- Departamento de Química y Bioquímica, Facultad de Farmacia, Universidad San Pablo-CEU, CEU Universities, Urbanización Montepríncipe, Boadilla del Monte, Madrid, Spain
Pleiotrophin (PTN) is a cytokine which has been for long studied at the level of the central nervous system, however few studies focus on its role in the peripheral organs. The main aim of this review is to summarize the state of the art of what is known up to date about pleiotrophin and its implications in the main metabolic organs. In summary, pleiotrophin promotes the proliferation of preadipocytes, pancreatic β cells, as well as cells during the mammary gland development. Moreover, this cytokine is important for the structural integrity of the liver and the neuromuscular junction in the skeletal muscle. From a metabolic point of view, pleiotrophin plays a key role in the maintenance of glucose and lipid as well as whole-body insulin homeostasis and favors oxidative metabolism in the skeletal muscle. All in all, this review proposes pleiotrophin as a druggable target to prevent from the development of insulin-resistance-related pathologies.
Introduction
Pleiotrophin (PTN) is an 18-kDa neurotrophic heparin-binding factor that was simultaneously described by several groups around 1990 (1–5). Ptn gene encodes a basic protein of 168 amino acids, that after post-transcriptional modifications, renders the active protein composed of 136 amino acids and a 32 amino acid signal peptide (3, 5, 6). In addition, PTN maintains a highly conserved sequence among species (sequence identity >90%) and shares more than 50% sequence identity with midkine, the other member of the family (7).
Ptn expression is highly upregulated during embryonic development and during early cell differentiation (2, 4, 5). In the adulthood, its expression is decreased in most of the tissues except for the bone and the nervous system, where the highest expression levels are maintained (1–5). In humans, circulating PTN levels are significantly associated with advancing chronological age, what confirms a retention of pleiotrophin expression in adult tissues (8). In particular, a residual expression of pleiotrophin has been found in the adult liver, brain, adipose tissue, testis, and pancreas (9–11).
Additionally, upregulated pleiotrophin expression has been associated with biological events that involve cellular proliferation and differentiation such as tissue regeneration (12), bone repair (13), inflammatory processes (14), hypoxia (15), tumor growth (16) and angiogenesis (17). In fact, Ptn gene is a proto-oncogene and high levels of this cytokine are associated to perineural invasion in pancreatic cancer (18) and metastasis in prostate cancer (19). Accordingly, determination of PTN levels has been proposed as a potential tool for the diagnosis and prognosis of breast cancer (20, 21). Moreover, the expression of this cytokine has shown to be enhanced in the presence of several growth factors and cytokines, like androgens (22), TNFα and epidermal growth factor (EGF) (23), platelet-derived growth factor (PDGF) and basic fibroblast growth factor (FGF) (24), and to be downregulated in a dose-dependent manner by 1α,25-Dihydroxyvitamin D(3) (25).
Additionally, several miRNAs are also regulators of PTN expression. miR-143 represses Ptn expression and enhances preadipocyte differentiation (26), miR-182 downregulates PTN levels and participates in the development of endometrial receptivity (27). PTN levels are negatively correlated with the levels of miR-137 a miRNA that is decreased in hypertrophic scars (28) and of those of miR‐384 a microRNA with potential tumor suppressor activity (29). Moreover, some miRNAs (miR-499 and miR-1709) regulate Ptn expression by affecting the DNA methylation status of the Ptn gene, and hence, favor the initiation and progression of some tumoral processes (30).
From a pharmacodynamic point of view, PTN is a ligand for several receptors that are differentially expressed in the different tissues. So far, PTN has been described to interact with nucleolin (19), neuropilin-1 (31), syndecans (32, 33), integrin αvβ3 (34), integrin αMβ2 (Mac-1) (35), anaplastic lymphoma kinase (ALK) (36), and receptor protein phosphatase β/ζ (RPTP β/ζ) (37, 38). PTN interacts with the glycosaminoglycans of syndecans and induces its oligomerization affecting its interactions with other membrane receptors, what has shown to activate focal adhesion kinase and ERK1/2 (39). Similarly, PTN can also bind to the extracellular domain of RPTP β/ζ, inducing its dimerization, what inhibits the intracellular tyrosine phosphatase activity and favors the increment in the phosphorylated forms of the RPTP β/ζ substrates including β-catenin, ALK, c-Src, PKC, integrin αVβ3, p190RhoGAP and ERK1/2 (40). Although, ALK has been described as a receptor for pleiotrophin (36) it is not clear if pleiotrophin directly modulates ALK signaling or if it is a target of the PTN/RPTP β/ζ signaling pathway (41). PTN treatment induces the phosphorylation of the cytoplasmic domains of β3 of αvβ3 integrin (34). Furthermore αvβ3 forms a multi receptor complex with RPTP β/ζ and nucleolin (42). Pleiotrophin interacts with nucleolin that participates in PTN nuclear translocation what have been suggested that may modify gene expression (43). Altogether, the binding of PTN to these receptors triggers the activation of intracellular signaling cascades involved in cell migration and adhesion (19, 44, 45), cancer and metastasis (19, 44, 45), inflammation (35, 46, 47) and neurite outgrowth (2).
Pleiotrophin in metabolic organs: implications for metabolism and disease
Metabolic diseases are characterized by the disruption of normal metabolic processes which alter the capacity to process carbohydrates, proteins or lipids. Metabolic disorders include a wide range of diseases that can be either inherited or acquired during lifetime. Metabolic syndrome is characterized by the presence of insulin resistance, central obesity, hypercholesterolemia and hypertension and poses a higher risk of developing diabetes and cardiovascular disease (48).
The role of the central nervous system (CNS) in controlling peripheral metabolism has been extensively described in the literature (49). The continuous crosstalk between the brain and peripheral organs through different molecules is key in the regulation of whole-body metabolic homeostasis (50). Recently, the link between neurodegenerative diseases and metabolic disorders has gained more relevance. It has been proposed that the different molecules involved in the crosstalk between the brain and the adipose tissue, might contribute to the development of several metabolic and/or neurodegenerative disorders (49, 51). Thus, diabetic or obese patients are prone to develop neurodegenerative disorders such as Parkinson´s or Alzheimer´s Disease (52, 53). However, the mechanisms underlying these connections remain still unclear.
PTN is a potent modulator of neuroinflammation in the CNS (14) and participates in the repair, survival and differentiation of neurons (54). PTN modulates the tyrosine phosphorylation of substrates that are involved in neuroinflammation through RPTP β/ζ, which is mainly expressed in the adult CNS in both neurons and microglia (55). As PTN signaling pathway regulates the inflammatory condition related to metabolic disorders, pleiotrophin has been postulated as a promising candidate for CNS and peripheral metabolism crosstalk (55). Moreover, recent reports have evidenced that PTN is implicated in the regulation of peripheral metainflammation, metabolic homeostasis, thermogenesis, as well as insulin sensitivity in the peripheral tissues (11, 56, 57) Furthermore, Ptn deletion protects against neuroinflammation, mitochondrial dysfunction, and aberrant protein aggregation in a high fat diet (HFD) induced obesity model (47).
In this review we summarize the main roles of pleiotrophin in the development and metabolism of key metabolic organs (Figure 1), and how these effects may contribute to the development of metabolic disorders and more precisely, metabolic syndrome.
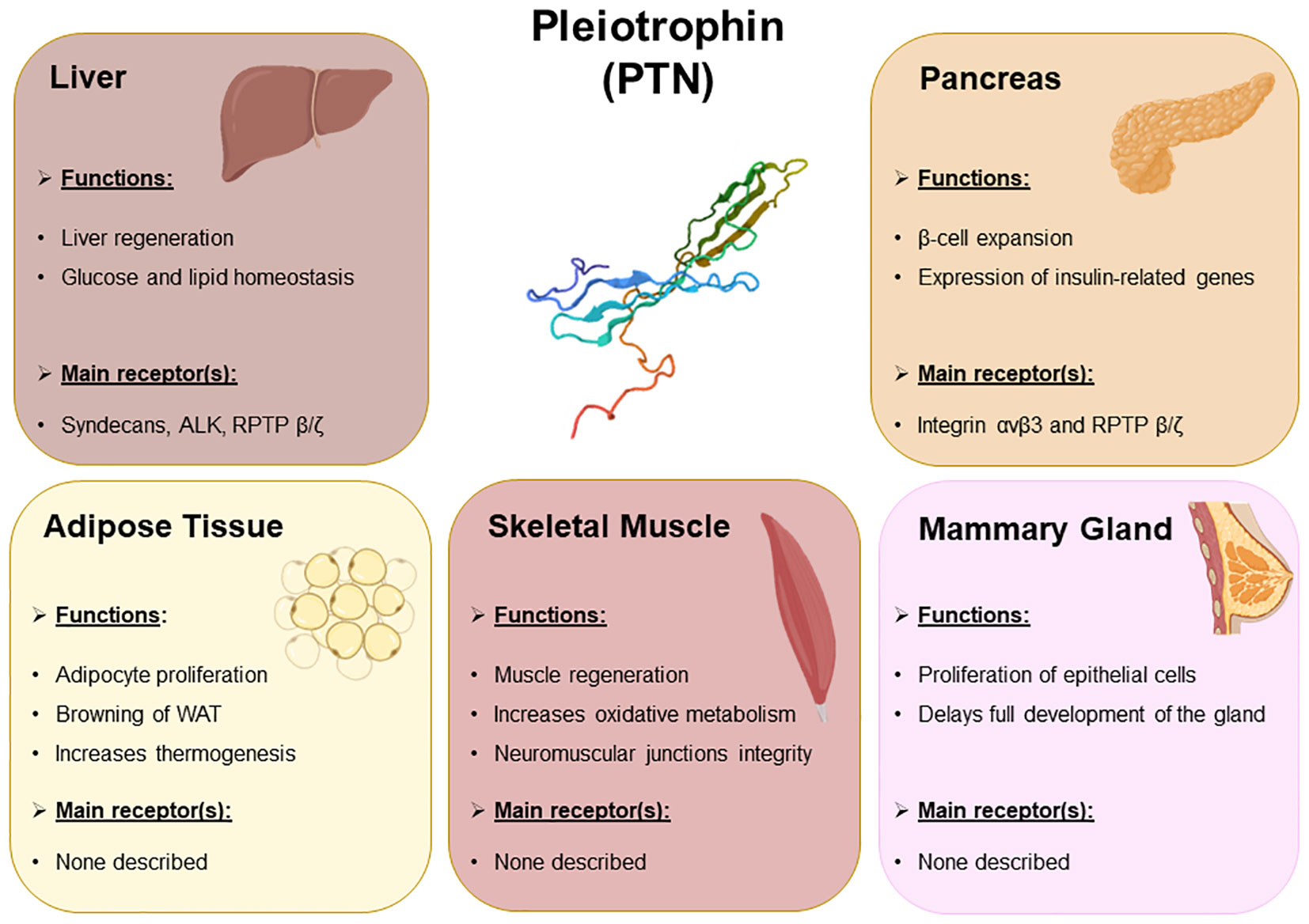
Figure 1 Summary of the main biological events regulated by PTN occurring in the metabolic organs. The main metabolic events regulated by PTN in liver, pancreas, adipose tissue, skeletal muscle, and mammary gland. The receptors of PTN expressed in these organs that presumably mediate PTN signaling pathways have also been included.
Pleiotrophin in liver regeneration and metabolic regulation
The liver is the main metabolic organ of the organism and regulates energy homeostasis by modulating glucose and lipid metabolism (58, 59). Liver integrity is crucial for liver functionality. Accordingly, liver has a unique capacity to regenerate after tissue damage (60), and several cytokines and growth factors have been shown to be essential for liver regeneration (61–65). PTN exerts an antiapoptotic activity through the inhibition of caspase-3 (66) and is a potent mitogen for hepatocytes (67). In this line of evidence, PTN potentiates regeneration after partial hepatectomy (68), regulates the proliferation of bile ducts after liver injury and is overall implicated in liver regeneration and development (68, 69). In fact, after liver damage, under hypoxic conditions or in the presence of high PDGF levels (15), Ptn expression and secretion is induced in the hepatic stellate cells (HSCs) and once secreted, PTN exerts its mitogenic effects on hepatocytes (68, 70). Regarding the metabolic effects of pleiotrophin in hepatocytes, PTN binding to the N-syndecan activates the PI3K/Akt/mTORC1 pathway what increases the levels of sterol regulatory element‐binding protein 1c (SREBP‐1c) and the expression of lipogenic genes, inducing fatty acid synthesis (29).
In the liver, Ptn deletion in mice lowered lipid accumulation and decreased the mRNA levels of the enzymes involved in both fatty acid and triacylglyceride synthesis. Although the exposure to a HFD promoted hyperinsulinemia and insulin resistance in wild type mice, Ptn deletion blocks the HFD-induced hyperinsulinemia and the increment in the HOMA-IR index, and also protects against HFD-induced hepatic steatosis (57).
On the other hand, PTN has also shown to be necessary for hepatic homeostasis during pregnancy (56). Deletion of Ptn is associated with a defective hepatic peroxisome proliferator-activated receptor alpha (PPARα) and NUR77 activation that impairs lipid and carbohydrate metabolism. As a consequence, in the absence of pleiotrophin, a marked reduction in fatty acid, triacylglyceride and cholesterol synthesis is observed in the liver. Additionally, hepatic secretion of triacylglycerides and hepatic lipid accumulation are reduced in the pregnant Ptn knock out mice (56).
Role of pleiotrophin in skeletal muscle development and function
Pleiotrophin is expressed in all types of muscle cells (smooth muscle, cardiac muscle, and skeletal muscle) and it is distributed in the basement membranes and epithelial cell surfaces (71–73). First of all, expression of pleiotrophin has been reported in intestinal smooth muscle cells (24). Secondly, Ptn is upregulated during in vitro myogenesis as well as in rat soleus muscle during regeneration after crushing. The expression levels of PTN progressively increased during the differentiation process with a peak during the fusion of myoblasts into myotubes (74). Moreover, PTN staining was localized in differentiating skeletal muscles and in the outer mesenchymal layer of the stomach and the intestine, which gives rise to the smooth muscle layer (75). PTN has neurotrophic activity and induces clustering of acetylcholine receptors (AchR) at neuromuscular junctions (NMJ). During rat embryogenesis, at embryonic day E16, PTN is located close to AchR clusters on the muscle cell surface (76). Moreover, Ptn is expressed in macrophages and non-myelinating glial cells (Schwann cells) which are important cell types implicated in the NMJ (77). PTN also accumulates in extracellular structures that line growing axonal processes and disappears after neurite extension has been completed (78).
During the regeneration of adult muscle, in a general inflammatory milieu, platelets and myogenic precursor cells start secreting various growth factors, to counteract the deleterious effects of a muscle lesion (71–73). PDGF, a chemotactic factor for satellite cells, is released by platelets from injured vessels and may contribute to the increment in the expression of PTN in the damaged area (74). Pleiotrophin not only enhances vascularization mechanisms but is equally involved in the formation of new myofibers (74). PTN mRNA and protein expression increase during the regeneration process of a crushed muscle with a peak at day 5 in the newly formed myotubes and in the activated myoblasts, being the levels restored 15 days after the damage (74).
Skeletal muscle plays a key role in metabolism, uptaking up to 80% of postprandial glucose and it is one of the key players in the development of whole-body insulin resistance (79). Moreover, muscle functions as an endocrine organ given its capacity to secrete molecules known as myokines that are released in the circulation (80). These myokines are essential for the crosstalk between the skeletal muscle and other organs, such as the adipose tissue, the brain, or the bone and may favor the development of some metabolic diseases (80). Transgenic mice over-expressing PTN in bone (PtnTg), under the control of the human bone specific osteocalcin promoter, exhibit higher oxidative metabolism in soleus muscle. This increment in oxidative metabolism may be the consequence of the enrichment in the number of the highly oxidative type 1 fibers, and higher mRNA levels of ATP-sensitive K+ channel (Abcc8), cytochrome c oxidase subunit IV (Cox4i1), and citrate synthase (Cs), and increased vascularization (72). Although these effects observed in these mice overexpressing PTN seem to be related to a paracrine action of this cytokine, from a metabolic point of view, a higher oxidative metabolism in the skeletal muscle may have a protective action against the development of insulin resistance and type 2 diabetes and highlights the role of this cytokine in the metabolic homeostasis in the skeletal muscle.
Role of pleiotrophin in adipogenesis and adipose tissue browning
An impairment of adipogenesis favors the development of obesity, insulin resistance and diabetes (81). Consequently, the molecules regulating preadipocyte proliferation and adipogenesis might be targets for the treatment of these metabolic diseases. PTN is expressed in the adipose tissue (82), and differential expression of PTN was found between omental and subcutaneous adipose tissue. As increased omental adipose tissue mass has a higher risk of obesity-associated metabolic diseases, PTN expression has therefore been proposed as a possible link between obesity, diabetes, and cardiovascular diseases (82).
Pleiotrophin gene expression varies during adipocyte differentiation suggesting a possible role of PTN in adipogenesis (26, 83). Particularly, Ptn expression is almost undetectable in preadipocytes, increases during confluence and quickly decreases when differentiation is induced. Moreover, as Ptn expression is induced by ADAMTS1 (ADAM metallopeptidase with thrombospondin type 1 motif 1) via the Wnt/β-catenin pathway, the activation of ADAMTS1 could also play a role in preadipocyte differentiation (84). In fact, when brown adipocytes differentiate, the levels of Adamts1 (84) are decreased and so the levels of Ptn (11), supporting the idea that PTN might be implicated in adipocyte proliferation but not in differentiation. In fact, as shown in the 3T3-L1 cell line, PTN impairs preadipocyte differentiation through the inhibition of PPARγ (Peroxisome proliferator-activated gamma) and through a crosstalk between the PTN/PI3K/Akt/GSK-3β/β-catenin and the Wnt/Fz/GSK-3β/β-catenin signaling pathways (83).
Some micro-RNAs that regulate adipogenesis have been involved in the development of metabolic disorders such as obesity and diabetes (85). miR-143 favors preadipocyte differentiation, as its levels begin to increase when adipocyte differentiation is induced. Furthermore, miR-143 interacts with the coding region of Ptn gene and it is able to repress Ptn expression (26). An up-regulated expression of miR-143 in association with an increased expression of Pparγ and aP2 (adipocyte protein 2), two adipocyte genes involved in the pathophysiology of obesity and insulin resistance, was found in the adipose tissue of obese mice fed with HFD (86).
Recent studies using a Ptn knock out mice model (Ptn-/-) reveal that Ptn deletion is associated with decreased body weight and adiposity, altered fat distribution, impaired periovarian white adipose tissue expandability, increased catecholamine-induced lipolysis and reduced inhibitory response to insulin of lipolysis (11). Moreover, Ptn-/- mice are glucose intolerant and develop insulin resistance in later life and preferentially oxidize fatty acids as main energy source instead of glucose both in the light and dark periods (11). In fact, Ptn deletion is associated with an increased conversion of T4 to T3 by deiodinase 2 (DIO2) and increased fatty acid thermogenesis in brown adipose tissue (11). Additionally, pleiotrophin deletion is associated with browning and increased expression of uncoupling protein-1 (UCP-1) and other specific markers of brown/beige adipocytes in periovarian adipose tissue of female Ptn-/- mice (57). Therefore, it has been proposed that absence of pleiotrophin has a protective role against high fat diet-induced insulin resistance and obesity in rodents.
Role of pleiotrophin in mammary gland development
PTN stimulates angiogenesis and promotes invasion and metastasis in breast cancer. Thus, PTN protein was purified for the first time from tissue culture supernatants of human breast cancer cells (87). PTN is expressed in primary breast cancers and in estrogen receptor-negative breast cancer cell lines (88), and is upregulated in carcinogen induced mammary carcinomas in rats (88).
Nevertheless, Ptn expression is also detected in the normal human breast (89) and in the normal mouse mammary gland (90). In the human, Ptn is expressed mainly in the alveolar epithelial and myoepithelial cells (89) and it is secreted in human milk and colostrum (91). In the mouse, Ptn is expressed in the adipocytes and in the epithelial cells (89) and the differentiation of mammary gland starts after weaning, and hormonal stimuli induces ductal tree proliferation, branching and invasion of the mammary fat pads. Treatment with a monoclonal anti-PTN neutralizing antibody has demonstrated that PTN is required to maintain mammary epithelial cells in a proliferative state and delays ductal outgrowth, branching and terminal end formation. In particular, PTN seems to inhibit differentiation and to delay mammary gland maturation through the inhibition of phospho ERK1/2 signaling (92).
The levels of Ptn mRNA also vary during the intense remodeling of mammary gland during pregnancy and lactation. Ptn expression is not modified during the first 10 days of pregnancy when ductal epithelial cells proliferate in mammary gland. However, by day 15, when the epithelial ductal cells start undergoing lobular-alveolar differentiation, Ptn mRNA levels are downregulated 30-fold. After weaning, during the apoptosis of mammary epithelial cells after lactation, Ptn mRNA levels are restored to the ones of non-pregnant animals (92). Moreover, lower Ptn mRNA levels were detected in the mammary gland of parous dams when compared to nulliparous dams in rodents (93).
Pleiotrophin and pancreas: the pancreatic beta cell
Pleiotrophin is highly expressed during embryonic and fetal development in those organs that undergo branching morphogenesis, like the pancreas (75). During the branching ductal morphogenesis of the embryonic pancreas in the mice, PTN modulates cell proliferation and angiogenesis (94). Prior to pancreatic evagination, PTN is located in the areas of vasculogenesis adjacent to the differentiating ductal epithelium. At the embryonic day E11-13 in mice, PTN is localized in the basement membranes of the pancreatic epithelium. With the progress of differentiation, PTN becomes localized only to the undifferentiated “cord” region, and by E18 only to blood vessels. Furthermore, in mouse 3D culture of E11 pancreatic explants, the antisense inhibition of Ptn expression impaired the differentiation of endocrine precursors and blunted glucagon and insulin expression (94).
Pancreatic β cells share with neurons that are excitable cells (95) and that retain high levels of Ptn expression in the adult. In fact, PTN maintains a high level of expression in the β cells of pancreatic islets of adult rats [10], mice [10] and humans [10]. The expression of Ptn in β cells could potentially contribute to adaptive increases in β cell mass as PTN is prominently expressed in Ins+ cells, the immature pancreatic β cells that have multipotential islet endocrine cell lineage potentiality, and retain the proliferative capacity (10).
Although αvβ3 integrin is a PTN receptor that is expressed in the pancreatic islets (96), RPTP β/ζ is the most probable target for PTN in pancreatic islets (10). Treatment with rPTN increased DNA synthesis and upregulated the expression of Pdx-1 and insulin genes in the INS1E rat insulinoma cells suggesting that PTN modulates β cell expansion through RPTP β/ζ and/or other surface binding proteins (10).
On the other hand, the excitability of β cells is dependent of glucose uptake and triggers the opening of the voltage-dependent calcium channels, allowing the entrance of calcium inside the β cell which leads to insulin secretion (97, 98). In previous studies using female Ptn-/- mice, glucose tolerance and insulin responsiveness were improved in 3-month-old Ptn-/- mice, but in later life these mice become hyperinsulinemic, insulin-resistant and glucose intolerant (11). Therefore, Ptn deletion may favor a prediabetic state that increases the susceptibility to develop diabetes in later stages of life. Besides, late pregnant Ptn knock out mice are hypoinsulinemic, hyperglycemic and glucose intolerant and have a decreased expression of key proteins involved in glucose and lipid uptake and metabolism (56).
Conclusions and future perspectives
Pleiotrophin has been widely studied in the field of cancer and much work has been also carried out to analyze its implications in the CNS. In this review, we summarize the main actions of PTN at the main metabolic peripheral organs obtained from the experiments in which pleiotrophin levels are increased (by recombinant pleiotrophin administration and transgenic overexpression) or reduced (by miRNA, siRNA, neutralizing antibodies or Ptn deletion).
In the mammary gland, this cytokine favors tumor growth and progression and delays the full development of the tissue. In this line of evidence, blocking PTN with anti-PTN antibody enhanced ductal development in the mammary glands. Pleiotrophin is expressed in the young endocrine pancreas and in the adult pancreas and it is overexpressed during β cell regeneration after streptozotocin treatment. Moreover, the administration of recombinant pleiotrophin in an insulinoma cell line induces β cell expansion and enhances the expression of insulin-related genes. In the muscle, pleiotrophin is overexpressed during tissue regeneration. Transgenic overexpression of Ptn in the muscle enhances vascularization and oxidative muscle metabolism through an increment in the activity of citric acid cycle and electron transport system. No evidence is available yet regarding the effects of Ptn deletion on muscle oxidative metabolism. However, the actions of PTN on oxidative metabolism and whole-body energy expenditure are also derived from the effects of PTN on adipose tissue and liver. Ptn expression increases in preadipocytes during confluence and quickly decreases once differentiation starts. In fact, when adipocyte differentiation starts miR-143 levels start to increase what may repress Ptn expression and treatment with rPTN inhibits preadipocyte differentiation. Nevertheless, pleiotrophin deletion impairs fat accumulation and the expansibility of adipose tissue and favors lipolysis of triacylglycerides in the adipose tissue and free fatty acid release. Moreover, pleiotrophin deletion induces browning in white adipose tissue and increases the thermogenic activity of the brown adipose tissue increasing the use of fatty acids instead of carbohydrates for energy production. The role of PTN on hepatic lipid metabolism and development has also been clearly evidenced. PTN expression increases during liver regeneration and development. Additionally, the overexpression of pleiotrophin has also shown to increase fatty acid synthesis and the expression of lipogenic genes. However, Ptn deletion decreases both the liver size and the gene expression of proteins involved in lipogenesis, both during late pregnancy and in a HFD-induced obesity mice model, and thus may protect against steatosis.
These findings suggest a role for pleiotrophin in the development of pathologies related to insulin resistance such as aging or diabetes, however, much effort is still needed to fully understand and deepen the knowledge of the field. We believe in the importance of a more profound and further characterization of Ptn knock out and transgenic animal models to fully elucidate the implications of this cytokine in pancreas functionality and muscle metabolism.
Author contributions
All authors contributed to the writing and review of the manuscript. All authors have read and agreed to the published version of the manuscript. The article is an original work, that hasn’t received prior publication and isn’t under consideration for publication elsewhere.
Funding
This research was funded by grants from the Ministerio de Ciencia, Innovación y Universidades of Spain (PID2021-123865OB-I00) and by Comunidad de Madrid (P2022/BMD-7227) to MPR-A. CB-P, JS and MS-A were recipients of CEU‐Santander mobility grant.
Conflict of interest
The authors declare that the research was conducted in the absence of any commercial or financial relationships that could be construed as a potential conflict of interest.
Publisher’s note
All claims expressed in this article are solely those of the authors and do not necessarily represent those of their affiliated organizations, or those of the publisher, the editors and the reviewers. Any product that may be evaluated in this article, or claim that may be made by its manufacturer, is not guaranteed or endorsed by the publisher.
References
1. Kovesdi I, Fairhurst JL, Kretschmer PJ, Böhlen P. Heparin-binding neurotrophic factor (HBNF) and MK, members of a new family of homologous, developmentally regulated proteins. Biochem Biophys Res Commun (1990) 172:850–4. doi: 10.1016/0006-291X(90)90753-A
2. Rauvala H. An 18-kd heparin-binding protein of developing brain that is distinct from fibroblast growth factors. EMBO J (1989) 8:2933–41. doi: 10.1002/j.1460-2075.1989.tb08443.x
3. Tezuka K, Takeshita S, Hakeda Y, Kumegawa M, Kikuno R, Hashimoto-Gotoh T. Isolation of mouse and human cDNA clones encoding a protein expressed specifically in osteoblasts and brain tissues. Biochem Biophys Res Commun (1990) 173:246–51. doi: 10.1016/S0006-291X(05)81048-4
4. Vanderwinden JM, Mailleux P, Schiffmann SN, Vanderhaeghen JJ. Cellular distribution of the new growth factor pleiotrophin (HB-GAM) mRNA in developing and adult rat tissues. Anat Embryol (1992) 186:387–406. doi: 10.1007/BF00185989
5. Merenmies J, Rauvala H. Molecular cloning of the 18-kDa growth-associated protein of developing brain. J Biol Chem (1990) 265:16721–4. doi: 10.1016/S0021-9258(17)44817-4
6. Li YS, Milner PG, Chauhan AK, Watson MA, Hoffman RM, Kodner CM, et al. Cloning and expression of a developmentally regulated protein that induces mitogenic and neurite outgrowth activity. Sci (New York NY) (1990) 250:1690–4. doi: 10.1126/science.2270483
7. Muramatsu T. Midkine (MK), the product of a retinoic acid responsive gene, and pleiotrophin constitute a new protein family regulating growth and differentiation. Int J Dev Biol (1993) 37:183–8. doi: 10.1387/ijdb.8507561
8. Menni C, Kiddle SJ, Mangino M, Viñuela A, Psatha M, Steves C, et al. Circulating proteomic signatures of chronological age. journals gerontol Ser A Biol Sci Med Sci (2015) 70:809–16. doi: 10.1093/gerona/glu121
9. Wang X. Pleiotrophin: activity and mechanism. Adv Clin Chem (2020) 98:51–89. doi: 10.1016/bs.acc.2020.02.003
10. Sevillano J, Liang A, Strutt B, Hill TG, Szlapinski S, Ramos-Álvarez MP, et al. Pleiotrophin expression and actions in pancreatic β-cells. Front Endocrinol (2022) 13:777868. doi: 10.3389/fendo.2022.777868
11. Sevillano J, Sánchez-Alonso MG, Zapatería B, Calderón M, Alcalá M, Limones M, et al. Pleiotrophin deletion alters glucose homeostasis, energy metabolism and brown fat thermogenic function in mice. Diabetologia (2019) 62:123–35. doi: 10.1007/s00125-018-4746-4
12. Deuel TF, Zhang N, Yeh HJ, Silos-Santiago I, Wang ZY. Pleiotrophin: a cytokine with diverse functions and a novel signaling pathway. Arch Biochem Biophys (2002) 397:162–71. doi: 10.1006/abbi.2001.2705
13. Lamprou M, Kaspiris A, Panagiotopoulos E, Giannoudis PV, Papadimitriou E. The role of pleiotrophin in bone repair. Injury (2014) 45:1816–23. doi: 10.1016/j.injury.2014.10.013
14. Fernández-Calle R, Vicente-Rodríguez M, Gramage E, Pita J, Pérez-García C, Ferrer-Alcón M, et al. Pleiotrophin regulates microglia-mediated neuroinflammation. J Neuroinflamm (2017) 14:46. doi: 10.1186/s12974-017-0823-8
15. Antoine M, Tag CG, Wirz W, Borkham-Kamphorst E, Sawitza I, Gressner AM, et al. Upregulation of pleiotrophin expression in rat hepatic stellate cells by PDGF and hypoxia: implications for its role in experimental biliary liver fibrogenesis. Biochem Biophys Res Commun (2005) 337:1153–64. doi: 10.1016/j.bbrc.2005.09.173
16. Zhou J, Yang Y, Zhang Y, Liu H, Dou Q. A meta-analysis on the role of pleiotrophin (PTN) as a prognostic factor in cancer. PloS One (2018) 13:e0207473. doi: 10.1371/journal.pone.0207473
17. Perez-Pinera P, Berenson JR, Deuel TF. Pleiotrophin, a multifunctional angiogenic factor: mechanisms and pathways in normal and pathological angiogenesis. Curr Opin Hematol (2008) 15:210–4. doi: 10.1097/MOH.0b013e3282fdc69e
18. Yao J, Hu XF, Feng XS, Gao SG. Pleiotrophin promotes perineural invasion in pancreatic cancer. World J Gastroenterol (2013) 19:6555–8. doi: 10.3748/wjg.v19.i39.6555
19. Lamprou M, Koutsioumpa M, Kaspiris A, Zompra K, Tselios T, Papadimitriou E. Binding of pleiotrophin to cell surface nucleolin mediates prostate cancer cell adhesion to osteoblasts. Tissue Cell (2022) 76:101801. doi: 10.1016/j.tice.2022.101801
20. Chang Y, Zuka M, Perez-Pinera P, Astudillo A, Mortimer J, Berenson JR, et al. Secretion of pleiotrophin stimulates breast cancer progression through remodeling of the tumor microenvironment. Proc Natl Acad Sci USA (2007) 104:10888–93. doi: 10.1073/pnas.0704366104
21. Ma J, Kong Y, Nan H, Qu S, Fu X, Jiang L, et al. Pleiotrophin as a potential biomarker in breast cancer patients. Clinica chimica acta; Int J Clin Chem (2017) 466:6–12. doi: 10.1016/j.cca.2016.12.030
22. Vacherot F, Laaroubi K, Caruelle D, Delbe J, Barritault D, Caruelle JP, et al. Upregulation of heparin-affin regulatory peptide by androgen. In vitro cellular & developmental biology. Animal (1995) 31:647–8. doi: 10.1007/BF02634082
23. Pufe T, Bartscher M, Petersen W, Tillmann B, Mentlein R. Expression of pleiotrophin, an embryonic growth and differentiation factor, in rheumatoid arthritis. Arthritis Rheum (2003) 48:660–7. doi: 10.1002/art.10839
24. Li YS, Gurrieri M, Deuel TF. Pleiotrophin gene expression is highly restricted and is regulated by platelet-derived growth factor. Biochem Biophys Res Commun (1992) 184:427–32. doi: 10.1016/0006-291X(92)91211-8
25. Tamura M, Ichikawa F, Guillerman RP, Deuel TF, Nodal M. 1α,25-dihydroxyvitamin D(3) down-regulates pleiotrophin messenger RNA expression in osteoblast-like cells. Endocrine (1995) 3:21–4. doi: 10.1007/BF02917444
26. Yi C, Xie WD, Li F, Lv Q, He J, Wu J, et al. MiR-143 enhances adipogenic differentiation of 3T3-L1 cells through targeting the coding region of mouse pleiotrophin. FEBS Lett (2011) 585:3303–9. doi: 10.1016/j.febslet.2011.09.015
27. Zhang L, Liu X, Liu J, Zhou Z, Song Y, Cao B, et al. miR-182 aids in receptive endometrium development in dairy goats by down-regulating PTN expression. PloS One (2017) 12:e0179783. doi: 10.1371/journal.pone.0179783
28. Zhang Q, Guo B, Hui Q, Chang P, Tao K. miR-137 inhibits proliferation and metastasis of hypertrophic scar fibroblasts via targeting pleiotrophin. Cell Physiol Biochem (2018) 49:985–95. doi: 10.1159/000493236
29. Bai PS, Xia N, Sun H, Kong Y. Pleiotrophin, a target of miR-384, promotes proliferation, metastasis and lipogenesis in HBV-related hepatocellular carcinoma. J Cell Mol Med (2017) 21:3023–43. doi: 10.1111/jcmm.13213
30. Lee JY, Jeong W, Lim W, Kim J, Bazer FW, Han JY, et al. Chicken pleiotrophin: regulation of tissue specific expression by estrogen in the oviduct and distinct expression pattern in the ovarian carcinomas. PloS One (2012) 7:e34215. doi: 10.1371/journal.pone.0034215
31. Elahouel R, Blanc C, Carpentier G, Frechault S, Cascone I, Destouches D, et al. Pleiotrophin exerts its migration and invasion effect through the neuropilin-1 pathway. Neoplasia (New York NY) (2015) 17:613–24. doi: 10.1016/j.neo.2015.07.007
32. Deepa SS, Yamada S, Zako M, Goldberger O, Sugahara K. Chondroitin sulfate chains on syndecan-1 and syndecan-4 from normal murine mammary gland epithelial cells are structurally and functionally distinct and cooperate with heparan sulfate chains to bind growth factors. a novel function to control binding of midkine, pleiotrophin, and basic fibroblast growth factor. J Biol Chem (2004) 279:37368–76. doi: 10.1074/jbc.M403031200
33. Landgraf P, Wahle P, Pape HC, Gundelfinger ED, Kreutz MR. The survival-promoting peptide y-P30 enhances binding of pleiotrophin to syndecan-2 and -3 and supports its neuritogenic activity. J Biol Chem (2008) 283:25036–45. doi: 10.1074/jbc.M800963200
34. Mikelis C, Sfaelou E, Koutsioumpa M, Kieffer N, Papadimitriou E. Integrin alpha(v)beta(3) is a pleiotrophin receptor required for pleiotrophin-induced endothelial cell migration through receptor protein tyrosine phosphatase beta/zeta. FASEB J (2009) 23:1459–69. doi: 10.1096/fj.08-117564
35. Shen D, Podolnikova NP, Yakubenko VP, Ardell CL, Balabiyev A, Ugarova TP, et al. Pleiotrophin, a multifunctional cytokine and growth factor, induces leukocyte responses through the integrin mac-1. J Biol Chem (2017) 292:18848–61. doi: 10.1074/jbc.M116.773713
36. Stoica GE, Kuo A, Aigner A, Sunitha I, Souttou B, Malerczyk C, et al. Identification of anaplastic lymphoma kinase as a receptor for the growth factor pleiotrophin. J Biol Chem (2001) 276:16772–9. doi: 10.1074/jbc.M010660200
37. Meng K, Rodriguez-Peña A, Dimitrov T, Chen W, Yamin M, Noda M, et al. Pleiotrophin signals increased tyrosine phosphorylation of beta beta-catenin through inactivation of the intrinsic catalytic activity of the receptor-type protein tyrosine phosphatase beta/zeta. Proc Natl Acad Sci USA (2000) 97:2603–8. doi: 10.1073/pnas.020487997
38. Tanaka M, Maeda N, Noda M, Marunouchi T. A chondroitin sulfate proteoglycan PTPzeta /RPTPbeta regulates the morphogenesis of purkinje cell dendrites in the developing cerebellum. J Neurosci (2003) 23:2804–14. doi: 10.1523/JNEUROSCI.23-07-02804.2003
39. Diamantopoulou Z, Kitsou P, Menashi S, Courty J, Katsoris P. Loss of receptor protein tyrosine phosphatase β/ζ (RPTPβ/ζ) promotes prostate cancer metastasis. J Biol Chem (2012) 287:40339–49. doi: 10.1074/jbc.M112.405852
40. Sevillano J, Sánchez-Alonso MG, Pizarro-Delgado J, Ramos-Álvarez MDP. Role of receptor protein tyrosine phosphatases (RPTPs) in insulin signaling and secretion. Int J Mol Sci (2021) 22(11):5812. doi: 10.3390/ijms22115812
41. Deuel TF. Anaplastic lymphoma kinase: "Ligand independent activation" mediated by the PTN/RPTPβ/ζ signaling pathway. Biochim Biophys Acta (2013) 1834:2219–23. doi: 10.1016/j.bbapap.2013.06.004
42. Koutsioumpa M, Polytarchou C, Courty J, Zhang Y, Kieffer N, Mikelis C, et al. Interplay between αvβ3 integrin and nucleolin regulates human endothelial and glioma cell migration. J Biol Chem (2013) 288:343–54. doi: 10.1074/jbc.M112.387076
43. Koutsioumpa M, Drosou G, Mikelis C, Theochari K, Vourtsis D, Katsoris P, et al. Pleiotrophin expression and role in physiological angiogenesis in vivo: potential involvement of nucleolin. Vasc Cell (2012) 4:4. doi: 10.1186/2045-824X-4-4
44. Feng ZJ, Gao SB, Wu Y, Xu XF, Hua X, Jin GH. Lung cancer cell migration is regulated via repressing growth factor PTN/RPTP β/ζ signaling by menin. Oncogene (2010) 29:5416–26. doi: 10.1038/onc.2010.282
45. Muramatsu T. Midkine and pleiotrophin: two related proteins involved in development, survival, inflammation and tumorigenesis. J Biochem (2002) 132:359–71. doi: 10.1093/oxfordjournals.jbchem.a003231
46. Yokoi H, Kasahara M, Mori K, Ogawa Y, Kuwabara T, Imamaki H, et al. Pleiotrophin triggers inflammation and increased peritoneal permeability leading to peritoneal fibrosis. Kidney Int (2012) 81:160–9. doi: 10.1038/ki.2011.305
47. Cañeque-Rufo H, Sánchez-Alonso MG, Zuccaro A, Sevillano J, Ramos-Álvarez MDP, Herradón G. Pleiotrophin deficiency protects against high-fat diet-induced neuroinflammation: implications for brain mitochondrial dysfunction and aberrant protein aggregation. Food Chem Toxicol (2023) 172:113578. doi: 10.1016/j.fct.2022.113578
48. Samson SL, Garber AJ. Metabolic syndrome. Endocrinol Metab Clinics North America (2014) 43:1–23. doi: 10.1016/j.ecl.2013.09.009
49. Gonçalves RA, De Felice FG. The crosstalk between brain and periphery: implications for brain health and disease. Neuropharmacology (2021) 197:108728. doi: 10.1016/j.neuropharm.2021.108728
50. Schwartz MW, Porte D Jr. Diabetes, obesity, and the brain. Sci (New York N.Y.) (2005) 307:375–9. doi: 10.1126/science.1104344
51. Nguyen TT, Hulme J, Vo TK, Van Vo G. The potential crosstalk between the brain and visceral adipose tissue in alzheimer's development. Neurochem Res (2022) 47:1503–12. doi: 10.1007/s11064-022-03569-1
52. Flores-Dorantes MT, Díaz-López YE, Gutiérrez-Aguilar R. Environment and gene association with obesity and their impact on neurodegenerative and neurodevelopmental diseases. Front Neurosci (2020) 14:863. doi: 10.3389/fnins.2020.00863
53. Pugazhenthi S, Qin L, Reddy PH. Common neurodegenerative pathways in obesity, diabetes, and alzheimer's disease. Biochim Biophys Acta Mol basis Dis (2017) 1863:1037–45. doi: 10.1016/j.bbadis.2016.04.017
54. Herradón G, Pérez-García C. Targeting midkine and pleiotrophin signalling pathways in addiction and neurodegenerative disorders: recent progress and perspectives. Br J Pharmacol (2014) 171:837–48. doi: 10.1111/bph.12312
55. Herradon G, Ramos-Alvarez MP, Gramage E. Connecting metainflammation and neuroinflammation through the PTN-MK-RPTPβ/ζ axis: relevance in therapeutic development. Front Pharmacol (2019) 10:377. doi: 10.3389/fphar.2019.00377
56. Zapatería B, Sevillano J, Sánchez-Alonso MG, Limones M, Pizarro-Delgado J, Zuccaro A, et al. Deletion of pleiotrophin impairs glucose tolerance and liver metabolism in pregnant mice: moonlighting role of glycerol kinase. FASEB J (2021) 35:e21911. doi: 10.1096/fj.202101181R
57. Zuccaro A, Zapatería B, Sánchez-Alonso MG, Haro M, Limones M, Terrados G, et al. Pleiotrophin deficiency induces browning of periovarian adipose tissue and protects against high-fat diet-induced hepatic steatosis. Int J Mol Sci (2021) 22(17):9261. doi: 10.3390/ijms22179261
59. Saltiel AR, Kahn CR. Insulin signalling and the regulation of glucose and lipid metabolism. Nature (2001) 414:799–806. doi: 10.1038/414799a
60. Michalopoulos GK, Bhushan B. Liver regeneration: biological and pathological mechanisms and implications. Nat Rev Gastroenterol Hepatol (2021) 18:40–55. doi: 10.1038/s41575-020-0342-4
61. Böhm F, Köhler UA, Speicher T, Werner S. Regulation of liver regeneration by growth factors and cytokines. EMBO Mol Med (2010) 2:294–305. doi: 10.1002/emmm.201000085
62. Fausto N, Laird AD, Webber EM. Liver regeneration. 2. role of growth factors and cytokines in hepatic regeneration. FASEB J (1995) 9:1527–36. doi: 10.1096/fasebj.9.15.8529831
63. Hoffmann K, Nagel AJ, Tanabe K, Fuchs J, Dehlke K, Ghamarnejad O, et al. Markers of liver regeneration-the role of growth factors and cytokines: a systematic review. BMC Surg (2020) 20:31. doi: 10.1186/s12893-019-0664-8
64. Lowes KN, Croager EJ, Olynyk JK, Abraham LJ, Yeoh GC. Oval cell-mediated liver regeneration: role of cytokines and growth factors. J Gastroenterol Hepatol (2003) 18:4–12. doi: 10.1046/j.1440-1746.2003.02906.x
65. Murtha-Lemekhova A, Fuchs J, Ghamarnejad O, Nikdad M, Probst P, Hoffmann K. Influence of cytokines, circulating markers and growth factors on liver regeneration and post-hepatectomy liver failure: a systematic review and meta-analysis. Sci Rep (2021) 11:13739. doi: 10.1038/s41598-021-92888-4
66. Park TJ, Jeong BR, Tateno C, Kim HS, Ogawa T, Lim IK, et al. Pleiotrophin inhibits transforming growth factor beta1-induced apoptosis in hepatoma cell lines. Mol carcinogenesis (2008) 47:784–96. doi: 10.1002/mc.20438
67. Ochiai K, Muramatsu H, Yamamoto S, Ando H, Muramatsu T. The role of midkine and pleiotrophin in liver regeneration. Liver Int (2004) 24:484–91. doi: 10.1111/j.1478-3231.2004.0990.x
68. Asahina K, Sato H, Yamasaki C, Kataoka M, Shiokawa M, Katayama S, et al. Pleiotrophin/heparin-binding growth-associated molecule as a mitogen of rat hepatocytes and its role in regeneration and development of liver. Am J Pathol (2002) 160:2191–205. doi: 10.1016/S0002-9440(10)61167-4
69. Michelotti GA, Tucker A, Swiderska-Syn M, Machado MV, Choi SS, Kruger L, et al. Pleiotrophin regulates the ductular reaction by controlling the migration of cells in liver progenitor niches. Gut (2016) 65:683–92. doi: 10.1136/gutjnl-2014-308176
70. Sato H, Funahashi M, Kristensen DB, Tateno C, Yoshizato K. Pleiotrophin as a Swiss 3T3 cell-derived potent mitogen for adult rat hepatocytes. Exp Cell Res (1999) 246:152–64. doi: 10.1006/excr.1998.4304
71. Chen HW, Yu SL, Chen WJ, Yang PC, Chien CT, Chou HY, et al. Dynamic changes of gene expression profiles during postnatal development of the heart in mice. Heart (British Cardiac Society) (2004) 90:927–34. doi: 10.1136/hrt.2002.006734
72. Camerino GM, Pierno S, Liantonio A, De Bellis M, Cannone M, Sblendorio V, et al. Effects of pleiotrophin overexpression on mouse skeletal muscles in normal loading and in actual and simulated microgravity. PloS One (2013) 8:e72028. doi: 10.1371/journal.pone.0072028
73. Li J, Wei H, Chesley A, Moon C, Krawczyk M, Volkova M, et al. The pro-angiogenic cytokine pleiotrophin potentiates cardiomyocyte apoptosis through inhibition of endogenous AKT/PKB activity. J Biol Chem (2007) 282:34984–93. doi: 10.1074/jbc.M703513200
74. Caruelle D, Mazouzi Z, Husmann I, Delbé J, Duchesnay A, Gautron J, et al. Upregulation of HARP during in vitro myogenesis and rat soleus muscle regeneration. J Muscle Res Cell Motil (2004) 25:45–53. doi: 10.1023/B:JURE.0000021387.92378.2e
75. Mitsiadis TA, Salmivirta M, Muramatsu T, Muramatsu H, Rauvala H, Lehtonen E, et al. Expression of the heparin-binding cytokines, midkine (MK) and HB-GAM (pleiotrophin) is associated with epithelial-mesenchymal interactions during fetal development and organogenesis. Development (1995) 121:37–51. doi: 10.1242/dev.121.1.37
76. Szabat E, Rauvala H. Role of HB-GAM (heparin-binding growth-associated molecule) in proliferation arrest in cells of the developing rat limb and its expression in the differentiating neuromuscular system. Dev Biol (1996) 178:77–89. doi: 10.1006/dbio.1996.0199
77. Blondet B, Carpentier G, Ferry A, Courty J. Exogenous pleiotrophin applied to lesioned nerve impairs muscle reinnervation. Neurochem Res (2006) 31:907–13. doi: 10.1007/s11064-006-9095-x
78. Husmann I, Soulet L, Gautron J, Martelly I, Barritault D. Growth factors in skeletal muscle regeneration. Cytokine Growth Factor Rev (1996) 7:249–58. doi: 10.1016/S1359-6101(96)00029-9
79. Merz KE, Thurmond DC. Role of skeletal muscle in insulin resistance and glucose uptake. Compr Physiol (2020) 10:785–809. doi: 10.1002/cphy.c190029
80. Chen W, Wang L, You W, Shan T. Myokines mediate the cross talk between skeletal muscle and other organs. J Cell Physiol (2021) 236:2393–412. doi: 10.1002/jcp.30033
81. McLaughlin T, Sherman A, Tsao P, Gonzalez O, Yee G, Lamendola C, et al. Enhanced proportion of small adipose cells in insulin-resistant vs insulin-sensitive obese individuals implicates impaired adipogenesis. Diabetologia (2007) 50:1707–15. doi: 10.1007/s00125-007-0708-y
82. Hoggard N, Cruickshank M, Moar KM, Bashir S, Mayer CD. Using gene expression to predict differences in the secretome of human omental vs. subcutaneous adipose tissue. Obes (Silver Spring Md) (2012) 20:1158–67. doi: 10.1038/oby.2012.14
83. Gu D, Yu B, Zhao C, Ye W, Lv Q, Hua Z, et al. The effect of pleiotrophin signaling on adipogenesis. FEBS Lett (2007) 581:382–8. doi: 10.1016/j.febslet.2006.12.043
84. Wong JC, Krueger KC, Costa MJ, Aggarwal A, Du H, McLaughlin TL, et al. A glucocorticoid- and diet-responsive pathway toggles adipocyte precursor cell activity in vivo. Sci Signaling (2016) 9:ra103. doi: 10.1126/scisignal.aag0487
85. Landrier JF, Derghal A, Mounien L. MicroRNAs in obesity and related metabolic disorders. Cells (2019) 8(8):859. doi: 10.3390/cells8080859
86. Takanabe R, Ono K, Abe Y, Takaya T, Horie T, Wada H, et al. Up-regulated expression of microRNA-143 in association with obesity in adipose tissue of mice fed high-fat diet. Biochem Biophys Res Commun (2008) 376:728–32. doi: 10.1016/j.bbrc.2008.09.050
87. Wellstein A, Fang WJ, Khatri A, Lu Y, Swain SS, Dickson RB, et al. A heparin-binding growth factor secreted from breast cancer cells homologous to a developmentally regulated cytokine. J Biol Chem (1992) 267:2582–7. doi: 10.1016/S0021-9258(18)45920-0
88. Fang W, Hartmann N, Chow DT, Riegel AT, Wellstein A. Pleiotrophin stimulates fibroblasts and endothelial and epithelial cells and is expressed in human cancer. J Biol Chem (1992) 267:25889–97. doi: 10.1016/S0021-9258(18)35692-8
89. Ledoux D, Caruelle D, Sabourin JC, Liu J, Crepin M, Barritault D, et al. Cellular distribution of the angiogenic factor heparin affin regulatory peptide (HARP) mRNA and protein in the human mammary gland. J Histochem Cytochem (1997) 45:1239–45. doi: 10.1177/002215549704500907
90. Kouros-Mehr H, Werb Z. Candidate regulators of mammary branching morphogenesis identified by genome-wide transcript analysis. Dev dynamics (2006) 235:3404–12. doi: 10.1002/dvdy.20978
91. Bernard-Pierrot I, Delbé J, Heroult M, Rosty C, Soulié P, Barritault D, et al. Heparin affin regulatory peptide in milk: its involvement in mammary gland homeostasis. Biochem Biophys Res Commun (2004) 314:277–82. doi: 10.1016/j.bbrc.2003.12.101
92. Rosenfield SM, Bowden ET, Cohen-Missner S, Gibby KA, Ory V, Henke RT, et al. Pleiotrophin (PTN) expression and function and in the mouse mammary gland and mammary epithelial cells. PloS One (2012) 7:e47876. doi: 10.1371/journal.pone.0047876
93. D'Cruz CM, Moody SE, Master SR, Hartman JL, Keiper EA, Imielinski MB, et al. Persistent parity-induced changes in growth factors, TGF-beta3, and differentiation in the rodent mammary gland. Mol Endocrinol (Baltimore Md) (2002) 16:2034–51. doi: 10.1210/me.2002-0073
94. Li Z, Mehta SS, Prasadan K, Hembree M, Holcomb GW, Ostlie DJ, et al. Pleiotrophin signaling in pancreatic organogenesis and differentiation. J Surg Res (2003) 114:283–4. doi: 10.1016/j.jss.2003.08.043
95. Eberhard D. Neuron and beta-cell evolution: learning about neurons is learning about beta-cells. BioEssays News Rev Molecular Cell Dev Biol (2013) 35:584. doi: 10.1002/bies.201300035
96. Cirulli V, Beattie GM, Klier G, Ellisman M, Ricordi C, Quaranta V, et al. Expression and function of alpha(v)beta(3) and alpha(v)beta(5) integrins in the developing pancreas: roles in the adhesion and migration of putative endocrine progenitor cells. J Cell Biol (2000) 150:1445–60. doi: 10.1083/jcb.150.6.1445
97. Antunes CM, Salgado AP, Rosário LM, Santos RM. Differential patterns of glucose-induced electrical activity and intracellular calcium responses in single mouse and rat pancreatic islets. Diabetes (2000) 49:2028–38. doi: 10.2337/diabetes.49.12.2028
Keywords: pleiotrophin, receptor protein tyrosine phosphatase β/ζ (RPTP β/ζ), metabolism, metabolic disorders, peripheral organs
Citation: Ballesteros-Pla C, Sánchez-Alonso MG, Pizarro-Delgado J, Zuccaro A, Sevillano J and Ramos-Álvarez MP (2023) Pleiotrophin and metabolic disorders: insights into its role in metabolism. Front. Endocrinol. 14:1225150. doi: 10.3389/fendo.2023.1225150
Received: 18 May 2023; Accepted: 26 June 2023;
Published: 07 July 2023.
Edited by:
Yanshan Dai, Bristol Myers Squibb, United StatesReviewed by:
Xiang Ou, University of South China Affiliated Changsha Central Hospital, ChinaKe Wu, Wuhan University, China
Copyright © 2023 Ballesteros-Pla, Sánchez-Alonso, Pizarro-Delgado, Zuccaro, Sevillano and Ramos-Álvarez. This is an open-access article distributed under the terms of the Creative Commons Attribution License (CC BY). The use, distribution or reproduction in other forums is permitted, provided the original author(s) and the copyright owner(s) are credited and that the original publication in this journal is cited, in accordance with accepted academic practice. No use, distribution or reproduction is permitted which does not comply with these terms.
*Correspondence: Julio Sevillano, jsevilla@ceu.es