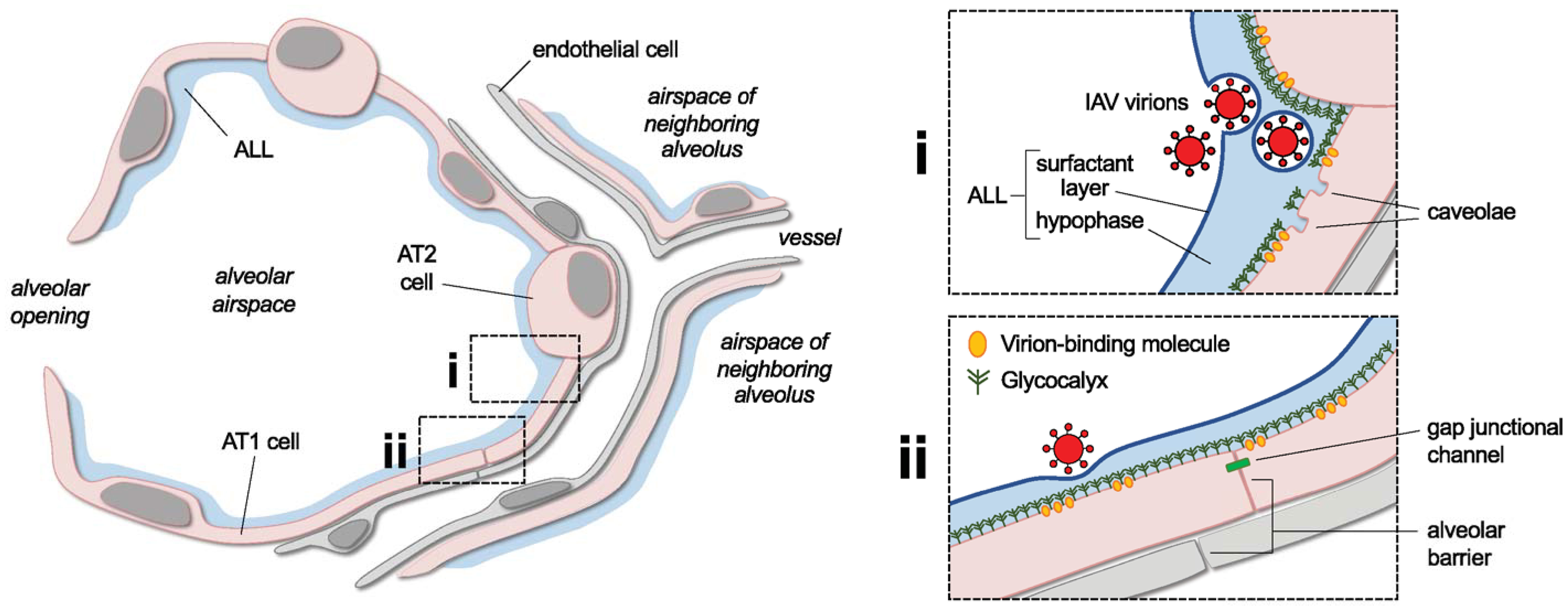
Figure 1 Overview of lung alveolar structure and selected features to be addressed in future research of the alveolar endocytosis of IAV virions. The cartoon shows a lung alveolus and parts of neighboring alveoli and microvessels. The alveolar lining layer (ALL) and alveolar epithelial type 1 (AT1) and 2 (AT2) cells are indicated. Boxed cartoons at right show enlarged views of area i, an alveolar corner, and area ii, a flat alveolar region. In i, the corner is depicted as a site of aggregation of inhaled IAV virions. Two virions are shown covered by a phospholipid film derived from the ALL’s surfactant layer. Labels highlight ALL components and plasma membrane caveolae, which are abundant in AT1 cells and located primarily near AT1-AT2 cell junctions. In ii, a virion interacts with the alveolar surface. Symbols approximate the epithelial glycocalyx and virion-binding molecules in alveolar epithelial membranes, and labels point out epithelial and endothelial components of the alveolar barrier and the intercellular gap junctional channels that conduct epithelial cell-cell signaling. See main text for discussion of how the depicted structural features might bear on the alveolar pathogenesis of IAV and may be addressed in future research. Note, in the cartoon, AT1 cells are shown as thicker than neighboring structures in order to emphasize the epithelium. However, publications including reference #153 indicate that the epithelium is, in fact, thinner than the interstitium and endothelial cells in alveoli of mammalian lungs. Size relationships between virions, AT1 cells, the glycocalyx, and the ALL are drawn approximately to scale.
Whether inhaled particles, including IAV virions, reach alveoli is partly governed by the balance of convective and diffusive transport of gases in the lung. Broadly, convection refers to bulk transport of gases in the airways, while diffusion relates to gas mixing in acinar regions (32). The balance between convection and diffusion determines particle transport in non-lung model systems (33, 34). In healthy lungs, the convective-diffusive balance is neither static nor uniform. Rather, it varies with breathing pattern (24, 32) and across acinar geometries (35) that, themselves, change with age (36, 37) and vary by lung region (38, 39). At the acinar level, air flow represents a combination of convection and diffusion that generates chaotic gas mixing where inhaled and residual acinar gases meet (40–42). Going forward, better understanding is needed of how local and regional gas transport mechanisms affect the alveolar inhalation of IAV virions, since the inhalation of virion-sized particles into acini and alveoli is influenced by factors that affect the convective-diffusive balance (24, 35, 37, 43).
There is notably little understanding of how chronic lung diseases bear on the alveolar inhalation of IAV virions. Human aerosol inhalation studies (44–47) and computational models (48) suggest that airway obstruction due to asthma and chronic obstructive pulmonary disease (COPD) promotes the deposition of inhaled particles in central lung airways. By contrast, lung compliance changes related to aging and obesity may promote particle deposition in more distal lung regions, including alveoli (49–51). Clinical studies that associate severe IAV infection with aging and obesity – but not asthma or COPD (52–55) – raise the possibility that the tendency to inhale particles into distal lung regions promotes IAV pathogenesis. Experimental studies that relate airway obstruction, lung compliance, and IAV pathogenesis to the microanatomical location of inhaled particles might clarify these issues.
Finally, virion inhalation into alveoli is likely to be influenced by virion morphology. IAV virions assume spherical, bacilliform, or filamentous shapes in response to host and viral factors (56–58). Spherical and bacilliform virions range 120-150 nm in length, but filamentous virions can extend tens of microns long (56). Although studies of fixed lung tissue demonstrate the presence of all virion morphologies in IAV-infected human lungs (59, 60), the relevance of virion morphology to the alveolar pathogenesis of IAV is not clear. While electron microscopic data suggest that spherical and bacilliform virions associate with the alveolar epithelium (60), the relatively large size of filamentous virions may inhibit their inhalation into alveoli. Future studies might clarify how virion morphology affects alveolar inhalation dynamics and the extent to which inhaled filamentous virions initiate alveolar IAV infection.
2.2 Significance of alveolar structure for IAV virion inhalation
Once inhaled IAV virions reach alveoli, alveolar structure may influence how virions establish contact with alveolar walls. Alveolar structure is defined by flat tissue surfaces that alternate with curved, corner-like regions to form a unique tissue architecture. At flat septal regions, a thin band of tissue and surface lining liquid separates alveolar airspaces from the microvascular lumens of the adjacent capillary meshwork (23, 61). Flat septal regions converge to form more than five corner-like structures per alveolus (62), where the radius of corner curvature varies with the degree of lung inflation (63).
Alveolar structure might determine viral entry mechanisms by shaping how virions are presented to alveolar cells. Our group has shown, by live imaging of intact lungs, that alveolar corners are sites of accumulation for bacteria, inert particles, and liquid (64, 65). The extent to which virions also accumulate at alveolar corners is not clear. If such accumulation occurs, it might facilitate contact between IAV virions and AT2 cells, which are located primarily at alveolar corners (66, 67) and serve functions critical to alveolar homeostasis and injury responses.
Virion localization to corners or other alveolar sites may be determined by air flow patterns that arise from the unique structure of alveoli. Thus, an encounter with an alveolar opening causes fluid flowing in an airway to change from a linear flow pattern to a curved flow pattern, oriented into the alveolus (37, 40, 41). Simulation and experimental data show that the vectors of curved flow form a gradient, with vectors of lesser curvature intersecting with alveolar walls and vectors of greater curvature forming vortices in airspaces (37, 40, 41). Such rotational flow patterns may promote the deposition of particles on alveolar walls (37, 68), but the extent to which they determine virion deposition in alveoli is not clear. Better understanding in this area seems particularly important in the lungs of young children, whose developing acinar structure may promote rotational air flows and particle deposition in alveoli (37, 68), and who experience disproportionately high mortality from IAV infection (69–73).
Breathing also causes changes in alveolar structure that might further influence the deposition of inhaled IAV virions in alveoli. Using live imaging of intact, perfused lungs, our group showed that lung inflation causes a heterogeneous distribution of alveolar distention, with the greatest increase of alveolar septal length occurring at flat segments lined by AT1 cells (67). Interestingly, alveolar expansion is lost with aging in a manner that correlates with age-related increases of perialveolar and subpleural collagen density (74), suggesting that age-related changes in lung tissue have a constraining effect on alveolar micromechanics. Further research is needed to understand how breathing-induced changes of alveolar structure affect particle and virion localization in alveoli and to characterize how aging bears on these issues.
3 IAV virion attachment to the alveolar surface
To initiate viral entry, virions must stabilize on the alveolar surface by attaching to epithelial cells. The alveolar epithelium consists of two cell populations: large, thin AT1 cells that cover the bulk of the alveolar surface, and small, cuboidal AT2 cells. IAV attaches to both AT1 and AT2 cells of fixed lung tissue (75, 76), indicating that both cell types are susceptible to virion attachment.
3.1 Significance of the alveolar glycocalyx for IAV virion attachment
Attachment of IAV virions to host cells is mediated by interactions between the viral HA protein and sialic acids on the host cell surface. The HA protein protrudes from the virion surface and binds sialic acid moieties at a site on the HA head domain (77–79). Sialic acids are 9-carbon sugars typically found at the distal end of glycolipid and glycoprotein components of the host cell glycocalyx, a layer of carbohydrates, proteins, and lipids that covers the surface of all mammalian cells. Strong evidence supports the presence of an airspace-facing glycocalyx on the alveolar epithelial surface (80) that provides potential attachment sites for IAV virions inhaled into alveoli. This, coupled with the high density of HA protein on the virion surface – more than 300 per spherical virion (81) – seems to create ample opportunity for HA-sialic acid interactions in alveoli.
The alveolar pathogenesis of IAV is thought to derive, in part, from the configuration of chemical bonds between sialic acids and the galactose residues that connect them to glycocalyx glycolipids and glycoproteins (82–84). Two such configurations are recognized for their potential relevance to lung infection: an α2,3- link that connects the quaternary C2 carbon of sialic acid to the C3 carbon of galactose, and an α2,6- link that connects the C2 carbon of sialic acid to the C6 carbon of galactose. Although studies using erythrocyte agglutination show that IAV virions bind sialic acids bearing either linkage configuration (85, 86), IAV has strain-specific differences in linkage binding. Thus, the erythrocyte-agglutinating capacity is greater when mammalian-origin IAV strains are exposed to α2,6-bearing erythrocytes, and when avian-origin IAV strains are exposed to α2,3-bearing erythrocytes (85, 86). These findings suggest that IAV virions originating from mammalian versus avian sources have unique capacities for host cell attachment, depending on the sialic acid linkage present at the host cell surface. Notably, landmark studies show that the α2,3- linkage is highly expressed on the alveolar epithelium, and that avian viruses that preferentially bind the α2,3- linkage attach to alveoli of fixed lung tissue and infect the alveolar epithelium of lung tissue blocks (87, 88). Hence, the presence of the α2,3-galactose linkage on the alveolar epithelium is suggested to underlie the extreme severity of lung infection by avian IAV strains (83, 84) that cause severe lung injury with high mortality (89–93).
However, recent findings suggest the role of sialic acid linkages in the alveolar pathogenesis of IAV is more complex. The alveolar epithelium expresses sialic acids of both the α2,3- (76, 87, 94) and α2,6- (76, 87) linkage configurations, and specific linkage configurations may not be required for IAV attachment and infection in the lung (95, 96). In fact, virion attachment to cultured cells is enhanced by a mix of sialic acid linkage configurations (97), suggesting that linkage diversity, rather than expression of a single configuration, might determine virion binding to the alveolar epithelium. Moreover, virion affinity for specific linkages may change over time, since HA-sialic acid interactions are affected by point mutations in the HA head domain (77–79), and mutations accumulate within strains and occur frequently in the course of infection (98–100). The intra-infection mutation rate may be considerable, since longitudinal sequencing data from IAV-infected human subjects show that multiple mutations causing amino acid changes in HA occurred in nearly all subjects in the first days of IAV infection (100). The potential impact of such mutations is evident from reports showing that changes in only two amino acids alters the sialic acid binding specificity of HA (101, 102). Taking the sialic acid data together, we interpret that epithelial sialic linkage configurations likely impact the spatial profile of virion attachment on the alveolar surface. However, future research might address how α2,3- and α2,6- linkages distribute in alveoli and how linkage partitioning or blending affects virion attachment.
Emerging data suggest that non-sialic acid components of the host cell surface, such as carcinoembryonic cell adhesion molecule 6 (CEACAM6, also known as CD66c) and macrophage galactose-type lectin 1 (MGL1), contribute to virion attachment mechanisms (103, 104). CEACAM6 and MGL1 may determine virion attachment to alveoli in vivo, since they are each expressed by the alveolar epithelium (105, 106). High-throughput approaches have identified other novel potential attachment mediators in alveolar epithelial-like A549 cells (107), but their relevance to virion attachment in alveoli has yet to be defined.
The extent to which virion attachment leads to endocytosis in intact alveoli may be determined by glycocalyx shedding. Intranasal instillation of IAV in mice induces shedding of the lung epithelial glycocalyx (108). Shedding releases glycocalyx components into airspaces (108), leading to a reduction of glycocalyx height and density (109). Shedding might defend against virion endocytosis by coating virions in shed matrix components and displacing them from the epithelial surface, but direct evidence for this possibility is lacking. Human studies that show evidence of glycocalyx shedding in severe lung infection (110) support the potential relevance of glycocalyx shedding to the alveolar pathogenesis of IAV. Further research might clarify how shedding of the alveolar epithelial glycocalyx affects viral entry in alveoli.
3.2 Significance of the alveolar lining layer for IAV virion attachment
The alveolar wall is covered by a lining layer that may provide a physical barrier to virion-glycocalyx interactions. The alveolar lining layer consists of an aqueous hypophase underlying a film of surfactant phospholipids and proteins at the air-liquid interface. Although the lining layer covers the alveolar wall in a continuous fashion, its depth varies across the alveolar surface (61). Thus, thin portions of the lining layer project an average height of 140 nm from the alveolar wall on flat surfaces of rat alveoli, and thicker portions project 1,000 nm or more at alveolar corners (61). The lining layer completely submerges alveolar epithelial cells and alveolar macrophages even at its thinnest parts (61), but whether the lining layer entirely submerges the glycocalyx remains unclear. Data generated by thorium dioxide-based electron microscopy show that the epithelial glycocalyx height also varies across alveolar regions, with its shortest level on the surfaces of AT1 cells that line flat alveolar walls (109). The extent to which the alveolar lining layer shields the epithelial glycocalyx from virion attachment in alveoli in vivo remains uncertain, but it may have implications for viral entry mechanisms, particularly on flat alveolar surfaces.
Surfactant proteins and phospholipids might also inhibit virion attachment to the alveolar epithelium. Reports indicate that particles inhaled into alveoli of rodent lungs become submerged in the alveolar lining layer and coated by a phospholipid film (111). There is little understanding of how surfactant phospholipids interact with inhaled IAV virions in alveoli. Since surfactant proteins enhance the uptake of IAV virions by macrophages in vitro (112), surfactant-virion interactions in alveoli in vivo might promote virion phagocytosis by alveolar macrophages. A protective role for surfactant proteins is supported by mouse models of IAV infection, in which mice with deficiencies of surfactant proteins A and D have impaired viral clearance from the lung (113, 114). Recombinant surfactant protein D binds the viral HA protein to protect against IAV uptake and replication in lungs of IAV-infected mice (115, 116), supporting the therapeutic potential of surfactant proteins in IAV infection.
The aqueous portion of the alveolar lining layer might further limit virion attachment to alveolar walls. Our group has shown that, under baseline conditions, the aqueous hypophase of the alveolar lining layer is secreted continuously by the alveolar epithelium (65, 117). The secretion generates a flow of alveolar wall liquid on the alveolar surface that clears particles from alveoli by convective transport (117). Alveolar wall liquid secretion also promotes the alveolar clearance of S. aureus bacteria (64, 65), but whether the secretion clears IAV virions from alveoli, thereby blocking their endocytosis, is not clear. Since we reported recently that IAV disrupts alveolar wall liquid secretion (65), the capacity of the secretion to clear virions may change in IAV-infected lungs over the course of lung infection.
4 IAV virion endocytosis by the alveolar epithelium
Binding of IAV virions to sialic acids is neither necessary (118) nor sufficient (119) to induce endocytosis by cultured cells, suggesting that the endocytosis is mediated by non-sialic acid interactions between virions and host membrane proteins. Here, we will address the known pathways of IAV virion endocytosis that are supported by evidence generated in alveoli, cultured alveolar cells, or cultured alveolar-like cells. Broadly, reports indicate that IAV virions exploit both clathrin-dependent and clathrin-independent endocytic pathways.
4.1 Clathrin-dependent mechanisms in alveoli
Evidence supports clathrin-mediated endocytosis (CME) as a mechanism of IAV virion uptake by cultured cells of non-alveolar origin (120–123). Briefly, CME is triggered by interactions between extracellular cargo and the plasma membrane phosphoinositide, PI(4,5)P2, that lead to binding of PI(4,5)P2 by the cytosolic adaptor protein 2 (AP2) (124, 125). AP2 stimulates the assembly of a protein complex that includes the scaffolding protein, clathrin. Lateral enlargement of the clathrin complex along the inner plasma membrane surface forms a polyhedral clathrin-containing “coat” that, along with polymerization of an actin filament network, induces plasma membrane curvature to form an endocytic vesicle. The developing vesicle is progressively constricted at the vesicle neck, then released into the cytosol by the cooperative activity of dynamin, an intracellular GTPase, and proteins of the Bin/amphiphysin/Rvs (BAR) domain family.
IAV virions are taken up by CME in cultured alveolar epithelial-like cells. Thus, data generated in cultured, alveolar epithelial-like A549 cells indicate that IAV virions interact with the epidermal growth factor receptor (EGFR), free fatty acid receptor 2 (FFAR2), or transferrin receptor 1 (TfR1) to initiate endocytosis by CME (126–128). Importantly, genetic inhibition of each protein blocks IAV entry and replication (126–128). Although these findings provide strong evidence that EGFR, FFAR2, TfR1, and CME drive viral entry mechanisms in A549 cells, future studies are needed to determine their roles the alveolar epithelium, since A549 cells are a cancer cell line (129) that exhibit features not shared with either AT1 or AT2 cells (129, 130). However, single cell transcriptomic datasets indicate EGFR, FFAR2, and TfR1 are each expressed by the alveolar epithelium of human lungs (131), bolstering the potential relevance of these proteins as initiators of CME for IAV virion endocytosis in alveoli.
4.2 Clathrin-independent mechanisms in alveoli
Clathrin-independent endocytic pathways may also mediate the alveolar epithelial uptake of IAV virions. In non-alveolar epithelial cells, IAV virions are taken up by multiple clathrin-independent pathways including caveolae-mediated endocytosis (132), macropinocytosis (133, 134) and a mechanism that involves the small GTPase, Cdc42 (135). Few studies have addressed whether such clathrin-independent pathways facilitate the endocytosis of IAV virions in alveoli. There is evidence that, in cultured alveolar epithelial-like A549 cells, the virion endocytic pathway initiated by EGFR that was discussed earlier in this review proceeds not only through CME, but also through an endocytic pathway that involves the caveolin-1 protein (126). While the mechanistic details of the caveolin-1 pathway remain uncertain, support for caveolin-1 as a potential mediator of virion endocytosis in alveoli comes from studies of uptake mechanisms of non-virion cargo. Genetic inhibition of caveolin-1 blocks endocytosis of P. aeruginosa (136) and nanoparticles (137, 138) in cultured alveolar epithelial-like cells, indicating caveolin-1 is critical to the endocytic mechanisms. Immunolabeling studies that identify caveolin-1 in AT1 and AT2 cells of fixed rat lungs (139) lend plausibility to a role for caveolin-1 in alveolar function. Taken together, these data support the possibility that caveolin-1 mediates endocytosis of IAV virions in the alveolar epithelium, but further studies are needed to draw this conclusion definitively.
Caveolin-1 is known to associate with plasma membrane domains called caveolae, but endocytic pathways mediated by caveolin-1 protein may occur independently of membrane caveolae in alveoli. Caveolae are plasma membrane regions defined by their pit-shaped architecture and specific lipid and protein composition, which includes core structural proteins such as caveolin-1. Membrane caveolae are traditionally thought to mediate endocytosis (140–143), but emerging views call into question the extent to which caveolae participate in endocytic mechanisms (144–146). This issue is relevant to alveoli because expression patterns of caveolin-1 protein and membrane caveolae are discordant in cells of the alveolar epithelium. Thus, caveolin-1 protein is expressed by both AT1 and AT2 cells (139, 147–151), but membrane caveolae are identified only in AT1 cells (139). Yet, immunofluorescence studies of cultured AT2 cells show caveolin-1 protein colocalizes with albumin taken up by endocytosis (152), suggesting caveolin-1 participates in endocytic mechanisms in alveolar epithelial cells that lack membrane caveolae.
In AT1 cells, the extent to which membrane caveolae and caveolin-1 protein mediate endocytosis remains unclear. Better understanding of their roles may be important, since alveolar epithelial expression of caveolin-1 protein occurs primarily in AT1 cells (139, 147–151), and AT1 cell membranes contain numerous caveolae. In fact, Gil et al. determined AT1 cells contain more than 150 vesicular structures per um2 of the luminal plasma membrane surface in unchallenged rabbit lungs (153). Even though immunolocalization studies suggest only some of the vesicular structures are caveolae (139), the calculations of Gil et al., taken in the context of the extensive surface area of AT1 cells (154), suggest caveolae number more than half a million in the AT1 cells that line alveolar walls. Immunolabeling studies identify caveolin-1 protein near AT1-AT2 cell junctions (139), and electron microscopic images demonstrate few caveolae in AT1 cell regions that line flat alveolar septa (23, 153, 155). This paucity of caveolin-1 protein and membrane caveolae at flat alveolar septa suggests that the endocytosis of IAV virions occurs at non-septal regions of the intact alveolus.
4.3 Virion motility across the alveolar surface
Reports indicate virion-epithelial interactions that lead to endocytosis are spatially and temporally dynamic and might localize virions to membrane sites conducive to endocytosis. After virion HA binds to host sialic acids, cooperative activity of HA and the viral neuraminidase (NA), an enzyme that cleaves sialic acids to release them from the glycocalyx, generates a series of sialic acid binding and cleavage events that induces IAV virion motility across a sialic acid-coated surface (156–158). If such motility occurs on the alveolar surface, it might move virions into proximity to EGFR, FFAR2, or TfR1. IAV virions also induce movement of host endocytic factors to sites of virion attachment. In cultured kidney epithelial-like cells, IAV virions induce clathrin accumulation at virion binding sites (120), perhaps promoting CME. Virion-induced plasma membrane clustering of the lipid raft ganglioside, GM1 (126) may lead to interactions between IAV virions and GM1 that are pro-endocytic (159). In alveoli, we do not identify evidence to support virion motility or to indicate whether such motility, if it occurs, promotes endocytosis.
5 Post-endocytic events that lead to alveolar barrier dysfunction
After endocytosis, IAV virions undergo a number of processing events that establish host cell infection: (i) endosomal trafficking; (ii) fusion with endosomal membranes to release viral nucleoproteins into the host cell cytosol; (iii) nucleoprotein trafficking to the host cell nucleus; (iv) viral RNA replication and transcription; (v) viral mRNA export to the cytosol; (vi) mRNA translation and viral protein processing; and (vii) virion formation and release via assembly and budding of viral proteins and nucleoproteins at the host cell plasma membrane. Here, we will briefly address the consequences of IAV virion endocytosis for alveolar function, specifically function of the alveolar barrier.
5.1 Alveolar barrier loss mechanisms
IAV lung infection causes dysfunction of the alveolar barrier, leading to pulmonary edema formation (160–166). Under baseline conditions, the alveolar barrier restricts the passage of proteins and small molecules from microvessels into airspaces to maintain airspace patency. The barrier is comprised of alveolar epithelial and microvascular endothelial cells that share a basement membrane (167). Barrier permeability is regulated at sites of contact between adjacent epithelial or adjacent endothelial cells by tight junctional protein complexes that incorporate claudins, occludins, junctional adhesion molecules, and cytoskeleton-binding adaptor proteins (168). Epithelial cells are the chief regulators of alveolar barrier permeability (169–171) due to the high density of junctional complexes at epithelial intercellular surfaces (172) and the large size of AT1 cells (154), which minimizes the total junctional surface area (173).
Mechanisms of alveolar barrier loss may include direct effects of IAV infection on alveolar epithelial barrier proteins. In cultured A549 cells, H1N1 IAV exposure causes loss of the tight junction proteins, occludin and ZO-1, and the adherens junction protein, E-cadherin by inhibiting junctional protein transcription (174). Although junctional protein loss is also reported in A549 cells exposed to H5N1 IAV, it results from protein degradation via the E3 ubiquitin ligase, Itch (93). Inhibition of the upstream kinase, TAK1 restores junctional protein expression in H5N1-exposed cultured cells, and intraperitoneal pretreatment with a TAK1 inhibitor restores alveolar junctional protein expression and increases survival in H5N1-infected mice (93). Findings by others confirm loss of the tight junction protein, claudin-4, in cells of the distal lung epithelial cancer line, NCI-H441, after exposure to either H5N1 or H1N1 IAV (175). Taken together, these findings provide evidence that IAV causes loss of alveolar epithelial junctional proteins by pre- and post-translational mechanisms, leading directly to loss of alveolar barrier function.
Indirect mechanisms may also mediate IAV-induced alveolar barrier loss. Reports indicate IAV induces proinflammatory signaling in alveolar epithelial and alveolar epithelial-like cells (176–184), leading to inflammation-induced alveolar damage (185–188). Numerous studies support the notion that IAV induces cell death in the alveolar epithelium (31, 161, 164, 189–191) and in cultured alveolar epithelial and alveolar epithelial-like cells (166, 192, 193), contributing to barrier loss. Once edema forms, IAV-induced alterations of alveolar epithelial ion channel function may further dysregulate alveolar liquid dynamics to potentiate the edema response (160, 194–198). Together, these data support the capacity of IAV to induce alveolar barrier loss by indirect effects on alveolar barrier function.
Although we do not address them in this review, other mechanisms may contribute to barrier loss and edema formation in IAV-infected lungs but are not well understood. For example, the interplay between surfactant, surface tension, and barrier permeability in alveoli of IAV-infected lungs may have implications for edema formation and virion distribution. Thus, IAV infection of AT2 cells (199, 200) may cause loss of surfactant secretion, leading to increase of alveolar surface tension and edema formation by a mechanism proposed by Pattle (201) and Clements (202) and supported by experimental data (203, 204). How this edema formation might affect the subsequent distribution of virions in alveoli is not clear. In addition, the specific mechanisms by which different IAV strains affect alveolar barrier function remain unclear, but they may relate to viral factors directly (205, 206) or to how viruses interact with the host (207–211). New insights in this area might address the disproportionately high morbidity and mortality caused by pandemic and avian IAV strains (212, 213). Finally, a growing literature supports a role for circadian rhythms in lung inflammation and repair in lungs that are mechanically ventilated (214) and IAV-infected (215–217). Better understanding of how sleep and circadian rhythm disruption affects alveolar responses to IAV may lead to new therapeutic approaches for infection-induced critical illness (218, 219).
5.2 Significance of alveolar communication pathways for barrier loss
Recent advances highlight the potential for cell-cell communication pathways present in intact alveoli to amplify the barrier dysfunction that follows virion endocytosis (220). Necropsy studies of human lungs with severe IAV infection identify little, if any, viral nucleic acids, protein, or cytopathic changes in the alveolar epithelium at sites of alveolar damage and barrier loss, at the same time that such evidence of active IAV infection is detectable elsewhere in the lungs (191, 221–224). Although these findings might reflect the tendency of tissue damage to linger after the resolution of viral infection, alternatively, they could reflect tissue damage that occurred due to spread of alveolar damage signals from infected to uninfected alveoli.
Interferon signaling might spread alveolar damage and barrier dysfunction in intact alveoli of IAV-infected lungs. Interferon signals spread from IAV-infected to uninfected cells in culture (225) and in alveoli (226), and interferon exposure causes loss of tight junctional proteins and barrier permeability in cultured intestinal epithelial-like cells (227, 228). These data raise the possibility that barrier-deteriorating interferon signals spread from infected to uninfected alveoli in IAV-exposed lungs, such that alveolar epithelial cells need not take up IAV virions directly to lose barrier function. A critical role for interferon signaling in influenza infection is supported by human data that identify genetic mutations in the interferon system in patients with influenza-induced critical illness (229–231).
Gap junctional communication in intact alveoli might also expand the scope of IAV-induced barrier loss. We have shown connexin 43-containing gap junctional channels conduct cytosolic Ca2+ signals in the alveolar epithelium (64, 232, 233) that, in S. aureus-infected alveoli, spread barrier loss from infected to uninfected alveolar regions (64). Since reports indicate IAV infection induces cytosolic Ca2+ increases in alveolar epithelial-like A549 cells (234, 235), IAV might also induce spread of cytosolic Ca2+ signals that amplify the barrier loss effects of virion endocytosis. Evidence is needed to determine whether this occurs in intact alveoli.
6 Conclusion
This review highlights key mechanistic steps underlying virion endocytosis in alveoli of IAV-infected lungs. Evidence from fixed lung tissue supports the notion that inhaled IAV virions can attach to AT1 and AT2 cells of the alveolar epithelium. The attachment mechanisms may involve virion interactions with sialic acids of the alveolar glycocalyx and alveolar epithelial surface proteins, such as CEACAM6 and MGL1. Data generated in cultured cells indicate that, after attachment, IAV virions are taken up by clathrin-dependent and clathrin-independent endocytic mechanisms. In this regard, EGFR-, FFAR2-, TfR1, and caveolin-1 may drive alveolar endocytic responses and provide new opportunities for the development of host-directed therapies that block viral entry at its earliest mechanistic steps. Finally, endocytosis of IAV virions leads to alveolar barrier permeability, and evidence derived from cultured cells suggests that the permeability responses might result from loss of alveolar barrier proteins. Targeting these responses may likewise yield new therapeutic approaches.
However, we point out that the unique structure of intact alveoli raises numerous, as yet unstudied questions about the mechanisms by which IAV virions are inhaled into alveoli, attach to the alveolar surface, and are taken up by the alveolar epithelium. For example, whether virions accumulate at alveolar corners is not clear but may determine which cells of the alveolar epithelium are exposed to and take up the virus. The extent to which virion attachment to the alveolar surface is opposed by glycocalyx shedding, surfactant binding, and alveolar wall liquid secretion in uncertain but may bear on the efficacy of virion attachment and internalization in intact alveoli. Alveolar cell-cell communication pathways may spread virus-induced interferon and Ca2+ responses, perhaps negating the need for virion endocytosis in barrier loss mechanisms. Thus, new insights are needed to generate a more fundamental understanding of IAV lung pathogenesis mechanisms that takes into account these unique structural features of intact alveoli. A better understanding of how alveolar structure shapes alveolar interactions with IAV may lead to novel approaches to therapy for IAV-induced lung injury that disrupt virion endocytosis and the barrier dysfunction that follows.
Author contributions
JH: Conceptualization, Writing – original draft, Writing – review & editing. JB: Conceptualization, Writing – review & editing.
Funding
The author(s) declare financial support was received for the research, authorship, and/or publication of this article. This work was supported by NIH grant R01HL164821, Cystic Fibrosis Foundation Research Grant 004792G222, and American Lung Association COVID-19 and Emerging Respiratory Viruses Research Award 1031520 to JH and R01HL036024 and R01HL122730 to JB.
Acknowledgments
We thank Dr. Sunita Bhattacharya for helpful suggestions.
Conflict of interest
The authors declare that the research was conducted in the absence of any commercial or financial relationships that could be construed as a potential conflict of interest.
Publisher’s note
All claims expressed in this article are solely those of the authors and do not necessarily represent those of their affiliated organizations, or those of the publisher, the editors and the reviewers. Any product that may be evaluated in this article, or claim that may be made by its manufacturer, is not guaranteed or endorsed by the publisher.
References
1. Taubenberger JK, Morens DM. Influenza: the once and future pandemic. Public Health Rep (2010) 125 Suppl 3(Suppl 3):16–26. doi: 10.1177/00333549101250S305
2. Saunders-Hastings PR, Krewski D. Reviewing the history of pandemic influenza: understanding patterns of emergence and transmission. Pathogens (2016) 5(4):66. doi: 10.3390/pathogens5040066
3. Iuliano AD, Roguski KM, Chang HH, Muscatello DJ, Palekar R, Tempia S, et al. Estimates of global seasonal influenza-associated respiratory mortality: a modelling study. Lancet (2018) 391(10127):1285–300. doi: 10.1016/S0140-6736(17)33293-2
4. Paget J, Spreeuwenberg P, Charu V, Taylor RJ, Iuliano AD, Bresee J, et al. Global mortality associated with seasonal influenza epidemics: new burden estimates and predictors from the GLaMOR Project. J Glob Health (2019) 9(2):020421. doi: 10.7189/jogh.09.020421
5. Louie JK, Acosta M, Winter K, Jean C, Gavali S, Schechter R, et al. Factors associated with death or hospitalization due to pandemic 2009 influenza A(H1N1) infection in California. JAMA (2009) 302(17):1896–902. doi: 10.1001/jama.2009.1583
6. Hsu JC, Lee IK, Huang WC, Chen YC, Tsai CY. Clinical characteristics and predictors of mortality in critically ill influenza adult patients. J Clin Med (2020) 9(4):1073. doi: 10.3390/jcm9041073
7. Bhattacharya J, Matthay MA. Regulation and repair of the alveolar-capillary barrier in acute lung injury. Annu Rev Physiol (2013) 75:593–615. doi: 10.1146/annurev-physiol-030212-183756
8. Ploin D, Chidiac C, Carrat F, Cohen B, Javouhey E, Mayaud C, et al. Complications and factors associated with severity of influenza in hospitalized children and adults during the pandemic wave of A(H1N1)pdm2009 infections–the Fluco French cohort. J Clin Virol (2013) 58(1):114–9. doi: 10.1016/j.jcv.2013.05.025
9. Wang H, Xiao X, Lu J, Chen Z, Li K, Liu H, et al. Factors associated with clinical outcome in 25 patients with avian influenza A (H7N9) infection in Guangzhou, China. BMC Infect Dis (2016) 16(1):534. doi: 10.1186/s12879-016-1840-4
10. Lobo SM, Watanabe ASA, Salomao MLM, Queiroz F, Gandolfi JV, de Oliveira NE, et al. Excess mortality is associated with influenza A (H1N1) in patients with severe acute respiratory illness. J Clin Virol (2019) 116:62–8. doi: 10.1016/j.jcv.2019.05.003
11. Shi Y, Chen W, Zeng M, Shen G, Sun C, Liu G, et al. Clinical features and risk factors for severe influenza in children: a study from multiple hospitals in Shanghai. Pediatr Neonatol. (2021) 62(4):428–36. doi: 10.1016/j.pedneo.2021.05.002
12. Hussain M, Galvin HD, Haw TY, Nutsford AN, Husain M. Drug resistance in influenza A virus: the epidemiology and management. Infect Drug Resist (2017) 10:121–34. doi: 10.2147/IDR.S105473
13. Hayden FG, de Jong MD. Emerging influenza antiviral resistance threats. J Infect Dis (2011) 203(1):6–10. doi: 10.1093/infdis/jiq012
14. Madhav N, Oppenheim B, Gallivan M, Mulembakani P, Rubin E, Wolfe N. Pandemics: risks, impacts, and mitigation. In: Jamison DT, Gelband H, Horton S, Jha P, Laxminarayan R, Mock CN, Nugent R, editors. Disease Control Priorities: Improving Health and Reducing Poverty, 3rd Edition. Washington, DC: The International Bank for Reconstruction and Development (2017).
15. Herold S, Becker C, Ridge KM, Budinger GR. Influenza virus-induced lung injury: pathogenesis and implications for treatment. Eur Respir J (2015) 45(5):1463–78. doi: 10.1183/09031936.00186214
16. Vanderlinden E, Naesens L. Emerging antiviral strategies to interfere with influenza virus entry. Med Res Rev (2014) 34(2):301–39. doi: 10.1002/med.21289
17. Li Y, Huo S, Yin Z, Tian Z, Huang F, Liu P, et al. The current state of research on influenza antiviral drug development: drugs in clinical trial and licensed drugs. mBio (2023) 14(5):e0127323. doi: 10.1128/mbio.01273-23
18. Shaw ML, Stone KL, Colangelo CM, Gulcicek EE, Palese P. Cellular proteins in influenza virus particles. PLoS Pathog (2008) 4(6):e1000085. doi: 10.1371/journal.ppat.1000085
19. Gerl MJ, Sampaio JL, Urban S, Kalvodova L, Verbavatz JM, Binnington B, et al. Quantitative analysis of the lipidomes of the influenza virus envelope and MDCK cell apical membrane. J Cell Biol (2012) 196(2):213–21. doi: 10.1083/jcb.201108175
20. Hutchinson EC, Charles PD, Hester SS, Thomas B, Trudgian D, Martinez-Alonso M, et al. Conserved and host-specific features of influenza virion architecture. Nat Commun (2014) 5:4816. doi: 10.1038/ncomms5816
21. Dou D, Revol R, Ostbye H, Wang H, Daniels R. Influenza A virus cell entry, replication, virion assembly and movement. Front Immunol (2018) 9:1581. doi: 10.3389/fimmu.2018.01581
22. Mercer RR, Russell ML, Roggli VL, Crapo JD. Cell number and distribution in human and rat airways. Am J Respir Cell Mol Biol (1994) 10(6):613–24. doi: 10.1165/ajrcmb.10.6.8003339
23. Weibel ER. The Pathway for Oxygen: Structure and Function in the Mammalian Respiratory System. Cambridge, Massachusetts: Harvard University Press (1984).
24. Heyder J, Gebhart J, Rudolf G, Schiller CF, Stahlofen W. Deposition of particles in the human respiratory tract in the size range 0.005-15 μm. J Aerosol Sci (1986) 17(5):811–25. doi: 10.1016/0021-8502(86)90035-2
25. Gralton J, Tovey ER, McLaws ML, Rawlinson WD. Respiratory virus RNA is detectable in airborne and droplet particles. J Med Virol (2013) 85(12):2151–9. doi: 10.1002/jmv.23698
26. Milton DK, Fabian MP, Cowling BJ, Grantham ML, McDevitt JJ. Influenza virus aerosols in human exhaled breath: particle size, culturability, and effect of surgical masks. PLoS Pathog (2013) 9(3):e1003205. doi: 10.1371/journal.ppat.1003205
27. Zhou J, Wei J, Choy KT, Sia SF, Rowlands DK, Yu D, et al. Defining the sizes of airborne particles that mediate influenza transmission in ferrets. Proc Natl Acad Sci USA (2018) 115(10):E2386–92. doi: 10.1073/pnas.1716771115
28. Loosli CG, Stinson SF, Ryan DP, Hertweck MS, Hardy JD, Serebrin R. The destruction of type 2 pneumocytes by airborne influenza PR8-A virus; its effect on surfactant and lecithin content of the pneumonic lesions of mice. Chest (1975) 67(2 Suppl):7S–14S. doi: 10.1378/chest.67.2_Supplement.7S
29. Stinson SF, Ryan DP, Hertweck S, Hardy JD, Hwang-Kow SY, Loosli CG. Epithelial and surfactant changes in influenzal pulmonary lesions. Arch Pathol Lab Med (1976) 100(3):147–53.
30. Gu J, Xie Z, Gao Z, Liu J, Korteweg C, Ye J, et al. H5N1 infection of the respiratory tract and beyond: a molecular pathology study. Lancet (2007) 370(9593):1137–45. doi: 10.1016/S0140-6736(07)61515-3
31. Wonderlich ER, Swan ZD, Bissel SJ, Hartman AL, Carney JP, O'Malley KJ, et al. Widespread virus replication in alveoli drives acute respiratory distress syndrome in aerosolized H5N1 influenza infection of macaques. J Immunol (2017) 198(4):1616–26. doi: 10.4049/jimmunol.1601770
32. Butler JP, Tsuda A. Transport of gases between the environment and alveoli–theoretical foundations. Compr Physiol (2011) 1(3):1301–16. doi: 10.1002/cphy.c090016
33. Gomes MSP, Pui DYH, Vincent JH, Liu BYH. Convective and diffusive dispersion of particles in laminar tube flow: effects on time-dependent concentration measurements. J Aerosol Sci (1993) 24(5):643–54. doi: 10.1016/0021-8502(93)90021-Z
34. Wu S, Chen Y, Qi C, Liu C, Li G, Zhu H. A 3D Monte Carlo simulation of convective diffusional deposition of ultrafine particles on fiber surfaces. Atmosphere (2022) 13(8):1319. doi: 10.3390/atmos13081319
35. Hofemeier P, Sznitman J. Role of alveolar topology on acinar flows and convective mixing. J Biomech Eng. (2014) 136(6):061007. doi: 10.1115/1.4027328
36. Vasilescu DM, Gao Z, Saha PK, Yin L, Wang G, Haefeli-Bleuer B, et al. Assessment of morphometry of pulmonary acini in mouse lungs by nondestructive imaging using multiscale microcomputed tomography. Proc Natl Acad Sci USA (2012) 109(42):17105–10. doi: 10.1073/pnas.1215112109
37. Henry FS, Tsuda A. Onset of alveolar recirculation in the developing lungs and its consequence on nanoparticle deposition in the pulmonary acinus. J Appl Physiol (1985). (2016) 120(1):38–54. doi: 10.1152/japplphysiol.01161.2014
38. Haefeli-Bleuer B, Weibel ER. Morphometry of the human pulmonary acinus. Anat Rec. (1988) 220(4):401–14. doi: 10.1002/ar.1092200410
39. Kizhakke Puliyakote AS, Vasilescu DM, Newell JD Jr., Wang G, Weibel ER, Hoffman EA. Morphometric differences between central vs. surface acini in A/J mice using high-resolution micro-computed tomography. J Appl Physiol (1985). (2016) 121(1):115–22. doi: 10.1152/japplphysiol.00317.2016
40. Tsuda A, Henry FS, Butler JP. Chaotic mixing of alveolated duct flow in rhythmically expanding pulmonary acinus. J Appl Physiol (1985). (1995) 79(3):1055–63. doi: 10.1152/jappl.1995.79.3.1055
41. Tsuda A, Rogers RA, Hydon PE, Butler JP. Chaotic mixing deep in the lung. Proc Natl Acad Sci USA (2002) 99(15):10173–8. doi: 10.1073/pnas.102318299
42. Henry FS, Butler JP, Tsuda A. Kinematically irreversible acinar flow: a departure from classical dispersive aerosol transport theories. J Appl Physiol (1985). (2002) 92(2):835–45. doi: 10.1152/japplphysiol.00385.2001
43. Valberg PA, Brain JD, Sneddon SL, LeMott SR. Breathing patterns influence aerosol deposition sites in excised dog lungs. J Appl Physiol Respir Environ Exerc Physiol (1982) 53(4):824–37. doi: 10.1152/jappl.1982.53.4.824
44. Lin MS, Goodwin DA. Pulmonary distribution of an inhaled radioaerosol in obstructive pulmonary disease. Radiology (1976) 118(3):645–51. doi: 10.1148/118.3.645
45. Smaldone GC, Messina MS. Flow limitation, cough, and patterns of aerosol deposition in humans. J Appl Physiol (1985). (1985) 59(2):515–20. doi: 10.1152/jappl.1985.59.2.515
46. Svartengren M, Philipson K, Camner P. Individual differences in regional deposition of 6-micron particles in humans with induced bronchoconstriction. Exp Lung Res (1989) 15(1):139–49. doi: 10.3109/01902148909069613
47. O'Riordan TG, Walser L, Smaldone GC. Changing patterns of aerosol deposition during methacholine bronchoprovocation. Chest (1993) 103(5):1385–9. doi: 10.1378/chest.103.5.1385
48. Zhang H, Papadakis G. Computational analysis of flow structure and particle deposition in a single asthmatic human airway bifurcation. J Biomech. (2010) 43(13):2453–9. doi: 10.1016/j.jbiomech.2010.05.031
49. Darquenne C, Prisk GK. The effect of aging on aerosol bolus deposition in the healthy adult lung: a 19-year longitudinal study. J Aerosol Med Pulm Drug Deliv. (2020) 33(3):133–9. doi: 10.1089/jamp.2019.1566
50. Bennett WD, Zeman KL. Effect of body size on breathing pattern and fine-particle deposition in children. J Appl Physiol (1985) (2004) 97(3):821–6. doi: 10.1152/japplphysiol.01403.2003
51. Afshar-Mohajer N, Wu TD, Shade R, Brigham E, Woo H, Wood M, et al. Obesity, tidal volume, and pulmonary deposition of fine particulate matter in children with asthma. Eur Respir J (2022) 59(3):2100209. doi: 10.1183/13993003.00209-2021
52. Nguyen-Van-Tam JS, Openshaw PJ, Hashim A, Gadd EM, Lim WS, Semple MG, et al. Risk factors for hospitalisation and poor outcome with pandemic A/H1N1 influenza: United Kingdom first wave (May-September 2009). Thorax (2010) 65(7):645–51. doi: 10.1136/thx.2010.135210
53. Van Kerkhove MD, Vandemaele KA, Shinde V, Jaramillo-Gutierrez G, Koukounari A, Donnelly CA, et al. Risk factors for severe outcomes following 2009 influenza A (H1N1) infection: a global pooled analysis. PLoS Med (2011) 8(7):e1001053. doi: 10.1371/journal.pmed.1001053
54. Yu H, Feng Z, Uyeki TM, Liao Q, Zhou L, Feng L, et al. Risk factors for severe illness with 2009 pandemic influenza A (H1N1) virus infection in China. Clin Infect Dis (2011) 52(4):457–65. doi: 10.1093/cid/ciq144
55. McLean HQ, Hanson KE, Foster AD, Olson SC, Kemble SK, Belongia EA. Serious outcomes of medically attended, laboratory-confirmed influenza illness among school-aged children with and without asthma, 2007-2018. Influenza Other Respir Viruses. (2020) 14(2):173–81. doi: 10.1111/irv.12710
56. Dadonaite B, Vijayakrishnan S, Fodor E, Bhella D, Hutchinson EC. Filamentous influenza viruses. J Gen Virol (2016) 97(8):1755–64. doi: 10.1099/jgv.0.000535
57. Vahey MD, Fletcher DA. Low-fidelity assembly of influenza A virus promotes escape from host cells. Cell (2019) 176(1-2):281–94 e19. doi: 10.1016/j.cell.2018.10.056
58. Seladi-Schulman J, Steel J, Lowen AC. Spherical influenza viruses have a fitness advantage in embryonated eggs, while filament-producing strains are selected in vivo. J Virol (2013) 87(24):13343–53. doi: 10.1128/JVI.02004-13
59. Basu A, Shelke V, Chadha M, Kadam D, Sangle S, Gangodkar S, et al. Direct imaging of pH1N1 2009 influenza virus replication in alveolar pneumocytes in fatal cases by transmission electron microscopy. J Electron Microsc (Tokyo). (2011) 60(1):89–93. doi: 10.1093/jmicro/dfq081
60. Kataoka M, Ishida K, Ogasawara K, Nozaki T, Satoh YI, Sata T, et al. Serial section array scanning electron microscopy analysis of cells from lung autopsy specimens following fatal A/H1N1 2009 pandemic influenza virus infection. J Virol (2019) 93(19):e00644–19. doi: 10.1128/JVI.00644-19
61. Bastacky J, Lee CY, Goerke J, Koushafar H, Yager D, Kenaga L, et al. Alveolar lining layer is thin and continuous: low-temperature scanning electron microscopy of rat lung. J Appl Physiol (1985). (1995) 79(5):1615–28. doi: 10.1152/jappl.1995.79.5.1615
62. Boren HG. Alveolar fenestrae. Relationship to the pathology and pathogenesis of pulmonary emphysema. Am Rev Respir Dis (1962) 85:328–44. doi: 10.1164/arrd.1962.85.3.328
63. Gil J, Weibel ER. Morphological study of pressure-volume hysteresis in rat lungs fixed by vascular perfusion. Respir Physiol (1972) 15(2):190–213. doi: 10.1016/0034-5687(72)90098-9
64. Hook JL, Islam MN, Parker D, Prince AS, Bhattacharya S, Bhattacharya J. Disruption of staphylococcal aggregation protects against lethal lung injury. J Clin Invest. (2018) 128(3):1074–86. doi: 10.1172/JCI95823
65. Tang S, De Jesus AC, Chavez D, Suthakaran S, Moore SK, Suthakaran K, et al. Rescue of alveolar wall liquid secretion blocks fatal lung injury by influenza-staphylococcal coinfection. J Clin Invest. (2023) 133(19):e163402. doi: 10.1101/2022.07.07.499169
66. Mensah EA, Kumar NM, Nielsen L, Lwebuga-Mukasa JS. Distribution of alveolar type II cells in neonatal and adult rat lung revealed by RT-PCR in situ. Am J Physiol (1996) 271(1 Pt 1):L178–85. doi: 10.1152/ajplung.1996.271.1.L178
67. Perlman CE, Bhattacharya J. Alveolar expansion imaged by optical sectioning microscopy. J Appl Physiol (1985). (2007) 103(3):1037–44. doi: 10.1152/japplphysiol.00160.2007
68. Semmler-Behnke M, Kreyling WG, Schulz H, Takenaka S, Butler JP, Henry FS, et al. Nanoparticle delivery in infant lungs. Proc Natl Acad Sci USA (2012) 109(13):5092–7. doi: 10.1073/pnas.1119339109
69. Bhat N, Wright JG, Broder KR, Murray EL, Greenberg ME, Glover MJ, et al. Influenza-associated deaths among children in the United States, 2003-2004. N Engl J Med (2005) 353(24):2559–67. doi: 10.1056/NEJMoa051721
70. Sachedina N, Donaldson LJ. Paediatric mortality related to pandemic influenza A H1N1 infection in England: an observational population-based study. Lancet (2010) 376(9755):1846–52. doi: 10.1016/S0140-6736(10)61195-6
71. Peebles PJ, Dhara R, Brammer L, Fry AM, Finelli L. Influenza-associated mortality among children - United States: 2007-2008. Influenza Other Respir Viruses. (2011) 5(1):25–31. doi: 10.1111/j.1750-2659.2010.00166.x
72. Centers for Disease Control and Prevention. Influenza-associated pediatric deaths–United States, September 2010-August 2011. MMWR Morb Mortal Wkly Rep (2011) 60(36):1233–8.
73. Torres SF, Iolster T, Schnitzler EJ, Farias JA, Bordogna AC, Rufach D, et al. High mortality in patients with influenza A pH1N1 2009 admitted to a pediatric intensive care unit: a predictive model of mortality. Pediatr Crit Care Med (2012) 13(2):e78–83. doi: 10.1097/PCC.0b013e318219266b
74. Lee S, Islam MN, Boostanpour K, Aran D, Jin G, Christenson S, et al. Molecular programs of fibrotic change in aging human lung. Nat Commun (2021) 12(1):6309. doi: 10.1038/s41467-021-26603-2
75. van Riel D, Munster VJ, de Wit E, Rimmelzwaan GF, Fouchier RA, Osterhaus AD, et al. Human and avian influenza viruses target different cells in the lower respiratory tract of humans and other mammals. Am J Pathol (2007) 171(4):1215–23. doi: 10.2353/ajpath.2007.070248
76. Eriksson P, Lindskog C, Engholm E, Blixt O, Waldenstrom J, Munster V, et al. Characterization of avian influenza virus attachment patterns to human and pig tissues. Sci Rep (2018) 8(1):12215. doi: 10.1038/s41598-018-29578-1
77. Rogers GN, Paulson JC, Daniels RS, Skehel JJ, Wilson IA, Wiley DC. Single amino acid substitutions in influenza haemagglutinin change receptor binding specificity. Nature (1983) 304(5921):76–8. doi: 10.1038/304076a0
78. Xiong X, Martin SR, Haire LF, Wharton SA, Daniels RS, Bennett MS, et al. Receptor binding by an H7N9 influenza virus from humans. Nature (2013) 499(7459):496–9. doi: 10.1038/nature12372
79. Xiong X, Coombs PJ, Martin SR, Liu J, Xiao H, McCauley JW, et al. Receptor binding by a ferret-transmissible H5 avian influenza virus. Nature (2013) 497(7449):392–6. doi: 10.1038/nature12144
80. Ochs M, Hegermann J, Lopez-Rodriguez E, Timm S, Nouailles G, Matuszak J, et al. On top of the alveolar epithelium: surfactant and the glycocalyx. Int J Mol Sci (2020) 21(9):3075. doi: 10.3390/ijms21093075
81. Harris A, Cardone G, Winkler DC, Heymann JB, Brecher M, White JM, et al. Influenza virus pleiomorphy characterized by cryoelectron tomography. Proc Natl Acad Sci USA (2006) 103(50):19123–7. doi: 10.1073/pnas.0607614103
82. Liu Y, Childs RA, Matrosovich T, Wharton S, Palma AS, Chai W, et al. Altered receptor specificity and cell tropism of D222G hemagglutinin mutants isolated from fatal cases of pandemic A(H1N1) 2009 influenza virus. J Virol (2010) 84(22):12069–74. doi: 10.1128/JVI.01639-10
83. Kuiken T, Holmes EC, McCauley J, Rimmelzwaan GF, Williams CS, Grenfell BT. Host species barriers to influenza virus infections. Science (2006) 312(5772):394–7. doi: 10.1126/science.1122818
84. Flerlage T, Boyd DF, Meliopoulos V, Thomas PG, Schultz-Cherry S. Influenza virus and SARS-CoV-2: pathogenesis and host responses in the respiratory tract. Nat Rev Microbiol (2021) 19(7):425–41. doi: 10.1038/s41579-021-00542-7
85. Rogers GN, Paulson JC. Receptor determinants of human and animal influenza virus isolates: differences in receptor specificity of the H3 hemagglutinin based on species of origin. Virology (1983) 127(2):361–73. doi: 10.1016/0042-6822(83)90150-2
86. Rogers GN, D'Souza BL. Receptor binding properties of human and animal H1 influenza virus isolates. Virology (1989) 173(1):317–22. doi: 10.1016/0042-6822(89)90249-3
87. Shinya K, Ebina M, Yamada S, Ono M, Kasai N, Kawaoka Y. Avian flu: influenza virus receptors in the human airway. Nature (2006) 440(7083):435–6. doi: 10.1038/440435a
88. van Riel D, Munster VJ, de Wit E, Rimmelzwaan GF, Fouchier RA, Osterhaus AD, et al. H5N1 virus attachment to lower respiratory tract. Science (2006) 312(5772):399. doi: 10.1126/science.1125548
89. Oner AF, Bay A, Arslan S, Akdeniz H, Sahin HA, Cesur Y, et al. (H5N1) infection in eastern Turkey in 2006. N Engl J Med (2006) 355(21):2179–85. doi: 10.1056/NEJMoa060601
90. Gao HN, Lu HZ, Cao B, Du B, Shang H, Gan JH, et al. Clinical findings in 111 cases of influenza A (H7N9) virus infection. N Engl J Med (2013) 368(24):2277–85. doi: 10.1056/NEJMoa1305584
91. Li Q, Zhou L, Zhou M, Chen Z, Li F, Wu H, et al. Epidemiology of human infections with avian influenza A(H7N9) virus in China. N Engl J Med (2014) 370(6):520–32. doi: 10.1056/NEJMoa1304617
92. Xu T, Qiao J, Zhao L, Wang G, He G, Li K, et al. Acute respiratory distress syndrome induced by avian influenza A (H5N1) virus in mice. Am J Respir Crit Care Med (2006) 174(9):1011–7. doi: 10.1164/rccm.200511-1751OC
93. Ruan T, Sun Y, Zhang J, Sun J, Liu W, Prinz RA, et al. H5N1 infection impairs the alveolar epithelial barrier through intercellular junction proteins via Itch-mediated proteasomal degradation. Commun Biol (2022) 5(1):186. doi: 10.1038/s42003-022-03131-3
94. Ibricevic A, Pekosz A, Walter MJ, Newby C, Battaile JT, Brown EG, et al. Influenza virus receptor specificity and cell tropism in mouse and human airway epithelial cells. J Virol (2006) 80(15):7469–80. doi: 10.1128/JVI.02677-05
95. Nicholls JM, Chan MC, Chan WY, Wong HK, Cheung CY, Kwong DL, et al. Tropism of avian influenza A (H5N1) in the upper and lower respiratory tract. Nat Med (2007) 13(2):147–9. doi: 10.1038/nm1529
96. Davis AS, Chertow DS, Kindrachuk J, Qi L, Schwartzman LM, Suzich J, et al. 1918 Influenza receptor binding domain variants bind and replicate in primary human airway cells regardless of receptor specificity. Virology (2016) 493:238–46. doi: 10.1016/j.virol.2016.03.025
97. Liu M, Huang LZX, Smits AA, Bull C, Narimatsu Y, van Kuppeveld FJM, et al. Human-type sialic acid receptors contribute to avian influenza A virus binding and entry by hetero-multivalent interactions. Nat Commun (2022) 13(1):4054. doi: 10.1038/s41467-022-31840-0
98. Liu M, Bakker AS, Narimatsu Y, van Kuppeveld FJM, Clausen H, de Haan CAM, et al. H3N2 influenza A virus gradually adapts to human-type receptor binding and entry specificity after the start of the 1968 pandemic. Proc Natl Acad Sci USA (2023) 120(31):e2304992120. doi: 10.1073/pnas.2304992120
99. Yang W, Punyadarsaniya D, Lambertz RLO, Lee DCC, Liang CH, Hoper D, et al. Mutations during the adaptation of H9N2 avian influenza virus to the respiratory epithelium of pigs enhance sialic acid binding activity and virulence in mice. J Virol (2017) 91(8):e02125–16. doi: 10.1128/JVI.02125-16
100. Cushing A, Kamali A, Winters M, Hopmans ES, Bell JM, Grimes SM, et al. Emergence of hemagglutinin mutations during the course of influenza infection. Sci Rep (2015) 5:16178. doi: 10.1038/srep16178
101. Connor RJ, Kawaoka Y, Webster RG, Paulson JC. Receptor specificity in human, avian, and equine H2 and H3 influenza virus isolates. Virology (1994) 205(1):17–23. doi: 10.1006/viro.1994.1615
102. Tumpey TM, Maines TR, Van Hoeven N, Glaser L, Solorzano A, Pappas C, et al. A two-amino acid change in the hemagglutinin of the 1918 influenza virus abolishes transmission. Science (2007) 315(5812):655–9. doi: 10.1126/science.1136212
103. Rahman SK, Ansari MA, Gaur P, Ahmad I, Chakravarty C, Verma DK, et al. The immunomodulatory CEA cell adhesion molecule 6 (CEACAM6/CD66c) is a protein receptor for the influenza A virus. Viruses (2021) 13(5):726. doi: 10.3390/v13050726
104. Ng WC, Liong S, Tate MD, Irimura T, Denda-Nagai K, Brooks AG, et al. The macrophage galactose-type lectin can function as an attachment and entry receptor for influenza virus. J Virol (2014) 88(3):1659–72. doi: 10.1128/JVI.02014-13
105. Chapin C, Bailey NA, Gonzales LW, Lee JW, Gonzalez RF, Ballard PL. Distribution and surfactant association of carcinoembryonic cell adhesion molecule 6 in human lung. Am J Physiol Lung Cell Mol Physiol (2012) 302(2):L216–25. doi: 10.1152/ajplung.00055.2011
106. Jondle CN, Sharma A, Simonson TJ, Larson B, Mishra BB, Sharma J. Macrophage Galactose-Type Lectin-1 deficiency is associated with increased neutrophilia and hyperinflammation in gram-negative pneumonia. J Immunol (2016) 196(7):3088–96. doi: 10.4049/jimmunol.1501790
107. Sobotzki N, Schafroth MA, Rudnicka A, Koetemann A, Marty F, Goetze S, et al. HATRIC-based identification of receptors for orphan ligands. Nat Commun (2018) 9(1):1519. doi: 10.1038/s41467-018-03936-z
108. Langouet-Astrie C, Oshima K, McMurtry SA, Yang Y, Kwiecinski JM, LaRiviere WB, et al. The influenza-injured lung microenvironment promotes MRSA virulence, contributing to severe secondary bacterial pneumonia. Cell Rep (2022) 41(9):111721. doi: 10.1016/j.celrep.2022.111721
109. Timm S, Lettau M, Hegermann J, Rocha ML, Weidenfeld S, Fatykhova D, et al. The unremarkable alveolar epithelial glycocalyx: a thorium dioxide-based electron microscopic comparison after heparinase or pneumolysin treatment. Histochem Cell Biol (2023) 160(2):83–96. doi: 10.1007/s00418-023-02211-7
110. Rizzo AN, Haeger SM, Oshima K, Yang Y, Wallbank AM, Jin Y, et al. Alveolar epithelial glycocalyx degradation mediates surfactant dysfunction and contributes to acute respiratory distress syndrome. JCI Insight (2022) 7(2):e154573. doi: 10.1172/jci.insight.154573
111. Schurch S, Gehr P, Im Hof V, Geiser M, Green F. Surfactant displaces particles toward the epithelium in airways and alveoli. Respir Physiol (1990) 80(1):17–32. doi: 10.1016/0034-5687(90)90003-H
112. Benne CA, Benaissa-Trouw B, van Strijp JA, Kraaijeveld CA, van Iwaarden JF. Surfactant protein A, but not surfactant protein D, is an opsonin for influenza A virus phagocytosis by rat alveolar macrophages. Eur J Immunol (1997) 27(4):886–90. doi: 10.1002/eji.1830270413
113. LeVine AM, Whitsett JA, Hartshorn KL, Crouch EC, Korfhagen TR. Surfactant protein D enhances clearance of influenza A virus from the lung in vivo. J Immunol (2001) 167(10):5868–73. doi: 10.4049/jimmunol.167.10.5868
114. LeVine AM, Hartshorn K, Elliott J, Whitsett J, Korfhagen T. Absence of SP-A modulates innate and adaptive defense responses to pulmonary influenza infection. Am J Physiol Lung Cell Mol Physiol (2002) 282(3):L563–72. doi: 10.1152/ajplung.00280.2001
115. Hartshorn KL, White MR, Voelker DR, Coburn J, Zaner K, Crouch EC. Mechanism of binding of surfactant protein D to influenza A viruses: importance of binding to haemagglutinin to antiviral activity. Biochem J (2000) 351 Pt 2(Pt 2):449–58. doi: 10.1042/bj3510449
116. van Eijk M, Hillaire MLB, Rimmelzwaan GF, Rynkiewicz MJ, White MR, Hartshorn KL, et al. Enhanced antiviral activity of human surfactant protein D by site-specific engineering of the carbohydrate recognition domain. Front Immunol (2019) 10:2476. doi: 10.3389/fimmu.2019.02476
117. Lindert J, Perlman CE, Parthasarathi K, Bhattacharya J. Chloride-dependent secretion of alveolar wall liquid determined by optical-sectioning microscopy. Am J Respir Cell Mol Biol (2007) 36(6):688–96. doi: 10.1165/rcmb.2006-0347OC
118. Stray SJ, Cummings RD, Air GM. Influenza virus infection of desialylated cells. Glycobiology (2000) 10(7):649–58. doi: 10.1093/glycob/10.7.649
119. Chu VC, Whittaker GR. Influenza virus entry and infection require host cell N-linked glycoprotein. Proc Natl Acad Sci USA (2004) 101(52):18153–8. doi: 10.1073/pnas.0405172102
120. Rust MJ, Lakadamyali M, Zhang F, Zhuang X. Assembly of endocytic machinery around individual influenza viruses during viral entry. Nat Struct Mol Biol (2004) 11(6):567–73. doi: 10.1038/nsmb769
121. Chen C, Zhuang X. Epsin 1 is a cargo-specific adaptor for the clathrin-mediated endocytosis of the influenza virus. Proc Natl Acad Sci USA (2008) 105(33):11790–5. doi: 10.1073/pnas.0803711105
122. Wang H, Jiang C. Influenza A virus H5N1 entry into host cells is through clathrin-dependent endocytosis. Sci China C Life Sci (2009) 52(5):464–9. doi: 10.1007/s11427-009-0061-0
123. Zhang Y, Whittaker GR. Influenza entry pathways in polarized MDCK cells. Biochem Biophys Res Commun (2014) 450(1):234–9. doi: 10.1016/j.bbrc.2014.05.095
124. Wills RC, Hammond GRV. PI(4,5)P2: signaling the plasma membrane. Biochem J (2022) 479(21):2311–25. doi: 10.1042/BCJ20220445
125. Mettlen M, Chen PH, Srinivasan S, Danuser G, Schmid SL. Regulation of clathrin-mediated endocytosis. Annu Rev Biochem (2018) 87:871–96. doi: 10.1146/annurev-biochem-062917-012644
126. Eierhoff T, Hrincius ER, Rescher U, Ludwig S, Ehrhardt C. The epidermal growth factor receptor (EGFR) promotes uptake of influenza A viruses (IAV) into host cells. PLoS Pathog (2010) 6(9):e1001099. doi: 10.1371/journal.ppat.1001099
127. Wang G, Jiang L, Wang J, Zhang J, Kong F, Li Q, et al. The G protein-coupled receptor FFAR2 promotes internalization during influenza A virus entry. J Virol (2020) 94(2):e01707. doi: 10.1128/JVI.01707-19
128. Mazel-Sanchez B, Niu C, Williams N, Bachmann M, Choltus H, Silva F, et al. Influenza A virus exploits transferrin receptor recycling to enter host cells. Proc Natl Acad Sci USA (2023) 120(21):e2214936120. doi: 10.1073/pnas.2214936120
129. Lieber M, Smith B, Szakal A, Nelson-Rees W, Todaro G. A continuous tumor-cell line from a human lung carcinoma with properties of type II alveolar epithelial cells. Int J Cancer. (1976) 17(1):62–70. doi: 10.1002/ijc.2910170110
130. Foster KA, Oster CG, Mayer MM, Avery ML, Audus KL, et al. Characterization of the A549 cell line as a type II pulmonary epithelial cell model for drug metabolism. Exp Cell Res (1998) 243(2):359–66. doi: 10.1006/excr.1998.4172
131. The LungMAP Consortium. The LungMAP Database. Available at: https://lungmap.net/ (Accessed July 31, 2023).
132. Nunes-Correia I, Eulalio A, Nir S, Pedroso de Lima MC. Caveolae as an additional route for influenza virus endocytosis in MDCK cells. Cell Mol Biol Lett (2004) 9(1):47–60.
133. de Vries E, Tscherne DM, Wienholts MJ, Cobos-Jimenez V, Scholte F, Garcia-Sastre A, et al. Dissection of the influenza A virus endocytic routes reveals macropinocytosis as an alternative entry pathway. PLoS Pathog (2011) 7(3):e1001329. doi: 10.1371/journal.ppat.1001329
134. Rossman JS, Leser GP, Lamb RA. Filamentous influenza virus enters cells via macropinocytosis. J Virol (2012) 86(20):10950–60. doi: 10.1128/JVI.05992-11
135. Hunziker A, Glas I, Pohl MO, Stertz S. Phosphoproteomic profiling of influenza virus entry reveals infection-triggered filopodia induction counteracted by dynamic cortactin phosphorylation. Cell Rep (2022) 38(4):110306. doi: 10.1016/j.celrep.2022.110306
136. Zaas DW, Duncan MJ, Li G, Wright JR, Abraham SN. Pseudomonas invasion of type I pneumocytes is dependent on the expression and phosphorylation of caveolin-2. J Biol Chem (2005) 280(6):4864–72. doi: 10.1074/jbc.M411702200
137. Thorley AJ, Ruenraroengsak P, Potter TE, Tetley TD. Critical determinants of uptake and translocation of nanoparticles by the human pulmonary alveolar epithelium. ACS Nano. (2014) 8(11):11778–89. doi: 10.1021/nn505399e
138. Detampel P, Tehranian S, Mukherjee P, Foret M, Fuerstenhaupt T, Darbandi A, et al. Caveolin-initiated macropinocytosis is required for efficient silica nanoparticles' transcytosis across the alveolar epithelial barrier. Sci Rep (2022) 12(1):9474. doi: 10.1038/s41598-022-13388-7
139. Newman GR, Campbell L, von Ruhland C, Jasani B, Gumbleton M. Caveolin and its cellular and subcellular immunolocalisation in lung alveolar epithelium: implications for alveolar epithelial type I cell function. Cell Tissue Res (1999) 295(1):111–20. doi: 10.1007/s004410051217
140. Mayor S, Pagano RE. Pathways of clathrin-independent endocytosis. Nat Rev Mol Cell Biol (2007) 8(8):603–12. doi: 10.1038/nrm2216
141. Doherty GJ, McMahon HT. Mechanisms of endocytosis. Annu Rev Biochem (2009) 78:857–902. doi: 10.1146/annurev.biochem.78.081307.110540
142. Mayor S, Parton RG, Donaldson JG. Clathrin-independent pathways of endocytosis. Cold Spring Harb Perspect Biol (2014) 6(6):a016758. doi: 10.1101/cshperspect.a016758
143. Thottacherry JJ, Sathe M, Prabhakara C, Mayor S. Spoiled for choice: diverse endocytic pathways function at the cell surface. Annu Rev Cell Dev Biol (2019) 35:55–84. doi: 10.1146/annurev-cellbio-100617-062710
144. Parton RG. Caveolae: structure, function, and relationship to disease. Annu Rev Cell Dev Biol (2018) 34:111–36. doi: 10.1146/annurev-cellbio-100617-062737
145. Parton RG, Del Pozo MA, Vassilopoulos S, Nabi IR, Le Lay S, Lundmark R, et al. Caveolae: the FAQs. Traffic (2020) 21(1):181–5. doi: 10.1111/tra.12689
146. Rennick JJ, Johnston APR, Parton RG. Key principles and methods for studying the endocytosis of biological and nanoparticle therapeutics. Nat Nanotechnol. (2021) 16(3):266–76. doi: 10.1038/s41565-021-00858-8
147. Ramirez MI, Pollack L, Millien G, Cao YX, Hinds A, Williams MC. The alpha-isoform of caveolin-1 is a marker of vasculogenesis in early lung development. J Histochem Cytochem (2002) 50(1):33–42. doi: 10.1177/002215540205000104
148. Kogo H, Aiba T, Fujimoto T. Cell type-specific occurrence of caveolin-1alpha and -1beta in the lung caused by expression of distinct mRNAs. J Biol Chem (2004) 279(24):25574–81. doi: 10.1074/jbc.M310807200
149. Fuchs S, Hollins AJ, Laue M, Schaefer UF, Roemer K, Gumbleton M, et al. Differentiation of human alveolar epithelial cells in primary culture: morphological characterization and synthesis of caveolin-1 and surfactant protein-C. Cell Tissue Res (2003) 311(1):31–45. doi: 10.1007/s00441-002-0653-5
150. Dahlin K, Mager EM, Allen L, Tigue Z, Goodglick L, Wadehra M, et al. Identification of genes differentially expressed in rat alveolar type I cells. Am J Respir Cell Mol Biol (2004) 31(3):309–16. doi: 10.1165/rcmb.2003-0423OC
151. Campbell L, Hollins AJ, Al-Eid A, Newman GR, von Ruhland C, Gumbleton M. Caveolin-1 expression and caveolae biogenesis during cell transdifferentiation in lung alveolar epithelial primary cultures. Biochem Biophys Res Commun (1999) 262(3):744–51. doi: 10.1006/bbrc.1999.1280
152. John TA, Vogel SM, Minshall RD, Ridge K, Tiruppathi C, Malik AB. Evidence for the role of alveolar epithelial gp60 in active transalveolar albumin transport in the rat lung. J Physiol (2001) 533(Pt 2):547–59. doi: 10.1111/j.1469-7793.2001.0547a.x
153. Gil J, Silage DA, McNiff JM. Distribution of vesicles in cells of air-blood barrier in the rabbit. J Appl Physiol Respir Environ Exerc Physiol (1981) 50(2):334–40. doi: 10.1152/jappl.1981.50.2.334
154. Crapo JD, Barry BE, Gehr P, Bachofen M, Weibel ER. Cell number and cell characteristics of the normal human lung. Am Rev Respir Dis (1982) 126(2):332–7. doi: 10.1164/arrd.1982.126.2.332
155. DeFouw DO. Vesicle numerical densities and cellular attenuation: comparisons between endothelium and epithelium of the alveolar septa in normal dog lungs. Anat Rec. (1984) 209(1):77–84. doi: 10.1002/ar.1092090110
156. Sakai T, Nishimura SI, Naito T, Saito M. Influenza A virus hemagglutinin and neuraminidase act as novel motile machinery. Sci Rep (2017) 7:45043. doi: 10.1038/srep45043
157. Guo H, Rabouw H, Slomp A, Dai M, van der Vegt F, van Lent JWM, et al. Kinetic analysis of the influenza A virus HA/NA balance reveals contribution of NA to virus-receptor binding and NA-dependent rolling on receptor-containing surfaces. PLoS Pathog (2018) 14(8):e1007233. doi: 10.1371/journal.ppat.1007233
158. Vahey MD, Fletcher DA. Influenza A virus surface proteins are organized to help penetrate host mucus. Elife (2019) 8:e43764. doi: 10.7554/eLife.43764
159. Verma DK, Gupta D, Lal SK. Host lipid rafts play a major role in binding and endocytosis of influenza A virus. Viruses (2018) 10(11):650. doi: 10.3390/v10110650
160. Wolk KE, Lazarowski ER, Traylor ZP, Yu EN, Jewell NA, Durbin RK, et al. Influenza A virus inhibits alveolar fluid clearance in BALB/c mice. Am J Respir Crit Care Med (2008) 178(9):969–76. doi: 10.1164/rccm.200803-455OC
161. Herold S, Steinmueller M, von Wulffen W, Cakarova L, Pinto R, Pleschka S, et al. Lung epithelial apoptosis in influenza virus pneumonia: the role of macrophage-expressed TNF-related apoptosis-inducing ligand. J Exp Med (2008) 205(13):3065–77. doi: 10.1084/jem.20080201
162. Wang S, Le TQ, Kurihara N, Chida J, Cisse Y, Yano M, et al. Influenza virus-cytokine-protease cycle in the pathogenesis of vascular hyperpermeability in severe influenza. J Infect Dis (2010) 202(7):991–1001. doi: 10.1086/656044
163. Armstrong SM, Wang C, Tigdi J, Si X, Dumpit C, Charles S, et al. Influenza infects lung microvascular endothelium leading to microvascular leak: role of apoptosis and claudin-5. PLoS One (2012) 7(10):e47323. doi: 10.1371/journal.pone.0047323
164. Hogner K, Wolff T, Pleschka S, Plog S, Gruber AD, Kalinke U, et al. Macrophage-expressed IFN-beta contributes to apoptotic alveolar epithelial cell injury in severe influenza virus pneumonia. PLoS Pathog (2013) 9(2):e1003188. doi: 10.1371/journal.ppat.1003188
165. Gotts JE, Abbott J, Matthay MA. Influenza causes prolonged disruption of the alveolar-capillary barrier in mice unresponsive to mesenchymal stem cell therapy. Am J Physiol Lung Cell Mol Physiol (2014) 307(5):L395–406. doi: 10.1152/ajplung.00110.2014
166. Wan X, Li J, Wang Y, Yu X, He X, Shi J, et al. H7N9 virus infection triggers lethal cytokine storm by activating gasdermin E-mediated pyroptosis of lung alveolar epithelial cells. Natl Sci Rev (2021) 9(1):nwab137. doi: 10.1093/nsr/nwab137
168. Chiba H, Osanai M, Murata M, Kojima T, Sawada N. Transmembrane proteins of tight junctions. Biochim Biophys Acta (2008) 1778(3):588–600. doi: 10.1016/j.bbamem.2007.08.017
169. Gorin AB, Stewart PA. Differential permeability of endothelial and epithelial barriers to albumin flux. J Appl Physiol Respir Environ Exerc Physiol (1979) 47(6):1315–24. doi: 10.1152/jappl.1979.47.6.1315
170. Wiener-Kronish JP, Albertine KH, Matthay MA. Differential responses of the endothelial and epithelial barriers of the lung in sheep to Escherichia coli endotoxin. J Clin Invest. (1991) 88(3):864–75. doi: 10.1172/JCI115388
171. Schneeberger-Keeley EE, Karnovsky MJ. The ultrastructural basis of alveolar-capillary membrane permeability to peroxidase used as a tracer. J Cell Biol (1968) 37(3):781–93. doi: 10.1083/jcb.37.3.781
172. Schneeberger EE, Karnovsky MJ. Substructure of intercellular junctions in freeze-fractured alveolar-capillary membranes of mouse lung. Circ Res (1976) 38(5):404–11. doi: 10.1161/01.RES.38.5.404
173. Crapo JD. New concepts in the formation of pulmonary edema. Am Rev Respir Dis (1993) 147(4):790–2. doi: 10.1164/ajrccm/147.4.790
174. Ruan T, Sun J, Liu W, Prinz RA, Peng D, Liu X, et al. H1N1 influenza virus cross-activates Gli1 to disrupt the intercellular junctions of alveolar epithelial cells. Cell Rep (2020) 31(13):107801. doi: 10.1016/j.celrep.2020.107801
175. Short KR, Kasper J, van der Aa S, Andeweg AC, Zaaraoui-Boutahar F, Goeijenbier M, et al. Influenza virus damages the alveolar barrier by disrupting epithelial cell tight junctions. Eur Respir J (2016) 47(3):954–66. doi: 10.1183/13993003.01282-2015
176. Ronni T, Matikainen S, Sareneva T, Melen K, Pirhonen J, Keskinen P, et al. Regulation of IFN-alpha/beta, MxA, 2',5'-oligoadenylate synthetase, and HLA gene expression in influenza A-infected human lung epithelial cells. J Immunol (1997) 158(5):2363–74. doi: 10.4049/jimmunol.158.5.2363
177. Chan MC, Cheung CY, Chui WH, Tsao SW, Nicholls JM, Chan YO, et al. Proinflammatory cytokine responses induced by influenza A (H5N1) viruses in primary human alveolar and bronchial epithelial cells. Respir Res (2005) 6(1):135. doi: 10.1186/1465-9921-6-135
178. Guillot L, Le Goffic R, Bloch S, Escriou N, Akira S, Chignard M, et al. Involvement of toll-like receptor 3 in the immune response of lung epithelial cells to double-stranded RNA and influenza A virus. J Biol Chem (2005) 280(7):5571–80. doi: 10.1074/jbc.M410592200
179. Bernasconi D, Amici C, La Frazia S, Ianaro A, Santoro MG. The IkappaB kinase is a key factor in triggering influenza A virus-induced inflammatory cytokine production in airway epithelial cells. J Biol Chem (2005) 280(25):24127–34. doi: 10.1074/jbc.M413726200
180. Herold S, von Wulffen W, Steinmueller M, Pleschka S, Kuziel WA, Mack M, et al. Alveolar epithelial cells direct monocyte transepithelial migration upon influenza virus infection: impact of chemokines and adhesion molecules. J Immunol (2006) 177(3):1817–24. doi: 10.4049/jimmunol.177.3.1817
181. Veckman V, Osterlund P, Fagerlund R, Melen K, Matikainen S, Julkunen I. TNF-alpha and IFN-alpha enhance influenza-A-virus-induced chemokine gene expression in human A549 lung epithelial cells. Virology (2006) 345(1):96–104. doi: 10.1016/j.virol.2005.09.043
182. Wang J, Nikrad MP, Phang T, Gao B, Alford T, Ito Y, et al. Innate immune response to influenza A virus in differentiated human alveolar type II cells. Am J Respir Cell Mol Biol (2011) 45(3):582–91. doi: 10.1165/rcmb.2010-0108OC
183. Yu WC, Chan RW, Wang J, Travanty EA, Nicholls JM, Peiris JS, et al. Viral replication and innate host responses in primary human alveolar epithelial cells and alveolar macrophages infected with influenza H5N1 and H1N1 viruses. J Virol (2011) 85(14):6844–55. doi: 10.1128/JVI.02200-10
184. Ito Y, Correll K, Zemans RL, Leslie CC, Murphy RC, Mason RJ. Influenza induces IL-8 and GM-CSF secretion by human alveolar epithelial cells through HGF/c-Met and TGF-alpha/EGFR signaling. Am J Physiol Lung Cell Mol Physiol (2015) 308(11):L1178–88. doi: 10.1152/ajplung.00290.2014
185. Lin KL, Suzuki Y, Nakano H, Ramsburg E, Gunn MD. CCR2+ monocyte-derived dendritic cells and exudate macrophages produce influenza-induced pulmonary immune pathology and mortality. J Immunol (2008) 180(4):2562–72. doi: 10.4049/jimmunol.180.4.2562
186. Walsh KB, Teijaro JR, Wilker PR, Jatzek A, Fremgen DM, Das SC, et al. Suppression of cytokine storm with a sphingosine analog provides protection against pathogenic influenza virus. Proc Natl Acad Sci USA (2011) 108(29):12018–23. doi: 10.1073/pnas.1107024108
187. Narasaraju T, Yang E, Samy RP, Ng HH, Poh WP, Liew AA, et al. Excessive neutrophils and neutrophil extracellular traps contribute to acute lung injury of influenza pneumonitis. Am J Pathol (2011) 179(1):199–210. doi: 10.1016/j.ajpath.2011.03.013
188. Aoyagi T, Newstead MW, Zeng X, Kunkel SL, Kaku M, Standiford TJ. IL-36 receptor deletion attenuates lung injury and decreases mortality in murine influenza pneumonia. Mucosal Immunol (2017) 10(4):1043–55. doi: 10.1038/mi.2016.107
189. Mori I, Komatsu T, Takeuchi K, Nakakuki K, Sudo M, Kimura Y. In vivo induction of apoptosis by influenza virus. J Gen Virol (1995) 76(Pt 11):2869–73. doi: 10.1099/0022-1317-76-11-2869
190. Uiprasertkul M, Kitphati R, Puthavathana P, Kriwong R, Kongchanagul A, Ungchusak K, et al. Apoptosis and pathogenesis of avian influenza A (H5N1) virus in humans. Emerg Infect Dis (2007) 13(5):708–12. doi: 10.3201/eid1305.060572
191. Mauad T, Hajjar LA, Callegari GD, da Silva LF, Schout D, Galas FR, et al. Lung pathology in fatal novel human influenza A (H1N1) infection. Am J Respir Crit Care Med (2010) 181(1):72–9. doi: 10.1164/rccm.200909-1420OC
192. Daidoji T, Koma T, Du A, Yang CS, Ueda M, Ikuta K, et al. H5N1 avian influenza virus induces apoptotic cell death in mammalian airway epithelial cells. J Virol (2008) 82(22):11294–307. doi: 10.1128/JVI.01192-08
193. Kosmider B, Messier EM, Janssen WJ, Nahreini P, Wang J, Hartshorn KL, et al. Nrf2 protects human alveolar epithelial cells against injury induced by influenza A virus. Respir Res (2012) 13:43. doi: 10.1186/1465-9921-13-43
194. Chen XJ, Seth S, Yue G, Kamat P, Compans RW, Guidot D, et al. Influenza virus inhibits ENaC and lung fluid clearance. Am J Physiol Lung Cell Mol Physiol (2004) 287(2):L366–73. doi: 10.1152/ajplung.00011.2004
195. Lazrak A, Iles KE, Liu G, Noah DL, Noah JW, Matalon S. Influenza virus M2 protein inhibits epithelial sodium channels by increasing reactive oxygen species. FASEB J (2009) 23(11):3829–42. doi: 10.1096/fj.09-135590
196. Peteranderl C, Morales-Nebreda L, Selvakumar B, Lecuona E, Vadasz I, Morty RE, et al. Macrophage-epithelial paracrine crosstalk inhibits lung edema clearance during influenza infection. J Clin Invest. (2016) 126(4):1566–80. doi: 10.1172/JCI83931
197. Chan MC, Kuok DI, Leung CY, Hui KP, Valkenburg SA, Lau EH, et al. Human mesenchymal stromal cells reduce influenza A H5N1-associated acute lung injury in vitro and in vivo. Proc Natl Acad Sci USA (2016) 113(13):3621–6. doi: 10.1073/pnas.1601911113
198. Brand JD, Lazrak A, Trombley JE, Shei RJ, Adewale AT, Tipper JL, et al. Influenza-mediated reduction of lung epithelial ion channel activity leads to dysregulated pulmonary fluid homeostasis. JCI Insight (2018) 3(20):e123467. doi: 10.1172/jci.insight.123467
199. Weinheimer VK, Becher A, Tonnies M, Holland G, Knepper J, Bauer TT, et al. Influenza A viruses target type II pneumocytes in the human lung. J Infect Dis (2012) 206(11):1685–94. doi: 10.1093/infdis/jis455
200. Koerner I, Matrosovich MN, Haller O, Staeheli P, Kochs G. Altered receptor specificity and fusion activity of the haemagglutinin contribute to high virulence of a mouse-adapted influenza A virus. J Gen Virol (2012) 93(Pt 5):970–9. doi: 10.1099/vir.0.035782-0
201. Pattle RE. Properties, function and origin of the alveolar lining layer. Nature (1955) 175(4469):1125–6. doi: 10.1038/1751125b0
202. Clements JA. Pulmonary edema and permeability of alveolar membranes. Arch Environ Health (1961) 2:280–3. doi: 10.1080/00039896.1961.10662852
203. Nieman GF, Bredenberg CE. High surface tension pulmonary edema induced by detergent aerosol. J Appl Physiol (1985). (1985) 58(1):129–36. doi: 10.1152/jappl.1985.58.1.129
204. Islam MN, Gusarova GA, Das SR, Li L, Monma E, Anjaneyulu M, et al. The mitochondrial calcium uniporter of pulmonary type 2 cells determines severity of acute lung injury. Nat Commun (2022) 13(1):5837. doi: 10.1038/s41467-022-33543-y
205. Salomon R, Franks J, Govorkova EA, Ilyushina NA, Yen HL, Hulse-Post DJ, et al. The polymerase complex genes contribute to the high virulence of the human H5N1 influenza virus isolate A/Vietnam/1203/04. J Exp Med (2006) 203(3):689–97. doi: 10.1084/jem.20051938
206. Soszynska-Jozwiak M, Pszczola M, Piasecka J, Peterson JM, Moss WN, Taras-Goslinska K, et al. Universal and strain specific structure features of segment 8 genomic RNA of influenza A virus-application of 4-thiouridine photocrosslinking. J Biol Chem (2021) 297(6):101245. doi: 10.1016/j.jbc.2021.101245
207. Loveday EK, Svinti V, Diederich S, Pasick J, Jean F. Temporal- and strain-specific host microRNA molecular signatures associated with swine-origin H1N1 and avian-origin H7N7 influenza A virus infection. J Virol (2012) 86(11):6109–22. doi: 10.1128/JVI.06892-11
208. Ocana-Macchi M, Ricklin ME, Python S, Monika GA, Stech J, Stech O. Avian influenza A virus PB2 promotes interferon type I inducing properties of a swine strain in porcine dendritic cells. Virology (2012) 427(1):1–9. doi: 10.1016/j.virol.2012.01.037
209. Gnirss K, Zmora P, Blazejewska P, Winkler M, Lins A, Nehlmeier I, et al. Tetherin sensitivity of influenza A viruses is strain specific: role of hemagglutinin and neuraminidase. J Virol (2015) 89(18):9178–88. doi: 10.1128/JVI.00615-15
210. Narkpuk J, Jongkaewwattana A, Teeravechyan S. The avian influenza virus PA segment mediates strain-specific antagonism of BST-2/tetherin. Virology (2018) 525:161–9. doi: 10.1016/j.virol.2018.09.016
211. Mostafa A, Mahmoud SH, Shehata M, Muller C, Kandeil A, El-Shesheny R, et al. PA from a recent H9N2 (G1-like) avian influenza A virus (AIV) strain carrying lysine 367 confers altered replication efficiency and pathogenicity to contemporaneous H5N1 in mammalian systems. Viruses (2020) 12(9):1046. doi: 10.3390/v12091046
212. Reed C, Chaves SS, Perez A, D'Mello T, Daily Kirley P, Aragon D, et al. Complications among adults hospitalized with influenza: a comparison of seasonal influenza and the 2009 H1N1 pandemic. Clin Infect Dis (2014) 59(2):166–74. doi: 10.1093/cid/ciu285
213. Wu ZQ, Zhang Y, Zhao N, Yu Z, Pan H, Chan TC, et al. Comparative epidemiology of human fatal infections with novel, high (H5N6 and H5N1) and low (H7N9 and H9N2) pathogenicity avian influenza A viruses. Int J Environ Res Public Health (2017) 14(3):263. doi: 10.3390/ijerph14030263
214. Felten M, Ferencik S, Teixeira Alves LG, Letsiou E, Lienau J, Muller-Redetzky HC, et al. Ventilator-induced lung injury is modulated by the circadian clock. Am J Respir Crit Care Med (2023) 207(11):1464–74. doi: 10.1164/rccm.202202-0320OC
215. Sundar IK, Ahmad T, Yao H, Hwang JW, Gerloff J, Lawrence BP, et al. Influenza A virus-dependent remodeling of pulmonary clock function in a mouse model of COPD. Sci Rep (2015) 4:9927. doi: 10.1038/srep09927
216. Sengupta S, Tang SY, Devine JC, Anderson ST, Nayak S, Zhang SL, et al. Circadian control of lung inflammation in influenza infection. Nat Commun (2019) 10(1):4107. doi: 10.1038/s41467-019-11400-9
217. Naik A, Forrest KM, Paul O, Issah Y, Valekunja UK, Tang SY, et al. Circadian regulation of lung repair and regeneration. JCI Insight (2023) 8(16):e164720. doi: 10.1172/jci.insight.164720
218. Knauert MP, Ayas NT, Bosma KJ, Drouot X, Heavner MS, Owens RL, et al. Causes, consequences, and treatments of sleep and circadian disruption in the ICU: an official American Thoracic Society Research Statement. Am J Respir Crit Care Med (2023) 207(7):e49–68. doi: 10.1164/rccm.202301-0184ST
219. Zampieri FG, Wilcox ME. Understanding sleep and circadian disruption in the intensive care unit. Ann Am Thorac Soc (2023) 20(11):1558–60. doi: 10.1513/AnnalsATS.202308-699ED
220. Sanches Santos Rizzo Zuttion M, Moore SKL, Chen P, Beppu AK, Hook JL. New insights into the alveolar epithelium as a driver of acute respiratory distress syndrome. Biomolecules (2022) 12(9):1273. doi: 10.3390/biom12091273
221. To KF, Chan PK, Chan KF, Lee WK, Lam WY, Wong KF, et al. Pathology of fatal human infection associated with avian influenza A H5N1 virus. J Med Virol (2001) 63(3):242–6. doi: 10.1002/1096-9071(200103)63:3<242::AID-JMV1007>3.0.CO;2-N
222. Chan PK. Outbreak of avian influenza A(H5N1) virus infection in Hong Kong in 1997. Clin Infect Dis (2002) 34 Suppl 2:S58–64. doi: 10.1086/338820
223. Uiprasertkul M, Puthavathana P, Sangsiriwut K, Pooruk P, Srisook K, Peiris M, et al. Influenza A H5N1 replication sites in humans. Emerg Infect Dis (2005) 11(7):1036–41. doi: 10.3201/eid1107.041313
224. Gill JR, Sheng ZM, Ely SF, Guinee DG, Beasley MB, Suh J, et al. Pulmonary pathologic findings of fatal 2009 pandemic influenza A/H1N1 viral infections. Arch Pathol Lab Med (2010) 134(2):235–43. doi: 10.5858/134.2.235
225. Ramos I, Smith G, Ruf-Zamojski F, Martinez-Romero C, Fribourg M, Carbajal EA, et al. Innate immune response to influenza virus at single-cell resolution in human epithelial cells revealed paracrine induction of interferon lambda 1. J Virol (2019) 93(20):e00559. doi: 10.1128/JVI.00559-19
226. Stifter SA, Bhattacharyya N, Sawyer AJ, Cootes TA, Stambas J, Doyle SE, et al. Visualizing the selectivity and dynamics of interferon signaling in vivo. Cell Rep (2019) 29(11):3539–50 e4. doi: 10.1016/j.celrep.2019.11.021
227. Bruewer M, Luegering A, Kucharzik T, Parkos CA, Madara JL, Hopkins AM, et al. Proinflammatory cytokines disrupt epithelial barrier function by apoptosis-independent mechanisms. J Immunol (2003) 171(11):6164–72. doi: 10.4049/jimmunol.171.11.6164
228. Utech M, Ivanov AI, Samarin SN, Bruewer M, Turner JR, Mrsny RJ, et al. Mechanism of IFN-gamma-induced endocytosis of tight junction proteins: myosin II-dependent vacuolarization of the apical plasma membrane. Mol Biol Cell (2005) 16(10):5040–52. doi: 10.1091/mbc.e05-03-0193
229. Ciancanelli MJ, Huang SX, Luthra P, Garner H, Itan Y, Volpi S, et al. Infectious Disease. Life-threatening influenza and impaired interferon amplification in human IRF7 deficiency. Science (2015) 348(6233):448–53. doi: 10.1126/science.aaa1578
230. Hernandez N, Melki I, Jing H, Habib T, Huang SSY, Danielson J, et al. Life-threatening influenza pneumonitis in a child with inherited IRF9 deficiency. J Exp Med (2018) 215(10):2567–85. doi: 10.1084/jem.20180628
231. Lim HK, Huang SXL, Chen J, Kerner G, Gilliaux O, Bastard P, et al. Severe influenza pneumonitis in children with inherited TLR3 deficiency. J Exp Med (2019) 216(9):2038–56. doi: 10.1084/jem.20181621
232. Westphalen K, Gusarova GA, Islam MN, Subramanian M, Cohen TS, Prince AS, et al. Sessile alveolar macrophages communicate with alveolar epithelium to modulate immunity. Nature (2014) 506(7489):503–6. doi: 10.1038/nature12902
233. Ichimura H, Parthasarathi K, Lindert J, Bhattacharya J. Lung surfactant secretion by interalveolar Ca2+ signaling. Am J Physiol Lung Cell Mol Physiol (2006) 291(4):L596–601. doi: 10.1152/ajplung.00036.2006
234. Fujioka Y, Tsuda M, Nanbo A, Hattori T, Sasaki J, Sasaki T, et al. A Ca(2+)-dependent signalling circuit regulates influenza A virus internalization and infection. Nat Commun (2013) 4:2763. doi: 10.1038/ncomms3763
Keywords: endocytosis, influenza A virus, blood-air barrier, pulmonary edema, acute lung injury
Citation: Hook JL and Bhattacharya J (2024) The pathogenesis of influenza in intact alveoli: virion endocytosis and its effects on the lung’s air-blood barrier. Front. Immunol. 15:1328453. doi: 10.3389/fimmu.2024.1328453
Received: 26 October 2023; Accepted: 03 January 2024;
Published: 26 January 2024.
Edited by:
István Vadász, Universities of Giessen and Marburg Lung Center, GermanyReviewed by:
Fariba Rezaee, Cleveland Clinic, United StatesMatthias Felten, Charité University Medicine Berlin, Germany
Copyright © 2024 Hook and Bhattacharya. This is an open-access article distributed under the terms of the Creative Commons Attribution License (CC BY). The use, distribution or reproduction in other forums is permitted, provided the original author(s) and the copyright owner(s) are credited and that the original publication in this journal is cited, in accordance with accepted academic practice. No use, distribution or reproduction is permitted which does not comply with these terms.
*Correspondence: Jaime L. Hook, jaime.hook@mssm.edu