- 1Department of Medicine, University of Wisconsin-Madison, Madison, WI, United States
- 2Department of Medical Microbiology and Immunology, University of Wisconsin-Madison, Madison, WI, United States
Invasive candidiasis frequently involves medical device placement. On the surfaces of these devices, Candida can form biofilms and proliferate in adherent layers of fungal cells surrounded by a protective extracellular matrix. Due in part to this extracellular matrix, biofilms resist host defenses and antifungal drugs. Previous work (using neutrophils from healthy donors) found that one mechanism employed to resist host defenses involves the inhibition of neutrophil extracellular traps (NET) formation. NETs contain nuclear DNA, as well as antimicrobial proteins that can ensnare pathogens too large or aggregated to be effectively killed by phagocytosis. Given that these neutrophil structures are anticipated to have activity against the large aggregates of C. albicans biofilms, understanding the role of this inhibition in patients could provide insight into new treatment strategies. However, prior work has not included patients. Here, we examine NET formation by neutrophils collected from patients with invasive candidiasis. When compared to neutrophils from healthy participants, we show that patient neutrophils exhibit a heightened background level of NET release and respond to a positive stimulus by producing 100% more NETs. However, despite these physiologic differences, patient neutrophil responses to C. albicans were similar to healthy neutrophils. For both groups, planktonic cells induce strong NET release and biofilms inhibit NET formation. These results show that a mechanism of immune evasion for fungal biofilms translates to the clinical setting.
Introduction
Candida albicans, a widespread nosocomial fungal pathogen, is an avid biofilm-former and a frequent cause of invasive fungal infection (1). On the surface of medical devices, such as vascular catheters, Candida spp. adopt a biofilm lifestyle and grow as adherent communities encased in an extracellular matrix with protective properties (2). Candida biofilms infections are notoriously difficult to treat, as they resist high levels of antifungal drugs and withstand host defenses (3–10). Despite advancements in antifungal therapies and diagnostics, the mortality for Candida-associated bloodstream infection remains exceedingly high, near 30% (1, 11).
Neutrophils are essential for eradicating many fungal infections, including invasive candidiasis (12–15). Neutropenic patients are at high risk for critical disease, and those remaining neutropenic are more likely to relapse (16). However, in the clinical setting, neutrophils are ineffective at controlling Candida device-associated biofilm infections. Eradication of infection most often requires device removal, even for patients with normal numbers of neutrophils (11). Moreover, mortality rates increase for patients when biofilm-infected devices are retained (1, 11).
Ex vivo, C. albicans biofilms are approximately 5-fold more resistant to killing by neutrophils when compared to non-biofilm, planktonic cells (7, 8, 17, 18). When no biofilm is present, neutrophils phagocytose the smaller yeast form of C. albicans and release neutrophil extracellular traps (NETs) in response to the larger, elongated hyphal form (19, 20). NETs consist of extracellular web-like structures of DNA associated with citrullinated histones and other antimicrobial proteins that contain and kill pathogens. These structures exhibit antifungal activity against both yeast and hyphae (19, 21, 22). NETs are anticipated to be crucial for controlling Candida infections because they can target hyphae, which are too large to be phagocytosed (20). Given their role in combatting hyphae and their activity against aggregative pathogens, the formation of NETs would seem to be an ideal response against Candida biofilm. However, neutrophils (collected from healthy study participants) fail to release NETs in response to Candida biofilms (18, 23, 24). This impaired innate immune response likely contributes to the resilient nature of device-associated Candida infections.
While our understanding neutrophil-Candida biofilm interactions has advanced significantly using healthy participant neutrophils, studies have not delineated the relevance of these interactions for patients with invasive candidiasis. We considered that infection with Candida may influence neutrophil responses. The conventional concept poses that neutrophils are uniformly short-lived, terminally differentiated cells. However, recent investigations reveal phenomena of significant neutrophil plasticity and heterogeneity in a variety of clinical settings (25–27). Here we compare neutrophil responses between healthy participants and those with invasive candidiasis, focusing on NET formation.
Methods
Organisms and Inoculum
C. albicans (SC5314) was stored in 15% glycerol stock (vol/vol) at -80°C and plated on yeast extract-peptone-dextrose agar (YPD-agar) plates (1% yeast extract, 2% peptone, 2% dextrose, 2% agar) prior to experiments. Cultures were grown overnight in YPD media (1% yeast extract, 2% peptone, and 2% dextrose) at 200 RPM at 30°C in an orbital shaker. For experiments using biofilms, C. albicans was resuspended in RPMI-MOPS at a concentration of 1.5 × 106 cells/ml and added at volumes specified in individual assays, followed by a 24 h incubation at 37°C. For experiments with planktonic cells, 1 ml of an overnight culture was added to 10 ml fresh YPD broth for 2 h at 180 RPM at 30°C. Following the incubation, the planktonic C. albicans cells were washed twice with phosphate-buffered saline (-calcium and -magnesium) DPBS (Hyclone Laboratories Inc., Logan, UT) and counted with a hemocytometer. For Sytox Green and reactive oxygen species (ROS) production assays, a burden of planktonic cells (3 × 106 cells/well) equivalent to biofilm burden was established, as described previously (18). For other experiments, planktonic cells were added at concentrations described below.
Study Participants
We obtained blood samples from patients with invasive candidiasis, through protocol 2017-0685 approved by the University of Wisconsin Internal Review Board (IRB). Patients were eligible upon identification of Candida growing as a culture from a normally sterile site, in accordance with clinical diagnosis of invasive candidiasis (16). All patients were 18–89 and admitted for hospitalization at the University of Wisconsin Hospital. We collected blood samples following written informed consent. Patient characteristics are presented in Table 1. Blood from healthy participants was collected through protocol 2013-1758 approved by the University of Wisconsin IRB. Healthy participants were 18 years of age or older and the group included 9 males and 10 females.
Neutrophil Isolation
We isolated primary human neutrophils from whole blood by negative antibody selection using the MACSxpress Neutrophil Isolation and MACSxpress Erythrocyte Depletion kit (Miltenyi Biotec Inc., Auburn, CA). Experiments with isolated neutrophils were performed in RPMI 1640 media (without phenol red) supplemented with 2% heat-inactivated fetal bovine serum (FBS) and glutamine (0.3 mg/ml). All incubations were at 37°C with 5% CO2. We collected neutrophils from up to 12 ml of blood, which resulted in 1.0 × 107 to 6.1 × 107 neutrophils per patient.
Sytox Green Assays
For a quantitative measure of NET formation, we used Sytox Green assays, as described previously (18, 23). To measure NET release in the presence of biofilm, we grew C. albicans as a biofilm for 24 h in 96-well opaque plates. To measure NET formation in the presence of planktonic cells, we added 3 × 106 planktonic cells/well prior to the addition of neutrophils. For a subset of experiments, phorbol myristate acetate (PMA) was added at a final concentration of 100 nM. Neutrophils were added at a final concentration of 2 × 105 neutrophils/well. After a 4 h incubation, the cell-impermeable free DNA fluorescent dye Sytox Green (Life Technologies, Eugene, OR) was added and read at excitation 500 nm/emission 528 nm, as previously described (18, 28). In experiments with C. albicans, fluorescence for C. albicans biofilms or planktonic cells alone was analyzed and subtracted as background fluorescence.
ROS Production Assays
To measure ROS production, we used an oxidative stress assay modified for biofilm (18, 23). Planktonic cells or biofilm were added to 96-well opaque plates, as described above. Neutrophils were stained at room temperature with fluorescent dye CM-H2DCFDA (Life Technologies, Eugene, OR) for 10 min in DPBS prior to addition to a 96-well opaque plate at a final concentration of 2 × 105 neutrophils/well. For some experiments, PMA was added at a final concentration of 100 nM. Fluorescence was recorded prior to incubation at 37°C and at every 30 min for 4 h (excitation, 495 nm; emission, 527 nm). In experiments with C. albicans, fluorescence for C. albicans biofilms or planktonic cells alone was recorded and subtracted as background fluorescence.
Scanning Electron Microscopy
For electron microscopy experiments, we utilized poly-L-lysine-treated plastic coverslips (13 mm, Thermonax plastic for cell culture). Neutrophil (5 × 105) were added to coverslips containing C. albicans biofilm, planktonic cells, or PMA. For biofilm experiments, biofilms were grown on coverslips, as previously described (18, 23). Briefly, 1.5 × 106 cells/ml C. albicans were applied to coverslips at 30°C for 30 min. After removing non-adherent cells, fresh RPMI-MOPS was added and biofilms were grown for 24 h at 37°C and then washed with DPBS. For planktonic interactions, planktonic cells were added to coverslips (1.5 × 107 cells/coverslip) for 1 h prior to the addition of neutrophils. For a subset of experiments, PMA was included at a final concentration of 100 nM. Following a 4 h incubation, coverslips were processed for scanning electron microscopy, as described previously (18, 23). Briefly, samples were washed in DPBS and fixed overnight in a 4% formaldehyde and 1% glutaraldehyde solution in DPBS. Following fixation, samples were treated with 1% osmium tetroxide for 1 h, and then washed with DPBS. The samples then underwent ethanol dehydration, which was immediately followed with critical point drying. Samples were then placed on aluminum stubs and sputter-coated with 10 nm platinum. The samples were imaged at 3 kV by a LEO 1530 scanning electron microscope.
Immunofluorescence Microscopy
We utilized immunofluorescence microscopy to visualize the location of neutrophil elastase. Neutrophils were analyzed in 8-well Ibidi devices. Briefly, neutrophils (1 × 105/well) were added to wells containing biofilms, planktonic cells, or PMA (100 nM). To examine neutrophil-biofilm interactions, we seeded 100 µl of C. albicans in RPMI-MOPS at 1.5 × 106 cells/ml and incubated for 24 h on a 45° incline to propagate biofilm. After 6 h, 50 µl of RPMI-MOPS was gently added to prevent biofilm growth at the air interface. Following 24 h of growth, biofilms were removed from incline and gently rinsed with DPBS prior adding neutrophils. For planktonic studies, we added 4 × 105 planktonic C. albicans cells per device. Following a 4 h incubation, samples were fixed for 2 h in 1% formaldehyde in DPBS. Samples were then washed with DPBS and incubated with blocking buffer (2% w/v bovine serum albumin (BSA) and 0.02% v/v Tween 20 in DPBS) overnight at 4°C. Samples were exposed to anti-Neutrophil elastase rabbit pAb (MilliporeSigma, Burlington, MA) or anti-Histone 4 (citrulline 3) rabbit pAB (EMD Millipore, Temecula, CA) at a 1:200 dilution in binding buffer (0.1% BSA w/v and 0.005% v/v Tween 20 in DPBS) overnight at 4°C. Samples were washed 3× for 5 min in fresh binding buffer and then incubated with chicken anti-rabbit IgG, DyLight 594 conjugated secondary antibody (ImmunoReagents, Inc., Raleigh, NC) at a 1:500 dilution in binding buffer for 1 h at room temperature and washed 3× for 5 min in binding buffer prior to being imaged in DPBS. Images were obtained on a Nikon eclipse-TI2 inverted microscope equipped with a TI2-S-SS-E motorized stage, ORCA-Flash 4.0LT sCMOS camera, and NIS elements imaging software.
Statistics
Experiments were performed at least 4 times using neutrophils from different donors on different days. Statistical analyses were performed by ANOVA or Student’s t-test using GraphPad Prism8 software. Differences of p < 0.05 were considered significant.
Results
Neutrophils From Invasive Candidiasis Patients Exhibit a Heightened Capacity for NET Formation
To delineate neutrophil responses for patients with invasive candidiasis, we collected blood from hospitalized patients and performed ex vivo neutrophil studies (Table 1). We also included neutrophils from healthy participants as a comparison, as prior Candida-neutrophil work has primarily utilized neutrophils from this population (6–8, 17–20, 23, 29, 30). In the absence of a stimulus, neutrophils from both study groups appeared rounded and NETs were only rarely observed by scanning electron microscopy over the course of 4 h (Figure 1A). To quantify NET release, we utilized a Sytox Green assay to measure free DNA (Figure 1B). Consistent with the scanning electron microscopy images, unstimulated neutrophils released very little free DNA. Neutrophils from patients with invasive candidiasis trended toward a higher background level of NET release, but this did not reach statistical significance. We next examined neutrophil responses to PMA, a strong inducer of NETs. Strikingly, in response to PMA, neutrophils from patients with invasive candidiasis released nearly 100% more DNA than neutrophils from healthy participants (Figure 1B). Scanning electron imaging revealed the formation of NET structures for both groups of neutrophils exposed to PMA, with more frequent NETs in the patient neutrophil group (Figure 1A).
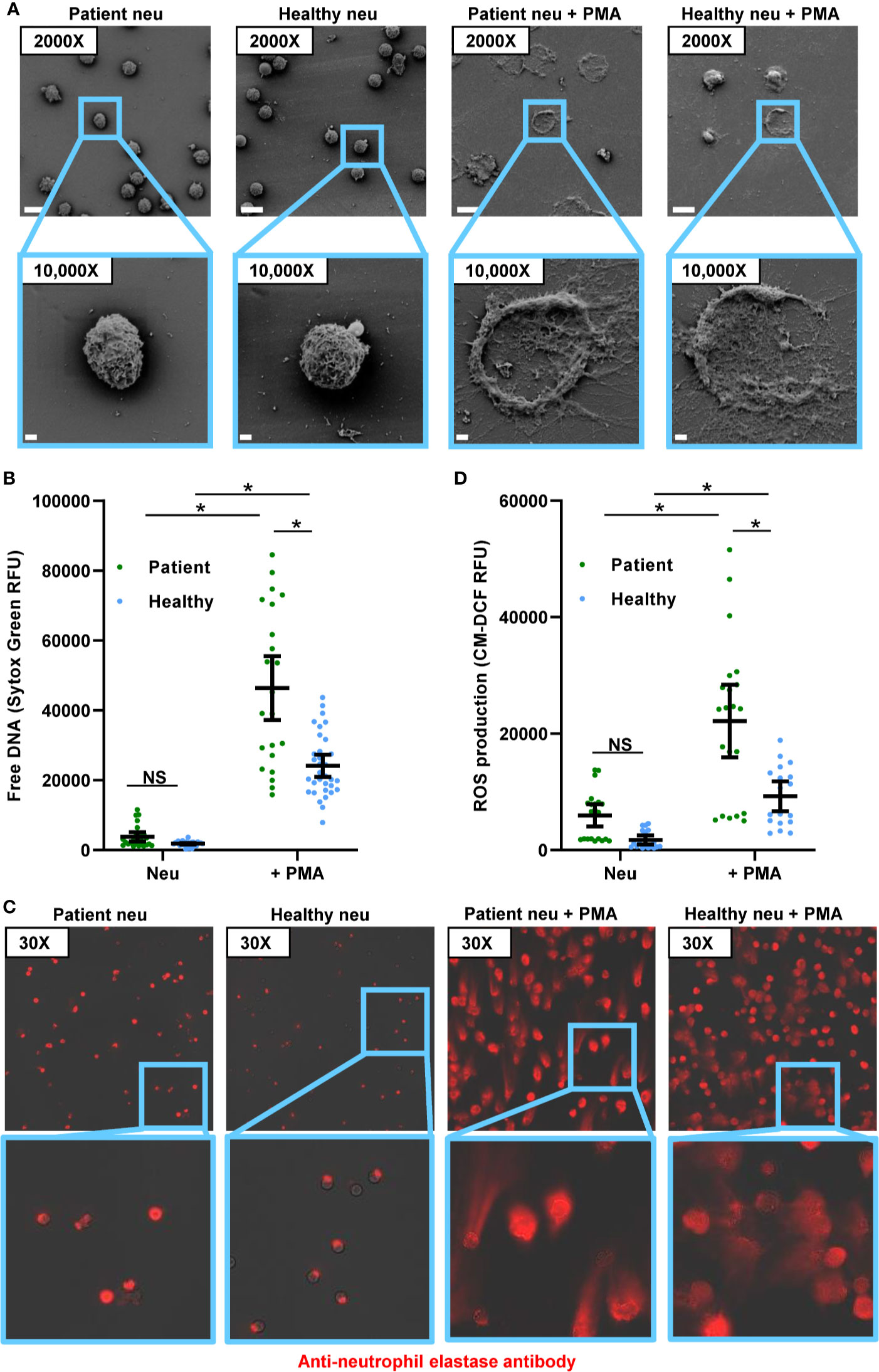
Figure 1 Comparison of PMA-induced NET formation and ROS production by neutrophils from healthy participants and patients with invasive candidiasis. (A) Scanning electron microscopy of patient and healthy neutrophils in the absence and presence of the NET stimulus PMA for 4 h. Measurement bars represent 10 µm and 1 µm for images taken at 2000X and 10,000X, respectively. (B) Patient and healthy neutrophils were incubated with or without PMA for 4 h and NET release was estimated by free DNA measurement following staining with cell-impermeable Sytox Green. (C) Neutrophils were incubated with or without PMA for 4 h, fixed, and stained with anti-neutrophil elastase rabbit primary antibody and chicken anti-rabbit IgG, DyLight 594 conjugated secondary antibody. (D) To measure ROS, neutrophils were pre-incubated with free radical sensor CM-H2DCFDA prior to incubation with or without PMA for 4 h, and fluorescence was measured. Statistical significance was analyzed by two-way ANOVA with Sidak’s multiple comparisons test, *p < 0.05, NS, not significant, mean with 95% confidence interval shown.
During NET formation, neutrophil elastase translocates from the granules to the nucleus, where it cleaves histones and ultimately releases extracellularly with DNA as part of the web-like structures (30, 31). Using immunohistochemistry, we analyzed the location of neutrophil elastase. As described previously for unstimulated neutrophils from healthy people, neutrophil elastase remained intracellular and appeared punctate (Figure 1C). This is consistent with prior work that has shown an intracellular, granular localization for neutrophil elastase in resting neutrophils (Figure 1C) (30). While unstimulated neutrophils from patients also displayed intracellular neutrophil elastase, in a subset of neutrophils, staining was more diffuse. This suggested that neutrophil elastase may be translocating to the cytosol or nucleus, as has been described for NET release, even in absence of a stimulus. In response to PMA, both neutrophil groups exhibited web-like structures of extracellular neutrophil elastase, consistent with NET formation (Figure 1C). The extensive formation of NETs limited a semi-quantitative comparison.
ROS triggers the release of neutrophil elastase into the cytosol (30). Because ROS production is a key step in PMA-induced NET release, we considered the possibility that activation of this pathway might differ between neutrophils from patients and healthy participants (32). We prelabeled neutrophils with an oxidative stress indicator and measured ROS production over the course of 4 h in the presence and absence of PMA (Figure 1D and Supplementary Figure 1A). In the absence of a stimulus, neutrophils from patients with invasive candidiasis generated more than 3-fold higher ROS levels compared to neutrophils from healthy people. In response to PMA, ROS production mirrored the pattern observed for NET formation, with neutrophils from invasive candidiasis patients generating twice the levels of ROS (Figures 1B, C).
Because of the heighten ROS production observed for patient neutrophils, we considered if they may exhibit higher ROS levels at baseline. We found neutrophils from invasive candidiasis patients to generate approximately 2-fold higher levels of ROS compared to healthy participant neutrophils (Supplementary Figure 1B). Considering the role of ROS in PMA-induced NET formation, this trait may contribute to the increased NET formation observed for patient neutrophils. To further explore NET formation pathways, we examined histone citrullination. This enzymatic modification of histone arginine residues to citrulline residues mediates chromatin decondensation and NET formation (33). By immunofluorescence, we did not detect histone citrullination in healthy participant neutrophils (Supplementary Figure 1C). However, we did observe histone citrullination for a small number of neutrophils from patients with invasive candidiasis, suggesting some cells may have activation of NET formation pathways in the absence of a stimulus. Taken together, the studies reveal that neutrophils from patients with invasive candidiasis display an increased capacity for ROS production and PMA-induced NET formation that differs from neutrophils collected from healthy participants.
Patient and Healthy Participant Neutrophils Respond Similarly to C. albicans
We next questioned how neutrophils from invasive candidiasis patients respond to C. albicans and if these interactions differ from those that have been observed for healthy people. As previously reported, healthy participant neutrophils form NETs upon encounter with planktonic C. albicans (19, 20). Consistent with this, scanning electron microscopy imaging revealed healthy participant neutrophils forming web-like structures coating planktonic cells (Figure 2A), and the free DNA of NETs was detected at levels similar to PMA induction (Figures 2B and 1B). Neutrophils from invasive candidiasis patients formed an equivalent amount of NETs upon encounter with planktonic C. albicans (Figures 2A, B). This was somewhat surprising in light of the heightened capacity of patient neutrophils for PMA-induced NET formation (Figure 1B). For both neutrophil groups, immunofluorescence imaging revealed strands of extracellular neutrophil elastase indicating the presence of NETs in response to planktonic C. albicans (Figure 2C). Upon examination of NET pathways following exposure to planktonic cells, we found that invasive candidiasis patient neutrophils generated 3-fold more ROS (Figure 2D and Supplementary Figure 2A). The finding that this elevation of ROS did not translate to increased NET formation by patient neutrophils is consistent with the involvement of ROS-independent pathways, as has been described for C. albicans (29, 31).
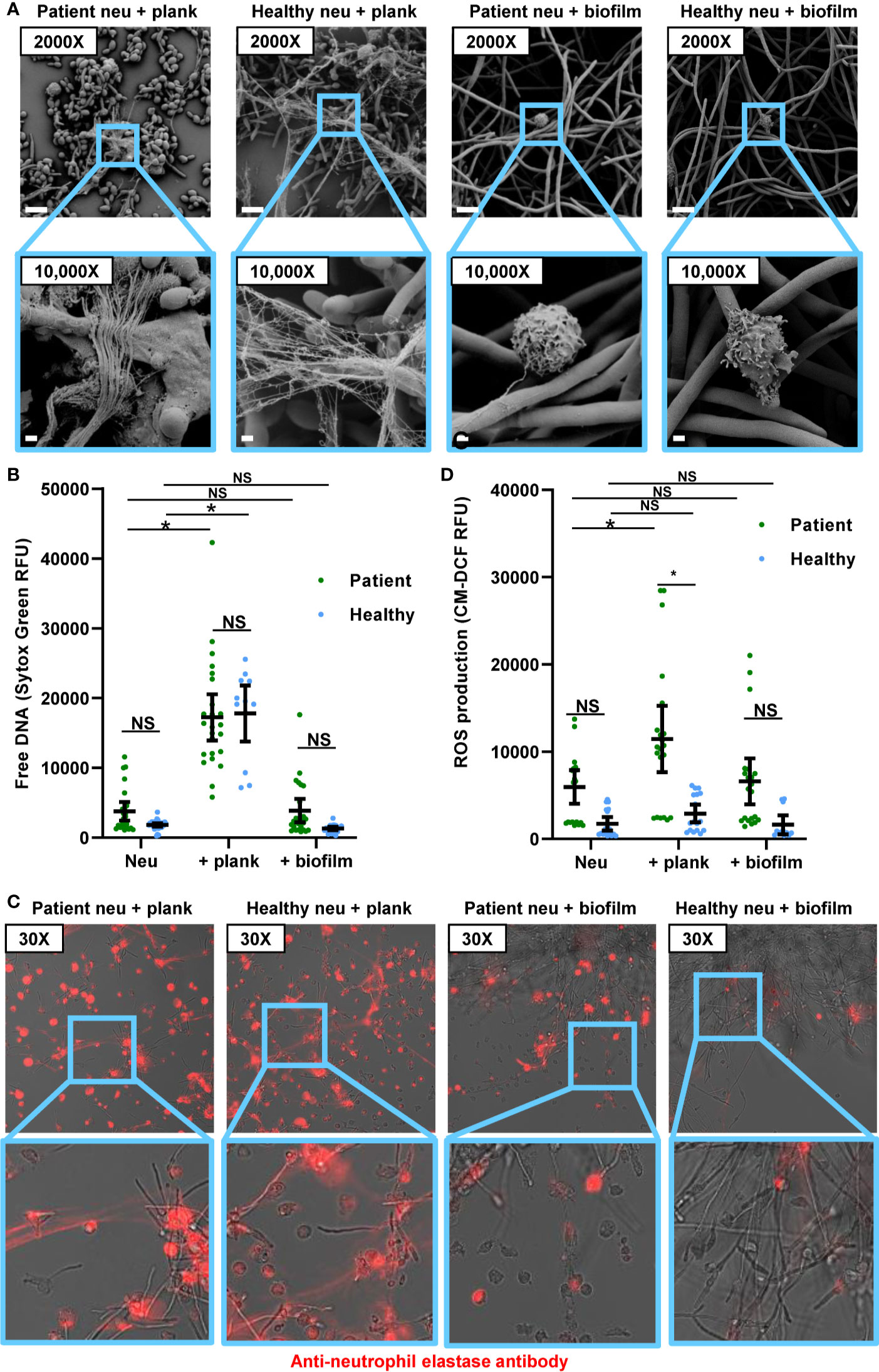
Figure 2 Response to planktonic and biofilm C. albicans for neutrophils from healthy participants and patients with invasive candidiasis. (A) Scanning electron microscopy of patient and healthy neutrophils co-incubated with planktonic or biofilm C. albicans for 4 h. Measurement bars represent 10 µm and 1 µm for images taken at 2,000× and 10,000×, respectively. (B) Patient and healthy neutrophils were co-incubated with planktonic or biofilm C. albicans for 4 h, and NETs were estimated by measurement of free DNA using Sytox Green. (C) Patient and healthy neutrophils were incubated with C. albicans planktonic cells or biofilm for 4 h, fixed, and stained with anti-neutrophil elastase rabbit primary antibody and chicken anti-rabbit IgG, DyLight 594 conjugated secondary antibody. Following fixation, neutrophils were stained for neutrophil elastase. (D) To measure ROS, neutrophils were pre-incubated with free radical sensor CM-H2DCFDA prior to incubation with planktonic or biofilm C. albicans for 4 h, and fluorescence was measured. Statistical significance was analyzed by two-way ANOVA with Sidak’s multiple comparisons test, *p < 0.05, NS, not significant, mean with 95% confidence interval shown.
Upon co-culture with C. albicans biofilms, both groups of neutrophils failed to form NETs (Figures 2A, B). By scanning electron microscopy, neutrophils remained intact without the extrusion of extracellular structures (Figure 2A). Although neutrophils from invasive candidiasis patients released slightly more free DNA than neutrophils from healthy people, this was not above the background detected in the neutrophil only control (Figure 2B). Neutrophil elastase remained primarily intracellular for both neutrophil groups (Figure 2C). The staining appeared slightly more diffuse for the invasive candidiasis patient neutrophils, suggesting early translocation of neutrophil elastase from the granules, similar to the appearance of these neutrophils without a stimulus present (Figure 1C). Similarly, biofilms did not induce ROS above the background for either neutrophil control group (Figure 2D). Thus, the lack of NET production in response to Candida biofilm is a clinically relevant phenotype that translates to patients.
C. albicans Biofilms Inhibit The Activity of Neutrophils From Patients With Invasive Candidiasis
Prior work with healthy participant neutrophils has shown that not only do C. albicans biofilms fail to induce NET formation, but also that NET release to other stimulants is suppressed by biofilm (18). Here, we wanted to determine if this phenotype translated to neutrophils from patients with invasive candidiasis. To test this, we measured NET formation by patient neutrophils in the presence of C. albicans biofilm and/or a stimulus for NET release (PMA). Consistent with our other experiments with patient neutrophils, PMA induced NET formation and biofilms did not (Figure 3A). When both biofilm and PMA were present, neutrophils failed to form NETs, indicating that biofilm suppresses PMA-induced NET formation. Similarly, biofilm formation inhibited PMA-induced ROS production (Figure 3B and Supplementary Figure 2B). These data support the finding that C. albicans biofilms suppress NET formation, even in the presence of a strong stimulant.
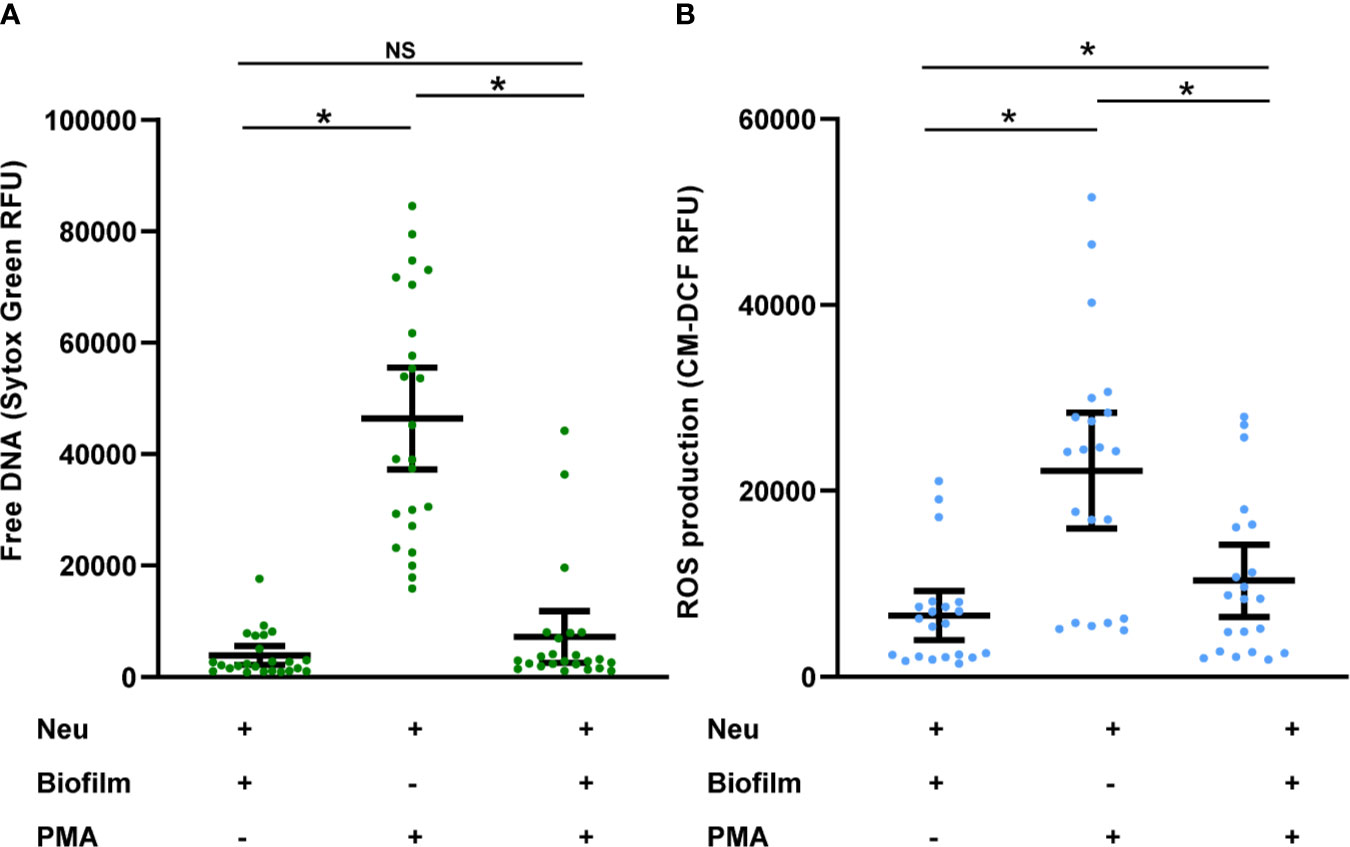
Figure 3 C. albicans biofilms inhibit PMA-induced activation of neutrophils collected from patients with invasive candidiasis. (A) Patient neutrophils were co-incubated with C. albicans biofilm in the absence or presence of PMA for 4 h and NET formation was estimated by measurement of free DNA using Sytox Green. (B) To measure ROS, neutrophils were pre-incubated with free radical sensor CM-H2DCFDA prior to incubation with C. albicans biofilm in the presence or absence of PMA for 4 h, and fluorescence was measured. Statistical significance was analyzed by one-way ANOVA with Tukey’s multiple comparisons test, *p < 0.05, NS, not significant, mean with 95% confidence interval shown.
Discussion
Invasive candidiasis is a widespread nosocomial infection with high mortality (1, 11). The vast majority of cases involve medical devices that become infected with Candida biofilms. While prior work has shown poor neutrophil responses to Candida biofilm, the role of these processes for patients with invasive candidiasis had not been elucidated (7, 8, 18, 23). Here, we characterize NET formation for patients with invasive candidiasis. With these patient neutrophils, we observe a heightened capacity for PMA-induced NET formation and activation of NET signalling pathways when compared to healthy participant neutrophils. Despite these different characteristics of patient neutrophils, we found that the neutrophils respond to C. albicans in a manner that mirrors healthy participant neutrophils. Both patient and healthy participant neutrophils formed NETs in response planktonic C. albicans but not upon encounter with C. albicans growing as a biofilm. These findings help explain why Candida biofilm infections are difficult to eradicate clinically. The studies also support the use of neutrophils from healthy people to model neutrophil responses during invasive candidiasis.
We were somewhat surprised to find that neutrophils from invasive candidiasis patients exhibited a heightened capacity to form NETs in response to PMA, but not to planktonic C. albicans. These differences suggest neutrophils from patients with invasive candidiasis might be primed for certain NET release pathways, but not others, depending on the stimuli (34). For example, PMA-induced NET release is ROS-dependent, whereas planktonic C. albicans can induce NETs through ROS-independent pathways (29, 31, 35, 36). We did observe a difference in ROS levels for patients and healthy participants, with patient neutrophils exhibiting elevated baseline ROS levels and higher ROS upon PMA treatment (Figure 1D and Supplementary Figures 1A, B). The current studies were not designed to determine the cause of the higher ROS production in patients. While this may be mainly due to candidiasis, our population included many patients with polymicrobial intra-abdominal infections (Table 1). Therefore, we are not able to exclude a contribution from bacterial infection. Furthermore, the studies did not include patients with serious bacterial infections or inflammatory conditions who did not have candidiasis. It is quite possible that neutrophils from these patient cohorts would also exhibit activation of NET pathways. Bacterial species, including Enterobacteriaceae and Streptococcus spp. have been shown to induce NETs through ROS-generating pathways (32, 37, 38). Furthermore, multiple factors may be influencing the neutrophil ROS production in these patients. However, despite the intrinsic heterogeneity of the patient population, the consistency of ROS elevation suggests this phenotype may be common among invasive candidiasis patients.
Prior work has shown that C. albicans biofilms inhibit NET release in healthy neutrophils, even in the presence of the potent stimulus (PMA) (18). Here, we show that biofilms also inhibit PMA-induced NET release in the neutrophils of patients with invasive candidiasis. This inhibition is striking in light of their increased baseline activation. Beyond this lack of activity against biofilms, suppression of neutrophils by C. albicans biofilm may impair the activation of other cells involved in innate and adaptive immunity (39, 40). Further study is needed to understand how fungal biofilms may influence immune responses to other pathogens in the clinical setting.
The subset of invasive candidiasis patients enrolled in our study are representative of larger cohort studies (1, 11, 41). For example, the study participants carried the diagnosis of immune-compromising diseases, received immunosuppressive drugs, and frequently required central lines (11, 41). However, as expected, we observed differences in co-morbidities, sites of infection, and Candida species. Despite the varied patient characteristics, we observed rather uniform neutrophil responses for all studies. This suggests that the findings may be broadly applicable to variety of patients with invasive candidiasis.
Data availability Statement
The raw data supporting the conclusions of this article will be made available by the authors, without undue reservation.
Ethics Statement
The studies involving human participants were reviewed and approved by the University of Wisconsin Internal Review Board. The patients/participants provided their written informed consent to participate in this study.
Author Contributions
JK, CJ, and JN planned the study and designed the experiments. CJ, JK, and MB performed the experiments. JK, CJ, JC, and JN analyzed data, performed statistical analyses, and wrote the manuscript. JN supervised the work. All authors offered input to the manuscript and approved the final draft. All authors contributed to the article and approved the submitted version.
Funding
This work was supported by the National Institutes of Health (R01 AI145939), the Burroughs Wellcome Fund (1012299), and the Doris Duke Charitable Foundation (2017074).
Conflict of Interest
The authors declare that the research was conducted in the absence of any commercial or financial relationships that could be construed as a potential conflict of interest.
Supplementary Material
The Supplementary Material for this article can be found online at: https://www.frontiersin.org/articles/10.3389/fimmu.2020.587956/full#supplementary-material
References
1. Pfaller MA, Diekema DJ. Epidemiology of invasive candidiasis: a persistent public health problem. Clin Microbiol Rev (2007) 20(1):133–63. doi: 10.1128/cmr.00029-06
2. Hawser SP, Baillie GS, Douglas LJ. Production of extracellular matrix by Candida albicans biofilms. J Med Microbiol (1998) 47(3):253–6. doi: 10.1099/00222615-47-3-253
3. Donlan RM. Biofilm formation: a clinically relevant microbiological process. Clin Infect Dis (2001) 33(8):1387–92. doi: 10.1086/322972
4. Kojic EM, Darouiche RO. Candida infections of medical devices. Clin Microbiol Rev (2004) 17(2):255–67. doi: 10.1128/CMR.17.2.255-267.2004
5. Kumamoto CA, Vinces MD. Alternative Candida albicans lifestyles: growth on surfaces. Annu Rev Microbiol (2005) 59:113–33. doi: 10.1146/annurev.micro.59.030804.121034
6. Katragkou A, Kruhlak MJ, Simitsopoulou M, Chatzimoschou A, Taparkou A, Cotten CJ, et al. Interactions between human phagocytes and Candida albicans biofilms alone and in combination with antifungal agents. J Infect Dis (2010) 201(12):1941–9. doi: 10.1086/652783
7. Katragkou A, Chatzimoschou A, Simitsopoulou M, Georgiadou E, Roilides E. Additive antifungal activity of anidulafungin and human neutrophils against Candida parapsilosis biofilms. J Antimicrob Chemother (2011) 66(3):588–91. doi: 10.1093/jac/dkq466
8. Katragkou A, Simitsopoulou M, Chatzimoschou A, Georgiadou E, Walsh TJ, Roilides E. Effects of interferon-g and granulocyte colony-stimulating factor on antifungal activity of human polymorphonuclear neutrophils against Candida albicans grown as biofilms or planktonic cells. Cytokine (2011) 55(3):330–4. doi: 10.1016/j.cyto.2011.05.007
9. Ramage G, Bachmann S, Patterson TF, Wickes BL, Lopez-Ribot JL. Investigation of multidrug efflux pumps in relation to fluconazole resistance in Candida albicans biofilms. J Antimicrob Chemother (2002) 49(6):973–80. doi: 10.1093/jac/dkf049
10. Chandra J, Kuhn DM, Mukherjee PK, Hoyer LL, McCormick T, Ghannoum MA. Biofilm formation by the fungal pathogen Candida albicans: development, architecture, and drug resistance. J Bacteriol (2001) 183(18):5385–94. doi: 10.1128/JB.183.18.5385-5394.2001
11. Andes DR, Safdar N, Baddley JW, Playford G, Reboli AC, Rex JH, et al. Impact of treatment strategy on outcomes in patients with candidemia and other forms of invasive candidiasis: a patient-level quantitative review of randomized trials. Clin Infect Dis (2012) 54(8):1110–22. doi: 10.1093/cid/cis021
12. Shoham S, Levitz SM. The immune response to fungal infections. Br J Haematol (2005) 129(5):569–82. doi: 10.1111/j.1365-2141.2005.05397.x
13. Edwards JE Jr., Lehrer RI, Stiehm ER, Fischer TJ, Young LS. Severe candidal infections: clinical perspective, immune defense mechanisms, and current concepts of therapy. Ann Intern Med (1978) 89(1):91–106. doi: 10.7326/0003-4819-89-1-91
14. Fidel PL Jr. Immunity to Candida. Oral Dis (2002) 8 Suppl 2:69–75. doi: 10.1034/j.1601-0825.2002.00015.x
15. Erwig LP, Gow NA. Interactions of fungal pathogens with phagocytes. Nat Rev Microbiol (2016) 14(3):163–76. doi: 10.1038/nrmicro.2015.21
16. Pappas PG, Kauffman CA, Andes DR, Clancy CJ, Marr KA, Ostrosky-Zeichner L, et al. Clinical practice guideline for the management of candidiasis: 2016 update by the Infectious Diseases Society of America. Clin Infect Dis (2016) 62(4):e1–50. doi: 10.1093/cid/civ933
17. Xie Z, Thompson A, Sobue T, Kashleva H, Xu H, Vasilakos J, et al. Candida albicans biofilms do not trigger reactive oxygen species and evade neutrophil killing. J Infect Dis (2012) 206(12):1936–45. doi: 10.1093/infdis/jis607
18. Johnson CJ, Cabezas-Olcoz J, Kernien JF, Wang SX, Beebe DJ, Huttenlocher A, et al. The extracellular matrix of Candida albicans biofilms impairs formation of neutrophil extracellular traps. PLoS Pathog (2016) 12(9):1–23. doi: 10.1371/journal.ppat.1005884
19. Urban CF, Reichard U, Brinkmann V, Zychlinsky A. Neutrophil extracellular traps capture and kill Candida albicans yeast and hyphal forms. Cell Microbiol (2006) 8(4):668–76. doi: 10.1111/j.1462-5822.2005.00659.x
20. Branzk N, Lubojemska A, Hardison SE, Wang Q, Gutierrez MG, Brown GD, et al. Neutrophils sense microbe size and selectively release neutrophil extracellular traps in response to large pathogens. Nat Immunol (2014) 15(11):1017–25. doi: 10.1038/ni.2987
21. Brinkmann V, Reichard U, Goosmann C, Fauler B, Uhlemann Y, Weiss DS, et al. Neutrophil extracellular traps kill bacteria. Science (2004) 303(5663):1532–5. doi: 10.1126/science.1092385
22. Urban CF, Ermert D, Schmid M, Abu-Abed U, Goosmann C, Nacken W, et al. Neutrophil extracellular traps contain calprotectin, a cytosolic protein complex involved in host defense against Candida albicans. PLoS Pathog (2009) 5(10):e1000639. doi: 10.1371/journal.ppat.1000639
23. Kernien JF, Johnson CJ, Nett JE. Conserved Inhibition of Neutrophil Extracellular Trap Release by Clinical Candida albicans Biofilms. J Fungi (Basel) (2017) 3(3):1–11. doi: 10.3390/jof3030049
24. Johnson CJ, Kernien JF, Hoyer AR, Nett JE. Mechanisms involved in the triggering of neutrophil extracellular traps (NETs) by Candida glabrata during planktonic and biofilm growth. Sci Rep (2017) 7(1):13065. doi: 10.1038/s41598-017-13588-6
25. Kolaczkowska E, Kubes P. Neutrophil recruitment and function in health and inflammation. Nat Rev Immunol (2013) 13(3):159–75. doi: 10.1038/nri3399
26. Pillay J, Ramakers BP, Kamp VM, Loi AL, Lam SW, Hietbrink F, et al. Functional heterogeneity and differential priming of circulating neutrophils in human experimental endotoxemia. J Leukoc Biol (2010) 88(1):211–20. doi: 10.1189/jlb.1209793
27. Beyrau M, Bodkin JV, Nourshargh S. Neutrophil heterogeneity in health and disease: a revitalized avenue in inflammation and immunity. Open Biol (2012) 2(11):120134. doi: 10.1098/rsob.120134
28. Brinkmann V, Laube B, Abu Abed U, Goosmann C, Zychlinsky A. Neutrophil extracellular traps: how to generate and visualize them. J Vis Exp (2010) 36:1–3. doi: 10.3791/1724
29. Byrd AS, O’Brien XM, Johnson CM, Lavigne LM, Reichner JS. An extracellular matrix-based mechanism of rapid neutrophil extracellular trap formation in response to Candida albicans. J Immunol (2013) 190(8):4136–48. doi: 10.4049/jimmunol.1202671
30. Metzler KD, Goosmann C, Lubojemska A, Zychlinsky A, Papayannopoulos V. A myeloperoxidase-containing complex regulates neutrophil elastase release and actin dynamics during NETosis. Cell Rep (2014) 8(3):883–96. doi: 10.1016/j.celrep.2014.06.044
31. Wu SY, Weng CL, Jheng MJ, Kan HW, Hsieh ST, Liu FT, et al. Candida albicans triggers NADPH oxidase-independent neutrophil extracellular traps through dectin-2. PLoS Pathog (2019) 15(11):e1008096. doi: 10.1371/journal.ppat.1008096
32. Fuchs TA, Abed U, Goosmann C, Hurwitz R, Schulze I, Wahn V, et al. Novel cell death program leads to neutrophil extracellular traps. J Cell Biol (2007) 176(2):231–41. doi: 10.1083/jcb.200606027
33. Wang Y, Li M, Stadler S, Correll S, Li P, Wang D, et al. Histone hypercitrullination mediates chromatin decondensation and neutrophil extracellular trap formation. J Cell Biol (2009) 184(2):205–13. doi: 10.1083/jcb.200806072
34. Papayannopoulos V. Neutrophil extracellular traps in immunity and disease. Nat Rev Immunol (2017) 18:1–14. doi: 10.1038/nri.2017.105
35. Hakkim A, Fuchs TA, Martinez NE, Hess S, Prinz H, Zychlinsky A, et al. Activation of the Raf-MEK-ERK pathway is required for neutrophil extracellular trap formation. Nat Chem Biol (2011) 7(2):75–7. doi: 10.1038/nchembio.496
36. Keshari RS, Jyoti A, Kumar S, Dubey M, Verma A, Srinag BS, et al. Neutrophil extracellular traps contain mitochondrial as well as nuclear DNA and exhibit inflammatory potential. Cytometry A (2012) 81(3):238–47. doi: 10.1002/cyto.a.21178
37. Pieterse E, Rother N, Yanginlar C, Hilbrands LB, van der Vlag J. Neutrophils Discriminate between Lipopolysaccharides of Different Bacterial Sources and Selectively Release Neutrophil Extracellular Traps. Front Immunol (2016) 7:484. doi: 10.3389/fimmu.2016.00484
38. Ma F, Chang X, Wang G, Zhou H, Ma Z, Lin H, et al. Streptococcus Suis Serotype 2 Stimulates Neutrophil Extracellular Traps Formation via Activation of p38 MAPK and ERK1/2. Front Immunol (2018) 9(2854):1–12. doi: 10.3389/fimmu.2018.02854
39. Kumar V, Sharma A. Neutrophils: Cinderella of innate immune system. Int Immunopharmacol (2010) 10(11):1325–34. doi: 10.1016/j.intimp.2010.08.012
40. Mantovani A, Cassatella MA, Costantini C, Jaillon S. Neutrophils in the activation and regulation of innate and adaptive immunity. Nat Rev Immunol (2011) 11(8):519–31. doi: 10.1038/nri3024
Keywords: Candida, biofilm, neutrophil, neutrophil extracellular trap, patients, invasive candidiasis, reactive oxygen species
Citation: Kernien JF, Johnson CJ, Bayless ML, Chovanec JF and Nett JE (2020) Neutrophils From Patients With Invasive Candidiasis Are Inhibited by Candida albicans Biofilms. Front. Immunol. 11:587956. doi: 10.3389/fimmu.2020.587956
Received: 27 July 2020; Accepted: 05 November 2020;
Published: 03 December 2020.
Edited by:
Luisa Martinez-Pomares, University of Nottingham, United KingdomReviewed by:
Joshua J. Obar, Dartmouth College, United StatesMaria Rapala-Kozik, Jagiellonian University, Poland
Copyright © 2020 Kernien, Johnson, Bayless, Chovanec and Nett. This is an open-access article distributed under the terms of the Creative Commons Attribution License (CC BY). The use, distribution or reproduction in other forums is permitted, provided the original author(s) and the copyright owner(s) are credited and that the original publication in this journal is cited, in accordance with accepted academic practice. No use, distribution or reproduction is permitted which does not comply with these terms.
*Correspondence: Jeniel E. Nett, jenett@medicine.wisc.edu