- Ministry of Education (MOE), Key Laboratory of Tumor Molecular Biology and Key Laboratory of Functional Protein Research of Guangdong Higher Education Institutes, Institute of Life and Health Engineering, Jinan University, Guangzhou, China
Gastrointestinal cancer is a leading cause of cancer-related mortality and remains a major challenge for cancer treatment. Despite the combined administration of modern surgical techniques and chemoradiotherapy (CRT), the overall 5-year survival rate of gastrointestinal cancer patients in advanced stage disease is less than 15%, due to rapid disease progression, metastasis, and CRT resistance. A better understanding of the mechanisms underlying cancer progression and optimized treatment strategies for gastrointestinal cancer are urgently needed. With increasing evidence highlighting the protective role of immune responses in cancer initiation and progression, immunotherapy has become a hot research topic in the integrative management of gastrointestinal cancer. Here, an overview of the molecular understanding of colorectal cancer, esophageal cancer and gastric cancer is provided. Subsequently, recently developed immunotherapy strategies, including immune checkpoint inhibitors, chimeric antigen receptor T cell therapies, tumor vaccines and therapies targeting other immune cells, have been described. Finally, the underlying mechanisms, fundamental research and clinical trials of each agent are discussed. Overall, this review summarizes recent advances and future directions for immunotherapy for patients with gastrointestinal malignancies.
Introduction
Gastrointestinal (GI) cancers are among the top 10 most prevalent and deadliest tumors worldwide, accounting for 26% of global cancer incidence and 35% of all cancer-related deaths (1). To date, surgical resection remains the primary treatment option for patients with colorectal cancer (CRC), gastric cancer (GC) and esophageal cancer (EC). Despite advances in adjuvant and neoadjuvant chemoradiotherapy (CRT), a fair number of patients still develop distant metastases and therapy resistance (2). To improve the prognosis of GI cancers, new therapeutic strategies are urgently needed. Over the last few decades, immune-targeted therapy has emerged as a revolutionary option for cancer treatment (3); however, the regulatory role of the immune system underlying GI cancers remains to be clarified. Fortunately, with the development of immunotherapy, cancer immunotherapy mainly based on checkpoint inhibitors has shown great prospects in clinical research, which shows the importance of immunotherapy in cancer treatment.
In this review, we first provide an overview of GI in terms of the epidemiology, molecular pathogenesis and standard therapy regimens. In subsequent sections, we outline the functional and molecular basis of oncoimmunology, with an emphasis on novel immune checkpoint targets and examples of applications in both laboratory research and clinical trials. We hope that this review will bring new insight into cancer immunotherapy for oncologists and immunologists.
Gastrointestinal Cancers: A General Overview
Esophageal and Gastric Cancer
The esophagus and stomach are part of the upper GI tract, which is part of the digestive system. As two major types of EC, esophageal squamous cell carcinoma (ESCC) occurs more commonly in the upper or middle part of the esophagus, while esophageal adenocarcinoma (SCC) occurs in the lower part of the esophagus. GC can develop in any part of the stomach and can spread throughout the stomach and to other organs, such as the small intestines, lymph nodes, liver, pancreas and colon (4). EC and GC are listed as the seventh and fourth most prevalent cancers worldwide (5). Based on estimates from 2018, 36.4% of digestive cancers, including stomach, liver and esophageal cancers, in China have a very poor prognosis, and the 5-year overall survival (OS) rate is quite low (less than 35% from 2013 to 2015) (6).
In general, surgery plays a key role in the treatment of GC as well as EC at an early stage. Moreover, systemic therapy of advanced, metastatic esophageal and gastric cancer utilizes a combination of multiple cytotoxic chemotherapeutic agents. Combination chemotherapy with a platinum and fluoropyrimidine doublet, such as FOLFOX, CAPOX, cisplatin/5-fluorouracil (5-FU), or cisplatin/capecitabine, is a common regimen with the addition of trastuzumab for the treatment of HER2-positive disease (5, 7, 8). Other agents, such as irinotecan or taxanes, can be utilized with fluoropyrimidines, platinum and/or ramicurumab or applied as monotherapy for those unfit for combination regimens (9, 10).
Colorectal Cancer
In recent decades, the incidence rate of CRC has shown an upward trend worldwide, especially in developing countries. In China, CRC has an incidence rate exceeding 14.2/100,000, a mortality rate exceeding 7.4/100,000 and a 5-year prevalence exceeding 52/100,000. In addition, the prevalence rate of CRC is obviously higher in senior citizens aged over 60 (11). Currently, traditional therapies for CRC include endoscopic and surgical local excision, downstaging preoperative radiotherapy and systemic therapy, extensive surgery for locoregional and metastatic disease, local ablative therapies for metastases, palliative chemotherapy, targeted therapy, and immunotherapy. Although these new treatment options have doubled the OS for advanced disease to 3 years, the best survival rates still occur in patients without metastasis.
The Rationale for Immunotherapy in Gastrointestinal Cancer
The immune system exists within the body, and the execution of immune function is performed by the entire immune system. The immune system consists of immune organs, immune cells and immune molecules. These cell types surrounding cancer cells, including fibroblasts, endothelial cells, immune cells, and extracellular molecules, which include cytokines, hormones, cellular matrix, and growth factors, constitute the tumor microenvironment (TME) (12). According to relevant reports, some immune components in the TME can regulate the occurrence and development of tumors, and these components constitute the tumor immune microenvironment (TIME), which is expected to become a promising target for cancer immunotherapy (13–15) (Figure 1).
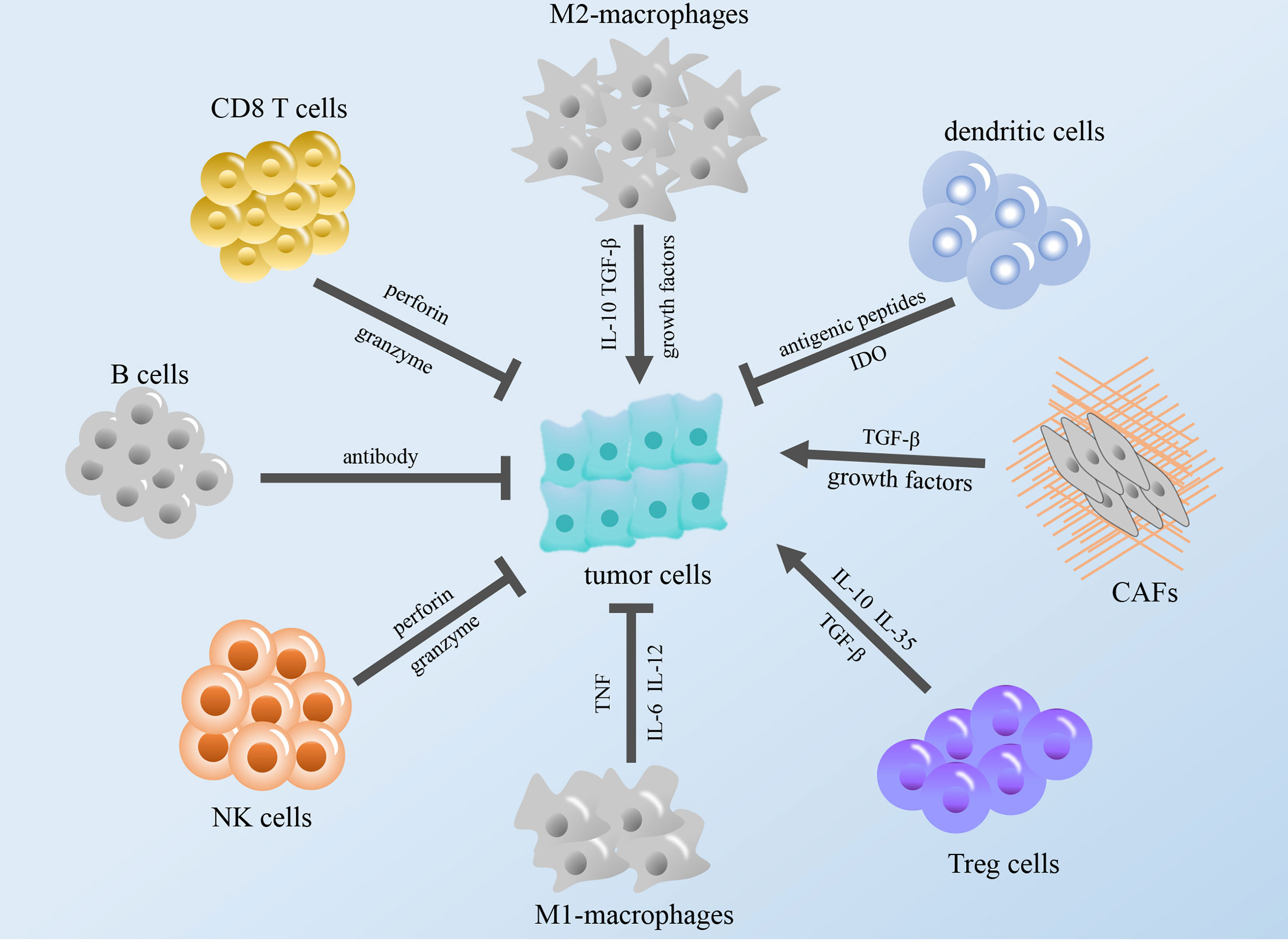
Figure 1 Modulation of the tumor immune microenvironment. The figure shows that immune cells in TME regulate tumor growth through cytokines and other regulatory factors. TGF-β, transforming growth factor β; IL, interleukin; NK cells, natural killer cells; TNF, tumor necrosis factor; IDO, indoleamine 2,3-dioxygenase; CAFs, cancer-associated fibroblasts; Treg cells, regulatory T cells.
Components of the TME
Tumor Cells
Although many improvements in molecularly directed therapies have been achieved, the prognosis of GI cancers remains poor (12). Breakthroughs in the immune checkpoint blockade offer potential therapeutic avenues, particularly with tools to overcome the mechanisms of immunosuppression in the TME. Currently, extensive publications highlight critical roles of the TIME in GI cancers, including CRC and GC (16, 17). As an important determinant of tumor progression and outcome in GI cancers, the TIME can shape cancer cell phenotypes and therapy responses through interplay with cancerous cells via chemokine and cytokine signaling or direct contact (18–20). Several reports have revealed that tumor progression and metastasis are subjected to not only genetic alterations within tumor cells but also the TIME elements. In brief, CD4+ T helper cells, CD8+ CTLs, NK cells, M1 macrophages, and DCs have been shown to be associated with a good prognosis (21). Conversely, CD4+ FOXP3+ Th2 cells, M2 macrophages, and myeloid-derived suppressor cells (MDSCs) have been attributed to a poor outcome (18).
Immune Cells
Tumor-infiltrating lymphocytes (TILs) are immune cells that have migrated to tumor tissue and the local microenvironment. This population is indicative of an immune response generated by the patient against the malignancy. TIL populations across GI tumors generally contain T lymphocytes, particularly CD8+ cytotoxic T lymphocytes (CTLs) (12). In EC cells, blocking the programmed cell death 1 (PD-1)/programmed cell death ligand 1 (PD-L1) and TGF-β signaling pathways can synergistically restore the function of antigen-specific CD8+ T cells and the capacity of antitumor T cells (22). In addition, functional MAGE-A3-specific CD8+ T cells have an independent prognostic effect on the survival of patients with ESCC (22). Recent studies have demonstrated that higher numbers of CD3+, CD8+, or CD45RO+ T cells in tumor tissue are significantly correlated with a superior disease outcome in patients with GC, and an imbalance in Th1 and Th2 cells can lead to an immunosuppressive state dominated by Th2-type cells (23). The Th1/Th2 cell ratio in peripheral blood in GC can be used to predict postoperative prognosis (24). Similarly, the type, density, and location of immune cells in CRC also have prognostic value that is superior to and independent of those of the tumor node metastasis (TNM) classification (25). In addition to T cells, there are many other immune cell types that infiltrate GI cancers.
Tregs, as a subtype of CD4+ T cells, can inhibit effector T cells via a series of chemokine signaling (26). FOXP3+ Tregs, a subtype of Tregs, their roles are ambiguous. Some studies have shown that a high density of FOXP3+ Tregs is beneficial to the prognosis of CRC after undergoing chemo or chemoimmunotherapy (27). On the other hand, it has been shown that Tregs in the esophageal mucosa and peripheral blood of patients with esophageal cancer increase significantly (28).
DCs, on the one hand, express MHC Class II and can present their antigenic peptides to CD4+ T cells. They activate effector T cells to attack tumors and play a crucial role in shaping the host response to cancerous cells. GC patients with good DC infiltration had lower lymph node metastases and lymphatic invasion and better 5-year survival rates (78%) than patients with less DC infiltration (29). On the other hand, activated DCs help in the expansion of Tregs, consequently leading to regulation of immune responses and thereby tumor immune escape (30). Meanwhile, DCs also stimulate the formation of M2 macrophages, thereby increasing the secretion of IL-10 and TGF-β (31), which reduces the expression of IL-12 expressed by DCs and inhibits the activation of adaptive responses (32).
Tissue-resident macrophages are present prior to the development of any malignancy (33, 34). Tumor-associated macrophages (TAMs) can differentiate into two distinct subtypes, M1 and M2. M1 macrophages secrete IL-6 and IL-12 to mitigate resistance during tumor development; they can also be activated by IFN-γ to secrete TNF to kill cancer cells, while M2 macrophages secrete growth factors that promote neoangiogenesis and tumor proliferation (35). In various types of cancers, increased numbers of TAMs are often related to a poor prognosis. However, the roles of TAMs in CRC remain controversial. According to some reports, on the one hand, a high density of TAMs predicts a better postoperative outcome (36), and on the other hand, TAMs also secrete cytokines that favor tumor development (37), which indicates that the impact of TAMs on CRC needs to be further explored. Additionally, in accordance with some studies, TGF-β and other growth factors secreted by cancer-associated fibroblasts (CAFs) promoted the proliferation of CRC cells through the Smad2/Smad4 pathway (38) and MAPK/PI3K/AKT pathway (39).
Neutrophils are similar to TAMs in classification. Neutrophils differentiate into N1 and N2 according to their polarization state. N1 has antitumor activity, which directly kill tumor cells by releasing reactive oxygen species (ROS) and reactive nitrogen species (RNS) (40, 41) and can also recruit M1 macrophages and promote T cell activation. In contrast, N2 has tumor-promoting activity, promoting angiogenesis and inhibiting the function of NK cells by releasing matrix metalloproteinase 9 (MMP9) (42). In addition, N2 recruits M2 macrophages and Treg cells and suppresses the function of CD8+ T cells, as well as other native neutrophils (43). Neutrophil extracellular traps (NETs) activate Toll-like receptor 9 on CRC cells through the MAPK pathway, which leads to the growth, migration and invasion of CRC cells (44).
B lymphocytes play an important role in tumor immunity and cancer biology. However, several studies have revealed that B lymphocytes can take part in carcinogenesis and tumor progression by producing antibodies to facilitate chronic inflammation in the TIME (45, 46). Moreover, regulatory B cells can also restrain antitumor responses mediated by T cells in cancer (47). Regulatory B cells (Breg cells), a novel subset of B lymphocytes, appear to facilitate tumor growth and progression via the production of IL-10 to suppress the activity of CD8+ T cells in squamous carcinoma (48). In CRC, the presence of B cells seems to be detrimental to prognostic outcome (49).
NK cells are unique in that they have both innate and adaptive immune properties. NK cells participate in the antitumor immune response through the production of proinflammatory cytokines, which recruit and induce the proliferation of other immune cells (12). Activated NK cells can directly kill some tumor cells and virus-infected cells. In EC, expanded NK cells had high cytotoxicity against ESCC cells, especially those with the epithelial-mesenchymal transition phenotype (50). In another investigation, a higher NK cell density was shown to be significantly related to a higher survival rate in GC, especially for advanced patients (51).
Cytokines/Chemokines
Cytokines are important players in the tumor microenvironment, and cytokines/chemokines are used to establish connections between various immune cells, such as IL-6 (52), IL-10 (53), IL-12 (54), IL-35 (55), epidermal growth factor (EGF), vascular endothelial growth factor (VEGF), tumor necrosis factor alpha (TNF-α) (56), interferon γ (IFN-γ) (57), indoleamine-2,3-dioxygenase (IDO) (58), and transforming growth factor beta (TGF-β) (59). For example, IL-10 and TGF-β switch macrophages from a M1-like (proinflammatory or classically activated) state to a M2-like (anti-inflammatory or alternatively activated) state. M1 macrophages induce antitumor immune signaling and correlate with tumor killing capacity. Conversely, M2 macrophages exhibit protumor effects and contribute to fibrosis and the production of matrix proteins (60) as well as angiogenesis, metastasis, and the suppression of adaptive immunity (61, 62). Human CRC cell lines cultured in vitro are able to polarize macrophages toward the M2 phenotype (63). In GC patient samples, M2 macrophages in the stroma may be correlated with the presence of a lesion (64). The CCL2-CCR2 axis in esophageal carcinogenesis contributes to immune evasion and tumor promotion through the PD-1 signaling pathway (65). At the same time, the activity of NK cells and effector T cells was suppressed due to the increased secretion of cytokines such as IL-10, TGF-β, EGF and VEGF (50).
Cells in the Extracellular Matrix (ECM)
Unlike strictly defined immune cells, fibroblasts are present within the stromal microenvironment and serve to produce extracellular matrix (ECM) proteins in particular collagen. They actively manufacture and respond to cytokines in cooperation with immune cells within the stromal microenvironment. Fibroblasts are also associated with epithelial cell polarity, proliferation, and, to some extent, tumorigenic potential. CAFs have been shown to drive increased tumor growth compared to normal fibroblasts (66). They contribute to cancer cell survival and progression via a series of nutrient-rich ECM proteins or ECM-degrading proteases, resulting in persistent chronic inflammation within the tumor microenvironment and enhanced epithelial mesenchymal transition (EMT) of tumor cells (67, 68).
Recent studies have demonstrated that CAFs have the capacity to produce proinflammatory cytokines, which disrupt the normal cytokine balance to stimulate tumor growth by initiating angiogenesis and inhibiting CTLs (69, 70). Additionally, CAFs have been shown to secrete high levels of the proinflammatory cytokines IL-1β, IL-8, IL-10, tumor necrosis factor-alpha (TNF-α), monocyte chemoattractant protein-1 (CCL2), stromal derived factor-1 (CXCL12) and interferon-beta (IFN-β) (71). The crucial role of CAFs in tumorigenesis has been addressed by genetic analyses showing that their gene expression profiles are very different from those of normal breast fibroblasts. Moreover, the expression profiles of CAFs obtained from tumors with poor versus good prognosis are also very different (72). Good-outcome fibroblasts were associated with immune modulators involved in the Th1 immune response. This includes the expression of T cell receptor complexes (CD8a, CD247, and CD3D), MHC class I protein binding and granzyme A/B activity. The poor-outcome stroma had increased levels of hypoxia and angiogenesis and decreased chemokines that stimulate NK migration and T cell survival (73).
Mechanisms of Tumor Immune Escape
One role of the immune system in mediating tumorigenesis is called “cancer immune editing”, and it can be classified into 3 stages: elimination, stalemate, and escape. The elimination stage includes innate and adaptive immune responses to specific tumor-related antigens and is characterized by the effector functions of T cells, B cells and NK cells mediated by cytokines, including IFN-α, IFN-γ and IL-12. The stalemate stage of immune killing is mediated by the adaptive immune system and the persistence of a small number of malignant clones. The escape stage involves malignant clones gaining the ability to evade surveillance carried out by the adaptive immune system.
The understanding of the mechanisms of tumor immune escape changes with each passing day, and the established mechanisms are as follows. First, there can be a lack of a specific antigen or an alteration in antigen processing. In a study of SCC cell lines and tumor tissues, levels of MIR125a-5p and MIR148a-3p were found to be increased, reducing levels of ATP binding cassette subfamily B member 3 (TAP2) and MHC I, both of which are required for antigen presentation (74). Tumor cells lack the expression of major MHC I molecules or lose the intracellular processing mechanism, enabling the transfer of tumor antigens to the surface of tumor cells for T cells to recognize (75). The high expression of MHC I molecules in SCC is related to markers of the adaptive immune response and significantly decreased OS time in patients (74). Second, tumors can promote the formation of an immune-tolerant microenvironment by affecting the levels of cytokines, such as by increasing the secretion of IL-6, IL-10 and TGF-β or by consuming IL-2. The changes in these cytokines in EC cells promote the infiltration of Treg cells, MDSCs and other types of cells, thus suppressing the function of cytotoxic T cells (76). Third, tumors can upregulate the expression of PD-1 and PD-L1 to induce peripheral T cell depletion (77). Finally, many oncogenic cell signaling pathways were originally thought to be used only to accelerate cell division and growth, but they are now thought to be factors mediating immune escape. For example, constitutively activated KIT signaling in GI tumors can lead to overexpression of indoleamine-2,3-dioxygenase (IDO), thus increasing Treg cell infiltration and promoting tumor growth; furthermore, melanoma cells activated by β-catenin/Wnt signaling can inhibit DC-mediated antigen presentation and prevent CD8+ T cell infiltration (78).
Immunotherapeutic Strategies in Gastrointestinal Cancer: The Current Scenario and Future Perspectives
Immunotherapy refers to the activation of the body’s immune system to fight against tumor cells. It manipulates the immune system to target cancer antigens or break the barriers of T cell infiltration. Immunotherapy methods mainly include cytokines, immune checkpoint inhibitors, CAR T cell treatments, tumor vaccines, and treatments involving other immune cells in the TME.
Activating the Immune Response - Cytokines
Several cytokines impede cancer cell growth via direct antiproliferative or proapoptotic actions or by indirectly enhancing the cytotoxic effect of immune responses against cancer cells. For example, autocrine IL-10 activity on CD8+ T lymphocytes has been shown to be crucial for prolonging the effector activity of cytotoxic CD8+ T cells (79, 80). This concept has been evaluated in a phase I clinical trial in advanced treatment-refractory tumors (NCT02009449) using IL-10 conjugated with PEG to increase its half-life. The administration of PEGylated cytokines (termed pegilodecakin) resulted in partial responses in patients with uveal melanoma, renal cell carcinoma (RCC) and CRC (81). In addition, CCR5 is the receptor for both CCL3 and CCL5, while CCR2 binds to CCL2. In patients with metastatic CRC, CCR2/CCR5 inhibitors, coupled with either chemotherapy or nivolumab, suppressed myeloid cell recruitment by blocking the activity of these chemokines (82). Another paradigmatic case is IFN-α, first discovered in 1957, which induces antitumor efficacy by directly augmenting NK cell-mediated killing and acting on T and B lymphocytes to modulate their activity and/or survival.
Immune Checkpoint Inhibitors
Immune checkpoints play key roles in the innate immune system by ensuring that immune cells are capable of distinguishing self-antigens from exogenous antigens. In the TME, tumors often display self-antigens and escape immune surveillance. By blocking the interaction between immune cells and tumor cells expressing immune checkpoint molecules, checkpoint inhibitors allow the immune system to recognize tumor-associated antigens and consequently destroy malignant cells. As shown in Figure 2, when CTLA-4 and LAG-3 are bound by their corresponding monoclonal antibodies, T cells become activated and differentiate and proliferate; TIM-3 and PD-1 on T cells and PD-L1 on tumor cells are bound by their corresponding antibodies, which prevents T cell death, inhibits tumor cell evasion and promotes tumor cell apoptosis (Figure 2).
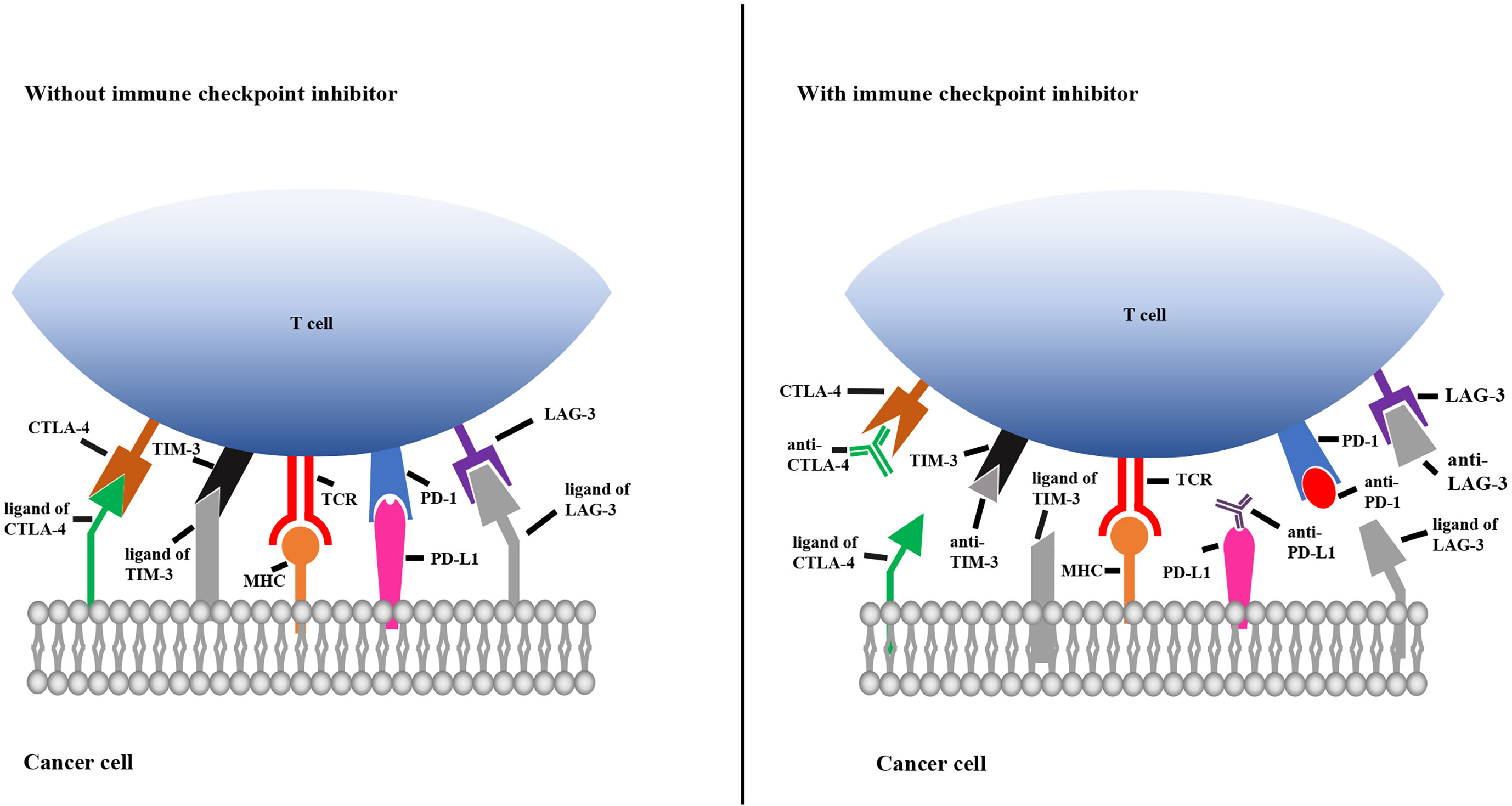
Figure 2 Mechanisms of immune checkpoint inhibitors. The left picture shows that the immune checkpoint molecules on T cells combine with the corresponding ligands on cancer cells, resulting in immunosuppression of T cell. The right picture shows that immune checkpoint molecules bind to the corresponding antibody to prevent T cell death. TCR, T cell receptor; MHC, major histocompatibility complex; TIM-3, T cell immunoglobulin 3; CTLA-4, cytotoxic T-lymphocyte-associated protein 4; PD-1, programmed cell death 1; PD-L1, programmed cell death ligand 1; LAG-3, lymphocyte activation gene 3.
CTLA-4
As the first inhibitory receptor identified, CTLA-4 is mainly located on activated T lymphocytes and is also highly expressed in several types of cancer (83). There is a competitive interaction between CD28 and CTLA-4 in binding with CD80/CD86 molecules. CTLA-4 transmits an inhibitory signal to T cells, whereas CD28 transmits a stimulatory signal. A recent study revealed that the combined consideration of CTLA-4 expression and the platelet-lymphocyte ratio (PLR) has potential prognostic value for people suffering from ESCC (84). Therefore, it is not surprising that CTLA-4-targeted agents have shown great potential in the treatment of many cancers. In clinical trials, the tumor response rates of two anti-CTLA-4 agents, ipilimumab and tremelimumab, were approximately 10% (85). A large phase II clinical study explored the efficacy and safety of nivolumab ( ± ipilimumab) in the treatment of advanced CRC. The results revealed that most patients with microsatellite instability-high (MSI-H) metastatic CRC (mCRC) benefited from the nivolumab + ipilimumab regimen, and the regimen was well tolerated, but whether the combination therapy is superior to either drug as monotherapy therapy is still uncertain (86).
PD-1 and PD-L1/L2
PD-1 is one of the crucial immune checkpoint receptor proteins on T cells, B cells and NK cells and can bind to PD-L1 and/or PD-L2, which are located on the surface of multifarious tumor cells as well as hematopoietic cells. The combination of PD-1 and PD-L1/L2 expressed on tumor cells can dramatically inhibit the apoptosis of tumor cells, promoting the depletion of peripheral effector T cells and catalyzing the transformation of effector T cells into Treg cells (87). In 2017, the US Food and Drug Administration (FDA) approved pembrolizumab for the treatment of unresectable or metastatic MSI-H or mismatch repair deficient (dMMR) CRC that progressed following treatment with fluoropyrimidine, oxaliplatin, and irinotecan. This approval was based on results from the phase II CheckMate-142 trial (88), in which the objective response rate (ORR) was 28% in mCRC patients who received prior fluoropyrimidine, oxaliplatin, and irinotecan; in the trial, 1 patient had a complete response (CR), and 14 patients had partial responses (PRs). Furthermore, the expression of PD-L1 in cancer cells and the TME may contribute to the development of EBV-associated GC, and PD-L1 overexpression predicts large tumor size, lymph node metastasis, and poor prognosis in GC (89). A recent study presented at the American Society of Clinical Oncology (ASCO) meeting revealed that an anti-PD-1 monoclonal antibody could significantly prolong the OS of PD-L1-positive patients with advanced EC regardless of whether it was used alone or in combination with chemotherapy; furthermore, the antibody was highly safe (90, 91).
TIM-3
TIM-3 is a cell surface molecule expressed on DCs, monocytes, CD8 T cells and Th1 cells. The ligands of TIM-3 are frequently overexpressed in tumor cells. TIM-3 is a negative regulator of the antitumor immune response and has been proven to be associated with the occurrence and development of several malignant tumors. At both the mRNA and protein levels, the expression of TIM-3 in ESCC is remarkably higher than that in matched adjacent normal tissues; furthermore, researchers have found a close connection between the overexpression of TIM−3 and poor survival in patients with ESCC. Similarly, TIM-3 knockdown significantly inhibited the propagation, migration and invasion of ESCC cell lines. Further research explored whether the depletion of TIM-3 could restrain several signal transduction pathways, including the snail, p-GSK-3β and p-AKT pathways (92). In addition, some studies have suggested that the increased expression of TIM-3 on T cells may be partially responsible for the development of GC by inducing the secretion of IFN-γ and TNF-α (93). Another study has shown that reduced TIM-3 expression induced by genetic polymorphisms of TIM-3 may promote CRC invasion and metastasis (94). Taken together, these findings show that TIM-3 is a critical mediator in the progression of various cancers and may serve as a potential therapeutic target.
LAG-3
LAG-3 is another membrane protein expressed on B cells, some T cells, NK cells and TILs. LAG-3 can enhance the activation of Treg cells and prevent T cells from proliferating and differentiating into effector cells by binding with MHC II. In addition, some basic research has shown that dual blockade of LAG-3 and PD-1 can induce strong antitumor action (95). Furthermore, high expression of LAG-3 is associated with an improved survival rate in patients with ESCC or CRC and is being evaluated as a biomarker to predict response to antitumor treatment. These results indicate that LAG-3 can be used as a marker of the immune response for patients with ESCC or CRC (96, 97).
SIRPα
Signal regulatory protein-α (SIRPα) acts as an inhibitory receptor and is expressed on myeloid cells such as macrophages, DCs, mast cells and neutrophils. It interacts with the broadly expressed transmembrane protein CD47, also called the “don’t eat me” signal. In addition, the interaction between CD47 and SIRPα can help tumor cells avoid phagocytosis in the TME (98). The biological and preclinical relevance of the interaction of SIRPα and CD47 has been extensively investigated. A recent study showed that blocking SIRPα/CD47 signaling in ESCC cell lines could enhance the phagocytosis of ESCC cells in a dose-dependent manner (99). In addition, with the evolution of cancer immunotherapy, SIRPα/CD47 immunotherapy to stimulate the innate immune system as a cancer treatment has drawn increasing interest. Other reports have confirmed that the high expression of CD47 is an independent prognostic factor in GC, and the OS rate of patients with CD47-positive tumors was significantly lower than that of patients with CD47-negative tumors (100). Aberrant expression of CD47 has also been reported to strongly promote the proliferation of tumor cells (101).
CAR T Cell Treatments
Chimeric antigen receptor (CAR) T cell therapy includes T cell modifications that enable activated T cells to recognize specific tumor cell surface antigens independent of MHC and eventually eliminate malignant cells (102). After many years of medical and laboratory research as well as specific clinical trials, in 2017, the US FDA approved the use of two CAR T cell therapies (103, 104). In recent years, some laboratories have attempted to exploit EphA2. CAR T cells recognizing EphA2 have demonstrated the capacity to identify and attack ESCC cells in vitro. These findings open a new avenue for future immunotherapies for ESCC (105). Coincidentally, CAR T cell therapy has been widely used in the treatment of other GI cancers and liver cancers. The HER2 gene plays an important role in the occurrence and development of GC, which highly expresses the protein p185; p185 shows negative expression in healthy individuals, and thus, it could be used as an ideal target for antitumor therapy with CAR T cells (106). Carcinoembryonic antigen (CEA), a sensitive tumor biomarker, was found to be a potent target for CAR T cell therapy for GI cancers (106). Therefore, a great deal of research has been carried out on the treatment of different cancers, such as liver cancer, cholangiocarcinoma and pancreatic cancer. Combined treatment integrating CAR T cell therapy with targeted therapies, such as therapies targeting CEA, HER2, GPC3, MUC1, CD133, EpCAM or EGFR, has exhibited great potential (107–110).
Targeted Therapy Mediating Immune Cells
NK Cells
NK cells are innate lymphocytes and play a pivotal role in host immunity by killing virally infected and/or cancerous cells. Several clinical studies have suggested NK cell-based immunotherapy as a potential therapy for cancer. Inhibitory receptors, such as killer-cell immunoglobulin-like receptors (KIRs) and CD94/NK group 2 member A (NKG2A), recognize human leukocyte antigen (HLA) class I molecules on normal host cells. By lacking inhibitory receptor ligands, tumor cells that have downregulated surface MHC-I expression become susceptible to attack by NK cells. Current NK cell-based cancer immunotherapy aims to reverse tumor-induced NK cell dysfunction and sustain NK cell effector functions (111). To fight against tumors, NK cells can also be modulated to target the cell types responsible for maintaining the immunosuppressive TME, including M2-polarized macrophages, MDSCs, Treg cells, and fibroblasts (112). According to recent reports, adoptive cell therapy (ACT) has become a powerful tool to improve host antitumor activity (113), mainly including autologous NK cell transfer, allogeneic NK cell transfer and CAR-engineered NK cells (114). The three methods all artificially activate and expand NK cells in vitro and then transfer the modified NK cells to patients. Under the stimulation of some cytokines and costimulators, the antitumor ability of NK cells has been significantly improved (113, 115), and NK cells are expected to achieve staged success in the course of immunotherapy.
NKT Cells
Natural killer T cells (NKTs) are unique T lymphocytes that have the characteristics of conventional T cells and natural killer cells (116). They play an important role in connecting the natural immune system with the adaptive immune system and are also an important intermediary for mediating the immune response and tumor monitoring (117). It is usually composed of two parts, namely, the invariant TCRα chain and the semivariant TCRβ chain, recognizing the lipid antigen presented by the nonclassical MHC class I molecule CD1d (118). More importantly, the activation of CD1d-dependent invariant natural killer T cells (iNKT cells) can promote the release of cytokines such as IL-5, IL-6, IL-10, IL-17, IL-21, TNF-α, TGF-β and granulocyte-macrophage colony stimulating factor (GM-CSF) (116, 119, 120) and indirectly activate the antitumor mechanism. At present, several cancer immunotherapies based on iNKT cells have been developed, mainly including antigen-presenting cells (APCs) pulsed with α-GalCer, the transfer of ex vivo–expanded and/or activated iNKT cells, and iNKT cell–activating ligands (117). First, a clinical experimental study has not indicated any major toxicity or severe side effects in patients who have received repeated injection of the tumor immunity agonist α-GalCer (121), implying its clinical value in improving tumor immunity. In addition, patients with head and neck squamous cell carcinoma, who were treated with the combination of iNKT cells and α-GalCer-pulsed DCs, the antitumor immunity was greatly improved (122, 123)
Macrophages
Tumor-infiltrating immune cells play an ambiguous role in tumor development and progression. It has been extensively reported that TAMs promote or inhibit the expansion and dissemination of cancer cells depending on their functional states. Tumor-infiltrating macrophages are mainly recruited by CCL2 or CSF-1, and TAM depletion therapy (e.g., CCR2 or CSF1R antagonists) has already been tested in clinical trials for malignant solid tumors (124, 125). Some studies have also shown that CSF1R inhibitors represent a promising combination partner for T cell-enhancing immunotherapies (126, 127). Further investigation of the synergistic effects of these agents with immunotherapies will lead to the improvement of ongoing immunotherapeutic strategies. At the same time, some cancer immunotherapies targeting TAMs are on the rise, and most of them follow the principle of directly reducing the formation of TAMs or polarizing TAMs from the pro-tumor phenotype (M2-like), which helps tumor development to an antitumor phenotype (M1-like) (128). By preventing the recruitment of TAMs or blocking the CSF1/CSF1R axis, the survival rate of TAMs can be significantly reduced (129, 130), as mentioned above. In addition, some studies have shown that the polarization of TAMs to M1 macrophages can be promoted by targeting antibody-dependent cellular phagocytosis (ADCP) induced by the SIRPα/CD47 axis, thus enhancing the tumor immune response (131). Finally, a possible strategy for cancer treatment is the use of natural living macrophages as drug delivery vehicles (128).
γδT Cells
As a subtype of T lymphocytes, γδT cells have a wide range of antigen recognition abilities and can be used to recognize a series of antigens, including mitochondrial ATPase, human mutS homolog 2 and nonpeptide phosphoantigens (132–134), without using traditional APC to mediate antigen recognition (135, 136). The mechanism of antigen recognition depends on its peptide sequence. Since the sequence of complementarity determining region 3 (CDR3) of the delta chain is longer and more varied, it is more important in the process of antigen recognition (137). In addition to expressing NK cell receptors such as NGG2D, it may be a bridge between the innate immune system and adaptive immunity (138). According to related reports, under certain conditions, γδT lymphocytes may be activated in the late stage of the immune response (139, 140), which shows cytotoxicity by secreting perforin and granzyme and can also regulate the secretion of IFN-γ and TNF-α (141). Conversely, some cytokines may drive γδT lymphocytes to remodel tumor-immunosuppressive functions; thus, there is an urgent need to optimize γδT lymphocytes to obtain new antitumor targets (142). A recent study demonstrated for the first time that CAR-γδT cells obtained by modification using CAR technology are able to migrate to tumor cells and cross-express antigens (143), which opens a new avenue for cancer immunotherapy research.
IDO1
IDO1 is the rate-limiting enzyme that participates in tryptophan metabolism by converting the amino acid L-tryptophan (Trp) into L-kynurenine (Kyn). This enzyme assists tumor cells in maximally utilizing essential amino acids such as tryptophan in the TME to support tumor growth. However, as immune cells lose tryptophan, they lose their ability to fight cancer cells. Overexpression of IDO1 in tumor cells inhibits T cell function and impairs immune surveillance, leading to immune escape (144). In addition, it has been verified that the expression of IDO1 is associated with prognosis in EC and could be used as a prognostic biomarker (145). In a multivariate analysis, IDO1 expression was proven to be an independent prognostic factor for developing recurrence (146). Chen et al. reported that the enzyme activity of IDO1 directly affected the radiosensitivity of CRC, and IDO1 inhibition made CRC cells more sensitive to radiation-induced cell death and protected the normal small intestinal epithelium from radiation toxicity, indicating that IDO1 inhibition enhances the radiotherapy effect in CRC (147). According to relevant studies, IDO1 can also cooperate with COL12A1 to promote GC metastasis. This new discovery suggests that IDO1 and COL12A1 may be promising targets for anticancer therapy in GC (148).
Tumor Vaccines
Tumor vaccines are a therapeutic method that can help educate the immune system to recognize cancer-related antigens and achieve antitumor effects. Tumor vaccines mainly include whole-cell vaccines, molecular vaccines and DC vaccines. DCs are one of the most effective APCs found in humans thus far. In addition, DC vaccines have achieved ideal results in clinical trials of malignant melanoma and prostate cancer (149). Due to the lack of MHC molecules and costimulatory molecules located on the surface of tumor cells, T lymphocyte immunity cannot be activated. Therefore, the immune response triggered by tumor vaccines mainly depends on the primary processing and further presentation of antigens by APCs, which is a key step in the development of effective adaptive immunity. As a new treatment strategy, immunotherapy based on tumor vaccines has received increasing attention in the treatment of advanced EC and other GI cancers (124, 150).
Clinical Application of Immunotherapies in Gastrointestinal Cancers
Colorectal Cancer
Immunotherapies have been studied extensively in CRC due to a better understanding of CRC subsets (particularly MSI/MMR status) and how they respond to immune modulation. Initial work on checkpoint inhibitors in CRC showed limited success. Neither the CTLA-4 inhibitor tremelimumab nor the PD-1 inhibitor nivolumab showed an objective response in CRC patients (151, 152). The Check-Mate-142 trial (phase II) evaluated single-agent nivolumab and nivolumab plus ipilimumab in patients with metastatic CRC with either MSI-H/dMMR or MSS/pMMR status. This study first investigated whether MSI-H tumors may be more responsive to immune checkpoint inhibitor therapy (88). Subsequent studies investigated targeting both CTLA-4 and PD-1 with ipilimumab/nivolumab in the pretreated population. The results were encouraging in that the ORR was 55%, the disease control rate for ≥ 12 weeks was 80%, and the progression-free survival (PFS) rates were 76 and 71% (9 and 12 months, respectively) (153). In the KEYNOTE-164 phase II trial, pembrolizumab monotherapy achieved an ORR of 28% and an excellent 1-year OS rate of 72% in heavily pretreated patients with MSI-H mCRC (154) responses to immune checkpoint inhibitors (155, 156).
Various regimens have been implemented to enhance immunogenicity in MSS/pMMR mCRC. The combination of atezolizumab and the MEK inhibitor conbimetinib showed limited effectiveness in CRC patients at advanced stages compared to regorafenib (157). A combination of FOLFOX and PD-1/PD-L1 inhibitors achieved an ORR of up to 50% and increased PFS (from 14 to 16 months) (158, 159). Preliminary data from the AVETUX trial demonstrated that a combination of FOLFOX, cetuximab, and the PD-L1 antibody avelumab showed a 75% ORR in patients with treatment-naïve microsatellite stable (MSS) mCRC (160). In contrast, the combination of atezolizumab (anti-PD-L1) with maintenance therapy with fluoropyrimidines and bevacizumab failed to improve the PFS of mCRC patients (161). In conclusion, all mCRCs should be tested for their microsatellite status. In MSI-H/dMMR CRC, checkpoint inhibitors should be used after therapy with fluoropyrimidine, oxaliplatin, and irinotecan. Currently, the value of checkpoint inhibitors in MSS CRC is still unclear.
Gastroesophageal Cancer
Compared to CRC, the application of immunotherapy has been limited in gastroesophageal cancer due to tumor heterogeneity and complex immunosuppressive mechanisms. Checkpoint inhibition therapy showed some success in early trials, which is likely to be related to a specific phenotype of GC and its histopathology (162).
According to the outcomes of the KEYNOTE-059 trial, pembrolizumab has been approved by the FDA for the management of refractory GC. In addition, a series of KEYNOTE trials have been conducted in EC, including KEYNOTE-180, KEYNOTE-181, and KEYNOTE-590 (90, 163, 164). Nivolumab, based on the outcomes of ATTRACTION-2, was also approved in heavily pretreated tumors in Japan (165). Checkmate-032 confirmed that the combination of ipilimumab with nivolumab was superior to nivolumab monotherapy (166, 167). ATTRACTION-3 compared nivolumab to chemotherapy in refractory OC and demonstrated significant improvement in survival (median OS 10.9 months vs. 8.4 months; hazard ratio for death 0.77, p = 0.019) (168). Understanding the role of immunotherapy in GC has been improved with a shift in the classification of tumors, and four genomic subtypes of GC have been identified. Similar to CRC, the MSI-H population in GC has shown an encouraging response, as indicated by a subset analysis of trials (169). The EBV subtype is even more promising (170), with all patients of this subtype responding to pembrolizumab immunotherapy in a phase II trial (171). Meanwhile, there are still ongoing trials to evaluate the efficacy of different available immunotherapy agents, such as KEYNOTE-062 and KEYNOTE-061 (Table 1).
Concluding Remarks
In summary, this review systematically describes immunological aspects of GI cancer and discusses the latest progress in cancer immunotherapy. Although immune-targeted therapy has gradually become the mainstream strategy for cancer treatment, side effects, drug resistance and a low response rate still represent challenges for researchers and clinicians. In addition, because immunotherapy may not benefit all the cancer types, there are some patient populations who cannot receive immunotherapy. Based on the above, we should strive to improve the understanding of tumor immunology, and the curative effect should be emphasized in further research. Over the next few decades, we expect to see the advent of more effective immunotherapy, the development of predictive biomarkers or more personalized treatment to help patients who are less likely to respond to current treatments to improve the treatment effect.
Author Contributions
D-KW and QZ drafted the manuscript. BL and Q-YH were involved in design of the study and revision of the manuscript. All authors contributed to the article and approved the submitted version.
Funding
This work was supported by National Natural Science Foundation of China (81973339, 31961160727, 81773085, 31770888) and the National Key Research and Development Program of China (2017YFA0505100).
Conflict of Interest
The authors declare that the research was conducted in the absence of any commercial or financial relationships that could be construed as a potential conflict of interest.
Publisher’s Note
All claims expressed in this article are solely those of the authors and do not necessarily represent those of their affiliated organizations, or those of the publisher, the editors and the reviewers. Any product that may be evaluated in this article, or claim that may be made by its manufacturer, is not guaranteed or endorsed by the publisher.
Glossary
References
1. Arnold M, Abnet CC, Neale RE, Vignat J, Giovannucci EL, McGlynn KA, et al. Global Burden of 5 Major Types of Gastrointestinal Cancer. Gastroenterology (2020) 159:335–49.e315. doi: 10.1053/j.gastro.2020.02.068
2. Smyth EC, Moehler M. Late-Line Treatment in Metastatic Gastric Cancer: Today and Tomorrow. Ther Adv Med Oncol (2019) 11:1758835919867522. doi: 10.1177/1758835919867522
3. Daskivich TJ, Belldegrun A. Words of Wisdom. Re: Safety, Activity, and Immune Correlates of Anti-PD-1 Antibody in Cancer. Eur Urol (2015) 67:816–7. doi: 10.1016/j.eururo.2014.12.052
4. Pourhoseingholi MA, Vahedi M, Baghestani AR. Burden of Gastrointestinal Cancer in Asia; An Overview. Gastroenterol Hepatol Bed Bench (2015) 8:19–27. doi: 10.22037/ghfbb.v1i1.608
5. Lorenzen S, Schuster T, Porschen R, Al-Batran SE, Hofheinz R, Thuss-Patience P, et al. Cetuximab Plus Cisplatin-5-Fluorouracil Versus Cisplatin-5-Fluorouracil Alone in First-Line Metastatic Squamous Cell Carcinoma of the Esophagus: A Randomized Phase II Study of the Arbeitsgemeinschaft Internistische Onkologie. Ann Oncol (2009) 20:1667–73. doi: 10.1093/annonc/mdp069
6. Zeng H, Chen W, Zheng R, Zhang S, Ji JS, Zou X, et al. Changing Cancer Survival in China During 2003-15: A Pooled Analysis of 17 Population-Based Cancer Registries. Lancet Glob Health (2018) 6:e555–67. doi: 10.1016/S2214-109X(18)30127-X
7. Bang YJ, Van Cutsem E, Feyereislova A, Chung HC, Shen L, Sawaki A, et al. Trastuzumab in Combination With Chemotherapy Versus Chemotherapy Alone for Treatment of HER2-Positive Advanced Gastric or Gastro-Oesophageal Junction Cancer (ToGA): A Phase 3, Open-Label, Randomised Controlled Trial. Lancet (2010) 376:687–97. doi: 10.1016/S0140-6736(10)61121-X
8. Al-Batran SE, Hartmann JT, Probst S, Schmalenberg H, Hollerbach S, Hofheinz R, et al. Phase III Trial in Metastatic Gastroesophageal Adenocarcinoma With Fluorouracil, Leucovorin Plus Either Oxaliplatin or Cisplatin: A Study of the Arbeitsgemeinschaft Internistische Onkologie. J Clin Oncol (2008) 26:1435–42. doi: 10.1200/JCO.2007.13.9378
9. Albertsson M, Johansson B, Friesland S, Kadar L, Letocha H, Frykholm G, et al. Phase II Studies on Docetaxel Alone Every Third Week, or Weekly in Combination With Gemcitabine in Patients With Primary Locally Advanced, Metastatic, or Recurrent Esophageal Cancer. Med Oncol (2007) 24:407–12. doi: 10.1007/s12032-007-0028-6
10. Bouche O, Raoul JL, Bonnetain F, Giovannini M, Etienne PL, Lledo G, et al. Randomized Multicenter Phase II Trial of a Biweekly Regimen of Fluorouracil and Leucovorin (LV5FU2), LV5FU2 Plus Cisplatin, or LV5FU2 Plus Irinotecan in Patients With Previously Untreated Metastatic Gastric Cancer: A Federation Francophone De Cancerologie Digestive Group Study–FFCD 9803. J Clin Oncol (2004) 22:4319–28. doi: 10.1200/JCO.2004.01.140
11. Zhu J, Tan Z, Hollis-Hansen K, Zhang Y, Yu C, Li Y. Epidemiological Trends in Colorectal Cancer in China: An Ecological Study. Dig Dis Sci (2017) 62:235–43. doi: 10.1007/s10620-016-4362-4
12. Zhang Y, Xu J, Zhang N, Chen M, Wang H, Zhu D. Targeting the Tumour Immune Microenvironment for Cancer Therapy in Human Gastrointestinal Malignancies. Cancer Lett (2019) 458:123–35. doi: 10.1016/j.canlet.2019.05.017
13. Grizzi F, Basso G, Borroni EM, Cavalleri T, Bianchi P, Stifter S, et al. Evolving Notions on Immune Response in Colorectal Cancer and Their Implications for Biomarker Development. Inflamm Res (2018) 67:375–89. doi: 10.1007/s00011-017-1128-1
14. Sanmamed MF, Chen L. A Paradigm Shift in Cancer Immunotherapy: From Enhancement to Normalization. Cell (2018) 175:313–26. doi: 10.1016/j.cell.2018.09.035
15. Forde PM, Chaft JE, Smith KN, Anagnostou V, Cottrell TR, Hellmann MD, et al. Neoadjuvant PD-1 Blockade in Resectable Lung Cancer. N Engl J Med (2018) 378:1976–86. doi: 10.1056/NEJMoa1716078
16. Roelands J, Kuppen PJK, Vermeulen L, Maccalli C, Decock J, Wang E, et al. Immunogenomic Classification of Colorectal Cancer and Therapeutic Implications. Int J Mol Sci (2017) 18:2229. doi: 10.3390/ijms18102229
17. Yang L, Wang Y, Wang H. Use of Immunotherapy in the Treatment of Gastric Cancer. Oncol Lett (2019) 18:5681–90. doi: 10.3892/ol.2019.10935
18. Elinav E, Nowarski R, Thaiss CA, Hu B, Jin C, Flavell RA. Inflammation-Induced Cancer: Crosstalk Between Tumours, Immune Cells and Microorganisms. Nat Rev Cancer (2013) 13:759–71. doi: 10.1038/nrc3611
19. Topalian SL, Taube JM, Anders RA, Pardoll DM. Mechanism-Driven Biomarkers to Guide Immune Checkpoint Blockade in Cancer Therapy. Nat Rev Cancer (2016) 16:275–87. doi: 10.1038/nrc.2016.36
20. Nagarsheth N, Wicha MS, Zou W. Chemokines in the Cancer Microenvironment and Their Relevance in Cancer Immunotherapy. Nat Rev Immunol (2017) 17:559–72. doi: 10.1038/nri.2017.49
21. McAllister SS, Weinberg RA. The Tumour-Induced Systemic Environment as a Critical Regulator of Cancer Progression and Metastasis. Nat Cell Biol (2014) 16:717–27. doi: 10.1038/ncb3015
22. Chen X, Wang L, Li P, Song M, Qin G, Gao Q, et al. Dual TGF-Beta and PD-1 Blockade Synergistically Enhances MAGE-A3-Specific CD8(+) T Cell Response in Esophageal Squamous Cell Carcinoma. Int J Cancer (2018) 143:2561–74. doi: 10.1002/ijc.31730
23. Wang XC, Zhang JQ, Shen YQ, Miao FQ, Xie W. Loss of Heterozygosity at 6p21.3 Underlying HLA Class I Downregulation in Gastric Cancer. J Exp Clin Cancer Res (2006) 25:115–9. doi: 10.1111/j.1399-0039.2008.01078.x
24. Ubukata H, Motohashi G, Tabuchi T, Nagata H, Konishi S, Tabuchi T. Evaluations of Interferon-γ/Interleukin-4 Ratio and Neutrophil/Lymphocyte Ratio as Prognostic Indicators in Gastric Cancer Patients. J Surg Oncol (2010) 102:742–7. doi: 10.1002/jso.21725
25. Galon J, Costes A, Sanchez-Cabo F, Kirilovsky A, Mlecnik B, Lagorce-Pagès C, et al. Type, Density, and Location of Immune Cells Within Human Colorectal Tumors Predict Clinical Outcome. Science (2006) 313:1960–4. doi: 10.1126/science.1129139
26. van Herk EH, Te Velde AA. Treg Subsets in Inflammatory Bowel Disease and Colorectal Carcinoma: Characteristics, Role, and Therapeutic Targets. J Gastroenterol Hepatol (2016) 31:1393–404. doi: 10.1111/jgh.13342
27. Correale P, Rotundo MS, Del Vecchio MT, Remondo C, Migali C, Ginanneschi C, et al. Regulatory (FoxP3+) T-Cell Tumor Infiltration Is a Favorable Prognostic Factor in Advanced Colon Cancer Patients Undergoing Chemo or Chemoimmunotherapy. J Immunother (2010) 33:435–41. doi: 10.1097/CJI.0b013e3181d32f01
28. Ichihara F, Kono K, Takahashi A, Kawaida H, Sugai H, Fujii H. Increased Populations of Regulatory T Cells in Peripheral Blood and Tumor-Infiltrating Lymphocytes in Patients With Gastric and Esophageal Cancers. Clin Cancer Res (2003) 9:4404–8.
29. Ishigami S, Natsugoe S, Tokuda K, Nakajo A, Xiangming C, Iwashige H, et al. Clinical Impact of Intratumoral Natural Killer Cell and Dendritic Cell Infiltration in Gastric Cancer. Cancer Lett (2000) 159:103–8. doi: 10.1016/S0304-3835(00)00542-5
30. Yamazaki S, Iyoda T, Tarbell K, Olson K, Velinzon K, Inaba K, et al. Direct Expansion of Functional CD25+ CD4+ Regulatory T Cells by Antigen-Processing Dendritic Cells. J Exp Med (2003) 198:235–47. doi: 10.1084/jem.20030422
31. Dijkgraaf EM, Heusinkveld M, Tummers B, Vogelpoel LT, Goedemans R, Jha V, et al. Chemotherapy Alters Monocyte Differentiation to Favor Generation of Cancer-Supporting M2 Macrophages in the Tumor Microenvironment. Cancer Res (2013) 73:2480–92. doi: 10.1158/0008-5472.CAN-12-3542
32. Ruffell B, Chang-Strachan D, Chan V, Rosenbusch A, Ho CM, Pryer N, et al. Macrophage IL-10 Blocks CD8+ T Cell-Dependent Responses to Chemotherapy by Suppressing IL-12 Expression in Intratumoral Dendritic Cells. Cancer Cell (2014) 26:623–37. doi: 10.1016/j.ccell.2014.09.006
33. Coussens LM, Pollard JW. Leukocytes in Mammary Development and Cancer. Cold Spring Harb Perspect Biol (2011) 3:a003285. doi: 10.1101/cshperspect.a003285
34. Inman JL, Robertson C, Mott JD, Bissell MJ. Mammary Gland Development: Cell Fate Specification, Stem Cells and the Microenvironment. Development (2015) 142:1028–42. doi: 10.1242/dev.087643
35. Arango Duque G, Descoteaux A. Macrophage Cytokines: Involvement in Immunity and Infectious Diseases. Front Immunol (2014) 5:491. doi: 10.3389/fimmu.2014.00491
36. Larionova I, Cherdyntseva N, Liu T, Patysheva M, Rakina M, Kzhyshkowska J. Interaction of Tumor-Associated Macrophages and Cancer Chemotherapy. Oncoimmunology (2019) 8:1596004. doi: 10.1080/2162402X.2019.1596004
37. Barbera-Guillem E, Nyhus JK, Wolford CC, Friece CR, Sampsel JW. Vascular Endothelial Growth Factor Secretion by Tumor-Infiltrating Macrophages Essentially Supports Tumor Angiogenesis, and IgG Immune Complexes Potentiate the Process. Cancer Res (2002) 62:7042–9.
38. Peddareddigari VG, Wang D, Dubois RN. The Tumor Microenvironment in Colorectal Carcinogenesis. Cancer Microenviron (2010) 3:149–66. doi: 10.1007/s12307-010-0038-3
39. Torres S, Bartolomé RA, Mendes M, Barderas R, Fernandez-Aceñero MJ, Peláez-García A, et al. Proteome Profiling of Cancer-Associated Fibroblasts Identifies Novel Proinflammatory Signatures and Prognostic Markers for Colorectal Cancer. Clin Cancer Res (2013) 19:6006–19. doi: 10.1158/1078-0432.CCR-13-1130
40. Gershkovitz M, Caspi Y, Fainsod-Levi T, Katz B, Michaeli J, Khawaled S, et al. TRPM2 Mediates Neutrophil Killing of Disseminated Tumor Cells. Cancer Res (2018) 78:2680–90. doi: 10.1158/0008-5472.CAN-17-3614
41. Finisguerra V, Di Conza G, Di Matteo M, Serneels J, Costa S, Thompson AA, et al. MET Is Required for the Recruitment of Anti-Tumoural Neutrophils. Nature (2015) 522:349–53. doi: 10.1038/nature14407
42. Ardi VC, Kupriyanova TA, Deryugina EI, Quigley JP. Human Neutrophils Uniquely Release TIMP-Free MMP-9 to Provide a Potent Catalytic Stimulator of Angiogenesis. Proc Natl Acad Sci USA (2007) 104:20262–7. doi: 10.1073/pnas.0706438104
43. Giese MA, Hind LE, Huttenlocher A. Neutrophil Plasticity in the Tumor Microenvironment. Blood (2019) 133:2159–67. doi: 10.1182/blood-2018-11-844548
44. Tohme S, Yazdani HO, Al-Khafaji AB, Chidi AP, Loughran P, Mowen K, et al. Neutrophil Extracellular Traps Promote the Development and Progression of Liver Metastases After Surgical Stress. Cancer Res (2016) 76:1367–80. doi: 10.1158/0008-5472.CAN-15-1591
45. Berntsson J, Svensson MC, Leandersson K, Nodin B, Micke P, Larsson AH, et al. The Clinical Impact of Tumour-Infiltrating Lymphocytes in Colorectal Cancer Differs by Anatomical Subsite: A Cohort Study. Int J Cancer (2017) 141:1654–66. doi: 10.1002/ijc.30869
46. de Visser KE, Korets LV, Coussens LM. De Novo Carcinogenesis Promoted by Chronic Inflammation Is B Lymphocyte Dependent. Cancer Cell (2005) 7:411–23. doi: 10.1016/j.ccr.2005.04.014
47. He Y, Qian H, Liu Y, Duan L, Li Y, Shi G. The Roles of Regulatory B Cells in Cancer. J Immunol Res (2014) 2014:215471. doi: 10.1155/2014/215471
48. Schioppa T, Moore R, Thompson RG, Rosser EC, Kulbe H, Nedospasov S, et al. B Regulatory Cells and the Tumor-Promoting Actions of TNF-Alpha During Squamous Carcinogenesis. Proc Natl Acad Sci USA (2011) 108:10662–7. doi: 10.1073/pnas.1100994108
49. Berntsson J, Nodin B, Eberhard J, Micke P, Jirström K. Prognostic Impact of Tumour-Infiltrating B Cells and Plasma Cells in Colorectal Cancer. Int J Cancer (2016) 139:1129–39. doi: 10.1002/ijc.30138
50. Lim KS, Mimura K, Kua LF, Shiraishi K, Kono K. Implication of Highly Cytotoxic Natural Killer Cells for Esophageal Squamous Cell Carcinoma Treatment. J Immunother (2018) 41:261–73. doi: 10.1097/CJI.0000000000000227
51. Peng LS, Zhang JY, Teng YS, Zhao YL, Wang TT, Mao FY, et al. Tumor-Associated Monocytes/Macrophages Impair NK-Cell Function Via Tgfβ1 in Human Gastric Cancer. Cancer Immunol Res (2017) 5:248–56. doi: 10.1158/2326-6066.CIR-16-0152
52. Fisher DT, Appenheimer MM, Evans SS. The Two Faces of IL-6 in the Tumor Microenvironment. Semin Immunol (2014) 26:38–47. doi: 10.1016/j.smim.2014.01.008
53. Mannino MH, Zhu Z, Xiao H, Bai Q, Wakefield MR, Fang Y. The Paradoxical Role of IL-10 in Immunity and Cancer. Cancer Lett (2015) 367:103–7. doi: 10.1016/j.canlet.2015.07.009
54. Choi JN, Sun EG, Cho SH. IL-12 Enhances Immune Response by Modulation of Myeloid Derived Suppressor Cells in Tumor Microenvironment. Chonnam Med J (2019) 55:31–9. doi: 10.4068/cmj.2019.55.1.31
55. Turnis ME, Sawant DV, Szymczak-Workman AL, Andrews LP, Delgoffe GM, Yano H, et al. Interleukin-35 Limits Anti-Tumor Immunity. Immunity (2016) 44:316–29. doi: 10.1016/j.immuni.2016.01.013
56. Josephs SF, Ichim TE, Prince SM, Kesari S, Marincola FM, Escobedo AR, et al. Unleashing Endogenous TNF-Alpha as a Cancer Immunotherapeutic. J Transl Med (2018) 16:242. doi: 10.1186/s12967-018-1611-7
57. Burke JD, Young HA. IFN-γ: A Cytokine at the Right Time, Is in the Right Place. Semin Immunol (2019) 43:101280. doi: 10.1016/j.smim.2019.05.002
58. Munn DH, Mellor AL. IDO in the Tumor Microenvironment: Inflammation, Counter-Regulation, and Tolerance. Trends Immunol (2016) 37:193–207. doi: 10.1016/j.it.2016.01.002
59. Batlle E, Massagué J. Transforming Growth Factor-β Signaling in Immunity and Cancer. Immunity (2019) 50:924–40. doi: 10.1016/j.immuni.2019.03.024
60. Mills CD, Ley K. M1 and M2 Macrophages: The Chicken and the Egg of Immunity. J Innate Immun (2014) 6:716–26. doi: 10.1159/000364945
61. Siveen KS, Kuttan G. Role of Macrophages in Tumour Progression. Immunol Lett (2009) 123:97–102. doi: 10.1016/j.imlet.2009.02.011
62. Schmieder A, Michel J, Schonhaar K, Goerdt S, Schledzewski K. Differentiation and Gene Expression Profile of Tumor-Associated Macrophages. Semin Cancer Biol (2012) 22:289–97. doi: 10.1016/j.semcancer.2012.02.002
63. Popena I, Abols A, Saulite L, Pleiko K, Zandberga E, Jekabsons K, et al. Effect of Colorectal Cancer-Derived Extracellular Vesicles on the Immunophenotype and Cytokine Secretion Profile of Monocytes and Macrophages. Cell Commun Signal (2018) 16:17. doi: 10.1186/s12964-018-0229-y
64. Gambardella V, Castillo J, Tarazona N, Gimeno-Valiente F, Martinez-Ciarpaglini C, Cabeza-Segura M, et al. The Role of Tumor-Associated Macrophages in Gastric Cancer Development and Their Potential as a Therapeutic Target. Cancer Treat Rev (2020) 86:102015. doi: 10.1016/j.ctrv.2020.102015
65. Yang H, Zhang Q, Xu M, Wang L, Chen X, Feng Y, et al. CCL2-CCR2 Axis Recruits Tumor Associated Macrophages to Induce Immune Evasion Through PD-1 Signaling in Esophageal Carcinogenesis. Mol Cancer (2020) 19:41. doi: 10.1186/s12943-020-01165-x
66. Joshi RS, Kanugula SS, Sudhir S, Pereira MP, Jain S, Aghi MK. The Role of Cancer-Associated Fibroblasts in Tumor Progression. Cancers (Basel) (2021) 13:1399. doi: 10.3390/cancers13061399
67. Smith HA, Kang Y. The Metastasis-Promoting Roles of Tumor-Associated Immune Cells. J Mol Med (Berl) (2013) 91:411–29. doi: 10.1007/s00109-013-1021-5
68. Bonnans C, Chou J, Werb Z. Remodelling the Extracellular Matrix in Development and Disease. Nat Rev Mol Cell Biol (2014) 15:786–801. doi: 10.1038/nrm3904
69. Landskron G, de la Fuente M, Thuwajit P, Thuwajit C, Hermoso MA. Chronic Inflammation and Cytokines in the Tumor Microenvironment. J Immunol Res (2014) 2014:149185. doi: 10.1155/2014/149185
70. Chang CP, Hu MH, Hsiao YP, Wang YC. ST2 Signaling in the Tumor Microenvironment. Adv Exp Med Biol (2020) 1240:83–93. doi: 10.1007/978-3-030-38315-2_7
71. Unsworth A, Anderson R, Britt K. Stromal Fibroblasts and the Immune Microenvironment: Partners in Mammary Gland Biology and Pathology? J Mammary Gland Biol Neoplasia (2014) 19:169–82. doi: 10.1007/s10911-014-9326-8
72. Xing F, Saidou J, Watabe K. Cancer Associated Fibroblasts (CAFs) in Tumor Microenvironment. Front Biosci (Landmark Ed) (2010) 15:166–79. doi: 10.2741/3613
73. Freeman P, Mielgo A. Cancer-Associated Fibroblast Mediated Inhibition of CD8+ Cytotoxic T Cell Accumulation in Tumours: Mechanisms and Therapeutic Opportunities. Cancers (Basel) (2020) 12:2687. doi: 10.3390/cancers12092687
74. Mari L, Hoefnagel SJM, Zito D, van de Meent M, van Endert P, Calpe S, et al. microRNA 125a Regulates MHC-I Expression on Esophageal Adenocarcinoma Cells, Associated With Suppression of Antitumor Immune Response and Poor Outcomes of Patients. Gastroenterology (2018) 155:784–98. doi: 10.1053/j.gastro.2018.06.030
75. Zaretsky JM, Garcia-Diaz A, Shin DS, Escuin-Ordinas H, Hugo W, Hu-Lieskovan S, et al. Mutations Associated With Acquired Resistance to PD-1 Blockade in Melanoma. N Engl J Med (2016) 375:819–29. doi: 10.1056/NEJMoa1604958
76. Guo F, Wang Y, Liu J, Mok S, Xue F, Zhang W. CXCL12/CXCR4: A Symbiotic Bridge Linking Cancer Cells and Their Stromal Neighbors in Oncogenic Communication Networks. Oncogene (2016) 35:816–26. doi: 10.1038/onc.2015.139
77. Chen DS, Mellman I. Elements of Cancer Immunity and the Cancer-Immune Set Point. Nature (2017) 541:321–30. doi: 10.1038/nature21349
78. Spranger S, Gajewski TF. Impact of Oncogenic Pathways on Evasion of Antitumour Immune Responses. Nat Rev Cancer (2018) 18:139–47. doi: 10.1038/nrc.2017.117
79. Mumm JB, Emmerich J, Zhang X, Chan I, Wu L, Mauze S, et al. IL-10 Elicits IFNgamma-Dependent Tumor Immune Surveillance. Cancer Cell (2011) 20:781–96. doi: 10.1016/j.ccr.2011.11.003
80. Fioravanti J, Di Lucia P, Magini D, Moalli F, Boni C, Benechet AP, et al. Effector CD8(+) T Cell-Derived Interleukin-10 Enhances Acute Liver Immunopathology. J Hepatol (2017) 67:543–8. doi: 10.1016/j.jhep.2017.04.020
81. Naing A, Papadopoulos KP, Autio KA, Ott PA, Patel MR, Wong DJ, et al. Safety, Antitumor Activity, and Immune Activation of Pegylated Recombinant Human Interleukin-10 (AM0010) in Patients With Advanced Solid Tumors. J Clin Oncol (2016) 34:3562–9. doi: 10.1200/JCO.2016.68.1106
82. Zhao Q. Dual Targeting of CCR2 and CCR5: Therapeutic Potential for Immunologic and Cardiovascular Diseases. J Leukoc Biol (2010) 88:41–55. doi: 10.1189/jlb.1009671
83. Buchbinder E, Hodi FS. Cytotoxic T Lymphocyte Antigen-4 and Immune Checkpoint Blockade. J Clin Invest (2015) 125:3377–83. doi: 10.1172/JCI80012
84. Zhang C, Zhang J, Ma Y, Zhe H, Zhao R, Wang Y. Prognostic Value of Combined Analysis of CTLA-4 and PLR in Esophageal Squamous Cell Carcinoma (ESCC) Patients. Dis Markers (2019) 2019:1601072–1601072. doi: 10.1155/2019/1601072
85. Kim DW, Trinh VA, Hwu WJ. Ipilimumab in the Treatment of Advanced Melanoma - A Clinical Update. Expert Opin Biol Ther (2014) 14:1709–18. doi: 10.1517/14712598.2014.963053
86. Toh JWT, de Souza P, Lim SH, Singh P, Chua W, Ng W, et al. The Potential Value of Immunotherapy in Colorectal Cancers: Review of the Evidence for Programmed Death-1 Inhibitor Therapy. Clin Colorectal Cancer (2016) 15:285–91. doi: 10.1016/j.clcc.2016.07.007
87. Topalian SL, Taube JM, Pardoll DM. Neoadjuvant Checkpoint Blockade for Cancer Immunotherapy. Science (2020) 367:6477. doi: 10.1126/science.aax0182
88. Overman MJ, McDermott R, Leach JL, Lonardi S, Lenz HJ, Morse MA, et al. Nivolumab in Patients With Metastatic DNA Mismatch Repair-Deficient or Microsatellite Instability-High Colorectal Cancer (CheckMate 142): An Open-Label, Multicentre, Phase 2 Study. Lancet Oncol (2017) 18:1182–91. doi: 10.1016/S1470-2045(17)30422-9
89. Saito R, Abe H, Kunita A, Yamashita H, Seto Y, Fukayama M. Overexpression and Gene Amplification of PD-L1 in Cancer Cells and PD-L1(+) Immune Cells in Epstein-Barr Virus-Associated Gastric Cancer: The Prognostic Implications. Mod Pathol (2017) 30:427–39. doi: 10.1038/modpathol.2016.202
90. Kato K, Shah MA, Enzinger P, Bennouna J, Shen L, Adenis A, et al. KEYNOTE-590: Phase III Study of First-Line Chemotherapy With or Without Pembrolizumab for Advanced Esophageal Cancer. Future Oncol (2019) 15:1057–66. doi: 10.2217/fon-2018-0609
91. Metges JP, Francois E, Shah MA, Adenis A, Enzinger PC, Kojima T, et al. The Phase 3 KEYNOTE-181 Study: Pembrolizumab Versus Chemotherapy as Second-Line Therapy for Advanced Esophageal Cancer. Ann Oncol (2019) 30:iv130. doi: 10.1093/annonc/mdz154.011
92. Shan B, Man H, Liu J, Wang L, Zhu T, Ma M, et al. TIM-3 Promotes the Metastasis of Esophageal Squamous Cell Carcinoma by Targeting Epithelial-Mesenchymal Transition Via the Akt/GSK-3β/Snail Signaling Pathway. Oncol Rep (2016) 36:1551–61. doi: 10.3892/or.2016.4938
93. Yu J, Zhang H, Sun S, Sun S, Li L. The Effects of Tim-3 Activation on T-Cells in Gastric Cancer Progression. Oncol Lett (2019) 17:1461–6. doi: 10.3892/ol.2018.9743
94. Zhang P, Wang Y, Liu XR, Hong SR, Yao J. Downregulated Tim-3 Expression Is Responsible for the Incidence and Development of Colorectal Cancer. Oncol Lett (2018) 16:1059–66. doi: 10.3892/ol.2018.8697
95. Andrews LP, Marciscano AE, Drake CG, Vignali DA. LAG3 (CD223) as a Cancer Immunotherapy Target. Immunol Rev (2017) 276:80–96. doi: 10.1111/imr.12519
96. Zhang Y, Liu Y, Luo Y, Liu B, Huang Q, Wang F, et al. Prognostic Value of Lymphocyte Activation Gene-3 (LAG-3) Expression in Esophageal Squamous Cell Carcinoma. J Cancer (2018) 9:4287–93. doi: 10.7150/jca.26949
97. Saleh R, Taha RZ, Toor SM, Sasidharan Nair V, Murshed K, Khawar M, et al. Expression of Immune Checkpoints and T Cell Exhaustion Markers in Early and Advanced Stages of Colorectal Cancer. Cancer Immunol Immunother (2020) 69:1989–99. doi: 10.1007/s00262-020-02593-w
98. Zhang W, Huang Q, Xiao W, Zhao Y, Pi J, Xu H, et al. Advances in Anti-Tumor Treatments Targeting the CD47/SIRPalpha Axis. Front Immunol (2020) 11:18. doi: 10.3389/fimmu.2020.00018
99. Zhao CL, Yu S, Wang SH, Li SG, Wang ZJ, Han SN. Characterization of Cluster of Differentiation 47 Expression and Its Potential as a Therapeutic Target in Esophageal Squamous Cell Cancer. Oncol Lett (2017) 15:2017–23. doi: 10.3892/ol.2017.7447
100. Yoshida K, Tsujimoto H, Matsumura K, Kinoshita M, Takahata R, Matsumoto Y, et al. CD47 Is an Adverse Prognostic Factor and a Therapeutic Target in Gastric Cancer. Cancer Med (2015) 4:1322–33. doi: 10.1002/cam4.478
101. Tzatzarakis E, Hissa B, Reissfelder C, Schölch S. The Overall Potential of CD47 in Cancer Immunotherapy: With a Focus on Gastrointestinal Tumors. Expert Rev Anticancer Ther (2019) 19:993–9. doi: 10.1080/14737140.2019.1689820
102. Sadelain M, Brentjens R, Riviere I. The Basic Principles of Chimeric Antigen Receptor Design. Cancer Discov (2013) 3:388–98. doi: 10.1158/2159-8290.CD-12-0548
103. Sandlund JT, Martin MG. Non-Hodgkin Lymphoma Across the Pediatric and Adolescent and Young Adult Age Spectrum. Hematology Am Soc Hematology Educ Program (2016) 2016:589–97. doi: 10.1182/asheducation-2016.1.589
104. Al-Mansour M, Al-Foheidi M, Ibrahim E. Efficacy and Safety of Second-Generation CAR T-Cell Therapy in Diffuse Large B-Cell Lymphoma: A Meta-Analysis. Mol Clin Oncol (2020) 13:33. doi: 10.3892/mco.2020.2103
105. Shi H, Yu F, Mao Y, Ju Q, Wu Y, Bai W, et al. EphA2 Chimeric Antigen Receptor-Modified T Cells for the Immunotherapy of Esophageal Squamous Cell Carcinoma. J Thorac Dis (2018) 10:2779–88. doi: 10.21037/jtd.2018.04.91
106. Zhang Q, Zhang Z, Peng M, Fu S, Xue Z, Zhang R. CAR-T Cell Therapy in Gastrointestinal Tumors and Hepatic Carcinoma: From Bench to Bedside. Oncoimmunology (2016) 5:e1251539. doi: 10.1080/2162402X.2016.1251539
107. Holzinger A, Abken H. CAR T Cells Targeting Solid Tumors: Carcinoembryonic Antigen (CEA) Proves to be a Safe Target. Cancer Immunol Immunother (2017) 66:1505–7. doi: 10.1007/s00262-017-2045-4
108. Chi X, Yang P, Zhang E, Gu J, Xu H, Li M, et al. Significantly Increased Anti-Tumor Activity of Carcinoembryonic Antigen-Specific Chimeric Antigen Receptor T Cells in Combination With Recombinant Human IL-12. Cancer Med (2019) 8:4753–65. doi: 10.1002/cam4.2361
109. Zhou JT, Liu JH, Song TT, Ma B, Amidula N, Bai C. EGLIF-CAR-T Cells Secreting PD-1 Blocking Antibodies Significantly Mediate the Elimination of Gastric Cancer. Cancer Manag Res (2020) 12:8893–902. doi: 10.2147/CMAR.S260915
110. Tabernero J, Hoff PM, Shen L, Ohtsu A, Shah MA, Cheng K, et al. Pertuzumab Plus Trastuzumab and Chemotherapy for HER2-Positive Metastatic Gastric or Gastro-Oesophageal Junction Cancer (JACOB): Final Analysis of a Double-Blind, Randomised, Placebo-Controlled Phase 3 Study. Lancet Oncol (2018) 19:1372–84. doi: 10.1016/S1470-2045(18)30481-9
111. Chester C, Fritsch K, Kohrt HE. Natural Killer Cell Immunomodulation: Targeting Activating, Inhibitory, and Co-Stimulatory Receptor Signaling for Cancer Immunotherapy. Front Immunol (2015) 6:601. doi: 10.3389/fimmu.2015.00601
112. Hasmim M, Messai Y, Ziani L, Thiery J, Bouhris JH, Noman MZ, et al. Critical Role of Tumor Microenvironment in Shaping NK Cell Functions: Implication of Hypoxic Stress. Front Immunol (2015) 6:482. doi: 10.3389/fimmu.2015.00482
113. Alici E, Konstantinidis KV, Sutlu T, Aints A, Gahrton G, Ljunggren HG, et al. Anti-Myeloma Activity of Endogenous and Adoptively Transferred Activated Natural Killer Cells in Experimental Multiple Myeloma Model. Exp Hematol (2007) 35:1839–46. doi: 10.1016/j.exphem.2007.08.006
114. Lin C, Zhang J. Reformation in Chimeric Antigen Receptor Based Cancer Immunotherapy: Redirecting Natural Killer Cell. Biochim Biophys Acta Rev Cancer (2018) 1869:200–15. doi: 10.1016/j.bbcan.2018.01.005
115. Siegler U, Kalberer CP, Nowbakht P, Sendelov S, Meyer-Monard S, Wodnar-Filipowicz A. Activated Natural Killer Cells From Patients With Acute Myeloid Leukemia Are Cytotoxic Against Autologous Leukemic Blasts in NOD/SCID Mice. Leukemia (2005) 19:2215–22. doi: 10.1038/sj.leu.2403985
116. Bendelac A, Savage PB, Teyton L. The Biology of NKT Cells. Annu Rev Immunol (2007) 25:297–336. doi: 10.1146/annurev.immunol.25.022106.141711
117. McEwen-Smith RM, Salio M, Cerundolo V. The Regulatory Role of Invariant NKT Cells in Tumor Immunity. Cancer Immunol Res (2015) 3:425–35. doi: 10.1158/2326-6066.CIR-15-0062
118. Wolf BJ, Choi JE, Exley MA. Novel Approaches to Exploiting Invariant NKT Cells in Cancer Immunotherapy. Front Immunol (2018) 9:384. doi: 10.3389/fimmu.2018.00384
119. Coquet JM, Kyparissoudis K, Pellicci DG, Besra G, Berzins SP, Smyth MJ, et al. IL-21 Is Produced by NKT Cells and Modulates NKT Cell Activation and Cytokine Production. J Immunol (2007) 178:2827–34. doi: 10.4049/jimmunol.178.5.2827
120. Heczey A, Liu D, Tian G, Courtney AN, Wei J, Marinova E, et al. Invariant NKT Cells With Chimeric Antigen Receptor Provide a Novel Platform for Safe and Effective Cancer Immunotherapy. Blood (2014) 124:2824–33. doi: 10.1182/blood-2013-11-541235
121. Crowe NY, Uldrich AP, Kyparissoudis K, Hammond KJ, Hayakawa Y, Sidobre S, et al. Glycolipid Antigen Drives Rapid Expansion and Sustained Cytokine Production by NK T Cells. J Immunol (2003) 171:4020–7. doi: 10.4049/jimmunol.171.8.4020
122. Yamasaki K, Horiguchi S, Kurosaki M, Kunii N, Nagato K, Hanaoka H, et al. Induction of NKT Cell-Specific Immune Responses in Cancer Tissues After NKT Cell-Targeted Adoptive Immunotherapy. Clin Immunol (2011) 138:255–65. doi: 10.1016/j.clim.2010.11.014
123. Kunii N, Horiguchi S, Motohashi S, Yamamoto H, Ueno N, Yamamoto S, et al. Combination Therapy of In Vitro-Expanded Natural Killer T Cells and Alpha-Galactosylceramide-Pulsed Antigen-Presenting Cells in Patients With Recurrent Head and Neck Carcinoma. Cancer Sci (2009) 100:1092–8. doi: 10.1111/j.1349-7006.2009.01135.x
124. Nywening TM, Wang-Gillam A, Sanford DE, Belt BA, Panni RZ, Cusworth BM, et al. Targeting Tumour-Associated Macrophages With CCR2 Inhibition in Combination With FOLFIRINOX in Patients With Borderline Resectable and Locally Advanced Pancreatic Cancer: A Single-Centre, Open-Label, Dose-Finding, Non-Randomised, Phase 1b Trial. Lancet Oncol (2016) 17:651–62. doi: 10.1016/S1470-2045(16)00078-4
125. Cannarile MA, Weisser M, Jacob W, Jegg AM, Ries CH, Rüttinger D. Colony-Stimulating Factor 1 Receptor (CSF1R) Inhibitors in Cancer Therapy. J Immunother Cancer (2017) 5:53. doi: 10.1186/s40425-017-0257-y
126. Ruffell B, Coussens LM. Macrophages and Therapeutic Resistance in Cancer. Cancer Cell (2015) 27:462–72. doi: 10.1016/j.ccell.2015.02.015
127. Quail DF, Bowman RL, Akkari L, Quick ML, Schuhmacher AJ, Huse JT, et al. The Tumor Microenvironment Underlies Acquired Resistance to CSF-1R Inhibition in Gliomas. Science (2016) 352:aad3018. doi: 10.1126/science.aad3018
128. Li X, Liu R, Su X, Pan Y, Han X, Shao C, et al. Harnessing Tumor-Associated Macrophages as Aids for Cancer Immunotherapy. Mol Cancer (2019) 18:177. doi: 10.1186/s12943-019-1102-3
129. Ries CH, Cannarile MA, Hoves S, Benz J, Wartha K, Runza V, et al. Targeting Tumor-Associated Macrophages With Anti-CSF-1R Antibody Reveals a Strategy for Cancer Therapy. Cancer Cell (2014) 25:846–59. doi: 10.1016/j.ccr.2014.05.016
130. Pyonteck SM, Akkari L, Schuhmacher AJ, Bowman RL, Sevenich L, Quail DF, et al. CSF-1R Inhibition Alters Macrophage Polarization and Blocks Glioma Progression. Nat Med (2013) 19:1264–72. doi: 10.1038/nm.3337
131. Willingham SB, Volkmer JP, Gentles AJ, Sahoo D, Dalerba P, Mitra SS, et al. The CD47-Signal Regulatory Protein Alpha (SIRPa) Interaction Is a Therapeutic Target for Human Solid Tumors. Proc Natl Acad Sci USA (2012) 109:6662–7. doi: 10.1073/pnas.1121623109
132. Kabelitz D, Wesch D, He W. Perspectives of Gammadelta T Cells in Tumor Immunology. Cancer Res (2007) 67:5–8. doi: 10.1158/0008-5472.CAN-06-3069
133. Scotet E, Martinez LO, Grant E, Barbaras R, Jenö P, Guiraud M, et al. Tumor Recognition Following Vgamma9Vdelta2 T Cell Receptor Interactions With a Surface F1-ATPase-Related Structure and Apolipoprotein A-I. Immunity (2005) 22:71–80. doi: 10.1016/j.immuni.2004.11.012
134. Chen H, He X, Wang Z, Wu D, Zhang H, Xu C, et al. Identification of Human T Cell Receptor Gammadelta-Recognized Epitopes/Proteins Via CDR3delta Peptide-Based Immunobiochemical Strategy. J Biol Chem (2008) 283:12528–37. doi: 10.1074/jbc.M708067200
135. Hayday AC. [Gamma][Delta] Cells: A Right Time and a Right Place for a Conserved Third Way of Protection. Annu Rev Immunol (2000) 18:975–1026. doi: 10.1146/annurev.immunol.18.1.975
136. Lin C, Zhang J. Chimeric Antigen Receptor Engineered Innate Immune Cells in Cancer Immunotherapy. Sci China Life Sci (2019) 62:633–9. doi: 10.1007/s11427-018-9451-0
137. Rock EP, Sibbald PR, Davis MM, Chien YH. CDR3 Length in Antigen-Specific Immune Receptors. J Exp Med (1994) 179:323–8. doi: 10.1084/jem.179.1.323
138. Wu YL, Ding YP, Tanaka Y, Shen LW, Wei CH, Minato N, et al. γδ T Cells and Their Potential for Immunotherapy. Int J Biol Sci (2014) 10:119–35. doi: 10.7150/ijbs.7823
139. Khairallah C, Déchanet-Merville J, Capone M. γδ T Cell-Mediated Immunity to Cytomegalovirus Infection. Front Immunol (2017) 8:105. doi: 10.3389/fimmu.2017.00105
140. Mamedov MR, Scholzen A, Nair RV, Cumnock K, Kenkel JA, Oliveira JHM, et al. A Macrophage Colony-Stimulating-Factor-Producing γδ T Cell Subset Prevents Malarial Parasitemic Recurrence. Immunity (2018) 48:350–63.e357. doi: 10.1016/j.immuni.2018.01.009
141. Born WK, Reardon CL, O’Brien RL. The Function of Gammadelta T Cells in Innate Immunity. Curr Opin Immunol (2006) 18:31–8. doi: 10.1016/j.coi.2005.11.007
142. Morrow ES, Roseweir A, Edwards J. The Role of Gamma Delta T Lymphocytes in Breast Cancer: A Review. Transl Res (2019) 203:88–96. doi: 10.1016/j.trsl.2018.08.005
143. Capsomidis A, Benthall G, Van Acker HH, Fisher J, Kramer AM, Abeln Z, et al. Chimeric Antigen Receptor-Engineered Human Gamma Delta T Cells: Enhanced Cytotoxicity With Retention of Cross Presentation. Mol Ther (2018) 26:354–65. doi: 10.1016/j.ymthe.2017.12.001
144. Liu M, Wang X, Wang L, Ma X, Gong Z, Zhang S, et al. Targeting the IDO1 Pathway in Cancer: From Bench to Bedside. J Hematol Oncol (2018) 11:100. doi: 10.1186/s13045-018-0644-y
145. Kiyozumi Y, Baba Y, Okadome K, Yagi T, Ishimoto T, Iwatsuki M, et al. IDO1 Expression Is Associated With Immune Tolerance and Poor Prognosis in Patients With Surgically Resected Esophageal Cancer. Ann Surg (2019) 269:1101–8. doi: 10.1097/SLA.0000000000002754
146. Zhou S, Zhao L, Liang Z, Liu S, Li Y, Liu S, et al. Indoleamine 2,3-Dioxygenase 1 and Programmed Cell Death-Ligand 1 Co-Expression Predicts Poor Pathologic Response and Recurrence in Esophageal Squamous Cell Carcinoma After Neoadjuvant Chemoradiotherapy. Cancers (Basel) (2019) 11:169. doi: 10.3390/cancers11020169
147. Chen B, Alvarado DM, Iticovici M, Kau NS, Park H, Parikh PJ, et al. Interferon-Induced IDO1 Mediates Radiation Resistance and Is a Therapeutic Target in Colorectal Cancer. Cancer Immunol Res (2020) 8:451–64. doi: 10.1158/2326-6066.CIR-19-0282
148. Xiang Z, Li J, Song S, Wang J, Cai W, Hu W, et al. A Positive Feedback Between IDO1 Metabolite and COL12A1 Via MAPK Pathway to Promote Gastric Cancer Metastasis. J Exp Clin Cancer Res (2019) 38:314. doi: 10.1186/s13046-019-1318-5
149. Ozao-Choy J, Lee DJ, Faries MB. Melanoma Vaccines: Mixed Past, Promising Future. Surg Clinics North Am (2014) 94:1017–1030, viii. doi: 10.1016/j.suc.2014.07.005
150. Bouzid R, Peppelenbosch M, Buschow SI. Opportunities for Conventional and in Situ Cancer Vaccine Strategies and Combination With Immunotherapy for Gastrointestinal Cancers, A Review. Cancers (Basel) (2020) 12:1121. doi: 10.3390/cancers12051121
151. Topalian SL, Hodi FS, Brahmer JR, Gettinger SN, Smith DC, McDermott DF, et al. Safety, Activity, and Immune Correlates of Anti-PD-1 Antibody in Cancer. N Engl J Med (2012) 366:2443–54. doi: 10.1056/NEJMoa1200690
152. Chung KY, Gore I, Fong L, Venook A, Beck SB, Dorazio P, et al. Phase II Study of the Anti-Cytotoxic T-Lymphocyte-Associated Antigen 4 Monoclonal Antibody, Tremelimumab, in Patients With Refractory Metastatic Colorectal Cancer. J Clin Oncol (2010) 28:3485–90. doi: 10.1200/JCO.2010.28.3994
153. Overman MJ, Lonardi S, Wong KYM, Lenz HJ, Gelsomino F, Aglietta M, et al. Durable Clinical Benefit With Nivolumab Plus Ipilimumab in DNA Mismatch Repair-Deficient/Microsatellite Instability-High Metastatic Colorectal Cancer. J Clin Oncol (2018) 36:773–9. doi: 10.1200/JCO.2017.76.9901
154. Middha S, Zhang L, Nafa K, Jayakumaran G, Wong D, Kim HR, et al. Reliable Pan-Cancer Microsatellite Instability Assessment by Using Targeted Next-Generation Sequencing Data. JCO Precis Oncol (2017) 1:1–17. doi: 10.1200/PO.17.00084
155. Asaoka Y, Ijichi H, Koike K. PD-1 Blockade in Tumors With Mismatch-Repair Deficiency. N Engl J Med (2015) 373:1979. doi: 10.1056/NEJMc1510353
156. Morse MA, Overman MJ, Hartman L, Khoukaz T, Brutcher E, Lenz HJ, et al. Safety of Nivolumab Plus Low-Dose Ipilimumab in Previously Treated Microsatellite Instability-High/Mismatch Repair-Deficient Metastatic Colorectal Cancer. Oncologist (2019) 24:1453–61. doi: 10.1634/theoncologist.2019-0129
157. Eng C, Kim TW, Bendell J, Argiles G, Tebbutt NC, Di Bartolomeo M, et al. Atezolizumab With or Without Cobimetinib Versus Regorafenib in Previously Treated Metastatic Colorectal Cancer (IMblaze370): A Multicentre, Open-Label, Phase 3, Randomised, Controlled Trial. Lancet Oncol (2019) 20:849–61. doi: 10.1016/S1470-2045(19)30027-0
158. Tapia Rico G, Price TJ. Atezolizumab for the Treatment of Colorectal Cancer: The Latest Evidence and Clinical Potential. Expert Opin Biol Ther (2018) 18:449–57. doi: 10.1080/14712598.2018.1444024
159. Barroso-Sousa R, Krop IE, Trippa L, Tan-Wasielewski Z, Li T, Osmani W, et al. A Phase II Study of Pembrolizumab in Combination With Palliative Radiotherapy for Hormone Receptor-Positive Metastatic Breast Cancer. Clin Breast Cancer (2020) 20:238–45. doi: 10.1016/j.clbc.2020.01.012
160. Bourhis J, Stein A, Paul de Boer J, Van Den Eynde M, Gold KA, Stintzing S, et al. Avelumab and Cetuximab as a Therapeutic Combination: An Overview of Scientific Rationale and Current Clinical Trials in Cancer. Cancer Treat Rev (2021) 97:102172. doi: 10.1016/j.ctrv.2021.102172
161. Schmoll HJ, Arnold D, de Gramont A, Ducreux M, Grothey A, O’Dwyer PJ, et al. MODUL-A Multicenter Randomized Clinical Trial of Biomarker-Driven Maintenance Therapy Following First-Line Standard Induction Treatment of Metastatic Colorectal Cancer: An Adaptable Signal-Seeking Approach. J Cancer Res Clin Oncol (2018) 144:1197–204. doi: 10.1007/s00432-018-2632-6
162. Dolcetti R, De Re V, Canzonieri V. Immunotherapy for Gastric Cancer: Time for a Personalized Approach? Int J Mol Sci (2018) 19:1602. doi: 10.3390/ijms19061602
163. Shah MA, Kojima T, Hochhauser D, Enzinger P, Raimbourg J, Hollebecque A, et al. Efficacy and Safety of Pembrolizumab for Heavily Pretreated Patients With Advanced, Metastatic Adenocarcinoma or Squamous Cell Carcinoma of the Esophagus: The Phase 2 KEYNOTE-180 Study. JAMA Oncol (2019) 5:546–50. doi: 10.1001/jamaoncol.2018.5441
164. Kojima T, Shah MA, Muro K, Francois E, Adenis A, Hsu CH, et al. Randomized Phase III KEYNOTE-181 Study of Pembrolizumab Versus Chemotherapy in Advanced Esophageal Cancer. J Clin Oncol (2020) 38:4138–48. doi: 10.1200/JCO.20.01888
165. Ilson DH. Advances in the Treatment of Gastric Cancer. Curr Opin Gastroenterol (2018) 34:465–8. doi: 10.1097/MOG.0000000000000475
166. Janjigian YY, Bendell J, Calvo E, Kim JW, Ascierto PA, Sharma P, et al. CheckMate-032 Study: Efficacy and Safety of Nivolumab and Nivolumab Plus Ipilimumab in Patients With Metastatic Esophagogastric Cancer. J Clin Oncol (2018) 36:2836–44. doi: 10.1200/JCO.2017.76.6212
167. Ready N, Farago AF, de Braud F, Atmaca A, Hellmann MD, Schneider JG, et al. Third-Line Nivolumab Monotherapy in Recurrent SCLC: CheckMate 032. J Thorac Oncol (2019) 14:237–44. doi: 10.1016/j.jtho.2018.10.003
168. Kato K, Cho BC, Takahashi M, Okada M, Lin CY, Chin K, et al. Nivolumab Versus Chemotherapy in Patients With Advanced Oesophageal Squamous Cell Carcinoma Refractory or Intolerant to Previous Chemotherapy (ATTRACTION-3): A Multicentre, Randomised, Open-Label, Phase 3 Trial. Lancet Oncol (2019) 20:1506–17. doi: 10.1016/S1470-2045(19)30626-6
169. Lee JJ, Chu E. Recent Advances in the Clinical Development of Immune Checkpoint Blockade Therapy for Mismatch Repair Proficient (pMMR)/Non-MSI-H Metastatic Colorectal Cancer. Clin Colorectal Cancer (2018) 17:258–73. doi: 10.1016/j.clcc.2018.06.004
170. Derks S, Liao X, Chiaravalli AM, Xu X, Camargo MC, Solcia E, et al. Abundant PD-L1 Expression in Epstein-Barr Virus-Infected Gastric Cancers. Oncotarget (2016) 7:32925–32. doi: 10.18632/oncotarget.9076
Keywords: gastrointestinal cancer, colorectal cancer, gastric cancer, esophageal cancer, immune checkpoint inhibitor, immunotherapy
Citation: Wang D-K, Zuo Q, He Q-Y and Li B (2021) Targeted Immunotherapies in Gastrointestinal Cancer: From Molecular Mechanisms to Implications. Front. Immunol. 12:705999. doi: 10.3389/fimmu.2021.705999
Received: 06 May 2021; Accepted: 26 July 2021;
Published: 10 August 2021.
Edited by:
Hubing Shi, Sichuan University, ChinaReviewed by:
Chu Lin, Peking University People’s Hospital, ChinaNavin Chintala, Memorial Sloan Kettering Cancer Center, United States
Reem Saleh, Peter MacCallum Cancer Centre, Australia
Copyright © 2021 Wang, Zuo, He and Li. This is an open-access article distributed under the terms of the Creative Commons Attribution License (CC BY). The use, distribution or reproduction in other forums is permitted, provided the original author(s) and the copyright owner(s) are credited and that the original publication in this journal is cited, in accordance with accepted academic practice. No use, distribution or reproduction is permitted which does not comply with these terms.
*Correspondence: Bin Li, libinjnu@163.com
†These authors have contributed equally to this work and share first authorship