- Department of Medicine, Section of General Pathology, University of Verona, Verona, Italy
CD8+ lymphocytes are adaptive immunity cells with the particular function to directly kill the target cell following antigen recognition in the context of MHC class I. In addition, CD8+ T cells may release pro-inflammatory cytokines, such as tumor necrosis factor-α (TNF-α) and interferon-γ (IFN-γ), and a plethora of other cytokines and chemoattractants modulating immune and inflammatory responses. A role for CD8+ T cells has been suggested in aging and several diseases of the central nervous system (CNS), including Alzheimer’s disease, Parkinson’s disease, multiple sclerosis, amyotrophic lateral sclerosis, limbic encephalitis-induced temporal lobe epilepsy and Susac syndrome. Here we discuss the phenotypic and functional alterations of CD8+ T cell compartment during these conditions, highlighting similarities and differences between CNS disorders. Particularly, we describe the pathological changes in CD8+ T cell memory phenotypes emphasizing the role of senescence and exhaustion in promoting neuroinflammation and neurodegeneration. We also discuss the relevance of trafficking molecules such as selectins, mucins and integrins controlling the extravasation of CD8+ T cells into the CNS and promoting disease development. Finally, we discuss how CD8+ T cells may induce CNS tissue damage leading to neurodegeneration and suggest that targeting detrimental CD8+ T cells functions may have therapeutic effect in CNS disorders.
Introduction
The central nervous system (CNS) has been previously viewed as an immune-privileged site inaccessible to peripheral immune cells during normal, steady-state conditions (1). The role of neuroinflammation in neurodegenerative disorders, such as Alzheimer’s disease (AD) and Parkinson’s disease (PD), has been disregarded for a long period of time. However, current research has completely redefined the concept of CNS immunity, shifting it from the belief that the brain is an isolated organ, impervious to peripheral immune cells, to the recognition of the key role for immune mechanisms and neuroimmune interactions during physiological and pathological conditions (2–7). Whereas the role of innate immunity, especially microglia, in neurodegeneration was the focus of numerous studies, the involvement of adaptive immune cells, particularly CD8+ T lymphocytes, in neurological disorders was less explored (2, 5, 6, 8–19). The reason for this discrepancy may be due to a heterogeneous and plastic CD8+ T cell compartment, with T cell subsets that have not been yet well characterized in both normal and diseased conditions (7, 20, 21). Indeed, recent studies started to unveil the phenotypic and functional alterations occurring in the subpopulations of CD8+ T lymphocytes in various CNS diseases, but this research area is still in its infancy and many questions remain unanswered (6, 12, 14, 17–19, 22–25). Although CD8+ T lymphocytes are present in significant numbers in the brains of healthy individuals (22), their activity needs to be properly regulated in order to prevent potential detrimental local effects (15). Aging is widely recognized as a major risk factor for the development of neurodegenerative diseases and the aged CNS is characterized by a gradual loss of naïve and memory CD8+ T cells and an exponential increase in the number of transcriptionally altered exhausted and senescent T lymphocytes (20, 23, 26–28). However, the role of aging-induced CD8+ T cell alterations in brain disorders is poorly understood and a comprehensive view of the molecular mechanisms through which CD8+ T lymphocytes contribute to the development of diseases is lacking.
In this review, we discuss the role of CD8+ T lymphocytes in various neuroinflammatory pathologies, including common disorders such as AD, PD, multiple sclerosis (MS), and amyotrophic lateral sclerosis (ALS), as well as rare brain disorders such as limbic encephalitis-induced temporal lobe epilepsy (LE-induced TLE) and Susac syndrome (Sus). We highlight the heterogeneity of CD8+ T cell populations and their multifaced roles and discuss common disease pathways but also how CD8+ T cells may specifically promote aging and the development of neurodegenerative diseases.
CD8+ T cells origins and differentiation
CD8+ T lymphocytes are adaptive immune cells that arise from the bone marrow and mature in the thymus (26). After the release in the bloodstream, mature naïve CD8+ T cells search for their cognate antigen presented in the context of major histocompatibility complex-I (MHC-I) molecules expressed on the surface of antigen-presenting cells (APC) (29, 30). Upon antigen encounter, naïve CD8+ T lymphocytes become effector cells (31), whose main role is to mediate the apoptosis of the target cell via direct and indirect immune mechanisms, known as T cell-mediated cytotoxicity (32, 33). During this process, conventional T cells first establish contacts with the target cell via FasL-CD95 (FasR) binding, inducing the activation of the caspase cascade and releasing granzymes and perforins to facilitate apoptosis (33). Secondly, they produce pro-inflammatory cytokines, such as tumor necrosis factor-α (TNF-α) and interferon-γ (IFN-γ), which stimulate the expression of MHC-I and FasR molecules on the surface of the target cell, further promoting its death (32, 34–36). After antigen clearance, most effector CD8+ T lymphocytes undergo a controlled apoptosis during the “contraction phase” of the immune response, with only a small fraction of cells surviving as memory CD8+ T cells, providing immune protection from experienced antigens in the circulation and inside the tissues (37). Importantly, memory CD8+ T lymphocytes are maintained throughout lifetime, but their numbers may vary over time and during certain disease conditions (24, 26, 28, 30).
Naïve CD8+ T cells
Naïve CD8+ T lymphocytes are mature circulating cells that can acquire various effector functions depending on external clues (38). Their differentiation program is not pre-determined, but is shaped instead by conditions such as inflammatory states and ageing (39). In mice, the naïve phenotype is characterized by the expression of surface markers CD62L (L-selectin) and CD197 (CCR7), while in humans also includes the expression of CD45RA epitope (32, 39, 40) (Figure 1). Upon activation, naïve CD8+ T cells lose the expression of homing receptors, initiating a proliferation and differentiation program resulting in an army of effector CD8+ T lymphocytes (31, 40, 41) (Figure 1). In addition to the traditional method of identifying cells based on their classical surface markers, a new approach has emerged that focuses on their metabolic traits. Recent research has shown that naïve and memory T cells rely on oxidative phosphorylation and fatty acid oxidation, while effector T cells use aerobic glycolysis and amino acid metabolism to maintain their active state (42, 43).
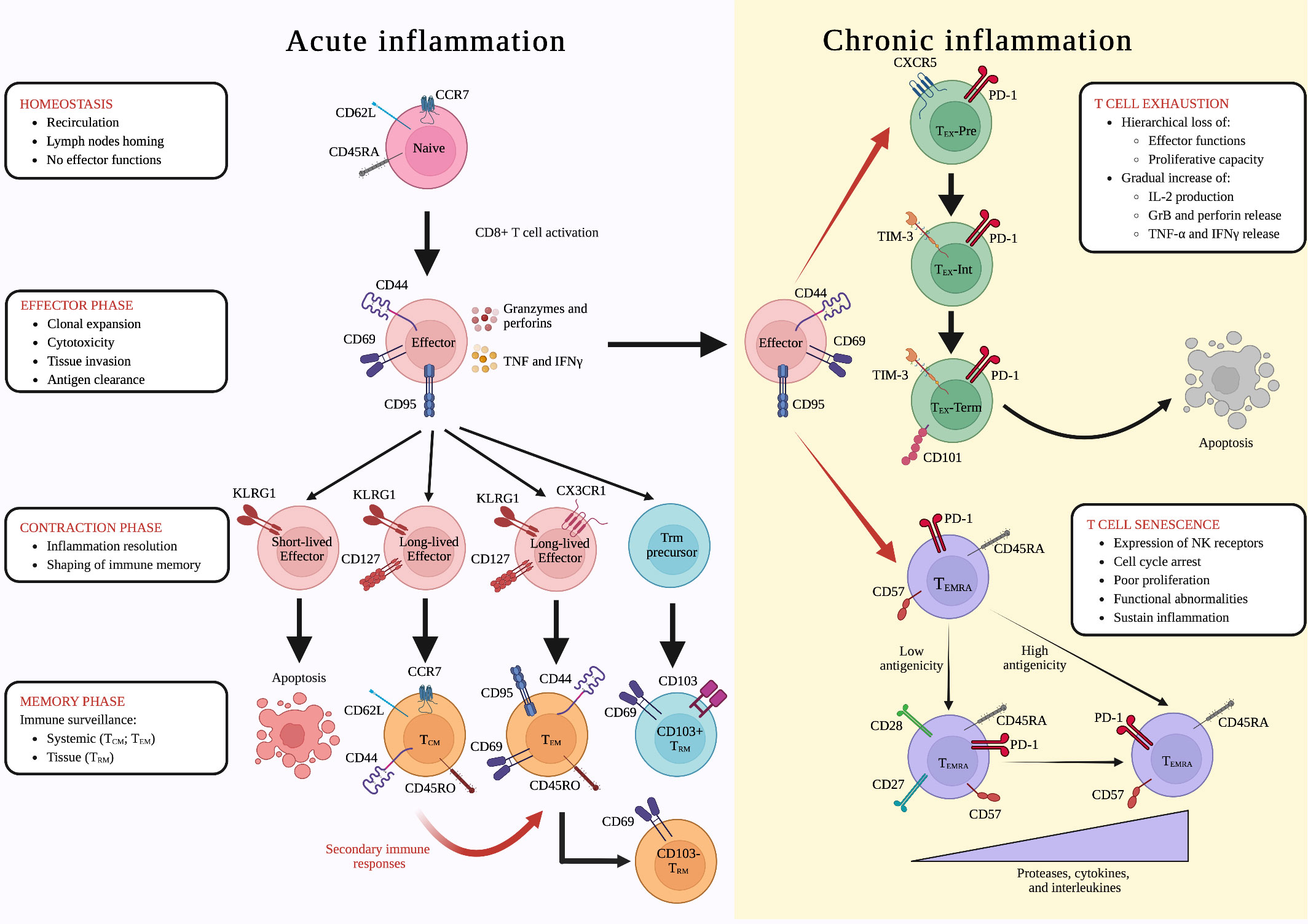
Figure 1 Differentiation of CD8+ T lymphocytes during acute and chronic inflammation. Inflammatory reactions are associated to several conditions including infections, tumors, autoimmune disorders and ageing. During acute inflammatory conditions, such as infections, naïve lymphocytes undergo cell activation becoming effector cells and leading to the “effector phase” of the immune response. Clonally expanded CD8+ T lymphocytes will then invade peripheral tissues, where they can exert cytotoxic functions. After antigen clearance, during the “contraction phase” of the immune response, KLRG1+ CD127- CX3CR1- short-lived effector cells undergo a controlled apoptosis, while long-lived KLRG1+ CD127+ CX3CR1- and KLRG1+ CD127+ CX3CR1+ give origin to central memory (TCM) and T effector memory (TEM) cells, respectively, shaping immunological memory. Once in the tissues, TEM lymphocytes could also undergo to another differentiation step, leading to the formation of CD69+ CD103- tissue resident memory (TRM) cells, whereas the CD69+ CD103+ TRM subset arise from KLRG1- effector TRM precursors. In addition, in case of re-infection with the same antigen, during the so-called “secondary immune response”, TCM lymphocytes are rapidly re-activated, differentiating into TEM cells. This differentiation cascade is altered under chronic immune and inflammatory reactions in which antigen stimulation is prolonged and effector CD8+ T lymphocytes undergo two distinct differentiation steps: (i) exhaustion, which is characterized by a hierarchical loss of effector functions and a gradual increase of intrleukin-2 (IL-2) production and granzyme B, perforin, tumor necrosis factor (TNF), and interferon gamma (IFNγ) release (CXCR5+ PD-1+ TEX-pre ➔ TIM-3+ PD-1+ TEX -Int ➔ CD101+ TIM-3+ PD-1+ TEX-Term); and (ii) senescence, during which CD57+ PD-1+ CD45RA+ TEMRA CD8+ T lymphocytes expressing natural killer (NK) receptors undergo cell cycle arrest, poor proliferation, and functional abnormalities, sustaining inflammation. Depending on the strength of antigenic stimulation, TEMRA cells release increased levels of senescent-associated secretory phenotype (SASP)-related proteases, cytokines, and interleukins (CD27+ CD28+ TEMRA ➔ CD27- CD28- TEMRA). Created with Biorender.com.
Effector CD8+ T cells
In contrast to naïve CD8+ T cells, the fate of effector T lymphocytes is more clearly defined (44, 45). Indeed, these cells are specifically activated and directed toward pathogen-derived or tumor-derived peptides, (29, 46, 47). Effector CD8+ T lymphocytes classically express CD44 and CD69 surface molecules, allowing them to enter peripheral tissues (32, 48, 49) (Figure 1). Moreover, they can be identified by the expression of CD95 (FasR) molecule, which contributes to the direct CD8-mediated cytotoxic process, and by the Ki-67 proliferation marker, which is important during clonal expansion (50–52). CD8+ T cells also possess a high cytotoxic potential by secreting various effector and cytotoxic molecules, including granzymes, perforins, IFN-γ, TNF-α and interleukin-2 (IL-2), which enable them to effectively combat infections (32, 33, 53, 54) (Figure 1).
Following antigen clearance, a two-tiered contraction occurs in the CD8+ T cell population, defined by the expression of the killer cell lectin-like receptor G member 1 (KLRG1). Short-lived effector KLRG1+ CD127- CD8+ T cells undergo selective apoptosis, whereas effector KLRG1+ CD127+ CD8+ T lymphocytes are preserved and evolve into exKLRG1 long-lived memory T cells, providing immunological memory (37, 41, 44) (Figure 1). However, a subset of effector CD8+ T cells, called memory precursors effector (TMPE) cells, has been found to lack KLRG1 expression, and this was associated with enhanced survival during the contraction phase and a higher developmental plasticity (37). Accordingly, TMPE cells retain the capability to differentiate in all the subsets of memory cells, playing a critical role in long-term protective immunity (37).
Memory CD8+ T cells
During the memory phase of the immune response, CD8+ T cells display immunological memory, which enhances their ability to rapidly and effectively respond to previously encountered pathogens, thus safeguarding the body against known threats (55, 56).
The memory compartment of CD8+ T lymphocytes comprises three different cell subsets: (i) T central memory (TCM), (ii) T effector memory (TEM), and (iii) tissue-resident memory (TRM) (55, 57). It is largely known that effector CD8+ T lymphocytes may generate all these memory populations during the “contraction phase” of the immune response and CX3CR1 expression has a key role in this process. Specifically, CX3CR1+ effector T cells appear to preferentially differentiate into TEM cells, while CX3CR1- precursors give rise to TCM cells (37, 58, 59) (Figure 1). The different origin of TCM and TEM reflects their phenotypical and functional differences. Similarly to naïve cells, TCM CD8+ T lymphocytes express CD62L and CD197 homing receptors, which are responsible for their recirculating behavior (32, 48, 60). However, TCM cells also express CD44 molecule in mice, and both CD44 and CD45RO epitopes in humans, indicating their memory-like phenotype (39, 61) (Figure 1). During the second expansion phase, TCM CD8+ T cells encounter their cognate antigen and differentiate into TEM CD8+ T lymphocytes, losing the expression of homing receptors and migrating into peripheral tissues, where they can release cytotoxic molecules (39, 62) (Figure 1). When the antigen is effectively cleared, TEM cells evolve into antigen-specific TCM CD8+ T lymphocytes, and the ultimate goal of these two populations is to provide systemic immunity.
Differently, local protective immune responses are orchestrated by TRM CD8+ T lymphocytes, which have a distinct profile from other CD8+ T cell subsets (59, 63). Notably, TRM CD8+ T cells are not recirculating, but are mainly organized in lymphoid niches close to anatomical and physiological barriers, acting as sentinels and protecting against reinfections (64). While further research is needed to fully understand the microenvironmental signals needed to establish and maintain the population of TRM CD8+ T cells into different peripheral tissues, it is clear that IL-15, IL-7, TGF-β, IL-21, TNF-α, and IL-33 play a crucial role in the formation of this CD8+ T cell subset (21, 65–68). The retention of TRM CD8+ T cells in peripheral tissues is mediated by CD69 and CD103 molecules, which are classically expressed on the surface of both human and murine TRM CD8+ T lymphocytes (22, 37). CD69 inhibits the expression of sphingosine-1-phosphate receptor 1 (S1PR1) molecule, promoting T cell residency, while CD103 integrin, an adhesion receptor for E-cadherin, contributes to CD8+ T cells persistence inside the tissues (69–71). Notably, all TRM CD8+ T lymphocytes express CD103 integrin in lymphoid tissues, but its expression may be lost in non-lymphoid tissues (64, 69). Accordingly, several studies reported the presence of both CD103+ CD69+ and CD103- CD69+ TRM CD8+ T lymphocytes in non-lymphoid tissues, such as liver, brain, gut, skin, and lungs (17, 22, 63, 65, 72–76). Recently, it has been demonstrated that CD103+ and CD103- TRM CD8+ T cells originate from two separate differentiation paths and are characterized by distinct effector functions (22, 37). ExKLRG1 effector CD8+ T cells give rise to CD103- TRM lymphocytes which can be distinguished from CD103+ counterpart due to their cytotoxic potential (37, 65) (Figure 1). In contrast, the precursors of CD103+ TRM cells, featuring a lower expression of granzymes and other effector molecules, seem to originate from KLRG1- TMPE CD8+ T lymphocytes (22, 37, 65) (Figure 1).
It is now well established that the TRM compartment of CD8+ T lymphocytes upregulates CXCR6 homing receptor gene, and ITGA1 gene, encoding CD49a collagen-binding integrin, while downregulating SELL and CX3CR1 genes, encoding for CD62L and CX3CR1 molecules, respectively (17, 57, 77). Moreover, Runx3, Notch, Bhlhe40, Blimp1 and its homolog Hobit, and the AP-1 family members, including Jun, Junb, Jund, Fos, Fosb, and Batf have been identified as crucial transcription factors (TFs) in the regulation of TRM cells formation (59). Additionally, TFs induced by interferon signaling, such as Stat1, Irf1, Irf7, and Irf9, or related to the NF-κB signaling pathway, including Bcl3, Rela, Relb, Rel, and Nfkb2 are enriched in TRM T lymphocytes, adding further markers for this CD8+ T cell population (59). Altogether, these data highlight the heterogenicity and complexity within the TRM compartment of the CD8+ T cell population.
Exhausted CD8+ T cells
All stages of the immune response and their players are perfectly coordinated and functioning under acute inflammatory states, when the immune reaction successfully clears antigens. However, persistent antigen stimulation leads to chronic inflammation, disrupting this harmoniously synchronized mechanism (78, 79). Effector T cells in this condition become dysfunctional, undergoing exhaustion, resulting in poor effector functions and reduced proliferative potential (78, 79) (Figure 1; Table 1). Importantly, T cell exhaustion is not just an alteration of cell phenotype and functions, but also represents a distinct differentiation state, with different characteristics compared to the memory features (24, 81). Exhausted (TEX) CD8+ T cells maintain the same characteristics under different inflammatory conditions, with a well-defined gene signature, including TCR-signaling related genes such as Batf, Egr2, Ezh2, Irf4, Nfatc1, Nfatc2, Nr4a1, Nr4a2, and Nr4a3 (94–96), confirming that continuous exposure to persistent antigens is a key factor in T cell exhaustion, whereas short antigen exposures lead to exhaustion recovery (80) (81) (Table 1).
Hyporesponsive TEX cells are defined by their high surface expression of programmed cell death-1 (PD-1), lymphocyte-activation gene 3 (LAG-3), CD244 (2B4), T-cell immunoglobulin and mucin domain-3 (TIM-3), cytotoxic T-lymphocyte-associated protein-4 (CTLA-4), and CD160 inhibitory receptors (24, 82–85) (Table 1). These receptors typically expressed on the surface of TEX CD8+ T bind to a variety of ligands, suggesting that microenvironment clues, such as ligand availability, may regulate the functionality of TEX CD8+ T lymphocytes (78, 79).
TEX lymphocyte compartment is heterogeneous showing three differentiation states: (i) T cell factor 1+ (TCF1+) PD1+ CXCR5+ TEX precursors (TEX-Pre), expressing the T-bet TF and showing memory-like features, such as the expression of Sell, Ccr7, Id3, and Bcl6 genes; (ii) PD1+ TIM-3+ TCF1- Intermediate TEX (TEX-Int), not expressing Zeb1 gene, which encodes for the ZEB-1 TF; and (iii) PD1+ TIM-3+ CD101+ TCF1- terminally differentiated TEX (TEX-Term) expressing the TFs ZEB-1, Blimp-1, and Eomesodermin (EOMES) (24, 97, 98) (Figure 1). Along the differentiation process, TEX-Pre, TEX-Int, and TEX-Term show a hierarchical decrease of effector activity, marked by mitochondrial dysfunctions, and proliferative capacity, while gradually increasing the expression of inhibitory receptors, ultimately leading to cell death (78, 98, 99) (Figure 1). However, TEX lymphocytes do not entirely lack effector functions, exhibiting instead a gradual increase of (i) IL-2 production, (ii) cytotoxicity mediated by granzyme B and perforin, and (iii) release of pro-inflammatory molecules such as IFNγ and TNF-α (78, 79, 98) (Figure 1; Table 1).
Senescent CD8+ T cells
Senescent CD8+ T cells exhibit cell cycle arrest and poor proliferation along with severe functional abnormalities similar to those occurring during T cell exhaustion (78, 87, 88) (Figure 1; Table 1). They differentiate from effector CD8+ T cells typically occurring during conditions associated to chronic inflammation, including auto-immune diseases, cancer and ageing (88–91) (Table 1). Unlike hypo-functional TEX lymphocytes, senescent CD8+ T lymphocytes continue to secrete a range of factors including proteases such as cathepsins and serine proteases, and cytokines such as CCL5, CCL16, CCL23, TNF-α, IL-29, and IL-18, which in turn may induce IFN-γ production, suggesting a pro-inflammatory senescent-associated secretory phenotype (SASP) (87, 92) (Table 1). Moreover, CD45RA molecule is re-express on the surface of these hyper-functional CD8+ T lymphocytes, which are commonly referred as effector memory CD45RA+ (TEMRA) cells (91). TEMRA cells are characterized by the simultaneous expression of PD-1, KLRG-1, and CD57 on their surface, which also represent classical phenotypical markers of senescent CD8+ T lymphocytes (82, 91, 100–103) (Figure 1). A certain degree of heterogenicity has been also shown among TEMRA lymphocytes. Accordingly, a recent report revealed that effector CD8+ T cells differentiate into CD27- CD28- or CD27+ CD28+ TEMRA cells based on the strength of TCR engagement and the immunogenicity of the tumor antigens (91) (Figure 1), while other studies have shown that the lack of CD27 and CD28 surface molecules is associated with the expression of p16 and p21 proteins, causing G1 cell cycle arrest and replicative senescence (86, 87) (Table 1).
Senescent CD8+ T cells possess the capability to express natural killer (NK)-associated receptors (NKR), such as NKG2D, NKG2C, NKG2A, and killer immunoglobulin-like receptor (KIR) families, allowing them to be reprogrammed into hyper-functional lymphocytes with the ability to recognize and kill target cells through both TCR and NKR recognition mechanisms (93) (Figure 1; Table 1). Notably, senescent CD8+ T lymphocytes expressing NKR need to be distinguished from invariant natural killer T (iNKT) cells, which are a subset of natural killer T (NKT) innate immune cells that decrease with age (104, 105). Thus, senescent CD8+ T cells seem to have beneficial as well as detrimental roles during aging, having the potential to retain a broad spectrum of effector functions to kill malignant and infected cells, whilst also having the capacity to induce or sustain autoimmunity and other chronic disorders (106). However, further studies are required to better clarify the involvement of senescent CD8+ T cells during homeostasis and disease.
CD8+ T cells during ageing
CD8+ T cell compartment is essential in providing long-term immune protection, but its composition may be altered during aging (26, 28, 89). Naïve CD8+ T cells are mainly located in the blood, spleen and lymph nodes, where they can respond to new antigens, while memory CD8+ T cells are found predominantly in tissues such as lung, gut, and brain to rapidly protect against potential infections (11, 22, 26, 60, 77, 107). Infancy is characterized by the egress of a large number of naïve CD8+ T cells from the thymus, which then differentiate into memory cells upon antigen exposure (26, 28, 108). Differently, aging is associated with immunosenescence, resulting in a decrease in the number of naïve and memory CD8+ T cells and an increase of senescent CD8+ T lymphocytes, particularly in the blood and blood-rich sites, such as spleen and lungs (26, 28, 60, 77, 82, 109). During aging, the production of naïve CD8+ T lymphocytes significantly decreases due to age-related thymic involution. This process also leads to changes in the phenotype of CD8+ T cells, such as an increased generation of self-reactive T cells (110, 111), potentially explaining the higher occurrence of autoimmune disorders in older individuals (112).
Moreover, the reduced adaptive immune response during aging promotes innate immunity responses and the release of pro-inflammatory mediators, leading to a state of low-grade systemic inflammation, creating a vicious cycle that further contributes to immunosenescence (113–115). Inflammageing also causes the accumulation of TEX and senescent CD8+ T cells in various organs, where they can make up to 60% of all CD8+ T cells, contributing to significant immune alterations during aging (115, 116). This overall decline in T cell functionality, combined with an increased apoptotic rate of T lymphocytes, can lead to the development of age-related diseases, including neurodegeneration (114). Accordingly, it was recently demonstrated that accumulation of CD8+ T cells in the CNS of aged mice drives axonal degeneration and contributes to age-related cognitive and motor decline through the release of the cytotoxic molecule granzyme B (20). In addition, it was shown that clonally expanded INF-γ-producing CD8+ T cells infiltrate old neurogenic niches in the healthy brain, inhibiting the proliferation of neural stem-cells, potentially contributing to age-related deterioration of brain functions (117). These data suggest that targeting CD8+ CNS-associated T cells in older adults might mitigate aging-related decline of brain structures and functions.
Despite these evidences showing that alterations in CD8+ T lymphocyte characterize ageing and sustain neurodegeneration, it is still debated whether these are the cause or consequence of age-related micro-environment perturbations. Recent findings suggest that reduced extrinsic nutritional availability of glucose, amino-acids, and lipids in older tissues may negatively affect CD8+ T cell functioning. In support of this, metabolic alterations are considered among the main differences between young and old T lymphocytes (113, 118). Thus, the immune changes considered as characteristics of ageing could be instead viewed as the manifestation of elderly-related environmental interferences, which can be modulated by lifestyle factors. Overall, age-related T cell dysfunctions can be regarded as alterations potentially mitigated by a nutrition- and exercise-based approach to improve human health and longevity (118, 119).
CD8+ T cells in brain diseases
Immunosenescence and inflammageing play a role in patients with neurodegenerative and neuroinflammatory disorders such as AD, PD, and MS, in which the well-balanced inflammatory and anti-inflammatory equilibrium is lost, leading to a prolonged and uncontrolled state of chronic low-grade inflammation (114, 120). In addition, senescent CD8+ T lymphocytes were also detected in the brain of patients affected by LE-induced TLE and Sus, suggesting common pathogenic mechanisms underlying different brain disorders (13, 18).
Alzheimer’s disease
AD is a progressive neurodegenerative disorder characterized by neuronal death and accumulation of amyloid beta (Aβ) deposits and hyperphosphorylated tau protein in the brain, leading to memory loss and cognitive impairment (121). To date, approximately 35 million people worldwide have been affected by AD, making this disease the most common cause of dementia (122). Approximatively 75% of AD subjects are 75 years old or older, indicating a strong correlation between the development of AD and aging (123). Moreover, a shorter telomere length in CD8+ T cells was correlated to a greater AD severity, together with a lower CD28 expression and an increase of cytotoxic molecules and sensitivity to apoptosis, suggesting the presence of dysfunctional CD8+ T cells in AD (124, 125). The majority of studies performed in AD showed changes in the overall CD8+ T cell population in mice developing amyloid-related pathology, that may not closely reflect the human condition in which tau pathology also represents a disease hallmark (8, 10, 15, 17). Indeed, the association between tau pathology and CD8 T cells was previously suggested, although more recent studies found no correlation between tau hyperphosphorylation and the presence of cytotoxic T cells (15, 126, 127).
The heterogenicity of the CD8+ T compartment has been only recently studied in AD, showing clonally expanded CD8+ TEMRA cells with cytotoxic potential in the cerebrospinal fluid (CSF) of AD individuals (6). Surprisingly, TEMRA lymphocytes were not clonally expanded against AD-specific antigens such as Aβ peptides or tau protein, but they were reactive in the presence of Epstein-Barr virus (EBV) antigens (6) (Figure 2). These data do not provide a causal link between EBV infection and AD, but suggest that senescence of CD8+ T lymphocytes may play a role in AD (128). Furthermore, the absence of clonal expansion toward disease-specific antigens suggests that CD8+ T cell trafficking into the CNS could be a stochastic phenomenon (6, 17). Accordingly, the damage of CNS barriers previously described in AD can favor nonspecific CD8+ T cells brain invasion from the blood circulation (129) (Figure 2). The increased expression of lymphocyte function-associated antigen 1 (LFA-1) integrin on infiltrating total T cells in the AD brain, and the augmented expression of Itgb2 gene, encoding for the CD18 subunit of LFA-1 molecule, in the hippocampus of a mouse model of tauopathy compared to controls suggest a role for LFA-1-intercellular adhesion molecule-1 (ICAM-1) interactions in the migration of CD8+ T cells in the CNS during AD (8, 126). This is also supported by the increased expression of ICAM-1 detected on brain endothelial cells of mice with AD-like disease and higher levels of soluble ICAM-1 in the plasma from AD subjects compared to controls (3, 130).
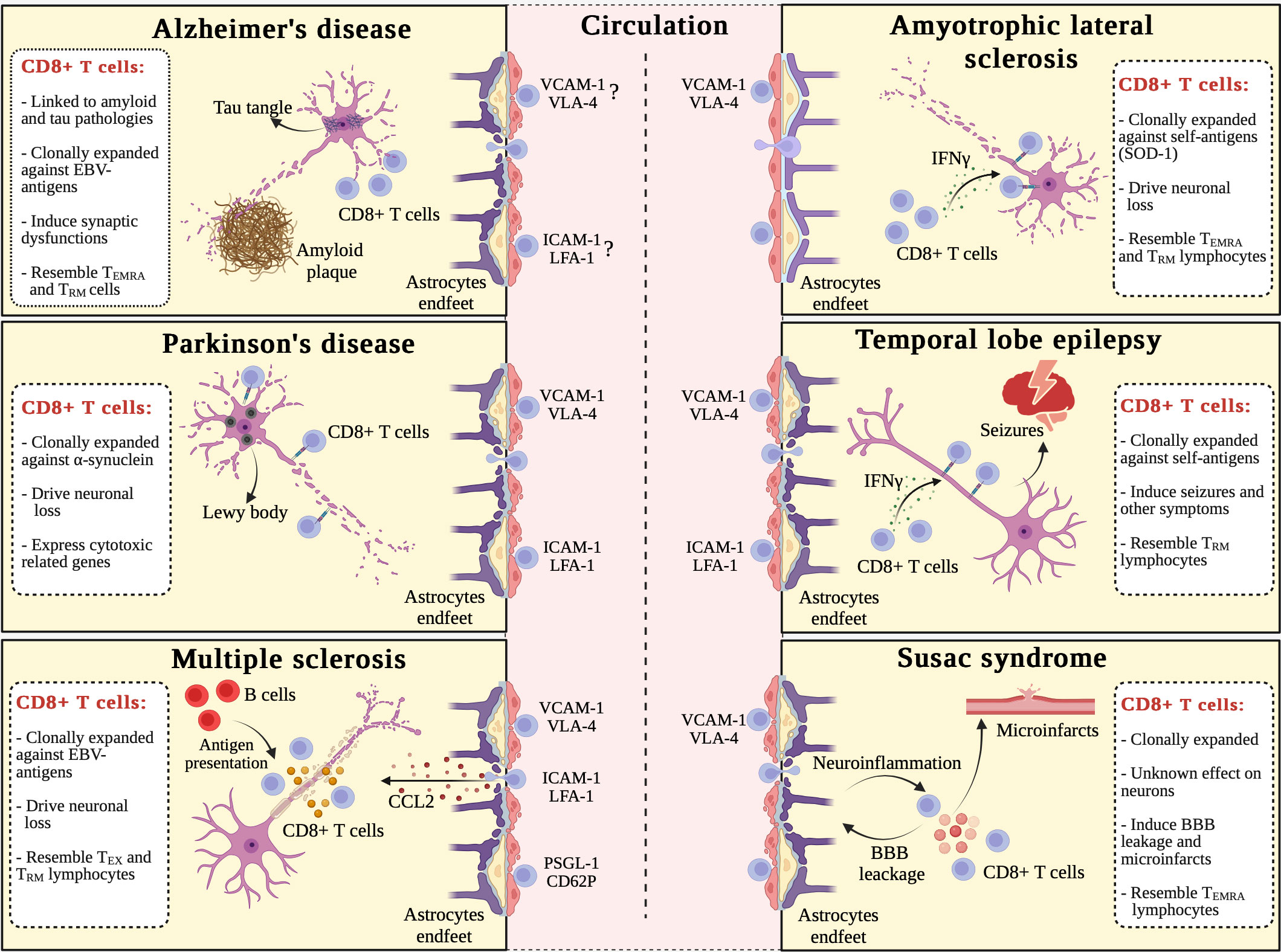
Figure 2 Commonalities and differences in CD8+ T cell-driven immune responses in brain disorders. Alzheimer’s disease (AD), Parkinson’s disease (PD), multiple sclerosis (MS), amyotrophic lateral sclerosis (ALS), limbic encephalitis (LE)-induced temporal lobe epilepsy (TLE), and Susac syndrome (Sus) are all characterized by the migration of circulating CD8+ T lymphocytes into the CNS. The following molecular pathways drive the extravasation of CD8+ T cells in these disorders: (i) VCAM-1/VLA-4 adhesion pathway contributes to all diseases discussed in this review; (ii) ICAM-1/LFA-1 adhesion pathway has a role in PD, MS, and LE-induced TLE; and (iii) PSGL-1/CD62P adhesion pathway is involved only in MS. Once in the CNS, CD8+ T lymphocytes clonally expanded against non-self (AD, MS, and Sus) or self (PD, ALS, and LE-induced TLE) antigens: (i) induce synaptic dysfunctions in AD; (ii) drive neuronal loss in PD, MS, and ALS; (iii) support seizures and other epilepsy-related symptoms in LE-induced TLE; and (iv) promote blood-brain barrier (BBB) leakage, neuroinflammation, and microinfarcts damaging endothelial cells in Sus. CD8+ T cell-driven cellular alterations are induced by direct cytotoxicity (TCR-MHC-I binding) in PD, ALS, and LE-induced TLE, as well as soluble factors, including granzymes, in MS and Sus. In addition, two district features characterize MS: (i) B cells present Epstein Barr-derived (EBV) antigens to T cells; and (ii) CCL2 is crucially involved in the homing of blood-derived CD8+ T lymphocytes to the inflamed CNS. ALS is apparently the only disorder not yet associated to BBB dysfunction. CD8+ T cells from patients with AD, ALS, and Sus show a TEMRA senescent-like phenotype, while in MS CD8+ T cells have an “exhausted” phenotype. Except for PD, CD8+ T lymphocytes from all discussed CNS disorders, have tissue resident memory (TRM) traits. Created with Biorender.com.
Although ablation of CD8+ T cells in AD mice did not reduce Aβ deposition and cognitive deficits in APP-PS1 mice at later disease stages, recent studies suggested that CD8+ T cells migrate in the brain parenchyma in subjects with AD and its mouse models (15, 127) (Figure 2). The phenotype of CD8+ T lymphocytes in aged and AD transgenic mice have a TRM gene signature, but the characterization is still in its infancy (17, 20) (Figure 2). In summary, existing literature suggests CD8+ T cell heterogeneity in AD, highlighting a potential role for TEMRA and TRM cells subsets in the pathogenesis of this disorder. However, the involvement of CD8+ T cells in AD is still unclear and more studies are needed to identify the molecular mechanisms mediating a potential CD8+ T cell-dependent damage.
Parkinson’s disease
PD is the second most common form of neurodegenerative disorder, affecting more than 10 millions people around the world, with an increased prevalence in the aged population (131). The main neuropathological hallmark of the disease is the presence α-synuclein (α-syn) aggregates, referred to as Lewy bodies, and Lewy neurites, observed in neuromelanin-containing neurons of the substantia nigra (SN) (132). This is associated with the classical PD symptoms of bradykinesia, rest tremor, muscular rigidity, motor and cognitive alterations, as well as autonomic dysfunctions (132). Similarly to AD, the initiators of the pathogenic cascade leading to neuronal death and to the related neurological alterations are presently poorly understood in PD.
Recently, a study conducted in PD patients provided novel insights into the T cell-mediated adaptive immune responses by performing single-cell transcriptome and TCR sequencing, revealing a continuous progression of CD8+ T cells from a central memory to a terminal effector phenotype (133). In addition, previous data showed that CD8+ T cells are increased in the substantia nigra of diagnosed PD cases and positively correlate with neuronal death (5). Interestingly, CD8+ T cells infiltration is present since early disease stages, preceding neuronal loss and α-syn aggregation, suggesting a role for these cells in disease development (5). Notably, although a direct demonstration is still lacking, CD8+ T cells with a TRM phenotype were detected near altered neurons in the substantia nigra, suggesting that CD8+ T cells may exert cytotoxic functions potentially contributing to the pathological changes in PD (5) (Figure 2). Furthermore, a longitudinal case study and analysis of two independent cohorts revealed that elevated α-syn-specific CD8+ T cell responses in the blood were present prior to and after diagnosis of motor PD, and were significantly associated with age (134) (Figure 2). These studies suggest that CD8+ T cell infiltration is an early event in PD, paralleling the progression of neuronal death and synucleinopathy, providing insight into new disease mechanisms and early diagnosis in PD. Accordingly, T cells from PD patients do not react to common antigens, but are activated by α-syn-derived antigens, suggesting that the T cell responses observed in PD may be prevalently directed towards autoantigens (135).
Recent data showed a core gene signature for α-syn-reactive CD8+ T lymphocytes in PD, which includes the expression of CX3CR1, CCR5, CCR1 pro-inflammatory genes, but also genes expressed in neurons such as LRRK2, LAMP3, and aquaporin genes, previously associated with PD worsening (136). Interestingly, the increased expression of the leucine-rich repeat kinase 2 (LRRK2) in CD8+ T lymphocytes from PD patients correlated with an increased secretion of pro-inflammatory molecules and cell activation, suggesting that LRRK2 may represent a therapeutic target in PD (137). Accordingly, increased expression of granzymes and perforin 1 in clonally expanded CD8+ T cells are present in the blood and CSF of PD patients (133) (Figure 2). Also, the increased cytotoxicity of CD8+ T lymphocytes in PD correlates with a terminal effector phenotype and the expression of proteins involved in cell migration, such as CX3CR1 and the adhesion G protein-coupled receptor G1 (ADGRG1) (133). The migration of CD8+ T lymphocytes into the CNS during PD (5) has been associated with the upregulation of ITGAM and ITGB1 genes, suggesting a role for CD11b and CD29, subunits of LFA-1 and very late antigen-4 (VLA-4) integrins, respectively (133) (Figure 2). Moreover, the disruption of the blood-brain barrier (BBB) in PD (138, 139), indicate that vascular phenomena may also contribute to CD8+ T cell infiltration into the CNS (Figure 2). Overall, these findings emphasize an increased immune reactivity of CD8+ T cells against CNS antigens in PD, which is associated with an enhanced clonally expanded T cell infiltration and cytotoxicity in the brain, suggesting a circulating origin of α-syn-reactive CD8+ T lymphocytes in the PD brains (133, 135). Future studies exploring the phenotype and functions of CD8+ T lymphocyte subsets in PD could lead to the identification of novel and specific therapeutic targets for this neurodegenerative disease.
Multiple sclerosis
MS is a chronic inflammatory and autoimmune disorder of the CNS affecting approximatively 2.8 million people worldwide (140). Multifocal inflammatory lesions develop in both brain and spinal cord (SC), resulting in demyelination and neurodegeneration and leading to a progressive decline in motor, sensory, and cognitive functions (141). In most patients, MS is characterized by a relapsing and remitting onset, followed by a chronic, prolonged, and progressive inflammatory state during which the neurological symptoms gradually worse (141). Several studies have shown that CD8+ T lymphocytes predominate in active MS lesions (142–144). Furthermore, CD8+ T cells from in MS lesions are clonally expanded against common antigens and have been probably recruited from the periphery (12, 142, 145) (Figure 2). Indeed, it has been demonstrated that blood, CSF and brain CD8+ T cell clones share a high degree of phenotypic similarity further supporting the view that CD8+ T cells invading the MS brain originate from the periphery and contribute to MS progression (144, 145). The homing of clonally expanded CD8+ T lymphocytes to the CNS of MS patients may be further promoted by BBB damage and increased expression of adhesion molecules and chemoattractants on brain endothelial cells (143, 146) (Figure 2). Moreover, previous studies have shown a role for platelet and endothelial cell adhesion molecule 1 (PECAM1), P-selectin glycoprotein ligand-1 (PSGL-1), vascular cell adhesion protein-1 (VCAM-1), intracellular adhesion molecule-1 (ICAM-1), VLA-4 and LFA-1 in the migration of CD8+ T cells into the CNS (143, 144, 147, 148) (Figure 2). Once infiltrated to the CNS, CD8+ T cells express higher levels of cytotoxic molecules (19, 143, 144), thus suggesting their detrimental role in the progression of MS-related neurological alterations. Indeed, CD49d+ CD8+ T lymphocytes invading the MS brain exhibited a pro-inflammatory effector phenotype, expressing CD137 and CD95L, as well as inhibitory receptors TIM-3 and PD-1 on their surface, in addition to the transcriptional factor EOMES (12, 14, 19, 143) (Figure 2). Importantly, an increased production of granzyme B by CD8+ T cells in MS lesions, has been suggested to contribute to neuronal alterations (143, 144) (Figure 2). In line with these findings, in early MS brain lesions the majority of CD8+ T lymphocytes express CD69, but not CD103, and were shown to contain granzyme B (12). Although a link has been suggested between T cell exhaustion and the progression of chronic neuroinflammation in MS, the contribution of TEX CD8+ T lymphocytes in MS course is unclear (149). EBV infection is now considered a key environmental factor for chronic CNS inflammation during MS and CD8+ T cells are clonally expanded against EBV-derived antigens presented by B cells in MS patients (12). EBV can establish a prolonged latent and intermittent reactivation within B cells (150), potentially resulting in a series of CD8+ T cells immune responses, suggesting that B cells may represent essential players in promoting chronic CD8+ T lymphocyte activity in MS (151). Overall, MS represents the neurodegenerative disease in which CD8+ T cells were studied more into detail, although the molecular mechanisms leading to brain damage are not yet fully understood.
Amyotrophic lateral sclerosis
ALS is an incurable and devastating neurodegenerative disorder, that causes a progressive degeneration of motor neurons leading to a loss of voluntary muscle control and, in severe cases, to respiratory failure (152). Most commonly, symptoms of ALS appear between the ages of 40 and 70, and several genes were associated to the development of this disease, including superoxide dismutase 1 (SOD1) (152–155). The normal function of SOD1 protein is to protect cells from oxidative damage, and its alterations lead to increased oxidative stress and mitochondrial dysfunctions (156). In addition, oxidative stress levels are substantially increased by neuroinflammation, which has been recently included among the hallmarks of ALS (157, 158).
A role for CD8+ T lymphocytes in SOD1-associated ALS form was recently suggested (159–161). Particularly, peripheral CD8+ T cell ablation has been shown to increase motoneuron survival in a mouse model of ALS, whereas in vitro studies showed that SOD-1 expressing CD8+ T lymphocytes recognize and selectively kill motoneurons via binding MHC-I molecules expressed on these cells, suggesting a possible autoimmune origin for ALS (159) (Figure 2). Moreover, activated CD8+ T lymphocytes expressing mutant SOD-1 produce high levels of IFNγ and eliminate a subset of motoneurons in ALS through an antigen restricted, MHC-I-dependent cytotoxic pathway, suggesting a neurotoxic role for self-reactive CD8+ T cells in ALS (159) (Figure 2). These data were supported by studies performed in ALS patients showing an increased activation of peripheral and intrathecal CD8+ T cells, with the activation status of CD8+ lymphocytes in the blood being higher in ALS compared to MS and dementia, further suggesting a role for cytotoxic lymphocytes in ALS (160).
Clonally expanded TEMRA CD8+ T cells originating from the circulation have been reported in the CNS of Setx knock-in (KI) mice developing ALS4-like disease, as well as in ALS4 patients, who typically have mutations on senataxin (SETX) gene (18) (Figure 2). These data point to TEMRA cells as negative contributors during ALS and are further supported by a recent retrospective study demonstrating higher frequencies of senescent-like T cells in ALS individuals, suggesting that lymphocyte senescence may drive disease progression (162). Furthermore, the immunophenotyping of TEMRA CD8+ T lymphocytes detected in the CNS of Setx KI mice revealed a CD49d+ PD-1+ CD103- profile (18), which is consistent with their peripheral origin in ALS4-like mice, suggesting pathological changes of the TRM CD8+ T cell compartment similar to those observed in MS (12) (Figure 2). Nevertheless, differently from MS, the severity of ALS does not appear to be correlated with BBB leakage, suggesting that the infiltration of CD8+ T cells into the CNS of ALS patients and animal models could represent a tightly regulated process, rather than being favored by stochastic events (163, 164) (Figure 2). Recent data obtained in two ALS mouse models showed that blocking α4-integrins reduces the migration of peripheral immune cells into the CNS and decreases IFNγ, which is primarily produced by CD8+ T lymphocytes and NK cells, further supporting a role for peripheral cytotoxic T cells in ALS (159, 161, 165). However, more research is needed to identify the molecular mechanisms governing the extravasation of CD8+ T cells into the CNS during ALS.
Limbic encephalitis-induced temporal lobe epilepsy
Limbic encephalitis (LE)-induced temporal lobe epilepsy (TLE) is a rare form of epilepsy characterized by different types of epileptic seizures, including focal seizures, secondarily generalized seizures, and status epilepticus (166). This subtype of encephalitis is also characterized by a potent inflammatory reaction predominantly against neurons in the grey matter of the medial temporal lobes of the brain, leading to the generation of recurrent seizures (167, 168). Previous studies associated neuroinflammation, BBB dysfunction and leukocyte migration to the induction of seizures (169–172).
LE is triggered by an autoimmune response favored by various underlying causes, including viral infections and paraneoplastic syndromes (173–175). Indeed, the presence of autoantibodies directed toward neuronal surface antigens (NSAbs) was well described in LE patients (176–180). Moreover, it was suggested that IFNγ-producing CD8+ T lymphocytes promote MHC-I upregulation on LE neurons, thus supporting a neuron-directed CD8+ T cell attack, similar to the immune reactivity demonstrated in ALS (16, 181) (Figure 2). CD8-mediated neurotoxicity has been suggested to contribute to neuronal excitability and acute seizure generation, promoting psychiatric disturbances, memory impairment and behavioral changes (182). Furthermore, it has been hypothesized that chronic neuroinflammation can induce persistent changes in the structural and electrical properties of certain neuronal networks, resulting in the development of chronic spontaneous seizures and epilepsy (16, 181, 182) (Figure 2). Notably, infiltration of granzyme B-producing CD8+ T lymphocytes, which may attack and destroy neurons expressing MHC-I, was found among other leukocyte populations in the hippocampi of TLE patients (2, 16, 183–188) (Figure 2). Interestingly, cytotoxic CD8+ T cells accumulate in the brain of LE patients, suggesting that self-reactive CD8+ T cells can directly cause neurotoxicity, but can also contribute to the conversion of LE into TLE (178) (Figure 2).
Recent studies showed that activated CD44+ ovalbumin (OVA)-specific CD8+ T cells, directed against the “SIINFEKL” chicken peptide (OVA 257-264) expressed exclusively by hippocampal neurons, migrate into the brain and promote seizures (16). Furthermore, CD8+ T lymphocyte infiltration leads to prolonged epileptic activity and memory deficits, suggesting that circulating CD8+ T cells may play a role in the induction and progression of LE-induced TLE (16) (Figure 2). Brain invasion by circulating CD8+ T lymphocytes correlate to BBB dysfunction in experimental models of TLE and magnetic resonance imaging revealed BBB alterations after the transfer of OVA-specific CD8+ T cells, followed by a size reduction and degeneration of the hippocampus (2, 16, 169) (Figure 2). Moreover, brain-invading CD8+ T lymphocytes showed an increased expression of CD69 surface marker, suggesting that these cells may acquire a TRM phenotype once migrated into the brain (16), similarly to what has been previously described in MS (12). This may be supported by previous studies showing that ICAM-1 and VCAM-1 are upregulated in TLE hippocampi and after seizure induction and that preventive blockade of α4 integrins or ICAM-1 adhesion receptor abrogates seizure induction (2, 169). Of note, TLE patients display a T cell activated phenotype in peripheral blood (185). Thus, CD8+ T lymphocytes could migrate into the inflamed brain in TLE using LFA-1 and/or α-4 integrins, as shown in ALS and as previously suggested in patients with MS and epilepsy (189) (Figure 2).
Susac syndrome
Sus is a rare disorder characterized by neuroinflammation and CNS dysfunction, due to focal microangiopathy affecting small and medium size vessels of the brain, retina and the inner ear (190, 191). The etiology of Sus remains largely unknown, and the role of neuroinflammation has only recently started to be addressed. However, the successful use of immunosuppressive and immunoregulatory drugs in Sus cases, support an autoimmune origin for this disease (192, 193). Accordingly, an increased immune cell infiltration into the brain, with the majority of cells being CD8+ T lymphocytes, has been found in Sus patients compared to healthy controls (9, 194). Also, it has been suggested that circulating self-reactive CD8+ T lymphocytes may promote endothelial cell alterations and BBB dysfunction during Sus, potentially leading to microinfarcts and leukocyte transmigration across the BBB (13) (Figure 2). Moreover, Sus patients treated with natalizumab, which inhibits lymphocyte migration into the CNS, or mice treated with an antibody blocking anti-α4 integrins, showed a reduction in disease severity compared to controls, further supporting the idea that Sus shares several key immunopathological elements with MS (13) (Figure 2). Indeed, VLA-4 neutralization was found to restrict infiltration of CD8+ T lymphocytes into the CNS of EAE mice (143, 195), suggesting this may be the case also in Sus. Despite several common disease mechanism between MS and Sus, the total number of leukocytes detected in the CSF is in the normal range in Sus patients, whereas it is significantly increased in MS cases (13). However, the proportion of CD8+ T cells is selectively and significantly increased in the CSF of Sus subjects, suggesting a role for these cells in Sus (13). Sus patients have a larger proportion of clonally expanded CD57+ CD8+ TEMRA lymphocytes of circulating origin close to disease onset, with lower clonal expansion levels during remission, further supporting a relationship between Sus pathogenesis and CD8+ T cells (13). Moreover, immunophenotyping of CD8+ T lymphocytes in brain of Sus patients revealed a cytotoxic capacity, as evidenced by the higher expression of granzyme B lytic molecule compared to healthy controls (13) (Figure 2). Overall, several lines of evidence point to a key role for CD8+ cells in Sus, but more studies are needed to understand how these cells contribute to BBB dysfunction and neuronal damage.
Conclusions
CD8+ T lymphocytes are adaptive immune cells that, upon antigen recognition, undergo a complex differentiation process (Figure 1). In acute inflammatory responses, when antigen is effectively cleared, short-lived effector T cells undergo controlled apoptosis, while long-lived effector T lymphocytes differentiate into memory T cells, thus efficiently resolving the inflammatory reaction. However, during chronic inflammatory conditions, this natural resolution is impaired, and CD8+ T lymphocytes become exhausted or senescent, retaining a neurotoxic potential and contributing to several neurodegenerative diseases. CD8+ T cells, reacting against self and non-self antigens are clonally expanded in all brain disorders discussed in this review: AD, PD, MS, ALS, LE-induced TLE and Sus. It is worth noting that although these disorders may have distinct causes, occurrence rates, and clinical presentations, they share common immunopathological characteristics. These include the circulating origin of CNS-invading CD8+ T lymphocytes, the clonal expansion of CD8+ T cells, and phenotypical traits that resemble senescence (Figure 2). In the light of growing evidence suggesting that senescent and exhausted CD8+ T cells contribute to aging and various brain disorders, a promising therapeutic approach for these conditions may be represented by targeting deleterious functions of CD8+ T cells. Indeed, targeting senescent and exhausted CD8+ T cells may create a personalized neuroimmunotherapy, with the ultimate goal to rejuvenate T cells through tailored diagnostic and therapeutic protocols (87, 196). Strategies such as epigenetic modulation and using senolytic compounds to induce apoptosis in senescent and exhausted CD8+ T cells may also be explored. Several studies are ongoing to prove the effectiveness of interventions targeting tissue-damaging senescent cells, which may slow, prevent, and alleviate disorders in preclinical models (197). The development of senolytic small-molecules that can specifically eliminate senescent cells, may represent a promising strategy for treating multiple CD8+ T cell senescent-mediated disorders and age-related conditions in humans (197). Also, the epigenetic modulation of senescent and exhausted CD8+ T cells involving small molecules and biologics to target the molecular pathways involved in developing and maintaining these cell types, can modify the senescence and exhaustion, potentially reversing these deleterious phenotypes (198–200). For example, it has been recently observed that EZH2-expressing T cells are precursors to KLRG1+ effector lymphocytes, while EZH2LOW-expressing T cells predominantly produce non-cytotoxic CD103+ CD69+ TRM CD8+ T cells (201). Thus, the silencing or deficiency of the Ezh2 gene, which mediates these epigenetic modifications in CD8+ T lymphocytes, may be therefore targeted to induce repression of the exhausted phenotype (200). Overall, these approaches may help to reduce the number of neurotoxic CD8+ T cells and potentially mitigate the effects of aging and neuroinflammatory disorders.
Author contributions
All authors listed have made a substantial, direct, and intellectual contribution to the work and approved it for publication.
Funding
This work was supported in part by the European Research Council (ERC) advanced grant no. 695714 IMMUNOALZHEIMER and the ERC Proof of Concept grant nr. 101069397 NeutrAD; The National Recovery and Resilience Plan (PNRR) National Centers Program (National Biodiversity Future Center – NBFC), PNRR “Partenariati estesi” Program, grant MNESYS (PE0000006) (to GC).
Conflict of interest
The authors declare that the research was conducted without any commercial or financial relationships that could be construed as a potential conflict of interest.
Publisher’s note
All claims expressed in this article are solely those of the authors and do not necessarily represent those of their affiliated organizations, or those of the publisher, the editors and the reviewers. Any product that may be evaluated in this article, or claim that may be made by its manufacturer, is not guaranteed or endorsed by the publisher.
References
1. Castellani G, Croese T, Peralta Ramos JM, Schwartz M. Transforming the understanding of brain immunity. Science (2023) 380:eabo7649. doi: 10.1126/science.abo7649
2. Zattoni M, Mura ML, Deprez F, Schwendener RA, Engelhardt B, Frei K, et al. Brain infiltration of leukocytes contributes to the pathophysiology of temporal lobe epilepsy. J Neurosci (2011) 31:4037–50. doi: 10.1523/JNEUROSCI.6210-10.2011
3. Zenaro E, Pietronigro E, Della Bianca V, Piacentino G, Marongiu L, Budui S, et al. Neutrophils promote Alzheimer's disease-like pathology and cognitive decline via lfa-1 integrin. Nat Med (2015) 21:880–6. doi: 10.1038/nm.3913
4. Negi N, Das BK. Cns: not an immunoprivilaged site anymore but A virtual secondary lymphoid organ. Int Rev Immunol (2018) 37:57–68. doi: 10.1080/08830185.2017.1357719
5. Galiano-Landeira J, Torra A, Vila M, Bove J. Cd8 T cell nigral infiltration precedes synucleinopathy in early stages of Parkinson's disease. Brain (2020) 143:3717–33. doi: 10.1093/brain/awaa269
6. Gate D, Saligrama N, Leventhal O, Yang AC, Unger MS, Middeldorp J, et al. Clonally expanded cd8 T cells patrol the cerebrospinal fluid in Alzheimer's disease. Nature (2020) 577:399–404. doi: 10.1038/s41586-019-1895-7
7. Urban SL, Jensen IJ, Shan Q, Pewe LL, Xue HH, Badovinac VP, et al. Peripherally induced brain tissue-resident memory cd8(+) T cells mediate protection against cns infection. Nat Immunol (2020) 21:938–49. doi: 10.1038/s41590-020-0711-8
8. Togo T, Akiyama H, Iseki E, Kondo H, Ikeda K, Kato M, et al. Occurrence of T cells in the brain of Alzheimer’s disease and other neurological diseases. J Of Neuroimmunol (2002) 124:83–92. doi: 10.1016/S0165-5728(01)00496-9
9. Hardy TA, O'brien B, Gerbis N, Barnett MH, Reddel SW, Brewer J, et al. Brain histopathology in three cases of susac's syndrome: implications for lesion pathogenesis and treatment. J Neurol Neurosurg Psychiatry (2015) 86:582–4. doi: 10.1136/jnnp-2014-308240
10. Ferretti MT, Merlini M, Spani C, Gericke C, Schweizer N, Enzmann G, et al. T-cell brain infiltration and immature antigen-presenting cells in transgenic models of Alzheimer's disease-like cerebral amyloidosis. Brain Behav Immun (2016) 54:211–25. doi: 10.1016/j.bbi.2016.02.009
11. Ritzel RM, Crapser J, Patel AR, Verma R, Grenier JM, Chauhan A, et al. Age-associated resident memory cd8 T cells in the central nervous system are primed to potentiate inflammation after ischemic brain injury. J Immunol (2016) 196:3318–30. doi: 10.4049/jimmunol.1502021
12. Van Nierop GP, Van Luijn MM, Michels SS, Melief MJ, Janssen M, Langerak AW, et al. Phenotypic and functional characterization of T cells in white matter lesions of multiple sclerosis patients. Acta Neuropathol (2017) 134:383–401. doi: 10.1007/s00401-017-1744-4
13. Gross CC, Meyer C, Bhatia U, Yshii L, Kleffner I, Bauer J, et al. Cd8(+) T cell-mediated endotheliopathy is a targetable mechanism of neuro-inflammation in susac syndrome. Nat Commun (2019) 10:5779. doi: 10.1038/s41467-019-13593-5
14. Fransen NL, Hsiao CC, van der Poel M, Engelenburg HJ, Verdaasdonk K, Vincenten MCJ, et al. Tissue-resident memory T cells invade the brain parenchyma in multiple sclerosis white matter lesions. Brain (2020) 143:1714–30. doi: 10.1093/brain/awaa117
15. Unger MS, Li E, Scharnagl L, Poupardin R, Altendorfer B, Mrowetz H, et al. Cd8(+) T-cells infiltrate Alzheimer's disease brains and regulate neuronal- and synapse-related gene expression in app-ps1 transgenic mice. Brain Behav Immun (2020) 89:67–86. doi: 10.1016/j.bbi.2020.05.070
16. Pitsch J, Van Loo KMJ, Gallus M, Dik A, Kamalizade D, Baumgart AK, et al. Cd8(+) T-lymphocyte-driven limbic encephalitis results in temporal lobe epilepsy. Ann Neurol (2021) 89:666–85. doi: 10.1002/ana.26000
17. Altendorfer B, Unger MS, Poupardin R, Hoog A, Asslaber D, Gratz IK, et al. Transcriptomic profiling identifies cd8(+) T cells in the brain of aged and Alzheimer's disease transgenic mice as tissue-resident memory T cells. J Immunol (2022) 209:1272–85. doi: 10.4049/jimmunol.2100737
18. Campisi L, Chizari S, Ho JSY, Gromova A, Arnold FJ, Mosca L, et al. Clonally expanded cd8 T cells characterize amyotrophic lateral sclerosis-4. Nature (2022) 606:945–52. doi: 10.1038/s41586-022-04844-5
19. Koetzier SC, Van Langelaar J, Melief MJ, Wierenga-Wolf AF, Corsten CEA, Blok KM, et al. Distinct effector programs of brain-homing cd8(+) T cells in multiple sclerosis. Cells (2022) 11. doi: 10.3390/cells11101634
20. Groh J, Knopper K, Arampatzi P, Yuan X, Losslein L, Saliba AE, et al. Accumulation of cytotoxic T cells in the aged cns leads to axon degeneration and contributes to cognitive and motor decline. Nat Aging (2021) 1:357–67. doi: 10.1038/s43587-021-00049-z
21. Crowl JT, Heeg M, Ferry A, Milner JJ, Omilusik KD, Toma C, et al. Tissue-resident memory cd8(+) T cells possess unique transcriptional, epigenetic and functional adaptations to different tissue environments. Nat Immunol (2022) 23:1121–31. doi: 10.1038/s41590-022-01229-8
22. Smolders J, Heutinck KM, Fransen NL, Remmerswaal EBM, Hombrink P, Ten Berge IJM, et al. Tissue-resident memory T cells populate the human brain. Nat Commun (2018) 9:4593. doi: 10.1038/s41467-018-07053-9
23. Mogilenko DA, Shpynov O, Andhey PS, Arthur L, Swain A, Esaulova E, et al. Comprehensive profiling of an aging immune system reveals clonal gzmk(+) cd8(+) T cells as conserved hallmark of inflammaging. Immunity (2021) 54:99–115.e12. doi: 10.1016/j.immuni.2020.11.005
24. Giles JR, Ngiow SF, Manne S, Baxter AE, Khan O, Wang P, et al. Shared and distinct biological circuits in effector, memory and exhausted cd8(+) T cells revealed by temporal single-cell transcriptomics and epigenetics. Nat Immunol (2022) 23:1600–13. doi: 10.1038/s41590-022-01338-4
25. Aydin S, Pareja J, Schallenberg VM, Klopstein A, Gruber T, Page N, et al. Antigen recognition detains cd8(+) T cells at the blood-brain barrier and contributes to its breakdown. Nat Commun (2023) 14:3106. doi: 10.1038/s41467-023-38703-2
26. Kumar BV, Connors TJ, Farber DL. Human T cell development, localization, and function throughout life. Immunity (2018) 48:202–13. doi: 10.1016/j.immuni.2018.01.007
27. Cui L, Hou NN, Wu HM, Zuo X, Lian YZ, Zhang CN, et al. Prevalence of Alzheimer's disease and Parkinson's disease in China: an updated systematical analysis. Front Aging Neurosci (2020) 12:603854. doi: 10.3389/fnagi.2020.603854
28. Lu J, Ahmad R, Nguyen T, Cifello J, Hemani H, Li J, et al. Heterogeneity and transcriptome changes of human cd8(+) T cells across nine decades of life. Nat Commun (2022) 13:5128. doi: 10.1038/s41467-022-32869-x
29. Van Stipdonk MJ, Lemmens EE, Schoenberger SP. Naïve ctls require A single brief period of antigenic stimulation for clonal expansion and differentiation. Nat Immunol (2001) 2(5):423–9. doi: 10.1038/87730
30. Diao H, Pipkin M. Stability and flexibility in chromatin structure and transcription underlies memory cd8 T-cell differentiation. F1000res (2019) 8. doi: 10.12688/f1000research.18211.1
31. Kaech S, Ahmed R. Memory cd8+ T cell differentiation: initial antigen encounter triggers A developmental program in naïve cells. Nat Immunol (2001) 2(5):415–22. doi: 10.1038/87720
32. Sallusto F. Two subsets of memory T lymphocytes with distinct homing potentials and effector functions. Nature (1999) 401:708–12. doi: 10.1038/44385
33. Hosking MP, Flynn CT, Whitton JL. Antigen-specific naive cd8+ T cells produce A single pulse of ifn-gamma in vivo within hours of infection, but without antiviral effect. J Immunol (2014) 193:1873–85. doi: 10.4049/jimmunol.1400348
34. Cho BK, Lian KC, Lee P, Brunmark A, Mckinley C, Chen J, et al. Differences in antigen recognition and cytolytic activity of cd8(+) and cd8(-) T cells that express the same antigen-specific receptor. Proc Natl Acad Sci USA (2001) 98:1723–7. doi: 10.1073/pnas.98.4.1723
35. Shrestha B, Diamond MS. Fas ligand interactions contribute to cd8+ T-cell-mediated control of west nile virus infection in the central nervous system. J Virol (2007) 81:11749–57. doi: 10.1128/JVI.01136-07
36. Bhat P, Leggatt G, Waterhouse N, Frazer IH. Interferon-gamma derived from cytotoxic lymphocytes directly enhances their motility and cytotoxicity. Cell Death Dis (2017) 8:E2836. doi: 10.1038/cddis.2017.67
37. Herndler-Brandstetter D, Ishigame H, Shinnakasu R, Plajer V, Stecher C, Zhao J, et al. Klrg1(+) effector cd8(+) T cells lose klrg1, differentiate into all memory T cell lineages, and convey enhanced protective immunity. Immunity (2018) 48:716–729.e8. doi: 10.1016/j.immuni.2018.03.015
38. Plumlee CR, Sheridan BS, Cicek BB, Lefrancois L. Environmental cues dictate the fate of individual cd8+ T cells responding to infection. Immunity (2013) 39:347–56. doi: 10.1016/j.immuni.2013.07.014
39. Arsenio J, Kakaradov B, Metz PJ, Kim SH, Yeo GW, Chang JT. Early specification of cd8+ T lymphocyte fates during adaptive immunity revealed by single-cell gene-expression analyses. Nat Immunol (2014) 15:365–72. doi: 10.1038/ni.2842
40. Picker LJ, Treer JR, Ferguson-Darnell B, Collins PA, Bergstresser PR, Terstappen LW. Control of lymphocyte recirculation in man. Ii. Differential regulation of the cutaneous lymphocyte-associated antigen, A tissue-selective homing receptor for skin-homing T cells. J Immunol (1993) 150:1122–36. doi: 10.4049/jimmunol.150.3.1122
41. Buchholz VR, Flossdorf M, Hensel I, Kretschmer L, Weissbrich B, Gräf P, et al. Disparate individual fates compose robust cd8+ T cell immunity. Science (2013) 340:630–5. doi: 10.1126/science.1235454
42. Buck MD, O'sullivan D, Pearce EL. T cell metabolism drives immunity. J Exp Med (2015) 212:1345–60. doi: 10.1084/jem.20151159
43. O'sullivan D. The metabolic spectrum of memory T cells. Immunol Cell Biol (2019) 97:636–46. doi: 10.1111/imcb.12274
44. Obar JJ, Lefrancois L. Early signals during cd8 T cell priming regulate the generation of central memory cells. J Immunol (2010) 185:263–72. doi: 10.4049/jimmunol.1000492
45. Olson JA, Mcdonald-Hyman C, Jameson SC, Hamilton SE. Effector-like cd8(+) T cells in the memory population mediate potent protective immunity. Immunity (2013) 38:1250–60. doi: 10.1016/j.immuni.2013.05.009
46. Joffre OP, Segura E, Savina A, Amigorena S. Cross-presentation by dendritic cells. Nat Rev Immunol (2012) 12:557–69. doi: 10.1038/nri3254
47. Macnabb BW, Chen X, Tumuluru S, Godfrey J, Kasal DN, Yu J, et al. Dendritic cells can prime anti-tumor cd8(+) T cell responses through major histocompatibility complex cross-dressing. Immunity (2022) 55:2206–8. doi: 10.1016/j.immuni.2022.09.015
48. Lugli E, Galletti G, Boi SK, Youngblood BA. Stem, effector, and hybrid states of memory cd8(+) T cells. Trends Immunol (2020) 41:17–28. doi: 10.1016/j.it.2019.11.004
49. Andreatta M, Corria-Osorio J, Muller S, Cubas R, Coukos G, Carmona SJ. Interpretation of T cell states from single-cell transcriptomics data using reference atlases. Nat Commun (2021) 12:2965. doi: 10.1038/s41467-021-23324-4
50. Suzuki I, Martin S, Boursalian TE, Beers C, Fink PJ. Fas ligand costimulates the in vivo proliferation of cd8+ T cells. J Immunol (2000) 165:5537–43. doi: 10.4049/jimmunol.165.10.5537
51. Gupta S, Gollapudi S. Cd95-mediated apoptosis in naive, central and effector memory subsets of cd4+ and cd8+ T cells in aged humans. Exp Gerontol (2008) 43:266–74. doi: 10.1016/j.exger.2007.12.006
52. Soares A, Govender L, Hughes J, Mavakla W, De Kock M, Barnard C, et al. Novel application of ki67 to quantify antigen-specific in vitro lymphoproliferation. J Immunol Methods (2010) 362:43–50. doi: 10.1016/j.jim.2010.08.007
53. Takata H, Takiguchi M. Three memory subsets of human cd8+ T cells differently expressing three cytolytic effector molecules. J Immunol (2006) 177:4330–40. doi: 10.4049/jimmunol.177.7.4330
54. De Araujo-Souza PS, Hanschke SCH, Nardy A, Secca C, Oliveira-Vieira B, Silva KL, et al. Differential interferon-gamma production by naive and memory-like cd8 T cells. J Leukoc Biol (2020) 108:1329–37. doi: 10.1002/JLB.2AB0420-646R
55. Martin MD, Badovinac VP. Defining memory cd8 T cell. Front Immunol (2018) 9:2692. doi: 10.3389/fimmu.2018.02692
56. Parga-Vidal L, Van Gisbergen K. Area under immunosurveillance: dedicated roles of memory cd8 T-cell subsets. Cold Spring Harb Perspect Biol (2020) 12. doi: 10.1101/cshperspect.a037796
57. Van Der Gracht ETI, Beyrend G, Abdelaal T, Pardieck IN, Wesselink TH, Van Haften FJ, et al. Memory cd8(+) T cell heterogeneity is primarily driven by pathogen-specific cues and additionally shaped by the tissue environment. Iscience (2021) 24:101954. doi: 10.1016/j.isci.2020.101954
58. Gerlach C, Moseman EA, Loughhead SM, Alvarez D, Zwijnenburg AJ, Waanders L, et al. The chemokine receptor cx3cr1 defines three antigen-experienced cd8 T cell subsets with distinct roles in immune surveillance and homeostasis. Immunity (2016) 45:1270–84. doi: 10.1016/j.immuni.2016.10.018
59. Chen Y, Shen J, Kasmani MY, Topchyan P, Cui W. Single-cell transcriptomics reveals core regulatory programs that determine the heterogeneity of circulating and tissue-resident memory cd8(+) T cells. Cells (2021) 10. doi: 10.3390/cells10082143
60. Thome JJ, Yudanin N, Ohmura Y, Kubota M, Grinshpun B, Sathaliyawala T, et al. Spatial map of human T cell compartmentalization and maintenance over decades of life. Cell (2014) 159:814–28. doi: 10.1016/j.cell.2014.10.026
61. Gattinoni L, Lugli E, Ji Y, Pos Z, Paulos CM, Quigley MF, et al. A human memory T cell subset with stem cell-like properties. Nat Med (2011) 17:1290–7. doi: 10.1038/nm.2446
62. Nolz JC, Starbeck-Miller GR, Harty JT. Naive, effector and memory cd8 T-cell trafficking: parallels and distinctions. Immunotherapy (2011) 3:1223–33. doi: 10.2217/imt.11.100
63. Wakim LM, Woodward-Davis A, Liu R, Hu Y, Villadangos J, Smyth G, et al. The molecular signature of tissue resident memory cd8 T cells isolated from the brain. J Immunol (2012) 189:3462–71. doi: 10.4049/jimmunol.1201305
64. Takamura S. Niches for the long-term maintenance of tissue-resident memory T cells. Front Immunol (2018) 9:1214. doi: 10.3389/fimmu.2018.01214
65. Mackay LK, Rahimpour A, Ma JZ, Collins N, Stock AT, Hafon ML, et al. The developmental pathway for cd103(+)Cd8+ Tissue-resident memory T cells of skin. Nat Immunol (2013) 14:1294–301. doi: 10.1038/ni.2744
66. Adachi T, Kobayashi T, Sugihara E, Yamada T, Ikuta K, Pittaluga S, et al. Hair follicle-derived il-7 and il-15 mediate skin-resident memory T cell homeostasis and lymphoma. Nat Med (2015) 21:1272–9. doi: 10.1038/nm.3962
67. Ren HM, Kolawole EM, Ren M, Jin G, Netherby-Winslow CS, Wade Q, et al. Il-21 from high-affinity cd4 T cells drives differentiation of brain-resident cd8 T cells during persistent viral infection. Sci Immunol (2020) 5. doi: 10.1126/sciimmunol.abb5590
68. Marx AF, Kallert SM, Brunner TM, Villegas JA, Geier F, Fixemer J, et al. The alarmin interleukin-33 promotes the expansion and preserves the stemness of tcf-1(+) cd8(+) T cells in chronic viral infection. Immunity (2023) 56:813–828.e10. doi: 10.1016/j.immuni.2023.01.029
69. Kutlesa S, Wessels JT, Speiser A, Steiert I, Muller CA, Klein G. E-cadherin-mediated interactions of thymic epithelial cells with cd103+ Thymocytes lead to enhanced thymocyte cell proliferation. J Cell Sci (2002) 115:4505–15. doi: 10.1242/jcs.00142
70. Hofmann M, Pircher H. E-cadherin promotes accumulation of A unique memory cd8 T-cell population in murine salivary glands. Proc Natl Acad Sci USA (2011) 108:16741–6. doi: 10.1073/pnas.1107200108
71. Walsh DA, Borges Da Silva H, Beura LK, Peng C, Hamilton SE, Masopust D, et al. The functional requirement for cd69 in establishment of resident memory cd8(+) T cells varies with tissue location. J Immunol (2019) 203:946–55. doi: 10.4049/jimmunol.1900052
72. Wu T, Hu Y, Lee YT, Bouchard KR, Benechet A, Khanna K, et al. Lung-resident memory cd8 T cells (Trm) are indispensable for optimal cross-protection against pulmonary virus infection. J Leukoc Biol (2014) 95:215–24. doi: 10.1189/jlb.0313180
73. Steinbach K, Vincenti I, Kreutzfeldt M, Page N, Muschaweckh A, Wagner I, et al. Brain-resident memory T cells represent an autonomous cytotoxic barrier to viral infection. J Exp Med (2016) 213:1571–87. doi: 10.1084/jem.20151916
74. Mcnamara HA, Cai Y, Wagle MV, Sontani Y, Roots CM, Miosge LA, et al. Up-regulation of lfa-1 allows liver-resident memory T cells to patrol and remain in the hepatic sinusoids. Sci Immunol (2017) 2. doi: 10.1126/sciimmunol.aaj1996
75. Bottois H, Ngollo M, Hammoudi N, Courau T, Bonnereau J, Chardiny V, et al. Klrg1 and cd103 expressions define distinct intestinal tissue-resident memory cd8 T cell subsets modulated in crohn's disease. Front Immunol (2020) 11:896. doi: 10.3389/fimmu.2020.00896
76. Fitzpatrick MEB, Provine NM, Garner LC, Powell K, Amini A, Irwin SL, et al. Human intestinal tissue-resident memory T cells comprise transcriptionally and functionally distinct subsets. Cell Rep (2021) 34:108661. doi: 10.1016/j.celrep.2020.108661
77. Poon MML, Caron DP, Wang Z, Wells SB, Chen D, Meng W, et al. Tissue adaptation and clonal segregation of human memory T cells in barrier sites. Nat Immunol (2023) 24:309–19. doi: 10.1038/s41590-022-01395-9
79. Verdon DJ, Mulazzani M, Jenkins MR. Cellular and molecular mechanisms of cd8(+) T cell differentiation, dysfunction and exhaustion. Int J Mol Sci (2020) 21. doi: 10.3390/ijms21197357
80. Schietinger A, Philip M, Krisnawan VE, Chiu EY, Delrow JJ, Basom RS, et al. Tumor-specific T cell dysfunction is A dynamic antigen-driven differentiation program initiated early during tumorigenesis. Immunity (2016) 45:389–401. doi: 10.1016/j.immuni.2016.07.011
81. Tonnerre P, Wolski D, Subudhi S, Aljabban J, Hoogeveen RC, Damasio M, et al. Differentiation of exhausted cd8(+) T cells after termination of chronic antigen stimulation stops short of achieving functional T cell memory. Nat Immunol (2021) 22:1030–41. doi: 10.1038/s41590-021-00982-6
82. Rodriguez IJ, Lalinde Ruiz N, Llano Leon M, Martinez Enriquez L, Montilla Velasquez MDP, Ortiz Aguirre JP, et al. Immunosenescence study of T cells: A systematic review. Front Immunol (2020) 11:604591. doi: 10.3389/fimmu.2020.604591
83. Saleh R, Taha RZ, Toor SM, Sasidharan Nair V, Murshed K, Khawar M, et al. Expression of immune checkpoints and T cell exhaustion markers in early and advanced stages of colorectal cancer. Cancer Immunol Immunother (2020) 69:1989–99. doi: 10.1007/s00262-020-02593-w
84. Grebinoski S, Zhang Q, Cillo AR, Manne S, Xiao H, Brunazzi EA, et al. Autoreactive cd8(+) T cells are restrained by an exhaustion-like program that is maintained by lag3. Nat Immunol (2022) 23:868–77. doi: 10.1038/s41590-022-01210-5
85. Zhao Y, Liao P, Huang S, Deng T, Tan J, Huang Y, et al. Increased tox expression associates with exhausted T cells in patients with multiple myeloma. Exp Hematol Oncol (2022) 11:12. doi: 10.1186/s40164-022-00267-0
86. Xu W, Larbi A. Markers of T cell senescence in humans. Int J Mol Sci (2017) 18. doi: 10.3390/ijms18081742
87. Janelle V, Neault M, Lebel ME, De Sousa DM, Boulet S, Durrieu L, et al. P16(Ink4a) regulates cellular senescence in pd-1-expressing human T cells. Front Immunol (2021) 12:698565. doi: 10.3389/fimmu.2021.698565
88. Tedeschi V, Paldino G, Kunkl M, Paroli M, Sorrentino R, Tuosto L, et al. Cd8(+) T cell senescence: lights and shadows in viral infections, autoimmune disorders and cancer. Int J Mol Sci (2022) 23. doi: 10.3390/ijms23063374
89. Quinn KM, Fox A, Harland KL, Russ BE, Li J, Nguyen THO, et al. Age-related decline in primary cd8(+) T cell responses is associated with the development of senescence in virtual memory cd8(+) T cells. Cell Rep (2018) 23:3512–24. doi: 10.1016/j.celrep.2018.05.057
90. Zhao Y, Shao Q, Peng G. Exhaustion and senescence: two crucial dysfunctional states of T cells in the tumor microenvironment. Cell Mol Immunol (2020) 17:27–35. doi: 10.1038/s41423-019-0344-8
91. Lee SW, Choi HY, Lee GW, Kim T, Cho HJ, Oh IJ, et al. Cd8(+) tils in nsclc differentiate into temra via A bifurcated trajectory: deciphering immunogenicity of tumor antigens. J Immunother Cancer (2021) 9. doi: 10.1136/jitc-2021-002709
92. Callender LA, Carroll EC, Beal RWJ, Chambers ES, Nourshargh S, Akbar AN, et al. Human cd8(+) emra T cells display A senescence-associated secretory phenotype regulated by P38 mapk. Aging Cell (2018) 17. doi: 10.1111/acel.12675
93. Pereira BI, De Maeyer RPH, Covre LP, Nehar-Belaid D, Lanna A, Ward S, et al. Sestrins induce natural killer function in senescent-like cd8(+) T cells. Nat Immunol (2020) 21:684–94. doi: 10.1038/s41590-020-0643-3
94. Man K, Gabriel SS, Liao Y, Gloury R, Preston S, Henstridge DC, et al. Transcription factor irf4 promotes cd8(+) T cell exhaustion and limits the development of memory-like T cells during chronic infection. Immunity (2017) 47:1129–1141.e5. doi: 10.1016/j.immuni.2017.11.021
95. Mognol GP, Spreafico R, Wong V, Scott-Browne JP, Togher S, Hoffmann A, et al. Exhaustion-associated regulatory regions in cd8(+) tumor-infiltrating T cells. Proc Natl Acad Sci USA (2017) 114:E2776–85. doi: 10.1073/pnas.1620498114
96. Khan O, Giles JR, Mcdonald S, Manne S, Ngiow SF, Patel KP, et al. Tox transcriptionally and epigenetically programs cd8(+) T cell exhaustion. Nature (2019) 571:211–8. doi: 10.1038/s41586-019-1325-x
97. Im SJ, Ha SJ. Re-defining T-cell exhaustion: subset, function, and regulation. Immune Netw (2020) 20:e2. doi: 10.4110/in.2020.20.e2
98. Zhang Z, Chen L, Chen H, Zhao J, Li K, Sun J, et al. Pan-cancer landscape of T-cell exhaustion heterogeneity within the tumor microenvironment revealed A progressive roadmap of hierarchical dysfunction associated with prognosis and therapeutic efficacy. Ebiomedicine (2022) 83:104207. doi: 10.1016/j.ebiom.2022.104207
99. Yu YR, Imrichova H, Wang H, Chao T, Xiao Z, Gao M, et al. Disturbed mitochondrial dynamics in cd8(+) tils reinforce T cell exhaustion. Nat Immunol (2020) 21:1540–51. doi: 10.1038/s41590-020-0793-3
100. Voehringer D, Blaser C, Brawand P, Raulet DH, Hanke T, Pircher H. Viral infections induce abundant numbers of senescent cd8 T cells. J Immunol (2001) 167:4838–43. doi: 10.4049/jimmunol.167.9.4838
101. Brenchley JM, Karandikar NJ, Betts MR, Ambrozak DR, Hill BJ, Crotty LE, et al. Expression of cd57 defines replicative senescence and antigen-induced apoptotic death of cd8+ T cells. Blood (2003) 101:2711–20. doi: 10.1182/blood-2002-07-2103
102. Henson SM, Macaulay R, Riddell NE, Nunn CJ, Akbar AN. Blockade of pd-1 or P38 map kinase signaling enhances senescent human cd8(+) T-cell proliferation by distinct pathways. Eur J Immunol (2015) 45:1441–51. doi: 10.1002/eji.201445312
103. Wang TW, Johmura Y, Suzuki N, Omori S, Migita T, Yamaguchi K, et al. Blocking pd-L1-pd-1 improves senescence surveillance and ageing phenotypes. Nature (2022) 611:358–64. doi: 10.1038/s41586-022-05388-4
104. Peralbo E, Alonso C, Solana R. Invariant nkt and nkt-like lymphocytes: two different T cell subsets that are differentially affected by ageing. Exp Gerontol (2007) 42:703–8. doi: 10.1016/j.exger.2007.05.002
105. Crosby CM, Kronenberg M. Tissue-specific functions of invariant natural killer T cells. Nat Rev Immunol (2018) 18:559–74. doi: 10.1038/s41577-018-0034-2
106. Covre LP, De Maeyer RPH, Gomes DCO, Akbar AN. The role of senescent T cells in immunopathology. Aging Cell (2020) 19:e13272. doi: 10.1111/acel.13272
107. Sathaliyawala T, Kubota M, Yudanin N, Turner D, Camp P, Thome JJ, et al. Distribution and compartmentalization of human circulating and tissue-resident memory T cell subsets. Immunity (2013) 38:187–97. doi: 10.1016/j.immuni.2012.09.020
108. Thome JJ, Bickham KL, Ohmura Y, Kubota M, Matsuoka N, Gordon C, et al. Early-life compartmentalization of human T cell differentiation and regulatory function in mucosal and lymphoid tissues. Nat Med (2016) 22:72–7. doi: 10.1038/nm.4008
109. Li M, Yao D, Zeng X, Kasakovski D, Zhang Y, Chen S, et al. Age related human T cell subset evolution and senescence. Immun Ageing (2019) 16:24. doi: 10.1186/s12979-019-0165-8
110. Palmer DB. The effect of age on thymic function. Front Immunol (2013) 4:316. doi: 10.3389/fimmu.2013.00316
111. Thomas R, Wang W, Su DM. Contributions of age-related thymic involution to immunosenescence and inflammaging. Immun Ageing (2020) 17:2. doi: 10.1186/s12979-020-0173-8
112. Goronzy JJ, Weyand CM. Immune aging and autoimmunity. Cell Mol Life Sci (2012) 69:1615–23. doi: 10.1007/s00018-012-0970-0
113. Aiello A, Farzaneh F, Candore G, Caruso C, Davinelli S, Gambino CM, et al. Immunosenescence and its hallmarks: how to oppose aging strategically? A review of potential options for therapeutic intervention. Front Immunol (2019) 10:2247. doi: 10.3389/fimmu.2019.02247
114. Fessler J, Angiari S. The role of T cell senescence in neurological diseases and its regulation by cellular metabolism. Front Immunol (2021) 12:706434. doi: 10.3389/fimmu.2021.706434
115. Mogilenko DA, Shchukina I, Artyomov MN. Immune ageing at single-cell resolution. Nat Rev Immunol (2022) 22:484–98. doi: 10.1038/s41577-021-00646-4
116. Xia S, Zhang X, Zheng S, Khanabdali R, Kalionis B, Wu J, et al. An update on inflamm-aging: mechanisms, prevention, and treatment. J Immunol Res (2016) 2016:8426874. doi: 10.1155/2016/8426874
117. Dulken BW, Buckley MT, Navarro Negredo P, Saligrama N, Cayrol R, Leeman DS, et al. Single-cell analysis reveals T cell infiltration in old neurogenic niches. Nature (2019) 571:205–10. doi: 10.1038/s41586-019-1362-5
118. Diwan B, Sharma R. Nutritional components as mitigators of cellular senescence in organismal aging: A comprehensive review. Food Sci Biotechnol (2022) 31:1089–109. doi: 10.1007/s10068-022-01114-y
119. Donovan T, Bain AL, Tu W, Pyne DB, Rao S. Influence of exercise on exhausted and senescent T cells: A systematic review. Front Physiol (2021) 12:668327. doi: 10.3389/fphys.2021.668327
120. Tan EK, Chao YX, West A, Chan LL, Poewe W, Jankovic J. Parkinson disease and the immune system - associations, mechanisms and therapeutics. Nat Rev Neurol (2020) 16:303–18. doi: 10.1038/s41582-020-0344-4
121. Knopman DS, Amieva H, Petersen RC, Chetelat G, Holtzman DM, Hyman BT, et al. Alzheimer disease. Nat Rev Dis Primers (2021) 7:33. doi: 10.1038/s41572-021-00269-y
122. Gustavsson A, Norton N, Fast T, Frolich L, Georges J, Holzapfel D, et al. Global estimates on the number of persons across the Alzheimer's disease continuum. Alzheimers Dement (2023) 19:658–70. doi: 10.1002/alz.12694
123. Alzheimer’s Association. Alzheimer's Disease facts and figures 2022. Alzheimers Dement (2022) 18:700–89. doi: 10.1002/alz.12638
124. Panossian LA, Porter VR, Valenzuela HF, Zhu X, Reback E, Masterman D, et al. Telomere shortening in T cells correlates with Alzheimer’s disease status. Neurobiol Aging (2003) 24(1):77–84. doi: 10.1016/S0197-4580(02)00043-X
125. Jenkins EC, Velinov MT, Ye L, Gu H, Li S, Jenkins EC Jr., et al. Telomere shortening in T lymphocytes of older individuals with down syndrome and dementia. Neurobiol Aging (2006) 27:941–5. doi: 10.1016/j.neurobiolaging.2005.05.021
126. Laurent C, Dorothee G, Hunot S, Martin E, Monnet Y, Duchamp M, et al. Hippocampal T cell infiltration promotes neuroinflammation and cognitive decline in a mouse model of tauopathy. Brain (2017) 140:184–200. doi: 10.1093/brain/aww270
127. Merlini M, Kirabali T, Kulic L, Nitsch RM, Ferretti MT. Extravascular cd3+ T cells in brains of Alzheimer disease patients correlate with tau but not with amyloid pathology: an immunohistochemical study. Neurodegener Dis (2018) 18:49–56. doi: 10.1159/000486200
128. Gericke C, Kirabali T, Flury R, Mallone A, Rickenbach C, Kulic L, et al. Early β-amyloid accumulation in the brain is associated with peripheral T cell alterations. Alzheimer’s Dement (2023) 1–21. doi: 10.1002/alz.13136
129. Van Olst L, Coenen L, Nieuwland JM, Rodriguez-Mogeda C, De Wit NM, Kamermans A, et al. Crossing borders in Alzheimer's disease: A T cell's perspective. Adv Drug Delivery Rev (2022) 188:114398. doi: 10.1016/j.addr.2022.114398
130. Zenaro E, Piacentino G, Constantin G. The blood-brain barrier in Alzheimer's disease. Neurobiol Dis (2017) 107:41–56. doi: 10.1016/j.nbd.2016.07.007
131. Bloem BR, Okun MS, Klein C. Parkinson's disease. Lancet (2021) 397:2284–303. doi: 10.1016/S0140-6736(21)00218-X
132. Kalia LV, Lang AE. Parkinson's disease. Lancet (2015) 386:896–912. doi: 10.1016/S0140-6736(14)61393-3
133. Wang P, Yao L, Luo M, Zhou W, Jin X, Xu Z, et al. Single-cell transcriptome and tcr profiling reveal activated and expanded T cell populations in Parkinson's disease. Cell Discovery (2021) 7:52. doi: 10.1038/s41421-021-00280-3
134. Lindestam Arlehamn CS, Dhanwani R, Pham J, Kuan R, Frazier A, Rezende Dutra J, et al. Alpha-synuclein-specific T cell reactivity is associated with preclinical and early Parkinson's disease. Nat Commun (2020) 11:1875. doi: 10.1038/s41467-020-15626-w
135. Williams GP, Muskat K, Frazier A, Xu Y, Mateus J, Grifoni A, et al. Unaltered T cell responses to common antigens in individuals with Parkinson's disease. J Neurol Sci (2023) 444:120510. doi: 10.1016/j.jns.2022.120510
136. Dhanwani R, Lima-Junior JR, Sethi A, Pham J, Williams G, Frazier A, et al. Transcriptional analysis of peripheral memory T cells reveals Parkinson's disease-specific gene signatures. NPJ Parkinsons Dis (2022) 8:30. doi: 10.1038/s41531-022-00282-2
137. Cook DA, Kannarkat GT, Cintron AF, Butkovich LM, Fraser KB, Chang J, et al. Lrrk2 levels in immune cells are increased in Parkinson's disease. NPJ Parkinsons Dis (2017) 3:11. doi: 10.1038/s41531-017-0010-8
138. Al-Bachari S, Naish JH, Parker GJM, Emsley HCA, Parkes LM. Blood-brain barrier leakage is increased in Parkinson's disease. Front Physiol (2020) 11:593026. doi: 10.3389/fphys.2020.593026
139. Ruan Z, Zhang D, Huang R, Sun W, Hou L, Zhao J, et al. Microglial activation damages dopaminergic neurons through mmp-2/-9-mediated increase of blood-brain barrier permeability in A Parkinson's disease mouse model. Int J Mol Sci (2022) 23. doi: 10.3390/ijms23052793
140. Walton C, King R, Rechtman L, Kaye W, Leray E, Marrie RA, et al. Rising prevalence of multiple sclerosis worldwide: insights from the atlas of ms, third edition. Multiple Sclerosis J (2020) 26(14):1816–21. doi: 10.1177/1352458520970841
141. Thompson AJ, Baranzini SE, Geurts J, Hemmer B, Ciccarelli O. Multiple sclerosis. Lancet (2018) 391:1622–36. doi: 10.1016/S0140-6736(18)30481-1
142. Babbe H, Roers H, Waisman A, Lassmann H, Goebels N, Hohlfeld R, et al. Clonal expansions of cd81 T cells dominate the T cell infiltrate in active multiple sclerosis lesions as shown by micromanipulation and single cell polymerase chain reaction. J Of Experimenral Med (2000) 92(3):393–404. doi: 10.1084/jem.192.3.393
143. Ifergan I, Kebir H, Alvarez JI, Marceau G, Bernard M, Bourbonniere L, et al. Central nervous system recruitment of effector memory cd8+ T lymphocytes during neuroinflammation is dependent on alpha4 integrin. Brain (2011) 134:3560–77. doi: 10.1093/brain/awr268
144. Salou M, Garcia A, Michel L, Gainche-Salmon A, Loussouarn D, Nicol B, et al. Expanded cd8 T-cell sharing between periphery and cns in multiple sclerosis. Ann Clin Transl Neurol (2015) 2:609–22. doi: 10.1002/acn3.199
145. Skulina C, Schmidt S, Dornmair K, Babbe. H, Roers A, Rajewsky K, et al. Multiple sclerosis: brain-infiltrating cd8 T cells persist as clonal expansions in the cerebrospinal fluid and blood. Pnas (2004) 101(8):2428–33. doi: 10.1073/pnas.0308689100
146. Zeitelhofer M, Adzemovic MZ, Moessinger C, Stefanitsch C, Strell C, Muhl L, et al. Blocking pdgf-cc signaling ameliorates multiple sclerosis-like neuroinflammation by inhibiting disruption of the blood-brain barrier. Sci Rep (2020) 10:22383. doi: 10.1038/s41598-020-79598-z
147. Battistini L, Piccio L, Rossi B, Bach S, Galgani S, Gasperini C, et al. Cd8+ T cells from patients with acute multiple sclerosis display selective increase of adhesiveness in brain venules: A critical role for P-selectin glycoprotein ligand-1. Blood (2003) 101:4775–82. doi: 10.1182/blood-2002-10-3309
148. Schwab N, Schneider-Hohendorf T, Wiendl H. Therapeutic uses of anti-alpha4-integrin (Anti-vla-4) antibodies in multiple sclerosis. Int Immunol (2015) 27:47–53. doi: 10.1093/intimm/dxu096
149. Smolders J, Hamann J. Programmed cell death protein 1-positive cd8(+) T cells in multiple sclerosis: exhausted fighters or peacekeepers. Neurol Neuroimmunol Neuroinflamm (2022) 9. doi: 10.1212/NXI.0000000000001173
150. Taylor GS, Long HM, Brooks JM, Rickinson AB, Hislop AD. The immunology of epstein-barr virus-induced disease. Annu Rev Immunol (2015) 33:787–821. doi: 10.1146/annurev-immunol-032414-112326
151. Veroni C, Aloisi F. The cd8 T cell-epstein-barr virus-B cell trialogue: A central issue in multiple sclerosis pathogenesis. Front Immunol (2021) 12:665718. doi: 10.3389/fimmu.2021.665718
152. Feldman EL, Goutman SA, Petri S, Mazzini L, Savelieff MG, Shaw PJ, et al. Amyotrophic lateral sclerosis. Lancet (2022) 400:1363–80. doi: 10.1016/S0140-6736(22)01272-7
153. Rosen DR, Siddique T, Patterson D, Figlewicz DA, Sapp P, Hentati A, et al. Mutations in cu/zn superoxide dismutase gene are associated with familial amyotrophic lateral sclerosis. Nature (1993) 362:59–62. doi: 10.1038/362059a0
154. Wicks P, Abrahams S, Papps B, Al-Chalabi A, Shaw CE, Leigh PN, et al. Sod1 and cognitive dysfunction in familial amyotrophic lateral sclerosis. J Neurol (2009) 256:234–41. doi: 10.1007/s00415-009-0078-0
155. Al-Chalabi A, Jones A, Troakes C, King A, Al-Sarraj S, Van Den Berg LH. The genetics and neuropathology of amyotrophic lateral sclerosis. Acta Neuropathol (2012) 124:339–52. doi: 10.1007/s00401-012-1022-4
156. Tokuda E, Furukawa Y. Copper homeostasis as A therapeutic target in amyotrophic lateral sclerosis with sod1 mutations. Int J Mol Sci (2016) 17. doi: 10.3390/ijms17050636
157. Blasco H, Garcon G, Patin F, Veyrat-Durebex C, Boyer J, Devos D, et al. Panel of oxidative stress and inflammatory biomarkers in als: A pilot study. Can J Neurol Sci (2017) 44:90–5. doi: 10.1017/cjn.2016.284
158. Xiong L, Mccoy M, Komuro H, West XZ, Yakubenko V, Gao D, et al. Inflammation-dependent oxidative stress metabolites as A hallmark of amyotrophic lateral sclerosis. Free Radic Biol Med (2022) 178:125–33. doi: 10.1016/j.freeradbiomed.2021.11.031
159. Coque E, Salsac C, Espinosa-Carrasco G, Varga B, Degauque N, Cadoux M, et al. Cytotoxic cd8(+) T lymphocytes expressing als-causing sod1 mutant selectively trigger death of spinal motoneurons. Proc Natl Acad Sci USA (2019) 116:2312–7. doi: 10.1073/pnas.1815961116
160. Rolfes L, Schulte-Mecklenbeck A, Schreiber S, Vielhaber S, Herty M, Marten A, et al. Amyotrophic lateral sclerosis patients show increased peripheral and intrathecal T-cell activation. Brain Commun (2021) 3:Fcab157. doi: 10.1093/braincomms/fcab157
161. Garofalo S, Cocozza G, Bernardini G, Savage J, Raspa M, Aronica E, et al. Blocking immune cell infiltration of the central nervous system to tame neuroinflammation in amyotrophic lateral sclerosis. Brain Behav Immun (2022) 105:1–14. doi: 10.1016/j.bbi.2022.06.004
162. Yildiz O, Schroth J, Tree T, Turner MR, Shaw PJ, Henson SM, et al. Senescent-like blood lymphocytes and disease progression in amyotrophic lateral sclerosis. Neurol Neuroimmunol Neuroinflamm (2023) 10. doi: 10.1212/NXI.0000000000200042
163. Prell T, Vlad B, Gaur N, Stubendorff B, Grosskreutz J. Blood-brain barrier disruption is not associated with disease aggressiveness in amyotrophic lateral sclerosis. Front Neurosci (2021) 15:656456. doi: 10.3389/fnins.2021.656456
164. Cao MC, Cawston EE, Chen G, Brooks C, Douwes J, Mclean D, et al. Serum biomarkers of neuroinflammation and blood-brain barrier leakage in amyotrophic lateral sclerosis. BMC Neurol (2022) 22:216. doi: 10.1186/s12883-022-02730-1
165. Garofalo S, Cocozza G, Porzia A, Inghilleri M, Raspa M, Scavizzi F, et al. Natural killer cells modulate motor neuron-immune cell cross talk in models of amyotrophic lateral sclerosis. Nat Commun (2020) 11:1773. doi: 10.1038/s41467-020-15644-8
166. Bien CG, Elger CE. Limbic encephalitis: A cause of temporal lobe epilepsy with onset in adult life. Epilepsy Behav (2007) 10:529–38. doi: 10.1016/j.yebeh.2007.03.011
167. Graus F, Titulaer MJ, Balu R, Benseler S, Bien CG, Cellucci T, et al. A clinical approach to diagnosis of autoimmune encephalitis. Lancet Neurol (2016) 15:391–404. doi: 10.1016/S1474-4422(15)00401-9
168. Michael BD, Griffiths MJ, Granerod J, Brown D, Davies NW, Borrow R, et al. Characteristic cytokine and chemokine profiles in encephalitis of infectious, immune-mediated, and unknown aetiology. PloS One (2016) 11:e0146288. doi: 10.1371/journal.pone.0146288
169. Fabene PF, Navarro Mora G, Martinello M, Rossi B, Merigo F, Ottoboni L, et al. A role for leukocyte-endothelial adhesion mechanisms in epilepsy. Nat Med (2008) 14:1377–83. doi: 10.1038/nm.1878
170. Fabene PF, Bramanti P, Constantin G. The emerging role for chemokines in epilepsy. J Neuroimmunol (2010) 224:22–7. doi: 10.1016/j.jneuroim.2010.05.016
171. Bertini G, Bramanti P, Constantin G, Pellitteri M, Radu BM, Radu M, et al. New players in the neurovascular unit: insights from experimental and clinical epilepsy. Neurochem Int (2013) 63:652–9. doi: 10.1016/j.neuint.2013.08.001
172. Fabene PF, Laudanna C, Constantin G. Leukocyte trafficking mechanisms in epilepsy. Mol Immunol (2013) 55:100–4. doi: 10.1016/j.molimm.2012.12.009
173. Budhram A, Leung A, Nicolle MW, Burneo JG. Diagnosing autoimmune limbic encephalitis. Cmaj (2019) 191:E529–34. doi: 10.1503/cmaj.181548
174. Ding JB, Dongas J, Hu K, Ding M. Autoimmune limbic encephalitis: A review of clinicoradiological features and the challenges of diagnosis. Cureus (2021) 13:e17529. doi: 10.7759/cureus.17529
175. Ismail FS, Spatola M, Woermann FG, Popkirov S, Jungilligens J, Bien CG, et al. Diagnostic challenges in patients with temporal lobe seizures and features of autoimmune limbic encephalitis. Eur J Neurol (2022) 29:1303–10. doi: 10.1111/ene.15026
176. Lancaster E, Martinez- Hernandez E, Dalmau J. Encephalitis and antibodies to synaptic and neuronal cell surface proteins. Neurology (2011) 77:179–89. doi: 10.1212/WNL.0b013e318224afde
177. Lancaster E, Dalmau J. Neuronal autoantigens–pathogenesis, associated disorders and antibody testing. Nat Rev Neurol (2012) 8:380–90. doi: 10.1038/nrneurol.2012.99
178. Ng AS, Kramer J, Centurion A, Dalmau J, Huang E, Cotter JA, et al. Clinico-pathological correlation in adenylate kinase 5 autoimmune limbic encephalitis. J Neuroimmunol (2015) 287:31–5. doi: 10.1016/j.jneuroim.2015.08.009
179. Lancaster E. The diagnosis and treatment of autoimmune encephalitis. J Clin Neurol (2016) 12:1–13. doi: 10.3988/jcn.2016.12.1.1
180. Do L, Chanson E, Desestret V, Joubert B, Ducray F, Yohann Couté SB, et al. Characteristics in limbic encephalitis with anti–adenylate kinase 5 autoantibodies. Am Acad Of Neurol (2017) 88(6):514–24. doi: 10.1212/WNL.0000000000003586
181. Meuth SG, Herrmann AM, Simon OJ, Siffrin V, Melzer N, Bittner S, et al. Cytotoxic cd8+ T cell-neuron interactions: perforin-dependent electrical silencing precedes but is not causally linked to neuronal cell death. J Neurosci (2009) 29:15397–409. doi: 10.1523/JNEUROSCI.4339-09.2009
182. Ehling P, Melzer N, Budde T, Meuth SG. Cd8(+) T cell-mediated neuronal dysfunction and degeneration in limbic encephalitis. Front Neurol (2015) 6:163. doi: 10.3389/fneur.2015.00163
183. Bauer J, Bien CG. Neuropathology of autoimmune encephalitides. Handb Clin Neurol (2016) 133:107–20. doi: 10.1016/B978-0-444-63432-0.00007-4
184. Golombeck KS, Bonte K, Monig C, Van Loo KM, Hartwig M, Schwindt W, et al. Evidence of A pathogenic role for cd8(+) T cells in anti-gabab receptor limbic encephalitis. Neurol Neuroimmunol Neuroinflamm (2016) 3:e232. doi: 10.1212/NXI.0000000000000232
185. Vieira ELM, De Oliveira GNM, Lessa JMK, Goncalves AP, Oliveira ACP, Bauer ME, et al. Peripheral leukocyte profile in people with temporal lobe epilepsy reflects the associated proinflammatory state. Brain Behav Immun (2016) 53:123–30. doi: 10.1016/j.bbi.2015.11.016
186. Xu D, Robinson AP, Ishii T, Duncan DS, Alden TD, Goings GE, et al. Peripherally derived T regulatory and gammadelta T cells have opposing roles in the pathogenesis of intractable pediatric epilepsy. J Exp Med (2018) 215:1169–86. doi: 10.1084/jem.20171285
187. Troscher AR, Sakaraki E, Mair KM, Kock U, Racz A, Borger V, et al. T cell numbers correlate with neuronal loss rather than with seizure activity in medial temporal lobe epilepsy. Epilepsia (2021) 62:1343–53. doi: 10.1111/epi.16914
188. Troscher AR, Mair KM, Verdu De Juan L, Kock U, Steinmaurer A, Baier H, et al. Temporal lobe epilepsy with gad antibodies: neurons killed by T cells not by complement membrane attack complex. Brain (2023) 146:1436–52. doi: 10.1093/brain/awac404
189. Sotgiu S, Murrighile MR, Constantin G. Treatment of refractory epilepsy with natalizumab in a patient with multiple sclerosis. Case report. BMC Neurol (2010) 10:84. doi: 10.1186/1471-2377-10-84
190. Susac JO, Hardman JM, Selhorst JB. Microangiopathy of the brain and retina. Neurology (1979) 29:313–6. doi: 10.1212/WNL.29.3.313
191. Kleffner I, Ringelstein EB, Stupp N, Niederstadt TU, Young P. Neurological picture. Susac's syndrome: effective combination of immunosuppression and antiplatelet treatment. J Neurol Neurosurg Psychiatry (2006) 77:1335. doi: 10.1136/jnnp.2006.095596
192. Rennebohm RM, Egan RA, Susac JO. Reatment of Susac’s syndrome. Curr Treat Options In Neurol (2008) 10:67–4. doi: 10.1007/s11940-008-0008-y
193. Fernando SL, Boyle T, Smith A, Parratt JDE. The successful use of infliximab in A relapsing case of susac's syndrome. Case Rep Neurol Med (2020) 2020:9317232. doi: 10.1155/2020/9317232
194. Agamanolis DP, Klonk C, Bigley K, Rennebohm RM. Neuropathological findings in susac syndrome: an autopsy report. J Neuropathol Exp Neurol (2019) 78:515–9. doi: 10.1093/jnen/nlz031
195. Schwab SR, Cyster JG. Finding A way out: lymphocyte egress from lymphoid organs. Nat Immunol (2007) 8:1295–301. doi: 10.1038/ni1545
196. Levite M. T cells plead for rejuvenation and amplification; with the brain's neurotransmitters and neuropeptides we can make it happen. Front Immunol (2021) 12:617658. doi: 10.3389/fimmu.2021.617658
197. Chaib S, Tchkonia T, Kirkland JL. Cellular senescence and senolytics: the path to the clinic. Nat Med (2022) 28:1556–68. doi: 10.1038/s41591-022-01923-y
198. Fisicaro P, Barili V, Montanini B, Acerbi G, Ferracin M, Guerrieri F, et al. Targeting mitochondrial dysfunction can restore antiviral activity of exhausted hbv-specific cd8 T cells in chronic hepatitis B. Nat Med (2017) 23:327–36. doi: 10.1038/nm.4275
199. Blake MK, O'connell P, Aldhamen YA. Fundamentals to therapeutics: epigenetic modulation of cd8(+) T cell exhaustion in the tumor microenvironment. Front Cell Dev Biol (2022) 10:1082195. doi: 10.3389/fcell.2022.1082195
200. Quezada LK, Jin W, Liu YC, Kim ES, He Z, Indralingam CS, et al. Early transcriptional and epigenetic divergence of cd8+ T cells responding to acute versus chronic infection. PloS Biol (2023) 21:e3001983. doi: 10.1371/journal.pbio.3001983
Keywords: CD8+ T lymphocytes, tissue resident memory CD8+ T cells, neurotoxicity, neurodegenerative diseases, cytotoxicity
Citation: Terrabuio E, Zenaro E and Constantin G (2023) The role of the CD8+ T cell compartment in ageing and neurodegenerative disorders. Front. Immunol. 14:1233870. doi: 10.3389/fimmu.2023.1233870
Received: 07 June 2023; Accepted: 13 July 2023;
Published: 28 July 2023.
Edited by:
Dong-Ming Su, University of North Texas Health Science Center, United StatesReviewed by:
Weikan Wang, University of North Texas Health Science Center, United StatesShiyun Xiao, University of Georgia, United States
Copyright © 2023 Terrabuio, Zenaro and Constantin. This is an open-access article distributed under the terms of the Creative Commons Attribution License (CC BY). The use, distribution or reproduction in other forums is permitted, provided the original author(s) and the copyright owner(s) are credited and that the original publication in this journal is cited, in accordance with accepted academic practice. No use, distribution or reproduction is permitted which does not comply with these terms.
*Correspondence: Eleonora Terrabuio, eleonora.terrabuio@univr.it