Animal Coloration in the Anthropocene
- School of Biological Sciences, University of Bristol, Bristol, United Kingdom
Natural habitats are increasingly affected by anthropogenically driven environmental changes resulting from habitat destruction, chemical and light pollution, and climate change. Organisms inhabiting such habitats are faced with novel disturbances that can alter their modes of signaling. Coloration is one such sensory modality whose production, perception and function is being affected by human-induced disturbances. Animals that acquire pigment derivatives through diet are adversely impacted by the introduction of chemical pollutants into their environments as well as by general loss of natural habitat due to urbanization or logging leading to declines in pigment sources. Those species that do manage to produce color-based signals and displays may face disruptions to their signaling medium in the form of light pollution and turbidity. Furthermore, forest fragmentation and the resulting breaks in canopy cover can expose animals to predation due to the influx of light into previously dark environments. Global climate warming has been decreasing snow cover in arctic regions, causing birds and mammals that undergo seasonal molts to appear conspicuous against a snowless background. Ectotherms that rely on color for thermoregulation are under pressure to change their appearances. Rapid changes in habitat type through severe fire events or coral bleaching also challenge animals to match their backgrounds. Through this review, we aim to describe the wide-ranging impacts of anthropogenic environmental changes on visual ecology and suggest directions for the use of coloration both as an indicator of ecological change and as a tool for conservation.
Introduction
The recent intersection of multiple global crises—climate change and public health—has brought the aesthetic enjoyment of “nature” to the forefront of collective human consciousness. As a species that tends to place the visual above other senses of perception, our knowledge systems are often centered around visual observations of natural phenomena and natural life, color in particular. As such, changes in our visual environments are often the first to be noticed and studied. Natural environments have been shaped by human societies for millennia (Sullivan et al., 2017), but recent decades have seen an acceleration of habitat loss and destruction due to changing land-use and climate. The rate of these changes outpaces natural rates of adaptation (Heinrichs et al., 2016; Greenspoon and Spencer, 2021). As these systems face threats from climate change, habitat loss and pollution, visual ecologists must reckon with the ramifications for the production, perception, evolution, and application of coloration. The introduction of novel stressors and the amplification of pre-existing ones beyond levels that animals can behaviorally ameliorate presents a range of consequences for the evolutionary and ecological dynamics of these populations. While visual ecologists and biologists have thus far been engaged in questions around the mechanisms of color production and perception, the field has yet to provide a synthetic understanding of the effects of anthropogenic stressors on these mechanisms. Conversely, conservation science has yet to incorporate cues from visual ecology as way to monitor populations at risk.
Over the course of this review, we attempt to lay out the documented effects of various anthropogenic stressors on different aspects of visual ecology. The production of color, either through de novo synthesis or dietary acquisition, is impacted from multiple directions by environmental pollution and habitat loss (Peneaux et al., 2021). The fragmentation or loss of a habitat also leads to a fragmentation of the dietary resources essential for the sequestering of pigmentary compounds such as carotenoids. In order for color phenotypes to function properly, the color patterns must be perceived under optimal lighting conditions, whether in ambient air or water. Rapid changes to the photic environment due to environmental pollution and landscape change disrupt the perception and processing of signals that are often crucial to species’ recognition and mate choice. Furthermore, color patterns used to achieve crypticity or thermoregulation can be rendered ineffective, and even counterproductive, due to accelerated changes to climate patterns and the seasonal composition of habitats. Additionally, epidermal color traits present great potential as non-invasive yet powerful indicators of environmental change, while the colorscape of an ecosystem, measured through the color composition and variation within and across species in the community presents an interesting alternative metric to report on the health of ecosystems. Finally, we outline present uses of visual information from the natural world for ecological monitoring and possibilities for the future incorporation of visual ecology for conservation.
Color Production
Central to the study of animal coloration is the presence of pigment sources in the animal’s habitat, whether as prey or plant material. This dependence of coloration on environmental conditions means that the color traits are often honest indicators of individual quality (Hamilton and Zuk, 1982; Naguib and Nemitz, 2007). Only individuals with good access to the necessary nutrients or the ability to overcome stresses can express costly phenotypes. Color in animals may be presented through either pigment deposition in the epidermis or through nanostructures such as those found in bird feathers and lepidopteran wings. Of the many pigment classes, the two most used, especially in birds, are melanin and carotenoids, each of which have different mechanisms of production. Carotenoids are primarily acquired through diet and are costly and energy intensive to produce, thereby indicative of the ability of individuals to compete and forage for the necessary dietary components (McGraw, 2006a). Melanin (eumelanin and phaeomelanin) is synthesized endogenously in melanocytes and may be limited by oxidative stress, hormones, and amino acid precursors (McGraw, 2006b). Pteridines are another class of pigments that are not as well studied as carotenoids or melanin but are increasingly regarded as indicators of individual quality (Stuart-Fox et al., 2021). Although structural coloration is primarily dependent on the incidence of light in the environment, some aspects of structural colors may be condition-dependent and constrained by protein availability in the diet (Prum, 2006). Anthropogenic landscape and climate change have introduced novel environmental constraints ranging from a direct loss of nutrient sources due to habitat loss to the effects of temperature change on endocrinology and biochemical pathways essential to pigment production.
Carotenoids
Carotenoid-based ornamental traits have been established as condition-dependent and therefore honestly indicative of individual quality (Hill, 1995, 2006) based on experimental and correlational studies (Weaver et al., 2018). The term “individual quality” is an index of body condition, used primarily in the context of female mate choice (Weaver et al., 2017) and sexual selection for male ornamental traits (Møller, 1991), which include highly plastic color signals. Several studies that experimentally manipulate the availability of carotenoid sources in the diet have demonstrated this plasticity and subsequent link between the habitat and quality (Peneaux et al., 2021; Stuart-Fox et al., 2021). This link may be disrupted when the carotenoid-based coloration is constrained by the environment in two ways—(a) through lack of availability of carotenoid sources such as insects, fruits and young shoots in the habitat and (b) constraints on other non-carotenoid macro-nutrients in the habitat, such as proteins and fats that are necessary for carotenoid absorption and expression through signals. As landscapes and habitats decline in quality, carotenoid-based coloration may start to be construed as an indicator of habitat condition rather than individual quality (Lifshitz and St Clair, 2016; Weaver et al., 2018; Peneaux et al., 2021).
The concept of using carotenoids as biological indicators of environmental changes is not a novel one (Hill, 1995). There is extensive literature on the relationship between the amount of carotenoid or carotenoid precursors available in the environment and the signal quality of animals, primarily avian species, which use carotenoid-based coloration. While a considerable proportion of the literature in this field is dedicated to experimentally inducing variation in carotenoid availability through captive breeding and diet manipulation to show the direct link between carotenoids in the environment and the level of display as ornaments in birds (McGraw et al., 2005; McGraw and Parker, 2006; Shawkey et al., 2006), some studies have relied on field observations of nesting in the wild. Free-ranging birds with wider growth bands on their tails (dependent on better overall nutrition) exhibit more saturated colorful plumage (dependent on carotenoid availability) (Hill and Montgomerie, 1994; Senar et al., 2003). The American kestrel (Falco sparverius) sequesters carotenoid-based yellow coloration in patches around the eyes, above the bill and on the tarsi (Figure 1). These patches of color are on the skin, and not on plumage and therefore can be directly linked to pigment deposition in the epidermis and not optic structures as seen in feathers. Bortolotti et al. (2000) present data on the genetic vs. environmental contributions to variation in carotenoid coloration in kestrels, collected through cameras set up in nest boxes to record prey variability and through spectrophotometry to quantify carotenoid levels. They found that the abundance of voles in territories was negatively associated with plasma carotenoid concentration in the predatory birds. Those that consumed relatively higher proportions of voles over other prey types, such as grasshoppers, frogs, dragonflies, or small birds, had lower concentrations of carotenoids, suggesting that environmental limits on the diversity of prey can lead to compromised color-based signaling as well as poorer health and quality. In a second study (Bortolotti et al., 2003) the authors exposed juvenile and adult kestrels to polychlorinated biphenyls (PCBs) through diet and measured plasma carotenoid concentrations and plumage coloration before and during the breeding period. Their results showed that PCBs disrupted both color deposition and plasma carotenoid levels during the breeding period. In male kestrels that were fed PCBs, plumage coloration became significantly duller. PCB exposed females did not lose carotenoid-based color during the breeding session, as expected and as seen in control females. Female kestrels fed the control diet lost their carotenoid coloration due to the routine allocation of carotenoids to eggs. The disruption of this mechanism by PCBs highlights the many ways organic pollutants can affect reproductive fitness. Another study by Fernie et al. (2001) showed that PCB contaminated females experienced delays in egg laying. In yet another case of carotenoid-based signaling reflective of individual health, Kristiansen et al. (2006) studied the coloration of bills and eye-rings in the great black-backed gull (Larus marinus). They found that adult male gulls in better condition (measured as residuals from the regression between body size and body weight) showed bills and eye-rings with higher color saturation while female gulls with higher color intensity laid larger eggs and had greater clutch sizes.
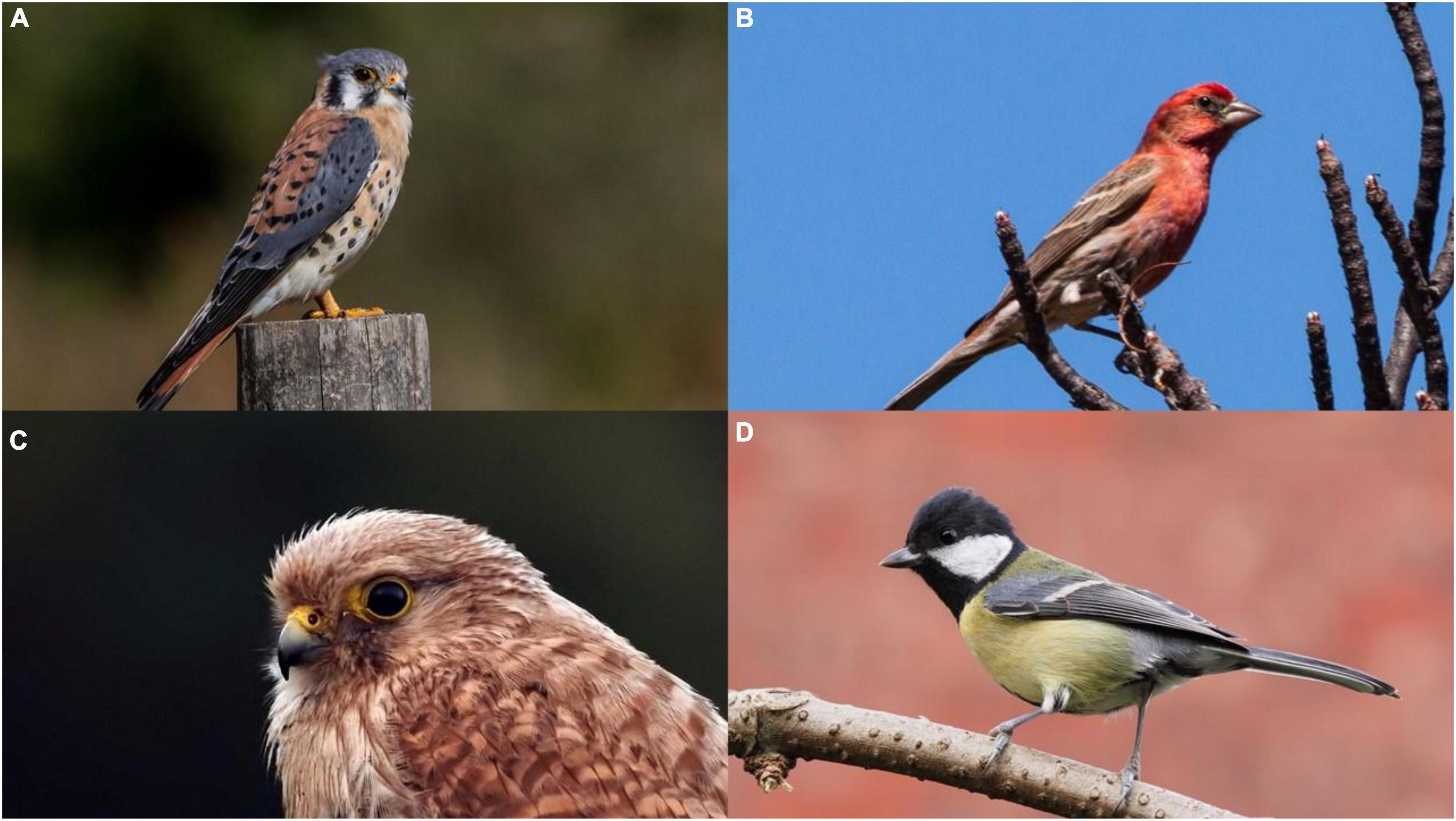
Figure 1. Birds whose pigmentation is affected by environmental pollution. (A) The American kestrel (Falco sparverius) in Florida, by Andrew Morrfew. Male kestrels that were fed PCBs in the laboratory setting showed duller plumage coloration (B) House finch (Haemorhous mexicanus) in Baja California, by Ron Knight. The house finch exhibits a decrease in red plumage coloration with exposure to herbicides. (C) Eurasian kestrel (Falco tinnunculus) in the Annamalai Hills, by T.R. Shankar Raman. The melanin-based plumage color has been shown to fluctuate in response to diet diversity (Fargallo et al., 2007). (D) Great tit (Parus major) in Lancashire by Francis Franklin. The yellow breast coloration has been shown to grow duller with exposure to metal pollutants, while melanin-based black stripes grow brighter.
Carotenoid based displays may be further environmentally constrained by the energetic costs of pigment production. Acquisition of carotenoids is dependent on both the availability of pigments as well as the availability of sufficient caloric content in the environment (Hill, 2000). Diet places energetic constraints on the biochemical pathways of pigment production as well as on the supplementary compounds such as lipoproteins that are just as essential to pigment synthesis and deposition (Tyczkowski and Hamilton, 1986). There is increasing evidence linking protein limitations in urban landscapes to declines in insect communities, a key feature of the avian diet (Tallamy and Shriver, 2021). Among the many multi-dimensional changes in climate and land-use patterns, urbanization and the resulting built environments may be the most far-reaching in this regard. Built environment is marked by a large-scale rapid transition from natural habitat to anthropogenic structures, accompanied by increased pollution, higher human population densities and significant increases in oxidative stress levels (Hutton and McGraw, 2016). As birds are one of the few classes of animals that continue to inhabit urban spaces after the transition from natural vegetation to built-up spaces, they may serve as a good indicator of rapid environmental change through the highly plastic carotenoid-based color patterns they use for conspecific signaling (Peneaux et al., 2021).
Melanin
Although melanin-based coloration has often been thought of as being primarily under genetic control with little influence from environmental factors (Griffith et al., 2006), inter-individual variation in melanin-based patches in the Eurasian kestrel (Falco tinnunculus) points to a different story. Fargallo et al. (2007) examined gray plumage in kestrels as an indicator of variation in environmental conditions during the early developmental stages of male nestlings. Through data collected from nest boxes over 3 years, they found that the production of gray color patches in male nestlings was correlated with prey richness in the environment and could not be attributed to genetically determined maternal quality allocated into eggs. Their results, combined with established knowledge about the biochemical pathway of melanogenesis and the higher cost of brown (phaeomelanin) vs. gray/black (eumelanin) pigmentation (Jawor and Breitwisch, 2003), demonstrate the dietary constraints on melanin production in kestrels. The incidence of gray color patches in male nestlings was higher in years with greater vole abundance. As melanin pigmentation in male kestrels may be attributed to quality signaling, either toward females for mate selection, or to advertise sex to parents for differential allocation of resources, large scale climate or land use changes could lead to fluctuations in prey supply and subsequently the quality of kestrel nestlings. New data (Fay et al., 2020) on the synchrony between vole population cycles and kestrel breeding patterns sets this dynamic in perspective as a possible case study for the effects of climate change on dietary sources of melanin pigmentation.
Structural Coloration
Sexually selected structural color ornaments are considered honest signals of quality due to their diet-driven plasticity. Protein is especially limiting for structural coloration of plumage and skin as well as tissue and feather formation through keratin and collagen. Certain colors commonly used for signals in birds are often produced through a combination of pigments and structural coloration (Prum and Torres, 2003). It is this association between pigment and optic structures that make macronutrients like proteins and fats important limiting factors in addition to carotenoid intake. Indeed, protein intake, or the lack thereof, has been found to affect other non-carotenoid based visual signals as well. Male blue tit nestlings with lower plasma protein concentrations exhibit tail feathers in a more vivid blue/UV (Peters et al., 2007). Lower levels of proteins circulating in the bloodstream are indicative of these proteins being allocated to the formation of nanostructures in feathers instead. In another study that investigated the effects of cholesterol level variation in zebra finch (Taeniopygia guttata) ornamental coloration, researchers found that bill coloration faded significantly in finches whose diets had less cholesterol (McGraw and Parker, 2006). Similarly, the iconic iridescent patch in male Anna’s hummingbirds (Calypte anna) is constrained by protein: hummingbirds that were fed higher protein levels grew brighter crown feathers (Meadows et al., 2012).
Pollutants and Color Production
In addition to being impacted by dietary constraints, integumentary pigmentation and structurally derived colors are also affected by anthropogenic pollution. These pollutants can range from pharmaceuticals, to pesticides, to industry-related compounds, to metals (Lifshitz and St Clair, 2016). As previously established here, color displays that act as honest indicators of quality can be heavily constrained by diet and nutritional deficiencies in their respective environments. Broadly, polluting compounds impact animal coloration through multiple pathways: by inhibiting or disrupting the acquisition of carotenoid precursors, by replacing similar compounds in key biochemical pathways and by causing general declines in overall health and condition (Pacyna et al., 2018). The abundance of literature (Table 1) on the effects of pollutants on plumage coloration in birds suggests that carotenoid-based coloration could be used as an indicator of pollution. Organic pollutants such as DDT (dichlorodiphenyl trichloride), PCB and PAH (polycylic aromatic hydrocarbons) have been shown to reduce hue and saturation in birds as varied as gulls and harriers (Pérez et al., 2010; García-Heras et al., 2017). Similarly, metallic pollution as a byproduct of industrial smelter plants has led to significant decreases in carotenoid-based color deposition. As a visual indicator that can be measured from a distance, pigmentary color display in birds is a far less intrusive method of assessing the impacts of anthropogenic pollution than sampling blood and tissue, especially in endangered or vulnerable species whose populations cannot afford to be sampled (Yang et al., 2021).
Perception and Signal Visibility
Visual modes of communication require that the signal passes to the receiver without undue disruption. Animals rely on ambient light for the visual processing of color signals—it can modulate the level of contrast between patches of color and thus the efficacy of the signal itself (Endler, 1980; Rosenthal and Stuart-Fox, 2012). For instance, for terrestrial animals that use warning coloration, the medium of ambient air is often subject to natural variation due to weather patterns and vegetation (Rosenthal, 2007). Anthropogenic changes in climate and habitat structure as well as the introduction of artificial light can alter the visual signaling environment beyond the normal threshold of variation (Lim et al., 2008). Forests are one such example of a habitat type whose lighting conditions are characterized by complex interplay between vegetation structure, weather, and time of day (Endler, 1993). While natural variation in ambient light in forests over the course of a day can be offset by minor behavioral adjustments, more dramatic changes brought about by logging and land clearing can result in animals being unable to communicate as effectively as before (Delhey and Peters, 2016). The presence of atmospheric aerosols due to anthropogenic emissions has been found to have decreased global clear sky visibility (Wang et al., 2009), with potential ramifications for taxa, such as sandhoppers, that rely on skylight radiance for orientation and navigation (Ciofini et al., 2021). The impacts of this decrease in irradiance on animal coloration have yet to be examined. Transitional light regimes, such as those seen at dawn and dusk, have also experienced changes due to light pollution (Spitschan et al., 2016). However, the impacts of these changes on the visual ecologies of non-human animals have yet to be investigated comprehensively. Similarly, elevated turbidity in aquatic systems due to eutrophication, sediment run-off, increased storm intensity from climate change and bottom dredging causes drastic changes in irradiance (van der Sluijs et al., 2011), thereby disrupting the medium of transmission and perception of visual signals.
The effects of water turbidity have been well documented in cichlids and guppies (Chapman et al., 2009; Camargo-dos-Santos et al., 2021). Seehausen et al. (1997, 2008) have ascertained, through lab experiments and field data, that changes in ambient light in Lake Victoria due to eutrophication have led to the hybridization of several differently colored cichlid species. As female cichlids prefer to mate with conspicuously colored males (Seehausen et al., 2008), the perception of these conspicuous colors is crucial to the maintenance of mating systems. Male nuptial coloration, often presented as bright reds or blues to offer a striking contrast against yellow light, is impacted by turbidity in two ways: (a) the lack of photosynthetically useful light causes declines in dietary sources of carotenoids and (b) the costliness of bright colors and the decline in ambient light causes males to develop duller colors over generations (Seehausen et al., 1997). Turbidity reduces the overall efficacy of color-based signals, followed by a loss or decline in male nuptial colors, and finally a decrease in overall species diversity and phenotypic diversity due to the loss of sensory barriers against hybridization. As sexual selection contributes significantly to species and phenotypic diversification and the maintenance of diversity in cichlids and guppies (Selz et al., 2014), a breakdown of these selective forces due to environmental conditions could lead to declines in the genetic diversity and by extension, perhaps to resilience of a population to further challenges.
Butterflies that rely on lighting environment for the efficacy of their defensive coloration mechanisms are particularly dependent on forest cover for survival. As they employ a combination of pigmentation and structural coloration to achieve a fine balance between color for thermoregulation and as an anti-predator strategy, significant changes in both climate and land use patterns mark them as especially vulnerable. In a wide-ranging comparative study of the effects of deforestation on coloration in Amazonian butterflies, Spaniol et al. (2020) show how in recent years, butterflies have experienced a reduction in diversity of defensive coloration strategies. Primary growth forests, especially in the tropics (Adams et al., 2014) offer a greater range of visual environments that are associated with specific color phenotypes. Species that inhabit low-light environments, i.e., primary growth forests with dense canopies, are more susceptible to population declines following disturbance events that convert them to higher-light environments (Patten and Smith-Patten, 2012). Through their sampling of butterflies in primary forests and early succession stage areas, Spaniol et al. (2020) find that butterflies that rely on dull colors and patterns (measured as saturation and hue) for camouflage continue to persist in recently disturbed forest sites, whereas conspicuously colored butterflies show declines. Brighter colored species may be exposed to greater predation risk due to the opening up of habitats from disturbance. This exposure is sometimes combined with the introduction of novel, invasive predators to the systems that have not undergone the learning processes that make conspicuous color patterns effective as warning signals (Ciuti et al., 2012). Overall, deforestation and the creation of smaller, more disturbed fragments of forest appear to select for lower color diversity as well as species diversity in neotropical butterflies (Spaniol et al., 2019, 2020). Butterflies are a particularly charismatic taxonomic group whose colors and patterns represent a large range of specialized adaptations closely associated with their habitats. As these habitats face extensive deforestation and changes to the light environment and predator composition, butterflies may be used as model taxa for the monitoring of forest composition and degradation.
Artificial light at night is a unique feature of modern human society that affects many aspects of animal behavior (Gaston et al., 2013). The advent of new lighting technologies such as the energy-efficient LED bulb to offset the energetic costs of older incandescent light bulbs has introduced a wider range of artificial spectra to nocturnal animals (Owens and Lewis, 2018; Desouhant et al., 2019). Fireflies and glow-worms are examples of nocturnal insects that use the darkness of the nighttime as a background against which to emit sexual signals. Elgert et al. (2021) investigated the effects of artificial light on sexual signaling in the glow-worm (Lampyris noctiluca) and found that female glow-worms ceased glowing when exposed to artificial light at night for an extended period of time. Briolat and others have shown how artificial light affects coloration too, specifically, using elephant hawkmoths (Deilephila elpenor) and the impact of different types of artificial lighting conditions on their pollination and anti-predator behavior (Briolat et al., 2021). As highly efficient and effective nocturnal pollinators, hawkmoths rely on their night vision systems to fly directly toward specific flowers. The authors tested the effect of artificial lights on the ability of moths to discriminate flower colors at night and found that broadband amber light, most commonly emitted from amber LED lights, inhibited color discrimination. With regard to anti-predator behaviors, they also found that narrow band light sources, such as orange LED, disrupt the moths’ ability to perceive and discriminate the color of backgrounds against which they choose to finally rest at night to avoid detection by diurnal avian predators the next day.
Functional Aspects of Coloration
Rapid environmental change can be expected to impact the functionality of color patterns as much as it does their acquisition and perception. One of the most striking effects of climate change on the adaptive function of coloration is seen in arctic mammals and birds that undergo seasonal color change to achieve crypsis against their changing backgrounds. As the duration of annual snow cover shortens with warmer temperatures each year (Post et al., 2009), arctic animals are faced with mismatching their backgrounds, leading to increased predation risk (Pedersen et al., 2017). Similarly, ectotherms that rely on coloration for thermoregulation must contend with warmer temperatures by either evolving lighter colored bodies or shifting their ranges to cooler and shadier regions. Changes to the landscape, in the form of forest fragmentation or wildfires, can compound on the effects of climate change and severely affect the distribution of morphotypes within a polymorphic population.
Climate Change in Mammals
Climate change has diverse effects on animal coloration (Figure 2). Some animals that inhabit upper temperate and polar regions or migrate between them undergo seasonal color change. These animals, primarily birds and mammals that use coat or plumage color polyphenism to camouflage against snow, are directly compromised by seasonal mismatches between daylength and modern changes in temperature and precipitation (Forrest and Miller-Rushing, 2010).
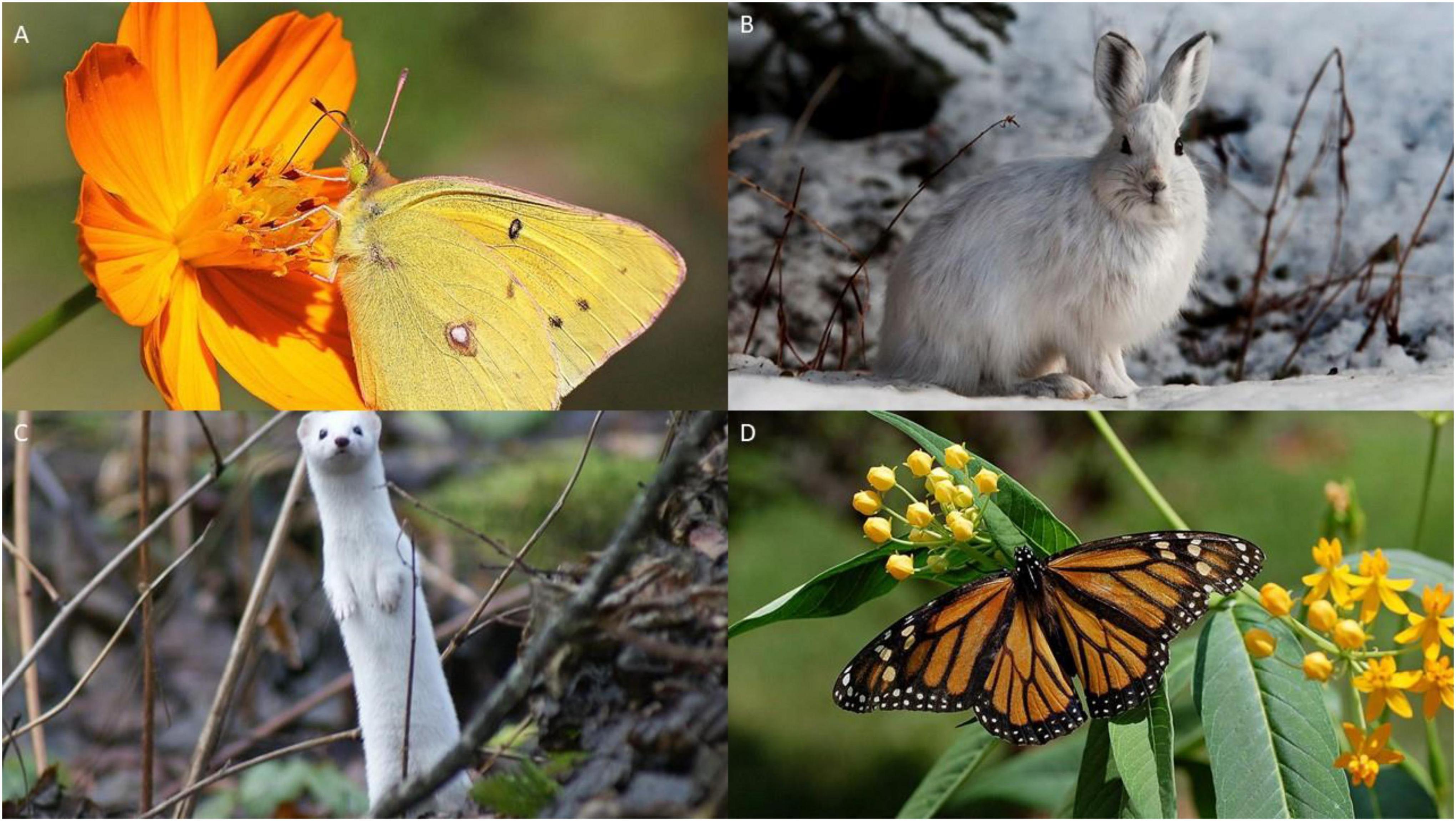
Figure 2. Animals whose colors are impacted by climate change. (A) The Orange sulfur butterfly (Colias eurytheme) in Nogales, Arizona by Alan Schmierer. C. eurytheme relies on wing color to reach optimal temperatures for flying and foraging. (B) Snowshoe hare (Lepus americanus) in Denali National Park by Tim Rains. Snowshoe hares are increasingly experiencing camouflage mismatch with decreases in snow cover due to climate change. (C) Least weasel (Mustela nivalis) in Bialowieza Forest by Stormbringer76. Weasels in their winter pelage are also experiencing camouflage mismatch with decreasing snow cover and period. (D) Monarch butterfly (Danaus plexippus) in Aston Township, Pennsylvania by Derek Ramsey. Monarch wing color darkness is important for flight endurance during migration.
Photoperiod is the primary driver of seasonal polyphenism in arctic mammals that undergo molting to match the backgrounds of their changing landscape (Zimova et al., 2018). Although photoperiod itself is not affected by climate change, the discrepancy between seasonal transitions and photoperiod is particularly important as a driver of increased mortality due to camouflage mismatch between coat color and habitat substrates that change with season (Zimova et al., 2020b). Snowshoe hares undergo seasonal molts to match their background, between a uniform brown coat in summer and a uniform white in winter, with intermediate phases of brown and white patches during the seasonal transitions in autumn and spring. In a series of studies on phenotypic plasticity of snow-shoe hares (Lepus americanus) in response to decreased snow cover and mismatches with coat color, researchers used data collected over 3 years on snowpack and color polyphenism to illustrate the extent of seasonal mismatch and associated mortality (Mills et al., 2013; Zimova et al., 2016, 2020a). A large portion of mortality is directly attributed to predation, making their predator interactions a primary selective pressure for local adaptations. Through their long-term study, the authors established a lack of or limited phenotypic plasticity in coat color phenology: onset of seasonal molt appeared to be fixed and determined by photoperiod rather than temperature and snow cover (Zimova et al., 2014). Increased color contrast between the hares’ coat color and their substrate led to increased mortality. Moreover, hares have not adjusted their behavioral defenses against predation to compensate for the decline in crypticity either (Zimova et al., 2014). Population and climate pattern modeling of mortality rates show that climate driven phenological mismatches are capable of driving severe population declines.
In another example, Atmeh et al. (2018) demonstrated the effects of seasonal camouflage mismatch on winter survival rates in the common weasel (Mustela nivalis). In the Białowieża Forest in Poland, two morphs of weasel—M. n. nivalis and M. n. vulgaris—occur sympatrically. While there are few differences in their summer coat coloration, the former undergoes a winter molt to a white coat, but the latter retains its brown coat year-round. Visually mediated predation by raptors and other larger mammals is often the largest mortality factor for weasels, making their ability to achieve crypsis against their seasonally changing background of utmost importance to survival (Zub et al., 2008). The investigators used a combination of weasel model-predator interactions and live-trapping of weasels over a decade to assess the population ratios of the two color morphs. In their field experiments, they used artificial weasel models that corresponded to the two morphs to determine predation risk induced by camouflage mismatch. They demonstrated that camouflage, or the lack thereof, was the most significant factor influencing detection by predators. They also found a negative but non-significant association between the detection rate and percentage snow cover. Through live trapping, they found that the proportion of M. n. nivalis, the morph that molts to white in winter, actually decreased parallel to the decrease in the number of days of snow cover in the forest. The authors thereby predicted that the two morphs will suffer different rates of mortality, with M. n. nivalis experiencing higher mortality than M. n. vulgaris with decreasing snow cover, perhaps leading to the fixation of the latter morph in the population over time.
Climate Change in Insects
Ectotherms that rely on body color for thermal regulation are particularly affected by changing global temperatures. Darker colored animals have an advantage over lighter colored animals in cooler climates as they are more efficient at increasing their body temperatures above that of the ambient atmosphere (Clusella-Trullas and Nielsen, 2020). In warmer climates, however, lighter colored ectotherms can remain active in the day for longer. This constraint on color and thermoregulatory abilities has led to seasonal polyphenisms in several insect species, particularly well documented in the North American sulfur butterflies of the genus Colias (Watt, 1968, 1969; Hoffman, 1978; Kingsolver, 1983a,b; Kingsolver and Buckley, 2015). Summer morphs of both species display orange or yellow colors on their hindwings, due to pteridine pigments, while spring and fall morphs show a darker underside of their hindwings, due to the replacement of pteridines with melanin. In their normal resting position, the hindwings are folded upward, exposing their undersides to the absorption of solar radiation. The lighter color of the summer morph minimizes overheating, enabling it to stay active longer in the day. The butterflies are triggered by a photoperiodic change from the longer summer months to shorter winter months to undergo morphological changes to their wings. Watt (1968, 1969) studied the mechanism of polyphenism in C. eurytheme and the effect of temperature on butterfly activity patterns, specifically, daily flying time. He demonstrated that the darker butterflies reached high body temperatures needed for flying longer in the day even under lower ambient temperatures, entailing an adaptive advantage according to season and photoperiod. Hoffman (1978) also investigated the correspondence between photoperiod and hindwing darkening through laboratory experiments and field observations in Central California by exposing larval instars of C. eurytheme at each stage to different photoperiods and ambient temperatures. He found that seasonal polyphenism is controlled by photoperiod during larval development, and that temperature during this time has no significant effect on seasonal hindwing darkening. Kingsolver and Buckley (2015) investigated melanin variation in alpine and sub-alpine populations of Colias meadii to estimate the impacts of climate change on butterfly fitness (measured as net reproductive rate) and thermoregulatory traits (wing solar absorptivity, measured as degree of melanism on ventral hindwings). They then used the results of experiments conducted previously (Kingsolver, 1983a,b; Buckley and Kingsolver, 2012) and historic climate data from 1955 to 2010 to construct models that predict the direction of selection and the evolutionary responses to climate change. Their models predicted selection for decreased wing melanin as temperatures rise, but the degree of selection remained weak, suggesting that evolutionary responses to climate change may not match the rate of change of environmental conditions.
Monarch butterflies, whose migration cycles are the source of much concern in recent years (Zylstra et al., 2021), depend on wing coloration for their flight ability. Indeed, the link between butterfly wing color and flight ability has been established through studies by of Pieris butterflies in North America (Kingsolver and Wiernasz, 1991; Kingsolver, 1995). The two types of pigments responsible for the iconic wing colors of monarchs (Figure 2) are pteridine and melanin. The ability of monarchs to successfully navigate their migratory path is dependent on the thermoregulatory capacity of their wings, which is a function of wing surface coloration. Wild-caught monarchs with redder or darker shades of orange flew longer distances in the laboratory setting (Davis et al., 2012). Another study by Davis (2009) found that migrating butterflies tended to be redder than individuals in the overwintering or breeding stages. These results build on a study (Hanley et al., 2013) that found wild monarchs captured from the Gulf coast (warmer temperatures) were more melanized than monarchs from the Great lakes (colder temperatures). Monarchs that fly longer distances, i.e., those sampled at the Gulf coast, exhibit darker colored wings in order to absorb more solar energy. While monarch migration has been put forth as a complex trait to be used to study the impacts of climate change on population dynamics (Green, 2021), the strong link between wing color and flight performance during migration may allow us to use of wing color traits as a biological indicator of environmental change.
Zeuss et al. (2014) extended the relationship between coloration and thermoregulation to entire clades by conducting a phylogenetic comparison of butterflies and dragonflies’ color lightness and thermal environments to demonstrate a correlation between the two. Their models showed that ventral wing surfaces became lighter with increasing temperatures in European butterflies. The authors also found that dragonfly assemblages across Europe became lighter colored over the last century. They attribute this directional change to climate warming forcing a skew in thermoregulatory coloration. They also predict range shifts in darker colored insects toward cooler and shadier regions.
As insect clades experience shifts toward color lightness or darkness due to broad changes in climate patterns, the plant assemblages they interact with are also expected to shift to adapt to an altered sensory and thermal landscape too (Shrestha et al., 2018). Flowers that generally rely on color to signal to pollinators must reach a trade-off between thermoregulation through pigmentary absorption of solar radiation and colors that are visible to their specific insect pollinator’s vision systems (Lacey et al., 2010). With increasing temperatures and altered precipitation patterns, an imbalance in this trade-off can arise (Koski et al., 2020). Although anthocyanin-based colors are a strong influence on plant-pollinator interaction, anthocyanin also functions as a photosynthetic and photoprotective pigment (Gould, 2004). The reproductive structures of flowers are heat-sensitive and rely on pigments to regulate internal temperatures (van der Kooi et al., 2019). Using historical data from herbarium specimens and historic climate data (temperature, precipitation, and vapor pressure deficit), Sullivan and Koski (2021) assessed changes in floral pigmentation over 124 years in 12 North American polymorphic species that spanned 10 genera. They scored herbarium species as pigmented (red, pink, blue, or purple), unpigmented (white) and mixed. Color polymorphic species that experienced larger increases in aridity showed an increase in frequency of pigmented specimens over the timeframe. On the other hand, species that underwent larger increases in temperature in their respective environments showed a decline in the number of pigmented specimens. As warmer temperatures are often accompanied by and even exacerbate the effects of aridity in regions like western and southwestern North America, this creates conflicting selective forces for anthocyanin pigmentation. We may then expect to see new distributions of pigmented and unpigmented morphs, based on novel microclimates and niches created by climate change.
Landscape Change
The interaction of habitat fragmentation and climate change as separate but interlinked creates new environments that force species to either adapt rapidly or perish. While climate change may cause directional selection for traits that ameliorate thermal and drought related stresses, landscape change narrows the breadth of adaptations possible by reducing the potential for range expansion (Figure 3). Deforestation and intense fire events that have increased in frequency in recent years (Cattau et al., 2020) are two such habitat modifying forces that severely affect the species composition of ecosystems as well as intraspecific phenotypic variation. The Eastern red-backed salamander (Plethodon cinereus) has been used by Cosentino et al. (2017) to study the combined effects of forest fragmentation and climate change on morphotype distribution. The loss of or fragmentation of forests leads to greater edge effects such as drier and warmer soil conditions (Murcia, 1995; Laurance, 2004). These effects are exacerbated by warmer temperatures caused by climate change. Selection for climate ameliorating traits may then be either intensified by the loss of habitat or dampened by the regeneration of forest patches. Coupled with warmer temperatures owing to climate change, forest fragments represent a shortage of thermal refuges (Monasterio et al., 2009). As a terrestrial amphibian that requires moist and cool conditions, the red-backed salamander is particularly vulnerable to changes in temperature and moisture. Plethodon cinereus occurs as two discrete, genetically based color morphs that are differentially distributed in the forests of eastern North America. The unstriped (black) individuals are linked to warmer and drier microclimates than striped individuals. Cosentino et al. (2017) used historical data from 1880 to 2013 as well as data they collected between 2013 and 2015 on salamander morph frequencies, climate variables and forest cover. They modeled the interactive effects of mean annual temperature and forest cover on the proportion of striped individuals and found that years with higher temperatures saw a greater proportion of striped individuals in areas with higher forest cover. The proportion of striped morphs was also lower in areas that had experienced forest loss due to urbanization and agriculture, owing to the loss of thermal refuges. Their results support the suggestion that landscape factors can either exacerbate or ameliorate the effects of climate change. This association between genetically based color morphs and microclimates also lays down the potential for sympatric speciation with further warming and forest fragmentation. The red-backed salamander may rely on the persistence of multiple color morphs through, frequency dependent selection (Fitzpatrick et al., 2009) to survive predation, the greatest contributor to salamander mortality (Grant et al., 2018). Each of these morphs has also been shown to exhibit different anti-predator behaviors (rates of tail autotomy) that may be genetically correlated with coloration (Venesky and Anthony, 2007). Sympatric speciation along the basis of color could perhaps put each resulting species at greater risk of extinction without the buffering effects of polymorphism and frequency dependent selection (Forsman et al., 2008).
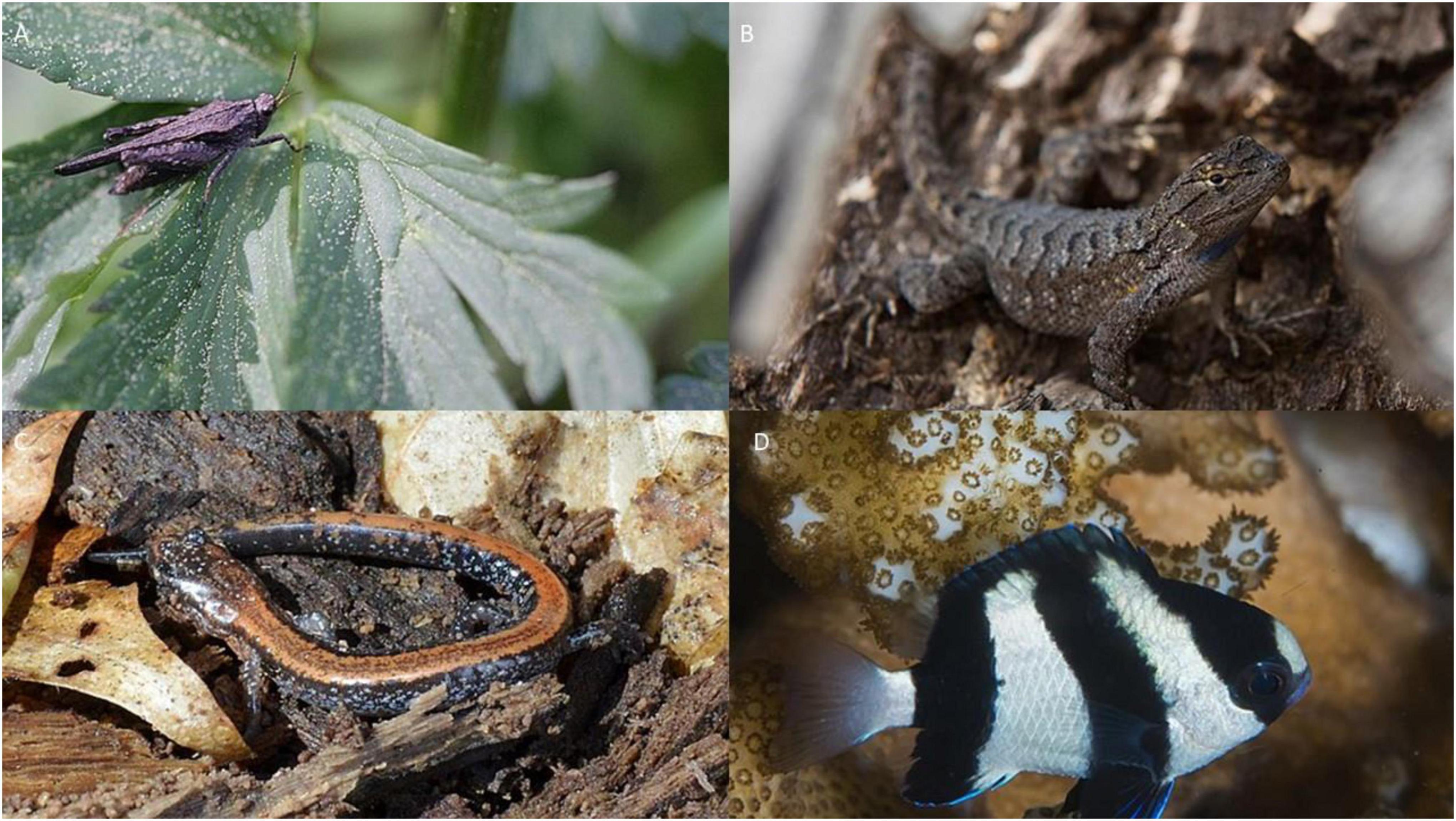
Figure 3. Animals whose coloration are impacted by landscape change. (A) Pygmy grasshopper (Tetrix subulata) by Hedwig Storch. Melanic morphs of the pygmy grasshopper are favored over non-melanic morphs after a fire event. (B) Western fence lizard (Sceloporus occidentalis) in Joshua Tree National Park by Hannah Schwalbe. The western fence lizard has been shown to perch on burnt twigs after a fire event to match its background better. (C) Eastern red-backed salamander (Plethodon cinereus) in Mississauga, Ontario by Ryan Hodnett. The striped morph of the eastern red-backed salamander relies on cooler and more moist microclimates that are declining due to forest fragmentation and climate change. (D) Humbug dacyllus (Dascyllus aruanus) in Lembeh, Indonesia by Rickard Zerpe. The dascyllus is one species of reef-dwelling fish that is experiencing greater predation rates due to coral bleaching.
Fire
Fire melanism is a well-documented trait occurring in animals that inhabit fire-dominant ecosystems (Karlsson et al., 2008). In regions that experience a fire season, habitat succession and the associated changes in visual environments are major drivers of polymorphisms and polyphenisms (Hocking, 1964). The stability of these succession cycles may be threatened by changes in climate and land use patterns that have led to a marked increase in frequency and intensity of fires in these regions (Steel et al., 2015). Color polymorphic animals that inhabit ecosystems shaped by fire often have melanistic and non-melanistic morphs. Rocha et al. (2015) used coloration, specifically, the frequency of darker individuals and darker species to assess the impact of fire disturbance events on the composition of Brazilian grassland insect assemblages. The authors sampled insects from five orders and categorized individuals into 52 morphotypes. Measuring color phenotype distribution through color density (high density meant brighter tones, low density meant darker tones), their results showed higher frequencies of darker colors in burned areas and higher frequencies of lighter colors in unburned areas. Rowell (1971) describes three possible mechanisms by which melanic morphs may be distributed in a fire shaped landscape—(a) natural selection for dark morphs on a dark substrate, (b) phenotypic plasticity in response to fire and associated darkened substrates, and (c) behaviorally different dispersal patterns. In order to establish a causal link between fire events and increased predation on lighter phenotypes in burned, darkened substrates, one would need direct evidence of altered predator-prey interactions.
Karpestam et al. (2012, 2013) do just this with the pygmy grasshopper (Tetrix subulata) in human predation trials. Over the course of two studies, the first a capture-mark-recapture study of natural populations of T. subulata in the wild in southwest Sweden, and the second a controlled human detection experiment, they examined differences in rates of detection for images of the three color morphs of grasshoppers against a range of naturally occurring backgrounds. In order to validate the use of humans as “predators” for their detection study, they compared the rate of detection of grasshopper images on computer screens to rates of capture of live individuals from their previous study (Karpestam et al., 2012) and to morph frequencies in the wild. They found that while no singular color morph was cryptic in all visual backgrounds, the detection rate of each of the three morphs was different across backgrounds that represented the various stages of habitat succession following a fire event. The authors established the context dependency of crypsis in a color polymorphic species and the idea that visual predation and background matching may contribute to the differential distribution of dark and light phenotypes in a post-fire environment. This association between adaptive color polymorphism and habitat heterogeneity provides a marker for the conservation of habitat complexity and the prevention of fragmentation.
The chaparral ecosystem of California is characterized by a fire season that is crucial to the community ecology and assemblages of that region. Over the course of a seasonal cycle, the landscape undergoes significant changes to its visual characteristics, ranging from dark and soot covered immediately post fire to verdant in the winter. Background matching and selective perching behavior in the western fence lizard (Sceloporus occidentalis) have been studied as adaptations to fire (Lillywhite et al., 1977). The lizards are dark colored and have been found, through field sampling after the Laguna fire of 1970 and laboratory-based choice tests, to selectively perch on darker colored stalks after a fire. As the successive stages of post-fire recovery ensue, the lizards perch on different substrates, such as dark colored rocks, to match their background. While the efficacy of their background matching has not been tested with predation trials, the authors hold that predation may be the selective force driving the maintenance of this adaptation.
Marine
The wide-ranging impacts of coral bleaching caused by climate change have been well documented, but the exact causes of declines in coral associated fish species have yet to be comprehensively laid out with empirical evidence. Coker et al. (2009) have attempted to address this knowledge gap with their study on the predation of reef fishes after a coral bleaching event. Coral reefs are a crucial feature of benthic ecosystems, providing complex habitat structure that mediates and maintains biotic interactions. The disappearance of colors from reefs due to bleaching events effectively removes the background against which reef-dwelling fish have evolved complex colors and patterns for a range of functions. The authors found that two species of Indo-Pacific damselfish (Pomacentrus moluccensis and Dascyllus aruanus) experienced greater rates of predation due to mismatches between the bleached coral background and body coloration.
Hemingson et al. (2022) recently extended this link between coral bleaching events and reef fish body coloration by developing and using a novel measure of color at the community level. Using reef community composition data collected over 27 years from the Great Barrier Reef, they looked at broad changes in the color composition of the cryptobenthic reef-dwelling fish community after mass bleaching events. Immediately following a bleaching event in 1998, the focal reef community saw a decline in color area (a quantitative measure of fish abundance derived from non-metric multidimensional scaling) of over 30%. The development of community-level measures of coloration is a crucial step toward connecting visual ecology and conservation biology. The authors of the study have also particularly used the human visual system (RGB colorspace) to quantify coloration and to draw attention to the aesthetic function of coral reefs in garnering interest in marine conservation.
Large scale changes to the marine landscape can include increased levels of anthropogenic noise pollution. While the bulk of literature addressing the ecological consequences of noise from ports and ships examines their effects on acoustic channels of communication and signaling, Carter et al. (2020) showed that noise may have far reaching effects on carapace color change in the shore crab (Carcinus maenas). They tested the effects of ship noise on changes to carapace luminance in juvenile shore crabs over molts using playback experiments over 8 weeks. Crabs exposed to ship noise were found to have changed luminance significantly less than those exposed to control ambient noise. Luminance is often the most elementary measure of background and thus lends itself as a good indicator trait for anthropogenic stresses. Ship noise also resulted in crabs being less camouflaged to predator vision as modeled digitally. As camouflage and other visual defenses are the first layer of protection against predators, crabs must also adopt secondary defense mechanisms to employ once spotted by a predator. The authors also examined the effects of loud ship noise on juvenile crabs’ ability to respond to an artificial predator. Individuals exposed to the ship noise treatment were found to react to predation less often, and retreat into refuges slower than those exposed to ambient control noise. While the study did not look at the physiological mechanisms behind the effects of loud noise on luminance and color change, the authors posit that stress induced by noise may affect differential investment of resources (Sokolova et al., 2012) as well as the endocrinology of individuals. Color change and luminance change are tied to molting, which is regulated by hormones that are also associated with stress (Chung et al., 1999; Chung and Zmora, 2008). Luminance change during a molt is an energetically costly process to undergo and the energy budget is strained further by the increased metabolic rate associated with noise-induced stress (Wale et al., 2013).
Application
Thus far in the review, we have presented the impacts of anthropogenic landscape and climate change on the production, perception, and functionality of color patterns in a range of taxa. In demonstrating the inhibitory effects of environmental pollutants on the acquisition of pigments and pigmentary precursors, we suggest the use of ornamental color traits as non-invasive indicators of environmental change. The disruption of color signals and communication in terrestrial and aquatic systems by forest fragmentation, artificial light, and turbidity raises implications for the conservation of these systems. These anthropogenic modifications to the environment are particularly important as novel stimuli that can severely affect species and populations with low genetic or phenotypic variation and low levels of plasticity to accommodate these changes. The close link between the functionality of adaptive color phenotypes and climate and landscape factors especially highlights the need for conservation policies and practices that incorporate coloration and visual ecology.
Environmental Indicators
Coloration can be an indicator of environmental change. For example, ocean color remote sensing (OCRS) monitors ocean phytoplankton at potentially very large geographic scales. Started in 1998, the advantages of OCRS are access to biogeochemical proxies in remote areas, coverage several times each day, and the capability of separating chlorophyll concentrations and irradiance. Since it is satellite driven, OCRS can be obscured by cloud cover, sea ice or turbidity of river water that limit coverage (Babin et al., 2015). Nonetheless, ocean coloration can give us insights into the role of phytoplankton in marine biogeochemistry, the global carbon cycle, and responses of marine systems to climate change. It is a means by which we can monitor algal blooms, sediment plumes, and coastal eutrophication (Groom et al., 2019).
At a smaller scale, coral bleaching is a sensitive measure of the effects of changing sea temperatures because coral polyps expel zooanthellae which produce reactive oxygen species with increasing temperatures, and these are toxic to corals. Other triggers of bleaching are solar irradiance, changes in salinity, pollution, and ocean acidification (Hoegh-Guldberg, 1999). Rapid bleaching in response to environmental stress and the impact it has on the public make it an effective rallying point for addressing rising sea temperatures.
Normalized difference vegetation index is a method of measuring reflectance of the planet in the red and near-infrared bands (NIR) as viewed by satellite (Carlson and Ripley, 1997). Live plants absorb solar radiation but leaves re-emit it in the near-infrared because wavelengths > 700 nm are too small to be involved in molecule synthesis within the plant. Thus, normalized difference vegetation index is the ratio of (NIR – red)/(NIR + red) such that positive values indicate dense vegetation cover and hence intact undisturbed forests. This tool is also compromised by cloud cover, soil reflectance and atmospheric effects, especially water vapor.
Coloration as an Instrument for Conservation
There are numerous anecdotes and limited evidence that coloration in nature attracts people to it and promotes conservation sympathy (Caro et al., 2017). Temperate deciduous regions that witness autumnal color changes in foliage color are sources of seasonal tourism (Hall et al., 2011). In North America, people speak fondly of the colors of autumnal deciduous leaves in New England, the mid-west and in Canada and vacations are structured around these events. “Leaf peeping” as a tourist activity has seen a recent resurgence with lockdowns due to the COVID-19 pandemic forcing people to rediscover natural spaces in their neighborhoods (McMahon, 2020). Similarly, spring wildflower super-blooms (Figure 4) in California deserts and vernal meadows attract tourists each year. Accumulating evidence on the effects of natural spaces on human health shows that ecosystems with higher species diversity are likely to provide greater eco-system services in urban areas (Aerts et al., 2018). As our review demonstrates the link between biodiversity and color diversity, human health benefits may present a more compelling angle from which to push for the protection of natural spaces.
The Woodland Trust in United Kingdom advertises membership using brightly colored spring flowers.1 Examples of colorful animals being used in conservation are numerous and include poison dart frogs, toucans, parrots, and birds of paradise. For instance, penguin species with brighter red or yellow coloration feature more in publications (Stokes, 2007). In an experimental study, 19–29 year-old Czech citizens preferred lighter colored passerines, especially blue and green birds, although they preferred patterned birds if they had been manipulated to a gray scale (Lišková et al., 2015). Thömmes and Hayn-Leichsenring (2021) discovered that the birds with red color patches received more “likes” on Instagram than those with yellow patches while blue received more than any other color (Figure 5). At an institutional level, many nations prefer colorful species as their national birds (Table 2 and Figure 6).
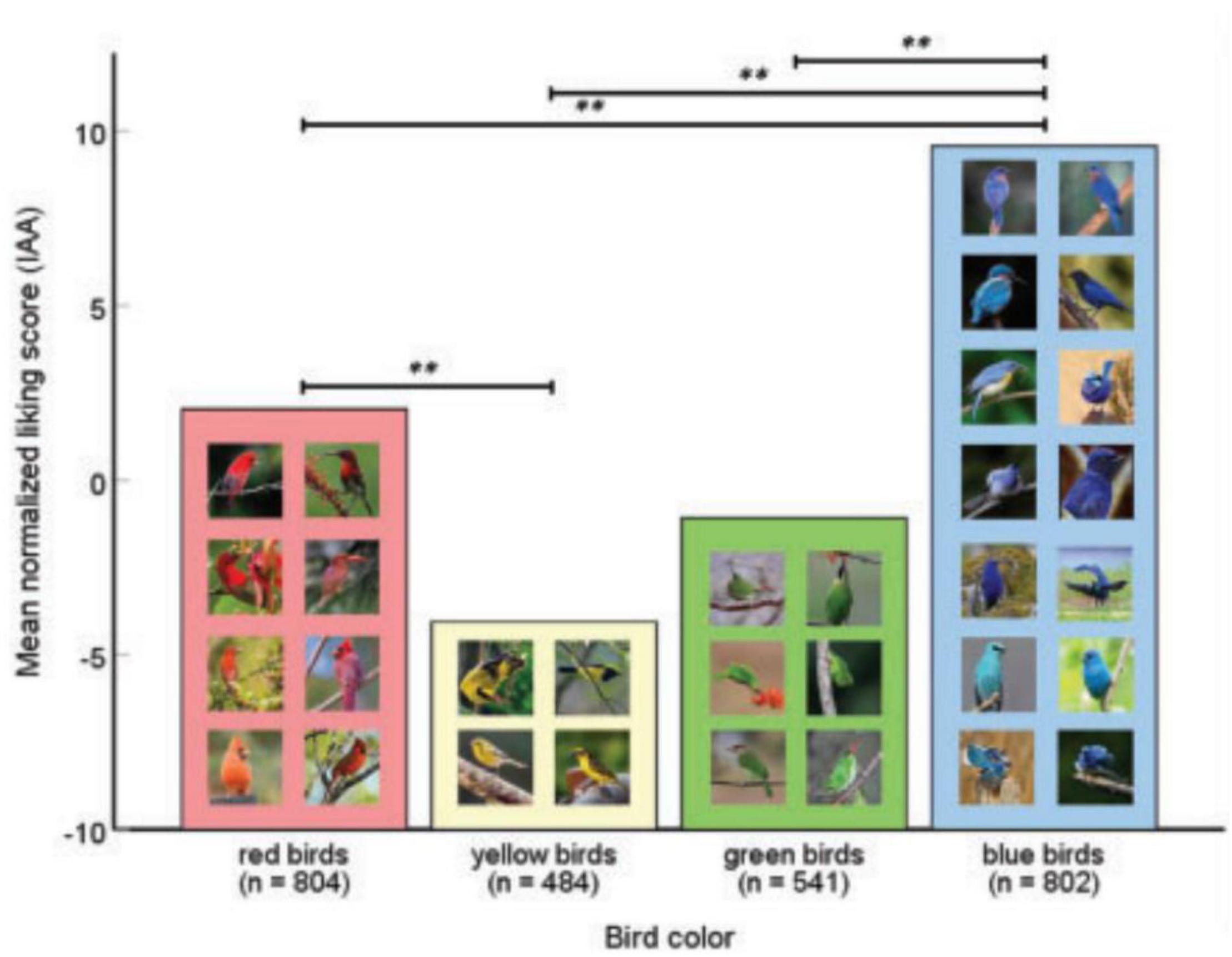
Figure 5. Human bird color preferences (η2 = 0.028). **The mean difference is significant at the 0.01 level. Adapted from Thömmes and Hayn-Leichsenring (2021).
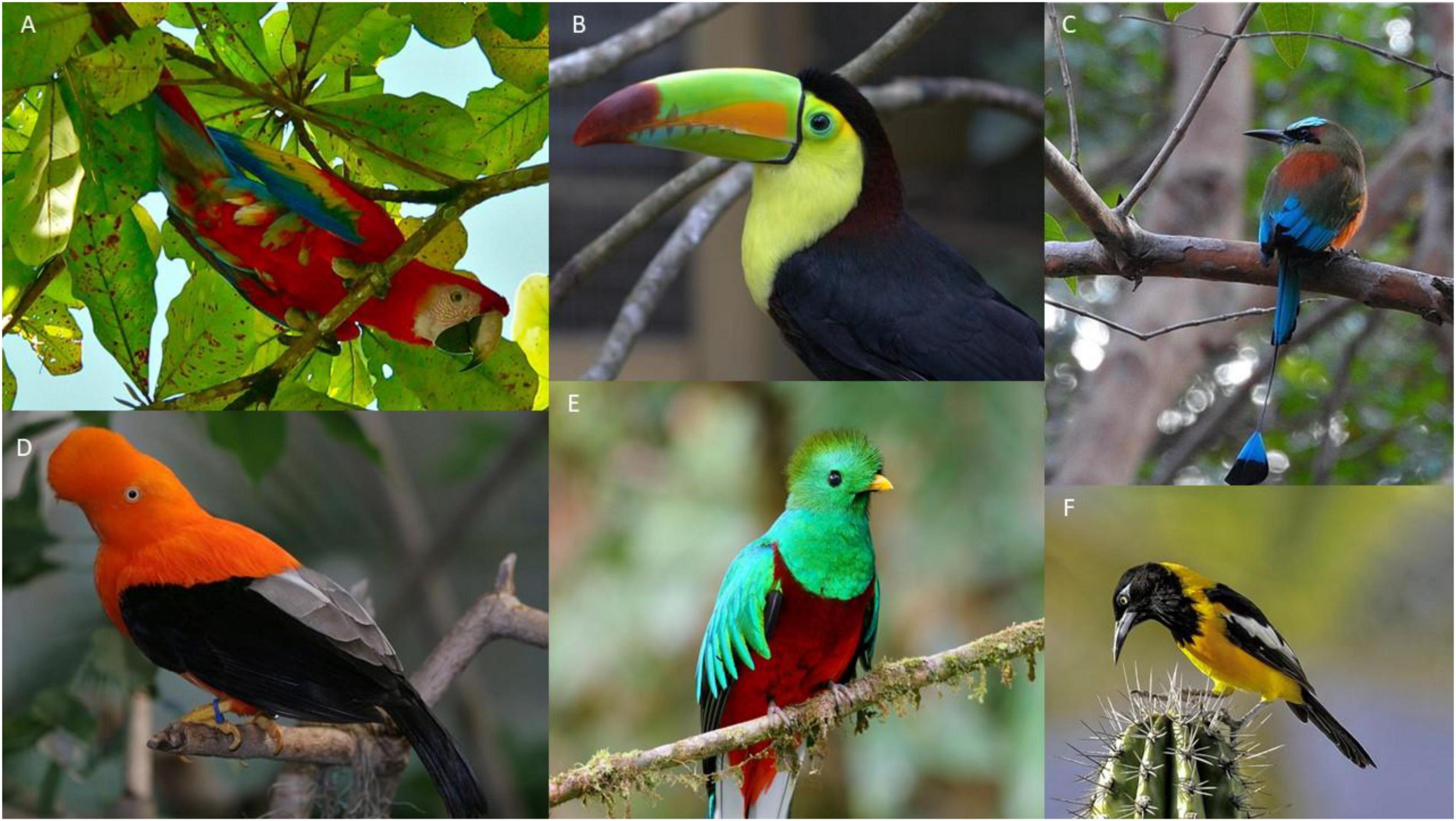
Figure 6. National birds of South American countries. Clockwise from the left: (A) Scarlet Macaw (Ara macao), the national bird of Honduras, by Bernard Dupont in Puerto Jimenez, Costa Rica. (B) Keel-billed toucan (Ramphastos sulfuratus), the national bird of Belize, by Lauri Vain at Macaw Mountain Bird Park and Nature Reserve, Honduras. (C) Turquoise browed motmot (Eumomota superciliosa), the national bird of Nicaragua and El Salvador, by Asa Berndtsson in Costa Rica. (D) Andean cock-of-the-rock (Rupicola peruviana), the national bird of Peru, by Jerry Thomspon at San Diego Zoo, United States. (E) Resplendent Quetzal (Pharomachrus mocinno), the national bird of Guatemala, by Cephas in Monteverde, Costa Rica. (F) Venezuelan Troupial (Icterus icterus), the national bird of Venezuela by Betty Wills at Bonaire, Venezuela.
Some systematic research has explored the ability of coloration in animals to generate willingness to pay for conservation activities. Prokop and Fančovičová (2013) manipulated pictures of conspicuous aposematic taxa and showed them to 10–20 year-old children and young adults in Slovakia. They found that conspicuous pictures elicited a greater willingness to protect the same species rendered cryptic. Curtin and Papworth (2020) found that British people from across a wide span of age-groups preferred imaginary animals that were multi-colored as opposed to those with fewer colors and that this went some way to predicting donations made to single species charities. These studies have implications for choosing flagship species that advertise nature and as educational tools, symbolize a nation’s natural heritage, promote the profile of an NGO, raise money, or help set up a reserve (Figure 7; Caro, 2010). But they carry a warning because colorful species may be more likely to be traded than duller species. Frynta et al. (2010) asked respondents to rank parrot species according to perceived beauty. They preferred species that were colorful, large, and long-tailed. Perceived beauty was positively correlated with size of the worldwide zoo population but not IUCN listing indicating zoos target beautiful but not endangered parrots for breeding.
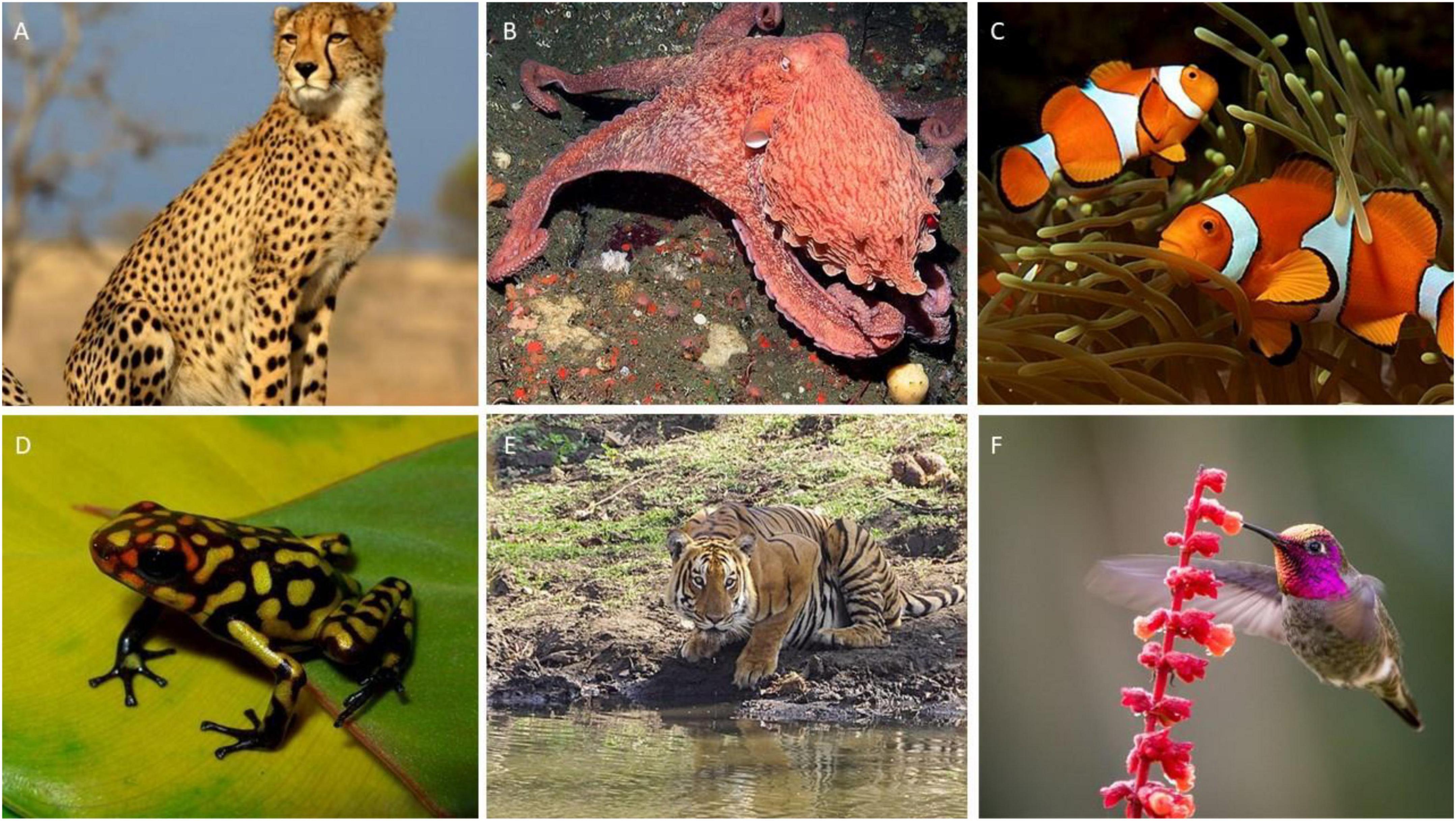
Figure 7. Charismatic and colorful flagship species from around the world used in conservation and restoration campaigns. Clockwise from top left: (A) Acinonyx jubatus (Cheetah in Sabi Sands by James Temple), one example of colorful and charismatic but endangered species used to head conservation campaigns and used often to promote eco-tourism and mammal conservation research in the southern African countries. (B) Enteroctopus dofleini (GFNMS—Giant Pacific Octopus—NOAA). Marine conservation organizations and the Monterey Bay and Seattle aquaria along the west coast of North America have used the Pacific giant octopus in promotional campaigns and merchandize to raise funds for their projects. (C) Amphiprion ocellaris (Clown anemonefish in Papua New Guinea by Nick Hobgood). The clown anemonefish (Amphiprion ocellaris) is instantly recognizable as a representative of tropical coral reefs and a recent study by Boudin et al. (2020) shows the effectiveness of the clownfish as a flagship to encourage conservation practices in young children, particularly in the context of the popular film Finding Nemo. (D) Oophaga histrionica (Harlequin poison frog by Mauricio Rivera Correa), often targeted by poachers for the illegal pet trade due to their striking color patterns. (E) Panthera tigris (Tiger drinking water, Mudumalai National Park by Timothy A. Gonsalves), the iconic symbol of Indian wildlife conservation, with the establishment of protected areas such as national parks and tiger reserves based around tiger populations. (F) Calypte anna (Anna’s hummingbird, UC Berkeley Botanic Garden by Becky Matsubara), with its striking patch of iridescence, is used by University of California Master Gardeners to encourage California residents to convert monoculture residential lawns to native wildlife supporting gardens.
Avenues for Future Research
Here, we briefly outline a series of future research possibilities on coloration and conservation as they relate to production, perception, function and application. Regarding the production of color, we see that the establishment of baseline levels of color diversity and saturation in populations is important for monitoring trends and trajectories of the evolutionary ecology of taxa. Using color as a qualitative measure of the health of an ecosystem presents a relatively non-invasive avenue for investigating the effects of large-scale landscape changes on phenotypic diversity, and by extension, the general biodiversity of a system. As this review and others (Lifshitz and St Clair, 2016; Peneaux et al., 2021) have established, environmental stressors such as metallic pollutants and herbicides have traceable impacts on the acquisition and deposition of pigments such as carotenoids and melanins in a range of avian species. The investigation of the effects of these stressors on other color pigments such as pteridines, psittacofulvins, and ommochromes presents another valuable path for future research. This would also widen the range of taxa capable of being monitored on the basis of coloration.
With regard to color perception in anthropogenically altered habitats, it is necessary to establish large scale quantitative measures of color traits along gradients of environmental change. These metrics may be constructed through systematic meta-analyses of each eco-system type to create standards against which climate change and landscape change can be assessed. By determining linkages between the phenotypic plasticity of color traits to genetic and epigenetic variation, we may more accurately evaluate the mechanisms by which color traits are affected by anthropogenic changes to the environment. Finally, color based measures of environmental changes may present more visually appealing ways of engaging with the general public when communicating climate and conservation science.
Conclusion
Reviews that bridge the link between visual ecology and human induced environmental changes are limited at present (Delhey and Peters, 2016; Peneaux et al., 2021; López-Idiáquez et al., 2022). While sensory ecology research relies heavily on the observation of changes in the environment—for example, seasonal camouflage and associated color change, seasonal polyphenism in butterflies—anthropogenic changes to the environments are only recently being factored into coloration studies. Delhey and Peters (2016) addresses the documented effects of changes in the visual environment on visual ecology, while we have attempted to cover the impacts of broader anthropogenic changes in climate and land-use. Peneaux et al. (2021) lays out an argument for carotenoid-based coloration in avian species to be used as an indicator of environmental pollution for monitoring the health of wild populations. While the bulk of literature studying the effects of environmental pollution on pigment production and deposition is skewed toward avian species, we attempt to make a case for using color as an indicator of pollution. The effects of anthropogenic noise on acoustic communication and signaling have been well-documented, with recent reviews by Chhaya et al. (2021) and Duquette et al. (2021) comprehensively covering the implications of anthropogenic noise and acoustic ecology for conservation.
In this review we have tried to unpick the relationship between coloration and anthropogenic change to show that coloration in nature as viewed in 1900 will not be the same as coloration viewed in 2100. Changes will be brought about by alterations in pigment production and structural coloration influenced by dietary changes, themselves mediated by landscape change. Changes in air or water transmission media will create new selection pressures on signal coloration usually making species less colorful. Changes in climate and landscape will alter the strength of selection by effecting coloration-background mismatch in color changing organisms and polymorphic species. More optimistically, coloration as an indicator of landscape change, and at a large scale has the potential to attract people’s interest in nature and promote funding and effort toward the conservation agenda. Nature’s palette is always changing due to alteration in natural and sexual selection pressures but now we can expect some rapid changes ahead.
Author Contributions
MK collected the literature and drafted the manuscript. TC conceived of the study, revised, and edited drafts. Both authors contributed to the final manuscript.
Conflict of Interest
The authors declare that the research was conducted in the absence of any commercial or financial relationships that could be construed as a potential conflict of interest.
Publisher’s Note
All claims expressed in this article are solely those of the authors and do not necessarily represent those of their affiliated organizations, or those of the publisher, the editors and the reviewers. Any product that may be evaluated in this article, or claim that may be made by its manufacturer, is not guaranteed or endorsed by the publisher.
Footnotes
References
Adams, J. M., Kang, C., and June-Wells, M. (2014). Are tropical butterflies more colorful? Ecol. Res. 29, 685–691. doi: 10.1007/s11284-014-1154-1
Aerts, R., Honnay, O., and Van Nieuwenhuyse, A. (2018). Biodiversity and human health: mechanisms and evidence of the positive health effects of diversity in nature and green spaces. Br. Med. Bull. 127, 5–22. doi: 10.1093/bmb/ldy021
Alonso-Alvarez, C., and Galván, I. (2011). Free radical exposure creates paler carotenoid-based ornaments: a possible interaction in the expression of black and red traits. PLoS One 6:e19403. doi: 10.1371/journal.pone.0019403
Atmeh, K., Andruszkiewicz, A., and Zub, K. (2018). Climate change is affecting mortality of weasels due to camouflage mismatch. Sci. Rep. 8:7648. doi: 10.1038/s41598-018-26057-5
Babin, M., Bélanger, S., Ellingsen, I., Forest, A., Le Fouest, V., Lacour, T., et al. (2015). Estimation of primary production in the Arctic Ocean using ocean colour remote sensing and coupled physical-biological models: strengths, limitations and how they compare. Prog. Oceanography 139, 197–220. doi: 10.1016/j.pocean.2015.08.008
Bortolotti, G. R., Fernie, K. J., and Smits, J. E. (2003). Carotenoid concentration and coloration of American Kestrels (Falco sparverius) disrupted by experimental exposure to PCBs. Funct. Ecol. 17, 651–657. doi: 10.1046/J.1365-2435.2003.00778.X
Bortolotti, G. R., Tella, J. L., Forero, M. G., Dawson, R. D., and Negro, J. J. (2000). Genetics, local environment and health as factors influencing plasma carotenoids in wild American kestrels (Falco sparverius). Proc. R. Soc. B: Biol. Sci. 267, 1433–1438. doi: 10.1098/rspb.2000.1160
Boudin, E., Carcaillet, F., Tribot, A. S., Carabeux, Q., Deter, J., Claverie, T., et al. (2020). Coral reef fish: not just a matter of beauty! Front. Young Minds 8:13. doi: 10.3389/frym.2020.00013
Briolat, E. S., Gaston, K. J., Bennie, J., Rosenfeld, E. J., and Troscianko, J. (2021). Artificial nighttime lighting impacts visual ecology links between flowers, pollinators and predators. Nat. Commun. 12:4163. doi: 10.1038/s41467-021-24394-0
Buckley, L. B., and Kingsolver, J. G. (2012). The demographic impacts of shifts in climate means and extremes on alpine butterflies. Funct. Ecol. 26, 969–977. doi: 10.1111/j.1365-2435.2012.01969.x
Camargo-dos-Santos, B., Gonçalves, B. B., Bellot, M. S., Guermandi, I. I., Barki, A., and Giaquinto, P. C. (2021). Water turbidity-induced alterations in coloration and courtship behavior of male guppies (Poecilia reticulata). Acta Ethol. 24, 127–136. doi: 10.1007/s10211-021-00369-8
Carlson, T. N., and Ripley, D. A. (1997). On the relation between NDVI, fractional vegetation cover, and leaf area index. Remote Sensing Environ. 62, 241–252. doi: 10.1016/S0034-4257(97)00104-1
Caro, T. (2010). Conservation by Proxy: Indicator, Umbrella, Keystone, Flagship, and Other Surrogate Species. Washington, DC: Island Press.
Caro, T., Stoddard, M. C., and Stuart-Fox, D. (2017). Animal coloration research: why it matters. Philos. Trans. R. Soc. B. 372:20170047. doi: 10.1098/rstb.2016.0333
Carter, E. E., Tregenza, T., and Stevens, M. (2020). Ship noise inhibits colour change, camouflage, and anti-predator behaviour in shore crabs. Curr. Biol. 30, R211–R212. doi: 10.1016/j.cub.2020.01.014
Cattau, M. E., Wessman, C., Mahood, A., and Balch, J. K. (2020). Anthropogenic and lightning-started fires are becoming larger and more frequent over a longer season length in the U.S.A. Global Ecol. Biogeography 29, 668–681. doi: 10.1111/geb.13058
Chapman, B. B., Morrell, L. J., and Krause, J. (2009). Plasticity in male courtship behaviour as a function of light intensity in guppies. Behav. Ecol. Sociobiol. 63, 1757–1763. doi: 10.1007/s00265-009-0796-4
Chatelain, M., Gasparini, J., and Frantz, A. (2016). Trace metals, melanin-based pigmentation and their interaction influence immune parameters in feral pigeons (Columbia livia). Ecotoxicology 25, 521–529. doi: 10.1007/s10646-016-1610-5
Chhaya, V., Lahiri, S., Jagan, A. M., Mohan, R., Pathaw, N. A., and Krishnan, A. (2021). Community bioacoustics: studying acoustic community structure for ecological and conservation insights. Front. Ecol. Evol. 9:706445. doi: 10.3389/fevo.2021.706445
Chung, J. S., and Zmora, N. (2008). Functional studies of crustacean hyperglycemic hormones (CHHs) of the blue crab, Callinectes sapidus - the expression and release of CHH in eyestalk and pericardial organ in response to environmental stress. FEBS J. 275, 693–704. doi: 10.1111/j.1742-4658.2007.06231.x
Chung, J. S., Dircksen, H., and Webster, S. G. (1999). A remarkable, precisely timed release of hyperglycemic hormone from endocrine cells in the gut is associated with ecdysis in the crab Carcinus maenas. Proc. Natl. Acad. Sci. U S A. 23, 13103–13107. doi: 10.1073/pnas.96.23.13103
Ciofini, A., Mercatelli, L., Hariyama, T., and Ugolini, A. (2021). Sky radiance and spectral gradient are orienting cues for the sandhopper Talitrus saltator (Crustacea, Amphipoda). J. Exp. Biol. 224:jeb239574. doi: 10.1242/jeb.239574
Ciuti, S., Northrup, J. M., Muhly, T. B., Simi, S., Musiani, M., Pitt, J. A., et al. (2012). Effects of humans on behaviour of wildlife exceed those of natural predators in a landscape of fear. PLoS One 7:e50611. doi: 10.1371/journal.pone.0050611
Clusella-Trullas, S., and Nielsen, M. (2020). The evolution of insect body coloration under changing climates. Curr. Opin. Insect Sci. 41, 25–32. doi: 10.1016/j.cois.2020.05.007
Coker, D. J., Pratchett, M. S., and Munday, P. L. (2009). Coral bleaching and habitat degradation increase susceptibility to predation for coral-dwelling fishes. Behav. Ecol. 20, 1204–1210. doi: 10.1093/beheco/arp113
Cosentino, B. J., Moore, J., Karraker, N. E., Oullete, M. J., and Gibbs, P. (2017). Evolutionary response to global change: climate and land use interact to shape color polymorphism in a woodland salamander. Ecol. Evol. 7, 5426–5434. doi: 10.1002/ece3.3118
Curtin, P., and Papworth, S. (2020). Coloring and size influence preferences for imaginary animals, and can predict actual donations to species-specific conservation charities. Conservation Lett. 13:e12723.
Dauwe, T., and Eens, M. (2008). Melanin- and carotenoid-dependent signals of great tits (Parus major) relate differently to metal pollution. Naturwissenschaften 95, 969–973. doi: 10.1007/s00114-008-0400-1
Davis, A. K. (2009). Wing color of monarch butterflies (Danaus plexippus) in Eastern North America across Life Stages: migrants are “Redder” than breeding and overwintering stages. Psyche: J. Entomol. 2009:705780. doi: 10.1155/2009/705780
Davis, A. K., Chi, J., Bradley, C., and Altizer, S. (2012). The redder the better: wing color predicts flight performance in monarch butterflies. PLoS One 7:e41323. doi: 10.1371/journal.pone.0041323
Delhey, K., and Peters, A. (2016). Implications for conservation of anthropogenic impacts on visual communication and camouflage. Conservation Biol. 31, 1–36. doi: 10.1111/cobi.12834
Desouhant, E., Gomes, E., Mondy, N., and Amat, I. (2019). Mechanistic, ecological, and evolutionary consequences of artificial light at night for insects: review and prospective. Entomol. Exp. Appl. 167, 37–58. doi: 10.1111/eea.12754
Duquette, C. A., Loss, S. R., and Hovick, T. J. (2021). A meta-analysis of the influence of anthropogenic noise on terrestrial wildlife communication strategies. J. Appl. Ecol. 58, 1112–1121. doi: 10.1111/1365-2664.13880
Elgert, C., Lehtonen, T. K., Kaitala, A., and Candolin, U. (2021). The duration of artificial light defines sexual signalling in the common glow-worm. Behav. Ecol. Sociobiol. 75:154. doi: 10.1007/s00265-021-03093-2
Endler, J. A. (1980). Natural selection on color patterns in Poecilia reticulata. Evolution 34:76. doi: 10.2307/2408316
Endler, J. A. (1993). The color of light in forests and its implications. Ecol. Monographs 43, 1–27. doi: 10.2307/2937121
Fargallo, J., Laaksonen, T., Korpimäki, E., and Wakamatsu, K. (2007). A melanin-based trait reflects environmental growth conditions of nestling male Eurasian kestrels. Evol. Ecol. 21, 157–171. doi: 10.1007/s10682-006-0020-1
Fay, R., Michler, S., Laesser, J., Jeanmonod, J., and Schaub, M. (2020). Large-Scale vole population synchrony in central europe revealed by kestrel breeding performance. Front. Ecol. Evol. 7:512. doi: 10.3389/fevo.2019.00512
Fernie, K. J., Smits, J. E., Bortolotti, G. R., and Bird, D. M. (2001). Reproduction success of American kestrels exposed to dietary polychlorinated biphenyls. Environ. Toxicol. Chem. 20, 776–781. doi: 10.1002/etc.5620200412
Fitzpatrick, B. M., Shook, K., and Izally, R. (2009). Frequency-dependent selection by wild birds promotes polymorphism in model salamanders. BMC Ecol. 9:12. doi: 10.1186/1472-6785-9-12
Forsman, A., Ahnesjö, J., Caesar, S., and Karlsson, M. (2008). A model of ecological and evolutionary consequences of color polymorphism. Ecology 89, 34–40. doi: 10.1890/07-0572.1
Forrest, J., and Miller-Rushing, A. J. (2010). Toward a synthetic understanding of the role of phenology in ecology and evolution. Philos. Trans. R. Soc. B 365, 3101–3112. doi: 10.1098/rstb.2010.0145
Frynta, D., Lišková, S., Bültmann, S., and Burda, H. (2010). Being attractive brings advantages: the case of parrot species in captivity. PLoS One 5:e12568. doi: 10.1371/journal.pone.0012568
García-Heras, M. S., Arroyo, B., Simmons, R. E., Camarero, P. R., Mateo, R., García, J. T., et al. (2017). Pollutants and diet influence carotenoid levels and integument coloration in nestlings of an endangered raptor. Sci. Total Environ. 60, 299–307. doi: 10.1016/J.SCITOTENV.2017.06.048
Gaston, K. J., Bennie, J., Davies, T. W., and Hopkins, J. (2013). The ecological impacts of nighttime light pollution: a mechanistic appraisal. Biol. Rev. 88, 912–927. doi: 10.1111/brv.12036
Giraudeau, M., Chavez, A., Toomey, M. B., and McGraw, K. J. (2015a). Effects of carotenoid supplementation and oxidative challenges on physiological parameters and carotenoid-based coloration in an urbanization context. Behav. Ecol. Sociobiol. 69, 957–970. doi: 10.1007/s00265-015-1908-y
Giraudeau, M., Mateos-Gonzalez, F., Cotín, J., Pagani-Nuñez, E., Torné-Noguera, A., and Senar, J. C. (2015b). Metal exposure influences the melanin and carotenoid-based colorations in great tits. Sci. Total Environ. 532, 512–516. doi: 10.1016/j.scitotenv.2015.06.021
Gould, K. S. (2004). Nature’s Swiss army knife: the diverse protective roles of anthocyanins in leaves. J. Biomed. Biotechnol. 2004, 314–320. doi: 10.1155/S1110724304406147
Grant, A. H., Ransom, T. S., and Liebgold, E. B. (2018). Differential survival and the effects of predation on a color polymorphic species, the red-backed salamander (Plethodon cinereus). J. Herpetol. 52, 127–135. doi: 10.1670/16-185
Green, D. A. (2021). Monarch butterfly migration as an integrative model of complex trait evolution. Am. Nat. 198, 142–157. doi: 10.1086/714526
Greenspoon, P. B., and Spencer, H. G. (2021). Avoiding extinction under nonlinear environmental change: models of evolutionary rescue with plasticity. Biol. Lett. 17:2021045920210459. doi: 10.1098/rsbl.2021.0459
Griffith, S. C., Parker, T. H., and Olson, V. A. (2006). Melanin- versus carotenoid-based sexual signals: is the difference really so black and red? Animal Behav. 71, 749–763. doi: 10.1016/j.anbehav.2005.07.016
Groom, S., Sathyendranath, S., Ban, Y., Bernard, S., Brewin, R., Brotas, V., et al. (2019). Satellite ocean colour: current status and future perspective. Front. Mar. Sci. 6:485. doi: 10.3389/fmars.2019.00485
Grunst, M. L., Grunst, A. S., Pinxten, R., Bervoets, L., and Eens, M. (2020). Carotenoid - but not melanin-based plumage coloration is negatively related to metal exposure and proximity to the road in an urban songbird. Environ. Pollut. 256:113473. doi: 10.1016/j.envpol.2019.113473
Hall, C. M., Scott, D., and Gössling, S. (2011). Forests, climate change and tourism. J. Heritage Tour. 6, 353–363. doi: 10.1080/1743873X.2011.620252
Hamilton, W. D., and Zuk, M. (1982). Heritable true fitness and bright birds: a role for parasites? Science 218, 384–387. doi: 10.1126/science.7123238
Hanley, D., Miller, N. G., Flockhart, D. T. T., and Norris, R. D. (2013). Forewing pigmentation predicts migration distance in wild-caught migratory monarch butterflies. Behav. Ecol. 24, 1108–1113. doi: 10.1093/beheco/art037
Heinrichs, J. A., Bender, D. J., and Schumaker, N. H. (2016). Habitat degradation and loss as key drivers of regional population extinction. Ecol. Modell. 335, 64–73. doi: 10.1016/j.ecolmodel.2016.05.009
Hemingson, C. R., Mihalitsis, M., and Bellwood, D. R. (2022). Are fish communities on coral reefs becoming less colourful? Glob. Change Biol. 1–12. doi: 10.1111/gcb.16095
Hill, G. E. (1995). Ornamental traits as indicators of environmental health: condition-dependent display traits hold promise as potent biomonitors. BioScience 45, 25–31. doi: 10.2307/1312532
Hill, G. E. (2000). Energetic constraints on expression of carotenoid-based plumage coloration. J. Avian Biol. 31, 559–566. doi: 10.1034/j.1600-048X.2000.310415.x
Hill, G. E. (2006). “Environmental regulation of ornamental coloration,” in Bird Coloration: Function and Evolution, eds G. E. Hill and K. J. McGraw (Cambridge, MA: Harvard University Press).
Hill, G. E., and Montgomerie, R. (1994). Plumage colour signals nutritional condition in the house finch. Proceedings of the Royal Society B: Biological Sciences, B. 258, 47–52. doi: 10.1098/rspb.1994.0140
Hocking, B. (1964). Fire melanism in some african grasshoppers. Evolution 18, 332–335. doi: 10.2307/2406407
Hoegh-Guldberg, O. (1999). Climate change, coral bleaching and the future of the world’s coral reefs. Mar. Freshwater Res. 50, 839–866. doi: 10.1071/MF99078
Hoffman, R. J. (1978). Environmental uncertainty and evolution of physiological adaptation in colias butterflies. Am. Natural. 112, 999–1015. doi: 10.1086/283343
Hutton, P., and McGraw, K. J. (2016). Urban impacts on oxidative balance and animal signals. Front. Ecol. Evol. 4:54. doi: 10.3389/fevo.2016.00054
Jawor, J. M., and Breitwisch, R. (2003). Melanin ornaments, honesty, and sexual selection. Auk 120, 249–265. doi: 10.1093/auk/120.2.249
Karlsson, M., Caesar, S., Ahnesjö, J., and Forsman, A. (2008). Dynamics of colour polymorphism in a changing environment: fire melanism and then what? Oecologia 154, 715–724. doi: 10.1007/s00442-007-0876-y
Karpestam, E., Merilaita, S., and Forsman, A. (2012). Reduced predation risk for melanistic pygmy grasshoppers in post-fire environments. Ecol. Evol. 2, 2204–2212. doi: 10.1002/ece3.338
Karpestam, E., Merilaita, S., and Forsman, A. (2013). Detection experiments with humans implicate visual predation as a driver of colour polymorphism dynamics in pygmy grasshoppers. BMC Ecol. 13:17. doi: 10.1186/1472-6785-13-17
Kingsolver, J. G. (1983a). Ecological significance of flight activity in Colias butterflies: implications for reproductive strategy and population structure. Ecology 64, 546–551. doi: 10.2307/1939974
Kingsolver, J. G. (1983b). Thermoregulation and flight in Colias butterflies: elevational patterns and mechanistic limitations. Ecology 64, 534–545. doi: 10.2307/1939973
Kingsolver, J. G. (1995). Fitness consequences of seasonal polyphenism in Western white butterflies. Evolution 49, 942–954. doi: 10.1111/j.1558-5646.1995.tb02329.x
Kingsolver, J. G., and Buckley, L. B. (2015). Climate variability slows evolutionary responses of Colias butterflies to recent climate change. Proc. R. Soc. B: Biol. Sci. 282:1802. doi: 10.1098/RSPB.2014.2470
Kingsolver, J. G., and Wiernasz, D. C. (1991). Seasonal polyphenism in wing-melanin pattern and thermoregulatory adaptation in pieris butterflies. Am. Nat. 137, 816–830. doi: 10.1086/285195
Koski, M. H., MacQueen, D., and Ashman, T.-L. (2020). Floral pigmentation has responded rapidly to global change in ozone and temperature. Curr. Biol. 30, 4425–4431. doi: 10.1016/j.cub.2020.08.077
Kristiansen, K. O., Bustnes, J. O., Folstad, I., and Helberg, M. (2006). Carotenoid coloration in great black-backed gull Larus marinus reflects individual quality. J. Avian Biol. 37, 6–12. doi: 10.1111/j.2006.0908-8857.03667.x
Lacey, E. P., Lovin, M. E., Richter, S. J., and Herington, D. A. (2010). Floral reflectance, color, and thermoregulation: what really explains geographic variation in thermal acclimation ability of ectotherms? Am. Natural. 175, 335–349. doi: 10.1086/650442
Laurance, W. F. (2004). Forest-climate interactions in fragmented tropical landscapes. Philos. Trans. R. Soc. Lond. B 359, 345–352. doi: 10.1098/rstb.2003.1430
Lifshitz, N., and St Clair, C. C. (2016). Coloured ornamental traits could be effective and non-invasive indicators of pollution exposure for wildlife. Conservation Physiol. 4:cow028. doi: 10.1093/conphys/cow028
Lifshitz, N., and St. Clair, C. C. (2019). Iridescent coloration of Tree Swallows relates to environmental metal pollution. Avian Conservation Ecol. 14:7. doi: 10.5751/ACE-01411-140207
Lillywhite, H. B., Friedman, G., and Ford, N. (1977). Color matching and perch selection by lizards in recently burned chaparral. Copeia 1977, 115–121. doi: 10.2307/1443512
Lim, M. L. M., Sodhi, N. S., and Endler, J. A. (2008). Conservation with sense. Science 319:581. doi: 10.1126/science.319.5861.281b
Lišková, S., Landová, E., and Frynta, D. (2015). Human preferences for colorful birds: vivid colors or pattern? Evol. Psychol. 13, 339–359. doi: 10.1177/147470491501300203
López-Idiáquez, D., Teplitsky, C., Grégoire, A., Fargevieille, A., Del Rey, M., de Franceschi, C., et al. (2022). Long-term decrease in coloration: a consequence of climate change? Am. Natural [Preprint]. doi: 10.1086/719655
McGraw, K. J. (2006a). “Mechanisms of carotenoid-based coloration,” in Bird Coloration, Mechanisms and Measurements, eds G. E. Hill and K. J. McGraw (Cambridge, MA: Harvard University Press).
McGraw, K. J. (2006b). “Mechanisms of melanin-based coloration,” in Bird Coloration, Mechanisms and Measurements, eds G. E. Hill and K. J. McGraw (Cambridge, MA: Harvard University Press).
McGraw, K. J., and Parker, R. S. (2006). A novel lipoprotein-mediated mechanism controlling sexual attractiveness in a colorful songbird. Physiol. Behav. 87, 103–108. doi: 10.1016/J.PHYSBEH.2005.09.001
McGraw, K. J., Hill, G. E., and Parker, R. S. (2005). The physiological costs of being colourful: nutritional control of carotenoid utilization in the American Goldfinch, Carduelis tristis. Animal Behav. 69, 653–660. doi: 10.1016/j.anbehav.2004.05.018
McMahon, S. (2020). America’s Fall Destinations will have to Balance Coronavirus Safety with a Tourism Comeback. Washington, DC: The Washington Post
Meadows, M. G., Roudybush, T. E., and McGraw, K. J. (2012). Dietary protein level affects iridescent coloration in Anna’s hummingbirds, Calypte anna. J. Exp. Biol. 215, 2742–2750. doi: 10.1242/JEB.069351
Mills, L. S., Zimova, M., Oyler, J., Running, S., Abatzoglou, J. T., and Lukacs, P. M. (2013). Camouflage mismatch in seasonal coat color due to decreased snow duration. Proc. Natl. Acad. Sci. U S A. 110, 7360–7365. doi: 10.1073/pnas.1222724110
Møller, A. P. (1991). Sexual ornament size and the cost of fluctuating asymmetry. Proc. R. Soc. B: Biol. Sci. 243, 59–62. doi: 10.1098/rspb.1991.0010
Monasterio, C., Salvador, A., Iraeta, P., and Díaz, J. A. (2009). The effects of thermal biology and refuge availability on the restricted distribution of an alpine lizard. J. Biogeogr. 36, 1673–1684. doi: 10.1111/j.1365-2699.2009.02113.x
Murcia, C. (1995). Edge effects in fragmented forests: implications for conservation. Trends Ecol. Evol. 10, 58–62. doi: 10.1016/S0169-5347(00)88977-6
Naguib, M., and Nemitz, A. (2007). Living with the past: nutritional stress in juvenile males has immediate effects on their plumage ornaments and on adult attractiveness in zebra finches. PLoS One 2:e901. doi: 10.1371/journal.pone.0000901
Owens, A. C. S., and Lewis, S. M. (2018). The impact of artificial light at night on nocturnal insects: a review and synthesis. Ecol. Evol. 8, 11337–11358. doi: 10.1002/ece3.4557
Pacyna, A. D., Ruman, M., Mazerski, J., and Polkowska, Ż (2018). Biological responses to environmental contamination. how can metal pollution impact signal honesty in avian species? Ecol. Evol. 8, 7733–7739. doi: 10.1002/ECE3.4192
Patten, M. A., and Smith-Patten, B. D. (2012). Testing the microclimate hypothesis: light environment and population trends of neotropical birds. Biol. Conservation 155, 85–93. doi: 10.1016/j.biocon.2012.06.004
Pedersen, S., Odden, M., and Pedersen, H. C. (2017). Climate change induced molting mismatch? mountain hare abundance reduced by duration of snow cover and predator abundance. Ecosphere 8:e01722. doi: 10.1002/ecs2.1722
Peneaux, C., Hansbro, P. M., and Griffin, A. S. (2021). The potential utility of carotenoid-based coloration as a biomonitor of environmental change. IBIS 163, 20–37. doi: 10.1111/ibi.12866
Pérez, C., Lores, M., and Velando, A. (2010). Oil pollution increases plasma antioxidants but reduces coloration in a seabird. Oecologia 163, 875–884. doi: 10.1007/s00442-010-1677-2
Peters, A., Delhey, K., Johnsen, A., and Kempenaers, B. (2007). The condition-dependent development of carotenoid based and structural plumage in nestling blue-tits: males and females differ. Am. Nat. 169, S122–S136. doi: 10.1086/510139
Post, E., Forchhammer, M. C., Ims, R. A., Jeppesen, E., Klein, D. R., Madsen, J., et al. (2009). Ecological dynamics across the arctic associated with recent climate change. Science 325, 1355–1358. doi: 10.1126/science.1173113
Prokop, P., and Fančovičová, J. (2013). Does colour matter? the influence of animal warning coloration on human emotions and willingness to protect them. Animal Conservation 16, 458–466. doi: 10.1111/acv.12014
Prum, R. O. (2006). “Anatomy, physics, and evolution of structural colors,” in Bird Coloration, Mechanisms and Measurements, eds G. E. Hill and K. J. McGraw (Cambridge, MA: Harvard University Press).
Prum, R. O., and Torres, R. (2003). Structural colouration of avian skin: convergent evolution of coherently scattering dermal collagen arrays. J. Exp. Biol. 206, 2409–2429. doi: 10.1242/JEB.00431
Rocha, E. A., Luz, F. A., Rossato, D. O., and Millan, C. (2015). Does fire disturbance change insect composition and the frequency of darker phenotypes in a grassland community? Braz. J. Biosci. 13, 219–223.
Rosenthal, G. G. (2007). Spatiotemporal dimensions of visual signals in animal communication. Annu. Rev. Ecol. Evol. Syst. 38, 155–178. doi: 10.1146/annurev.ecolsys.38.091206.095745
Rosenthal, G. G., and Stuart-Fox, D. (2012). “Environmental disturbance and animal communication,” in Behavioural Responses to a Changing World: Mechanisms and Consequences, eds U. Candolin and B. B. M. Wong (Oxford: Oxford University Press).
Rowell, C. H. F. (1971). The variable coloration of the Acridoid grasshoppers. Adv. Insect Physiol. 8, 145–198. doi: 10.1016/s0065-2806(08)60197-6
Seehausen, O., Terai, Y., Magalhaes, I. S., Carleton, K. L., Mrosso, H. D. J., Miyagi, R., et al. (2008). Speciation through sensory drive in cichlid fish. Nature 455, 620–626. doi: 10.1038/nature07285
Seehausen, O., van Alphen, J. J. M., and Witte, F. (1997). Cichlid fish diversity threatened by eutrophication that curbs sexual selection. Science 277, 1808–1811. doi: 10.1126/science.277.5333.1808
Selz, O. M., Pierotti, M. E. R., Maan, M. E., Schmid, C., and Seehausen, O. (2014). Female preference for male color is necessary and sufficient for assortative mating in 2 cichlid sister species. Behav. Ecol. 25, 612–626. doi: 10.1093/beheco/aru024
Senar, J. C., Figuerola, J., and Doménech, J. (2003). Plumage coloration and nutritional condition in the great tit Parus major: the roles of carotenoids and melanins differ. Naturwissenschaften 90, 234–237. doi: 10.1007/s00114-003-0414-7
Shawkey, M. D., Hill, G. E., McGraw, K. J., Hood, W. R., and Huggins, K. l. (2006). An experimental test of the contributions and condition dependence of microstructure and carotenoids in yellow plumage coloration. Proc. R. Soc. B: Biol. Sci. 273, 2985–2991. doi: 10.1098/rspb.2006.3675
Shrestha, M., Garcia, J. E., Bukovac, Z., Dorin, A., and Dyer, A. G. (2018). Pollination in a new climate: assessing the potential influence of flower temperature variation on insect pollinator behaviour. PLoS One 13:e0200549. doi: 10.1371/journal.pone.0200549
Sokolova, I. M., Frederich, M., Bagwe, R., Lannig, G., and Sukhotin, A. A. (2012). Energy homeostasis as an integrative tool for assessing limits of environmental stress tolerance in aquatic invertebrates. Mar. Environ. Res. 79, 1–15. doi: 10.1016/j.marenvres.2012.04.003
Spaniol, R. L., Duarte, L. D. S., Mendonca, M. D. S., and Iserhard, C. A. (2019). Combining functional traits and phylogeny to disentangling Amazonian butterfly assemblages on anthropogenic gradients. Ecosphere 10:e02837. doi: 10.1002/ecs2.2837
Spaniol, R. L., Mendonça, M. D. S., Hartz, S. M., Iserhad, C. A., and Stevens, M. (2020). Discolouring the Amazon Rainforest: how deforestation is affecting butterfly coloration. Biodiversity Conservation 29, 2821–2838. doi: 10.1007/s10531-020-01999-3
Spitschan, M., Aguirre, G., Brainard, D., and Sweeney, A. M. (2016). Variation of outdoor illumination as a function of solar elevation and light pollution. Sci. Rep. 6:26756. doi: 10.1038/srep26756
Steel, Z. L., Safford, H. D., and Viers, J. H. (2015). The fire frequency-severity relationship and the legacy of fire suppression in California forests. Ecosphere 6:8. doi: 10.1890/ES14-00224.1
Stokes, D. L. (2007). Things we like: human preferences among similar organisms and implications for conservation. Hum. Ecol. 35, 361–369. doi: 10.1007/s10745-006-9056-7
Stuart-Fox, D., Rankin, K. J., Lutz, A., Elliott, A., Hugall, A. F., McLean, C. A., et al. (2021). Environmental gradients predict the ratio of environmentally acquired carotenoids to self-synthesised pteridine pigments. Ecol. Lett. 24, 2207–2218. doi: 10.1111/ele.13850
Sullivan, A., Bird, D., and Perry, G. (2017). Human behaviour as a long-term ecological driver of non-human evolution. Nat. Ecol. Evol. 1:0065. doi: 10.1038/s41559-016-0065
Sullivan, C. E., and Koski, M. H. (2021). The effects of climate change on floral anthocyanin polymorphisms. Proc. R. Soc. B: Biol. Sci. 288:1946. doi: 10.1098/rspb.2020.2693
Tallamy, D. W., and Shriver, W. G. (2021). Are declines in insects and insectivorous birds related? Ornithol. Appl. 123:1. doi: 10.1093/ornithapp/duaa059
Thömmes, K., and Hayn-Leichsenring, G. (2021). What instagram can teach US about bird photography: the most photogenic bird and color preferences. I-Perception 12:20416695211003585. doi: 10.1177/20416695211003585
Tyczkowski, J. K., and Hamilton, P. B. (1986). Evidence for differential absorption of zeacarotene, cryptoxanthin, and lutein in young broiler chickens. Poultry Sci. 65, 1137–1140. doi: 10.3382/ps.0651137
van der Kooi, C. J., Kevan, P. G., and Koski, M. H. (2019). The thermal ecology of flowers. Ann. Bot. 124, 343–353. doi: 10.1093/aob/mcz073
van der Sluijs, I., Gray, S. M., Amorim, M. C. P., Barber, I., Candolin, U., Hendry, A. P., et al. (2011). Communication in troubled waters: responses of fish communication systems to changing environments. Evol. Ecol. 25, 623–640. doi: 10.1007/s10682-010-9450-x
Venesky, M. D., and Anthony, C. D. (2007). Antipredator adaptations and predator avoidance by two colour morphs of the Eastern red-backed salamander, Plethodon cinereus. Herpetologica 63, 450–458. doi: 10.1007/s10531-020-01999-3
Wale, M. A., Simpson, S. D., and Radford, A. N. (2013). Size-dependent physiological responses of shore crabs to single and repeated playback of ship noise. Biol. Lett. 9:20121194. doi: 10.1098/rsbl.2012.1194
Wang, K., Dickinson, R. E., and Liang, S. (2009). Clear sky visibility has decreased over land globally from 1973 to 2007. Science 323, 1468–1470. doi: 10.1126/science.1167549
Watt, W. B. (1968). Adaptive significance of pigment polymorphisms in Colias butterflies. I. variation of melanin pigment in relation to thermoregulation. Evolution 22, 437–458. doi: 10.1111/j.1558-5646.1968.tb03985.x
Watt, W. B. (1969). Adaptive significance of pigment polymorphisms in Colias butterflies. II. thermoregulation and photoperiodically controlled melanin variation in Colias eurytheme. Proc. Natl. Acad. Sci. U S A. 63, 767–774. doi: 10.1073/pnas.63.3.767
Weaver, R. J., Koch, R. E., and Hill, G. E. (2017). What maintains signal honesty in animal colour displays used in mate choice? Philos. Trans. R. Soc. B: Biol. Sci. 372:2016034320160343. doi: 10.1098/rstb.2016.0343
Weaver, R. J., Santos, E. S. A., Tucker, A. M., Wilson, A. E., and Hill, G. E. (2018). Carotenoid metabolism strengthens the link between feather coloration and individual quality. Nat. Commun. 9:73. doi: 10.1038/s41467-017-02649-z
Yang, C., Wang, J., Lyu, N., and Lloyd, H. (2021). Comparison of digital photography and spectrometry for evaluating colour perception in humans and other trichromatic species. Behav. Ecol. Sociobiol. 75:151. doi: 10.1007/s00265-021-03071-8
Zeuss, D., Brandl, R., Brändle, M., Rahbek, C., and Brunzel, S. (2014). Global warming favours light-coloured insects in Europe. Nat. Commun. 5:3874. doi: 10.1038/ncomms4874
Zimova, M., Hackländer, K., Good, J. M., Melo-Ferreira, J., Alves, P. C., and Mills, L. S. (2018). Function and underlying mechanisms of seasonal colour moulting in mammals and birds: what keeps them changing in a warming world? Biol. Rev. 93, 1478–1498. doi: 10.1111/brv.12405
Zimova, M., Mills, L. S., and Nowak, J. J. (2016). High fitness costs of climate change induced camouflage mismatch. Ecol. Lett. 19, 299–307. doi: 10.1111/ele.12568
Zimova, M., Mills, L. S., Lukacs, P. M., and Mitchell, M. S. (2014). Snowshoe hares display limited phenotypic plasticity to mismatch in seasonal camouflage. Proc. R. Soc. B: Biol. Sci. 281:20140029 doi: 10.1098/RSPB.2014.002
Zimova, M., Giery, S. T., Newey, S., Nowak, J. J., Spencer, M., and Mills, L. S. (2020a). Lack of phenological shift leads to increased camouflage mismatch in mountain hares. Proc. R. Soc. B: Biol. Sci. 287:20201786.
Zimova, M., Sirén, A. P. K., Nowak, J. J., Bryan, A. M., Ivan, J. S., Morelli, T. L., et al. (2020b). Local climate determines vulnerability to camouflage mismatch in snowshoe hares. Global Ecol. Biogeography 29, 503–515. doi: 10.1111/geb.13049
Zub, K., Sönnichsen, L., and Szafrańska, P. A. (2008). Habitat requirements of weasels Mustela nivalis constrain their impact on prey populations in complex ecosystems of the temperate zone. Oecologia 157, 571–582. doi: 10.1007/s00442-008-1109-8
Keywords: coloration, visual environment, climate change, landscape change, conservation
Citation: Koneru M and Caro T (2022) Animal Coloration in the Anthropocene. Front. Ecol. Evol. 10:857317. doi: 10.3389/fevo.2022.857317
Received: 18 January 2022; Accepted: 22 March 2022;
Published: 22 April 2022.
Edited by:
Daniel Marques Almeida Pessoa, Federal University of Rio Grande do Norte, BrazilReviewed by:
Pedro De Moraes, Federal University of Rio Grande do Norte, BrazilNathaniel Jay Dominy, Dartmouth College, United States
Copyright © 2022 Koneru and Caro. This is an open-access article distributed under the terms of the Creative Commons Attribution License (CC BY). The use, distribution or reproduction in other forums is permitted, provided the original author(s) and the copyright owner(s) are credited and that the original publication in this journal is cited, in accordance with accepted academic practice. No use, distribution or reproduction is permitted which does not comply with these terms.
*Correspondence: Manisha Koneru, io21732@bristol.ac.uk; Tim Caro, tmcaro@ucdavis.edu