The application and progress of stem cells in auricular cartilage regeneration: a systematic review
- 1Department of Burn and Plastic Surgery, West China Hospital, Sichuan University, Chengdu, China
- 2Department of Plastic Reconstructive and Aesthetic Surgery, West China Tianfu Hospital, Sichuan University, Chengdu, China
- 3West China Hospital, Sichuan University, Chengdu, China
Background: The treatment of microtia or acquired ear deformities by surgery is a significant challenge for plastic and ENT surgeons; one of the most difficult points is constructing the scaffold for auricular reconstruction. As a type of cell with multiple differentiation potentials, stem cells play an essential role in the construction of cartilage scaffolds, and therefore have received widespread attention in ear reconstructive research.
Methods: A literature search was conducted for peer-reviewed articles between 2005 and 2023 with the following keywords: stem cells; auricular cartilage; ear cartilage; conchal cartilage; auricular reconstruction, regeneration, and reparation of chondrocytes; tissue engineering in the following databases: PubMed, MEDLINE, Cochrane, and Ovid.
Results: Thirty-three research articles were finally selected and their main characteristics were summarized. Adipose-derived stem cells (ADSCs), bone marrow mesenchymal stem cells (BMMSCs), perichondrial stem/progenitor cells (PPCs), and cartilage stem/progenitor cells (CSPCs) were mainly used in chondrocyte regeneration. Injecting the stem cells into the cartilage niche directly, co-culturing the stem cells with the auricular cartilage cells, and inducing the cells in the chondrogenic medium in vitro were the main methods that have been demonstrated in the studies. The chondrogenic ability of these cells was observed in vitro, and they also maintained good elasticity and morphology after implantation in vivo for a period of time.
Conclusion: ADSC, BMMSC, PPC, and CSPC were the main stem cells that have been researched in craniofacial cartilage reconstruction, the regenerative cartilage performed highly similar to normal cartilage, and the test of AGA and type II collagen content also proved the cartilage property of the neo-cartilage. However, stem cell reconstruction of the auricle is still in the initial stage of animal experiments, transplantation with such scaffolds in large animals is still lacking, and there is still a long way to go.
Introduction
Ear deformities can be classified as congenital microtia and acquired trauma, such as injury, burn, or skin cancer excision (Otto et al., 2015; Jessop et al., 2016). Microtia is usually associated with atresia or stenosis with conductive hearing loss (80% of cases) (Mussi et al., 2019). In children, microtia with hearing impairment may be associated with delayed language development, learning difficulty in school, and difficulty interacting with others (Billings et al., 2016; Zhu and Chen, 2016). Deformities and absence of an ear can also lead to negative psychological effects due to esthetic modification of the face, lack of symmetry, differences in the appearance of the ears, and functional issues, for example, wearing glasses. Not by accident, 55% of people with microtia reported low confidence, dissatisfaction, and depression, and 52% of the subjects showed signs of anxiety, which compromised their quality of life (Horlock et al., 2005; Li et al., 2010).
Ear reconstruction continues to be one of the biggest challenges for ENT and plastic surgeons, regardless of whether it involves total auricular reconstruction for congenital microtia or auricular traumatic defect reparation (Wilkes et al., 2014). The auricle is one of the most complex three-dimensional structures in the human body, so being able to construct a satisfactory, complete outer ear has been a difficult goal for many years. In developed countries, there are more than a million patients who undergo some kind of operation involving cartilage reconstruction every year (Chang et al., 2003). However, adult human cartilage shows poor capability for repair and regeneration; at the same time, lack of blood vessels on the surface and inside of the cartilage also limits the survival of the cartilage itself and the skin on the cartilage surface (Dyson et al., 2019).
In recent years, several surgical procedures have been developed for repairing cartilage defects, which highly depend on technique and are limited to small areas of lesions (Ciorba and Martini, 2006). As for huge defects and microtia, simple surgical repair has been unable to meet their therapeutic requirements. The three mainstream treatment strategies are shown as follows: 1) silicone ear prostheses fixed through osseointegrated implants or adhesive; 2) auricular reconstruction with the synthetic material implant; 3) auricular reconstruction with autologous costal cartilage (Narges Baluch et al., 2014).
To enhance the strength of the implanted synthetic materials and the regeneration ability of cartilage, several kinds of stem cells were used in these scaffolds. Since cartilage has a very slow turnover at cellular and molecular levels, it has limited capability for self-renewal and self-repair. Cartilage tissue is complex and consists of chondrocytes and a cartilage-specific extracellular matrix (which is mainly composed of collagens and proteoglycans) (Ciorba and Martini, 2006). Adult stem cells/progenitor cells were first identified by Till and McCulloch (1961). These cells can produce multiline hematopoietic colonies in the spleen. The concept of mesenchymal stem cells (MSCs) equal to adult stem cells was first proposed by Caplan in 1991 (Ai, 1991) based on the early research results of Friedenstein and Gerasimov (1987). However, stem cells did not attract global attention until Pittenger et al. (1999)’s multilineage study found non-hematopoietic stem cells capable of multilineage differentiation. Since the original identification of MSC differentiation, its potential has expanded, (Spencer et al., 2021) and since the initial identification of bone marrow-derived MSC/progenitor cells, MSC/progenitor cells have also been identified in tendon (Bi et al., 2007), articular cartilage (Williams et al., 2010), auricular cartilage (Xue et al., 2016), trachea cartilage (Moshkbouymatin, 2019), ligament (Lee et al., 2019), fat (Rodeheffer et al., 2008), and muscle tissue (Mitchell et al., 2010).
Once the progenitor cells/stem cells have been isolated and expanded, the stem cells need to begin to differentiate into the target tissue. Differentiation into chondrocyte-“like” lineages has been achieved for more than 30 years, and there is a wide range of prospective not only put optimal growth factors but also on the use of mechanical conduction (Humphries et al., 2022). This review is to demonstrate the mechanisms of different kinds of stem cells in auricular reconstruction and auricular cartilage reparation.
Limitations of current techniques
In regular auricular reconstruction surgery, the prevailing gold standard requires three or four autologous costal cartilage segments that were harvested ipsilaterally or contralaterally (Thomson et al., 1995). Because of the poor regeneration ability of cartilage, the integrity and stability of the chest are damaged by such procedures. In these conditions, abnormally shaped ribs move backward under the force of respiratory muscles and negative thoracic pressure, leading to a local depression on the chest, especially in patients in the growth and development period (Park, 1997). Donor site morbidities were reported to be pneumothorax, atelectasis, pleural effusion, etc., at an early stage (Thomson et al., 1995; Kim et al., 2011; Moon et al., 2012), and in the delayed stage, the morbidities were described as persistent pain, thoracic scoliosis, clicking, seroma, abnormal scarring, contour deformity, etc. (Osorno, 1999; Osorno, 2007; Uppal et al., 2008; Moon et al., 2012). Although perioperative procedures are optimized to help reduce donor site morbidities, these problems remain incompletely solved (Walton and Beahm, 2002).
The reconstructed costal cartilage scaffold is different from normal auricular cartilage in terms of its mechanical properties (Brent, 1999). The skin tension of the flap leads to additional morphological distortion, and skin flap necrosis or postoperative infections also cause extrusion of the cartilage scaffold (Brent et al., 1992; Firmin, 1998). In addition, the calcification and resorption of costal cartilage after transplantation result in the stiffness and thickness of the scaffold, along with an indistinct contour and discomposed shape (Berghaus and Toplak, 1986; Firmin, 1998; Brent, 1999; Mori et al., 2002; Walton and Beahm, 2002)
Synthetic materials such as high-density porous polyethylene or Medpor (Porex Surgical, Inc., College Park, GA) were used in clinical treatment to eliminate donor site morbidities and achieve a durable shape. However, the immune response induced by the Medpor scaffold leads to a significantly higher rate of exposure than that of the autologous costal cartilage scaffold (Zhao et al., 2009). In terms of the esthetic outcomes of porous polyethylene and costal cartilage constructs, the former was superior in definition, shape, and size match but inferior in protrusion, location, and color (Constantine et al., 2014).
To decrease the risk of immune rejection, autologous cells are suggested in clinical application of tissue engineering. In auricular reconstruction, regenerating a full-size human auricular scaffold requires over 200 million isolated cells (Reiffel et al., 2013), which is impossible for microtia patients with minimal ear cartilage remnants. In vitro expansion of chondrocytes often results in dedifferentiation, showing an enhanced behavior of fibroblasts (deposition of type I collagen and reduction of cartilage matrix deposition such as type II collagen), which leads to a significant reduction in cartilage elasticity and mechanical strength (Schnabel et al., 2002; Mandl et al., 2004; Bichara et al., 2012; Reiffel et al., 2013; Cohen et al., 2016). Additionally, a 3D construct culture is required prior to implantation of monolayer containing expanded chondrocytes (Zhou et al., 2018).
Therefore, inducing the MSCs or progenitor cells (PCs) into chondrocytes is expected to alleviate the burden of microtia chondrocyte requirement (Yamamoto et al., 2004; McCorry et al., 2016; McCorry and Bonassar, 2017). MSC-derived auricular scaffolds should be paid attention to as a development trend for ear reconstruction in the future.
Methods
PubMed, MEDLINE, Cochrane, and Ovid databases were searched from 2005 to 2023 using the following key terms: stem cells; auricular cartilage; ear cartilage; conchal cartilage; auricular reconstruction, regeneration, and reparation of chondrocytes; tissue engineering. Across these databases, these search terms produced 729 results. After removing the duplicates, 662 results were reserved, and then we excluded those articles that were not in English and where the main interest in cartilage was not auricular. Inclusion criteria were studies focused on stem cell application in auricular reconstruction. Titles were screened and removed if not relevant. Abstracts were then screened and taken forward for full-text review, if appropriate. Finally, 33 articles focused on the topic were included in this review (Figure 1).
Stem cell types in chondrogenesis
Thirty-three research articles were selected, and the main characteristics were summarized. Four sorts of mainstream stem cells, i.e., adipose-derived stem cells, bone marrow mesenchymal stem cells, perichondrial stem/progenitor cells, and cartilage stem/progenitor cells, were generally applied to reconstruct the auricular cartilage. Furthermore, different cultures and inducement methods can present different results of chondrogenesis.
Adipose-derived stem cells
Adipose tissue is derived from the mesoderm during embryonic development and is present in all mammals throughout the body. Although two types of adipose tissue (brown and white) exist, white adipose tissue gives rise to the commonly studied ADSCs. The methods for isolating ADSCs include enzymatic digestion, mechanical separation, and tissue adherence. Enzymatic digestion is the most commonly used method, which uses various enzymes such as collagenase, trypsin, and hyaluronidase to digest the adipose tissue and isolate ADSCs (Minteer et al., 2013). ADSCs have multipotent differentiation potential and can differentiate into osteoblasts, chondrocytes, myocytes, and adipocytes. In addition, they have self-renewal and proliferation abilities and have a wide range of applications. Commonly used markers for ADSCs include CD29, CD44, CD73, CD90, and other mesenchymal stem cell markers, as well as CD34, CD45, and other markers for vascular endothelial and hematopoietic stem cells. These markers can help identify and purify ADSCs (Patricia et al., 2002).
According to the former study, it demonstrated that ADSCs are relatively easy to obtain (from adipose tissue) and to culture, and their extraction is less invasive in comparison with BMMSCs. (Raghunath et al., 2005). Based on these characteristics, ADSCs may represent an excellent source for cell therapy (Patricia et al., 2002; Yamamoto et al., 2007). The main characteristics of the studies focused on ADSC differentiation are analyzed and reported in Table 1.
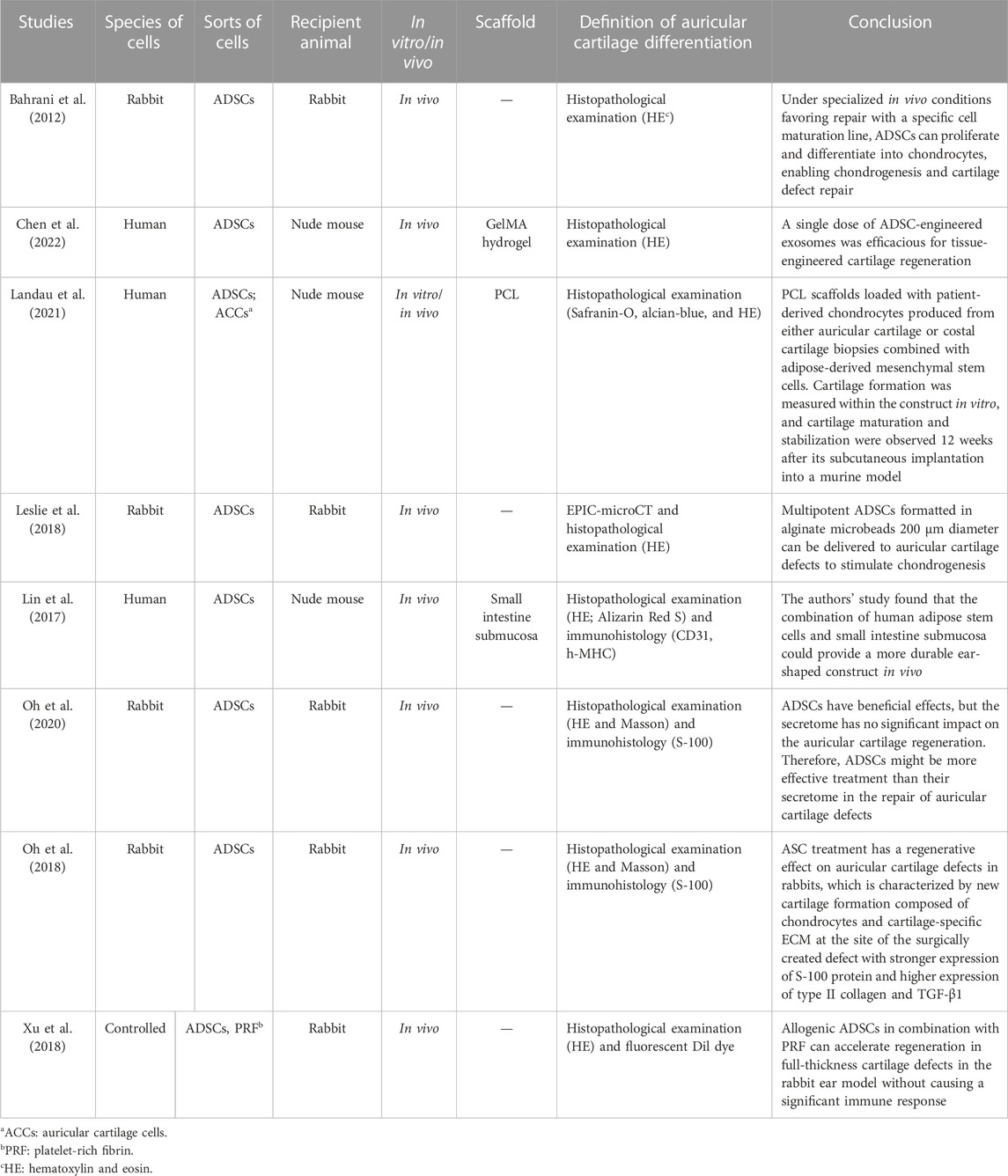
TABLE 1. Main characteristics of the eight studies included in the review on adipose-derived stem cells.
As we already know, ADSCs have multi-directional differentiation potential, and in different environments, they can be induced into different kinds of cells. ADSCs have been identified as novel potential candidates for the reconstruction of cartilage defects in vivo. In Bahrani’s study, rabbit ADSCs were isolated and cultured to passage 3; these stem cells were harvested and injected into the area where the auricular cartilage was removed by surgery, and then they found that the defects were repaired by neo-cartilage, which was differentiated from ADSCs (Bahrani et al., 2012). In another study, Leslie et al. (2018) found that rabbit ADSCs in alginate microbeads could regenerate auricular cartilage when they were placed in the auricular defect areas, but were not fully integrated with the surrounding tissues. In chondrogenic media, ADSCs expressed mRNAs for aggrecan (AGA), type II collagen, and type X collagen, which means these newborn cells have the characteristics of ear cartilage. Applying the same condition, Landau et al. (2021) used the 3D-printed clinical-grade polycaprolactone scaffold loaded with patient-derived chondrocytes generated from auricular cartilage or costal cartilage biopsy combined with ADSCs. Chondrogenesis potential was measured in vitro, and cartilage maturation and stability were observed 12 weeks after subcutaneous implantation in the mouse model. In the study by Se-Joon Oh et al. (2020), published in 2018 (57), rabbit ADSCs were injected into rabbit auricular defects; after 1 month, histopathology showed islands of new cartilage formation at the site defects. At the same time, the expressions of collagen type II and TGF-β1 were significantly higher in the ADSCs than in the blank control group. Except for this, in the other research studies, they contrasted the therapeutic efficacies of ADSCs and their secretome in terms of rabbit auricular cartilage regeneration, and they found that ADSCs could significantly enhance new cartilage formation, but their secretome did not. Therefore, ADSCs may be more effective in the repair of auricular cartilage defects. In the aforementioned studies, the main mechanism of ADSC differentiation into ear cartilage is that ADSCs can differentiate into ear cartilage in a large amount in a cartilage environment or chondrogenic medium.
However, in Chen et al.’s (2022) study, in contrast to Se-Joon Oh’s study, they isolated and purified the exosomes from human ADSCs, co-cultured them with microtia chondrocytes in Gelma hydrogel, and then implanted the tissue-engineered cartilage into subcutaneous pockets of nude mice for 6 weeks. Finally, they found that a single dose of Engineered exo was efficacious for tissue-engineered cartilage regeneration. Engineered exo effectively promoted the proliferation, survival, and mature cartilage formation of microtia chondrocytes through the hsa-miR-23a-3p/PTEN/PI3K/AKT/mTOR axis. They also designed Engineered exo by directly transfecting agomir-23a-3p into parental passage 4 ADSCs to isolate exosomes enriched with hsa-mir-23a-3p and optimize favorable effects on the cell viability and new cartilage formation.
In addition to direct injection of ADSCs into the cartilage site and culturing of ADSCs in chondrogenic medium, Lin et al. (2017) cultured and induced human ADSCs in the small intestine submucosa scaffold, which differentiated into osteocytes, but not chondrocytes. The ear-shaped human ADSCs/small intestine submucosa construct could maintain the shape in vivo for up to 1 year; angiogenesis was evident in human ADSCs/small intestinal submucosal structures at 6 months and persisted for 1 year; and the mechanical properties were highly equal to those of the natural auricular cartilage. Xu et al. (2018) evaluated the efficacy of allogeneic ADSCs combined with platelet-rich fibrin (PRF) in the treatment of ear cartilage defects in rabbits and found that ADSCs/PRF could promote the regeneration of full-thickness cartilage defects in rabbit ears without causing an obvious immune response. The results showed that allogeneic ADSCs plus PRF could be successfully used for cartilage regeneration.
Bone marrow mesenchymal stem cells
BMMSCs are a type of multipotent stem cells that were first discovered in the 1970s. BMMSCs can differentiate into various cell types, including osteoblasts, chondrocytes, adipocytes, and myocytes (Prockop, 1997). BMMSCs also produce many biologically active molecules, such as cytokines, growth factors, and extracellular matrix, which have important biological functions, such as stimulating cell proliferation, repairing tissue damage, and anti-inflammatory effects. The isolation methods of BMMSCs mainly include the adherent method and the density gradient centrifugation method. The adherent method refers to culturing bone marrow cells in plastic culture bottles, allowing MSCs to adhere to the bottle wall, and then removing non-adherent cells to leave MSCs. The density gradient centrifugation method separates MSCs from other cells by centrifugation in a density gradient centrifuge tube (Bianco et al., 2008). The markers of BMMSCs include CD73, CD90, and CD105. These markers are surface markers of MSCs and the main identification criteria for MSCs. In addition, BMMSCs also express some embryonic stem cell markers, such as Oct-4 and Nanog, indicating that BMMSCs have stem cell characteristics (Dominici et al., 2006; Jia et al., 2018).
In our review, BMMSCs might show better effects than ADSCs in auricular cartilage regeneration, and more research was conducted in the direction of BMMSCs. BMMSCs are one of the most important cells for repairing cartilage defects in vivo and have the characteristics of multipotent differentiation. In vitro, BMMSCs can be purified and cultured, have a durable phenotype and cellularity, and induce chondroblasts to secrete the cartilage matrix (Erickson et al., 2002; Jiang et al., 2002; Solchaga et al., 2004; Wagers and Weissman, 2004). The main characteristics of the studies focused on BMMSC differentiation are analyzed and reported in Table 2.
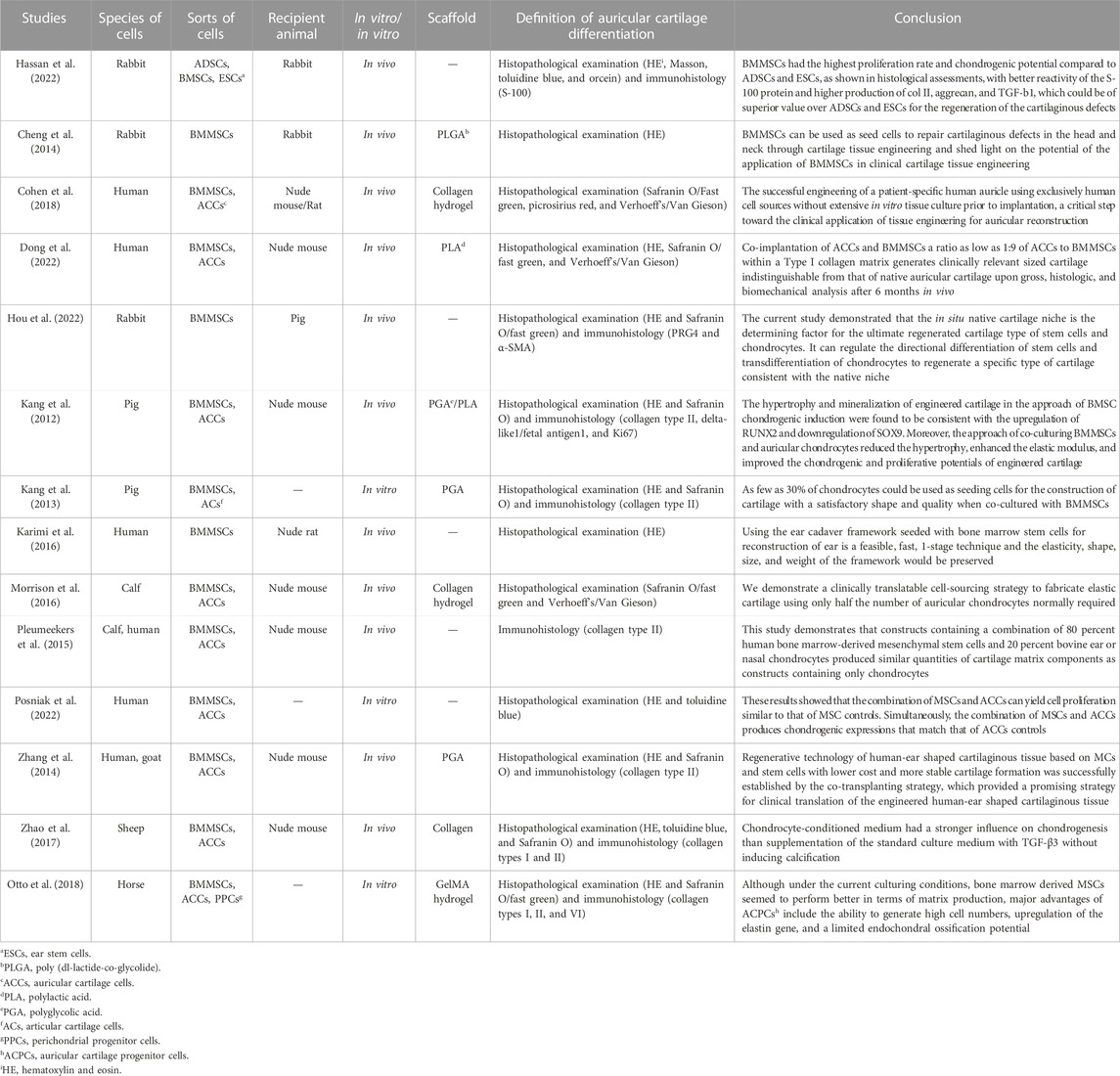
TABLE 2. Main characteristics of the 14 studies included in the review on bone marrow mesenchymal stem cells.
Although BMMSCs can differentiate into a variety of cells, they need certain circumstances that can cause their induction in target cells. In many studies, auricular cartilage cells were co-cultured with BMMSCs to obtain a significant number of cartilage cells for auricular reconstruction. In Zhang et al. (2014)’s study, they harvested and co-cultured the human microtia cartilage cells (MCs) and goat BMMSCs and implanted the cells and ear-shaped scaffolds in nude mice. After 12 weeks, a human-ear-shaped cartilaginous tissue with delicate structure and proper elasticity was successfully constructed. It shows that BMMSCs co-cultured with MCs would require fewer cartilage cells and construct a more stable scaffold than before. Moreover, the same results were also demonstrated in Karimi et al. (2016) and Morrison et al. (2016); they concluded that such an innovative cell sourcing strategy facilitates the efforts to achieve clinical translation of high fidelity. Kang et al. (2012); Kang et al. (2013) also co-cultured BMMSCs and chondrocytes in different ratios, and finally, the study found that 30% of chondrocytes are required to generate cartilage tissue of satisfactory shape and quality at least, and the MC: BMMSC ratio of 5:5 showed the highest Young’s modulus and the densest elastic fibers, which were consistent with the expression of DCN and LOXL2 genes (cartilage matrix-related genes). Nevertheless, in the study by Dong et al. (2022), they co-cultured human MCs and human BMMSCs and efficiently produced well-shaped human elastic cartilage without volume loss, even when human MCs accounted for only 10% of the total number of transplanted cells. In Cohen et al. (2018)’s study, they also found that BMMSCs and MCs co-cultured in a 1:1 ratio appeared as bundles of collagen fibers in the perichondrial layer, rich in proteoglycan deposits, forming an elastin fiber network similar to natural human ear cartilage, with the protein composition and mechanical stiffness of natural tissue. In Posniak et al. (2022)’s and Dong et al.’s study, they concluded that BMMSCs applied to replace auricular cartilage alleviate the requirement for large cartilage biopsies, which would otherwise be needed for sufficient cell numbers. To analyze the contribution of different cells in the co-culture system, Pleumeekers et al. (2015) used a xenogeneic co-culture system that included human BMMSCs and bovine ear chondrocytes or nasal chondrocytes in an 80:20 ratio. Based on these conditions, BMMSCs were found to play a trophic role in the co-culture system because aggrecan was expressed only by the chondrocytes.
Another strategy to obtain large-volume auricular chondrocytes is to induce BMMSCs in a chondrocyte induction medium (CM). BMMSCs were isolated from living bodies and amplified in CM in vitro. Cheng et al. (2014) confirmed these cells as chondrocytes and then implanted the cells onto a poly (D-L-lactide-co-glycolide) (PLGA) scaffold. After being cultured in vivo for 18 weeks, gross observation indicated that the cartilaginous defects were completely repaired by chondrocytes with smooth surfaces and similar color to the surrounding tissue. In Otto et al. (2018)’ s study, they cultured the BMMSCs in gelatin methacryloyl (gelMA). BMMSCs outperformed other cartilage-derived cell types in terms of matrix production and mechanical properties. Zhao et al. (2017) compared the different influences of BMMSCs in standard culture medium (SM) and CM for inducing BMMSCs. After amplifying for three passages in vitro, the cells were transplanted onto fibrous collagen scaffolds and precultured for 2 weeks, with or without transforming growth factor-beta 3 (TGF-β3). As shown in the results, after 12 weeks of in vivo culture, COL2A1 expression was upregulated in the CM compared to SM, and abundant neocartilage formation was observed in the implants that had been cultured in the CM, with or without TGF-β3. However, little cartilage matrix formation was observed in the SM group, regardless of the presence or absence of TGF-β3. It means that the effect of the CM on chondrogenesis was even stronger than that of the SM supplemented with TGF-β3, and there was no sign of endochondral osteogenesis.
For auricular cartilage reparation by injecting BMMSCs into the cartilage defect areas, Hassan et al. (2022) found that the auricle defects of the BMMSC group appeared completely healed with smooth surfaces and similar tissue color, and the treatment effects of BMMSCs were even better than those of ADSCs in their study. To research whether the anatomical location of cartilage could influence the differentiation of BMMSCs, Hou et al. (2022) transplanted the BMMSCs into native auricular and articular cartilage niches and found that the native cartilage niches were able to regulate BMMSC regeneration of elastic and hyaline cartilage despite the type of transplanted cartilage in these niches.
Perichondrial stem/progenitor cells
In spite of ADSCs and BMMSCs, another kind of stem cells was also experimented by researchers, which could be isolated from perichondrial named PPCs. Unlike CSPCs, the perichondrium is vascularized and innervated.
PPCs within the perichondrium have been described as proliferating more rapidly than mature chondrocytes and being able to differentiate into other mesenchymal tissues under specific conditions (Togo et al., 2006). PPCs are multipotent stem cells derived from the perichondrium, with good proliferation and differentiation potential, capable of differentiating into chondrocytes, osteoblasts, myocytes, adipocytes, and other cell types (Ho et al., 2022). The isolation of perichondrial stem/progenitor cells is mainly achieved by mechanical separation and enzymatic digestion methods, such as enzymatic digestion and collagenase digestion. PPCs can differentiate into multiple cell types and have good proliferation and differentiation potential. They can be cultured for a long time in vitro and in vivo. The markers of the cells include CD105, CD90, CD73, and other mesenchymal stem cell markers, as well as CD146, CD271, Stro-1, and other stem cell markers. In addition, they also express some chondrocyte-related markers such as Sox9 and Col2a1 (Arai et al., 2002).
Compared with BMMSCs, auricular cartilage PPCs are easily separated from a donor site without ectopic tissue formation, such as calcifications or fibrous tissue formation. The main characteristics of the studies concentrating on the PCC differentiation analyzed are reported in Table 3.
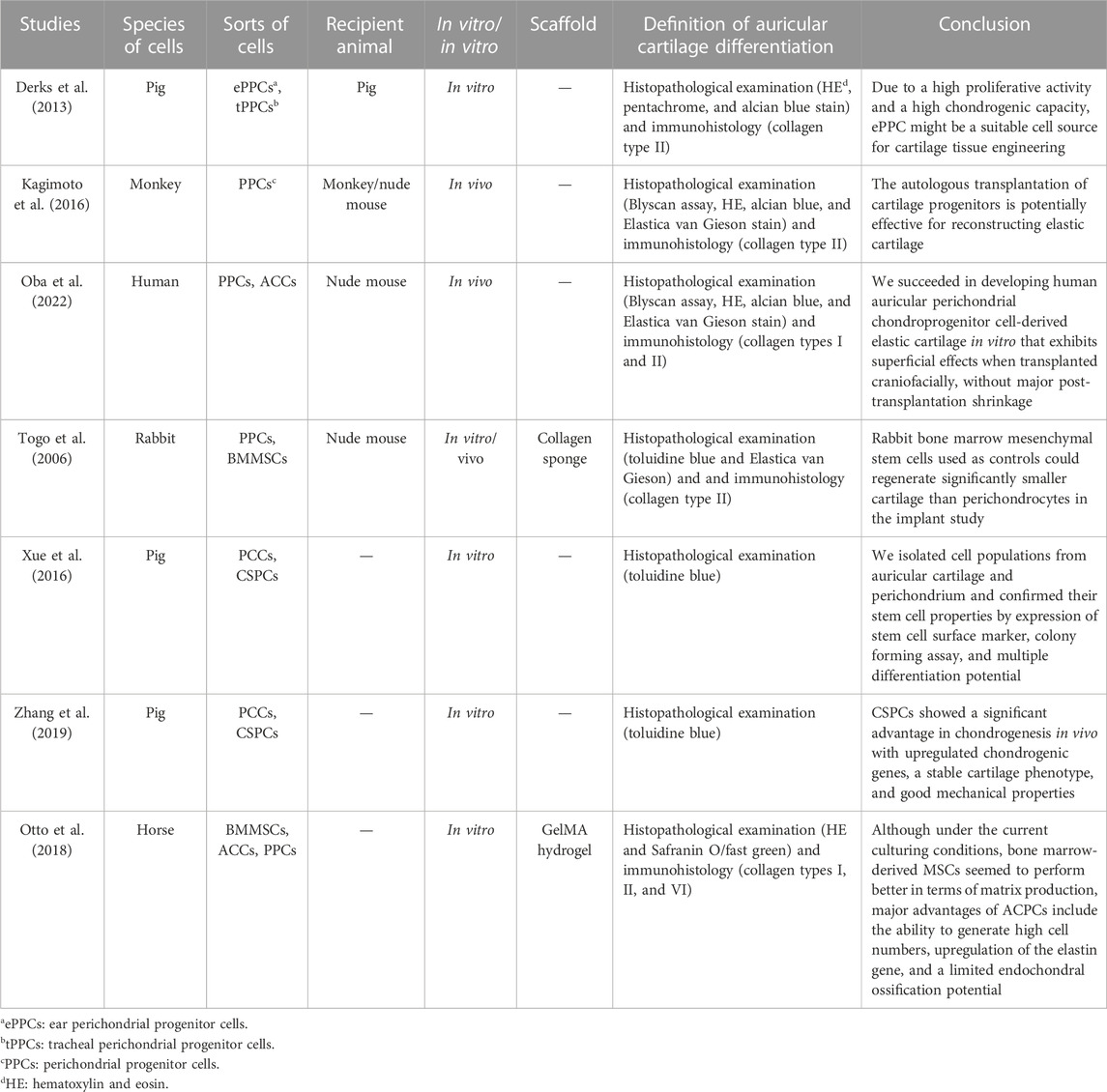
TABLE 3. Main characteristics of the seven studies included in the review on perichondrial stem/progenitor cells.
The presence of progenitor cells in the auricular perichondrial was first proved in Togo et al. (2006)’s study; they compared the adipogenic and osteogenic ability of rabbit PPCs, cartilage stem cells (CSCs), and BMMSCs, and progenitor cells and stem cells were implanted into the dorsum of nude mice with a collagen sponge scaffold. The results demonstrated that the adipogenic and osteogenic ability of PPCs and CSCs is equal to that of BMMSCs, and PPCs are superior to MSCs for cartilage reconstruction in vivo. In addition, both PPCs and CSCs could produce sulfated glycosaminoglycan and collagenous components and maintain a non-calcified phenotype in the reconstructed cartilage. In Derks et al. (2013)’s study, porcine ear perichondrial progenitor cells (ePPCs), tracheal perichondrial progenitor cells (tPPCs), and BMMSCs were compared; the cells were induced in CMs for 4 weeks; and the results indicated that the expressions of collagen II, aggrecan, and cartilage oligomeric matrix protein in ePPCs are higher than those of tPPCs and BMMSCs. However, the expression of collagen I was comparable in all cell types, which showed that due to their higher chondrogenic potential and accessibility, ePPCs may be more convenient than tPPCs. Meanwhile, after comparing the differentiation ability of PPCs and CSCs, Xue et al. (2016) and Zhang et al. (2019) found that the cells differentiate into osteogenic lines, chondrogenic lines, and adipogenic lines under different induction conditions, and the preformation in these aspects of PPCs was better.
In Kagimoto et al. (2016)’s research, they verified that monkey PPCs could be induced into chondrocytes in vitro and regenerated into elastic cartilage by xenotransplantation into a nude mouse. For autologous transplantation, the monkey progenitor cells were developed into mature elastic cartilage in the subcutaneous region of a craniofacial section.
Since the formation of morphologically stable scaffold-free elastic cartilage tissue is challenging, Oba et al. (2022) developed a method for in vitro scaffold-free cartilage reconstruction. The use of human auricular PPCs significantly increased the potential for chondrogenesis by inducing chondrogenesis using microspheres similar to the ear colliculus. After craniofacial transplantation in nude mice, the size and elasticity of the reconstructed tissue remained unchanged, indicating that the reconstructed tissue was morphologically stable.
Cartilage stem/progenitor cells
CSPCs are a type of stem cells found in human and animal ear cartilage, with the potential to differentiate into chondrocytes (Dowthwaite et al., 2004). The commonly used isolation methods for CSPCs include mechanical separation, enzymatic digestion, and magnetic bead sorting. Enzymatic digestion is currently the most commonly used method. By cutting ear cartilage tissue into small pieces, adding digestion enzymes (such as collagenase, pronase, or trypsin), and performing digestion on a constant temperature shaker at 37°C, single cells can be obtained. CSPCs have a differentiation ability that mainly tends toward chondrocytes. Studies have shown that, through appropriate inducers and culture conditions, CSPCs can differentiate into chondrocytes and synthesize cartilage matrix (Zucchelli et al., 2020). There is currently no unified standard for CSPC markers. However, researchers have discovered some markers associated with CSPCs, such as CD44 and CD90. The expression of these markers can help researchers identify and purify CSPCs (Kobayashi et al., 2011).
The main characteristics of the studies concentrating on CSPC differentiation analyzed are reported in Table 4.
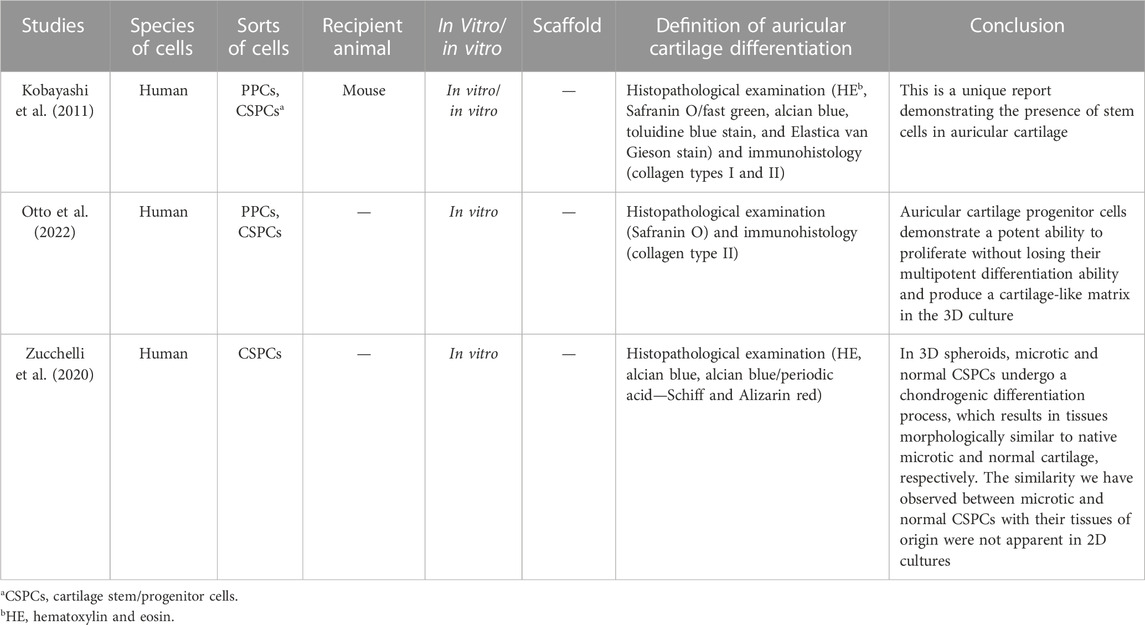
TABLE 4. Main characteristics of the three studies included in the review on cartilage stem/progenitor cells.
In 2011, Kobayashi et al. first reported the presence of stem cells in auricular cartilage; they acquired the cells from human auricular perichondrium, and after the clonogenic progeny of a single CD44+ and CD90+, CSPCs demonstrated several features of stem cells (Kobayashi et al., 2011). Otto et al., 2022 isolated the cartilage stem/progenitor cells and cultured them in the 3D gelatin-based hydrogel in vitro, with subsequent biochemical, mechanical, and histological analyses. Auricular CSPCs showed strong proliferative capacity in 3D culture without losing their multipotent differentiation capacity and cartilage-like matrix production.
Zucchelli et al. (2020)’s study showed that in 3D spheroids, microtia and normal CSPCs underwent a chondrogenic differentiation process, which resulted in tissue morphology similar to that of native microscopic and normal cartilage, respectively. The discovery of CSPCs provided a new direction for stem cell differentiation; researchers can use the CSPCs from microtia cartilage to construct the cartilage scaffold for auricular reconstruction without any other extra resections.
In spite of the cells we previously demonstrated, another kind of cell was also used in auricular reparation. Eslaminejad and Bordbar (2012) found the blastema cells in rabbit ears and compared them with the BMMSCS. In adipogenic, osteogenic, and chondrogenic cultures, blastema cells expressed more lineage-specific genes than BMMSCs. They also multiply faster than BMMSCs in vitro.
Stem cell differentiation method
Microtia is a significant challenge for plastic surgeons. In the past few decades, the use of the costal cartilage scaffold for auricular reconstruction has been dominant in such procedures. Nevertheless, the patients always have to suffer extra surgical incisions when harvesting the costal cartilage. The scaffold material for auricular reconstruction was very limited before application of the synthetic material. However, the synthetic material sometimes performed poorly (with low biocompatibility) in clinical applications; hence, the research to increase the biocompatibility of the scaffold material is becoming more and more vigorous. In recent years, the application of tissue engineering technology in reconstruction surgeries has increased dramatically. MSC/progenitor cells combined with or without those scaffold materials are being researched for producing durable ear cartilage replacements that conform to the functional and esthetic characteristics of the normal auricular function (Cao et al., 1997; Haisch et al., 2002; Shieh et al., 2004). To maintain the correct shape and elasticity of the tissue engineering scaffold after insertion under the skin, different strategies associated with culturing stem cells were invented.
Three main methods of stem cell differentiation were demonstrated in the studies we reviewed. The MSCs were injected directly into the cartilage niche to repair the defects of the auricular, where they were induced to differentiate into auricular cartilage in the physiological environment in vivo. Such kinds of cells can replicate and differentiate into different cell types, and the chondrogenic capacity of MSCs was improved (Raghunath et al., 2005; Robey and Bianco, 2006), and high-quality ear cartilage was formed in vivo (Bahrani et al., 2012). In this condition, chondrocyte growth was observed to go through a sequential phase from new, immature cartilage islands to mature, physically palpable cartilage plates, mimicking the formation of normal embryonic cartilage in many parts of the body, such as the auricle and nose. Although MSCs were observed to differentiate into auricular cartilage in the defective area of the ear in animal models, different kinds of MSCs also presented different capacities of chondrogenesis. In comparison, the researchers demonstrated that BMMSCs were superior to other stem cells in differentiation capacity (Chen et al., 2015; Oh et al., 2018; Hassan et al., 2022). BMMSCs are a representative cell source that promotes wound healing in multiple ways and develops into effector cells involved in angiogenesis, ECM formation, wound contraction, re-epithelialization, and matrix secretion (Hassan et al., 2022). This phenomenon was also observed in experiments in which stem cells were injected into cartilage defects. Compared to other induction methods, injecting MSCs directly into defect areas seems easier to implement, and since the regenerated cartilage only presents the properties of cartilage, it is not possible to further study the shape, quality, and support of the neo-cartilage.
The second main method of MSC induction was to co-culture the stem cells with the auricular cartilage cells in vitro. The results of co-culturing could be observed directly. In a further study, the mature cells were transplanted subcutaneously into animals for further in vivo observation. Since microtia cartilage is very limited in human beings and the development of microtia cartilage is congenital-insufficient, it is not enough to use microtia chondrocytes as the basis for ear cartilage culture. The microtia cartilage cells were isolated and co-cultured with MSCs. On one hand, those MSCs were induced into chondrocytes in a particular condition; on the other hand, a significant number of MSCs served as a complement to chondrocytes, increasing the total number of cells. In recent years, the co-culture of BMMSCs and chondrocytes has been developed to induce BMMSCs to form cartilage and inhibit cartilage hypertrophy (Yang et al., 2009; Fischer et al., 2010). The co-culture model also reduces the use of chondrocytes and makes it possible to obtain small pieces of cartilage for the repair of large defects. Comparison of the in vitro co-culture model with the BMMSC induction system alone showed that the co-culture of BMMSCs and chondrocytes reduced hypertrophy of tissue-engineered cartilage while enhancing its functional properties (Bian et al., 2011). After the co-culture model treatment, the cells were attached to the PLA/PCA scaffold, and the cartilage was formed in vitro and implanted into the subcutaneous tissue of the animal. After a period of observation, the elasticity and shape of the scaffold were well-maintained (Kang et al., 2012; Kang et al., 2013; Cheng et al., 2014; Zhang et al., 2014; Morrison et al., 2016; Zhao et al., 2017; Cohen et al., 2018; Dong et al., 2022). The results demonstrated that the co-culture model provided a high-quality strategy for auricular cartilage regeneration with significant potential for tissue-engineered auricular reconstruction.
Another method of stem cell induction was to culture the cells in a chondrogenic medium; the cells were induced in a particular medium in vitro, and some growth factors, antibiotics, and dexamethasone are added precisely to it (Cheng et al., 2014; Kagimoto et al., 2016). The MSCs could be induced into chondrocytes in these media; however, most of the chondrogenic ability of the cells was observed in vitro, although they showed good chondrogenic effects in vitro (Cheng et al., 2014; Kagimoto et al., 2016; Zhao et al., 2017; Otto et al., 2018). In a few in vivo experiments, Otto and Derks et al. found that cartilage induced in vitro could also show good morphology and type II collagen content after being implanted in animals for a period of time (Derks et al., 2013; Zhao et al., 2017); allogeneic stem cell-induced chondrocytes have also been studied as seed cells; the composite of functional chondrocytes and novel scaffolds can produce cartilage tissue after transplantation in vivo, due to the low immunity of cartilage; and the immune rejection of allogeneic functional cartilage transplantation is also weakened by the digestion, isolation, induction, in vitro culture, and carrier implantation of cartilage surface antigens. However, given that in vivo experiments are still in the preliminary stage of exploration, the in vivo transplantation effect of cartilage scaffolds prepared by this cartilage induction method needs to be further studied.
In addition to the aforementioned common induction methods, in recent years, stem cell exosomes, especially those derived from ADSCs, have been found to promote the differentiation of stem cells. Exosomes derived from tissue engineering can effectively promote the proliferation of microtia chondrocytes and the differentiation of mature cartilage (Chen et al., 2022). As a “cell-to-cell” messenger, ADSC exosomes have distinct characteristics and significant application potential in tissue regeneration by encapsulating various types of bioactive carriers. It can mechanistically play a role in different tissues by repairing specific functions such as cell migration and proliferation and promoting the formation of new blood vessels (Xiong et al., 2020). The research on stem cell-derived exosomes promoting ear cartilage regeneration and auricle reconstruction is a novel research area, which is more commonly used in wound healing, fat grafting, and articular cartilage reconstruction. The characteristics of stem cell exosomes that promote cell differentiation also provide direction for the future study of cell differentiation in ear reconstruction.
In the studies we reviewed, ADSCs, BMMSCs, PPCs, and CSPCs were the main stem cells that have been researched in craniofacial cartilage reconstruction, and each cell presented well-defined effects. The ADSCs were easily harvested and abundantly available in the body, given their well-known multipotent differentiation potential and the promoting effect of their exosomes on chondrogenic differentiation. ADSCs are a very important alternative stem cell in ear cartilage reconstruction. The BMMSCs also have multipotent differentiation ability and performed well in the chondrogenic assay of allografts. In the research comparing different MSCs’ chondrogenic abilities, BMMSCs were found to have the optimal chondrogenic capacity, as measured by cartilage morphology, elasticity, and AGA and collagen II content. PPCs and CPSCs are easily isolated from a donor site without ectopic tissue formation, such as calcifications or fibrous tissue formation, and have the advantage that they can be harvested in situ from the microtia without additional incisions. They also play an important role in chondrogenic differentiation, are more chondrogenic than ADSCs and BMMSCs, and have a good prospect in auricle reconstruction.
Conclusion and prospects
In conclusion, auricle reconstruction is a difficult task, and recent advances in biological tissue engineering, and collaborations between stem cell biologists and clinicians, offer an opportunity for auricular cartilage constructs that resemble the human ear in shape, size, and flexibility. At present, stem cell reconstruction of the auricle is still in the initial stage of animal experiments, and transplantation experiments with such scaffolds in large animals are still lacking. Inducing MSCs to differentiate into chondrocytes to construct an auricular scaffold and implanting it subcutaneously in large animals for long-term in vivo experiments should be the future research direction. At the same time, scaffolds carrying chondrocytes should be further screened for future research. There is still a long way to go to realize stem cell reconstruction of cartilage scaffolds instead of autologous materials and apply it to clinical practice.
Author contributions
YL, WW, CS, and ZL contributed equally to this article and should be considered co-first authors. YL: conceptualization, data curation, and writing—original draft and submission. WW: conceptualization, data curation, and writing—reviewing and editing. CS: conceptualization and writing—reviewing and editing. ZL: conceptualization and writing—reviewing and editing. YH: data curation and writing—reviewing and editing. KZ: data curation and writing—reviewing and editing. BW: data curation. ZC: data curation and writing—reviewing and editing. ZZ: conceptualization, writing—reviewing and editing, and supervision. All authors revised and approved the article and then agreed to be accountable for all aspects of the work. All authors contributed to the article and approved the submitted version.
Funding
This study was funded by the National Natural Science Foundation of China (NSFC) (81871574); the Sichuan Natural Science Foundation (2022NSFSC0717; 2022NSFSC1579; and 2022YFS0199); and 135 project for disciplines of excellence, West China Hospital Sichuan University (ZYPY20003 and ZYPY20004).
Conflict of interest
The authors declare that the research was conducted in the absence of any commercial or financial relationships that could be construed as a potential conflict of interest.
Publisher’s note
All claims expressed in this article are solely those of the authors and do not necessarily represent those of their affiliated organizations, or those of the publisher, the editors, and the reviewers. Any product that may be evaluated in this article, or claim that may be made by its manufacturer, is not guaranteed or endorsed by the publisher.
References
Arai, F., Ohneda, O., Miyamoto, T., Zhang, X. Q., and Suda, T. (2002). Mesenchymal stem cells in perichondrium express activated leukocyte cell adhesion molecule and participate in bone marrow formation. J. Exp. Med. 195 (12), 1549–1563. doi:10.1084/jem.20011700
Bahrani, H., Razmkhah, M., Ashraf, M. J., Tanideh, N., Chenari, N., Khademi, B., et al. (2012). Differentiation of adipose-derived stem cells into ear auricle cartilage in rabbits. J. laryngology otology 126 (8), 770–774. doi:10.1017/S0022215112001065
Berghaus, A., and Toplak, F. (1986). Surgical concepts for reconstruction of the auricle. History and current state of the art. Arch. Otolaryngol. Head. Neck Surg. 112 (4), 388–397. doi:10.1001/archotol.1986.03780040028007
Bi, Y., Ehirchiou, D., Kilts, T. M., Inkson, C. A., Embree, M. C., Sonoyama, W., et al. (2007). Identification of tendon stem/progenitor cells and the role of the extracellular matrix in their niche. Nat. Med. 13 (10), 1219–1227. doi:10.1038/nm1630
Bian, L., Zhai, D. Y., Mauck, R. L., and Burdick, J. A. (2011). Coculture of human mesenchymal stem cells and articular chondrocytes reduces hypertrophy and enhances functional properties of engineered cartilage. Tissue Eng. Part A 17 (7-8), 1137–1145. doi:10.1089/ten.TEA.2010.0531
Bianco, P., Robey, P. G., and Simmons, P. J. (2008). Mesenchymal stem cells: Revisiting history, concepts, and assays. Cell Stem Cell 2 (4), 313–319. doi:10.1016/j.stem.2008.03.002
Bichara, D. A., O'Sullivan, N. A., Pomerantseva, I., Zhao, X., Sundback, C. A., Vacanti, J. P., et al. (2012). The tissue-engineered auricle: Past, present, and future. Tissue Eng. Part B Rev. 18 (1), 51–61. doi:10.1089/ten.TEB.2011.0326
Billings, K. R., Qureshi, H., Gouveia, C., Ittner, C., and Hoff, S. R. (2016). Management of hearing loss and the normal ear in cases of unilateral Microtia with aural atresia. Laryngoscope 126 (6), 1470–1474. doi:10.1002/lary.25530
Brent, B., Tanzer, R. C., Rueckert, F., and Brown, F. E. (1992). Auricular repair with autogenous rib cartilage grafts: Two decades of experience with 600 cases. Plast. Reconstr. Surg. 90 (3), 375–376. doi:10.1097/00006534-199209000-00002
Brent, B. (1999). Technical advances in ear reconstruction with autogenous rib cartilage grafts: Personal experience with 1200 cases. Plast. Reconstr. Surg. 104 (2), 319–334; discussion 335-338. doi:10.1097/00006534-199908000-00001
Cao, Y. V. J., Paige, K. T., Upton, J., and Vacanti, C. A. (1997). Transplantation of chondrocytes utilizing a polymer-cell construct to produce tissue-engineered cartilage in the shape of a human ear. Plast. Reconstr. Surg. 100 (2), 297–302; discussion 303-304. doi:10.1097/00006534-199708000-00001
Chang, S. C., Tobias, G., Roy, A. K., Vacanti, C. A., and Bonassar, L. J. (2003). Tissue engineering of autologous cartilage for craniofacial reconstruction by injection molding. Plast. Reconstr. Surg. 112 (3), 793–799; discussion 800-801. doi:10.1097/01.PRS.0000069711.31021.94
Chen, J., Huang, T., Liu, R., Wang, C., Jiang, H., Sun, H., et al. (2022). Congenital microtia patients: The genetically engineered exosomes released from porous gelatin methacryloyl hydrogel for downstream small RNA profiling, functional modulation of microtia chondrocytes and tissue-engineered ear cartilage regeneration. J. Nanobiotechnology 20 (1), 164–169. doi:10.1097/BS9.0000000000000121
Chen, S., Shi, J., Zhang, M., Chen, Y., Wang, X., Zhang, L., et al. (2015). Mesenchymal stem cell-laden anti-inflammatory hydrogel enhances diabetic wound healing. Sci. Rep. 5, 18104. doi:10.1038/srep18104
Cheng, Y., Cheng, P., Xue, F., Wu, K. M., Jiang, M. J., Ji, J. F., et al. (2014). Repair of ear cartilage defects with allogenic bone marrow mesenchymal stem cells in rabbits. Cell Biochem. Biophys. 70 (2), 1137–1143. doi:10.1007/s12013-014-0033-2
Ciorba, A., and Martini, A. (2006). Tissue engineering and cartilage regeneration for auricular reconstruction. Int. J. Pediatr. Otorhinolaryngol. 70 (9), 1507–1515. doi:10.1016/j.ijporl.2006.03.013
Cohen, B. P., Bernstein, J. L., Morrison, K. A., Spector, J. A., and Bonassar, L. J. (2018). Tissue engineering the human auricle by auricular chondrocyte-mesenchymal stem cell co-implantation. PLoS One 13 (10), e0202356. doi:10.1371/journal.pone.0202356
Cohen, B. P., Hooper, R. C., Puetzer, J. L., Nordberg, R., Asanbe, O., Hernandez, K. A., et al. (2016). Long-term morphological and microarchitectural stability of tissue-engineered, patient-specific auricles in vivo. Tissue Eng. Part A 22 (5-6), 461–468. doi:10.1089/ten.TEA.2015.0323
Constantine, K. K., Gilmore, J., Lee, K., and Leach, J. (2014). Comparison of microtia reconstruction outcomes using rib cartilage vs porous polyethylene implant. JAMA Facial Plast. Surg. 16 (4), 240–244. doi:10.1001/jamafacial.2014.30
Derks, M., Sturm, T., Haverich, A., and Hilfiker, A. (2013). Isolation and chondrogenic differentiation of porcine perichondrial progenitor cells for the purpose of cartilage tissue engineering. Cells Tissues Organs 198 (3), 179–189. doi:10.1159/000354897
Dominici, M., Le Blanc, K., Mueller, I., Slaper-Cortenbach, I., Marini, F., Krause, D., et al. (2006). Minimal criteria for defining multipotent mesenchymal stromal cells. The International Society for Cellular Therapy position statement. Cytotherapy 8 (4), 315–317. doi:10.1080/14653240600855905
Dong, X., Askinas, C., Kim, J., Sherman, J. E., Bonassar, L. J., and Spector, J. A. (2022). Efficient engineering of human auricular cartilage through mesenchymal stem cell chaperoning. J. Tissue Eng. Regen. Med. 16 (9), 825–835. doi:10.1002/term.3332
Dowthwaite, G. P., Bishop, J. C., Redman, S. N., Khan, I. M., Rooney, P., Evans, D. J., et al. (2004). The surface of articular cartilage contains a progenitor cell population. J. Cell Sci. 117 (6), 889–897. doi:10.1242/jcs.00912
Dyson, M. E., Orangi, M., Goldberg, L. H., and Kimyai-Asadi, A. (2019). Repair of anterior ear defects using transcartilage island pedicle flaps. Dermatol Surg. 45 (10), 1222–1227. doi:10.1097/DSS.0000000000001799
Erickson, G. R., Gimble, J. M., Franklin, D. M., Rice, H. E., Awad, H., and Guilak, F. (2002). Chondrogenic potential of adipose tissue-derived stromal cells in vitro and in vivo. Biochem. Biophys. Res. Commun. 290 (2), 763–769. doi:10.1006/bbrc.2001.6270
Eslaminejad, B. M., and Bordbar, S. (2012). Blastema from rabbit ear contains progenitor cells comparable to marrow derived mesenchymal stem cells. Vet. Res. Forum 3 (3), 159–165.
Firmin, F. (1998). Ear reconstruction in cases of typical microtia, Personal experience based on 352 microtic ear corrections. Scand. J. Plast. Reconstr. Surg. Hand Surg. 32 (1), 35–47. doi:10.1080/02844319850158930
Fischer, J., Dickhut, A., Rickert, M., and Richter, W. (2010). Human articular chondrocytes secrete parathyroid hormone-related protein and inhibit hypertrophy of mesenchymal stem cells in coculture during chondrogenesis. Arthritis Rheum. 62 (9), 2696–2706. doi:10.1002/art.27565
Friedenstein, A. J. C. R., and Gerasimov, U. V. (1987). Bone marrow osteogenic stem cells: In vitro cultivation and transplantation in diffusion chambers. Cell Prolif. 20 (3), 263–272. doi:10.1111/j.1365-2184.1987.tb01309.x
Haisch, A., Kläring, S., Gröger, A., Gebert, C., and Sittinger, M. (2002). A tissue-engineering model for the manufacture of auricular-shaped cartilage implants. Eur. Arch. Otorhinolaryngol. 259 (6), 316–321. doi:10.1007/s00405-002-0446-1
Hassan, T. A., Maher, M. A., El Karmoty, A. F., Ahmed, Z. S. O., Ibrahim, M. A., Rizk, H., et al. (2022). Auricular cartilage regeneration using different types of mesenchymal stem cells in rabbits. Biol. Res. 55 (1), 40. doi:10.1186/s40659-022-00408-z
Ho, C. L., Huang, L. L. H., and Shieh, S. J. (2022). Perichondrial progenitor cells promote proliferation and chondrogenesis of mature chondrocytes. Regen. Biomater. 9, rbab078. doi:10.1093/rb/rbab078
Horlock, N., Vogelin, E., Bradbury, E. T., Grobbelaar, A. O., and Gault, D. T. (2005). Psychosocial outcome of patients after ear reconstruction: A retrospective study of 62 patients. Ann. Plast. Surg. 54 (5), 517–524. doi:10.1097/01.sap.0000155284.96308.32
Hou, M., Tian, B., Bai, B., Ci, Z., Liu, Y., Zhang, Y., et al. (2022). Dominant role of in situ native cartilage niche for determining the cartilage type regenerated by BMSCs. Bioact. Mater 13, 149–160. doi:10.1016/j.bioactmat.2021.11.007
Humphries, S., Joshi, A., Webb, W. R., and Kanegaonkar, R. (2022). Auricular reconstruction: Where are we now? A critical literature review. Eur. Arch. Otorhinolaryngol. 279 (2), 541–556. doi:10.1007/s00405-021-06903-5
Jessop, Z. M., Javed, M., Otto, I. A., Combellack, E. J., Morgan, S., Breugem, C. C., et al. (2016). Combining regenerative medicine strategies to provide durable reconstructive options: Auricular cartilage tissue engineering. Stem Cell Res. Ther. 7, 19. doi:10.1186/s13287-015-0273-0
Jia, Z., Liang, Y., Xu, X., Li, X., Liu, Q., Ou, Y., et al. (2018). Isolation and characterization of human mesenchymal stem cells derived from synovial fluid by magnetic-activated cell sorting (MACS). Cell Biol. Int. 42 (3), 262–271. doi:10.1002/cbin.10903
Jiang, Y., Jahagirdar, B. N., Reinhardt, R. L., Schwartz, R. E., Keene, C. D., Ortiz-Gonzalez, X. R., et al. (2002). Pluripotency of mesenchymal stem cells derived from adult marrow. Nature 418 (6893), 41–49. doi:10.1038/nature00870
Kagimoto, S., Takebe, T., Kobayashi, S., Yabuki, Y., Hori, A., Hirotomi, K., et al. (2016). Autotransplantation of monkey ear perichondrium-derived progenitor cells for cartilage reconstruction. Cell Transplant. 25 (5), 951–962. doi:10.3727/096368916X690917
Kang, N., Liu, X., Guan, Y., Wang, J., Gong, F., Yang, X., et al. (2012). Effects of co-culturing BMSCs and auricular chondrocytes on the elastic modulus and hypertrophy of tissue engineered cartilage. Biomaterials 33 (18), 4535–4544. doi:10.1016/j.biomaterials.2012.03.019
Kang, N., Liu, X., Yan, L., Wang, Q., Cao, Y., and Xiao, R. (2013). Different ratios of bone marrow mesenchymal stem cells and chondrocytes used in tissue-engineered cartilage and its application for human ear-shaped substitutes in vitro. Cells, tissues, organs 198 (5), 357–366. doi:10.1159/000357669
Karimi, H., Emami, S. A., and Olad-Gubad, M. K. (2016). Bone marrow stem cells and ear framework reconstruction. J. Craniofac Surg. 27 (8), 2192–2196. doi:10.1097/SCS.0000000000003146
Kim, Y. H., Namkung, J., Lim, B. G., Min, S. H., Shin, H. W., and Lim, C. H. (2011). Pleural effusion after microtia reconstructive surgery -A case report. Korean J. Anesthesiol. 61 (2), 166–168. doi:10.4097/kjae.2011.61.2.166
Kobayashi, S., Takebe, T., Zheng, Y-W., Mizuno, M., Yabuki, Y., Maegawa, J., et al. (2011). Presence of cartilage stem/progenitor cells in adult mice auricular perichondrium. PloS one 6 (10), e26393. doi:10.1371/journal.pone.0026393
Landau, S., Szklanny, A. A., Machour, M., Kaplan, B., Shandalov, Y., Redenski, I., et al. (2021). Human-engineered auricular reconstruction (hEAR) by 3D-printed molding with human-derived auricular and costal chondrocytes and adipose-derived mesenchymal stem cells. Biofabrication 14 (1), 015010. doi:10.1088/1758-5090/ac3b91
Lee, K. J., Comerford, E. J., Simpson, D. M., Clegg, P. D., and Canty-Laird, E. G. (2019). Identification and characterization of canine ligament progenitor cells and their extracellular matrix niche. J. Proteome Res. 18 (3), 1328–1339. doi:10.1021/acs.jproteome.8b00933
Leslie, S. K., Cohen, D. J., Hyzy, S. L., Dosier, C. R., Nicolini, A., Sedlaczek, J., et al. (2018). Microencapsulated rabbit adipose stem cells initiate tissue regeneration in a rabbit ear defect model. J. Tissue Eng. Regen. Med. 12 (7), 1742–1753. doi:10.1002/term.2702
Li, D., Chin, W., Wu, J., Zhang, Q., Xu, F., Xu, Z., et al. (2010). Psychosocial outcomes among microtia patients of different ages and genders before ear reconstruction. Aesthetic Plast. Surg. 34 (5), 570–576. doi:10.1007/s00266-010-9502-1
Lin, C. H., Yang, I. C., Tsai, C. H., Fang, H. W., and Ma, H. (2017). Auricular tissue engineering using osteogenic differentiation of adipose stem cells with small intestine submucosa. Plast. Reconstr. Surg. 140 (2), 297–305. doi:10.1097/PRS.0000000000003522
Mandl, E. W., Jahr, H., Koevoet, J. L., van Leeuwen, J. P., Weinans, H., Verhaar, J. A., et al. (2004). Fibroblast growth factor-2 in serum-free medium is a potent mitogen and reduces dedifferentiation of human ear chondrocytes in monolayer culture. Matrix Biol. 23 (4), 231–241. doi:10.1016/j.matbio.2004.06.004
McCorry, M. C., and Bonassar, L. J. (2017). Fiber development and matrix production in tissue-engineered menisci using bovine mesenchymal stem cells and fibrochondrocytes. Connect. Tissue Res. 58 (3-4), 329–341. doi:10.1080/03008207.2016.1267152
McCorry, M. C., Puetzer, J. L., and Bonassar, L. J. (2016). Characterization of mesenchymal stem cells and fibrochondrocytes in three-dimensional co-culture: Analysis of cell shape, matrix production, and mechanical performance. Stem Cell Res. Ther. 7, 39. doi:10.1186/s13287-016-0301-8
Minteer, D., Marra, K. G., and Rubin, J. P. (2013). Adipose-derived mesenchymal stem cells: Biology and potential applications. Adv. Biochem. Eng. Biotechnol. 129, 59–71. doi:10.1007/10_2012_146
Mitchell, K. J., Pannerec, A., Cadot, B., Parlakian, A., Besson, V., Gomes, E. R., et al. (2010). Identification and characterization of a non-satellite cell muscle resident progenitor during postnatal development. Nat. Cell Biol. 12 (3), 257–266. doi:10.1038/ncb2025
Moon, B. J., Lee, H. J., and Jang, Y. J. (2012). Outcomes following rhinoplasty using autologous costal cartilage. Arch. Facial Plast. Surg. 14 (3), 175–180. doi:10.1001/archfacial.2012.138
Mori, H., Tanaka, K., Umeda, T., and Hata, Y. (2002). Ear reconstruction in elderly patients: A two-part helix method in a framework. Br. J. Plast. Surg. 55 (7), 589–591. doi:10.1054/bjps.2002.3924
Morrison, K. A., Cohen, B. P., Asanbe, O., Dong, X., Harper, A., Bonassar, L. J., et al. (2016). Optimizing cell sourcing for clinical translation of tissue engineered ears. Biofabrication 9 (1), 015004. doi:10.1088/1758-5090/9/1/015004
Moshkbouymatin, N. (2019). Identification and Characterisation of tracheal cartilage derived stem cells for airway tissue engineering. United Kingdom: Swansea University.
Mussi, E., Furferi, R., Volpe, Y., Facchini, F., McGreevy, K. S., and Uccheddu, F. (2019). Ear reconstruction simulation: From handcrafting to 3D printing. Bioeng. (Basel) 6 (1), 14. doi:10.3390/bioengineering6010014
Narges Baluch, S. N., Chul Park, M. D., Wilkes, G. H., Reinisch, J., Kasrai, L., Fisher, D., et al. (2014). Auricular reconstruction for microtia: a review of available methods. Plast. Surg. 22 (1), 39–43. doi:10.1177/229255031402200102
Oba, T., Okamoto, S., Ueno, Y., Matsuo, M., Tadokoro, T., Kobayashi, S., et al. (2022). In vitro elastic cartilage reconstruction using human auricular perichondrial chondroprogenitor cell-derived micro 3D spheroids. J. tissue Eng. 13, 20417314221143484. doi:10.1177/20417314221143484
Oh, S-J., Choi, K-U., Choi, S-W., Kim, S-D., Kong, S-K., Lee, S., et al. (2020). Comparative analysis of adipose-derived stromal cells and their secretome for auricular cartilage regeneration. Stem cells Int. 2020, 8595940. doi:10.1155/2020/8595940
Oh, S-J., Park, H-Y., Choi, K-U., Choi, S-W., Kim, S-D., Kong, S-K., et al. (2018). Auricular cartilage regeneration with adipose-derived stem cells in rabbits. Mediat. Inflamm. 2018, 4267158. doi:10.1155/2018/4267158
Osorno, G. (2007). A 20-year experience with the brent technique of auricular reconstruction: Pearls and pitfalls. Plast. Reconstr. Surg. 119 (5), 1447–1463. doi:10.1097/01.prs.0000258572.57161.d8
Osorno, G. (1999). Autogenous rib cartilage reconstruction of congenital ear defects: Report of 110 cases with brent's technique. Plast. Reconstr. Surg. 104 (7), 1951–1962; discussion 1963-1964. doi:10.1097/00006534-199912000-00001
Otto, I. A., Bernal, P. N., Rikkers, M., van Rijen, M. H. P., Mensinga, A., Kon, M., et al. (2022). Human adult, pediatric and microtia auricular cartilage harbor fibronectin-adhering progenitor cells with regenerative ear reconstruction potential. Science 25 (9), 104979. doi:10.1016/j.isci.2022.104979
Otto, I. A., Levato, R., Webb, W. R., Khan, I. M., Breugem, C. C., and Malda, J. (2018). Progenitor cells in auricular cartilage demonstrate cartilage-forming capacity in 3D hydrogel culture. Eur. cells Mater. 35, 132–150. doi:10.22203/eCM.v035a10
Otto, I. A., Melchels, F. P., Zhao, X., Randolph, M. A., Kon, M., Breugem, C. C., et al. (2015). Auricular reconstruction using biofabrication-based tissue engineering strategies. Biofabrication 7 (3), 032001. doi:10.1088/1758-5090/7/3/032001
Park, C. (1997). Modification of two-flap method and framework construction for reconstruction of atypical congenital auricular deformities. Plast. Reconstr. Surg. 99 (7), 1846–1857. doi:10.1097/00006534-199706000-00007
Patricia, A., Zuk, M. Z., Peter, A., DanielDe Ugarte, A., Huang, J. I., Mizuno, H., et al. (2002). Human adipose tissue is a source of multipotent stem cells. Mol. Biol. Cell 13, 4279–4295. doi:10.1091/mbc.e02-02-0105
Pittenger, M. F., Mackay, A. M., Beck, S. C., Jaiswal, R. K., Douglas, R., Mosca, J. D., et al. (1999). Multilineage potential of adult human mesenchymal stem cells. Science 284 (5411), 143–147. doi:10.1126/science.284.5411.143
Pleumeekers, M. M., Nimeskern, L., Koevoet, W. L. M., Karperien, M., Stok, K. S., and van Osch, G. J. V. M. (2015). Cartilage regeneration in the head and neck area: Combination of ear or nasal chondrocytes and mesenchymal stem cells improves cartilage production. Plastic Reconstr. Surg. 136 (6), 762e–74e. doi:10.1097/PRS.0000000000001812
Posniak, S., Chung, J. H. Y., Liu, X., Mukherjee, P., Gambhir, S., Khansari, A., et al. (2022). Bioprinting of chondrocyte stem cell Co-cultures for auricular cartilage regeneration. ACS Omega 7 (7), 5908–5920. doi:10.1021/acsomega.1c06102
Prockop, D. J. (1997). Marrow stromal cells as stem cells for nonhematopoietic tissues. Science 276, 71–74. doi:10.1126/science.276.5309.71
Raghunath, J., Salacinski, H. J., Sales, K. M., Butler, P. E., and Seifalian, A. M. (2005). Advancing cartilage tissue engineering: The application of stem cell technology. Curr. Opin. Biotechnol. 16 (5), 503–509. doi:10.1016/j.copbio.2005.08.004
Reiffel, A. J., Kafka, C., Hernandez, K. A., Popa, S., Perez, J. L., Zhou, S., et al. (2013). High-fidelity tissue engineering of patient-specific auricles for reconstruction of pediatric microtia and other auricular deformities. PLoS One 8 (2), e56506. doi:10.1371/journal.pone.0056506
Robey, P. G., and Bianco, P. (2006). The use of adult stem cells in rebuilding the human face. J. Am. Dent. Assoc. 137 (7), 961–972. doi:10.14219/jada.archive.2006.0317
Rodeheffer, M. S., Birsoy, K., and Friedman, J. M. (2008). Identification of white adipocyte progenitor cells in vivo. Cell 135 (2), 240–249. doi:10.1016/j.cell.2008.09.036
Schnabel, M., Marlovits, S., Eckhoff, G., Fichtel, I., Gotzen, L., Vécsei, V., et al. (2002). Dedifferentiation-associated changes in morphology and gene expression in primary human articular chondrocytes in cell culture. Osteoarthr. Cartil. 10 (1), 62–70. doi:10.1053/joca.2001.0482
Shieh, S. J., Terada, S., and Vacanti, J. P. (2004). Tissue engineering auricular reconstruction: In vitro and in vivo studies. Biomaterials 25 (9), 1545–1557. doi:10.1016/s0142-9612(03)00501-5
Solchaga, L. A., Welter, J. F., Lennon, D. P., and Caplan, A. I. (2004). Generation of pluripotent stem cells and their differentiation to the chondrocytic phenotype. Methods Mol. Med. 100, 53–68. doi:10.1385/1-59259-810-2:053
Spencer, H., Moshkbouymatin, N., Webb, W. R., Joshi, A., and D'Souza, A. (2021). Update on the role of emerging stem cell technology in head and neck medicine. Head. Neck 43 (6), 1928–1938. doi:10.1002/hed.26674
Thomson, H. G., Kim, T. Y., and Ein, S. H. (1995). Residual problems in chest donor sites after microtia reconstruction: A long-term study. Plast. Reconstr. Surg. 95 (6), 961–968. doi:10.1097/00006534-199505000-00002
Till, J. E., and McCulloch, M. E. (1961). A direct measurement of the radiation sensitivity of normal mouse bone marrow cells. Radiat. Res. 14, 213. doi:10.1667/rrav01.1
Togo, T., Utani, A., Naitoh, M., Ohta, M., Tsuji, Y., Morikawa, N., et al. (2006). Identification of cartilage progenitor cells in the adult ear perichondrium: Utilization for cartilage reconstruction. Laboratory investigation; a J. Tech. methods pathology 86 (5), 445–457. doi:10.1038/labinvest.3700409
Uppal, R. S., Sabbagh, W., Chana, J., and Gault, D. T. (2008). Donor-site morbidity after autologous costal cartilage harvest in ear reconstruction and approaches to reducing donor-site contour deformity. Plast. Reconstr. Surg. 121 (6), 1949–1955. doi:10.1097/PRS.0b013e318170709e
Wagers, A. J., and Weissman, I. L. (2004). Plasticity of adult stem cells. Cell 116 (3), 639–648. doi:10.1016/s0092-8674(04)00208-9
Walton, R. L., and Beahm, E. K. (2002). Auricular reconstruction for microtia: Part II. Surgical techniques. Plast. Reconstr. Surg. 110 (1), 234–249; quiz 250-251, 387. doi:10.1097/00006534-200207000-00041
Wilkes, G. H., Wong, J., and Guilfoyle, R. (2014). Microtia reconstruction. Plast. Reconstr. Surg. 134 (3), 464e–79e. doi:10.1097/PRS.0000000000000526
Williams, R., Khan, I. M., Richardson, K., Nelson, L., McCarthy, H. E., Analbelsi, T., et al. (2010). Identification and clonal characterisation of a progenitor cell sub-population in normal human articular cartilage. PLoS One 5 (10), e13246. doi:10.1371/journal.pone.0013246
Xiong, M., Zhang, Q., Hu, W., Zhao, C., Lv, W., Yi, Y., et al. (2020). Exosomes from adipose-derived stem cells: The emerging roles and applications in tissue regeneration of plastic and cosmetic surgery. Front. Cell Dev. Biol. 8, 574223. doi:10.3389/fcell.2020.574223
Xu, F., Yang, Y., Yang, T., Dai, T., Shao, X., Xu, H., et al. (2018). The use of allogenic adipose-derived stem cells in combination with platelet-rich fibrin for the treatment of cartilage defects in rabbit ear. Am. J. Transl. Res. 10 (6), 1900–1907.
Xue, K., Zhang, X., Qi, L., Zhou, J., and Liu, K. (2016). Isolation, identification, and comparison of cartilage stem progenitor/cells from auricular cartilage and perichondrium. Am. J. Transl. Res. 8 (2), 732–741.
Yamamoto, N., Akamatsu, H., Hasegawa, S., Yamada, T., Nakata, S., Ohkuma, M., et al. (2007). Isolation of multipotent stem cells from mouse adipose tissue. J. Dermatol Sci. 48 (1), 43–52. doi:10.1016/j.jdermsci.2007.05.015
Yamamoto, Y., Mochida, J., Sakai, D., Nakai, T., Nishimura, K., Kawada, H., et al. (2004). Upregulation of the viability of nucleus pulposus cells by bone marrow-derived stromal cells: Significance of direct cell-to-cell contact in coculture system. Spine (Phila Pa 1976) 29 (14), 1508–1514. doi:10.1097/01.brs.0000131416.90906.20
Yang, H. N., Na, K., Woo, D. G., Kwon, Y. D., and Park, K. H. (2009). The use of green fluorescence gene (GFP)-modified rabbit mesenchymal stem cells (rMSCs) co-cultured with chondrocytes in hydrogel constructs to reveal the chondrogenesis of MSCs. Biomaterials 30, 6374–6385. doi:10.1016/j.biomaterials.2009.07.062
Zhang, L., He, A., Yin, Z., Yu, Z., Luo, X., Liu, W., et al. (2014). Regeneration of human-ear-shaped cartilage by co-culturing human microtia chondrocytes with BMSCs. Biomaterials 35 (18), 4878–4887. doi:10.1016/j.biomaterials.2014.02.043
Zhang, X., Qi, L., Chen, Y., Xiong, Z., Li, J., Xu, P., et al. (2019). The in vivo chondrogenesis of cartilage stem/progenitor cells from auricular cartilage and the perichondrium. Am. J. Transl. Res. 11 (5), 2855–2865.
Zhao, X., Hwang, N. S., Bichara, D. A., Saris, D. B., Malda, J., Vacanti, J. P., et al. (2017). Chondrogenesis by bone marrow-derived mesenchymal stem cells grown in chondrocyte-conditioned medium for auricular reconstruction. J. Tissue Eng. Regen. Med. 11 (10), 2763–2773. doi:10.1002/term.2171
Zhao, Y., Wang, Y., Zhuang, H., Jiang, H., Jiang, W., Hu, X., et al. (2009). Clinical evaluation of three total ear reconstruction methods. J. Plast. Reconstr. Aesthet. Surg. 62 (12), 1550–1554. doi:10.1016/j.bjps.2008.07.009
Zhou, G., Jiang, H., Yin, Z., Liu, Y., Zhang, Q., Zhang, C., et al. (2018). In vitro regeneration of patient-specific ear-shaped cartilage and its first clinical application for auricular reconstruction. EBioMedicine 28, 287–302. doi:10.1016/j.ebiom.2018.01.011
Zhu, P., and Chen, S. (2016). Clinical outcomes following ear reconstruction with adjuvant 3D template model. Acta Otolaryngol. 136 (12), 1236–1241. doi:10.1080/00016489.2016.1206967
Keywords: stem cells, auricular cartilage, adipose-derived stem cells, bone marrow mesenchymal stem cells, perichondrial stem/progenitor cells, auricular reconstruction
Citation: Liu Y, Wu W, Seunggi C, Li Z, Huang Y, Zhou K, Wang B, Chen Z and Zhang Z (2023) The application and progress of stem cells in auricular cartilage regeneration: a systematic review. Front. Cell Dev. Biol. 11:1204050. doi: 10.3389/fcell.2023.1204050
Received: 11 April 2023; Accepted: 10 July 2023;
Published: 26 July 2023.
Edited by:
Lingyong Jiang, Shanghai Jiao Tong University, ChinaReviewed by:
Livia Roseti, Rizzoli Orthopedic Institute (IRCCS), ItalyHiroe Ohnishi, Kyoto University, Japan
Copyright © 2023 Liu, Wu, Seunggi, Li, Huang, Zhou, Wang, Chen and Zhang. This is an open-access article distributed under the terms of the Creative Commons Attribution License (CC BY). The use, distribution or reproduction in other forums is permitted, provided the original author(s) and the copyright owner(s) are credited and that the original publication in this journal is cited, in accordance with accepted academic practice. No use, distribution or reproduction is permitted which does not comply with these terms.
*Correspondence: Zhenyu Zhang, zhangzy.wch@foxmail.com