What do we know about the microbiome of I. ricinus?
- 1Department of Microbiology and Virology, Faculty of Natural Sciences, Comenius University in Bratislava, Bratislava, Slovakia
- 2Institute of Zoology, Slovak Academy of Sciences, Bratislava, Slovakia
- 3Comenius University Science Park, Comenius University in Bratislava, Bratislava, Slovakia
I. ricinus is an obligate hematophagous parasitic arthropod that is responsible for the transmission of a wide range of zoonotic pathogens including spirochetes of the genus Borrelia, Rickettsia spp., C. burnetii, Anaplasma phagocytophilum and Francisella tularensis, which are part the tick´s microbiome. Most of the studies focus on “pathogens” and only very few elucidate the role of “non-pathogenic” symbiotic microorganisms in I. ricinus. While most of the members of the microbiome are leading an intracellular lifestyle, they are able to complement tick´s nutrition and stress response having a great impact on tick´s survival and transmission of pathogens. The composition of the tick´s microbiome is not consistent and can be tied to the environment, tick species, developmental stage, or specific organ or tissue. Ovarian tissue harbors a stable microbiome consisting mainly but not exclusively of endosymbiotic bacteria, while the microbiome of the digestive system is rather unstable, and together with salivary glands, is mostly comprised of pathogens. The most prevalent endosymbionts found in ticks are Rickettsia spp., Ricketsiella spp., Coxiella-like and Francisella-like endosymbionts, Spiroplasma spp. and Candidatus Midichloria spp. Since microorganisms can modify ticks’ behavior, such as mobility, feeding or saliva production, which results in increased survival rates, we aimed to elucidate the potential, tight relationship, and interaction between bacteria of the I. ricinus microbiome. Here we show that endosymbionts including Coxiella-like spp., can provide I. ricinus with different types of vitamin B (B2, B6, B7, B9) essential for eukaryotic organisms. Furthermore, we hypothesize that survival of Wolbachia spp., or the bacterial pathogen A. phagocytophilum can be supported by the tick itself since coinfection with symbiotic Spiroplasma ixodetis provides I. ricinus with complete metabolic pathway of folate biosynthesis necessary for DNA synthesis and cell division. Manipulation of tick´s endosymbiotic microbiome could present a perspective way of I. ricinus control and regulation of spread of emerging bacterial pathogens.
1 Introduction
Ticks are ubiquitous arthropod species belonging to the Ixodida order. They are hematophagous obligate ectoparasites and can be found in a high variety of habitats, ranging from driest (Foughali et al., 2021; Abdulsalam et al., 2022) to most humid (Kim et al., 2018; Yang et al., 2020; Dantas-Torres et al., 2021). Ixodida are represented by two major families: Argasidae (soft ticks) and Ixodidae (hard ticks) (Guglielmone et al., 2010). Majority of tick species belong to the Ixodidae family (Zachary, 2017). Their life cycle consists of four developmental stages (egg, larva, nymph, and adult) (Leal et al., 2020), and each active stage requires a single blood meal.
Due to their relatively low mobility, most ixodid ticks including Ixodes spp. adopt the questing strategy, where they climb on top of vegetation waiting for a suitable host (Nicholson et al., 2019). Ixodid ticks have extended feeding times, lasting from hours to weeks, depending on the species and developmental stage (Richter et al., 2013; Sonenshine and Anderson, 2014; Vancová et al., 2020). They have developed different feeding strategies that depend on the habitat and/or the opportunity of contact with the appropriate host. Based on the number of hosts that ticks feed on during their parasitic life cycle, they are classified into one-, two- and three-host ticks (Taylor and Coop, 2015). I. ricinus, the most common species in Europe, is a three host tick having a wide range of hosts with over 300 terrestrial vertebrate species (Gray et al., 2021). The generation time of I. ricinus is on average three years, albeit it can vary from two to six years (Anderson and Magnarelli, 2008).
Internal organs of a tick are placed in an open cavity and are surrounded by hemolymph which consists of plasma and hemocytes. Hemolymph circulation is aided by a simple “heart”. The main internal organs of ticks comprise the digestive tract, salivary glands, reproduction organs, Malpighian tubules, respiratory system and central nervous system (CNS). The CNS is fused into a single compact organ, synganglion, located in the anterior ventral region of the body (Sonenshine and Anderson, 2014). Paired salivary glands contain large grape-like clusters, known as acini, located in the anterolateral area of the body cavity. During feeding, salivary glands significantly expand in size (up to 25 times) and act as a complex multifunctional organ that regulates water balance and production of saliva and “cement”. Cement is used for the attachment of ticks to the host skin. Salivary glands products are injected via the tick mouthparts into the host skin. This route is pivotal for transmission of majority of tick-borne pathogens (Bowman et al., 2008; Sonenshine and Anderson, 2014). Given the high complexity of tick saliva, it carries many functions, including anti-haemostatic, anti-inflammatory, anti-wound healing, immunomodulatory and vasoactive (Prevot et al., 2006; Oliveira et al., 2011a; Pekáriková et al., 2015; Scholl et al., 2016; Tirloni et al., 2019; Zhou et al., 2020; Aounallah et al., 2021). The tick alimentary system is divided into three primary regions: the preoral canal and foregut, the midgut, and the hindgut. The midgut is the largest organ in the body of feeding ticks. The segmented midgut is well adapted to accommodate an enormous amount of host blood and fills most of the internal body space. Since digestion in ticks occurs intracellularly, the midgut also functions as a storage organ, enabling continuous digestion of its content over long periods (Sonenshine and Anderson, 2014; Mahmood et al., 2020). The hindgut is composed of the intestine, the rectal sac, a large bulbous excretory organ, and the rectum (Sonenshine and Anderson, 2014; Šimo and Park, 2014). The female reproductive system consists of U-shaped ovaries located in the posterior region of the body, paired oviducts, uterus, and vagina. Ovaries in unfed females appear as a thin band of cells and in fed females as a large organ with multiple oocytes of differing sizes (Sonenshine and Anderson, 2014).
As each animal species, ticks also possess specific microbial communities - microbiomes. A microbiome is defined as a community of commensal, symbiotic, and pathogenic microorganisms that inhabit assorted niches in the host’s body (Hooper and Gordon, 2001). In addition to eubacteria, the microbiome can be also composed of Archaea, viruses, and eukaryotic microorganisms such as protozoa or fungi. Transmission of maternal microbiota to the offspring, proved in humans (Dunn et al., 2017) and mice (Jašarević et al., 2021), constitutes a foundation for a healthy microbiome. In ticks, besides transovarially transmitted endosymbiotic bacteria (Baldridge et al., 2009), the maternal microbiome may provide initial inoculum of other microorganisms in eggs and developing larvae (Narasimhan et al., 2014).
Despite a diet limited to the host blood, the tick microbiome appears to be quite complex (Carpi et al., 2011; Pollet et al., 2020; Narasimhan et al., 2021; Rousseau et al., 2021; Kumar et al., 2022a). Tick microbial communities were found to include tick-borne pathogens causing diseases in humans and animals (viruses, bacteria, protozoa) or pathogens and parasites that infect ticks (microsporidia, fungi, nematodes, hymenopteran parasitoids), and non-pathogenic microorganisms such as commensals or endosymbiotic, mutualistic microbes comprising mainly bacteria. The diversity of the tick microbiome can be tied to tick species, its life stage, sex, and specific organ or tissue (Andreotti et al., 2011; Menchaca et al., 2013; Budachetri et al., 2014; Van Treuren et al., 2015; Zolnik et al., 2016; Pollet et al., 2020; Batool et al., 2021; Krawczyk et al., 2022). It may also be dependent on the geographical region, environment (soil and plants), season, and since ticks are obligate hematophagous ectoparasites, also on the microbiota of the host’s skin and blood (Carpi et al., 2011; Lalzar et al., 2012; Van Treuren et al., 2015; Gurfield et al., 2017; Swei and Kwan, 2017; Krawczyk et al., 2022).
Introducing high-throughput “omics” approaches can be considered as a milestone not only in analyzes of tick genomes and proteomes, but also of tick microbiomes (Valenzuela, 2002; Carpi et al., 2011; Michelet et al., 2014; Greay et al., 2018). Although in this field significant progress has been made, there is still a big knowledge gap regarding the tissue-specific microbiota identification, microbiota member characterization and functional role detection. In vitro studies and/or single-cell analysis that could help to fill in the gap in the understanding of the intra microbiota community interaction as well as inter host (tick) – microbiota interactions are still challenging.
2 Tick microbial coinhabitants
Beneficial symbiotic organisms can be separated into two categories: primary and secondary symbionts. Primary, or obligate, symbionts, mutualists, are essential organisms and are ubiquitous throughout the host population. Many obligate symbionts of ticks are intracellular endosymbionts (Duron et al., 2018; Ben-Yosef et al., 2020; Foughali et al., 2021), while secondary symbionts are usually extracellular (Guizzo et al., 2022). Secondary, or facultative, symbiotic organisms have the ability to improve host fitness or ecological traits but are not vital for the host’s survival. Facultative symbionts often lack specialization and various microorganisms can fulfil their secondary role (Salcedo-Porras et al., 2020). In symbiotic relations a genomic complementarity of biochemical pathways, which is required for the survival of both host and symbiotic organisms is often found (Hansen and Moran, 2011). Bacterial endosymbionts can be found in different host organs inside host cells (Klyachko et al., 2007; Qiu et al., 2014; Guizzo et al., 2017; Al-Khafaji et al., 2020; Oliver et al., 2021).
In addition to tick-borne pathogens, a diverse group of commensal and symbiotic microorganisms is present within the tick microbiome (Carpi et al., 2011; Williams-Newkirk et al., 2014; Duron et al., 2017). Since the majority of tick microbiome research has been aimed towards pathogens, the biology of symbionts and their effects on ticks remain largely unexplored. However, non-pathogenic microorganisms may play a role in nutritional adaptation, reproduction, development, immunity or aiding the transmission of tick-borne pathogens (Bonnet et al., 2017). Among the tick symbionts, Coxiella-like endosymbionts, Rickettsia-like endosymbionts (Noda et al., 1997), Francisella-like endosymbionts (Sun et al., 2000), Cand. Midichloria mitochondrii (Sassera et al., 2008), Arsenophonus-like endosymbionts (Clay et al., 2008; Dergousoff and Chilton, 2010), Rickettsiella spp. (Garcia-Vozmediano et al., 2022), and Wolbachia spp. (Zhang et al., 2011) are included (Figure 1).
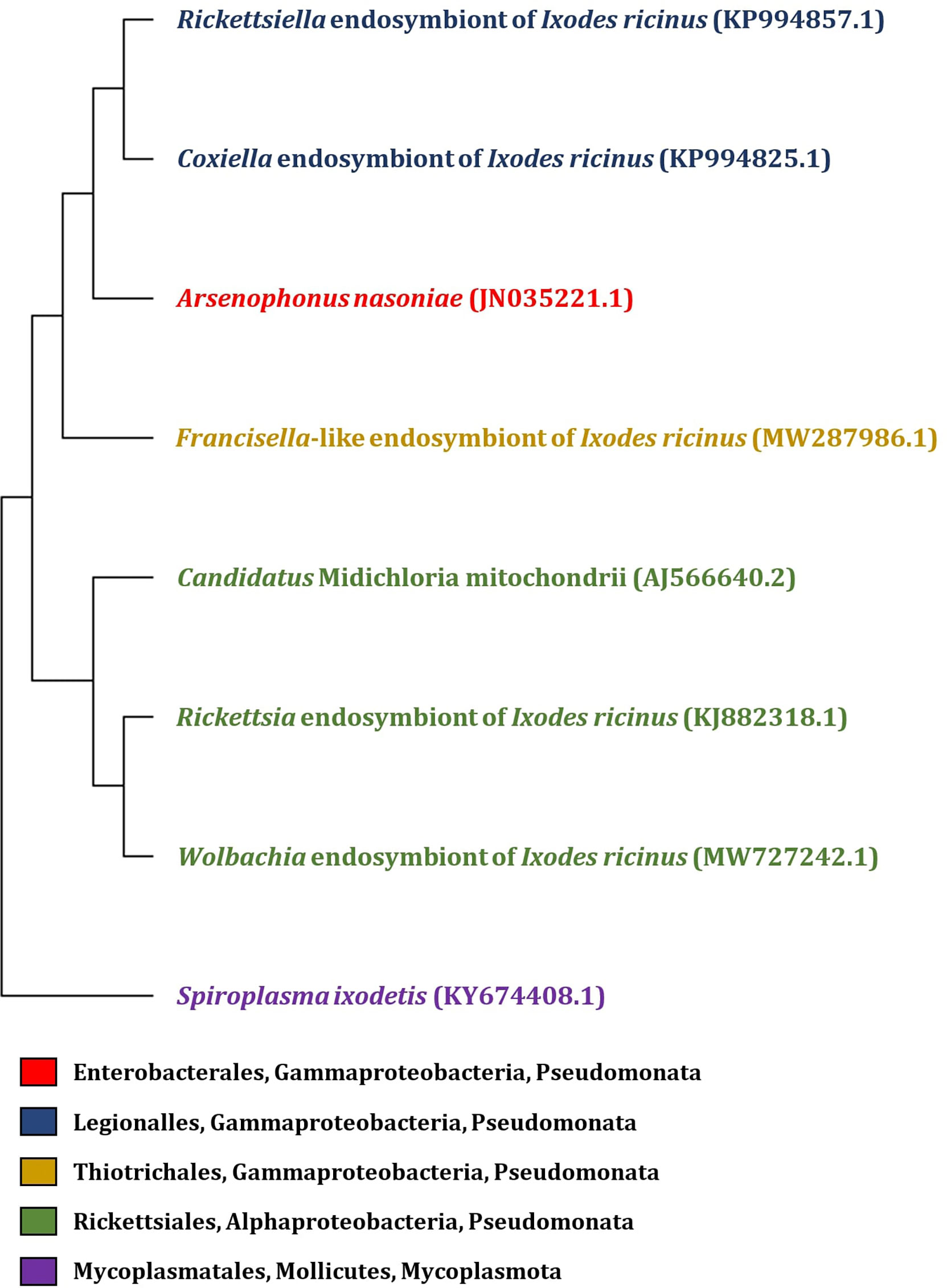
Figure 1 Phylogenetic tree of endosymbiotic bacteria based on 16S rRNA gene identified in I. ricinus. The phylogenetic tree was constructed by MEGA 11 program, with partial 16S rRNA gene sequence of endosymbionts isolated from the tick I. ricinus (the GenBank accession numbers are listed in the figure). Sequences were aligned using Muscle (Multiple Sequence Alignement) and the tree was constructed by Neighbor-joining method with Maximum Composite Likelyhood model.
2.1 Coxiella-like endosymbionts
Genus Coxiella contains only a single validly described species, C. burnetii (Shaw and Voth, 2019). C. burnetii is an obligate intracellular bacterium (Maurin and Raoult, 1999) and the etiological agent of Q fever in humans and animals. Q fever is a worldwide distributed zoonotic disease, with symptoms including fever, hepatitis and respiratory complications (Raoult, 1993). Although, many nonpathogenic Legionellales evolved together with the nonvertebrate hosts, ticks play an important role in the circulation of C. burnetii in natural foci and are partly responsible for the dissemination of the infection among animals (Psaroulaki et al., 2014; Duron et al., 2018; Špitalská et al., 2018). Coxiella-like bacteria have a certain degree of identity with C. burnetii, but this identity is not sufficient to consider them as the same species (Angelakis et al., 2016). Based on the sequence of the small ribosomal subunit (16S rRNA) gene, all Coxiella-like bacteria belong to the Coxiella genus (Gammaproteobacteria). The identity between C. burnetii and Coxiella-like bacteria was found to range from 91% to 98% (Zhong, 2012). Whole genome sequencing and multi-locus typing analyses showed that Coxiella-like bacteria represent a monophyletic and ancient group allied to ticks. Coxiella burnetti originated from progenitors of endosymbiotic Coxiella sp. hosted by ticks and was derived from a rare and quite recent event, likely by acquisition of virulence factor (Duron et al., 2015). Probably because of different approaches for C. burnetii detection, according to available literature, out of several studies on I. ricinus, only in one C. burnetii was detected.
Tick Coxiella-like endosymbionts (Coxiella-LE) cannot be found associated with any specific tissue. They are located across the majority of tick organs, however, organs that are infected most likely and in the highest concentration are Malpighian tubules and ovaries. Significant distribution of Coxiella-LE in tick reproductive organs suggests they are primarily transmitted transovarially and also transtadially (Clay et al., 2008; Zhong, 2012). Vertically transmitted Coxiella-LE that were detected in eggs and larvae of different individuals, beared 98% identity of the 16S rRNA sequence. Similar to other endosymbionts, localization of Coxiella in ovaries may be advantageous for its survival and transmission (Almeida et al., 2012; Lalzar et al., 2014; Duron et al., 2015). High concentration of Coxiella in Malpighian tubules may play a role in tick nutrition. Since Malpighian tubules are predominantly involved in osmoregulation and excretion (Sonnenshine and Anderson, 2014), there is a possibility that Coxiella-LE are able to utilize ticks’ nitrogenous metabolites to synthesize compounds such as B vitamins (Buysse et al., 2019). Since vitamin B is essential for cell growth and development and it is involved also in energetic metabolism, we can suppose that there are higher energetic requirements of ovaries and Malpigian tubules than of other organs.
This may be connected to the high frequency of Coxiella-LE, e.g. in the population of Rhipicephalus microplus ticks (Guizzo et al., 2017). However, also a common vertebrate host may serve as Coxiella-LE reservoir for further infection of ticks including Dermacentor and Hyalomma species. Furthermore, almost each tick genus, e. g. Amblyomma, Haemaphysalis, and also Ixodes spp. including I. ricinus were found to be positive for Coxiella-LE (Guizzo et al., 2017; Rahal et al., 2020). However, microbiome analyses revealed that prevalence of the Coxiella-LE is quite low in I. ricinus averaging around 5.5%, and depending on geography, tick stage and sex. Coxiella-LE were detected in 12% of adult females, 4.2% adult males and 18.3% nymphs. This lower prevalence, compared to other tick species that inhabit same locations (such as Dermacentor reticulatus and Heamaphysalis inermis, whose prevalence ranges from 25 to over 50%) (Papa et al., 2017; Špitalská et al., 2018) can be explained by significantly higher prevalence of Candidatus Midichloria mitochondrii in I. ricinus (Sassera et al., 2006). This endosymbiont most likely plays similar nutritional role and is able to outgrow population of either Coxiella-LE or Francisella-LE.
Since tick’s diet consists mainly of blood and vertebrate blood rarely contains sufficient quantities of amino acids and B-vitamin, it needs to be complemented with bacterially synthesized vitamins (Zhong, 2012). Vitamin B is required for blood digestion and is essential for the survival and reproduction of ticks. Coxiella-LE are thought to supply missing vitamin B (Buysse et al., 2019). Biosynthetic pathways for the vitamins Biotin (B7), riboflavin (B2), Pyridoxine (B6), Folic acid (B9) and Pantothenate (B5) were found in all Coxiella-like bacteria (Gottlieb et al., 2015; Guizzo et al., 2017).
2.2 Francisella-like endosymbionts
Francisella is a facultative intracellular Gram-negative Gammaproteobacterium. Within this genus, four valid species, Francisella tularensis, F. philomiragia, F. noatunensis, and F. hispaniensis, have been recognized (Liu et al., 2016). Francisella tularensis is naturally found in vertebrates, invertebrates, contaminated soil, water and vegetation (Mörner, 1992) and causes a zoonotic disease called tularemia (Hightower et al., 2014). Tick vectors of F. tularensis include species of genera Amblyomma, Dermacentor, Haemaphysalis, Ixodes and Ornithodoros (Gordon et al., 1983).
Multiple tick species were found to host bacteria closely related to F. tularensis, called Francisella-like endosymbionts (Francisella-LE) (Dergousoff and Chilton, 2012) that have a worldwide distribution in ixodid ticks, specifically in the genera Ixodes, Dermacentor and Amblyomma (de Carvalho et al., 2011). Špitalská et al. (2018) identified Francisella-LE in I. ricinus from Slovakia what correlated with previous findings of Hornok et al. (2013). Francisella-LE are predominantly localized in Malpighian tubules in clusters, surrounding nuclei. Francisella-LE were also observed in the poles of oocytes and scattered in salivary gland acini, where they appear to surround nuclei (Azagi et al., 2017). Francisella-LE replicate intracellularly and were found in 95 to 100% of eggs of Hyalomma ticks, which confirmed transovarial transmission. The presence of Francisella-LE in salivary glands might suggest a potential nutritional role in the supply of B vitamins that are deficient in the tick’s diet. Francisella-LE were detected in half of the analyzed questing I. ricinus ticks for the first time in Slovakia in 2017 (Azagi et al., 2017; Špitalská et al., 2018) and in I. ricinus larvae from Hungary (Hornok et al., 2013). On average, only 2.3% of analysed individuals of I. ricinus carried Francisella-LE. The prevalence was dependent on sex and stage, with higher being in female adult ticks (6.1%) (Hornok et al., 2013; Wójcik-Fatla et al., 2015; Špitalská et al., 2018). Similar to Coxiella-LE, this low prevalence of Francisella-LE in I. ricinus has most likely the same explanation as mentioned above in section 2.1. So far, there is a lack of knowledge concerning the pathogenic potential of Francisella-LE (Špitalská et al., 2018).
Previously, a microorganism, originally described as Wolbachia-like symbiont, was found in nearly all examined Dermacentor andersoni ticks, and based on the 16S rRNA sequence, it was characterized as an endosymbiont belonging to the genus Francisella with 95.4% identity to pathogenic F. tularensis (Niebylski et al., 1997a).
2.3 Rickettsia endosymbionts
Rickettsia spp. are Gram-negative obligate intracellular bacteria belonging to the class Alphaproteobacteria and include multiple known arthropod-borne human pathogens (Guillotte et al., 2021). Rickettsia spp. can occur both as vertebrate pathogens and arthropod symbionts, have a wide diversity and are classified into at least four groups: ancestral group (AG), typhus group (TG), transitional group (TRG), and spotted fever group (SFG) rickettsiae (Gillespie et al., 2012).
Rickettsiae of undetermined pathogenicity are commonly detected in ticks. Some are labelled as non-pathogenic beneficial endosymbionts and some are considered as potential pathogens (Kurtti et al., 2016). Analysis of the RIES (rickettsial endosymbiont of Ixodes scapularis) genome showed vast disruption of genome caused by mobile genetic elements and acquisition of genes responsible for intracellular parasitism. Over one-third of the REIS genome is made up of mobile genetic elements and transposases (Gillespie et al., 2012; Thorpe et al., 2021). Rickettsia buchneri and Rickettsia peacockii are non-pathogenic rickettsial endosymbionts of I. scapularis and Dermacentor andersoni, respectively, and are spread through transovarial transmission. They are mainly associated with tick ovarian tissue and both endosymbionts are non-infectious for vertebrate cells (Niebylski et al., 1997b; Kurtti et al., 2015). Pathogenic rickettsiae, transmitted by ticks to vertebrate hosts, are closely related to these endosymbionts. Riskettsia peacockii is closely related to R. rickettsii (causative agent of Rocky Mountain spotted fever), and R. buchneri to R. monacensis transmitted by I. ricinus (Simser et al., 2002; Kurtti et al., 2015). Compared to the related pathogens, both endosymbionts possess an extensively rearranged genome with gene mutations attenuating virulence and the capability to cause cytopathic effects (Kurtti et al., 2016). There is insufficient of research aimed towards detection of Rickettsia endosymbionts in I. ricinus. Rickettsia endosymbionts were identified in I. ricinus in Poland with prevalence of 20% (Stańczak et al., 2018), and also in Eastern Slovakia (Špitalská et al., 2020), nonetheless their interactions with ticks are unknown.
2.4 Rickettsiella spp.
Rickettsiella species (class Gammaproteobacteria, order Legionellales, family Coxiellaceae) are obligate intracellular bacteria that are associated with many arthropod species, including ticks. They are maternally inherited and, depending on the species and their arthropod host, they are either pathogenic with negative effects on the development and reproduction of arthropods (Rosenwald et al., 2020), or are essential for their survival, e.g. by supplying B-vitamins (Price et al., 2021). In contrast to insects and other tick species, only a few studies have dealt with presence of Rickettsiella in I. ricinus, while their biological role in this tick species is unknown (Carpi et al., 2011; Duron et al., 2015; Cerutti et al., 2018; Estrada-Peña et al., 2018; Aivelo et al., 2019; Garcia-Vozmediano et al., 2022). Recently, I. ricinus from Belgium, Italy, the Netherlands, Sweden, and the UK were screened for the presence of Rickettsiella spp. and their genetic diversity was studied (Garcia-Vozmediano et al., 2022). Moreover, associations of Rickettsiella spp. with the endosymbiont Midichloria mitochondrii and pathogenic Borrelia burgdorferi s.l. and Borrelia miyamotoi were investigated. Presence of Rickettsiella spp. was confirmed in majority of the studied I. ricinus populations across the studied regions and in all active tick stages. But, prevalence of Rickettsiella spp. was highest in adult ticks and differed between the sites. Four Rickettsiella clades were identified, with various patterns depending on the geographic locations. No associations of Rickettsiella spp. with the other investigated bacteria infecting I. ricinus were found. Based on these results, the authors suggest that Rickettsiella spp. are genetically and biologically diverse facultative symbionts of I. ricinus and environmental factors influence their presence, prevalence and geographic distribution.
2.5 Candidatus Midichloria mitochondrii
Candidatus Midichloria mitochondrii, formerly known as IricES1 (I. ricinus EndoSymbiont 1), is a Gram-negative Alphaproteobacterium, a member of the order Rickettsiales and intracellular endosymbiont of I. ricinus (Comandatore et al., 2021). The bacterium was detected in 100% of female ticks while only 44% of males were infected. Males, if infected, harbor a smaller quantity of bacteria than females. Cand. Midichloria mitochondrii was detected in every egg, suggesting that the major route of transmission is transovarial (Lo et al., 2006). Real-time PCR analysis showed the presence of Cand. Midichloria mitochondrii in every development stage (eggs, larvae, nymphs and adults) of I. ricinus (Sassera et al., 2006).
The bacterium resides in the mitochondria of tick ovaries, namely in the periplasmatic space between two membranes of these organelles. During the development of oocytes, the bacteria consume the inner part of mitochondria and reproduce inside (Sassera et al., 2006). According to the “mitochondrion-to-mitochondrion hypothesis”, the bacterium may be able to move in-between mitochondria, possibly within a mitochondrial network (Comandatore et al., 2021). The number of bacteria was observed to increase during feeding, which suggests a possible role in the metabolism of tick’s blood meal (Sassera et al., 2008). Tick populations maintained in the laboratory after a few generations eventually lost the symbiont. This can indicate that its role is important in natural habitat, e.g. for survival in a cold climate (Lo et al., 2006). Sequencing of Cand. Midichloria mitochondrii genome showed the presence of unique gene sets found in no other Rickettsiales. Among these was a cbb(3)-type cytochrome c oxidase, a haem-copper proton-pumping oxidase with the ability to perform oxidative phosphorylation at low oxygen tension. Due to its high oxygen affinity, this enzyme can allow respiration under microaerobic conditions, thus aiding ATP production when oxygen availability is scarce. This suggests that the bacterium could serve as a source of ATP for the tick under low-oxygen conditions (Sassera et al., 2011). Cand. Midichloria mitochondrii also possess complete metabolic pathways for de novo biosynthesis of vitamin B, which is necessary for tick development and is lacking in its blood meal (Sassera et al., 2011; Al-Khafaji et al., 2019).
In addition to I. ricinus, the distribution of Cand. Midichloria related endosymbionts was found in eight other hard tick species, though the prevalence levels differed (Epis et al., 2013).
2.6 Spiroplasma spp.
Spiroplasma spp. are helical bacteria and belong to the family Spiroplasmataceae, order Mycoplasmatales and the class Mollicutes (Tully et al., 1993). Bacteria of genus Spiroplasma are predominantly found in plants and arthropods. Some Spiroplasma spp. are potential pathogens of vertebrates, but most of them are symbionts (Henning et al., 2006). Only two Spiroplasma spp. were validated in ticks: Spiroplasma mirum and Spiroplasma ixodetis (Ogata et al., 2021). The first reported tick Spiroplasma, S. mirum, was isolated from the tick Haemaphysalis leporispalustris (Tully et al., 1982). Spiroplasma ixodetis was first found in Ixodes pacificus (Tully et al., 1981). The presence of a Spiroplasma strain, closely related to S. ixodetis, was also detected in I. ricinus (Tenckhoff et al., 1994; Henning et al., 2006; Bell-Sakyi et al., 2015)
2.7 Arsenophonus-like symbionts
The genus Arsenophonus is an endosymbiotic group of bacteria, mainly associated with insects (Nováková et al., 2009). The first isolated bacteria from this clade was Arsenophonus nasoniae, a male-killing symbiont of the parasitoid wasp Nasonia vitripennis (Gherna et al., 1991). A strain of A. nasoniae was also identified in ticks of genera Dermacentor and Amblyomma in the USA (Clay et al., 2008; Dergousoff and Chilton, 2012) and in nymphs of I. ricinus in Slovakia. Molecular screening of I. ricinus ticks from the same location identified A. nasoniae presence in 37% of nymphs and only in 3.6% of adults. The pathogenicity of this bacterium for vertebrate hosts is unknown (Clay et al., 2008; Dergousoff and Chilton, 2012; Subramanian et al., 2012). Bohacsova et al. (2016) detected A. nasoniae in adult Ixodiphagus hookeri wasps, which are parasites of I. ricinus nymphs. Arsenophonus nasoniae was not detected in tick nymphs that were not parasitized by wasps. Vertical transmission was also observed in Arsenophonus that was detected in eggs of Amblyomma ticks (Dale et al., 2006). Liu et al. (2013) detected Arsenophonus endosymbiont in multiple organs (ovaries, salivary glands, midgut and Malphigian tubules) of Dermacentor reticulatus ticks. Arsenophonus was present in different developmental stages (eggs, larvae, nymphs and adults), indicating possibility of vertical transmission.
2.8 Wolbachia spp.
Wolbachia are obligate intracellular endosymbiotic Alphaproteobacteria associated with arthropods and nematodes (Landmann, 2019). They are capable of manipulating the host reproductive system through cytoplasmic incompatibility, induction of parthenogenesis (Werren, 1997). The presence of Wolbachia endosymbionts in various hard ticks was reported in multiple studies (Benson et al., 2004; Andreotti et al., 2011; Thapa et al., 2019; Duan et al., 2020), however, it is uncertain if ticks themselves are infected with Wolbachia, or if the detected bacteria originate from parasitic wasps of the genus Ixodiphagus (Hu et al., 1998). The study conducted by Tijsse-Klasen et al. (2011) indicates the latter hypothesis. Presence of I. hookeri was detected in 9.5% of tested I. ricinus. Wolbachia was found in 87% of the ticks that were positive for I. hookeri and only in 1.6% of I. hookeri-free ticks tested positive for Wolbachia. Selective presence of Wolbachia in individual nymphs (Alafaci et al., 2021) correlates with the detection of Wolbachia in all of the analyzed I. ricinus nymphs parasitized by I. hookeri while all unparasitized nymphs were Wolbachia-free (Plantard et al., 2012). From these facts a question rose whether tick cells are capable of supporting the growth of Wolbachia. Khoo et al. (2020) tested in vitro Wolbachia infection susceptibility on cell lines derived from I. ricinus, I. scapularis and Rhipicephalus microplus and found that Wolbachia was able to invade and replicate in all tick cell lines. Zhang et al. (2011) investigated the distribution of Wolbachia in Amblyomma americanum in association witch nematodes. Association between tick parasite infection and Wolbachia prevalence was similar to results of Tijsse-Klasen et al. (2011). Wolbachia endosymbiont was significantly more prevalent in nematode-infected ticks.
Taking into consideration results from above mentioned studies, the lack of strict Wolbachia and tick-parasite co-infection concordance suggests that Wolbachia presence in ticks is not solely due to wasp or nematode infection. Furthermore, detection of Wolbachia endosymbiont in both female and male adult ticks and in nymphs may suggest a possible way of vertical transmission (Zhang et al., 2011; Chao et al., 2021).
Besides the reproduction system, Wolbachia distribution was detected also in various somatic tissues of its host, including the nervous tissue, and it might be able to regulate host behavior and physiology (Albertson et al., 2009; Albertson et al., 2013; Strunov et al., 2013).
Since it is still very difficult to identify the true microbiome it is necessary to focus also on the identification of potentially contaminating microorganisms originating in the environment, tick surface or laboratory reagents (see also Supplementary Table 1).
3 Pathogens
3.1 Viruses
I. ricinus is an important vector of viruses of public health interest (Charrel et al., 2004). Previously, research on the I. ricinus virome has been mainly focused on viruses from the family Flaviviridae that cause disease in humans, namely tick-borne encephalitis virus (TBEV), and Louping ill virus causing disease in domestic ruminants (Gritsun et al., 2003; Charrel et al., 2004; Bartíková et al., 2017). Uukuniemi phlebovirus (Phenuiviridae) (Papa et al., 2018), Tribeč and Lipovník virus (Kemerovo virus complex) (Grešíková, 1972) and Eyach virus (Rehse-Küpper et al., 1976) (Reoviridae), associated with human diseases, and Murid herpesvirus 4 (DNA virus, family) Herpesviridae) (Ficová et al., 2011) were also identified in I. ricinus. Recent metagenomics studies provided deeper insight into the virome composition of I. ricinus from several European countries and previously undetected or new viruses belonging to viral orders and families such as Flaviviridae, Rhabdoviridae, Bunyaviales, Mononegavirales, Nyamiviridae, Nairoviridae, Luteoviridae, Phenuiviridae, Partiviridae, Peribunyaviridae and Reoviridae were identified (Moutailler et al., 2016a; Pettersson et al., 2017; Vanmechelen et al., 2017; Kuivanen et al., 2019; Ohlendorf et al., 2019; Tomazatos et al., 2021; Sameroff et al., 2022) (see also Supplementary Table 2).
The knowledge on interactions between viruses and I. ricinus is limited (Nuttall, 2014; Kazimírová et al., 2017). The most explored and understood interaction is that between I. ricinus and TBEV. For example, Mansfield et al. (2017) observed induction of differential expression of genes responsible for cell survival and resistance in I. ricinus cells infected with TBEV and the Louping ill virus. The presence of these flaviviruses increased the gene expression of apoptosis-associated genes, and immune genes. However, an increase in expression of genes encoding proteins responsible for apoptosis inhibition was also observed, which may be influenced by pathogens to promote infection.
Tick saliva plays an important role in facilitating of TBEV transmission to the vertebrate host (i.e. salivary assisted transmission) (Nuttall and Labuda, 2008). Hart et al. (2020) found differences in the composition of the saliva between uninfected and TBEV-infected I. ricinus females during the early stages of feeding, particularly in the expression of uncategorized genes, proteases, Kunitz-type serine protease inhibitors, cytotoxins, and lipocalins. These changes in the tick sialome are probably significant in enhancing virus transmission to the vertebrate host. In the related species, I. scapularis, micro RNA expression was analyzed in salivary glands of ticks infected with Powassan virus. In virus-infected tick females, 35 salivary gland miRNAs were found to be significantly up-regulated, while 17 miRNAs were significantly down-regulated. Potential role of this difference in miRNA expression could be regulation of Powassan virus replication in host tissues (Hermance et al., 2019).
Laboratory studies also revealed that application of I. ricinus saliva significantly increased TBEV replication in murine dendritic cells in vitro and induced activation of Akt pathway (Lieskovská et al., 2018). This pathway plays an important regulatory role in numerous cellular processes and serves as anti-apoptotic signalling pathway in infected dendritic cells, which may aid TBEV replication and transmission. Another tool to explore virus-tick interactions is the study of protein-protein interactions (PPI). Lemasson et al. (2021) constructed a PPI network of TBEV and Louping ill virus, their hosts and vector, I. ricinus. The viral proteins interacted with numerous host cell proteins, particularly with pathways for cytoskeletal function, transcription, signal transduction and protein degradation. TBEV infection was found to change tick behavior as well in terms of higher mobility and questing activity (Alekseev et al., 1988; Belova et al., 2012).
3.2 Bacteria
I. ricinus is an important vector of a variety of infectious bacteria causing diseases in humans and domestic animals, e.g. Borrelia burgdorferi s. l. (Stanek et al., 2012; Strnad et al., 2017), Borrelia miyamotoi (Wagemakers et al., 2015), Anaplasma phagocytophilum (Strle, 2004; Stuen et al., 2013) Neoehrlichia mikurensis (Portillo et al., 2018), Rickettsia spp. (Oteo and Portillo, 2012; Tomassone et al., 2018), C. burnetii (Rehácek et al., 1991), and Francisella tularensis (Hubálek and Rudolf, 2011). In most cases, these bacteria cause zoonotic diseases and humans are their incidental and dead-end hosts. They are commonly transmitted from ticks to vertebrate hosts through hematophagous bites (Hofhuis et al., 2017). There are multiple ways of bacteria propagation in tick populations - vertical (transovarial) transmission (from females to eggs and the subsequent generation) (Harris et al., 2017; Ogata et al., 2021; Tufts and Diuk-Wasser, 2021) or horizontal transmission (from tick to tick through co-feeding on a host or by feeding on an infected host) (Belli et al., 2017; Moraes-Filho et al., 2018; Nah and Wu, 2021). The vertebrate pathogens may persist for long periods in infected ticks thanks to their ability to be transmitted from one stage to another (transstadial transmission) (Kalmár et al., 2015; Răileanu et al., 2020). Co-infection with multiple pathogens of vertebrates is a common feature observed in ticks. Thus, the possibility of co-transmission of these pathogens to human or animal hosts increases. Also, tick endosymbionts may be capable to interfere with pathogen transmission (Moutailler et al., 2016b; Cutler et al., 2021). According to Špitalská et al. (2018) co-occurrence of tick endosymbionts including Francisella-LE and Coxiella-LE with pathogenic bacteria like Rickettsia spp. and C. burnetii was detected in approximately 50% of field-collected adult D. reticulatus ticks, but only in a few I. ricinus adults and nymphs. However, not only endosymbionts modulate animal pathogen colonization in ticks; also the microbiota composition of individual tick organs can lead to dysbiosis, thus the ifluence of animal pathogens and tick endosymbionts may be bidirectional (Hussain et al., 2022).
Borrelia are helically shaped bacteria from the phylum Spirochaetota, order Spirochaetales. They comprise the B. burgdorferi sensu lato (s.l.) group which includes causative agents of Lyme disease, and the group containing causative agents of tick-borne relapsing infections.
The B. burgdorferi s. l. complex contains currently more than 20 geno-species, and their number is still increasing. Among them, B. burgdorferi sensu stricto (s. s.) in the USA and B. garinii and B. afzelii in Europe and Asia are the most important agents of Lyme borreliosis in humans (Steere et al., 2016; Marques et al., 2021). The dominant vectors of B. burgdorferi are species of the I. ricinus complex - I. ricinus and I. persulcatus in Europe and Asia, and I. scapularis and I. pacificus in North America (Steere et al., 2016).
Upon the entry in the tick midgut during feeding on infected host, B. burgdorferi s. s. cells begin to express the Outer surface protein A (OspA), which facilitates colonization of the tick midgut (Schwan et al., 1995; Pal et al., 2000). Expression of OspA continues in the midgut of moulted unfed ticks and is essential for maintaining the bacteria in the tick body (Pal et al., 2000). OspA is required for mediating attachment of B. burgorferi to the tick midgut by binding to the midgut receptor called Tick Receptor for OspA (TROSPA) (Pal et al., 2004). In the course of tick feeding, the spirochetes downregulate the expression of OspA and upregulate the expression of the Outer surface protein C (OspC). OspC is a lipoprotein that facilitates migration of B. burgdorferi from the gut to salivary glands and plays also an important role in subsequent infection of the vertebrate hosts (Grimm et al., 2004). To survive initial transmission from the tick salivary glands to vertebrate hosts, B. burgdorferi s. s. requires to be coated by tick salivary protein Salp15. This protein interacts with OspC and creates a coating around bacteria. The coating with Salp15 protects B. burgdorferi from vertebrate hosts antibody mediated immunity (Ramamoorthi et al., 2005). For transmission of bacteria from the tick gut to the host, a minimum 6-hour feeding period is required (Strle et al., 1996; Stanek and Kahl, 1999).
Majority of tick-borne borreliae causing relapsing fever are transmitted by Argasidae (soft ticks) (Trevisan et al., 2021). A single causative agent of tick-borne relapsing fever in humans (also called “Borrelia miyamotoi Disease” or BMD), Borrelia miyamotoi, belongs to hard-tick-borne relapsing fever borreliae and is transmitted by Ixodes spp., particularly I. ricinus in Europe, I. pacificus and I. scapularis in Northern America, and I. persulatus in Asia (Wagemakers et al., 2017; Trevisan et al., 2021). Rodents are suggested as natural reservoirs of B. miyamotoi. In contrast to B. burgdorferi s. l., for which horizontal and transstadial transmission are known, for B. miyamotoi transovarial transmission was confirmed (Krause et al., 2015).
C. burnetii belongs to Gammaproteobacteria and is the causative agent of Q fever (see above in Section 2). As an obligate intracellular pathogen of vertebrates, the bacterium replicates inside vacuoles of eukaryotic cells (Samoilis et al., 2010; Steiner et al., 2021). C. burnetii is able to propagate in a great variety of invertebrate and vertebrate hosts and persist for prolonged periods outside of the host (Seshadri et al., 2003). The most common mammal reservoirs for human infections are sheep, cattle and goats. C. burnetii has been isolated from multiple tick species from around the world, including I. ricinus (Hildebrandt et al., 2011; Koka et al., 2018; González et al., 2020; Jiao et al., 2021). Even though C. burnetii is rarely transmitted to humans through tick bites, ticks are also considered among its vectors. These bacteria possess the ability to penetrate the tick’s digestive tract and multiply in the cells of the midgut. Ticks disperse the bacteria through feces contaminating the fur or skin of animals or transmit the bacteria through saliva (Borawski et al., 2020). Transmission to the human host is most commonly achieved through inhalation of contaminated aerosol originating from pets or farm animals.
I. ricinus was found to harbour a novel obligate intracellular gamma-proteobacterium, Diplorickettsia massiliensis (order Legionellales, family Coxiellaceae) that was isolated from questing ticks by using mammalian and amphibian cell lines (Mediannikov et al., 2010) and was found to be pathogenic to humans (Mathew et al., 2012).
Rickettsioses are caused by obligate intercellular Alphaproteobacteria belonging to the genus Rickettsia and are transmitted by various arthropod vectors. Genus Rickettsia is divided into separate groups: spotted fever group rickettsiae, typhus group rickettsiae, Rickettsia canadensis group and Rickettsia belli group (Merhej and Raoult, 2011). tick-borne rickettsioses are caused by rickettsiae of the spotted fever group (SFG) and are distributed worldwide, whereby the distribution area of a specific species coincides with that of its vector. Certain species of SFG rickettsiae are primarily associated with single tick species. The initial infection of ticks with rickettsiae occurs during feeding on infected hosts or by co-feeding with infected ticks (Philip, 1959). The reservoirs are ticks (due to transovarial and transstadial transmission) (Burgdorfer and Brinton, 1975; Podboronov and Pchelkina, 1989; Socolovschi et al., 2009a; Hosseini-Chegeni et al., 2020) and probably small mammals and birds (Elfving et al., 2010; Essbauer et al., 2018; Fischer et al., 2018). The bacteria enter tick gut cells though intracellular digestion of host blood cells. Rickettsiae are able to escape tick immune responses and invade tick hemocytes (Kurtti et al., 2005; Ceraul et al., 2007), from where they subsequently disseminate to all tissues and organs, including salivary glands (Lejal et al., 2019). Rickettsiae also invade tick oocytes (probably during active oogenesis) and ovarian tissues, what leads to transovarial (vertical) transmission (Moore et al., 2018). Transstadial transmission is also essential for survival of Rickettsia spp. in ticks (Kelly and Mason, 1991).
In central Europe I. ricinus is the main vector and also reservoir of R. helvetica (Boretti et al., 2009; Sprong et al., 2009) and R. monacensis (Simser et al., 2002; Socolovschi et al., 2009b). Other Rickettsia spp. such R. bellii-like, R. limoniae-like (Tijsse-Klasen et al., 2011) and flea-borne/lice-borne Rickettsia (R. typhi, R. prowazekii) were also detected in I. ricinus, the latter probably as a result of cross-infection due to sharing rodents as reservoir hosts by ticks and fleas (Sprong et al., 2009). Recently, Rickettsia felis (causative agent of flea-borne spotted fever), strain “Danube” was isolated from a questing I. ricinus nymph in Slovakia (Danchenko et al., 2022).
Anaplasma phagocytophilum (order Rickettsiales, family Anaplasmataceae) is a Gram-negative obligate intracellular bacterium of medical and veterinary importance (Dumler et al., 2001). It invades white blood cells (predominantly neutrophils) and in humans, it causes human granulocytic anaplasmosis (HGA) (Blanco and Oteo, 2002), in ruminants it causes tick-borne fever (Woldehiwet, 1983). The main vectors are I. ricinus in Europe, I. pacificus and I. scapularis in Northern America, and I. persculcatus in Asia (Stuen et al., 2013). The reservoir hosts of the human pathogenic A. phagocytophilum strain in Northern America are mainly rodents. In Europe, the ecology of the bacterium is more complex and involves multiple strains associated with a great variety of vertebrate hosts, but the reservoir(s) of the human pathogenic strains have not been reliably defined (Stuen et al., 2013; Jahfari et al., 2014; Jaarsma et al., 2019). The infection in ticks is maintained through transstadial transmission, with nymphal and adult ticks infecting humans (Stuen et al., 2013).
Neoehrlichia mikurensis (family Anaplasmataceae) is an emerging tick-borne pathogenic bacterium occurring in Europe and Asia. In 1999, it was detected for the first time in I. ricinus from the Netherlands (Schouls et al., 1999) and was isolated in culture by Wass et al. (2019). The bacterium causes febrile disease mainly in immunocompromised human patients (Wennerås, 2015). The main vector of N. mikurensis in Europe is I. ricinus and rodents are suggested as its main reservoirs (Portillo et al., 2018). The bacterium is maintained in tick populations mainly transstadially, but transovarial transmission is suggested as well (Ondruš et al., 2020a).
Chlamydiae DNA was detected in I. ricinus ticks from Switzerland (Pilloux et al., 2015). Subsequently, a novel putative species, “Candidatus Rhabdochlamydia helvetica” was identified (Pillonel et al., 2019), however, it is still not clear if chlamydiae detected in ticks are pathogenic to mammals, tick endosymbionts, or tick parasites.
3.3 Apicomplexa
Babesia species (Piroplasmida, Babesiidae) are intraerythrocytic parasites that cause babesiosis in humans and animals (Yabsley and Shock, 2013). Babesia parasites undergo developmental changes in their vectors and vertebrate hosts: sexual phase (gamogony) in the midgut and asexual proliferation (sporogony) in salivary glands of ticks, and asexual reproduction (schizogony, merogony) in vertebrate erythrocytes (Jalovecka et al., 2018; Jalovecka et al., 2019). Transtadial transmission in Babesia is enabled by dormant sporoblasts present inside salivary gland cells. Transovarial transmission of Babesia in tick females occurs by the invasion of kinetes to the ovaries and is considered as a unique survival strategy in Babesia sensu stricto (Clade X, e.g. B. divergens, B. venatorum, B. canis) (Jalovecka et al., 2018; Jalovecka et al., 2019).
I. ricinus is the main vector of zoonotic Babesia spp. in Europe, namely Babesia divergens, B. microti and B. venatorum (Hildebrandt et al., 2021). Cattle and red deer are natural reservoirs of B. divergens, cervids (primarily roe deer) for B. venatorum and small rodents for B. microti (Yabsley and Shock, 2013). In addition to zoonotic species, Babesia sp. deer clade (B. cf. odocoilei) and B. capreoli causing asymptomatic infections in free ranging cervids have also been detected in I. ricinus (Azagi et al., 2021; Bajer and Dwużnik-Szarek, 2021) (see also Supplementary Table 3).
Dermacentor reticulatus is the main vector of Babesia canis, but the presence of this parasite was also detected in all active stages of questing I. ricinus (Liberska et al., 2021) suggesting transovarial and transstadial transmission of the parasite in I. ricinus populations. Another species associated with canines, particularly with the red fox, is Babesia vulpes. Ixodes hexagonus is probably its main vector, but the species was detected also in I. ricinus (Checa et al., 2018). However, the vector competence of I. ricinus for B. canis and B. vulpes has not been confirmed and needs further investigations (Bajer and Dwużnik-Szarek, 2021).
In spite of extensive information on their epidemiological importance, knowledge of molecular interactions of piroplasmids with their tick vectors, particularly I. ricinus, is limited (Antunes et al., 2017; Jalovecka et al., 2019). A few tick molecules potentially involved in piroplasmid acquisition, propagation and transmission by ticks through their saliva have been determined mainly in studies on Rhipicephalus microplus and Haemaphysalis longicornis (Antunes et al., 2017). They include molecules enabling the parasites to penetrate the tick midgut perithrophic membrane and invade epithelial cells (e.g. TROSPA), molecules involved in regulating Babesia infection (defensins and antimicrobial peptides – longicin, microplusin, longipain, LRR-domain and Kunitz-type protease inhibitors, Bm86, subolesin), invasion of other tick organs such as ovaries (calreticulin, glutamine synthetase, Kunitz-type serine protease inhibitors, vitellogenin receptor) and salivary glands (calreticulin, TROSPA).
Theileria spp. (Piroplasmida, Theileriidae) infect lymphocytes, erythrocytes and other cells of the internal organs of a variety of vertebrate hosts. In contrast to Babesia, they are characterized by schizogony in nucleated blood cells (monocytes, lymphocytes) and subsequent invasion of erythrocytes. In Europe, asymptomatic infections caused by Theileria spp. are known in free-living cervids and caprines, but none of these species has been found to cause zoonotic disease (Yabsley and Shock, 2013). Occurrence of Theileria spp. phylogenetically related with T. capreoli and Theileria sp. OT3 have been reported from I. ricinus feeding on infected cervids (Hamšíková et al., 2016a; Fernández et al., 2022), but I. ricinus is probably not their vector.
Hepatozoon spp. (Eucoccidiorida, Hepatozoidae) are intraerythrocytic parasites of different vertebrates. Hepatozoon canis DNA has been sporadically detected in questing I. ricinus (Hamšíková et al., 2016b) and in I. ricinus removed from dogs (Ciuca et al., 2021). However, the vector role of this tick species for H. canis has not been confirmed.
Toxoplasma gondii (Eucoccidiorida, Sarcocystidae) is an obligate intracellular parasite that causes toxoplasmosis in humans and animals and is capable of infecting all species of warm-blooded animals. Only Felidae are known as definitive hosts of the parasite and produce oocysts in their feces which develop into infectious sporozoites. Oral infection through contaminated food or water is considered the main route of infection. Congenital transmission is also possible. However, due to the wide range of infected hosts, other paths of infections have been suggested, including transmission through blood-feeding arthropods. By examining questing and host feeding I. ricinus ticks in Poland, over 10% of questing ticks were found infected suggesting that ticks may be involved in the spread of toxoplasmosis (Adamska and Skotarczak, 2017).
3.4 Kinetoplastea
Trypanosoma species (Trypanosomatida, Trypanosomatidae) is a group of parasitic flagellate protozoa transmitted by hematophagous insects (dipterans, heteropterans, fleas) causing serious diseases in humans and animals. Presence of trypanosomes has previously been detected also in different species of questing ticks, including I. ricinus (Rehacek et al., 1974). Recently, Trypanosoma sp. Bratislava1, was isolated from questing I. ricinus adults collected in Slovakia in tick cell culture and partially characterised (Luu et al., 2020). This may be a new species related to species detected in ticks in South America and Asia, and to Trypanosoma caninum isolated from dogs in Brazil. However, information about reservoirs, routes of transmission and pathogenicity of the new species are missing.
3.5 Microsporidia
Microsporidia is a large group of obligate, intracellular spore forming eukaryotic parasites. Seventeen species have been associated with human diseases (Han and Weiss, 2017). However, ticks are probably not vectors of zoonotic microsporidia (Trzebny et al., 2022), but they can occasionally be found infected with these parasites. In general, knowledge on microsporidial infections in ticks is limited and only three species have been detected in I. ricinus by microscopic methods: Nosema slovaca and Unikaryon (Nosema) ixodis in former Czechoslovakia by Weiser and Rehácek (1975) and Weiser et al. (1999) and a Nosema-like species in Moldova by Tokarev Iu and Movilé (2004). Recently, metabarcoding revealed low prevalence of microsporidian parasites in questing I. ricinus and in those collected from dogs in Poland. Encephalitozoon intestinalis, a potentially zoonotic species was detected in ticks that fed on dogs and a potentially new Endoreticulatus species in questing ticks (Trzebny et al., 2022). Nosema slovaca was also detected and isolated from D. reticulatus collected in Hungary. Laboratory infection of partially fed D. reticulatus females by the Nosema isolate caused an acute infection and death of the ticks. Thus, microsporidia could be ranked among potential biological control agents of ixodid ticks (Řeháček et al., 1996).
3.6 Fungi
In contrast to insects (Chakraborty et al., 2020; Višňovská et al., 2020; Kim et al., 2021a; Guo et al., 2022), the research on associations between fungi and ticks is limited. The majority of the available studies are focused on entomopathogenic fungi, mainly of the Beauveria and Metarhizium genera, and their prospective use in the biological control of ticks (Kalsbeek et al., 1995; Mitina et al., 2011; Munteanu et al., 2014; Wassermann et al., 2016). Entomopathogenic fungi invade ticks in the soil and leaf litter where development of ticks takes place. Beauveria and Metarhizium species were identified, for example, in adult I. ricinus and D. reticulatus from Poland and majority of the fungal isolates proved to be effective against both tick species (Szczepańska et al., 2020). Other fungi that could potentially be considered as biological control agents are contaminants found in laboratory tick colonies. Three different taxa have been isolated recently from I. ricinus colonies, Penicillium steckii, Aspergillus parasiticus and Scopulariopsis brevicaulis (Bonnet et al., 2021) (Supplementary Table 3).
3.7 Nematodes
I. ricinus ticks in nature were found parasitized by larvae of species belonging to the family Mermithidae (Lipa et al., 1997). I. ricinus is the intermediate host and vector of Cercopithifilaria rugosicauda (Spirurida, Onchocercidae), a subcutaneous filarial parasite of the European roe deer (Winkhardt, 1980). Nematodes from families Steinernematidae and Heterorhabditidae, are acaropahtogens, and are mainly of interest as tick biocontrol agents (Samish and Glazer, 2001; Hartelt et al., 2008) (see also Supplementary Table 3).
4 Microbiome in tick organs
The localization of symbionts in certain organs seems to be associated with two factors – nutritional needs and reproduction. Endosymbionts colonize specific tissues most likely due to nutrient availability and to ensure its survival and transmission. As mentioned earlier, localization of endosymbionts in tick Malpighian tubules is most likely tied to nutrient availability. Ticks’ metabolic waste concentrated in Malpighian tubules can be recycled by its endosymbiotic bacteria that produce necessary nutrients for the ticks, such as B vitamins (Reinhardt et al., 1972; Klyachko et al., 2007; Azagi et al., 2017; Zhang et al., 2022). The majority of endosymbionts can be found in ovarian tissues, including Francisella-LE, Coxiella-LE, Rickettsia endosymbionts, or Cand. Midichloria mitochondrii (Liu et al., 2016; Buysse et al., 2019; Olivieri et al., 2019). Coxiella-LE and Francisella-LE are capable of producing vitamin B2, B6 and B9. Increased requirements for micronutrients and macronutrient after female feeding, with subsequent oogenesis, may demand immediate and locally available source. Ovaries, and subsequently oocytes, represent a means for reproduction and transmission of the endosymbionts. Their prevalence also rapidly increases following blood-feeding (engorgement), and peaks during oogenesis due to the influx of nutrients (Sassera et al., 2008; Zhang et al., 2022). This increase in the proliferation of symbionts in fed females can supply the necessary nutrients and energy for oogenesis (Zhang et al., 2022). The microbiome of salivary glands (SG) is mostly associated with the presence of tick-borne pathogens, such as B. burgdorferi s.l., Rickettsia spp., or A. phagocytophylum. SG along with tick saliva enhance transmission of tick-borne pathogens to the vertebrate host during the tick feeding period (Liu et al., 2011; Lejal et al., 2019; Helble et al., 2021). Since most tick endosymbionts are closely related to tick-borne pathogens, their occurrence in SG can indicate their possible pathogenicity for a vertebrate host. However, only very scarce evidence on the transmission of such bacteria, e.g. Cand. M. mitochondrii from ticks SG to the vertebrate hosts blood (Cafiso et al., 2019; Sgroi, 2022) or presence of antibodies in human sera samples after tick engorgement (Mariconti, 2012) has been found so far. Rickettsia endosymbionts, Coxiella-LE or Francisella-LE are commonly found in SG. They are producents of B vitamins an possibly play a nutritional role in ticks’ diet. Vitamin B2 can be synthetized only by plants or microorganisms and eukyrotes cannot synthetise vitamin B6 at all. Thus, for ticks the only source of essential micronutrients are the bacteria which provide the host with the ability to metabolize macronutrients and produce energy (ATP). Moreover, vitamin B6 is also important for the metabolism of amino acids, neurotransmitters and nucleic acids.
4.1 Digestive system
For blood-feeding arthropods, blood is fundamental source of nutrients and is crucial for development – oogenesis and moulting (Brunner et al., 2011; Valzania et al., 2019). Arthropods with a diet primarily consisting of blood commonly possess a microbiota in their alimentary tract that influences their vector competence for pathogens (Dong et al., 2009; Cirimotich et al., 2011). Introduction of blood into the digestive system commonly leads to the multiplication of microbial community in the midgut of hematophagous arthropods, such as mosquitoes (Oliveira et al., 2011b). Ixodid ticks increase in size 100 to 1000 times during feeding on a vertebrate host due to the immense influx of blood which represents a nutritionally rich substrate and is possibly available also for microbiota present in the tick midgut. In contrast to other hematophagous arthropods whose digestion of blood is an extracellular process, ticks digest blood intracellularly in the functional midgut cells that are able to perform endocytosis (see above). It was also found that, in contrast to other blood-feeding arthropods, blood intake by ixodid ticks is not associated with growth of microbiome in the midgut (Ross et al., 2018; Guizzo et al., 2020). In fact, diversity and quantity of midgut bacteria of I. ricinus decrease after blood meal. There are various hypotheses explaining this phenomenon. According to the first hypothesis, hemoglobin fragments and complement system from host blood may have negative impact on midgut bacteria and are responsible for their decline (Fogaça et al., 1999; Nakajima et al., 2003; Sforça et al., 2005). Another hypothesis says that the tick digestive cells reduce midgut microbiota. During blood digestion, midgut bacteria might be together with blood elements endocytosed and digested by tick digestive cells (Lara et al., 2005). According to the third hypothesis, tick immunity affects the population of bacteria in the tick midgut by antimicrobial peptides and reactive oxygen species (ROS). I. ricinus midgut transcriptome shows that genes associated with immunity, such as lysozyme and defensin, were considerably upregulated during tick feeding (Isogai et al., 2009; Perner et al., 2016).
I. ricinus midgut was found to harbor a fluctuating bacterial population whose compositions differs in individual ticks, depending on the environment and/or infection with pathogens. Detection of bacterial taxa, such as Neisseria, Prevotella and Staphylococcus in midgut of I. ricinus and other tick species indicates that the host skin is the potential source of these bacteria. But most of bacteria identified in the midgut originated from the environment. Wild ticks have higher microbiome diversity compared to ticks raised in laboratory conditions (Dimitriu et al., 2019; Guizzo et al., 2020; Kumar et al., 2022b). Other commonly found bacteria in tick midgut are tick-borne pathogens, such as Borrelia spp. and Rickettsia spp. Borrelia spp. enter the tick mostly during feeding on an infected host, through blood, in early life stages and persist in the midgut throughout the rest of tick’s development, although B. miyamotoi can be transmitted also transovarially. Thanks to evolution of unique metabolic strategies, B. burgdorferi s. l. can persist in thiamine-limited environment of the tick midgut. Moreover, B. burgdorferi s. l. can outcompete other bacteria for limited nutrients and dominate the tick midgut microbiome (Benach et al., 1987; Ross et al., 2018). As no common bacterial genera of tick midgut were found amongst individual ticks, it is possible to conclude that tick midgut does not harbor a stable, core microbiome (Guizzo et al., 2020). However, studies on the microbiome of I. ricinus found large bacterial diversities, an overall unstable microbial composition, and an extremely low bacterial load in their midgut (Aivelo et al., 2019). The observations made by Guizzo et al. (2020) that I. ricinus midgut microbiome has a relatively high diversity but is low in abundance, except for tick-borne pathogens (genus Borrelia) and endosymbionts (Spiroplasma or Rickettsia) are in line with findings of Lejal et al. (2019). Furthermore, it has been shown that the bacterial composition in the I. ricinus is dynamic from temporal as well as geographical point of view. It is influenced also by life stage associated with feeding on different hosts as well as different environment. The majority of these environmentally gained bacteria are relatively quickly eliminated and are unable to colonize the gut (Lejal et al., 2019; Krawczyk et al., 2022).
4.2 Ovaries
Contrasting the midgut, tick ovaries harbor a stable microbial community, mostly of low diversity. Ovaries of I. ricinus are dominantly inhabited by Cand. Midichloria spp. endosymbionts (Sassera et al., 2008; Olivieri et al., 2019; Guizzo et al., 2020). Other dominant ovarian endosymbionts belong to the genus Coxiella. For example, Coxiella spp. endosymbionts in the ovaries of Rhipicephalus microplus were found to represent over 98% of the microbiome population (Andreotti et al., 2011). Rickettsia spp., Francisella spp. and Wolbachia spp. are also common in the ovaries of Ixodes spp., Rhipicephalus spp., Haemaphysalis spp. and Hyalomma spp. (Noda et al., 1997; Kurtti et al., 2015; Azagi et al., 2017).
4.3 Salivary glands
Salivary glands and saliva play an important role in transmission of most tick-borne pathogens to the vertebrate host. It is widely accepted that pathogens, such as B. burgdorferi s. l., mainly reproduce in tick gut and only migrate to SG at the beginning of the blood meal (des Vignes et al., 2001; Sertour et al., 2018) (see also in Section 3 on pathogens). However, Sertour et al. (2018) detected B. burgdorferi s. l. in SG of I. ricinus also prior to blood meal. Persistence of these bacteria in the SG of unfed ticks might suggest that this organ could serve as their potential reservoir (Lejal et al., 2019). In addition to B. burgdorferi s. l., Rickettsia spp., Anaplasma spp. and Neoehrlichia mikurensis were also detected in SG of I. ricinus (Lejal et al., 2019; Ondruš et al., 2020b). For comparison, the endosymbiont R. buchneri was found to colonize SG of I. scapularis (Al-Khafaji et al., 2020) and a Coxiella-like endosymbiont was observed in the granular acini of SG of Amblyomma americanum (Klyachko et al., 2007).
Di Venere et al. (2015) focused on proteomic analysis in ovaries and SG of I. ricinus and its endosymbiont Cand. Midichloria mitochondrii and created proteomic profiles of SG and ovaries. In similar manner, Cotté et al. (2014) investigated expression of proteins in I. ricinus SG in the presence of B. burgdorferi s. l. Presence of 12 of 120 identified proteins was modulated by B. burgdorferi s. l., most of which were upregulated and are involved in protein synthesis and cell defence. Liu et al. (2011) studied P11 protein secreted from I. ricinus SG which is important for migration of A. phagocytophilum from gut to SG.
Kim et al. (2021a) discovered that nymphs of I. scapularis infected with B. burgdorferi s. l. secreted significantly more saliva proteins while suppressing antimicrobial peptides up to 85-fold compared to uninfected ticks. Borrelia burgdorferi s. l. regulated protein composition in tick saliva to retain its survival at the tick feeding site. Saliva proteins that were upregulated in infected ticks most likely play a significant role in transmission and survival of the spirochaetes. An example of upregulated proteins is thioredoxin that neutralizes oxygen peroxide, which is highly toxic for B. burgdorferi, or pyruvate kinase that is required for pyruvate production, which protects the spirochaetes from effects of oxygen peroxide. On the contrary, saliva proteins that were suppressed in B. burgdorferi-infected ticks, e.g., copper/zinc superoxide dismutase that leads to production of oxygen peroxide, are probably harmful or hinder transmission of B. burgdorferi (Kim et al., 2021b).
Anaplasma phagocytophilum infecting SG of I. scapularis facilitates the infections by inhibiting the intrinsic apoptosis pathway. Tick cells are able to limit infections with the extrinsic apoptosis pathway while increasing feeding and survival (Ayllón et al., 2015; Villar et al., 2015; de la Fuente et al., 2016; Cabezas-Cruz et al., 2017).
5 Tick-microbiome relationships
5.1 Interactions of bacteria within the tick microbiome
Compared to other groups of arthropods, ticks host and transmit a greater diversity of pathogens. Majority of tick-borne pathogens are generally considered separately from the rest of the tick microbiome. Nonetheless, as they are able to reproduce within ticks, survive tick moulting and some can be transmitted transovarially, they can be considered members of the tick microbiome (Pollet et al., 2020).
For example, association between Rickettsia spp. and Cand. Midichloria mitochondrii was observed in I. ricinus. Cand. Midichloria mitochondrii abundance was significantly higher in Rickettsia-infected ticks suggesting that Cand. Midichloria facilitates colonisation of I. ricinus by Rickettsia spp. (Lejal et al., 2021). Similar interaction was observed in the tick Amblyomma maculatum, where in both unfed and fed females the quantity of Cand. Midichloria mitochondrii was notably higher in organs (ovaries, midgut and SG) of ticks infected with Rickettsia parkeri than in R. parkeri-free ticks. The same study also showed that in midgut of the females infected with R. parkeri reduced levels of Francisella-like endosymbionts were detected (Budachetri et al., 2018).
There are contradictory reports regarding the impact of Borrelia spp. on the tick microbial community. In earlier reports, I. scapularis females infected with rickettsial endosymbionts had notably smaller rates of B. burgdorferi s. l. infections in comparison to males that were lacking this symbiont (Steiner et al., 2008). Ixodes pacificus nymphs infected with B. burgdorferi s. l. had lower microbiome diversity than uninfected ticks, indicating that pathogen infection might be associated with microbiome diversity (Kwan et al., 2017). In contrast, no substantial differences in microbiome diversity of B. burgdorferi s. l. infected and uninfected I. pacificus ticks were observed by (Swei and Kwan, 2017). This observation was confirmed by Brinkerhoff et al. (2020) and Chauhan et al. (2019), who analyzed I. scapularis ticks infected with B. burgdorferi s. l. and did not find any association between diversity of tick microbiome and infections with B. burgdorferi. Though, these studies showed a connection between presence of certain bacterial taxa and their quantity, and B. burgdorferi (Chauhan et al., 2019; Brinkerhoff et al., 2020). Ticks infected with B. burgdorferi had higher amount of Cutibacterium spp. and lower number of species belonging to Beijerinckiaceae and Diplorickettsiaceae families, and bacteria of genus Rickettsia in their microbiome (Chauhan et al., 2019). It was also demonstrated that presence of bacterial genera such as Tepidomonas, Francisella and Fibriimonas were associated with the presence of B. burgdorferi s. l. (Brinkerhoff et al., 2020).
In I. ricinus, presence of human pathogenic genospecies of B. burgdorferi s. l. was found to shift the abundances of Cand. Midichloria, Rickettsia, Pseudomonas, Staphylococcus, and Cand. Neoehrlichia in the tick microbiome. The geographic location was less important in the tick microbiome composition but shifted the abundances of Pseudomonas and Wolbachia (Hoffmann et al., 2021). Another study has proved that tick larval dysbiosis after surface sterilization of eggs did not affect vector competence of I. ricinus for B. afzelii in the laboratory and the effect of egg surface sterilization on the tick bacterial microbiome disappeared in the moulted nymphs. However, the bacterial microbiome of I. ricinus nymphs that fed as larvae on Borrelia-infected mice was less abundant but more diverse than in nymphs fed on uninfected animals showing that infections in the vertebrate hosts can alter the microbiome of arthropod vectors (Hamilton et al., 2021).
5.2 Microbiota and tick behavior
Thanks to coevolution, ticks and tick-borne pathogens have created an intimate relationship. Physiological changes in pathogen-infected ticks modify Ixodes spp. behaviour in different ways (Benelli, 2020): changing mobility and questing activity (Alekseev et al., 2000; Faulde and Robbins, 2008; Kagemann and Clay, 2013), acquisition of bloodmeal (Dai et al., 2010; Narasimhan et al., 2014), modification of saliva production (Pham et al., 2021), or protection against unfavourable environmental conditions (Lefcort and Durden, 1996; Oliver et al., 2010; Ferrari and Vavre, 2011; Romashchenko et al., 2012).
5.3 Microbiota and tick´s vitamin supplementation
The tick symbiotic microbiome consists of relatively diverse species that form a comprehensive symbiotic system offering a nutritional adaptation for a constrained tick diet. Vertebrate blood is their exclusive source of food regardless of its nutritious imbalance. The tick genome includes genes related to heme and hemoglobin digestions, iron metabolism, osmotic homeostasis and vitamin shortage, but lacks genes for biosynthesis of essential vitamins (Jia et al., 2020; Buysse et al., 2021). The tick microbiome is dominated by intracellular bacterial symbionts that complement this vitamin deficiency (Figure 2). They possess genes for biosynthesis of a fundamental set of B vitamins: B7 (biotin), B2 (riboflavin) and B9 (folate) (Buysse et al., 2021).
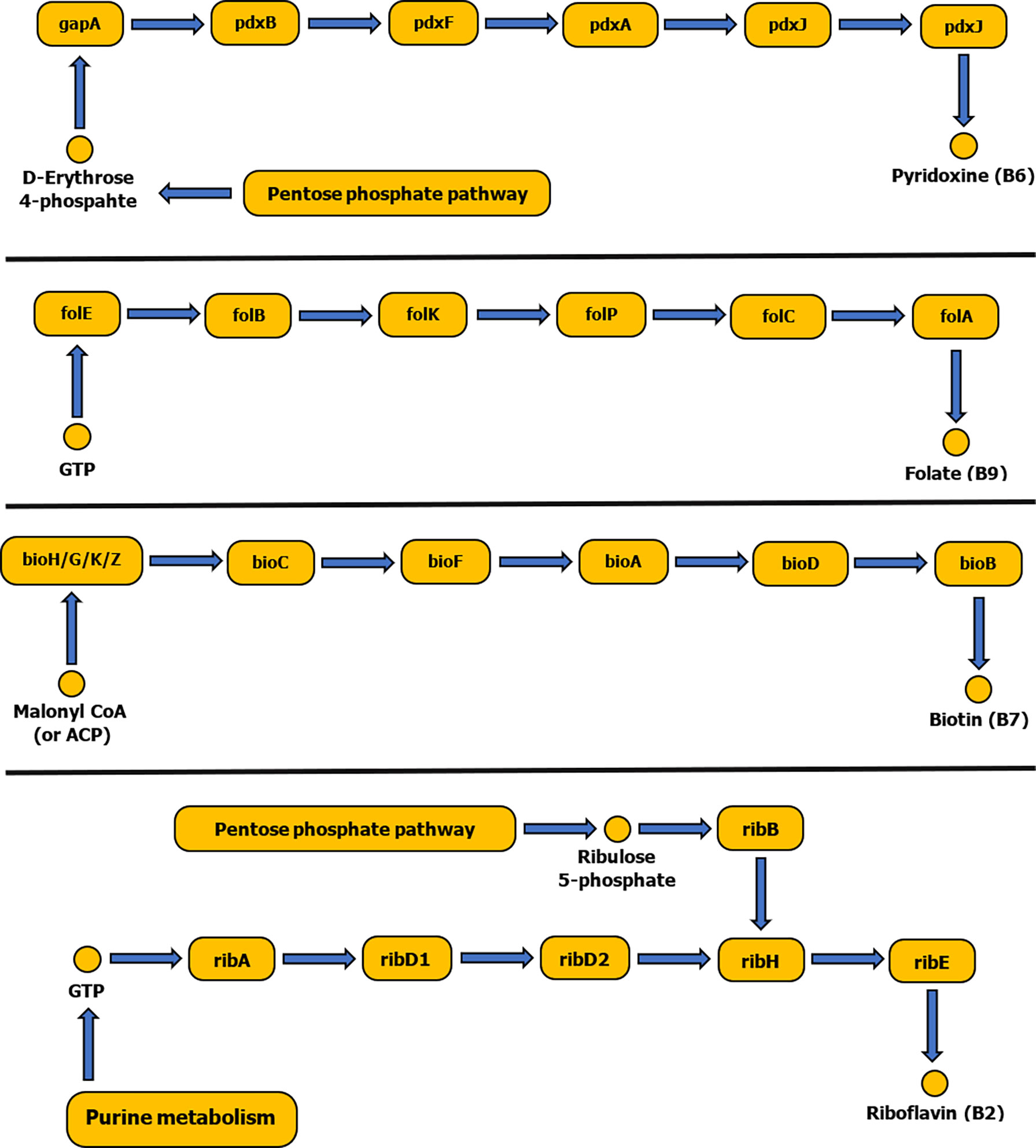
Figure 2 Schematic diagram showing biosynthetic pathways of vitamins B6, B9, B7 and B2. Pathways were constructed based on the KEGG reference database and pathways diagrams available in RAST SEED viewer.
Currently available genomic data of the representatives of tick endosymbionts and selected pathogens from GenBank show that tick-associated endosymbionts of the genus Rickettsia were found to be mainly producers of vitamin B9 (folate) with exception of R. buchneri, which also possesses genes for biosynthesis of vitamin B7 (biotin). Coxiella-like endosymbionts, Francisella-like endosymbionts and A. nasoniae were found to be the most beneficial bacteria in terms of vitamin B production. Both, Coxiella-like and Francisella-like endosymbionts possess complete metabolic pathways for biosynthesis of biotin, folate and vitamin B2 (riboflavin), while A. nasoniae is able to produce vitamin B6 (pyridoxine) (Figures 3, 4).
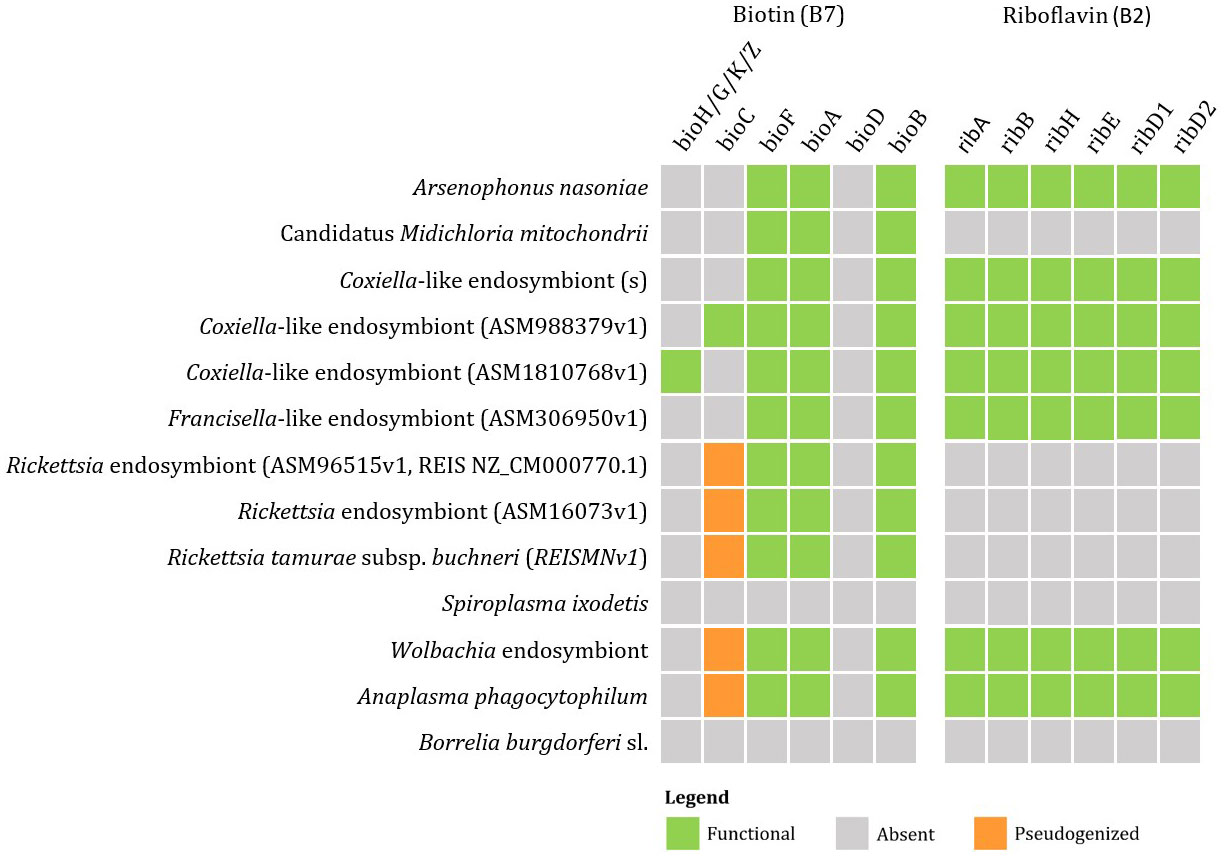
Figure 3 Schematic overview of endosymbiotic bacteria and pathogens transmitted by ticks with focus on gene clusters involved in vitamin B production (B7, B2). Color code: green – detected genes, grey – absent genes, orange – pseudogenized genes.
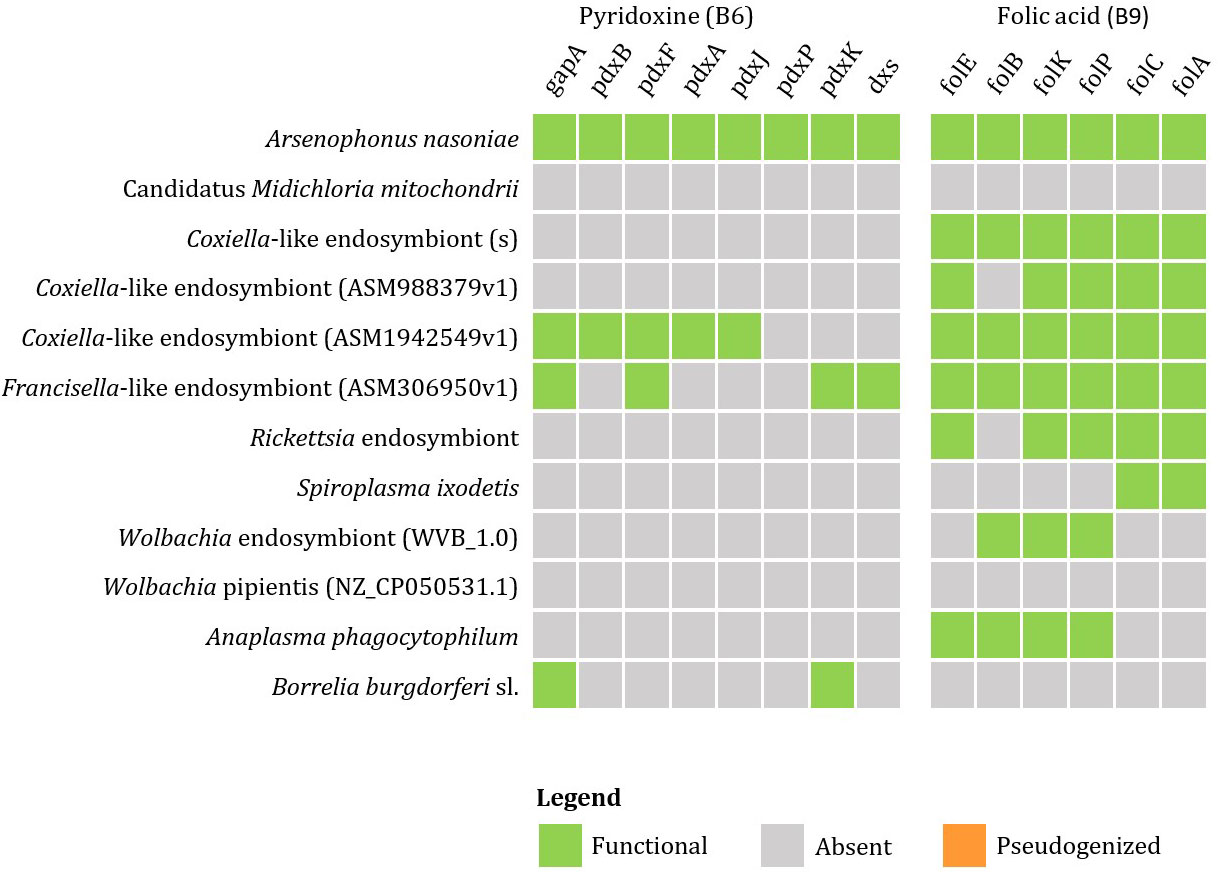
Figure 4 Schematic overview of endosymbiotic bacteria and pathogens transmitted by ticks with focus on gene clusters involved in vitamin B production (B6, B9). Color code: green – detected genes, grey – absent genes, orange – pseudogenized genes).
Wolbachia sp. and A. phagocytophilum were found to possess only the first half of the folate biosynthetic pathway (folB to folP). However, the complexity of the metabolic pathway for folate biosynthesis can be provided by coinfection with S. ixodetis, which possesses the second half of the folate biosynthesis pathway (enzymes folC and DHFR). Anaplasma phagocytophilum also possesses complete pathways for biosynthesis of biotin and riboflavin (Figure 5). No genes for production of vitamin B have been identified in B. burgdorferi s. l. associated with I. ricinus.
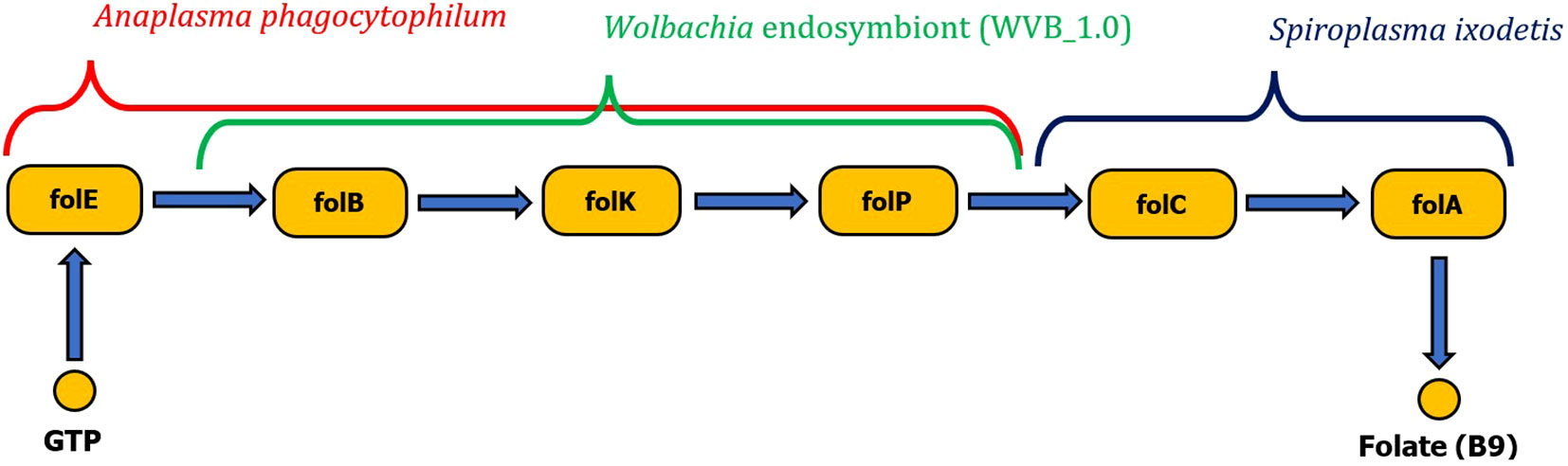
Figure 5 Schematic visualization of complete folate biosynthesis pathway formed by two gene clusters originating from either Wolbachia endosymbiont or Anaplasma phagocytophilum pathogen complementary with the Spiroplasma ixodetis gene cluster (folC, folA).
5.4 Microbiota and energetic metabolism
Whole-genome analysis of Cand. Midichloria mitochondrii confirmed the presence of cbb3 cytochrome oxidase. This cytochrome oxidase belongs to the C-family of the HCO (hem-copper oxidase) superfamily and has been described in proteobacteria (Myllykallio and Liebl, 2000). Compared to A- and B-family HCOs, the cbb3 has a higher affinity for O2 reduction, but is less efficient (Sassera et al., 2011). Oogenesis in ticks has high oxygen requirements (Aboul-Nasr and Bassal, 1972) that result in oxygen concentrations that are suboptimal for mitochondrion ATP synthesis. The presence of cbb3 oxidase, which is expressed only under these microanaerobic conditions, might enable Cand. Midichloria mitochondrii to synthesize ATP. As Cand. Midichloria mitochondrii is localized in ovaries, there is a possibility, that it may serve as a supplementary ATP source for host cells during oogenesis (Sassera et al., 2011). Regarding the possible impact of Cand. Midichloria mitochondrii on eggs development or supplementation of the needs of the eggs during their development has not been investigated so far.
In silico analysis of tick Coxiella-LE revealed the presence of a gene for deoxyhypusine synthase (DHPS). DHPS is required for the activation of eukaryotic initiation factor 5A (elF5A) with hypusine (Wolff et al., 1995). Hypusinated elF5A (elF5AH) affects mitochondrial respiration by promoting the efficient expression of a subset of mitochondrial proteins involved in the TCA cycle and oxidative phosphorylation (Puleston et al., 2019; Liang et al., 2021). Regulation of host mitochondria by Coxiella-LE is already known by mitochondrially-targeted effector proteins (Fielden et al., 2021) and the production of DHPS may serve as another tool for Coxiella spp. to modulate mitochondria function.
5.5 Microbiota and tick´s response to stress
In I. ricinus ticks infected with B. burgdorferi s. l. reduced mobility and higher tendency to stay immobile, rather than to move towards more humid environment was observed, which is more advantageous for keeping tick water balance (Herrmann and Gern, 2012). Infected ticks have increased survival under challenging thermohydrometric conditions. The pathogen is likely to change metabolism or physiology of tick organs that are involved in water regulation (Herrmann and Gern, 2010). It has also been observed that B. burgdorferi s. l.-infected ticks have higher fat reserves (i.e. higher energy reserves) (Herrmann et al., 2013). The spirochetes probably possess the ability to change their gene expression according to temperature and also might be able to influence tick gene expression depending on thermal conditions (Ojaimi et al., 2003). Compared to uninfected I. ricinus ticks that commonly increase their movement during desiccating conditions, infected ticks tend to slow down their metabolism and keep questing longer. This gives the pathogen more time and thus more opportunities to find and infect a vertebrate host (Perret et al., 2003).
Anaplasma phagocytophilum infection impacts tick questing, feeding, survival and can manipulate expression of tick genes (Cabezas-Cruz et al., 2016; Cabezas-Cruz et al., 2017). In contrast to B. burgdorferi s. l., A. phagocytophilum infection was found to increase tick questing speed during challenging thermohydrometric conditions. In A. phagocytophilum-infected I. scapularis ticks, the pathogen induces synthesis of heat shock protein hsp20 and hsp70. These proteins are involved in response to heat stress, thus decreasing chance of desiccation and increasing tick survival rates (Busby et al., 2012). Furthermore, hsp20 with immunogenic properties is similar to myosin-like proteins that have been shown to accumulate after infection. Increase of two myosin subunits has been associated also with infection of R. microplus with Babesia and so seems to be connected with successful pathogen transmission (Kim et al., 2016).
Anaplasma phagocytophilum infecting I. scapularis activates expression of the iafgp gene that is coding the antifreeze glycoprotein IAFGP (Ixodes scapularis AntiFreeze Glycoprotein). This protein serves as biofilm formation inhibitor. IAFGP binds to bacterial peptidoglycan, altering permeability and ability to form biofilm. By upregulating iafgp and thus inhibiting biofilm development and altering gut microbiome, A. phagocytophilum is able more effectively colonise the tick (Abraham et al., 2017). Another function of IAFGP is cold protection. Ixodes scapularis infected with A. phagocytophilum, which induces IAFGP production, have improved cold tolerance and thus increased survival in a cold environment (Neelakanta et al., 2010).
Glycine-rich proteins (GRP) are found in many organisms and their function varies; they are involved in processes from structural to cellular level. In ticks, GRP in saliva possess a fundamental role in formation of tick cement which is also suggested to protect the tick from the host-associated immune factors. Still, there is little evidence that they are involved also in host defense and stress response. Overexpression of GRP can provide the organism with tolerance to stress including abiotic (cold, heat, draught) or biotic factors (microbial homeostasis, hematophagy). Stress stimuli, including oxidative stress, or injury cause significant upregulation of GRPs, however, mechanism of stress mediation is yet unknown (Bullard et al., 2019). Higher expression of GRP can improve the resistance of organism and can even enhance its survival. GRPs can affect the microbial population. Within the SG microbial community of A. americanum involvement of GRP AamerSigP-41539 was established as one of the microbial homeostasis maintenance factors. Some of GRPs, such as Attacins, are immune proteins with antimicrobial activity, but they might switch their role in case of different type of stress conditions, or may serve other functions during molting, fasting or overwintering (Bullard et al., 2019). In Glossina morsitans, Attacins play a role in Trypanosoma resistance, and can maintain homeostasis in infected individuals (Wang et al., 2008). Also, in the study of Flores-Ramirez et al. (2019) three glycine-rich proteins have been identified as a response to infection of D. reticulatus ticks that was experimentally infected with Rickettsia slovaca. Except of glycine-rich proteins, also glycine-proline rich proteins have been detected in ticks as a response to infection. They are associated with ticks’ attachment and feeding on the host, especially they are involved in host´s immune system evasion (Leal et al., 2018). Although the above mentioned proteins are involved in stress responses of other ticks or arthropods than I. ricinus, there is a probability that future studies will reveal at least some of them also in I. ricinus.
5.6 Microbiota and the tick immune system
The immune system of ticks consists of cellular and humoral responses (Sonenshine and Hynes, 2008). Hemocytes, the tick blood cells, are able to recognize, attack and phagocytose microorganisms entering the tick´s body cavity. After proliferation and differentiation, they represent an effective immune system of the tick that can deal with microbiota reaching the hemocoel (Kuhn, 1996). Humoral defence in ticks is secured by a variety of antimicrobial compounds, such as defensins, ixodidin or microplusin (Fogaça et al., 2006; Hajdušek et al., 2013). Phagocytosis of invading microbes by tick hemocytes is most likely facilitated by a complement-like system composed of various lectins, thioesters, protease inhibitors, convertases or iron-binding proteins. Hemocyte-expressed thioester-containing proteins IrTEPs (I. ricinus thioester-containing proteins) were found to mediate phagocytosis of the Gram-negative bacterium Chryseobacterium indologenes and to lesser extent phagocytosis of yeast Candida albicans (Urbanová et al., 2015).
The protease inhibitor of the α2-macroglobulin family from I. ricinus (IrAm) and I. ricinus factor C2/factor B (IrC2/Bf) presence can be found in ticks hemolymph. Inactivation of IrAM significantly reduces the capability of hemocytes to phagocytise C. indologenes, however B. burgorferi was not affected. The specificity of IrAM may be linked to the metalloprotease of C. indologenes (Buresova et al., 2009). In contrast, the presence of Borrelia sp. and C. albicans significantly upregulated the expression if IrC2/Bf. Silencing of IrC2/Bf inhibited phagocytosis of Borrelia and C. albicans by ticks’ hemocytes. IrC2/Bf most likely functions as a convertase in the complement inactivation pathway leading to the elimination of Borrelia and yeast infection (Urbanová et al., 2018). Since iron is an indispensable element for most organisms, iron management is an important component of innate immunity. Hemocyte-produced ferritins of Haemaphysalis longicornis (HlFERs) take part in cellular immune response, most likely through their function of iron-sequestration. Infection of ticks by E. coli resulted in stimulation of HlFERs expression in hemocytes and silencing of HlFERs resulted in a significantly lower survival rate of infected ticks (Galay et al., 2016). Other important immune proteins are fibrinogen related proteins, Ixoderins. Some Ixoderins serve as opsonin’s in the tick hemolymph aiding phagocytosis. Ixoderin A (ixo-A) was found to be expressed in hemocytes and is most likely responsible for the hemagglutination activity of tick hemolymph and facilitates phagocytosis. Silencing of ixo-A expression significantly decreased phagocytosis of Gram-negative bacteria such as E. coli and C. indologenes and yeast C. albicans (Honig Mondekova et al., 2017). Additional essential factors of hemocyte-phagocytosis are hemocyte surface receptors. Class B scavenger receptor CD36 of H. logicornis (HlSRB) is part of first-line host defence and plays a vital role in hemocyte-mediated phagocytosis of exogenous pathogens, such as E. coli. Silencing of HlSRB impeded the ability of hemocytes to phagocytise E. coli, resulting in a significant increase in the mortality of infected ticks (Aung et al., 2012). We can conclude, that tick hemocytes play an integral role in tick immunity and in maintaining microbial homeostasis.
In I. scapularis ticks colonized by B. burgdorferi s. l. increased expression of pixr gene was observed. This gene encodes a gut secreted protein with Reeler domain called PIXR (Protein of I. scapularis with Reeler domain). PIXR most likely takes part in immune response and its function is to limit formation and growth of bacterial biofilm. Disabling pixr gene results in increased biofilm in tick gut and changes in gut microbiome (Narasimhan et al., 2017). PIXR homologues can be also found in I. ricinus (Kotsyfakis et al., 2015). Compared to uninfected nymphs, PIXR levels were increased approximately 1.5-fold in guts of B. burgdorferi infected nymphs. Decreased levels of PIXR led to decline of B. burgdorferi population in tick gut and consequently weakened its ability to infect vertebrate hosts (mice). By increasing expression of pixr, B. burgdorferi escapes tick immune responses that might be enhanced by increased biofilm formation and variations in microbiome composition (Narasimhan et al., 2017).
5.7 Microbiota, blood feeding and reproduction
Tick obligate endosymbionts may play a pivotal role in ticks’ biology by affecting tick’s feeding capability, molting, reproductive fitness or development. In H. longicornis, Coxiella spp. endosymbionts play an important role in the regulation of feeding. The bacteria possess genes for production of chlorismate, a precursor of tryptophan. Coxiella spp. endosymbionts produced chlorismate increases biosynthesis of 5-hydroxytryptamine (serotonin) through stimulation of the expression of aromatic amino acid decarboxylase, which is necessary for catalysis decarboxylation of 5-hydroxytryptophan to serotonin. The elevated levels of serotonin in tick synganglion and midgut promotes tick feeding activity. Treating ticks with glyphosate (inhibitor of chlorismate synthesis pathway) or tetracycline (reduced abundance of Coxiella symbiont) notably reduced tick feeding (Hofer, 2021; Zhong et al., 2021).
Ixodes scapularis produces the gut protein Is86, orthologue of Bm86 of R. microplus or Ir86 of I. ricinus. This protein harbors EGF-like (epidermal growth factor-like) domains and is upregulated during B. burgdorferi s. l. infection. Levels of Is86 in the gut after feeding were 1.8-fold higher in infected ticks compared to controls. Bm86 orthologues are associated with cell growth stimulation and membrane damage restoration and are possibly involved in reshaping of tick gut during feeding. Transmission of B. burgdorferi from ticks feeding on mice immunized with antibodies against Is86 was significantly decreased compared to controls. These antibodies did not impact persistence of spirochetes in the tick, but likely influenced their transmission (Koči et al., 2021).
Antibiotic treatment of tick females may result in prolonged oviposition, reduction of larvae viability and weight compared to untreated ticks. Such impact on reproduction capacity observed e.g., in A. americanum may be explained by contraction of essential nutrients provided by endosymbionts (Zhong et al., 2007; Smith et al., 2015). Similarly, H. longicornis females treated with antibiotics showed a significant reduction of Coxiella endosymbionts in ovaries and Malpighian tubules, which resulted in prolonged feeding and oviposition and reduced oviposition (Zhang et al., 2017).
6 Concluding remarks
The tick microbiome is quite complex, and its composition depends on tick developmental stage and gender, geographical location, and surrounding environment. Majority of the tick microbiome is represented by transient microbial inhabitants, consisting mainly of environmental bacteria, while the number of stable tick microbiome genera is not so high. However, still most of the attention is focused on tick-borne pathogens, since they are important causative agents of human diseases. These pathogenic bacteria evolved intricate mechanisms for suppressing, evading or co-opting tick immune responses, and modifying tick behavior in a way that is beneficial for the host and pathogen. Although, they are classified as pathogens, for the tick itself they represent no danger and can contribute to its physiological maintenance together with ticks´ symbiotic bacteria. However, current knowledge of ticks´ microbiome composition and its intimate involvement in tick´s physiology, behavior and survival is still very poor and more precise microbiota member characterization is urgently needed. The involvement of the plethora of microbes in a cascade of chain reactions with a common functional outcome still needs to be untangled. Evolution of the methods of molecular biology enable more precise cell-level identification, however, there are still big gaps in the understanding of the functional mechanisms and there is high demand for the in vitro studies.
Author contributions
All authors RH, MK, and KS have taken part on writing and manuscript editing and revision. RH has written, KS has planned, designed and written, and MK has designed and written, and all authors critically revised the manuscript and added ideas. All authors approved the final version of the manuscript.
Funding
This work was supported by project VEGA 01/0404/19.
Acknowledgments
Authors would like to acknowledge Marketa Derdakova and Juraj Koci for their support of experimental work associated and tightly connected with writing of this review.
Conflict of interest
The authors declare that the research was conducted in the absence of any commercial or financial relationships that could be construed as a potential conflict of interest.
Publisher’s note
All claims expressed in this article are solely those of the authors and do not necessarily represent those of their affiliated organizations, or those of the publisher, the editors and the reviewers. Any product that may be evaluated in this article, or claim that may be made by its manufacturer, is not guaranteed or endorsed by the publisher.
Supplementary material
The Supplementary Material for this article can be found online at: https://www.frontiersin.org/articles/10.3389/fcimb.2022.990889/full#supplementary-material
References
Abdulsalam, A. M., Saadawi, W. K., Kharwat, H. I., Shaibi, T. (2022). Species diversity of ticks (Acari: Ixodidae) in Tarhuna, Libya. Open Vet. J. 12 (3), 370–374. doi: 10.5455/OVJ.2022.v12.i3.10
Aboul-Nasr, A. E., Bassal, T. T. (1972). Biochemical and physiological studies of certain ticks (Ixodoidea). effect of mating, feeding, and oogenesis on oxygen consumption of Hyalomma (H.) dromedarii Koch (Ixodidae). J. Parasitol. 58 (4), 828–831. doi: 10.2307/3278328
Abraham, N. M., Liu, L., Jutras, B. L., Yadav, A. K., Narasimhan, S., Gopalakrishnan, V., et al. (2017). Pathogen-mediated manipulation of arthropod microbiota to promote infection. Proc. Natl. Acad. Sci. U.S.A. 114 (5), E781–e790. doi: 10.1073/pnas.1613422114
Adamska, M., Skotarczak, B. (2017). Molecular evidence for Toxoplasma gondii in feeding and questing I. ricinus ticks. Ticks Tick Borne Dis. 8 (2), 259–261. doi: 10.1016/j.ttbdis.2016.11.009
Aivelo, T., Norberg, A., Tschirren, B. (2019). Bacterial microbiota composition of I. ricinus ticks: the role of environmental variation, tick characteristics and microbial interactions. PeerJ 7, e8217. doi: 10.7717/peerj.8217
Alafaci, A., Crépin, A., Beaubert, S., Berjeaud, J. M., Delafont, V., Verdon, J. (2021). Exploring the individual bacterial microbiota of questing I. ricinus nymphs. Microorganisms 9 (7), 1526. doi: 10.3390/microorganisms9071526
Albertson, R., Casper-Lindley, C., Cao, J., Tram, U., Sullivan, W. (2009). Symmetric and asymmetric mitotic segregation patterns influence Wolbachia distribution in host somatic tissue. J. Cell Sci. 122 (Pt 24), 4570–4583. doi: 10.1242/jcs.054981
Albertson, R., Tan, V., Leads, R. R., Reyes, M., Sullivan, W., Casper-Lindley, C. (2013). Mapping Wolbachia distributions in the adult Drosophila brain. Cell Microbiol. 15 (9), 1527–1544. doi: 10.1111/cmi.12136
Alekseev, A. N., Burenkova, L. A., Chunikhin, S. P. (1988). Behavioral characteristics of Ixodes persulcatus P. Sch. ticks infected with the tick-borne encephalitis virus. Med. Parazitol. (Mosk.) 2, 71–75.
Alekseev, A. N., Jensen, P. M., Dubinina, H. V., Smirnova, L. A., Makrouchina, N. A., Zharkov, S. D. (2000). Peculiarities of behaviour of taiga (Ixodes persulcatus) and sheep (I. ricinus) ticks (Acarina: Ixodidae) determined by different methods. Folia Parasitol. (Praha) 47 (2), 147–153. doi: 10.14411/fp.2000.029
Al-Khafaji, A. M., Armstrong, S. D., Varotto Boccazzi, I., Gaiarsa, S., Sinha, A., Li, Z., et al. (2020). Rickettsia buchneri, symbiont of the deer tick Ixodes scapularis, can colonise the salivary glands of its host. Ticks Tick Borne Dis. 11 (1), 101299. doi: 10.1016/j.ttbdis.2019.101299
Al-Khafaji, A. M., Clegg, S. R., Pinder, A. C., Luu, L., Hansford, K. M., Seelig, F., et al. (2019). Multi-locus sequence typing of I. ricinus and its symbiont Candidatus Midichloria mitochondrii across Europe reveals evidence of local co-cladogenesis in Scotland. Ticks Tick Borne Dis. 10 (1), 52–62. doi: 10.1016/j.ttbdis.2018.08.016
Almeida, A. P., Marcili, A., Leite, R. C., Nieri-Bastos, F. A., Domingues, L. N., Martins, J. R., et al. (2012). Coxiella symbiont in the tick Ornithodoros rostratus (Acari: Argasidae). Ticks Tick Borne Dis. 3 (4), 203–206. doi: 10.1016/j.ttbdis.2012.02.003
Anderson, J. F., Magnarelli, L. A. (2008). Biology of ticks. Infect. Dis. Clin. North Am. 22 (2), 195–215. doi: 10.1016/j.idc.2007.12.006
Andreotti, R., Pérez de León, A. A., Dowd, S. E., Guerrero, F. D., Bendele, K. G., Scoles, G. A. (2011). Assessment of bacterial diversity in the cattle tick Rhipicephalus (Boophilus) microplus through tag-encoded pyrosequencing. BMC Microbiol. 11 (1), 6. doi: 10.1186/1471-2180-11-6
Angelakis, E., Mediannikov, O., Jos, S. L., Berenger, J. M., Parola, P., Raoult, D. (2016). Candidatus Coxiella massiliensis infection. Emerg. Infect. Dis. 22 (2), 285–288. doi: 10.3201/eid2202.150106
Antunes, S., Rosa, C., Couto, J., Ferrolho, J., Domingos, A. (2017). Deciphering Babesia-vector interactions. Front. Cell Infect. Microbiol. 7, 429. doi: 10.3389/fcimb.2017.00429
Aounallah, H., Fessel, M. R., Goldfeder, M. B., Carvalho, E., Bensaoud, C., Chudzinski-Tavassi, A. M., et al. (2021). rDromaserpin: A novel anti-hemostatic serpin, from the salivary glands of the hard tick Hyalomma dromedarii. Toxins (Basel) 13 (12), 913. doi: 10.3390/toxins13120913
Aung, K. M., Boldbaatar, D., Umemiya-Shirafuji, R., Liao, M., Tsuji, N., Xuenan, X., et al. (2012). HlSRB, a class b scavenger receptor, is key to the granulocyte-mediated microbial phagocytosis in ticks. PLoS One 7 (3), e33504. doi: 10.1371/journal.pone.0033504
Ayllón, N., Villar, M., Galindo, R. C., Kocan, K. M., Šíma, R., López, J. A., et al. (2015). Systems biology of tissue-specific response to Anaplasma phagocytophilum reveals differentiated apoptosis in the tick vector Ixodes scapularis. PLoS Genet. 11 (3), e1005120. doi: 10.1371/journal.pgen.1005120
Azagi, T., Jaarsma, R. I., Docters van Leeuwen, A., Fonville, M., Maas, M., Franssen, F. F. J., et al. (2021). Circulation of Babesia species and their exposure to humans through I. ricinus. Pathogens 10 (4), 386. doi: 10.3390/pathogens10040386
Azagi, T., Klement, E., Perlman, G., Lustig, Y., Mumcuoglu, K. Y., Apanaskevich, D. A., et al. (2017). Francisella-like endosymbionts and Rickettsia species in local and imported Hyalomma ticks. Appl. Environ. Microbiol. 83 (18), e01302-17. doi: 10.1128/aem.01302-17
Bajer, A., Dwużnik-Szarek, D. (2021). The specificity of Babesia-tick vector interactions: recent advances and pitfalls in molecular and field studies. Parasit. Vectors 14 (1), 507. doi: 10.1186/s13071-021-05019-3
Baldridge, G. D., Scoles, G. A., Burkhardt, N. Y., Schloeder, B., Kurtti, T. J., Munderloh, U. G. (2009). Transovarial transmission of Francisella-like endosymbionts and Anaplasma phagocytophilum variants in Dermacentor albipictus (Acari: Ixodidae). J. Med. Entomol. 46 (3), 625–632. doi: 10.1603/033.046.0330
Bartíková, P., Holíková, V., Kazimírová, M., Štibrániová, I. (2017). Tick-borne viruses. Acta Virol. 61 (4), 413–427. doi: 10.4149/av_2017_403
Batool, M., Blazier, J. C., Rogovska, Y. V., Wang, J., Liu, S., Nebogatkin, I. V., et al. (2021). Metagenomic analysis of individually analyzed ticks from Eastern Europe demonstrates regional and sex-dependent differences in the microbiota of I. ricinus. Ticks Tick Borne Dis. 12 (5), 101768. doi: 10.1016/j.ttbdis.2021.101768
Belli, A., Sarr, A., Rais, O., Rego, R. O. M., Voordouw, M. J. (2017). Ticks infected via co-feeding transmission can transmit Lyme borreliosis to vertebrate hosts. Sci. Rep. 7 (1), 5006. doi: 10.1038/s41598-017-05231-1
Bell-Sakyi, L., Palomar, A. M., Kazimirova, M. (2015). Isolation and propagation of a Spiroplasma sp. from Slovakian I. ricinus ticks in Ixodes spp. cell lines. Ticks Tick Borne Dis. 6 (5), 601–606. doi: 10.1016/j.ttbdis.2015.05.002
Belova, O. A., Burenkova, L. A., Karganova, G. G. (2012). Different tick-borne encephalitis virus (TBEV) prevalences in unfed versus partially engorged ixodid ticks–evidence of virus replication and changes in tick behavior. Ticks Tick Borne Dis. 3 (4), 240–246. doi: 10.1016/j.ttbdis.2012.05.005
Benach, J. L., Coleman, J. L., Skinner, R. A., Bosler, E. M. (1987). Adult Ixodes dammini on rabbits: a hypothesis for the development and transmission of Borrelia burgdorferi. J. Infect. Dis. 155 (6), 1300–1306. doi: 10.1093/infdis/155.6.1300
Benelli, G. (2020). Pathogens manipulating tick behavior-through a glass, darkly. Pathogens 9 (8), 664. doi: 10.3390/pathogens9080664
Benson, M. J., Gawronski, J. D., Eveleigh, D. E., Benson, D. R. (2004). Intracellular symbionts and other bacteria associated with deer ticks (Ixodes scapularis) from Nantucket and wellfleet, cape cod, Massachusetts. Appl. Environ. Microbiol. 70 (1), 616–620. doi: 10.1128/aem.70.1.616-620.2004
Ben-Yosef, M., Rot, A., Mahagna, M., Kapri, E., Behar, A., Gottlieb, Y. (2020). Coxiella-like endosymbiont of Rhipicephalus sanguineus is required for physiological processes during ontogeny. Front. Microbiol. 11. doi: 10.3389/fmicb.2020.00493
Blanco, J. R., Oteo, J. A. (2002). Human granulocytic ehrlichiosis in Europe. Clin. Microbiol. Infect. 8 (12), 763–772. doi: 10.1046/j.1469-0691.2002.00557.x
Bohacsova, M., Mediannikov, O., Kazimirova, M., Raoult, D., Sekeyova, Z. (2016). Arsenophonus nasoniae and rickettsiae infection of I. ricinus due to parasitic wasp Ixodiphagus hookeri. PLoS One 11 (2), e0149950. doi: 10.1371/journal.pone.0149950
Bonnet, S. I., Binetruy, F., Hernández-Jarguín, A. M., Duron, O. (2017). The tick microbiome: Why non-pathogenic microorganisms matter in tick biology and pathogen transmission. Front. Cell Infect. Microbiol. 7, 236. doi: 10.3389/fcimb.2017.00236
Bonnet, S. I., Blisnick, T., Al Khoury, C., Guillot, J. (2021). Of fungi and ticks: Morphological and molecular characterization of fungal contaminants of a laboratory-reared I. ricinus colony. Ticks tick-borne Dis. 12 (5), 101732. doi: 10.1016/j.ttbdis.2021.101732
Borawski, K., Dunaj, J., Czupryna, P., Pancewicz, S., Świerzbińska, R., Żebrowska, A., et al. (2020). Assessment of C. burnetii presence after tick bite in north-eastern Poland. Infection 48 (1), 85–90. doi: 10.1007/s15010-019-01355-w
Boretti, F. S., Perreten, A., Meli, M. L., Cattori, V., Willi, B., Wengi, N., et al. (2009). Molecular investigations of Rickettsia helvetica infection in dogs, foxes, humans, and Ixodes ticks. Appl. Environ. Microbiol. 75 (10), 3230–3237. doi: 10.1128/aem.00220-09
Bowman, A. S., Ball, A., Sauer, J. R. (2008). “Tick salivary glands: the physiology of tick water balance and their role in pathogen trafficking and transmission,” in Ticks: Biology, disease and control. Eds. Bowman, A. S., Nuttall, P. A. (Cambridge: Cambridge University Press), 73–91.
Brinkerhoff, R. J., Clark, C., Ocasio, K., Gauthier, D. T., Hynes, W. L. (2020). Factors affecting the microbiome of Ixodes scapularis and Amblyomma americanum. PLoS One 15 (5), e0232398. doi: 10.1371/journal.pone.0232398
Brunner, J. L., Cheney, L., Keesing, F., Killilea, M., Logiudice, K., Previtali, A., et al. (2011). Molting success of Ixodes scapularis varies among individual blood meal hosts and species. J. Med. Entomol. 48 (4), 860–866. doi: 10.1603/me10256
Budachetri, K., Browning, R. E., Adamson, S. W., Dowd, S. E., Chao, C. C., Ching, W. M., et al. (2014). An insight into the microbiome of the Amblyomma maculatum (Acari: Ixodidae). J. Med. Entomol. 51 (1), 119–129. doi: 10.1603/me12223
Budachetri, K., Kumar, D., Crispell, G., Beck, C., Dasch, G., Karim, S. (2018). The tick endosymbiont Candidatus Midichloria mitochondrii and selenoproteins are essential for the growth of Rickettsia parkeri in the gulf coast tick vector. Microbiome 6 (1), 141. doi: 10.1186/s40168-018-0524-2
Bullard, R., Sharma, S. R., Das, P. K., Morgan, S. E., Karim, S. (2019). Repurposing of glycine-rich proteins in abiotic and biotic stresses in the lone-star tick (Amblyomma americanum). Front. Physiol. 10, 744. doi: 10.3389/fphys.2019.00744
Buresova, V., Hajdusek, O., Franta, Z., Sojka, D., Kopacek, P. (2009). IrAM-an alpha2-macroglobulin from the hard tick I. ricinus: characterization and function in phagocytosis of a potential pathogen Chryseobacterium indologenes. Dev. Comp. Immunol. 33 (4), 489–498. doi: 10.1016/j.dci.2008.09.011
Burgdorfer, W., Brinton, L. P. (1975). Mechanisms of transovarial infection of spotted fever Rickettsiae in ticks. Ann. N Y Acad. Sci. 266, 61–72. doi: 10.1111/j.1749-6632.1975.tb35088.x
Busby, A. T., Ayllón, N., Kocan, K. M., Blouin, E. F., de la Fuente, G., Galindo, R. C., et al. (2012). Expression of heat shock proteins and subolesin affects stress responses, Anaplasma phagocytophilum infection and questing behaviour in the tick, Ixodes scapularis. Med. Vet. Entomol. 26 (1), 92–102. doi: 10.1111/j.1365-2915.2011.00973.x
Buysse, M., Floriano, A. M., Gottlieb, Y., Nardi, T., Comandatore, F., Olivieri, E., et al. (2021). A dual endosymbiosis supports nutritional adaptation to hematophagy in the invasive tick Hyalomma marginatum. Elife 10, e72747. doi: 10.7554/eLife.72747
Buysse, M., Plantard, O., McCoy, K. D., Duron, O., Menard, C. (2019). Tissue localization of Coxiella-like endosymbionts in three European tick species through fluorescence in situ hybridization. Ticks Tick Borne Dis. 10 (4), 798–804. doi: 10.1016/j.ttbdis.2019.03.014
Cabezas-Cruz, A., Alberdi, P., Ayllón, N., Valdés, J. J., Pierce, R., Villar, M., et al. (2016). Anaplasma phagocytophilum increases the levels of histone modifying enzymes to inhibit cell apoptosis and facilitate pathogen infection in the tick vector Ixodes scapularis. Epigenetics 11 (4), 303–319. doi: 10.1080/15592294.2016.1163460
Cabezas-Cruz, A., Estrada-Peña, A., Rego, R. O., de la Fuente, J. (2017). Tick-pathogen ensembles: Do molecular interactions lead ecological innovation? Front. Cell Infect. Microbiol. 7, 74. doi: 10.3389/fcimb.2017.00074
Cafiso, A., Sassera, D., Romeo, C., Serra, V., Hervet, C., Bandi, C., et al. (2019). Midichloria mitochondrii, endosymbiont of I. ricinus: evidence for the transmission to the vertebrate host during the tick blood meal. Ticks Tick Borne Dis. 10 (1), 5–12. doi: 10.1016/j.ttbdis.2018.08.008
Carpi, G., Cagnacci, F., Wittekindt, N. E., Zhao, F., Qi, J., Tomsho, L. P., et al. (2011). Metagenomic profile of the bacterial communities associated with I. ricinus ticks. PLoS One 6 (10), e25604. doi: 10.1371/journal.pone.0025604
Ceraul, S. M., Dreher-Lesnick, S. M., Gillespie, J. J., Rahman, M. S., Azad, A. F. (2007). New tick defensin isoform and antimicrobial gene expression in response to Rickettsia montanensis challenge. Infect. Immun. 75 (4), 1973–1983. doi: 10.1128/iai.01815-06
Cerutti, F., Modesto, P., Rizzo, F., Cravero, A., Jurman, I., Costa, S., et al. (2018). The microbiota of hematophagous ectoparasites collected from migratory birds. PLoS One 13 (8), e0202270. doi: 10.1371/journal.pone.0202270
Chakraborty, A., Modlinger, R., Ashraf, M. Z., Synek, J., Schlyter, F., Roy, A. (2020). Core mycobiome and their ecological relevance in the gut of five ips bark beetles (Coleoptera: Curculionidae: Scolytinae). Front. Microbiol. 11, 568853. doi: 10.3389/fmicb.2020.568853
Chao, L. L., Castillo, C. T., Shih, C. M. (2021). Molecular detection and genetic identification of Wolbachia endosymbiont in Rhipicephalus sanguineus (Acari: Ixodidae) ticks of Taiwan. Exp. Appl. Acarol. 83 (1), 115–130. doi: 10.1007/s10493-020-00574-3
Charrel, R. N., Attoui, H., Butenko, A. M., Clegg, J. C., Deubel, V., Frolova, T. V., et al. (2004). Tick-borne virus diseases of human interest in Europe. Clin. Microbiol. Infect. 10 (12), 1040–1055. doi: 10.1111/j.1469-0691.2004.01022.x
Chauhan, G., McClure, J., Hekman, J., Marsh, P. W., Bailey, J. A., Daniels, R. F., et al. (2019). Combining citizen science and genomics to investigate tick, pathogen, and commensal microbiome at single-tick resolution. Front. Genet. 10, 1322. doi: 10.3389/fgene.2019.01322
Checa, R., López-Beceiro, A. M., Montoya, A., Barrera, J. P., Ortega, N., Gálvez, R., et al. (2018). Babesia microti-like piroplasm (syn. Babesia vulpes) infection in red foxes (Vulpes vulpes) in NW Spain (Galicia) and its relationship with Ixodes hexagonus. Vet. Parasitol. 252, 22–28. doi: 10.1016/j.vetpar.2018.01.011
Cirimotich, C. M., Dong, Y., Clayton, A. M., Sandiford, S. L., Souza-Neto, J. A., Mulenga, M., et al. (2011). Natural microbe-mediated refractoriness to plasmodium infection in Anopheles gambiae. Science 332 (6031), 855–858. doi: 10.1126/science.1201618
Ciuca, L., Martinescu, G., Miron, L. D., Roman, C., Acatrinei, D., Cringoli, G., et al. (2021). Occurrence of Babesia species and co-infection with Hepatozoon canis in symptomatic dogs and in their ticks in Eastern Romania. Pathogens 10 (10), 1339. doi: 10.3390/pathogens10101339
Clay, K., Klyachko, O., Grindle, N., Civitello, D., Oleske, D., Fuqua, C. (2008). Microbial communities and interactions in the lone star tick, Amblyomma americanum. Mol. Ecol. 17 (19), 4371–4381. doi: 10.1111/j.1365-294x.2008.03914.x
Comandatore, F., Radaelli, G., Montante, S., Sacchi, L., Clementi, E., Epis, S., et al. (2021). Modeling the life cycle of the intramitochondrial bacterium "Candidatus Midichloria mitochondrii" using electron microscopy data. mBio 12 (3), e0057421. doi: 10.1128/mBio.00574-21
Cotté, V., Sabatier, L., Schnell, G., Carmi-Leroy, A., Rousselle, J. C., Arsène-Ploetze, F., et al. (2014). Differential expression of I. ricinus salivary gland proteins in the presence of the Borrelia burgdorferi sensu lato complex. J. Proteomics 96, 29–43. doi: 10.1016/j.jprot.2013.10.033
Cutler, S. J., Vayssier-Taussat, M., Estrada-Peña, A., Potkonjak, A., Mihalca, A. D., Zeller, H. (2021). Tick-borne diseases and co-infection: Current considerations. Ticks tick-borne Dis. 12 (1), 101607. doi: 10.1016/j.ttbdis.2020.101607
Dai, J., Narasimhan, S., Zhang, L., Liu, L., Wang, P., Fikrig, E. (2010). Tick histamine release factor is critical for Ixodes scapularis engorgement and transmission of the lyme disease agent. PLoS Pathog. 6 (11), e1001205. doi: 10.1371/journal.ppat.1001205
Dale, C., Beeton, M., Harbison, C., Jones, T., Pontes, M. (2006). Isolation, pure culture, and characterization of "Candidatus Arsenophonus arthropodicus," an intracellular secondary endosymbiont from the hippoboscid louse fly Pseudolynchia canariensis. Appl. Environ. Microbiol. 72 (4), 2997–3004. doi: 10.1128/aem.72.4.2997-3004.2006
Danchenko, M., Benada, O., Škultéty, Ľ., Sekeyová, Z. (2022). Culture isolate of Rickettsia felis from a tick. Int. J. Environ. Res. Publ. Health 19 (7), 4321. doi: 10.3390/ijerph19074321
Dantas-Torres, F., Braz, A., Sales, K., Sousa-Paula, L. C., Diniz, G. T. N., Correia, J. M. S. (2021). Tick infestation on birds in an urban Atlantic forest fragment in north-eastern Brazil. Exp. Appl. Acarol. 85 (2-4), 305–318. doi: 10.1007/s10493-021-00660-0
de Carvalho, I. L., Santos, N., Soares, T., Zé-Zé, L., Núncio, M. S. (2011). Francisella-like endosymbiont in Dermacentor reticulatus collected in Portugal. Vector Borne Zoonot. Dis. 11 (2), 185–188. doi: 10.1089/vbz.2010.0014
de la Fuente, J., Villar, M., Cabezas-Cruz, A., Estrada-Peña, A., Ayllón, N., Alberdi, P. (2016). Tick-host-pathogen interactions: Conflict and cooperation. PLoS Pathog. 12 (4), e1005488. doi: 10.1371/journal.ppat.1005488
Dergousoff, S. J., Chilton, N. B. (2010). Detection of a new Arsenophonus-type bacterium in Canadian populations of the rocky mountain wood tick, Dermacentor andersoni. Exp. Appl. Acarol. 52 (1), 85–91. doi: 10.1007/s10493-010-9340-5
Dergousoff, S. J., Chilton, N. B. (2012). Association of different genetic types of Francisella-like organisms with the rocky mountain wood tick (Dermacentor andersoni) and the American dog tick (Dermacentor variabilis) in localities near their northern distributional limits. Appl. Environ. Microbiol. 78 (4), 965–971. doi: 10.1128/aem.05762-11
des Vignes, F., Piesman, J., Heffernan, R., Schulze, T. L., Stafford, K. C., 3rd, Fish, D. (2001). Effect of tick removal on transmission of Borrelia burgdorferi and Ehrlichia phagocytophila by Ixodes scapularis nymphs. J. Infect. Dis. 183 (5), 773–778. doi: 10.1086/318818
Dimitriu, P. A., Iker, B., Malik, K., Leung, H., Mohn, W. W., Hillebrand, G. G. (2019). New insights into the intrinsic and extrinsic factors that shape the human skin microbiome. mBio 10 (4), e00839-00819. doi: 10.1128/mBio.00839-19
Di Venere, M., Fumagalli, M., Cafiso, A., De Marco, L., Epis, S., Plantard, O., et al. (2015). I. ricinus and its endosymbiont Midichloria mitochondrii: A comparative proteomic analysis of salivary glands and ovaries. PLoS One 10 (9), e0138842. doi: 10.1371/journal.pone.0138842
Dong, Y., Manfredini, F., Dimopoulos, G. (2009). Implication of the mosquito midgut microbiota in the defense against malaria parasites. PLoS Pathog. 5 (5), e1000423. doi: 10.1371/journal.ppat.1000423
Duan, X. Z., Sun, J. T., Wang, L. T., Shu, X. H., Guo, Y., Keiichiro, M., et al. (2020). Recent infection by Wolbachia alters microbial communities in wild Laodelphax striatellus populations. Microbiome 8 (1), 104. doi: 10.1186/s40168-020-00878-x
Dumler, J. S., Barbet, A. F., Bekker, C. P., Dasch, G. A., Palmer, G. H., Ray, S. C., et al. (2001). Reorganization of genera in the families Rickettsiaceae and Anaplasmataceae in the order Rickettsiales: unification of some species of Ehrlichia with Anaplasma, Cowdria with Ehrlichia and Ehrlichia with Neorickettsia, descriptions of six new species combinations and designation of Ehrlichia equi and 'HGE agent' as subjective synonyms of Ehrlichia phagocytophila. Int. J. Syst. Evol. Microbiol. 51 (Pt 6), 2145–2165. doi: 10.1099/00207713-51-6-2145
Dunn, A. B., Jordan, S., Baker, B. J., Carlson, N. S. (2017). The maternal infant microbiome: Considerations for labor and birth. MCN Am. J. Matern Child Nurs. 42 (6), 318–325. doi: 10.1097/nmc.0000000000000373
Duron, O., Binetruy, F., Noël, V., Cremaschi, J., McCoy, K. D., Arnathau, C., et al. (2017). Evolutionary changes in symbiont community structure in ticks. Mol. Ecol. 26 (11), 2905–2921. doi: 10.1111/mec.14094
Duron, O., Morel, O., Noël, V., Buysse, M., Binetruy, F., Lancelot, R., et al. (2018). Tick-bacteria mutualism depends on B vitamin synthesis pathways. Curr. Biol. 28 (12), 1896–1902.e1895. doi: 10.1016/j.cub.2018.04.038
Duron, O., Noël, V., McCoy, K. D., Bonazzi, M., Sidi-Boumedine, K., Morel, O., et al. (2015). The recent evolution of a maternally-inherited endosymbiont of ticks led to the emergence of the Q fever pathogen, C. burnetii. PLoS Pathog. 11 (5), e1004892. doi: 10.1371/journal.ppat.1004892
Elfving, K., Olsen, B., Bergström, S., Waldenström, J., Lundkvist, A., Sjöstedt, A., et al. (2010). Dissemination of spotted fever Rickettsia agents in Europe by migrating birds. PLoS One 5 (1), e8572. doi: 10.1371/journal.pone.0008572
Epis, S., Mandrioli, M., Genchi, M., Montagna, M., Sacchi, L., Pistone, D., et al. (2013). Localization of the bacterial symbiont Candidatus Midichloria mitochondrii within the hard tick I. ricinus by whole-mount FISH staining. Ticks Tick Borne Dis. 4 (1-2), 39–45. doi: 10.1016/j.ttbdis.2012.06.005
Essbauer, S., Hofmann, M., Kleinemeier, C., Wölfel, S., Matthee, S. (2018). Rickettsia diversity in southern Africa: A small mammal perspective. Ticks Tick Borne Dis. 9 (2), 288–301. doi: 10.1016/j.ttbdis.2017.11.002
Estrada-Peña, A., Cabezas-Cruz, A., Pollet, T., Vayssier-Taussat, M., Cosson, J. F. (2018). High throughput sequencing and network analysis disentangle the microbial communities of ticks and hosts within and between ecosystems. Front. Cell Infect. Microbiol. 8, 236. doi: 10.3389/fcimb.2018.00236
Faulde, M. K., Robbins, R. G. (2008). Tick infestation risk and Borrelia burgdorferi s.l. infection-induced increase in host-finding efficacy of female I. ricinus under natural conditions. Exp. Appl. Acarol. 44 (2), 137–145. doi: 10.1007/s10493-008-9131-4
Fernández, N., Revuelta, B., Aguilar, I., Soares, J. F., Zintl, A., Gray, J., et al. (2022). Babesia and Theileria identification in adult ixodid ticks from Tapada nature reserve, Portugal. Pathogens 11 (2), 222. doi: 10.3390/pathogens11020222
Ferrari, J., Vavre, F. (2011). Bacterial symbionts in insects or the story of communities affecting communities. Philos. Trans. R Soc. Lond B Biol. Sci. 366 (1569), 1389–1400. doi: 10.1098/rstb.2010.0226
Ficová, M., Betáková, T., Pančík, P., Václav, R., Prokop, P., Halásová, Z., et al. (2011). Molecular detection of murine herpesvirus 68 in ticks feeding on free-living reptiles. Microb. Ecol. 62 (4), 862–867. doi: 10.1007/s00248-011-9907-7
Fielden, L. F., Scott, N. E., Palmer, C. S., Khoo, C. A., Newton, H. J., Stojanovski, D. (2021). Proteomic identification of C. burnetii effector proteins targeted to the host cell mitochondria during infection. Mol. Cell Proteomics 20, 100005. doi: 10.1074/mcp.RA120.002370
Fischer, S., Spierling, N. G., Heuser, E., Kling, C., Schmidt, S., Rosenfeld, U. M., et al. (2018). High prevalence of Rickettsia helvetica in wild small mammal populations in Germany. Ticks Tick Borne Dis. 9 (3), 500–505. doi: 10.1016/j.ttbdis.2018.01.009
Flores-Ramirez, G., Sallay, B., Danchenko, M., Lakhneko, O., Špitalská, E., Skultety, L. (2019). Comparative proteomics of the vector Dermacentor reticulatus revealed differentially regulated proteins associated with pathogen transmission in response to laboratory infection with Rickettsia slovaca. Parasit. Vectors 12 (1), 318. doi: 10.1186/s13071-019-3564-y
Fogaça, A. C., Almeida, I. C., Eberlin, M. N., Tanaka, A. S., Bulet, P., Daffre, S. (2006). Ixodidin, a novel antimicrobial peptide from the hemocytes of the cattle tick Boophilus microplus with inhibitory activity against serine proteinases. Peptides 27 (4), 667–674. doi: 10.1016/j.peptides.2005.07.013
Fogaça, A. C., da Silva, P. I., Jr., Miranda, M. T., Bianchi, A. G., Miranda, A., Ribolla, P. E., et al. (1999). Antimicrobial activity of a bovine hemoglobin fragment in the tick Boophilus microplus. J. Biol. Chem. 274 (36), 25330–25334. doi: 10.1074/jbc.274.36.25330
Foughali, A. A., Jedidi, M., Dhibi, M., Mhadhbi, M., Sassi, L., Berber, A., et al. (2021). Infection by haemopathogens and tick infestation of sheep during summer season in Constantine region, northeast Algeria. Vet. Med. Sci. 7 (5), 1769–1777. doi: 10.1002/vms3.551
Galay, R. L., Takechi, R., Umemiya-Shirafuji, R., Talactac, M. R., Maeda, H., Kusakisako, K., et al. (2016). Impaired cellular immune response to injected bacteria after knockdown of ferritin genes in the hard tick Haemaphysalis longicornis. Parasitol. Int. 65 (3), 251–257. doi: 10.1016/j.parint.2016.01.007
Garcia-Vozmediano, A., Tomassone, L., Fonville, M., Bertolotti, L., Heylen, D., Fabri, N. D., et al. (2022). The genetic diversity of Rickettsiella symbionts in I. ricinus throughout Europe. Microb. Ecol. 84 (2), 613–626. doi: 10.1007/s00248-021-01869-7
Gherna, R. L., Werren, J. H., Weisburg, W., Cote, R., Woese, C. R., Mandelco, L., et al. (1991). NOTES: Arsenophonus nasoniae gen. nov., sp. nov., the causative agent of the son-killer trait in the parasitic wasp Nasonia vitripennis. Int. J. Syst. Evol. Microbiol. 41 (4), 563–565. doi: 10.1099/00207713-41-4-563
Gillespie, J. J., Joardar, V., Williams, K. P., Driscoll, T., Hostetler, J. B., Nordberg, E., et al. (2012). A Rickettsia genome overrun by mobile genetic elements provides insight into the acquisition of genes characteristic of an obligate intracellular lifestyle. J. Bacteriol. 194 (2), 376–394. doi: 10.1128/jb.06244-11
González, J., González, M. G., Valcárcel, F., Sánchez, M., Martín-Hernández, R., Tercero, J. M., et al. (2020). Prevalence of C. burnetii (Legionellales: Coxiellaceae) infection among wildlife species and the tick Hyalomma lusitanicum (Acari: Ixodidae) in a meso-Mediterranean ecosystem. J. Med. Entomol. 57 (2), 551–556. doi: 10.1093/jme/tjz169
Gordon, J. R., McLaughlin, B. G., Nitiuthai, S. (1983). Tularaemia transmitted by ticks (Dermacentor andersoni) in Saskatchewan. Can. J. Comp. Med. 47 (4), 408–411.
Gottlieb, Y., Lalzar, I., Klasson, L. (2015). Distinctive genome reduction rates revealed by genomic analyses of two Coxiella-like endosymbionts in ticks. Genome Biol. Evol. 7 (6), 1779–1796. doi: 10.1093/gbe/evv108
Gray, J., Kahl, O., Zintl, A. (2021). What do we still need to know about I. ricinus? Ticks tick-borne Dis. 12 (3), 101682. doi: 10.1016/j.ttbdis.2021.101682
Greay, T. L., Gofton, A. W., Paparini, A., Ryan, U. M., Oskam, C. L., Irwin, P. J. (2018). Recent insights into the tick microbiome gained through next-generation sequencing. Parasit. Vectors 11 (1), 12. doi: 10.1186/s13071-017-2550-5
Grešíková, M. (1972). Studies on tick-borne arboviruses isolated in central Europe. Biologické Práce 18 (2), 1–116.
Grimm, D., Tilly, K., Byram, R., Stewart, P. E., Krum, J. G., Bueschel, D. M., et al. (2004). Outer-surface protein C of the Lyme disease spirochete: a protein induced in ticks for infection of mammals. Proc. Natl. Acad. Sci. U.S.A. 101 (9), 3142–3147. doi: 10.1073/pnas.0306845101
Gritsun, T. S., Lashkevich, V. A., Gould, E. A. (2003). Tick-borne encephalitis. Antiviral Res. 57 (1-2), 129–146. doi: 10.1016/s0166-3542(02)00206-1
Guglielmone, A. A., Robbins, R. G., Apanaskevich, D. A., Petney, T. N., Estrada-Peña, A., Horák, I. G., et al. (2010). The Argasidae, Ixodidae and Nuttalliellidae (Acari: Ixodida) of the world: a list of valid species names. Zootaxa 2528 (1). doi: 10.11646/zootaxa.2528.1.1
Guillotte, M. L., Chandler, C. E., Verhoeve, V. I., Gillespie, J. J., Driscoll, T. P., Rahman, M. S., et al. (2021). Lipid a structural divergence in Rickettsia pathogens. mSphere 6 (3), e00184–21. doi: 10.1128/mSphere.00184-21
Guizzo, M. G., Dolezelikova, K., Neupane, S., Frantova, H., Hrbatova, A., Pafco, B., et al. (2022). Characterization and manipulation of the bacterial community in the midgut of I. ricinus. Parasit. Vectors 15 (1), 248. doi: 10.1186/s13071-022-05362-z
Guizzo, M. G., Neupane, S., Kucera, M., Perner, J., Frantová, H., da Silva Vaz, I., et al. (2020). Poor unstable midgut microbiome of hard ticks contrasts with abundant and stable monospecific microbiome in ovaries. Front. Cell Infect. Microbiol. 10, 211. doi: 10.3389/fcimb.2020.00211
Guizzo, M. G., Parizi, L. F., Nunes, R. D., Schama, R., Albano, R. M., Tirloni, L., et al. (2017). A Coxiella mutualist symbiont is essential to the development of Rhipicephalus microplus. Sci. Rep. 7 (1), 17554. doi: 10.1038/s41598-017-17309-x
Guo, Q., Yao, Z., Cai, Z., Bai, S., Zhang, H. (2022). Gut fungal community and its probiotic effect on Bactrocera dorsalis. Insect Sci. 29 (4), 1145–1158. doi: 10.1111/1744-7917.12986
Gurfield, N., Grewal, S., Cua, L. S., Torres, P. J., Kelley, S. T. (2017). Endosymbiont interference and microbial diversity of the pacific coast tick, Dermacentor occidentalis, in San Diego county, California. PeerJ 5, e3202. doi: 10.7717/peerj.3202
Hajdušek, O., Šíma, R., Ayllón, N., Jalovecká, M., Perner, J., de la Fuente, J., et al. (2013). Interaction of the tick immune system with transmitted pathogens. Front. Cell Infect. Microbiol. 3, 26. doi: 10.3389/fcimb.2013.00026
Hamilton, P. T., Maluenda, E., Sarr, A., Belli, A., Hurry, G., Duron, O., et al. (2021). Borrelia afzelii infection in the rodent host has dramatic effects on the bacterial microbiome of I. ricinus ticks. Appl. Environ. Microbiol. 87 (18), e0064121. doi: 10.1128/aem.00641-21
Hamšíková, Z., Kazimírová, M., Haruštiaková, D., Mahríková, L., Slovák, M., Berthová, L., et al. (2016a). Babesia spp. in ticks and wildlife in different habitat types of Slovakia. Parasit. Vectors 9 (1), 292. doi: 10.1186/s13071-016-1560-z
Hamšíková, Z., Silaghi, C., Rudolf, I., Venclíková, K., Mahríková, L., Slovák, M., et al. (2016b). Molecular detection and phylogenetic analysis of Hepatozoon spp. in questing I. ricinus ticks and rodents from Slovakia and Czech Republic. Parasitol. Res. 115 (10), 3897–3904. doi: 10.1007/s00436-016-5156-5
Hansen, A. K., Moran, N. A. (2011). Aphid genome expression reveals host-symbiont cooperation in the production of amino acids. Proc. Natl. Acad. Sci. U.S.A. 108 (7), 2849–2854. doi: 10.1073/pnas.1013465108
Han, B., Weiss, L. M. (2017). Microsporidia: Obligate intracellular pathogens within the fungal kingdom. Microbiol. Spectr. 5 (2), 5.2.03. doi: 10.1128/microbiolspec.FUNK-0018-2016
Harris, E. K., Verhoeve, V. I., Banajee, K. H., Macaluso, J. A., Azad, A. F., Macaluso, K. R. (2017). Comparative vertical transmission of Rickettsia by Dermacentor variabilis and Amblyomma maculatum. Ticks Tick Borne Dis. 8 (4), 598–604. doi: 10.1016/j.ttbdis.2017.04.003
Hartelt, K., Wurst, E., Collatz, J., Zimmermann, G., Kleespies, R., Oehme, R., et al. (2008). Biological control of the tick I. ricinus with entomopathogenic fungi and nematodes: Preliminary results from laboratory experiments. Int. J. Med. Microbiol. IJMM 298, 314–320. doi: 10.1016/j.ijmm.2007.10.003
Hart, C. E., Ribeiro, J. M., Kazimirova, M., Thangamani, S. (2020). Tick-borne encephalitis virus infection alters the sialome of I. ricinus ticks during the earliest stages of feeding. Front. Cell Infect. Microbiol. 10, 41. doi: 10.3389/fcimb.2020.00041
Helble, J. D., McCarthy, J. E., Hu, L. T. (2021). Interactions between Borrelia burgdorferi and its hosts across the enzootic cycle. Parasite Immunol. 43 (5), e12816. doi: 10.1111/pim.12816
Henning, K., Greiner-Fischer, S., Hotzel, H., Ebsen, M., Theegarten, D. (2006). Isolation of Spiroplasma sp. from an Ixodes tick. Int. J. Med. Microbiol. 296 Suppl 40, 157–161. doi: 10.1016/j.ijmm.2006.01.012
Hermance, M. E., Widen, S. G., Wood, T. G., Thangamani, S. (2019). Ixodes scapularis salivary gland microRNAs are differentially expressed during Powassan virus transmission. Sci. Rep. 9 (1), 13110. doi: 10.1038/s41598-019-49572-5
Herrmann, C., Gern, L. (2010). Survival of I. ricinus (Acari: Ixodidae) under challenging conditions of temperature and humidity is influenced by Borrelia burgdorferi sensu lato infection. J. Med. Entomol 47 (6), 1196–1204. doi: 10.1603/me10111
Herrmann, C., Gern, L. (2012). Do the level of energy reserves, hydration status and Borrelia infection influence walking by I. ricinus (Acari: Ixodidae) ticks? Parasitology 139 (3), 330–337. doi: 10.1017/s0031182011002095
Herrmann, C., Voordouw, M. J., Gern, L. (2013). I. ricinus ticks infected with the causative agent of Lyme disease, Borrelia burgdorferi sensu lato, have higher energy reserves. Int. J. Parasitol. 43 (6), 477–483. doi: 10.1016/j.ijpara.2012.12.010
Hightower, J., Kracalik, I. T., Vydayko, N., Goodin, D., Glass, G., Blackburn, J. K. (2014). Historical distribution and host-vector diversity of Francisella tularensis, the causative agent of tularemia, in Ukraine. Parasit. Vectors 7, 453. doi: 10.1186/s13071-014-0453-2
Hildebrandt, A., Straube, E., Neubauer, H., Schmoock, G. (2011). C. burnetii and coinfections in I. ricinus ticks in central Germany. Vector Borne Zoonot. Dis. 11 (8), 1205–1207. doi: 10.1089/vbz.2010.0180
Hildebrandt, A., Zintl, A., Montero, E., Hunfeld, K.-P., Gray, J. (2021). Human babesiosis in Europe. Pathogens 10 (9), 1165. doi: 10.3390/pathogens10091165
Hofer, U. (2021). Tick symbiont promotes blood feeding. Nat. Rev. Microbiol. 19 (12), 744. doi: 10.1038/s41579-021-00645-1
Hoffmann, A., Müller, T., Fingerle, V., Noll, M. (2021). Presence of human pathogens of the Borrelia burgdorferi sensu lato complex shifts the sequence read abundances of tick microbiomes in two German locations. Microorganisms 9 (9), 1814. doi: 10.3390/microorganisms9091814
Hofhuis, A., van de Kassteele, J., Sprong, H., van den Wijngaard, C. C., Harms, M. G., Fonville, M., et al. (2017). Predicting the risk of Lyme borreliosis after a tick bite, using a structural equation model. PLoS One 12 (7), e0181807. doi: 10.1371/journal.pone.0181807
Honig Mondekova, H., Sima, R., Urbanova, V., Kovar, V., Rego, R. O. M., Grubhoffer, L., et al. (2017). Characterization of I. ricinus fibrinogen-related proteins (Ixoderins) discloses their function in the tick innate immunity. Front. Cell. Infect. Microbiol. 7, 509. doi: 10.3389/fcimb.2017.00509
Hooper, L. V., Gordon, J. I. (2001). Commensal host-bacterial relationships in the gut. Science 292 (5519), 1115–1118. doi: 10.1126/science.1058709
Hornok, S., Csörgő, T., de la Fuente, J., Gyuranecz, M., Privigyei, C., Meli, M. L., et al. (2013). Synanthropic birds associated with high prevalence of tick-borne rickettsiae and with the first detection of Rickettsia aeschlimannii in Hungary. Vector Borne Zoonot. Dis. 13 (2), 77–83. doi: 10.1089/vbz.2012.1032
Hosseini-Chegeni, A., Tavakoli, M., Telmadarraiy, Z., Faghihi, F. (2020). Molecular detection of spotted fever group Rickettsia (Rickettsiales: Rickettsiaceae) in ticks of Iran. Arch. Razi Inst 75 (3), 317–325. doi: 10.22092/ari.2019.125746.1317
Hubálek, Z., Rudolf, I. (2011). Microbial Zoonoses and Sapronoses Heidelberg-London-New York: Springer Dordrecht.
Hu, R., Hyland, K. E., Oliver, J. H. (1998). A review on the use of Ixodiphagus wasps (Hymenoptera: Encyrtidae) as natural enemies for the control of ticks (Acari: Ixodidae). Syst. Appl. Acarol. 3 (1), 19–28,10. doi: 10.11158/saa.3.1.3
Hussain, S., Perveen, N., Hussain, A., Song, B., Aziz, M. U., Zeb, J., et al. (2022). The symbiotic continuum within ticks: Opportunities for disease control. Front. Microbiol. 13, 854803. doi: 10.3389/fmicb.2022.854803
Isogai, E., Isogai, H., Takahashi, K., Kobayashi-Sakamoto, M., Okumura, K. (2009). Antimicrobial activity of three tick defensins and four mammalian cathelicidin-derived synthetic peptides against Lyme disease spirochetes and bacteria isolated from the midgut. Exp. Appl. Acarol. 49 (3), 221–228. doi: 10.1007/s10493-009-9251-5
Jaarsma, R. I., Sprong, H., Takumi, K., Kazimirova, M., Silaghi, C., Mysterud, A., et al. (2019). Anaplasma phagocytophilum evolves in geographical and biotic niches of vertebrates and ticks. Parasit. Vectors 12 (1), 328. doi: 10.1186/s13071-019-3583-8
Jahfari, S., Coipan, E. C., Fonville, M., van Leeuwen, A. D., Hengeveld, P., Heylen, D., et al. (2014). Circulation of four Anaplasma phagocytophilum ecotypes in Europe. Parasit. Vectors 7, 365. doi: 10.1186/1756-3305-7-365
Jalovecka, M., Hajdusek, O., Sojka, D., Kopacek, P., Malandrin, L. (2018). The complexity of piroplasms life cycles. Front. Cell. Infect. Microbiol. 8, 248. doi: 10.3389/fcimb.2018.00248
Jalovecka, M., Sojka, D., Ascencio, M., Schnittger, L. (2019). Babesia life cycle - when phylogeny meets biology. Trends Parasitol. 35 (5), 356–368. doi: 10.1016/j.pt.2019.01.007
Jašarević, E., Hill, E. M., Kane, P. J., Rutt, L., Gyles, T., Folts, L., et al. (2021). The composition of human vaginal microbiota transferred at birth affects offspring health in a mouse model. Nat. Commun. 12 (1), 6289. doi: 10.1038/s41467-021-26634-9
Jiao, J., Zhang, J., He, P., OuYang, X., Yu, Y., Wen, B., et al. (2021). Identification of tick-borne pathogens and genotyping of C. burnetii in Rhipicephalus microplus in Yunnan province, China. Front. Microbiol. 12, 736484. doi: 10.3389/fmicb.2021.736484
Jia, N., Wang, J., Shi, W., Du, L., Sun, Y., Zhan, W., et al. (2020). Large-scale comparative analyses of tick genomes elucidate their genetic diversity and vector capacities. Cell 182 (5), 1328–1340.e1313. doi: 10.1016/j.cell.2020.07.023
Kagemann, J., Clay, K. (2013). Effects of infection by Arsenophonus and Rickettsia bacteria on the locomotive ability of the ticks Amblyomma americanum, Dermacentor variabilis, and Ixodes scapularis. J. Med. Entomol. 50 (1), 155–162. doi: 10.1603/me12086
Kalmár, Z., Cozma, V., Sprong, H., Jahfari, S., D'Amico, G., Mărcuțan, D. I., et al. (2015). Transstadial transmission of Borrelia turcica in Hyalomma aegyptium ticks. PLoS One 10 (2), e0115520. doi: 10.1371/journal.pone.0115520
Kalsbeek, V., Frandsen, F., Steenberg, T. (1995). Entomopathogenic fungi associated with I. ricinus ticks. Exp. Appl. Acarol. 19 (1), 45–51. doi: 10.1007/bf00051936
Kazimírová, M., Thangamani, S., Bartíková, P., Hermance, M., Holíková, V., Štibrániová, I., et al. (2017). Tick-borne viruses and biological processes at the tick-host-virus interface. Front. Cell. Infect. Microbiol. 7, 339. doi: 10.3389/fcimb.2017.00339
Kelly, P. J., Mason, P. R. (1991). Transmission of a spotted fever group Rickettsia by Amblyomma hebraeum (Acari: Ixodidae). J. Med. Entomol. 28 (5), 598–600. doi: 10.1093/jmedent/28.5.598
Khoo, J. J., Kurtti, T. J., Husin, N. A., Beliavskaia, A., Lim, F. S., Zulkifli, M. M. S., et al. (2020). Isolation and propagation of laboratory strains and a novel flea-derived field strain of Wolbachia in tick cell lines. Microorganisms 8 (7), 988. doi: 10.3390/microorganisms8070988
Kim, T. Y., Kwak, Y. S., Kim, J. Y., Nam, S. H., Lee, I. Y., Mduma, S., et al. (2018). Prevalence of tick-borne pathogens from ticks collected from cattle and wild animals in Tanzania in 2012. Korean J. Parasitol. 56 (3), 305–308. doi: 10.3347/kjp.2018.56.3.305
Kim, T. K., Tirloni, L., Bencosme-Cuevas, E., Kim, T. H., Diedrich, J. K., Yates, J. R., 3rd, et al. (2021b). Borrelia burgdorferi infection modifies protein content in saliva of Ixodes scapularis nymphs. BMC Genomics 22 (1), 152. doi: 10.1186/s12864-021-07429-0
Kim, T. K., Tirloni, L., Pinto, A. F., Moresco, J., Yates, J. R., 3rd, da Silva Vaz, I., Jr., et al. (2016). Ixodes scapularis tick saliva proteins sequentially secreted every 24 h during blood feeding. PLoS Negl. Trop. Dis. 10 (1), e0004323. doi: 10.1371/journal.pntd.0004323
Kim, J. Y., Yi, M. H., Lee, S., Lee, I. Y., Yong, D., Yoon, S. S., et al. (2021a). Microbiome and mycobiome interaction in house dust mites and impact on airway cells. Clin. Exp. Allergy 51 (12), 1592–1602. doi: 10.1111/cea.13962
Klyachko, O., Stein, B. D., Grindle, N., Clay, K., Fuqua, C. (2007). Localization and visualization of a Coxiella-type symbiont within the lone star tick, Amblyomma americanum. Appl. Environ. Microbiol. 73 (20), 6584–6594. doi: 10.1128/aem.00537-07
Koči, J., Bista, S., Chirania, P., Yang, X., Kitsou, C., Rana, V. S., et al. (2021). Antibodies against EGF-like domains in Ixodes scapularis BM86 orthologs impact tick feeding and survival of Borrelia burgdorferi. Sci. Rep. 11 (1), 6095. doi: 10.1038/s41598-021-85624-5
Koka, H., Sang, R., Kutima, H. L., Musila, L. (2018). C. burnetii detected in tick samples from pastoral communities in Kenya. BioMed. Res. Int. 2018, 8158102. doi: 10.1155/2018/8158102
Kotsyfakis, M., Kopáček, P., Franta, Z., Pedra, J. H., Ribeiro, J. M. (2015). Deep sequencing analysis of the I. ricinus haemocytome. PLoS Negl. Trop. Dis. 9 (5), e0003754. doi: 10.1371/journal.pntd.0003754
Krause, P. J., Fish, D., Narasimhan, S., Barbour, A. G. (2015). Borrelia miyamotoi infection in nature and in humans. Clin. Microbiol. Infect. 21 (7), 631–639. doi: 10.1016/j.cmi.2015.02.006
Krawczyk, A. I., Röttjers, L., Fonville, M., Takumi, K., Takken, W., Faust, K., et al. (2022). Quantitative microbial population study reveals geographical differences in bacterial symbionts of I. ricinus. Microbiome 10 (1), 120. doi: 10.1186/s40168-022-01276-1
Kuhn, K. H. (1996). Mitotic activity of the hemocytes in the tick I. ricinus (Acari; ixodidae). Parasitol. Res. 82 (6), 511–517. doi: 10.1007/s004360050154
Kuivanen, S., Levanov, L., Kareinen, L., Sironen, T., Jääskeläinen, A. J., Plyusnin, I., et al. (2019). Detection of novel tick-borne pathogen, Alongshan virus, in I. ricinus ticks, south-eastern Finland. Euro Surveill 24 (27), pii=1900394. doi: 10.2807/1560-7917.es.2019.24.27.1900394
Kumar, D., Downs, L. P., Adegoke, A., Machtinger, E., Oggenfuss, K., Ostfeld, R. S., et al. (2022a). An exploratory study on the microbiome of northern and southern populations of Ixodes scapularis ticks predicts changes and unique bacterial interactions. Pathogens 11 (2), 130. doi: 10.3390/pathogens11020130
Kumar, D., Sharma, S. R., Adegoke, A., Kennedy, A., Tuten, H. C., Li, A. Y., et al. (2022b). Recently evolved Francisella-like endosymbiont outcompetes an ancient and evolutionarily associated Coxiella-like endosymbiont in the lone star tick (Amblyomma americanum) linked to the alpha-gal syndrome. Front. Cell. Infect. Microbiol. 12, 787209. doi: 10.3389/fcimb.2022.787209
Kurtti, T. J., Burkhardt, N. Y., Heu, C. C., Munderloh, U. G. (2016). Fluorescent protein expressing Rickettsia buchneri and Rickettsia peacockii for tracking symbiont-tick cell interactions. Vet. Sci. 3 (4). doi: 10.3390/vetsci3040034
Kurtti, T. J., Felsheim, R. F., Burkhardt, N. Y., Oliver, J. D., Heu, C. C., Munderloh, U. G. (2015). Rickettsia buchneri sp. nov., a rickettsial endosymbiont of the blacklegged tick Ixodes scapularis. Int. J. Syst. Evol. Microbiol. 65 (Pt 3), 965–970. doi: 10.1099/ijs.0.000047
Kurtti, T. J., Simser, J. A., Baldridge, G. D., Palmer, A. T., Munderloh, U. G. (2005). Factors influencing in vitro infectivity and growth of Rickettsia peacockii (Rickettsiales: Rickettsiaceae), an endosymbiont of the rocky mountain wood tick, Dermacentor andersoni (Acari, ixodidae). J. Invertebr Pathol. 90 (3), 177–186. doi: 10.1016/j.jip.2005.09.001
Kwan, J. Y., Griggs, R., Chicana, B., Miller, C., Swei, A. (2017). Vertical vs. horizontal transmission of the microbiome in a key disease vector, Ixodes pacificus. Mol. Ecol. 26 (23), 6578–6589. doi: 10.1111/mec.14391
Lalzar, I., Friedmann, Y., Gottlieb, Y. (2014). Tissue tropism and vertical transmission of Coxiella in Rhipicephalus sanguineus and Rhipicephalus turanicus ticks. Environ. Microbiol. 16 (12), 3657–3668. doi: 10.1111/1462-2920.12455
Lalzar, I., Harrus, S., Mumcuoglu, K. Y., Gottlieb, Y. (2012). Composition and seasonal variation of Rhipicephalus turanicus and Rhipicephalus sanguineus bacterial communities. Appl. Environ. Microbiol. 78 (12), 4110–4116. doi: 10.1128/aem.00323-12
Landmann, F. (2019). The Wolbachia endosymbionts. Microbiol. Spectr. 7 (2). doi: 10.1128/microbiolspec.BAI-0018-2019
Lara, F. A., Lins, U., Bechara, G. H., Oliveira, P. L. (2005). Tracing heme in a living cell: hemoglobin degradation and heme traffic in digest cells of the cattle tick Boophilus microplus. J. Exp. Biol. 208 (Pt 16), 3093–3101. doi: 10.1242/jeb.01749
Leal, B. F., Alzugaray, M. F., Seixas, A., Da Silva Vaz, I., Ferreira, C. A. S. (2018). Characterization of a glycine-rich protein from Rhipicephalus microplus: tissue expression, gene silencing and immune recognition. Parasitology 145 (7), 927–938. doi: 10.1017/s0031182017001998
Leal, B., Zamora, E., Fuentes, A., Thomas, D. B., Dearth, R. K. (2020). Questing by tick larvae (Acari: Ixodidae): A review of the influences that affect off-host survival. Ann. Entomol Soc. Am. 113 (6), 425–438. doi: 10.1093/aesa/saaa013
Lefcort, H., Durden, L. A. (1996). The effect of infection with Lyme disease spirochetes (Borrelia burgdorferi) on the phototaxis, activity, and questing height of the tick vector Ixodes scapularis. Parasitology 113 (Pt 2), 97–103. doi: 10.1017/s0031182000066336
Lejal, E., Chiquet, J., Aubert, J., Robin, S., Estrada-Peña, A., Rue, O., et al. (2021). Temporal patterns in I. ricinus microbial communities: an insight into tick-borne microbe interactions. Microbiome 9 (1), 153. doi: 10.1186/s40168-021-01051-8
Lejal, E., Moutailler, S., Šimo, L., Vayssier-Taussat, M., Pollet, T. (2019). Tick-borne pathogen detection in midgut and salivary glands of adult I. ricinus. Parasit. Vectors 12 (1), 152. doi: 10.1186/s13071-019-3418-7
Lemasson, M., Caignard, G., Unterfinger, Y., Attoui, H., Bell-Sakyi, L., Hirchaud, E., et al. (2021). Exploration of binary protein-protein interactions between tick-borne flaviviruses and I. ricinus. Parasit. Vectors 14 (1), 144. doi: 10.1186/s13071-021-04651-3
Liang, Y., Piao, C., Beuschel, C. B., Toppe, D., Kollipara, L., Bogdanow, B., et al. (2021). eIF5A hypusination, boosted by dietary spermidine, protects from premature brain aging and mitochondrial dysfunction. Cell Rep. 35 (2), 108941. doi: 10.1016/j.celrep.2021.108941
Liberska, J., Michalik, J., Pers-Kamczyc, E., Wierzbicka, A., Lane, R. S., Rączka, G., et al. (2021). Prevalence of Babesia canis DNA in I. ricinus ticks collected in forest and urban ecosystems in west-central Poland. Ticks Tick Borne Dis. 12 (5), 101786. doi: 10.1016/j.ttbdis.2021.101786
Lieskovská, J., Páleníková, J., Langhansová, H., Chmelař, J., Kopecký, J. (2018). Saliva of I. ricinus enhances TBE virus replication in dendritic cells by modulation of pro-survival Akt pathway. Virology 514, 98–105. doi: 10.1016/j.virol.2017.11.008
Lipa, J., Eilenberg, J., Bresciani, J., Frandsen, F. (1997). Some observations on a newly recorded mermithid parasite of I. ricinus L. (Acarina: Ixodidae). Acta Parasitologica 42 (2).
Liu, L., Li, L., Liu, J., Hu, Y., Liu, Z., Guo, L., et al. (2013). Coinfection of Dermacentor silvarum Olenev (Acari: Ixodidae) by Coxiella-like, Arsenophonus-like, and Rickettsia-like symbionts. Appl. Environ. Microbiol. 79 (7), 2450–2454. doi: 10.1128/aem.03575-12
Liu, L., Narasimhan, S., Dai, J., Zhang, L., Cheng, G., Fikrig, E. (2011). Ixodes scapularis salivary gland protein P11 facilitates migration of Anaplasma phagocytophilum from the tick gut to salivary glands. EMBO Rep. 12 (11), 1196–1203. doi: 10.1038/embor.2011.177
Liu, J. N., Yu, Z. J., Liu, L. M., Li, N. X., Wang, R. R., Zhang, C. M., et al. (2016). Identification, distribution and population dynamics of Francisella-like endosymbiont in Haemaphysalis doenitzi (Acari: Ixodidae). Sci. Rep. 6, 35178. doi: 10.1038/srep35178
Lo, N., Beninati, T., Sassera, D., Bouman, E. A., Santagati, S., Gern, L., et al. (2006). Widespread distribution and high prevalence of an alpha-proteobacterial symbiont in the tick I. ricinus. Environ. Microbiol. 8 (7), 1280–1287. doi: 10.1111/j.1462-2920.2006.01024.x
Luu, L., Bown, K. J., Palomar, A. M., Kazimírová, M., Bell-Sakyi, L. (2020). Isolation and partial characterisation of a novel Trypanosoma from the tick I. ricinus. Ticks Tick Borne Dis. 11 (5), 101501. doi: 10.1016/j.ttbdis.2020.101501
Mahmood, S., Sima, R., Urbanova, V., Trentelman, J. J. A., Krezdorn, N., Winter, P., et al. (2020). Identification of tick I. ricinus midgut genes differentially expressed during the transmission of Borrelia afzelii spirochetes using a transcriptomic approach. Front. Immunol. 11. doi: 10.3389/fimmu.2020.612412
Mansfield, K. L., Cook, C., Ellis, R. J., Bell-Sakyi, L., Johnson, N., Alberdi, P., et al. (2017). Tick-borne pathogens induce differential expression of genes promoting cell survival and host resistance in I. ricinus cells. Parasit. Vectors 10 (1), 81. doi: 10.1186/s13071-017-2011-1
Mariconti, M., Epis, S., Gaibani, P., Dalla Valle, C., Sassera, D., Tomao, P., et al (2021). Humans parasitized by the hard tick I. ricinus are seropositive to Midichloria mitochondrii: is Midichloria a novel pathogen, or just a marker of tick bite? Pathog. Glob. Health 106 (7), 391–6. doi: 10.1179/2047773212y.0000000050
Marques, A. R., Strle, F., Wormser, G. P. (2021). Comparison of Lyme disease in the united states and Europe. Emerg. Infect. Dis. 27 (8), 2017–2024. doi: 10.3201/eid2708.204763
Mathew, M. J., Subramanian, G., Nguyen, T. T., Robert, C., Mediannikov, O., Fournier, P. E., et al. (2012). Genome sequence of Diplorickettsia massiliensis, an emerging I. ricinus-associated human pathogen. J. Bacteriol 194 (12), 3287. doi: 10.1128/jb.00448-12
Maurin, M., Raoult, D. (1999). Q fever. Clin. Microbiol. Rev. 12 (4), 518–553. doi: 10.1128/cmr.12.4.518
Mediannikov, O., Sekeyová, Z., Birg, M. L., Raoult, D. (2010). A novel obligate intracellular gamma-proteobacterium associated with ixodid ticks, Diplorickettsia massiliensis, gen. nov., sp. nov. PLoS One 5 (7), e11478. doi: 10.1371/journal.pone.0011478
Menchaca, A. C., Visi, D. K., Strey, O. F., Teel, P. D., Kalinowski, K., Allen, M. S., et al. (2013). Preliminary assessment of microbiome changes following blood-feeding and survivorship in the Amblyomma americanum nymph-to-adult transition using semiconductor sequencing. PLoS One 8 (6), e67129. doi: 10.1371/journal.pone.0067129
Merhej, V., Raoult, D. (2011). Rickettsial evolution in the light of comparative genomics. Biol. Rev. Camb Philos. Soc. 86 (2), 379–405. doi: 10.1111/j.1469-185X.2010.00151.x
Michelet, L., Delannoy, S., Devillers, E., Umhang, G., Aspan, A., Juremalm, M., et al. (2014). High-throughput screening of tick-borne pathogens in Europe. Front. Cell Infect. Microbiol. 4, 103. doi: 10.3389/fcimb.2014.00103
Mitina, G. V., Tokarev, Y. S., Movila, A. A., Yli-Mattila, T. (2011). Polymorphism of Beauveria bassiana (Deuteromycota: Hyphomycetes) strains isolated from I. ricinus (Acari: Ixodidae) in Moldova. Ticks tick-borne Dis. 2 (1), 50–54. doi: 10.1016/j.ttbdis.2010.10.005
Moore, T. C., Pulscher, L. A., Caddell, L., von Fricken, M. E., Anderson, B. D., Gonchigoo, B., et al. (2018). Evidence for transovarial transmission of tick-borne Rickettsiae circulating in northern Mongolia. PLoS Negl. Trop. Dis. 12 (8), e0006696. doi: 10.1371/journal.pntd.0006696
Moraes-Filho, J., Costa, F. B., Gerardi, M., Soares, H. S., Labruna, M. B. (2018). Rickettsia rickettsii co-feeding transmission among Amblyomma aureolatum ticks. Emerg. Infect. Dis. 24 (11), 2041–2048. doi: 10.3201/eid2411.180451
Mörner, T. (1992). The ecology of tularaemia. Rev. Sci. Tech 11 (4), 1123–1130. doi: 10.20506/rst.11.4.657
Moutailler, S., Popovici, I., Devillers, E., Vayssier-Taussat, M., Eloit, M. (2016a). Diversity of viruses in I. ricinus, and characterization of a neurotropic strain of Eyach virus. New Microbes New Infect. 11, 71–81. doi: 10.1016/j.nmni.2016.02.012
Moutailler, S., Valiente Moro, C., Vaumourin, E., Michelet, L., Tran, F. H., Devillers, E., et al. (2016b). Co-infection of ticks: The rule rather than the exception. PLoS Negl. Trop. Dis. 10 (3), e0004539. doi: 10.1371/journal.pntd.0004539
Munteanu, N. V., Mitkovets, P. V., Mitina, G. V., Movila, A., Tokarev, Y. S., Leclerque, A. (2014). Prevalence of Beauveria pseudobassiana among entomopathogenic fungi isolated from the hard tick, I. ricinus. Ticks tick-borne Dis. 5 (6), 641–648. doi: 10.1016/j.ttbdis.2014.04.015
Myllykallio, H., Liebl, U. (2000). Dual role for cytochrome cbb3 oxidase in clinically relevant proteobacteria? Trends Microbiol. 8 (12), 542–543. doi: 10.1016/s0966-842x(00)91831-6
Nah, K., Wu, J. (2021). Long-term transmission dynamics of tick-borne diseases involving seasonal variation and co-feeding transmission. J. Biol. Dyn. 15 (1), 269–286. doi: 10.1080/17513758.2021.1919322
Nakajima, Y., Ogihara, K., Taylor, D., Yamakawa, M. (2003). Antibacterial hemoglobin fragments from the midgut of the soft tick, Ornithodoros moubata (Acari: Argasidae). J. Med. Entomol 40 (1), 78–81. doi: 10.1603/0022-2585-40.1.78
Narasimhan, S., Rajeevan, N., Liu, L., Zhao, Y. O., Heisig, J., Pan, J., et al. (2014). Gut microbiota of the tick vector Ixodes scapularis modulate colonization of the Lyme disease spirochete. Cell Host Microbe 15 (1), 58–71. doi: 10.1016/j.chom.2013.12.001
Narasimhan, S., Schuijt, T. J., Abraham, N. M., Rajeevan, N., Coumou, J., Graham, M., et al. (2017). Modulation of the tick gut milieu by a secreted tick protein favors Borrelia burgdorferi colonization. Nat. Commun. 8 (1), 184. doi: 10.1038/s41467-017-00208-0
Narasimhan, S., Swei, A., Abouneameh, S., Pal, U., Pedra, J. H. F., Fikrig, E. (2021). Grappling with the tick microbiome. Trends Parasitol. 37 (8), 722–733. doi: 10.1016/j.pt.2021.04.004
Neelakanta, G., Sultana, H., Fish, D., Anderson, J. F., Fikrig, E. (2010). Anaplasma phagocytophilum induces Ixodes scapularis ticks to express an antifreeze glycoprotein gene that enhances their survival in the cold. J. Clin. Invest. 120 (9), 3179–3190. doi: 10.1172/jci42868
Nicholson, W. L., Sonenshine, D. E., Noden, B. H., Brown, R. N. (2019). “Chapter 27 - ticks (Ixodida),” in Medical and veterinary entomology (Third edition). Eds. Mullen, G. R., Durden, L. A. (London, UK; San Diego, CA, USA; Cambridge, MA, USA; Oxford UK: Academic Press), 603–672.
Niebylski, M. L., Peacock, M. G., Fischer, E. R., Porcella, S. F., Schwan, T. G. (1997a). Characterization of an endosymbiont infecting wood ticks, Dermacentor andersoni, as a member of the genus Francisella. Appl. Environ. Microbiol. 63 (10), 3933–3940. doi: 10.1128/aem.63.10.3933-3940.1997
Niebylski, M. L., Schrumpf, M. E., Burgdorfer, W., Fischer, E. R., Gage, K. L., Schwan, T. G. (1997b). Rickettsia peacockii sp. nov., a new species infecting wood ticks, Dermacentor andersoni, in western Montana. Int. J. Syst. Bacteriol 47 (2), 446–452. doi: 10.1099/00207713-47-2-446
Noda, H., Munderloh, U. G., Kurtti, T. J. (1997). Endosymbionts of ticks and their relationship to Wolbachia spp. and tick-borne pathogens of humans and animals. Appl. Environ. Microbiol. 63 (10), 3926–3932. doi: 10.1128/aem.63.10.3926-3932.1997
Nováková, E., Hypsa, V., Moran, N. A. (2009). Arsenophonus, an emerging clade of intracellular symbionts with a broad host distribution. BMC Microbiol. 9, 143. doi: 10.1186/1471-2180-9-143
Nuttall, P. A. (2014). “Tick-borne viruses,” in Biology of ticks. Eds. Sonenshine, D. E., Roe, M. R. (New York, NY: Oxford University Press), 180–210.
Nuttall, P. A., Labuda, M. (2008). “Saliva-assisted transmission of tick-borne pathogens,” in Ticks: Biology, disease and control. Eds. Bowman, A. S., Nuttall, P. A. (Cambridge: Cambridge University Press), 205–219.
Ogata, S., Mohamed, W. M. A., Kusakisako, K., Thu, M. J., Qiu, Y., Moustafa, M. A. M., et al. (2021). Spiroplasma infection among ixodid ticks exhibits species dependence and suggests a vertical pattern of transmission. Microorganisms 9 (2). doi: 10.3390/microorganisms9020333
Ohlendorf, V., Marklewitz, M., Kopp, A., Yordanov, S., Drosten, C., Junglen, S. (2019). Huge diversity of phleboviruses in ticks from Strandja nature park, Bulgaria. Ticks Tick Borne Dis. 10 (3), 697–703. doi: 10.1016/j.ttbdis.2019.03.001
Ojaimi, C., Brooks, C., Casjens, S., Rosa, P., Elias, A., Barbour, A., et al. (2003). Profiling of temperature-induced changes in Borrelia burgdorferi gene expression by using whole genome arrays. Infect. Immun. 71 (4), 1689–1705. doi: 10.1128/iai.71.4.1689-1705.2003
Oliveira, J. H., Gonçalves, R. L., Lara, F. A., Dias, F. A., Gandara, A. C., Menna-Barreto, R. F., et al. (2011b). Blood meal-derived heme decreases ROS levels in the midgut of Aedes aegypti and allows proliferation of intestinal microbiota. PLoS Pathog. 7 (3), e1001320. doi: 10.1371/journal.ppat.1001320
Oliveira, C. J., Sá-Nunes, A., Francischetti, I. M., Carregaro, V., Anatriello, E., Silva, J. S., et al. (2011a). Deconstructing tick saliva: non-protein molecules with potent immunomodulatory properties. J. Biol. Chem. 286 (13), 10960–10969. doi: 10.1074/jbc.M110.205047
Oliver, K. M., Degnan, P. H., Burke, G. R., Moran, N. A. (2010). Facultative symbionts in aphids and the horizontal transfer of ecologically important traits. Annu. Rev. Entomol. 55, 247–266. doi: 10.1146/annurev-ento-112408-085305
Oliver, J. D., Price, L. D., Burkhardt, N. Y., Heu, C. C., Khoo, B. S., Thorpe, C. J., et al. (2021). Growth dynamics and antibiotic elimination of symbiotic Rickettsia buchneri in the tick Ixodes scapularis (Acari: Ixodidae). Appl. Environ. Microbiol. 87 (3). doi: 10.1128/aem.01672-20
Olivieri, E., Epis, S., Castelli, M., Varotto Boccazzi, I., Romeo, C., Desirò, A., et al. (2019). Tissue tropism and metabolic pathways of Midichloria mitochondrii suggest tissue-specific functions in the symbiosis with I. ricinus. Ticks Tick Borne Dis. 10 (5), 1070–1077. doi: 10.1016/j.ttbdis.2019.05.019
Ondruš, J., Balážová, A., Baláž, V., Zechmeisterová, K., Novobilský, A., Široký, P. (2020a). Candidatus Neoehrlichia mikurensis is widespread in questing I. ricinus ticks in the Czech republic. Ticks Tick Borne Dis. 11 (3), 101371. doi: 10.1016/j.ttbdis.2020.101371
Ondruš, J., Kulich, P., Sychra, O., Široký, P. (2020b). Putative morphology of Neoehrlichia mikurensis in salivary glands of I. ricinus. Sci. Rep. 10 (1), 15987. doi: 10.1038/s41598-020-72953-0
Oteo, J. A., Portillo, A. (2012). Tick-borne rickettsioses in Europe. Ticks Tick Borne Dis. 3 (5-6), 271–278. doi: 10.1016/j.ttbdis.2012.10.035
Pal, U., de Silva, A. M., Montgomery, R. R., Fish, D., Anguita, J., Anderson, J. F., et al. (2000). Attachment of Borrelia burgdorferi within Ixodes scapularis mediated by outer surface protein A. J. Clin. Invest. 106 (4), 561–569. doi: 10.1172/jci9427
Pal, U., Li, X., Wang, T., Montgomery, R. R., Ramamoorthi, N., De Silva, A. M., et al. (2004). TROSPA, an Ixodes scapularis receptor for Borrelia burgdorferi. Cell 119 (4), 457–468. doi: 10.1016/j.cell.2004.10.027
Papa, A., Tsioka, K., Kontana, A., Papadopoulos, C., Giadinis, N. (2017). Bacterial pathogens and endosymbionts in ticks. Ticks Tick Borne Dis. 8 (1), 31–35. doi: 10.1016/j.ttbdis.2016.09.011
Papa, A., Zelená, H., Papadopoulou, E., Mrázek, J. (2018). Uukuniemi virus, Czech republic. Ticks Tick Borne Dis. 9 (5), 1129–1132. doi: 10.1016/j.ttbdis.2018.04.011
Pekáriková, D., Rajská, P., Kazimírová, M., Pecháňová, O., Takáč, P., Nuttall, P. A. (2015). Vasoconstriction induced by salivary gland extracts from ixodid ticks. Int. J. Parasitol. 45 (14), 879–883. doi: 10.1016/j.ijpara.2015.08.006
Perner, J., Provazník, J., Schrenková, J., Urbanová, V., Ribeiro, J. M., Kopáček, P. (2016). RNA-Seq analyses of the midgut from blood- and serum-fed I. ricinus ticks. Sci. Rep. 6, 36695. doi: 10.1038/srep36695
Perret, J. L., Guerin, P. M., Diehl, P. A., Vlimant, M., Gern, L. (2003). Darkness induces mobility, and saturation deficit limits questing duration, in the tick I. ricinus. J. Exp. Biol. 206 (Pt 11), 1809–1815. doi: 10.1242/jeb.00345
Pettersson, J. H., Shi, M., Bohlin, J., Eldholm, V., Brynildsrud, O. B., Paulsen, K. M., et al. (2017). Characterizing the virome of I. ricinus ticks from northern Europe. Sci. Rep. 7 (1), 10870. doi: 10.1038/s41598-017-11439-y
Pham, M., Underwood, J., Oliva Chávez, A. S. (2021). Changing the recipe: Pathogen directed changes in tick saliva components. Int. J. Environ. Res. Publ. Health 18 (4), 1806. doi: 10.3390/ijerph18041806
Philip, C. B. (1959). Some epidemiological considerations in rocky mountain spotted fever. Publ. Health Rep. (1896) 74 (7), 595–600. doi: 10.2307/4590519
Pillonel, T., Bertelli, C., Aeby, S., de Barsy, M., Jacquier, N., Kebbi-Beghdadi, C., et al. (2019). Sequencing the obligate intracellular Rhabdochlamydia helvetica within its tick host I. ricinus to investigate their symbiotic relationship. Genome Biol. Evol. 11 (4), 1334–1344. doi: 10.1093/gbe/evz072
Pilloux, L., Aeby, S., Gaümann, R., Burri, C., Beuret, C., Greub, G. (2015). The high prevalence and diversity of Chlamydiales DNA within I. ricinus ticks suggest a role for ticks as reservoirs and vectors of Chlamydia-related bacteria. Appl. Environ. Microbiol. 81 (23), 8177–8182. doi: 10.1128/aem.02183-15
Plantard, O., Bouju-Albert, A., Malard, M. A., Hermouet, A., Capron, G., Verheyden, H. (2012). Detection of Wolbachia in the tick I. ricinus is due to the presence of the Hymenoptera endoparasitoid Ixodiphagus hookeri. PLoS One 7 (1), e30692. doi: 10.1371/journal.pone.0030692
Podboronov, V. M., Pchelkina, A. A. (1989). Characteristics of the transphase and transovarial transmission of Rickettsia sibirica by ixodid and argasid ticks. Med. Parazitol. (Mosk). 4, 14–18.
Pollet, T., Sprong, H., Lejal, E., Krawczyk, A. I., Moutailler, S., Cosson, J. F., et al. (2020). The scale affects our view on the identification and distribution of microbial communities in ticks. Parasit. Vectors 13 (1), 36. doi: 10.1186/s13071-020-3908-7
Portillo, A., Santibáñez, P., Palomar, A. M., Santibáñez, S., Oteo, J. A. (2018). 'Candidatus Neoehrlichia mikurensis' in Europe. New Microbes New Infect. 22, 30–36. doi: 10.1016/j.nmni.2017.12.011
Prevot, P. P., Adam, B., Boudjeltia, K. Z., Brossard, M., Lins, L., Cauchie, P., et al. (2006). Anti-hemostatic effects of a serpin from the saliva of the tick I. ricinus. J. Biol. Chem. 281 (36), 26361–26369. doi: 10.1074/jbc.M604197200
Price, D. R. G., Bartley, K., Blake, D. P., Karp-Tatham, E., Nunn, F., Burgess, S. T. G., et al. (2021). A Rickettsiella endosymbiont is a potential source of essential B-vitamins for the poultry red mite, Dermanyssus gallinae. Front. Microbiol. 12, 695346. doi: 10.3389/fmicb.2021.695346
Psaroulaki, A., Chochlakis, D., Angelakis, E., Ioannou, I., Tselentis, Y. (2014). C. burnetii in wildlife and ticks in an endemic area. Trans. R. Soc. Trop. Med. Hyg. 108 (10), 625–631. doi: 10.1093/trstmh/tru134
Puleston, D. J., Buck, M. D., Klein Geltink, R. I., Kyle, R. L., Caputa, G., O'Sullivan, D., et al. (2019). Polyamines and eIF5A hypusination modulate mitochondrial respiration and macrophage activation. Cell Metab. 30 (2), 352–363.e358. doi: 10.1016/j.cmet.2019.05.003
Qiu, Y., Nakao, R., Ohnuma, A., Kawamori, F., Sugimoto, C. (2014). Microbial population analysis of the salivary glands of ticks; a possible strategy for the surveillance of bacterial pathogens. PLoS One 9 (8), e103961. doi: 10.1371/journal.pone.0103961
Rahal, M., Medkour, H., Diarra, A. Z., Bitam, I., Parola, P., Mediannikov, O. (2020). Molecular identification and evaluation of Coxiella-like endosymbionts genetic diversity carried by cattle ticks in Algeria. Ticks Tick Borne Dis. 11 (5), 101493. doi: 10.1016/j.ttbdis.2020.101493
Răileanu, C., Tauchmann, O., Vasić, A., Neumann, U., Tews, B. A., Silaghi, C. (2020). Transstadial transmission and replication kinetics of West Nile virus lineage 1 in laboratory reared I. ricinus ticks. Pathogens 9 (10), 780. doi: 10.3390/pathogens9100780
Ramamoorthi, N., Narasimhan, S., Pal, U., Bao, F., Yang, X. F., Fish, D., et al. (2005). The Lyme disease agent exploits a tick protein to infect the mammalian host. Nature 436 (7050), 573–577. doi: 10.1038/nature03812
Raoult, D. (1993). Treatment of Q fever. Antimicrob. Agents Chemother. 37 (9), 1733–1736. doi: 10.1128/aac.37.9.1733
Řeháček, J., Kováčová, E., Kocianová, E. (1996). Isolation of Nosema slovaca (Microsporidiae) from Dermacentor reticulatus ticks (Acari: Ixodidae) collected in Hungary. Exp. Appl. Acarol. 20 (1), 57–60. doi: 10.1007/BF00051477
Rehacek, J., Sixl, W., Sebek, Z. (1974). Trypanosomen in der Haemolymphe von Zecken. Mitt. Abt. Zool. Landesmus. Joanneum 3 (1), 33.
Rehácek, J., Urvölgyi, J., Kocianová, E., Sekeyová, Z., Vavreková, M., Kovácová, E. (1991). Extensive examination of different tick species for infestation with C. burnetii in Slovakia. Eur. J. Epidemiol. 7 (3), 299–303. doi: 10.1007/bf00145682
Rehse-Küpper, B., Casals, J., Rehse, E., Ackermann, R. (1976). Eyach–an arthropod-borne virus related to Colorado tick fever virus in the Federal Republic of Germany. Acta Virol. 20 (4), 339–342.
Reinhardt, C., Aeschlimann, A., Hecker, H. (1972). Distribution of Rickettsia-like microorganisms in various organs of an Ornithodorus moubata laboratory strain (Ixodoidea, Argasidae) as revealed by electron microscopy. Z Parasitenkd 39 (3), 201–209. doi: 10.1007/bf00329456
Richter, D., Matuschka, F. R., Spielman, A., Mahadevan, L. (2013). How ticks get under your skin: insertion mechanics of the feeding apparatus of I. ricinus ticks. Proc. Biol. Sci. 280 (1773), 20131758. doi: 10.1098/rspb.2013.1758
Romashchenko, A. V., Ratushnyak, A. S., Zapara, T. A., Tkachev, S. E., Moshkin, M. P. (2012). The correlation between tick (Ixodes persulcatus Sch.) questing behaviour and synganglion neuronal responses to odours. J. Insect Physiol. 58 (7), 903–910. doi: 10.1016/j.jinsphys.2012.04.004
Rosenwald, L. C., Sitvarin, M. I., White, J. A. (2020). Endosymbiotic Rickettsiella causes cytoplasmic incompatibility in a spider host. Proc. Biol. Sci. 287 (1930), 20201107. doi: 10.1098/rspb.2020.1107
Ross, B. D., Hayes, B., Radey, M. C., Lee, X., Josek, T., Bjork, J., et al. (2018). Ixodes scapularis does not harbor a stable midgut microbiome. ISME J. 12 (11), 2596–2607. doi: 10.1038/s41396-018-0161-6
Rousseau, R., Vanwambeke, S. O., Boland, C., Mori, M. (2021). The isolation of culturable bacteria in I. ricinus ticks of a Belgian peri-urban forest uncovers opportunistic bacteria potentially important for public health. Int. J. Environ. Res. Publ. Health 18 (22), 12134. doi: 10.3390/ijerph182212134
Salcedo-Porras, N., Umaña-Diaz, C., Bitencourt, R. O. B., Lowenberger, C. (2020). The role of bacterial symbionts in triatomines: An evolutionary perspective. Microorganisms 8 (9), 1438. doi: 10.3390/microorganisms8091438
Sameroff, S., Tokarz, R., Vucelja, M., Jain, K., Oleynik, A., Boljfetić, M., et al. (2022). Virome of I. ricinus, Dermacentor reticulatus, and Haemaphysalis concinna ticks from Croatia. Viruses 14 (5), 929. doi: 10.3390/v14050929
Samish, M., Glazer, I. (2001). Entomopathogenic nematodes for the biocontrol of ticks. Trends Parasitol. 17 (8), 368–371. doi: 10.1016/S1471-4922(01)01985-7
Samoilis, G., Aivaliotis, M., Vranakis, I., Papadioti, A., Tselentis, Y., Tsiotis, G., et al. (2010). Proteomic screening for possible effector molecules secreted by the obligate intracellular pathogen C. burnetii. J. Proteome Res. 9 (3), 1619–1626. doi: 10.1021/pr900605q
Sassera, D., Beninati, T., Bandi, C., Bouman, E. A. P., Sacchi, L., Fabbi, M., et al. (2006). 'Candidatus Midichloria mitochondrii', an endosymbiont of the tick I. ricinus with a unique intramitochondrial lifestyle. Int. J. Syst. Evol. Microbiol. 56 (Pt 11), 2535–2540. doi: 10.1099/ijs.0.64386-0
Sassera, D., Lo, N., Bouman, E. A., Epis, S., Mortarino, M., Bandi, C. (2008). "Candidatus Midichloria" endosymbionts bloom after the blood meal of the host, the hard tick I. ricinus. Appl. Environ. Microbiol. 74 (19), 6138–6140. doi: 10.1128/aem.00248-08
Sassera, D., Lo, N., Epis, S., D'Auria, G., Montagna, M., Comandatore, F., et al. (2011). Phylogenomic evidence for the presence of a flagellum and cbb(3) oxidase in the free-living mitochondrial ancestor. Mol. Biol. Evol. 28 (12), 3285–3296. doi: 10.1093/molbev/msr159
Scholl, D. C., Embers, M. E., Caskey, J. R., Kaushal, D., Mather, T. N., Buck, W. R., et al. (2016). Immunomodulatory effects of tick saliva on dermal cells exposed to Borrelia burgdorferi, the agent of Lyme disease. Parasit. Vectors 9 (1), 394. doi: 10.1186/s13071-016-1638-7
Schouls, L. M., Van De Pol, I., Rijpkema, S. G., Schot, C. S. (1999). Detection and identification of Ehrlichia, Borrelia burgdorferi sensu lato, and Bartonella species in Dutch I. ricinus ticks. J. Clin. Microbiol. 37 (7), 2215–2222. doi: 10.1128/jcm.37.7.2215-2222.1999
Schwan, T. G., Piesman, J., Golde, W. T., Dolan, M. C., Rosa, P. A. (1995). Induction of an outer surface protein on Borrelia burgdorferi during tick feeding. Proc. Natl. Acad. Sci. U.S.A. 92 (7), 2909–2913. doi: 10.1073/pnas.92.7.2909
Sertour, N., Cotté, V., Garnier, M., Malandrin, L., Ferquel, E., Choumet, V. (2018). Infection kinetics and tropism of Borrelia burgdorferi sensu lato in mouse after natural (via ticks) or artificial (needle) infection depends on the bacterial strain. Front. Microbiol. 9, 1722. doi: 10.3389/fmicb.2018.01722
Seshadri, R., Paulsen, I. T., Eisen, J. A., Read, T. D., Nelson, K. E., Nelson, W. C., et al. (2003). Complete genome sequence of the Q-fever pathogen C. burnetii. Proc. Natl. Acad. Sci. U.S.A. 100 (9), 5455–5460. doi: 10.1073/pnas.0931379100
Sforça, M. L., Machado, A., Figueredo, R. C., Oyama, S., Jr., Silva, F. D., Miranda, A., et al. (2005). The micelle-bound structure of an antimicrobial peptide derived from the alpha-chain of bovine hemoglobin isolated from the tick Boophilus microplus. Biochemistry 44 (17), 6440–6451. doi: 10.1021/bi0475323
Sgroi, G., Iatta, R., Lovreglio, P., Stufano, A., Laidoudi, Y., Mendoza-Roldan, J.A., et al (2022). Detection of endosymbiont Candidatus Midichloria mitochondrii and tick borne pathogens in humans exposed to tick bites, Italy. Emerg. Infect. Dis 28 (9), 1824–32. doi: 10.3201/eid2809.220329
Shaw, E. I., Voth, D. E. (2019). C. burnetii: A pathogenic intracellular acidophile. Microbiol. (Reading) 165 (1), 1–3. doi: 10.1099/mic.0.000707
Šimo, L., Park, Y. (2014). Neuropeptidergic control of the hindgut in the black-legged tick Ixodes scapularis. Int. J. Parasitol. 44 (11), 819–826. doi: 10.1016/j.ijpara.2014.06.007
Simser, J. A., Palmer, A. T., Fingerle, V., Wilske, B., Kurtti, T. J., Munderloh, U. G. (2002). Rickettsia monacensis sp. nov., a spotted fever group Rickettsia, from ticks (I. ricinus) collected in a European city park. Appl. Environ. Microbiol. 68 (9), 4559–4566. doi: 10.1128/aem.68.9.4559-4566.2002
Smith, T. A., Driscoll, T., Gillespie, J. J., Raghavan, R. (2015). A Coxiella-like endosymbiont is a potential vitamin source for the lone star tick. Genome Biol. Evol. 7 (3), 831–838. doi: 10.1093/gbe/evv016
Socolovschi, C., Huynh, T. P., Davoust, B., Gomez, J., Raoult, D., Parola, P. (2009a). Transovarial and trans-stadial transmission of Rickettsia africae in Amblyomma variegatum ticks. Clin. Microbiol. Infect. 15 Suppl 2, 317–318. doi: 10.1111/j.1469-0691.2008.02278.x
Socolovschi, C., Mediannikov, O., Raoult, D., Parola, P. (2009b). The relationship between spotted fever group Rickettsiae and ixodid ticks. Vet. Res. 40 (2), 34. doi: 10.1051/vetres/2009017
Sonenshine, D. E., Anderson, J. M. (2014). “Mouthparts and digestive system: antomy and molecular biology feeding and digestion,” in Biology of ticks, 2nd ed. Eds. Sonenshine, D. E., Roe, M. R. (New York: Oxford University Press), 122–162.
Sonenshine, D. E., Hynes, W. L. (2008). Molecular characterization and related aspects of the innate immune response in ticks. Front. Biosci. 13, 7046–7063. doi: 10.2741/3209
Špitalská, E., Kraljik, J., Miklisová, D., Boldišová, E., Sparagano, O. A. E., Stanko, M. (2020). Circulation of Rickettsia species and rickettsial endosymbionts among small mammals and their ectoparasites in Eastern Slovakia. Parasitol. Res. 119 (7), 2047–2057. doi: 10.1007/s00436-020-06701-8
Špitalská, E., Sparagano, O., Stanko, M., Schwarzová, K., Špitalský, Z., Škultéty, Ľ., et al. (2018). Diversity of Coxiella-like and Francisella-like endosymbionts, and Rickettsia spp., C. burnetii as pathogens in the tick populations of Slovakia, central Europe. Ticks tick-borne Dis. 9 (5), 1207–1211. doi: 10.1016/j.ttbdis.2018.05.002
Sprong, H., Wielinga, P. R., Fonville, M., Reusken, C., Brandenburg, A. H., Borgsteede, F., et al. (2009). I. ricinus ticks are reservoir hosts for Rickettsia helvetica and potentially carry flea-borne Rickettsia species. Parasit. Vectors 2 (1), 41. doi: 10.1186/1756-3305-2-41
Stańczak, J., Biernat, B., Racewicz, M., Zalewska, M., Matyjasek, A. (2018). Prevalence of different Rickettsia spp. in I. ricinus and Dermacentor reticulatus ticks (Acari: Ixodidae) in north-eastern Poland. Ticks Tick Borne Dis. 9 (2), 427–434. doi: 10.1016/j.ttbdis.2017.12.010
Stanek, G., Kahl, O. (1999). Chemoprophylaxis for Lyme borreliosis? Zentralbl. Bakteriol. 289 (5-7), 655–665. doi: 10.1016/s0934-8840(99)80025-5
Stanek, G., Wormser, G. P., Gray, J., Strle, F. (2012). Lyme Borreliosis. Lancet 379 (9814), 461–473. doi: 10.1016/s0140-6736(11)60103-7
Steere, A. C., Strle, F., Wormser, G. P., Hu, L. T., Branda, J. A., Hovius, J. W., et al. (2016). Lyme Borreliosis. Nat. Rev. Dis. Primers 2, 16090. doi: 10.1038/nrdp.2016.90
Steiner, S., Meir, A., Roy, C. R. (2021). C. burnetii encodes an LvgA-related protein important for intracellular replication. Cell Microbiol. 23 (6), e13331. doi: 10.1111/cmi.13331
Steiner, F. E., Pinger, R. R., Vann, C. N., Grindle, N., Civitello, D., Clay, K., et al. (2008). Infection and co-infection rates of Anaplasma phagocytophilum variants, Babesia spp., Borrelia burgdorferi, and the rickettsial endosymbiont in Ixodes scapularis (Acari: Ixodidae) from sites in Indiana, Maine, Pennsylvania, and Wisconsin. J. Med. Entomol. 45 (2), 289–297. doi: 10.1603/0022-2585(2008)45[289:iacroa]2.0.co;2
Strle, F. (2004). Human granulocytic ehrlichiosis in Europe. Int. J. Med. Microbiol. 293 Suppl 37, 27–35. doi: 10.1016/s1433-1128(04)80006-8
Strle, F., Maraspin, V., Furlan-Lotric, S., Cimperman, J. (1996). Epidemiological study of a cohort of adult patients with erythema migrans registered in Slovenia in 1993. Eur. J. Epidemiol. 12 (5), 503–507. doi: 10.1007/bf00144004
Strnad, M., Hönig, V., Růžek, D., Grubhoffer, L., Rego, R. O. M. (2017). Europe-wide meta-analysis of Borrelia burgdorferi sensu lato prevalence in questing I. ricinus ticks. Appl. Environ. Microbiol. 83 (15), e00609–00617. doi: 10.1128/aem.00609-17
Strunov, A., Kiseleva, E., Gottlieb, Y. (2013). Spatial and temporal distribution of pathogenic Wolbachia strain wMelPop in Drosophila melanogaster central nervous system under different temperature conditions. J. Invertebr Pathol. 114 (1), 22–30. doi: 10.1016/j.jip.2013.05.001
Stuen, S., Granquist, E. G., Silaghi, C. (2013). Anaplasma phagocytophilum–a widespread multi-host pathogen with highly adaptive strategies. Front. Cell. Infect. Microbiol. 3, 31. doi: 10.3389/fcimb.2013.00031
Subramanian, G., Sekeyova, Z., Raoult, D., Mediannikov, O. (2012). Multiple tick-associated bacteria in I. ricinus from Slovakia. Ticks Tick Borne Dis. 3 (5-6), 406–410. doi: 10.1016/j.ttbdis.2012.10.001
Sun, L. V., Scoles, G. A., Fish, D., O'Neill, S. L. (2000). Francisella-like endosymbionts of ticks. J. Invertebr Pathol. 76 (4), 301–303. doi: 10.1006/jipa.2000.4983
Swei, A., Kwan, J. Y. (2017). Tick microbiome and pathogen acquisition altered by host blood meal. ISME J. 11 (3), 813–816. doi: 10.1038/ismej.2016.152
Szczepańska, A., Kiewra, D., Plewa-Tutaj, K., Dyczko, D., Guz-Regner, K. (2020). Sensitivity of I. ricinus (L. 1758) and Dermacentor reticulatus (Fabr. 1794) ticks to entomopathogenic fungi isolates: preliminary study. Parasitol. Res. 119 (11), 3857–3861. doi: 10.1007/s00436-020-06805-1
Taylor, M., Coop, R. L. (2015). “Veterinary entomology,” in Veterinary parasitology, 4th ed. Eds. Taylor, M. A., Coop, R. L., Wall, R. (Chichester, UK: Wiley-Blackwell), 161–258.
Tenckhoff, B., Kölmel, H. W., Wolf, V., Lange, R. (1994). Production and characterization of a polyclonal antiserum against Spiroplasma mirum (ATCC 29335). Zentralbl. Bakteriol. 280 (3), 409–415. doi: 10.1016/s0934-8840(11)80605-5
Thapa, S., Zhang, Y., Allen, M. S. (2019). Bacterial microbiomes of Ixodes scapularis ticks collected from Massachusetts and Texas, USA. BMC Microbiol. 19 (1), 138. doi: 10.1186/s12866-019-1514-7
Thorpe, C. J., Wang, X. R., Munderloh, U. G., Kurtti, T. J. (2021). Tick cell culture analysis of growth dynamics and cellular tropism of Rickettsia buchneri, an endosymbiont of the blacklegged tick, Ixodes scapularis. Insects 12 (11), 968. doi: 10.3390/insects12110968
Tijsse-Klasen, E., Braks, M., Scholte, E. J., Sprong, H. (2011). Parasites of vectors–Ixodiphagus hookeri and its Wolbachia symbionts in ticks in the Netherlands. Parasit. Vectors 4, 228. doi: 10.1186/1756-3305-4-228
Tirloni, L., Kim, T. K., Berger, M., Termignoni, C., da Silva Vaz, I., Jr., Mulenga, A. (2019). Amblyomma americanum serpin 27 (AAS27) is a tick salivary anti-inflammatory protein secreted into the host during feeding. PLoS Negl. Trop. Dis. 13 (8), e0007660. doi: 10.1371/journal.pntd.0007660
Tokarev Iu, S., Movilé, A. A. (2004). A first record of microsporidia in the ixodid tick I. ricinus L. (Ixodidae) in the territory of the Commonwealth of independent states, Republic Moldova. Parazitologiia 38 (6), 552–556.
Tomassone, L., Portillo, A., Nováková, M., de Sousa, R., Oteo, J. A. (2018). Neglected aspects of tick-borne rickettsioses. Parasit. Vectors 11 (1), 263. doi: 10.1186/s13071-018-2856-y
Tomazatos, A., von Possel, R., Pekarek, N., Holm, T., Rieger, T., Baum, H., et al. (2021). Discovery and genetic characterization of a novel orthonairovirus in I. ricinus ticks from Danube delta. Infect. Genet. Evol. 88, 104704. doi: 10.1016/j.meegid.2021.104704
Trevisan, G., Cinco, M., Trevisini, S., di Meo, N., Ruscio, M., Forgione, P., et al. (2021). Borreliae part 2: Borrelia relapsing fever group and unclassified Borrelia. Biol. (Basel) 10 (11), 1117. doi: 10.3390/biology10111117
Trzebny, A., Liberska, J., Slodkowicz-Kowalska, A., Dabert, M. (2022). Metabarcoding reveals low prevalence of microsporidian infections in castor bean tick (I. ricinus). Parasit. Vectors 15 (1), 26. doi: 10.1186/s13071-022-05150-9
Tufts, D. M., Diuk-Wasser, M. A. (2021). Vertical transmission: A vector-independent transmission pathway of Babesia microti in the natural reservoir host Peromyscus leucopus. J. Infect. Dis. 223 (10), 1787–1795. doi: 10.1093/infdis/jiaa595
Tully, J. G., Bové, J. M., Laigret, F., Whitcomb, R. F. (1993). Revised taxonomy of the class Mollicutes: Proposed elevation of a monophyletic cluster of arthropod-associated Mollicutes to ordinal rank (Entomoplasmatales ord. nov.), with provision for familial rank to separate species with nonhelical morphology (Entomoplasmataceae fam. nov.) from helical species (Spiroplasmataceae), and emended descriptions of the order Mycoplasmatales, family Mycoplasmataceae. Int. J. Syst. Evolut. Microbiol. 43 (2), 378–385. doi: 10.1099/00207713-43-2-378
Tully, J. G., Rose, D. L., Yunker, C. E., Cory, J., Whitcomb, R. F., Williamson, D. L. (1981). Helical mycoplasmas (Spiroplasmas) from Ixodes ticks. Science 212 (4498), 1043–1045. doi: 10.1126/science.7233197
Tully, J. G., Whitcomb, R. F., Rose, D. L., Bové, J. M. (1982). Spiroplasma mirum, a new species from the rabbit tick (Haemaphysalis leporispalustris). Int. J. Syst. Evol. Microbiol. 32 (1), 92–100. doi: 10.1099/00207713-32-1-92
Urbanová, V., Hajdušek, O., Šíma, R., Franta, Z., Hönig-Mondeková, H., Grunclová, L., et al. (2018). IrC2/Bf - a yeast and Borrelia responsive component of the complement system from the hard tick I. ricinus. Dev. Comp. Immunol. 79, 86–94. doi: 10.1016/j.dci.2017.10.012
Urbanová, V., Šíma, R., Šauman, I., Hajdušek, O., Kopáček, P. (2015). Thioester-containing proteins of the tick I. ricinus: gene expression, response to microbial challenge and their role in phagocytosis of the yeast Candida albicans. Dev. Comp. Immunol. 48 (1), 55–64. doi: 10.1016/j.dci.2014.09.004
Valenzuela, J. G. (2002). High-throughput approaches to study salivary proteins and genes from vectors of disease. Insect Biochem. Mol. Biol. 32 (10), 1199–1209. doi: 10.1016/s0965-1748(02)00083-8
Valzania, L., Mattee, M. T., Strand, M. R., Brown, M. R. (2019). Blood feeding activates the vitellogenic stage of oogenesis in the mosquito Aedes aegypti through inhibition of glycogen synthase kinase 3 by the insulin and TOR pathways. Dev. Biol. 454 (1), 85–95. doi: 10.1016/j.ydbio.2019.05.011
Vancová, M., Bílý, T., Šimo, L., Touš, J., Horodyský, P., Růžek, D., et al. (2020). Three-dimensional reconstruction of the feeding apparatus of the tick I. ricinus (Acari: Ixodidae): a new insight into the mechanism of blood-feeding. Sci. Rep. 10 (1), 165. doi: 10.1038/s41598-019-56811-2
Vanmechelen, B., Laenen, L., Vergote, V., Maes, P. (2017). Grotenhout virus, a novel nairovirus found in I. ricinus in Belgium. Genome Announc. 5 (21), e00288–00217. doi: 10.1128/genomeA.00288-17
Van Treuren, W., Ponnusamy, L., Brinkerhoff, R. J., Gonzalez, A., Parobek, C. M., Juliano, J. J., et al. (2015). Variation in the microbiota of Ixodes ticks with regard to geography, species, and sex. Appl. Environ. Microbiol. 81 (18), 6200–6209. doi: 10.1128/aem.01562-15
Villar, M., Ayllón, N., Alberdi, P., Moreno, A., Moreno, M., Tobes, R., et al. (2015). Integrated metabolomics, transcriptomics and proteomics identifies metabolic pathways affected by Anaplasma phagocytophilum infection in tick cells. Mol. Cell Proteomics 14 (12), 3154–3172. doi: 10.1074/mcp.M115.051938
Višňovská, D., Pyszko, P., Šigut, M., Kostovčík, M., Kolařík, M., Kotásková, N., et al. (2020). Caterpillar gut and host plant phylloplane mycobiomes differ: a new perspective on fungal involvement in insect guts. FEMS Microbiol. Ecol. 96 (9), fiaa116. doi: 10.1093/femsec/fiaa116
Wagemakers, A., Jahfari, S., de Wever, B., Spanjaard, L., Starink, M. V., de Vries, H. J. C., et al. (2017). Borrelia miyamotoi in vectors and hosts in the Netherlands. Ticks Tick Borne Dis. 8 (3), 370–374. doi: 10.1016/j.ttbdis.2016.12.012
Wagemakers, A., Staarink, P. J., Sprong, H., Hovius, J. W. (2015). Borrelia miyamotoi: a widespread tick-borne relapsing fever spirochete. Trends Parasitol. 31 (6), 260–269. doi: 10.1016/j.pt.2015.03.008
Wang, J., Hu, C., Wu, Y., Stuart, A., Amemiya, C., Berriman, M., et al. (2008). Characterization of the antimicrobial peptide attacin loci from Glossina morsitans. Insect Mol. Biol. 17 (3), 293–302. doi: 10.1111/j.1365-2583.2008.00805.x
Wassermann, M., Selzer, P., Steidle, J. L. M., Mackenstedt, U. (2016). Biological control of I. ricinus larvae and nymphs with Metarhizium anisopliae blastospores. Ticks Tick Borne Dis. 7 (5), 768–771. doi: 10.1016/j.ttbdis.2016.03.010
Wass, L., Grankvist, A., Bell-Sakyi, L., Bergström, M., Ulfhammer, E., Lingblom, C., et al. (2019). Cultivation of the causative agent of human neoehrlichiosis from clinical isolates identifies vascular endothelium as a target of infection. Emerg. Microbes Infect. 8 (1), 413–425. doi: 10.1080/22221751.2019.1584017
Weiser, J., Rehácek, J. (1975). Nosema slovaca sp. n.: a second microsporidian of the tick I. ricinus. J. Invertebr. Pathol. 26 (3), 411. doi: 10.1016/0022-2011(75)90244-x
Weiser, J., Rehácek, J., Zizka, Z., Ciampor, F., Kocianová, E. (1999). Nosema slovaca Weiser et Rehacek 1975 and Unikaryon ixodis [Weiser 1957] comb. n. in ixodid ticks. Acta Parasitol. 44 (2), 99–107.
Wennerås, C. (2015). Infections with the tick-borne bacterium Candidatus Neoehrlichia mikurensis. Clin. Microbiol. Infect. 21 (7), 621–630. doi: 10.1016/j.cmi.2015.02.030
Werren, J. H. (1997). Biology of Wolbachia. Annu. Rev. Entomol 42, 587–609. doi: 10.1146/annurev.ento.42.1.587
Williams-Newkirk, A. J., Rowe, L. A., Mixson-Hayden, T. R., Dasch, G. A. (2014). Characterization of the bacterial communities of life stages of free living lone star ticks (Amblyomma americanum). PLoS One 9 (7), e102130. doi: 10.1371/journal.pone.0102130
Winkhardt, H. J. (1980). Untersuchungen uber den Entwicklungszyklus von Dipetalonema rugosicauda (syn. Wehrdikmansia rugosicauda) (Nematoda; Filarioidea). II. Die Entwicklung von Dipetalonema rugosicauda im Zwischenwirt I. ricinus und Untersuchungen uber das Vorkommen der Mikrofilarien im Reh (Capreolus capreolus). Tropenmedizin und Parasitologie 31 (1), 21–30.
Wójcik-Fatla, A., Zając, V., Sawczyn, A., Cisak, E., Sroka, J., Dutkiewicz, J. (2015). Occurrence of Francisella spp. in Dermacentor reticulatus and I. ricinus ticks collected in eastern Poland. Ticks Tick Borne Dis. 6 (3), 253–257. doi: 10.1016/j.ttbdis.2015.01.005
Woldehiwet, Z. (1983). Tick-borne fever: a review. Vet. Res. Commun. 6 (3), 163–175. doi: 10.1007/bf02214910
Wolff, E. C., Lee, Y. B., Chung, S. I., Folk, J. E., Park, M. H. (1995). Deoxyhypusine synthase from rat testis: purification and characterization. J. Biol. Chem. 270 (15), 8660–8666. doi: 10.1074/jbc.270.15.8660
Yabsley, M. J., Shock, B. C. (2013). Natural history of zoonotic Babesia: Role of wildlife reservoirs. Int. J. Parasitol. Parasites Wildl 2, 18–31. doi: 10.1016/j.ijppaw.2012.11.003
Yang, X., Gao, Z., Zhou, T., Zhang, J., Wang, L., Xiao, L., et al. (2020). Mapping the potential distribution of major tick species in China. Int. J. Environ. Res. Publ. Health 17 (14), 5145. doi: 10.3390/ijerph17145145
Zhang, X. Y., Li, S. S., Chen, K. L., Yang, C., Zhou, X. J., Liu, J. Z., et al. (2022). Growth dynamics and tissue localization of a Coxiella-like endosymbiont in the tick Haemaphysalis longicornis. Ticks Tick Borne Dis. 13 (5), 102005. doi: 10.1016/j.ttbdis.2022.102005
Zhang, C. M., Li, N. X., Zhang, T. T., Qiu, Z. X., Li, Y., Li, L. W., et al. (2017). Endosymbiont CLS-HI plays a role in reproduction and development of Haemaphysalis longicornis. Exp. Appl. Acarol 73 (3-4), 429–438. doi: 10.1007/s10493-017-0194-y
Zhang, X., Norris, D. E., Rasgon, J. L. (2011). Distribution and molecular characterization of Wolbachia endosymbionts and filarial nematodes in Maryland populations of the lone star tick (Amblyomma americanum). FEMS Microbiol. Ecol. 77 (1), 50–56. doi: 10.1111/j.1574-6941.2011.01089.x
Zhong, J. (2012). Coxiella-like endosymbionts. Adv. Exp. Med. Biol. 984, 365–379. doi: 10.1007/978-94-007-4315-1_18
Zhong, J., Jasinskas, A., Barbour, A. G. (2007). Antibiotic treatment of the tick vector Amblyomma americanum reduced reproductive fitness. PLoS One 2 (5), e405. doi: 10.1371/journal.pone.0000405
Zhong, Z., Zhong, T., Peng, Y., Zhou, X., Wang, Z., Tang, H., et al. (2021). Symbiont-regulated serotonin biosynthesis modulates tick feeding activity. Cell Host Microbe 29 (10), 1545–1557.e1544. doi: 10.1016/j.chom.2021.08.011
Zhou, W., Tahir, F., Wang, J. C., Woodson, M., Sherman, M. B., Karim, S., et al. (2020). Discovery of exosomes from tick saliva and salivary glands reveals therapeutic roles for CXCL12 and IL-8 in wound healing at the tick-human skin interface. Front. Cell Dev. Biol. 8, 554. doi: 10.3389/fcell.2020.00554
Keywords: I. ricinus, microbiome, symbiont, pathogen, interactions, vitamin B
Citation: Hodosi R, Kazimirova M and Soltys K (2022) What do we know about the microbiome of I. ricinus? Front. Cell. Infect. Microbiol. 12:990889. doi: 10.3389/fcimb.2022.990889
Received: 10 July 2022; Accepted: 17 October 2022;
Published: 16 November 2022.
Edited by:
Ryan Oliver Marino Rego, Academy of Sciences of the Czech Republic (ASCR), CzechiaReviewed by:
Serhii Filatov, Baylor College of Medicine, United StatesShahid Karim, University of Southern Mississippi, United States
Copyright © 2022 Hodosi, Kazimirova and Soltys. This is an open-access article distributed under the terms of the Creative Commons Attribution License (CC BY). The use, distribution or reproduction in other forums is permitted, provided the original author(s) and the copyright owner(s) are credited and that the original publication in this journal is cited, in accordance with accepted academic practice. No use, distribution or reproduction is permitted which does not comply with these terms.
*Correspondence: Katarina Soltys, katarina.soltys@gmail.com