Nanocarriers for methotrexate delivery/codelivery in the frame of cancer diagnostics and treatment: a review
- 1Faculty of Pharmacy, Institute of Pharmaceutical Technology and Regulatory Affairs, University of Szeged, Szeged, Hungary
- 2Department of Chemistry, Faculty of Science, Tshwane University of Technology (Arcadia Campus), Pretoria, South Africa
- 3Department of Biology, Faculty of Science, Nour Danesh Institute of Higher Education, Meymeh, Isfahan, Iran
- 4Medical Biomaterial Research Centre (MBRC), Tehran University of Medical Sciences, Tehran, Iran
- 5Department of Radiology, Zabol University of Medical Sciences, Zabol, Iran
- 6Department of Physics, University of Zabol, Zabol, Iran
- 7Department of Chemistry, Smt. Devkiba Mohansinhji Chauhan College of Commerce and Science, University of Mumbai, Mumbai, India
- 8Department of Applied Science and Technology, Institute of Materials Physics and Engineering, Politecnico di Torino, Turin, Italy
Cancer is one of the most life-threatening family of diseases that cause death worldwide. As a highly researched and successful therapeutic agent, methotrexate (MTX) treats many solid tumours, hematologic malignancies, and autoimmune illnesses. Despite many benefits, methotrexate induces drug resistance and limits plasma half-life due to its poor pharmacokinetics. The variable biological availability have prompted researchers to investigate innovative delivery strategies for enhancing its therapeutic qualities. To develop more suitable methotrexate formulations, nanoparticles (NPs) have recently gained a significant interest. A wide range of nanoparticles, including polymer-based nanoparticles, carbon-based nanoparticles, lipid-based nanoparticles, as well as inorganic nanoparticles, can be deliver cancer chemotherapeutics such as methotrexate. Loading methotrexate into NPs can provide a delivery system that has shown great promise to carcinoma therapy. In this review, we will describe the feasibility of NP-based strategies to deliver methotrexate in cancer therapy, outlining the current state of the art and the challenges/promises for the future.
1 Introduction
Cancer is among the most important reasons for decrement of life expectancy. In 2019, according to a study by the World Health Organization (WHO) in 112 countries, cancer has been estimated to be the main reason for death before the age of 70. In many countries, the death rate resulting from cancer has increased compared to the death rate from stroke and heart disease. The most frequently diagnosed cancers include female breast carcinoma (11.7% of all occurrences), lung carcinoma (11.4%), colorectal carcinoma (10%), prostate carcinoma (7.3%), and stoma carcinoma (5.6%). The most frequent deaths from cancers are due lung carcinoma (18% of all cancer deaths), followed by colorectal carcinoma (9.4%), liver carcinoma (8.3%), stomatal carcinoma (7.7%), and breast carcinoma (6.9%). Colorectal and prostate cancers are the most common cancers occurring and causing death among men population. In the women population, the most frequently leading reason of cancer death is breast cancer, followed by colorectal and lung cancers. Based on current clinical data, GLOBOCAN 2020 estimated the population with new cancer cases to be 19.3 millions per year, and reported around 10 million deaths from cancer globally (Fatima et al., 2021; Sung et al., 2021). Several immune-based strategies have been approved to treat cancer in recent years. Radiotherapy (RT) can affect the tumor immune state in a variety of ways, such as promoting the release of tumor-specific antigens by tumor cells and activating T-cells against tumors to overcome tumor immune tolerance mechanisms (Yang et al., 2022). RT treats patients by damaging DNA of tumor cells and generating cytotoxic reactive oxygen species (ROS) to control tumors. Meanwhile, the presence of hypoxic cells in tumors and toxic side effects in peripheral tissue lead to resistance to RT, which remains a challenge in RT research. At the same time, a minority of patients exhibit long-term response to immunotherapy due to the complexity of the mechanisms of tumor immune escape. As a result, single immunotherapy is restricted to a few tumors and can only benefit a fraction of patients. Due to the immunomodulatory effects of RT and reduced side effects in tumor immunotherapy, RT combined with immunotherapy has significantly improved the effective cure rate by eradicating tumor cells and amplifying the activity of immune cells in several clinical trials. Compared to immunotherapy, radioimmunotherapy has significantly shown better therapeutic effects with favorable efficacy to control the primary tumor and its metastases by activating the immune response, indicating a synergistic effect of RT and immunotherapy (Pointer et al., 2022; Colciago et al., 2023). Radioimmunotherapy leads to immunogenic cell death (ICD) by promoting the release of tumor-associated antigens (TAAs), changing the tumor microenvironment (TME), and activating the immune system to exert an anti-tumor immune response. The synergy between immunotherapy and RT has become a hot field in oncology therapy to boost immune response (Yu et al., 2022; Colciago et al., 2023). In oncotherapy, nanoparticles (NPs) are able to overcome biological barriers, thus reducing the dose of chemotherapeutics needed and the severity of chemotherapy-associated side effects (Hassanisaadi et al., 2021; Ajalli et al., 2022). Nanotechnology can target neoplastic cells through the enhanced permeability and retention (EPR) effects or active targeting. As EPR depends on the nature of carcinoma cell leakage, only 20% of the medication can be delivered to the cell while the body excretes the rest. Cancer treatment usually uses cytotoxic mediators, including DNA-damaging agents and microtubule inhibitors (Pourmadadi et al., 2022). Chemotherapy is provided at the maximum likely dose that is safe and agreeable to the highest tolerable concentration. It is established that conservative chemotherapy has side effects on health, such as hair loss, cardiac and neurotoxicity, liver cell harm, kidney damage, sickness, vomiting, immunosuppressive effects, etc.
A nanocarrier (Nc) is used to locally deliver cytotoxic compounds to reduce side effects and advance medical results. Nc is preferably deposited at the tumour location because of its size, high absorbency and EPR effect. The accumulation of NPs at the target sites yields incomplete neovascularization of tumor tissue and allows decreasing non-specific tissue damages and overcoming the barriers to chemotherapy. A mechanism called endocytosis can be more useful than conventional therapy. Targeted delivery of cytotoxic agents is due to ligands binding straight to the receptor, causing cell internalization (Sheikh et al., 2022). Numerous medical procedures have been performed with several projected anticancer therapies. When there is a real deal, the side effects and the tranfering to the desired location are important worries. Also, another problem is drug resistance and toxicity of new anti-cancer treatments. Nanotechnology is a very versatile approach with great promises in medical treatments. A key problem to tackle when dealing with cancer concerns the translation of outcomes from in vitro/in vivo to clinical applications with focus on some parameters, including therapeutic delivery, toxicity, targeting, resistance, and stability. Accordingly, many NP-based approaches for anti-tumour therapies are practically unfeasible or ineffective. Two important mechanisms for drug delivery are targeting and active targeting pathways in nanomaterials. Different types of NPs as targeted delivery systems have been used for a variety of cancer therapies. The delivery systems based on NPs are subdivided in four forms to treat cancer, including 1) Viral NPs, 2) Organic NPs (dendrimer, polymeric, and liposomal), 3) Inorganic NPs (carbon, magnetic, gold, silica, and some metals), and 4) composite NPs (lipid polymer). All NPs are often coated by other materials due to the side effects of chemotherapy. Chemotherapy is a non-specific treatment that can be spread to numerous tissues and organs by ineffective pharmacokinetics. The main structure of nano-liposomes is based on biomembrane phospholipid for encapsulating particles to enhance the solubility in aqueous systems. Polymer NPs as a system of drug delivery have been applied to form multifunctional organic NPs. Poly(lactic-c-hydroxyacetic acid) is used as a polymer for drug delivery due to its good biological compatibility and biodegradability. Inorganic NPs include Au NPs, Fe3O4 NPs and Ag NPs, which can be fabricated according to relatively simple processes, have good stability but lower biocompatibility compared to polymers. Silicon NPs are another example of more biocompatible inorganic NPs. In general, any type of NP, both inorganic and organic, has advantages and disadvantages to treat cancer with a different range of results (Al-Zoubi and Al-Zoubi, 2022). Methotrexate (MTX) can be loaded into and released from many of these nano-systems as an anticancer drug for targeted therapy.
2 Methotrexate
Methotrexate (MTX) has roles such as antimetabolic, anti-proliferative and anti-inflammatory, employing folate antagonist. MTX could decrease the level of inflammation by the immune system and some inflammatory cells, for example, monocytes, mast cells, neutrophils, helper T and lymphocytes B. MTX prevents purines and pyrimidines synthesis by inhibiting dihydrofolate reductase (HHFR) and also thymidylate synthase (TYMS) which has a role in the construction of thymine residues, then its suppressing protein, deoxyribonucleic acid (DNA) and ribonucleic acid (RNA), so it leads to accumulation of a plethora of inflammatory cells (Zhao et al., 2022). Some chemotherapeutic drugs are likely to produce an antimicrobial resistance in gut microbiota by inducing dysbiosis. Methotrexate works by blocking the enzyme dihydrofolate reductase in bacterial and eukaryotic cells in DNA synthesis and is used to treat skin, breast, head, and neck cancers and inflammatory diseases such as rheumatoid arthritis (RA) (Guðmundsdóttir et al., 2021). Kidney excretes MTX, and delayed clearance may cause toxicity. Poisoning depends on MTX serum concentration and the duration of complete excretion from the body. However, impaired MTX clearance causes kidney problems and the use of hemodialysis clears effectively, but data are not available in these patients (Solignac et al., 2021). In cancer treatment with chemotherapy, some cancer cells are resistant to drugs and do not undergo apoptosis. For the treatment to be targeted quickly and accurately and for patients to experience less unpleasant side effects of chemotherapy, the bioavailability and stability of the drugs used in chemotherapy must be considered. Based on this, intelligent drug delivery systems and new stimuli-responsive materials have been developed. Clinical trials have shown that intelligent drug delivery systems have increased the efficiency of cancer therapy compared to free drugs without nano-carriers. Limitations of anticancer drugs include short half-lives, nonspecificity, widespread distribution, and toxicity. Nanocarriers can minimize these limitations through controlled delivery and release, which is desired in cancer treatment. Nanocarriers increase drug uptake and bioavailability, thus reducing side effects and increasing cytotoxicity to cancer cells (Alqosaibi, 2022). Methotrexate is used to treat tumours and lymphomas. High intravenous doses increase serum enzymes and, in the long term, increase liver enzymes, cirrhosis, and fibrosis. Methotrexate is a yellowish-orange brown and crystalline, odourless powder that is used in chemotherapy to prevent the RNA and DNA synthesis. The molecular formula is C20H22N8O5. Oral methotrexate is indicated to treat acute lymphoblastic leukaemia in children (Bethesda MD: National Library of Medicine US, 2022). Figures 1A, B are depicted 2 and 3-dimensional.
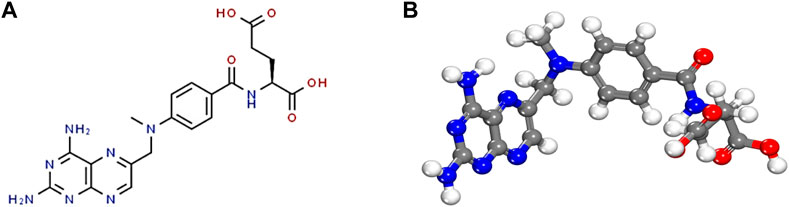
FIGURE 1. Methotrexate chemical structure. (A) 2-D methotrexate’s structure, and (B) 3-D methotrexate structure.
2.1 Methotrexate as an anticancer drug and resistance mechanisms
To decrease the unpleasant side effects on healthy tissues and cells, anticancer drugs require organic solvents and detergents for their complete dissolution and stability. Nano-formulations transport anticancer drugs to the target site through a nano-carrier, improving efficacy, pharmacokinetics and bioavailability (Connie and Ariya, 2015). In clinical observations, despite the advantages of D-α-tocopheryl polyethene glycol 1,000 succinate (TPGS) and poly(lactic-co-glycolic) acid (PLGA), no MTX delivery carrier was publicly claimed to use them (Almawash et al., 2022a). The remaining cells surviving after chemotherapy may develop drug resistance, possibly due to the presence of stem cells in heterogeneous tumours that cause resistant clones to form. Drug resistance occurs through the expression of genes that encode proteins resulting from the ATP-binding cassette (ABC) family. Multidrug flow pumps belong to this family, and drug withdrawal and overexpression pumps are among the main causes of chemotherapy failure. The capability of the drug to precisely target the cancer cell actively increases its concentration inside the cell and thus causes a localized cytotoxic effect (Xavier et al., 2022). In a clinical trial, it was shown that both MTX and folic acid, with a supramolecular approach, ligands are capable of interacting with folate receptor at the surface of cell, significantly reducing the side effects of the drug and the target cancer cells (Massaro et al., 2022). MTX is slightly soluble in water and has an oral bioavailability of less than 40%, which impairs its therapeutic effect. The oral uptake of MTX in the proton-dependent GIT depends on active transmission and dose. The oral bioavailability of MTX is decreased with increase in the dose above 25 mg; in fact, MTX is poorly bioavailability (∼18% for doses >40 mg/m2) and ∼80% is excreted unchanged into urine (Giri et al., 2022). Therefore, doses above 25% are more effective, although they may have side effects such as diarrhoea, anaemia, and intestinal toxicity. Liquid ion-based food (IL) systems are beneficial to improve pharmacokinetic properties and bioavailability, promote high intestinal absorption, better distribution, and limit drug metabolism. Preliminary results showed that MTX in the form of ionic fluids (IL) and body simulation fluids was 5,000 times more soluble and had a more anti-cancer effect than free MTX. Previous studies have shown that side effects in the slow distribution of MTX-IL are less than in the distribution of sodium MTX in organs such as the lungs, kidneys, heart and spleen without acute GIT accumulation (Moshikur et al., 2021). Therapeutic drug monitoring (TDM) is used as a valuable instrument for preventing the side effects of overdose and ineffective treatments that are unique to each patient and for assessing the effectiveness and safety of the treatment regimen. TDM is based on the level of active metabolite, which is usually plasma, and is adjusted by adjusting the dose of the drug for each individual, which aims to increase effectiveness and decrease toxicity and bioavailability (Zhou et al., 2022).
3 Methotrexate drug delivery systems
3.1 Polymeric nanoparticles
3.1.1 Non-responsive polymeric nanoparticles
The potential of MTX-loaded polymeric NPs has been exploited for various outcomes. MTX was loaded into methoxy poly(ethylene glycol) (MPEG)-grafted chitosan (chitoPEG) copolymer (Seo et al., 2009). The mean size of the NPs was 50–300 nm with spherical shape. 1H nuclear magnetic resonance (NMR) study demonstrated that MTX interacted with chitosan being embeeded in the inner core, and the outer shell of the NPs was composed of MPEG (Figure 2). The loading efficiency of MTX was demonstrated to be between 65.2% and 94%. The cytotocicity of the developed MTX-loaded NPs was evaluated on the B16F10 melanoma cells, and the outcomes revealed that MTX-loaded NPs presented less cytotoxicity compared to free MTX. In another study, MTX was enclosed into poly(lactide-co-glycolide) (PLGA) NPs by the process of solvent evaporation from a double emulsion (Jang et al., 2019).
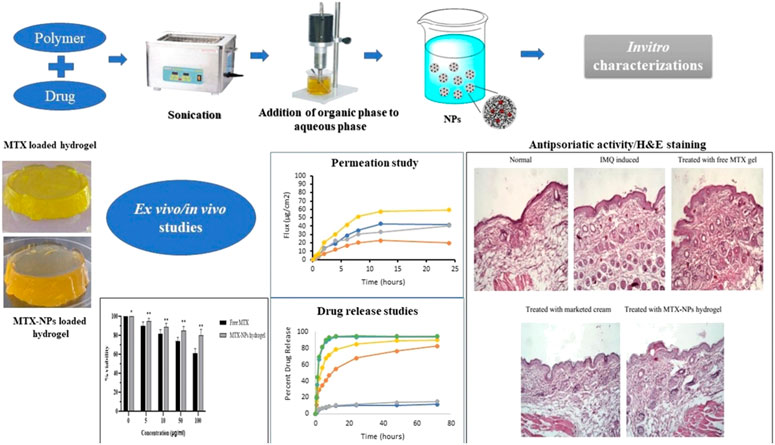
FIGURE 2. Schematic representation of topical hydrogel loaded with pH-sensitive polymeric NPs of methotrexate for effective treatment of psoriasis, reproduced from (Asad et al., 2021) published under an open access creative common cc by license.
The average size of the NPs was demonstrated to be 163.7 nm with an encapsulation efficiency of 93.3%, and the drug was released according to sustained kinetics (Figure 2). The cytotoxicity studies showed that the NPs presented higher antiproliferative activity against the cancerous cells, MFC-7 and CWR22Rv, in comparison with the free MTX. Moreover, the NPs were evaluated for properties of the pharmacokinetics and consequences of lymphatic delivery. The in vivo studies revealed high lymphatic efficiency and low accumulation of MTX in the kidney and liver. Furthermore, the fabricated NPs can be investigated by applying different administration routes, and MTX was still detectable in the blood for a long time in comparison with free MTX. To evaluate the anti-breast cancer activity, polymeric NPs comprised of PLGA and PEG were developed by the double emulsion solvent-evaporation method. The PLGA-PEG NPs were co-loaded with MTX and trapoxin A (TPX) (Akbari et al., 2021a). The developed PLGA-PEG NPs exhibited smooth morphology and nanosize particle distribution. Moreover, the NPs presented promising results against MCF-7 breast cancerous cells. The co-loaded NPs elicited an effective inhibition of MCF-7 cells. Also, the flow cytometry analysis demonstrated cell growth inhibition by induction of apoptosis. Furthermore, the cell cycle analysis was also done, which revealed that there was a stopped cell cycle at G1. The dual drug-loaded PLGA-PEG NPs also exhibited the mitochondrial apoptosis. Altogether, the developed NPs proved to be promising in breast cancer therapy. To improve the effective concentration of MTX at the target site for cancer therapy, a novel system was developed that combined physical targeting along with chemical targeting (Lin et al., 2015). Poly(lactic acid) (PLA) and MPEG were used to encapsulate MTX in the nanobacilli-shaped carriers, and the dye Cy5.5 was conjugated with them. The nanobacilli had improved intratumoral uptake in the H22 xenograft mouse model. Moreover, the MPEG-PLA- MTX-Cy5.5 nanospheres released the drug into the tumour cells in a controlled manner to inhibit tumour growth and improve therapeutic efficiency. Similarly, another MTX loaded PLGA-beta cyclodextrin nanosystem was developed using double emulsion method to improve drug atability and solubility (Gorjikhah et al., 2017). The mean particle size ranged from 70 to 200 nm with an entrapment of 80%. The PLGA-β cyclodextrin NPs increased the cytotoxicity after releasing the drug in T47D breast cancer cell lines. In another study, PLA-PEG-PLA triblock polymeric NPs were synthesized to deliver MTX in a controlled way to the cancer cells. The double emulsion method was adopted, and the prepared polymeric NPs were then covered with bovine serum albumin to enhance their biocompatibility. The average size of the NPs was reported to be in the range of 100–157 nm with an entrapping efficiency of 80.4% (Massadeh et al., 2016). In another study, biodegradable MPEG-PCL copolymer-based NPs were designed for their activity on breast cancer cells. MTX and curcumin were co-loaded in the NPs to improve the therapeutic activity against cancer cell lines. The nanoprecipitation method was adopted to develop self-assembled micelles. The average micelle size was found to be 88 nm. The cancer cell growth was inhibited with an increase in the concentration of the micelles in the treatment groups. The micelles improved the cytotoxicity against MCF-7 cancer cell lines (Saraei et al., 2019). Anti-inflammatory effects of MTX can also be relevant to the field of cancer treatment as some correlations were suggested between cancer development and persistent inflammation states. In this regard, MTX-loaded spherical chitosan and Pluronic F-127 NPs were developed using the ionic gelation technique and self-micellization for treating rheumatoid arthritis. The average size of the NPs was 181–417 nm, with a loading efficiency of 80.14%. The NPs prolonged the release of MTX for 48 h and improved the pharmacokinetic profile. There was an improved therapeutic activity with 3.48 times higher residence time in the inflamed tissues. The haematological study revealed that the NPs showed no adverse effects on the therapeutic activity. Chitosan was also employed to develop NPs and fucoidan to treat skin-related inflammatory issues. MTX was enclosed in the NPs with an entrapment efficiency of 80%–96% with a mean size of 300–500 nm. The NPs exhibited low cytotoxicity to the human keratinocytes and fibroblasts. In vivo studies showed that there was a significant reduction in the pro-inflammatory cytokines. Moreover, the NPs improved the permeation of MTX through the pig ear skin barrier as compared to free MTX. Altogether, this MTX topical delivery using this approach demonstrated promising anti-inflammatory activity (Barbosa et al., 2019). Polycaprolactone and polyethylene glycol (PCL-PEG) were used to develop NPs co-loaded with MTX and cisplatin for an effective treatment for non-small lung cancer (Zhang et al., 2017). The NPs were of spherical morphology and induced apoptosis in the tumour tissues as revealed by DAPI analysis. The PCL-PEG NPs improved the cytotoxicity against A549 cells by reducing bcl2 and cyclin D1 gene expression.
Iron oxide magnetic NPs (110–130 nm) were developed based on lignin as a natural polymeric-magnetic-nano-system via anti-solvent precipitation and ultrasonication for the targeted delivery of MTX. The drug loading and encapsulation efficiencies were calculated to be about 66.06% and 64.88%, respectively. The NPs, as a non-hemolytic material, exhibited a concentration-dependent release of MTX for the initial 24 h. MTT assay for NPs revealed higher cytotoxicity against breast cancer and macrophage cell lines in comparison to the free form of the drug by increasing caspase-3 activity and decresing glutathionelevels (Pathania et al., 2023).
Magnetic alginate beads coated with glutaraldehyde cross-linked chitosan were prepared to decrease the side effects and improve stability due to biodegradable and biocompatible nature of the NPs, resulting in an encapsulation efficiency of 75% and showing acceptable controlled release behavior. Cytotoxity assay based on MTT revealed higher anticancer effect compared to the free MTX by increasing expression of Bax and decreasing the expressions of Bcl-2 and TNF-α in MCF-7 cells (Taran et al., 2023). β-CD/MTX complex was fabricated to enhance the solubility and stability of MTX in aqueous solvents. β-CD as a nanocarrier had a good capacity for loading and releasing MTX. MTT assay exhibited improvement of the MTX performance (Hadi et al., 2023). Arrow et al. (2023) prepared poly(lactide-co-glycolide) complexes to load MTX, which demonstrated inhibitory effects on human PVR-cells. Samani et al. (2023) synthesized folic acid-functionalized MTX-loaded perfluorohexane nanodroplets (NDs) with an alginate shell (FA-MTX/PFH@alginate NDs) as a tool for smart ultrasound-guided chemoradiotherapy with controlled release. This composite could enhance the anticancer activity of chemotherapeutics and reduce side effects to normal tissue during breast cancer treatment in vitro [cancer cell (4T1)] and in vivo. In addition, MTX-loaded nanostructures with 26.7 ± 1.5 nm showed high inhibitory effects on tumor growth. Furthermore this nanostructure had no significant hemolytic activity and organ toxicity in combination with ultrasound exposure and X-ray irradiation. Lastly, FA-functionalized MTX/PFH@alginate NDs were accumulated selectively in the tumor region (Samani et al., 2023).
3.1.2 Stimuli-responsive polymeric nanoparticles
Various polymeric NPs have been developed to enhance the effective therapeutic concentration of the drug at the target site (Lodhi et al., 2022; Shahrousvand et al., 2022; Yoosefi, 2022). Delivery approaches such as stimuli-responsive NPs are being exploited. Such stimuli include chemical and physical changes, and the chemical stimuli are divided into pH, enzyme, and redox activity. In contrast, the physical stimuli can include light, magnetic field or temperature. Hence, using stimuli-responsive approaches can be advantageous in the targeted delivery of tumour cells and other disorders (Li et al., 2013a).
Asad et al. (2021) demonstrated the effectiveness of pH-responsive MTX NPs loaded into chitosan hydrogel to treat psoriasis. Eudragit-100 (E100) was used to make the hydrogel stimuli-responsive (pH-sensitive). The adopted method was the o/w emulsion solvent evaporation method (Figure 2). The average size of the NPs was 256 nm, with a loading efficiency of 86%. The ex-vivo permeation study showed that 81% of MTX was retained in the epidermis following the hydrogel application. No significant symptoms of hyperkeratosis and parakeratosis were observed. Moreover, the studies on the imiquimod-induced psoriasis model exhibited promising therapeutic activity. Histopathological studies showed reduction of inflammation and redness in the MTX-treated groups following topical administration.
In another research, chitosan was employed with fucoidan to assemble MTX-loaded NPs to study their effectiveness in lung carcinoma. It was shown that NPs with an average size of 300 nm were stable in the stomach acidic environment and improved the oral bioavailability in lung cancer. The NPs induced apoptosis of the lung cancer cells and were safe toward fibroblasts. The NPs exhibited biological activity and could suppress the growth and proliferation of lung carcinoma cells about 7-fold more as compared to free drug. Altogether, the nanosystem was found to be mucoadhesive and pH-responsive for the MTX oral delivery (Coutinho et al., 2020). In a novel study, Ganoderma lucidum polysaccharide (GLP) was utilized because of its antioxidant and antibacterial activity to develop a pH-sensitive nanosystem for the delivery of hydroxycamptothecin (HCPT) and MTX for cancer therapy. The developed NPs had a size of 190 nm and exhibited good loading capacity, i.e., 21.5% for MTX and 22.6% for HCPT. GLP NPs released the drugs under the acidic conditions of the tumour cells; however, the NPs remained stable at the other physiological pH. Moreover, tumour suppression was observed following the in vivo administration (Nezhad-Mokhtari et al., 2019). Similarly, an ultraviolet (UV) light and pH dual-responsive polymeric nanosystem was developed to deliver MTX in tumour therapy. Supramolecular polymeric NPs were fabricated from acetal-modified β-cyclodextrin-azobezene and poly(α-cyclodextrin) (Dai and Zhang, 2018).
Supramolecular polymeric NPs were discovered to have an average size of 85 nm, with an encapsulation efficiency of 21.3%. pH responsiveness was due to acetal group, and UV responsiveness was due to azobenzene, which was attributed to the controlled release of MTX upon UV irradiation. Based on the function of pH, over 65% of MTX was released in 12 h according to fast delivery kinetics. Upon UV irradiation, the drug was released in a slow manner after the initial burst release at the tumor site. A derivative of chitosan, thiolated carboxymethyl chitosan, was used to develop another kind of NPs. The polymer self-assembled via disulfide linkage in deionized water to synthesize NPs. NPs were discovered to have an average size of 160 nm, with an encapsulation efficiency of 43.4%. The slow and sustained release of MTX from the polymeric NPs was beneficial for tumour suppression. The inhibition rate of the MTX-loaded NPs was estimated to be 90% against HeLa cells. Owing to the existence of the carboxyl group on the polymer, the NPs were swelled and released the drug in the acidic environment based on the function of the surface area of NPs in contact with water and pH (Gao et al., 2014). A pH-responsive nanohydrogel was also established for MTX delivery in cancer therapy. In this regard, poly(hydroxyethyl methacrylate) (PHEMA) was crosslinked with poly(ethyleneglycol) diacrylate (PEG-DA) via chain transfer polymerization technique. The mean size of MTX-loaded nanohydrogel was 194 nm. MTX was coupled with polymeric structure by a pH-reponsive esteric bond, facilitating the drug release in acidic pH. PHEMA and PEG-DA conjugated nanohydrogel induced cytotoxicity in the MCF-7 cancer cells. pH-responsive conjugate enhanced the toxicity and cellular uptake in a cancer animal model (Farzanfar et al., 2021).
To successfully deliver MTX and curcumin for treating colorectal cancer, three-block copolymers polycaprolactone-poly(ethylene glycol)-poly(caprolactone) (PCL-PEG-PCL) were proposed (Zhang et al., 2021). PCL-PEG-PCL NPs presented good biocompatibility, low toxicity, good stability, and amphiphilicity. These NPs exhibited an encapsulation efficiency of 77%–95%, with an average particle size ranging from 95 to 135 nm. PCL-PEG-PCL NPs demonstrated dose-dependent toxicity to CL40 and SW1417 cells. The hybrid NPs showed improved cellular absorbance in the cancer cells and induced apoptosis, as revealed by the florescence staining assay.
In a novel hybrid approach, chitosan NPs loaded with MTX were functionalized with photocatalytic TiO2 NPs. The photocatalytic controlled release of MTX was facilitated through the developed nanosystem based on the function of TiO2. Upon irradiation with UV, the fabricated drug delivery system released MTX as TiO2 triggered the polymeric bond rupturing in the presence of light. The entrapment efficiency for MTX was around 75%, with a particle average size of 240 nm. CS NPs without TiO2 NPs coating did not release a significant percentage of a drug upon UV irradiation, whereas TiO2 NPs improved the release of MTX up to 40% in the presence of UV light. The cytotoxicity data confirmed that the developed nanosystem was cytotoxic against MCF-7 cells, hence showing promise in cancer therapy (Al-Nemrawi et al., 2022). In order to pass through the blood–brain barrier (BBB), which is as physical/biochemical barrier, the transactivator of transcription (TAT) peptide was used to produce mesoporous silica nanoparticles-linked TAT (MSN-NH-TAT) with the aim of improving MTX (MTX-loaded MSN-NH-TAT) penetration into the brain. As a result, brain-to-plasma concentration ratio and the drug blood terminal half-life was improved for delivery of MTX into the brain as compared with the use of free MTX (Shadmani et al., 2023). Poly(3-hydroxybutyrate)/chitosan-graft poly(acrylic acid)-conjugate hyaluronate was fabricated and used for targeted delivery of MTX to colon cancer cell lines (Caco-2). The overall copolymeric system showed the maximum drug loading efficiency about 69.7% ± 2.7% (4.65 mg/g) and the release about 98.6% ± 1.12%, at pH 7.4. The results of cytotoxicity exhibited IC50 of 11.7 μg/mL and enhanced level of DNA breakage as well as high reactive oxygen species (Hanna et al., 2023).
Biotin-PEG-conjugated nanogels of carboxymethyl polyethyleneimine (Biotin-PEG-CMPEI) were synthesized with a size of 100 nm and ζ-potential of +15 mV (pH = 7.4) for active targeted delivery of MTX in triple negative breast cancer (TNBC). This nano-system exhibited zero-order release kinetics of MTX at pH = 7.5 and a swelling-controlled release at pH = 5.5. The IC50 of the MTX-loaded Biotin-PEG-CMPEI was about 10 folds lower than the free drug, while the unloaded nanogels had no significant toxicity. In a mouse model, the volume of tumor, animal mortality rate, lung metastasis and glomerular damage were lower after treatment with the MTX-loaded Biotin-PEG-CMPEI as compared to the group receiving the free drug (Abolmaali et al., 2022).
ROS-responsive hyaluronic acid–MTX was prepared combining chemotherapy with photothermal therapy (PTT) for cancer treatment. The synthesized thioketal moiety linker (TK) of MTX and hyaluronic acid (HA) (HA-TK-MTX (HTM)) in the presence of the photothermal agent IR780 (IHTM NPs) allowed reaching a tumor inhibition rate of 70.95% for 4T1 tumor-bearing mice due to ROS-responsive NPs (Yun et al., 2022). Amphiphilic cross-linked poly(vinyl alcohol-methyl methacrylate) (PVA-PMMA) copolymer NPs (NPs) as pH-responsive system were fabricated to load MTX. It was shown that 47.24% and 65.39% of the drug were released at pH = 7.4 and pH = 5.8, respectively, after 96 h. MTT assay confirmed the effectiveness of NPs against MCF-7 cells (Ziyaee et al., 2023).
3.2 Carbon-based nanostructures
Recently, nanostructures based on carbon are gaining focus as medication delivery approaches to treat cancer effectively, inflammation, arthritis, and tumours. Carbon-based NPs have improved water solubility, biocompatibility, high stability, and tunable surface modification properties (Li et al., 2010). In one research, carbon NPs were synthesized using sucrose and glucose using ultrasounds. Later, these carbon NPs were anchored with ethylene diamine and MTX to be delivered to cancer. These developed carbon NPs were used as fluorescent nanocarriers for MTX delivery in human hung cancerous cells (H157) (Ajmal et al., 2015). The NPs were studied through cell adhesion assay, LDH assay, and sulforhodamine B bioassay in H157 cells and presented cytotoxicity and high affinity to the cancer cells. Similarly, other graphene-based NPs, for example, graphene oxide (GO), were applied to deliver drugs. Graphene-based nanomaterials have high loading ability, interesting release profile and can simultaneously incorporate both hydrophilic and hydrophobic drugs. A GO-based nano-delivery system was prepared using Hummer’s method followed by ultrasonication and loaded with MTX. The developed GO loaded with MTX presented cytotoxicity against different cell lines such as porcine skin fibroblasts (PEF), hepatocellular cancerous cells (HepG2 cells), and embryonic kidney cells derived from human (HEK293A cells) (Abdelhamid and Hussein, 2021). Various carbon-based drug delivery approaches are being exploited to deliver the drug to cancer cells. In a hybrid approach, multi-walled carbon nanotubes (MWCNTs) and C60-fullerenes were conjugated by 1,3-dipolar cycloaddition using glycin and paraformaldehyde together to deliver MTX in breast cancer. The nanosystem was studied regarding cytotoxicity, cellular uptake, hemocompatibility, and pharmacokinetics. C60-fullerenes/MWCNTs improved cellular uptake and retention along with improved cytotoxicity. C60-fullerenes/MWCNTs allowed achieving improved pharmacokinetic profile with enhanced AUC, improved bioavailability, decreased clearance, and reduced 1C50 values (Joshi et al., 2017). Another hybrid approach was composed of fluorescent carbon dots (CDspPEG) and PEG. The microwave pyrolysis approach used gelatin and PEG as starting materials to prepare CDs-PEG (Figure 3) (Arsalani et al., 2019). MTX-loaded CDs-PEG presented biocompatibility and anticancer activity. The average size of these nano-complexes was 6 nm with regular spherical morphology. CDs-PEG emitted blue photoluminescence with a highest quantum yield of 34%. The tumour growth was inhibited with improved targeted cancer therapy with good formulation stability.
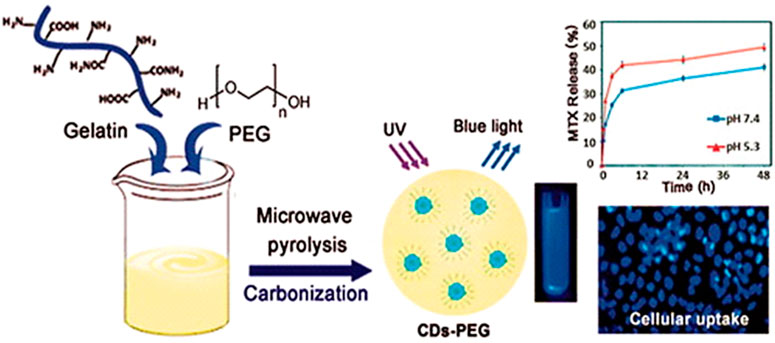
FIGURE 3. A diagram depicting the PEG-passivated carbon dot (CDs-PEG) synthesis via microwave carbonization, reproduced from (Arsalani et al., 2019), under the terms of the creative commons cc by license.
In another study, oxidized MWCNTs together with chitosan were used in conjugation to deliver MTX to lung cancer. The developed system exhibited pH-responsive with fast and higher release in the acidic environment. A biocompatibility test was conducted using NSCLC H1299 and MRC-5 cells, showing the anticancer properties of the developed nano-system (Cirillo et al., 2019). Currently, proteins are being used as coating agents on the surface of carbon biomaterials using amide groups to improve cell adhesion and attachment. Moreover, the protein coating imparts better stability and compatibility in the biological environment. The used bovine serum albumin coating on carbon NPs was loaded with MTX. The NPs exhibited spherical morphology with improved stability. The developed nanosystem presented cytotoxicity in A549 lung cancer cells (83% cell death) with high apoptosis rate. Around 79% of MTX was released in 48 h and no hemocompatibility issues were reported (Muthukumar et al., 2014). Another carbon-based system, fullerenols (derivatives of fullerenes), was used as a nanocarrier for its improved cellular permeation. Fullerenols were conjugated with MTX via a hydrolysable ester bond. The developed MTX conjugated fullerenols improved the bioavailability, cytotoxicity, and pharmacokinetics of the drug (Bahuguna et al., 2018). The average fullerenols size was 94.60 nm with limited size dispersion. Compared to the free MTX, the protein binding was shown to be lower. The MTX release was pH-dependent, with an improved release (85%) in cancer cells. There was a significant reduction in IC50 value for the MTX conjugated fullerenols. The florescent dye-tagged MTX conjugated fullerenols presented higher accumulation inside the cancer cells. Moreover, in vivo studies demonstrated the improved AUC by 6.15 times, and plasma half-life was improved by 2.45-fold as compared to the parenteral administration of a single dose. Also, conjugated fullerenols with MTX were compatible with erythrocytes while exhibiting higher toxicity to cancer cells.
3.3 Lipid-based nanoparticles
3.3.1 Non-responsive lipid-based nanoparticles
Lipid-based NPs are the most versatile drug delivery vehicles being exploited for their potential to treat various disorders, including cancer. Owing to the nature of lipids, these carriers can overcome the biological barriers. In one study, solid lipid NPs (SLNs) were developed using the fatty acid coacervation technique. Transferrin or insulin served as anchors, and they were stuffed with an MTX active lipophilic agent (Figure 4). Transferrin or insulin was selected because their proteins are overabundantly expressed on the blood-brain barriers. For the functionalization of proteins on SLNs, a PEG spacer was used. These developed SLNs presented higher cytotoxicity against glioblastoma cells and improved bioavailability in Wistar rats. Significant levels of SLNs were found in the tumour cells and suppressed the tumour growth in glioma models (Muntoni et al., 2019).
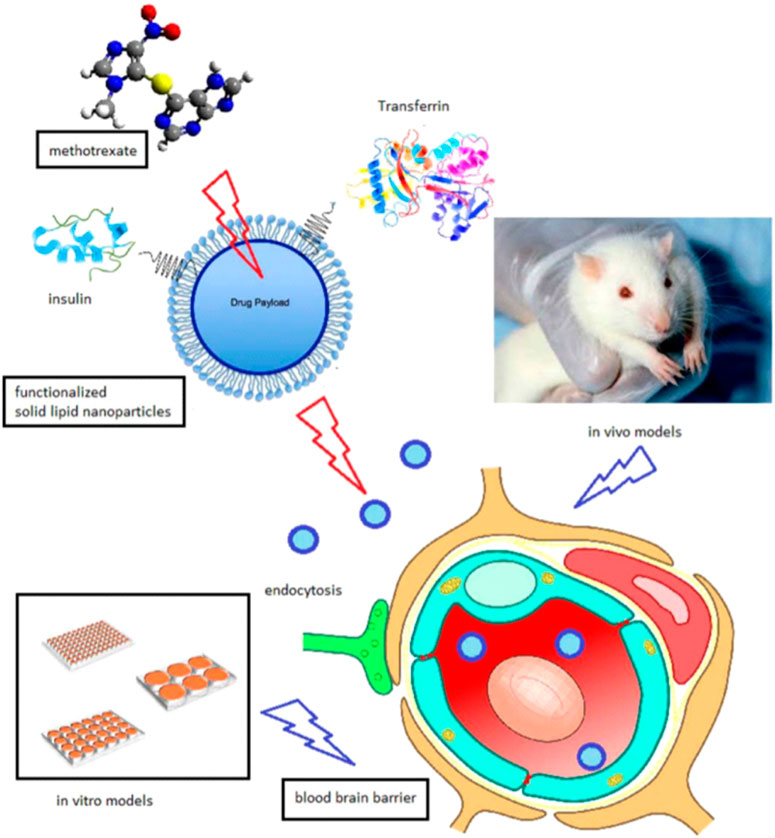
FIGURE 4. Schematic representation of solid lipid NPs loaded with MTX with surface conjugation of insulin or transferrin for the targeting of glioblastoma. Reproduced from (Muntoni et al., 2019), under the terms of the creative commons cc by license.
For the treatment of psoriasis systemic therapy, targeted therapy was developed using lipid NPs. MTX and etanercept were co-loaded into lipid NPs and mediated by carbopol hydrogel to deliver a drug into the skin with minimal transdermal permeation. The SNLs did not elicit cytotoxicity to the human keratinocytes and fibroblasts. Therapeutic amounts of drugs were deposited across the pig ear with reduced transdermal permeation. Cetyl palmitate was used as a lipid component, and the drugs were conjugated to the developed SLNs. The SLN size varied from 292 to 356 nm. The appearance of the SLN-loaded hydrogel was transparent, uniform, and glossy. The mucoadhesive characteristic of the hydrogel improved the MTX retention and reduced the systemic circulation in psoriasis (Ferreira et al., 2017). In a similar approach also addressed to MTX delivery, a pegylated lipid core containing strearic acid was created in order to overcome the limitation of low bioavailability of the drug. The pegylated SLN had an average size of 130 nm with limited size dispersion and spherical morphology. The developed SLNs were compatible with the blood cells and improved the pharmacokinetics profile in tumour models. SLNs improved the blood circulation profile with efficient tumour cell uptake, as evident through SPECT imaging. Because of the presence of stearic acid, the bioavailability of the SLNs was effectively improved in BALB/c mice (Kakkar et al., 2015). Another topical treatment approach for psoriasis was developed using MTX-loaded nanostructured lipid carriers incorporated into the gel. The solvent diffusion technique was utilized to synthesize the nanostructured lipid carriers, which were later loaded into the carbopol gel following optimization. The deposition studies of the nanostructured lipid carriers were carried out on human cadaver skin which exhibited significant deposition across the biological barrier (71%) and resulted in the reduction of the psoriasis stmptoms. The amount of pro-inflammatory cytokines were reduced following the gel application in the BALB/c mic, as compared to the marketed MTX gel (Agrawal et al., 2020). Lipid NPs have also shown promising results in osteosarcoma, where the traditional drug delivery systems could not slow the progression of the metastasis. MTX and edelfosine were co-loaded into the lipid NPs to synergistically improve the activity against osteosarcoma. Edelfosine suppressed the metastasis in the cancer cells, and MTX reduced the growth of osteosarcoma. Lipid NPs were found to be internalized in high concentration inside the cells irrespective of the expression of dihydrofolate reductase (González-Fernández et al., 2015). In another dual drug delivery approach, SLNs were fabricated to deliver MTX and calcipotriol for the topical therapy of psoriasis. Precirol ATO 5 and squalene were used in different ratios to synthesize the SLNs. The average particle size was in the range of 270–320 nm; however, the particle size increased with increasing concentration of Precirol. The developed SLNs improved the permeation of the loaded drugs across the skin (2.4–4.4 times higher in comparison with control). Confocal laser scanning microscopy (CLSM) exhibited improved permeation following the internalization of SLNs across the biological membrane. The promising outcome of the study was that drugs with different polarities were successfully embedded into one system without using any complex methodology. SLNs did not reveal any irritation to the skin and served as a potential vehicle for the efficient treatment of psoriasis (Lin et al., 2010).
SLNs have also been developed in a hybrid approach by anchorage with moieties such as fucose to target breast cancer. Gelucire 50/30 and stearic acid served as core, whereas stearyl amine and phospholipid 90 served as a coating. Fucose was anchored to the SLNs to target the breast cancer cells precisely while the off-site drug loading was minimized. SLNs demonstrated enhanced cellular absorbance in the breast cancerous cell with high drug release inside the cells and improved bioavailability. SLNs presented higher cytotoxicity at a lower IC50 value and facilitated programmed cell death, i.e., apoptosis. Moreover, the permeation profile was very promising, with improved anti-cancer activity due to the higher biodistribution of SLNS at the target site (Garg et al., 2016).
In order to prepare stable nanostructured lipid carriers (NLCs) for the encapsulation of MTX, glyceryl monostearate (GMS as solid lipids) and Miglyol 812 (MI1 as liquid lipids) were used to reduce adverse side effects and increase the low solubility and non-specific targeting of MTX. The nanoystems exhibited long-term stability and spherical morphology along with an internal cubic crystalline structure, as revealed by small-angle X-ray scattering (SAXS) analysis. MTX was encapsulated at 85 ± 0.9% and in vitro release of MTX was 52% ± 3.0 in 24 h. Furthermore, the MTT assay showed that MTX-loaded NLCs exhibited more toxicity against HeLa and MCF-7 cell lines as compared to free drug. In addition, hemolysis was assessed to be <10% (Li et al., 2019).
Dendritic nanocarriers such as lysine dendrimers were used for drug delivery owing to their high drug-loading capacity and high resistance to proteolytic degradation. The poly-lysine dendritic nanocarrier (P4LDN) for targeted chemotherapy was prepared and loaded with an epidermal growth factor receptor (EGFR)-specific short peptide E2 (ARSHVGYTGAR) and MTX. MTX was linked to the nanocarrier by using cathepsin B cleavable spacer (glycine–phenylalanine–leucine–glycine (GFLG)). The results showed that MTX released from the nanosystem decreased the percentage of cells in the G2/M phase and enhanced the percentage of cells in the S and G0 phase in both MDA-MB-231 (EGFR overexpressed) and SiHa (EGFR negative) cells (Narayanan et al., 2022).
3.3.2 Stimuli-responsive lipid-based nanoparticles
Currently, polymer and lipid-based hybrid NPs are being explored for their potential to deliver MTX in various disorders. Such hybrid NPs have high (Kumar et al., 2022) biocompatibility with improved blood circulation time (Surve et al., 2022). Also, these systems can further be modified in response to specific stimuli, including redox potential, pH, and temperature (Teixeira et al., 2017; Kumar et al., 2022; Chang et al., 2023).
Kumar et al. synthesized MTX-loaded nano-lipid polymeric system of poly(lactic-co-glycolic acid) (PLGA) in phosphatidylcholine (MTX-NLPHS) to release MTX through endocytosis against MCF-7 cell lines (encapsulation efficiency about 86.48% ± 0.31%). This system was not agglomerated due to highly negative zeta potential. In vitro release of MTX reached 100% in the long time. In a MTT study, the cell toxicity of MTX-NLPHS was lower with decreasing concentration of MTX. Meanwhile, at the higher concentration of MTX, toxicity was enhanced as compared to free MTX. ROS was also increased in the cells exposed to MTX-NLPHS, resulting from the controlled transport of drugs into the cells by PLGA (Kumar et al., 2023). In another study, NPs based on hyaluronanm (HA)-coated lipid with low molecular weight (<10 kDa) and high molecular weight (700 kDa) were fabricated and tested for tumor targeting towards the HA receptor (CD44). In vitro results showed that NPs with high molecular weight influenced significantly the affinity towards CD44 on B16F10 murine melanoma cells. In addition, low-molecular-weight NPs had weak binding on B16F10 murine melanoma cell lines. Lastly, MTX as a chemotherapy drug was loaded into high-molecular-weight HA–tsNPs, resulting in slow drug diffusion with a half-life of 13.75 days (Mizrahy et al., 2014). Lipid polymer hybrid NPs were also tested to co-deliver two therapeutic drugs (Teixeira et al., 2017). Fucose-anchored lipid-polymer hybrid nanoparticles (Fu-LPHNPs) were fabricated to co-encapsulate MTX and aceclofenac (ACL) for targeted/controlled delivery purposes against MCF-7 and MDA-MB-231 cells. In vitro drug release studies showed that the release of ACL and MTX was rapid in the early 12 h (over 25%), then slower and at last the release was sustained over 168 h. The drug bioavailability when LPHNP was used as carrier was greater than free MTX and ACL. It was reported that the therapeutic activity of MTX was improved by ACL and the inhibitory effect of MTX/ACL-loaded LPHNPs on cancer growth in a breast cancer mouse model was greater as compared to the simple combination of free MTX and ACL or MTX (Teixeira et al., 2017; Rui et al., 2022).
3.4 Inorganic nanostructures
At present, there has been a significant advancement in the use of inorganic compounds for developing drug delivery systems (Borse et al., 2023). Some selected inorganic NPs have low toxicity, tunable surface properties, improved intracellular drug delivery, and comprehensive functionality (Baqeri et al., 2022; Ekinci et al., 2023). Inorganic compounds such as calcium carbonate, iron oxide, silica, gold, and calcium phosphate are the most popular among the widely explored inorganic nanomaterials (Xu et al., 2006; Ram Prasad et al., 2019; Wu et al., 2022a). Currently, inorganic substances are being used to deliver MTX to different disorders.
Iron oxide and, specifically, Fe3O4 NPs have been studied to deliver MTX for effective anticancer treatment. Fe3O4 NPs (average size between 10 and 20 nm) were covered with a molecularly imprinted polymer (MIP) imparting thermal-resposive properties. These magnetic seeds could produce heat under the effect of an external magnetic field. Hence, the MIP-coated magnetic seeds exhibited superparamagnetic nature with an 80% MTX adsorption ratio. Under a magnetic field, these nanocarriers released MTX by cleavage of hydrogen bonds and demonstrated therapeutic activity in cancer cells. Therefore, this concept yielded a unique thermosensitive strategy for targeted drug delivery to cancerous tissue based on outer stimuli (Kubo et al., 2018). Although polymeric nanostructures are already being studied because of their biocompatibility, inorganic substances are often being used in conjunction with polymeric substances to increase their compatibility with healthy cells and tissues. The inclusion of gold NPs (Au NPs) into a polymeric shell facilitates imaging following the near-infrared photothermal stimulus. Following this approach, MTX and AuNPs were combined using the emulsion-diffusion-evaporation method and embedded into pegylated-poly(DL-lactic-co-glycolic acid) nanospheres (Lima and Reis, 2015). These developed hybrid nanostructures released MTX based on pH and temperature changes. Moreover, these Au-conjugated nanostructures demonstrated lower cytotoxicity to monocytes and macrophages as compared to Au NPs alone. The uptake of these nanostructures was higher in the cells based on clathrin and caveolae-dependent endocytosis. The presence of Au in the nanostructures dramatically decreased levels of pro-inflammatory cytokines that were produced by monocytes and macrophages. Moreover, based on the theranostic properties of Au, these nanostructures are novel vehicles for therapeutic as well as diagnostic function, showing highly effective for chemo-photothermal therapy.
Calcium phosphate is another inorganic compound that can be utilized for developing drug delivery systems. In one study, calcium phosphate NPs (CaP-NPs) were synthesized using the reverse micelles technique and loaded with MTX. The average size of the CaP-NPs was estimated to be 262 nm, with an entrapment performance of 58%. A minimum fraction of MTX was released at physiological pH, whereas over than 90% of the medication was released after three to 4 h at endosomal pH. The confocal imaging demonstrated the localization of CaP-NPs in CHO cell lines with promising intracellular drug delivery (Mukesh et al., 2009).
In another study, pH-sensitive magnetic hydrogels were developed via embedding of chemically precipitated Fe3O4 NPs into chitosan, carboxymethyl cellulose (CMC), and β-cyclodextrin (β-CD) hydrogel for the delivery of MTX (Naderi et al., 2020). The swelling capacity was observed for the hydrogels with magnetic NPs, and the maximum drug release (92%) was detected for the CMC-based hydrogels. The developed magnetic NPs were responsive to pH and magnetic field. A higher swelling index was observed for the magnetic hydrogel at high pH.
Silica is also used for fabricating nanocomposites for the delivery of MTX. Polypyrrole and mesoporous silica dioxide (PPy/mSiO2) were used to synthesize nanocarriers to deliver MTX for cancer therapy. Moreover, graphene quantum dots (GQDs) were coated onto the surface of these nanocarriers. mSiO2 improved the encapsulation efficiency of the nanocarrier, and PPy facilitated the heat development under exposure to outer near-infrared light. Upon irradiation with near-infrared light, the hydrogen bond between mSiO2 and GQDs was broken, and then MTX was released in a controlled manner. PPy/mSiO2 NPs coated with GQDs provided a better drug delivery platform based on the theranostic function of light (Zhao et al., 2017). Bovine serum albumin (BSA) was capped onto the AuNPs and loaded with MTX. The NPs demonstrated inhibition of breast carcinoma cells MCF-7. The NPs also induced the apoptosis based on the DNA ladder gel electrophoresis and flow cytometry analysis. These BSA-MTX-AuNPs improved uptake in MCF-7 cells as well as the stability and solubility of MTX, therefore demonstrating higher anti-cancer activity as compared to Au NPs alone. (Murawala et al., 2014). Some MTX-loaded nano-drug delivery systems are listed below with their composition and outcomes (Table 1).
The use of different phases including an inorganic/organic shell modified with polymer have been applied as multifunctional nanomaterials with the aim of protecting the inner core against oxidation, pH changes, high temperature, radiation, and aggressive agents. CoFe2O4/mSiO2-NH2/poly(methacrylic acid (MAA)-co-itaconic acid (IA))/MF3 nanocomposite as a smart core/double shells (CDS) carrier was synthesized for the simultaneous release of MTX and doxorubicin (DOX) against MCF-7 human breast cancer cells. This nano-composite system was sensitive to pH for a controlled drug release. In addition, the in vitro cytotoxic studies revealed a noticeable tumor inhibition as compared with the free forms of drugs (Ghazimoradi et al., 2023).
A novel triple-responsive nanocarrier including magnetic (for directing drug to cancerous cells), pH (due to lower pH values of the cancerous cells) and temperature responsiveness (due to higher temperature of the cancerous cells) has been developed for the targeted delivery of anticancer drug. For this aim, superparamagnetic Fe3O4 NPs coated with poly(N-isopropylacrylamide) grafted with chitosan (size of 85 nm) was fabricated and proposed for MTX delivery. This nanocarrier had the highest release percentage at pH = 5.5°C and 40°C, conditions which were similar to cancerous cell. The experiments of DAPI staining and MTT assay of MTX-loaded nanocarrier confirmed the effective anticancer performance by degrading the cell nucleus DNAs against the A549 lung cancer cell line (Moradi et al., 2022). In order to decrease severe adverse effects, combination of chemotherapy with photothermal therapy (PTT) or photodynamic therapy has been proposed (Muangsopa et al., 2023). Polydopamine (pDA)-modified ZIF-8 NPs loaded with MTX (pDA/MTX@ZIF-8 NPs) were fabricated for chemo-photothermal therapy: the nano-system displayed excellent photothermal effects under NIR irradiation, which yielded antitumor effect and allowed decreasing the dose of MTX. These pH-responsive NPs induced apoptosis through reducing mitochondrial membrane potentials (MMPs) in MG63 cells (Yin et al., 2022).
MoS2 nanoflower (MoS2 NF)-doped chitosan (CS)/oxidized dextran (OD) hydrogels were developed to treat colon cancer. 5-Fluorouracil (5-FU) was combined with polyethylenimine (PEI) and covered on MoS2 nanoflower (MoS2 NF)-doped CS/OD hydrogels to form 5-FU/PEI/MoS2. In the next stage, 5-FU/PEI/MoS2 was encapsulated by 1-tetradecanol (TD) to obtain TD/5-FU/PEI/MoS2 (TFPM), which was in turn co-encapsulated with MTX in the CS/OD hydrogels. Because the electrostatic attraction between CS and OD is pH-sensitive, MTX and TD/5-FU/PEI/MoS2 were released from the hydrogels at pH 7.4 (Liang et al., 2023).
In another study, Au and Fe NP conjugates with MTX were obtained by metal–vapor synthesis (MVS) and exhibited an average size of 8.3 and 1.8 nm, respectively. Anticancer activity based on MTT and NR assays was assessed. The results of Fe conjugates with MTX exhibited the highest toxicity as compared to MTX-loaded Au NPs against the lung adenocarcinoma cell line (Vasil’kov et al., 2023).
MnO2 NPs coated with biocompatible poly(dimethyl-amino-ethyl methacrylate-Co-itaconic acid) (DMAEMA-Co-IA)-conjugated MTX were prepared based on pH-sensitive targeted magnetic resonance imaging (MRI) contrast agent and innovative radio-sensitizing system. MTT assay for MnO2@Poly(DMAEMA-Co-IA)-MTX NPs showed higher inhibitory effect on MCF-7 cell viability as compared to free MTX after 24 and 48 h with insignificant hemolytic activity (Ziyaee et al., 2023).
4 Delivery and co-delivery systems of methotrexate with other drugs
The effectiveness of current cancer treatments is limited; therefore, researchers are studying the therapeutic and adverse effects of combining various drugs or treatment approaches (i.e., involving co-delivery drug systems) to achieve better outcomes (Mujokoro et al., 2020). Cancer treatments involving a single drug are often insufficient to treat cancer repeatedly due to potential drug resistance that might develop over time (Komarova and Wodarz, 2005; Zhang et al., 2017). Instead, a co-delivery drug system is required in combination therapies in the effort to avoid the development of drug resistance, just like in most clinical therapies where two or more therapeutic agents are used (Guo et al., 2014). The advantages offered by the co-delivery of drugs include the following: 1) extended half-lives of drugs, 2) enhanced drug targeting, 3) improved bioavailability, and 4) reduced adverse reactions to chemotherapy drugs (Torchilin, 2001; Qi et al., 2017). Co-delivery systems must be fabricated and designed according to the cargo (i.e., co-delivered drugs) and the vectors (i.e., the nanocarrier or nanoparticle) to finely tune the structural and physical properties. Therefore, effective co-delivery techniques should consider the interaction among the components, the balance of contradictory factors, and a synergistic treatment strategy (Yang et al., 2018; Meng et al., 2020). An example of co-delivered drugs currently in practice is paclitaxel (PTX) and cisplatin (DOP) which are delivered simultaneously for lung cancer therapy. Furthermore, choosing the appropriate vector type and drug loading strategy to successfully deliver cancer drugs is crucial. For example, when intravenously administering nanomedicine, factors to consider are 1) internalization, 2) circulation, 3) drug release, 4) accumulation, and 5) penetration to ensure a high level of therapeutic effectiveness (Blanco et al., 2015; Khan et al., 2015). Therefore, a weakness in one aspect of the co-delivery system will decrease its performance. In addition, designing co-delivery systems requires that vectors (i.e., micelles, organic/inorganic NPs, and microgels) and cargoes interact with each other and that the loaded reagents act synergistically (He et al., 2015; Xie et al., 2015; Xu et al., 2018). Moreover, it should be noted that although vectors (i.e., nanocarriers) pose some benefits (i.e., permeability and retention) as a result of their nanoscale, further modifications are still essential to enhance their functionality, for example, their loading methods (as indicated in Figure 5). Furthermore, loading methods involve physical intractions (i.e., hydrophobic and electrostatic intractions), and they can be classified as either physical (i.e., simple agitation of reagents over time, e.g., polyanions) or chemical (i.e., several sequential chemical reactions, e.g., mesoporous NPs) process. These loading methods are widely studied and are found in the experimental data obtained from forty (40) different nanoparticle systems for the co-delivery of methotrexate (MTX) combined with other cancer-inhibiting drugs, reported in the literature from 2018 to 2022. Table 2 reports an overview of these studies with their advantages and the indications from in vitro and in vivo experiments for various cancers.
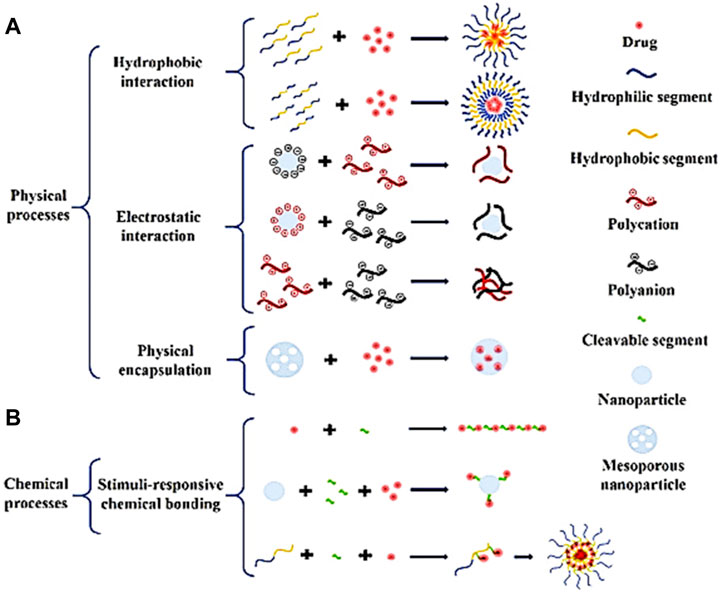
FIGURE 5. This illustration shows the typical loading methods for synthesizing nanoparticle systems co-delivery drugs: (A) Physical processes: hydrophobic interaction, electrostatic interaction and physical encapsulation; (B) Chemical processes: Stimuli-responsive chemical bonding. Figure reproduced with permission from Meng et al. (2020)).
Recently, Shakeran et al. (2022) were the first to report on a new nanoparticle-based co-delivery system involving signal transducer and activator of transcription 3 (STAT3) siRNA (STAT3 is a key player in cell proliferation, survival, and cell division) (Ezra Manicum et al., 2022) and MTX in breast carcinoma cells. In this research, the authors functionalized mesoporous silica NPs with the polysaccharide chitosan, grafted covalently by aminopropyl triethoxysilane and glutaraldehyde, as illustrated in Figure 6. In addition, the co-delivery system (i.e., MTX and STAT3 siRNA) was studied using analytical methods (i.e., confocal laser scanning fluorescence microscopy and flow cytometric analysis) to treat breast carcinoma (i.e., MCF-7 cells). This co-delivery system was useful to considerably decrease the viability of the breast cancer cells. In antoher study, biodegradable mPEG-PCL copolymer NPs were fabricated by polymerization of ε-caprolactone dehydrated monomer in the presence of the initiator (dry methoxy polyethylene glycol) and catalyst (Sn(Oct)2). These NPs were used as carriers for the delivery of MTX (average size of 51.89 ± 0.46 nm) or co-delivery of MTX and curcumin (average size of 88.21 ± 0.31 nm) after being conjugated with the drug(s). The amount of drug released from NPs at pH = 5.5 was higher than that released at pH = 7.4. MTT results showed lower cell survival with increasing drug concentration inside the NPs. The NPs conjugated with MTX and curcumin were associated to the highest cancer cell mortality due to pH sensitivity and synergistic effect/accumulation of drugs at tumor sites (Danafar et al., 2022).
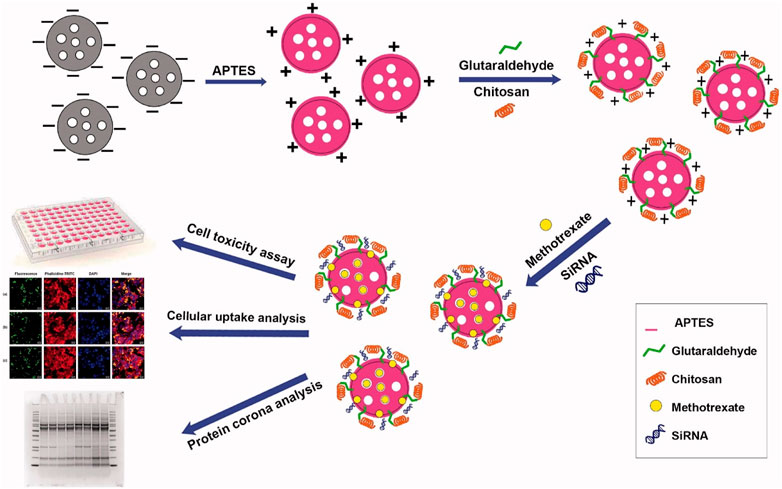
FIGURE 6. This illustration shows the delivery system involving signal transducer and activator of transcription 3 (STAT3) siRNA and MTX. Figure inserted with permission from (Shakeran et al., 2022).
In 2021, Shakeran et al. (2021) evaluated the cytotoxicity of the delivery system involving MTX-loaded, functionalized mesoporous silica NPs (MSNs) against breast cancer cells using confocal microscopy. Their findings were that the co-delivery system was effective against MCF-7 cells at a low dose of 0.5 μM MTX.
In addition, Rathee et al. (2022) demonstrated the promising carrier ability of nanostructured lipid carriers (NLCs) for MTX in the cytotoxic investigation (i.e., MTT assay) against MCF-7 and HeLa cell lines. This investigation revealed that the suggested drug delivery system exhibited significant cytotoxicity towards the specified cell lines, killing 55% of the HeLa cells and 40% of the MCF-7 cells.
Rui et al. (2022) recently synthesized a straightforward drug delivery system involving aminated mesoporous silica NPs (AMSNs) to load MTX, which was then encapsulated by electrostatic interaction with hyaluronic acid (HA). Spectroscopic methods (i.e., electron microscopic techniques, fourier transform infrared technique, and X-ray diffraction analysis) were used to confirm the successful synthesis of the drug delivery system. Flow cytometry experiments further showed increased inhibitory effects of the co-delivery system on hepatoma (SMMC-7721) cells, while the medication-free carrier was highly biocompatible.
In another study, Bruckmann et al. (2022) showed the usefulness of MTX and magnetic NPs in an in vitro study against melanoma cells. In addition, the investigation reported the high cytotoxicity displayed by the drug delivery drug for use in skin cancer treatment.
Mousazadeh et al. (2022) described the of niosomes comprising MTX and curcumin (CUR) for the co-delivery impact on human colorectal carcinoma cell lines (HCT-116). These niosomes were made utilising the thin-film htdration approach, and the codelivery system was effective against the indicated cancerous cells.
MTX and 10-hydroxycamptothecine (10-HCPT)-loaded pullulan NPs were assessed by Wu et al. (2022b) as a synergistic medication delivery mechanism for cancer therapy. Furthermore, MTX served as a targeting compound and a cytotoxic drug in the synthesized co-delivery system. According to the authors, MHNPs induced more cytotoxicity in HeLa cells when compared to individual drug-loaded NPs, suggesting that the co-delivery system make drugs to act synergistically and may be a practical antitumor agent.
In 2022, Mokhtar et al. (2022) described a hybrid nanocarrier co-delivery system for the concurrent distribution of MTX and aromatase inhibitor exemestane (EXE) for breast cancer treatment. First, EXE was enclosed physically in liquid crystalline NPs (LCNPs) using a carbodiimide reaction. Next, MTX was bonded chemically to lactoferrin (Lf), followed by the coating of the EXE-loaded LCNPs with MTX–Lf. The EXE and MTX exhibited a considerable synergistic effect on breast cancer cells after being embedded in the LCNPs. According to the study, dual drug-loaded LCNPs offer as a combined application of hormone treatment and chemotherapy agent for breast cancer.
Almawash et al. (2022b) synthesized a 5-Fluorouracil and MTX co-delivery system to be used as an anticancer agent in an in vivo study on female rats against breast carcinoma. The study outcomes showed that this nano-system could load and release medication sustainably over 14 days, as illustrated in Figure 7.
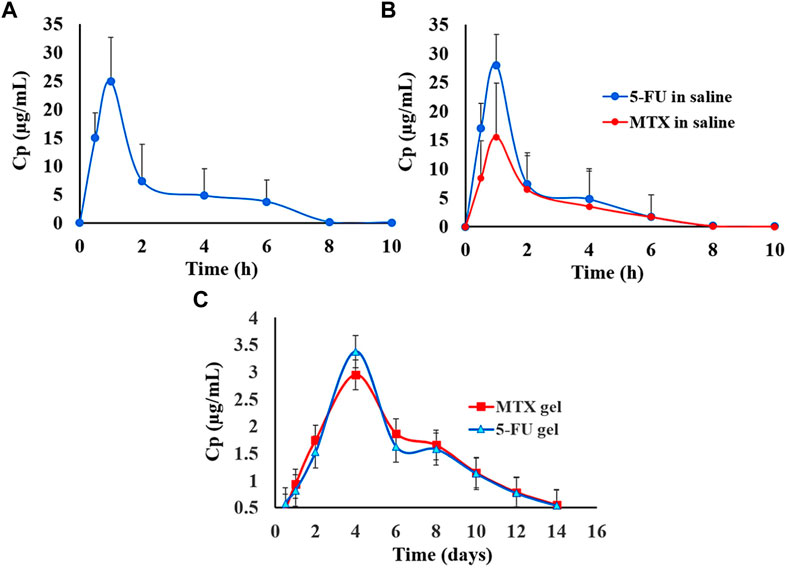
FIGURE 7. Graphical illustration comparing the in vivo release of 5-FU administered alone (A); 5-FU administered in combination with MTX (B); 5-FU administered alone and combined with MTX (C). Figure reproduced with permission from (Almawash et al., 2022b).
An additional study by Massaro et al. (2022) reported on a “smart” co-delivery system involving MTX and biotin loaded on halloysite nanotubes (HNTs) for anticancer applications with a specific focus on leukaemia. Thermographic and spectroscopic methods were used to characterize the nano-based drug delivery system. In the biological assays, both the biotinylated and MTX prodrugs played critical roles in the biological action of the cancerous treatment agents and showed promise.
Khatibi et al. (2022) created a delivery structure comprising a novel nanometal-organic framework (NMOF) covered with folic acid (FA) and chitosan (CS), referred to as MOF-CS-FA-MTX, for the delivery of MTX to colon carcinoma cells. The biological assays showed that the MOF-CS-FA-MTX co-delivery system has colon cancer inhibitory potential and is a viable system for cancer treatment.
Akbari et al. studied the efficient entrapment of Cisplatin (CDDP) and MTX into the bio-degradable poly(ε-caprolactone) (PCL)-poly(ethylene glycol) (PEG) copolymer, MTX@CDDP-loaded PCL-PEG, against lung cancer cell lines (Akbari et al., 2021b). Microscopic (i.e., scanning electron microscopy) characterization of the morphology and topology of the NPs, was carried out and it was established that the NPs had a spherical shape. The assay on lung cancer cells (A549) also showed that the formulation MTX@CDDP effectively killed lung cancer cells. Furthermore, the nanoparticle formulation MTX@CDDP-loaded PCL-PEG was promising in treating non-small-cell lung cancerous.
Khalili et al. (2021) synthesized a new co-delivery system consisting of MTX, conferone (CON), and a lipid/polymer hybrid NPs (i.e., MTX@CON-TLPN) for the concurrent delivery of multiple anticancer drugs. The synthesized material was characterized using spectroscopic methods (i.e., FTIR, 1H NMR, dynamic light scattering (DLS), transmission electron microscopy (TEM), and SEM). This nano-system was adequate to deliver medications to cancer cells according to cell cycle modulation, cell death evaluation, and cellular internalization assessments.
In another research by Akbari et al. (2021a), they demonstrated the synergistic anticancer impact of a co-delivery system consisting of Trapoxin A (TPX) and MTX that were loaded on poly(lactic-co-glycolic acid)-polyethylene glycol NPs (PLGA-PEG) for successfully treating breast cancer (i.e., MCF-7 cells).
Mahoutforoush et al. (2021) used nanostructured lipid carriers (NLCs) to prevent carcinoma cell proliferation with MTX as a as a targeting moiety and chemotherapy agent in conjugation with DTX and DOX (NLCs/DTX/DOX/CS-MTX). Spectroscopic methods were used to evaluate the physicochemical characteristics of the synthesized nano-complexes, and the compounds were tested against human breast carcinoma cells (i.e., MFC-7) and lung carcinoma cells (i.e., A549). Among other factors, the NLCs/DTX/DOX/CS-MTX co-delivery system demonstrated synergistic cytotoxicity against cancer cells, with good potential for successful treatment.
Zheng et al. (2021) produced a nanoparticle-based drug co-delivery system from Ganoderma lucidum polysaccharides, MTX, and 10-hydroxycamptothecin (HCPT) (i.e., GLP−APBA−MTX/HCPT). According to this study, GLP-APBA-MTX/HCPT NPs were more effective and had fewer in vivo side effects as compared to using the free drugs, indicating that they could be an effective tumor-suppressing treatment.
In order to reduce side effects, colon-targeted NPs based on biocompatible poly(methacrylic acid) (PMAA) nanohydrogels were investigated for possible use in combined therapy by simultaneous loading of MTX and chloroquine (CQ) (PMAA-MTX-CQ). Drug loading efficience of this NP system was 4.99% for MTX and 25.01% for CQ1; furthermore, pH/enzyme-triggered drug release behavior was observed. Higher CQ release rate (76%) was detected under simulated acidic microenvironment of tumor tissue whereas 39% of CQ was released under normal physiological conditions. MTX release was facilitated by the presence of proteinase K enzyme. The reported findings suggested that dual release exerted a significantly higher killing effect against SW480 colon cells as compared with single-drug therapy. Furthermore, nanohydrogels did not elicit hemolysis (Rashidzadeh et al., 2023).
5 Conclusion, outlook, and perspectives
MTX is a drug with significant therapeutic potential for various ailments, including cancer and immunological disorders. However, it is well proven that the residual tumour cells can resist a great deal of stress after the chemotherapy process, leading to a tumour with a more complex composition. Because of MTX’s poor pharmacokinetics, drug resistance, low bioavailability, and dose-dependent adverse effects, conventional drug delivery methods are constrained in their therapeutic potential. In this regard, the use of alternative delivery technologies has offered a significant chance to overcome these limitations of MTX. Drug delivery proposed platforms based on NPs, such as polymer and lipid-based NPs, as well as inorganic nanostructures, are extremely significant for improving the biological availability of MTX at the place of activity, as shown by in vitro studies and in vivo animal models. Consequently, the side effects of the drug on healthy tissues may be mitigated by synthesizing targeted NPs using a straightforward and effective procedure. However, a few main factors, such as nanomaterial-based MTX formulations, need to be considered to advance the use of NPs in delivery systems. In principle, the unique features of NPs allow for the controlled release of MTX. In this system, matrix type and drug loading technique are two important factors to consider for the delivery of anti-cancer medications. In another strategy, the co-delivery of small drug molecules like MTX and other pharmaceuticals may be regarded as an innovative technique to counteract some of the adverse effects of using MTX alone for an extended period. Co-delivery can reduce drug resistance, especially in long-term and overuse application. This strategy decreases undesirable effects without raising the initial dose, enabling clinical adoption for new objectives.
Due to uncontrolled drug release, lack of specificity in both normal and cancer cells, and drug resistance, novel drug delivery systems (DDSs) are needed not only to overcome adverse effects of MTX but also to increase therapeutic efficacies by improving bioavailability and targeting functions with the aid of delivery vehicles. Therefore, in the future, considerable attention should be paid to improve carriers including MOF- or COF-based stimuli-responsive DDSs such as pH-, temperature-, magnetic-, ion-, pressure-, H2S-, redox-, and photo-responsive nano-platform. In addition, other (nano)systems relying on photodynamic and photothermal effects as well as their combination with other stimuli-driven responses (e.g., stimuli-responsiveness to H2O2) and/or other chemotherapeutics deserve to be considered to overcome drug resistance and side effects in normal cells.
Nevertheless, it cannot be ignored that the clinical implementation of these multifaceted approaches in the routine clinical practice has to face several obstacles from the viewpoints of both functional validation (e.g., quantitative assessment of the synergy between drugs and other co-strategies) and regulatory approval. Therefore, many of these carriers and “intelligent” biomaterials still require additional investigation to ensure the highest level of safety and effectiveness for human use.
Author contributions
Conceptualization, AR; writing-original draft preparation, MM, A-LE, MS, RB, and RE; writing-review and editing, SG, FB, and AR; supervision, AR, FB, and SG. All authors contributed to the article and approved the submitted version.
Conflict of interest
The authors declare that the research was conducted in the absence of any commercial or financial relationships that could be construed as a potential conflict of interest.
Publisher’s note
All claims expressed in this article are solely those of the authors and do not necessarily represent those of their affiliated organizations, or those of the publisher, the editors and the reviewers. Any product that may be evaluated in this article, or claim that may be made by its manufacturer, is not guaranteed or endorsed by the publisher.
References
Abdelhamid, H. N., and Hussein, K. H. (2021). Graphene oxide as a carrier for drug delivery of methotrexate. Biointerface Res. Appl. Chem. 11, 14726–14735.
Abdelrady, H., Hathout, R. M., Osman, R., Saleem, I., and Mortada, N. D. (2019). Exploiting gelatin nanocarriers in the pulmonary delivery of methotrexate for lung cancer therapy. Eur. J. Pharm. Sci. 133, 115–126. doi:10.1016/j.ejps.2019.03.016
Abolmaali, S. S., Zarenejad, S., Mohebi, Y., Najafi, H., Javanmardi, S., Abedi, M., et al. (2022). Biotin receptor-targeting nanogels loaded with methotrexate for enhanced antitumor efficacy in triple-negative breast cancer in vitro and in vivo models. Int. J. Pharm. 624, 122049. doi:10.1016/j.ijpharm.2022.122049
Agrawal, Y. O., Mahajan, U. B., Mahajan, H. S., and Ojha, S. (2020). <p>Methotrexate-Loaded nanostructured lipid carrier gel alleviates imiquimod-induced psoriasis by moderating inflammation: Formulation, optimization, characterization, iIn-vVitro and iIn-vVivo studies</p>. Int. J. Nanomedicine 15, 4763–4778. doi:10.2147/ijn.s247007
Ahmadi, D., Zarei, M., Rahimi, M., Khazaie, M., Asemi, Z., Mir, S. M., et al. (2020). Preparation and in-vitro evaluation of pH-responsive cationic cyclodextrin coated magnetic nanoparticles for delivery of methotrexate to the Saos-2 bone cancer cells. J. Drug Deliv. Sci. Technol. 57, 101584. doi:10.1016/j.jddst.2020.101584
Ajalli, N., Pourmadadi, M., Yazdian, F., Rashedi, H., Navaei-Nigjeh, M., and Díez-Pascual, A. M. (2022). Chitosan/Gamma-Alumina/Fe3O4@ 5-FU nanostructures as promising nanocarriers: Physiochemical characterization and toxicity activity. Molecules 27, 5369. doi:10.3390/molecules27175369
Ajmal, M., Yunus, U., Matin, A., and Haq, N. U. (2015). Synthesis, characterization and in vitro evaluation of methotrexate conjugated fluorescent carbon nanoparticles as drug delivery system for human lung cancer targeting. J. Photochem. Photobiol. B Biol. 153, 111–120. doi:10.1016/j.jphotobiol.2015.09.006
Akbari, E., Mousazadeh, H., Hanifehpour, Y., Mostafavi, E., Gorabi, A. M., Nejati, K., et al. (2021). Co-loading of cisplatin and methotrexate in nanoparticle-based PCL-PEG system enhances lung cancer chemotherapy effects. J. Clust. Sci. 33, 1751–1762. doi:10.1007/s10876-021-02101-9
Akbari, E., Mousazadeh, H., Sabet, Z., Fattahi, T., Dehnad, A., Akbarzadeh, A., et al. (2021). Dual drug delivery of trapoxin A and methotrexate from biocompatible PLGA-PEG polymeric nanoparticles enhanced antitumor activity in breast cancer cell line. J. Drug Deliv. Sci. Technol. 61, 102294. doi:10.1016/j.jddst.2020.102294
Al-Nemrawi, N., Hameedat, F., Al-Husein, B., and Nimrawi, S. (2022). Photolytic controlled release formulation of methotrexate loaded in chitosan/TiO2 nanoparticles for breast cancer. Pharmaceuticals 15 (2), 149. doi:10.3390/ph15020149
Al-Rahim, A. M., Mahmood, R. I., Mohammed, M. M., and Omer, D. (2022). In vitro evaluation of antioxidant and cytotoxic activity of folate-methotrexate conjugated to bovine serum albumin nanoparticles against MCF-7, HepG2, and PC3 cell lines. Gene Rep. 29, 101666. doi:10.1016/j.genrep.2022.101666
Al-Zoubi, M. S., and Al-Zoubi, R. M. (2022). Nanomedicine tactics in cancer treatment: Challenge and hope. Crit. Rev. Oncology/Hematology 174, 103677. doi:10.1016/j.critrevonc.2022.103677
Almawash, S., Chaturvedi, S., Misra, C., Thotakura, N., Ibrahim, I. M., Sharma, G., et al. (2022). Vitamin E TPGS-PLGA-based nanoparticles for methotrexate delivery: Promising outcomes from preclinical studies. J. Drug Deliv. Sci. Technol. 72, 103276. doi:10.1016/j.jddst.2022.103276
Almawash, S., El Hamd, M. A., and Osman, S. K. (2022). Polymerized β-cyclodextrin-based injectable hydrogel for sustained release of 5-fluorouracil/methotrexate mixture in breast cancer management: In vitro and in vivo analytical validations. Pharmaceutics 14 (4), 817. doi:10.3390/pharmaceutics14040817
Alqosaibi, A. I. (2022). Nanocarriers for anticancer drugs: Challenges and perspectives. Saudi Journal of Biological Sciences. 103298.
Arrow, S. S., Felis, S. C., Hillenmayer, A., Strehle, L. D., Koenig, S. F., Vounotrypidis, E., et al. (2023). Inhibition of proliferative vitreoretinopathy by a newly developed methotrexate loaded drug carrier in vitro. Biomed. Pharmacother. 158, 114088. doi:10.1016/j.biopha.2022.114088
Arsalani, N., Nezhad-Mokhtari, P., and Jabbari, E. (2019). Microwave-assisted and one-step synthesis of PEG passivated fluorescent carbon dots from gelatin as an efficient nanocarrier for methotrexate delivery. Artif. cells, nanomedicine, Biotechnol. 47 (1), 540–547. doi:10.1080/21691401.2018.1562460
Asad, M. I., Khan, D., Rehman, A. u., Elaissari, A., and Ahmed, N. (2021). Development and in vitro/in vivo evaluation of pH-sensitive polymeric nanoparticles loaded hydrogel for the management of psoriasis. Nanomaterials 11 (12), 3433. doi:10.3390/nano11123433
Aslani, R., and Namazi, H. (2022). Simple fabrication of multifunctional hyperbranched copolymer based on l-lysine and citric acid for co-delivery of anticancer drugs to breast cancer cells. React. Funct. Polym. 170, 105101. doi:10.1016/j.reactfunctpolym.2021.105101
Bahuguna, S., Kumar, M., Sharma, G., Kumar, R., Singh, B., and Raza, K. (2018). Fullerenol-based intracellular delivery of methotrexate: A water-soluble nanoconjugate for enhanced cytotoxicity and improved pharmacokinetics. AAPS pharmscitech 19 (3), 1084–1092. doi:10.1208/s12249-017-0920-0
Baqeri, N., Shahsavari, S., Dahouee, I. A., and Shirmard, L. R. (2022). Design of slow-release methotrexate drug delivery system using PHBV magnetic nanoparticles and evaluation of its cytotoxicity. J. Drug Deliv. Sci. Technol. 77, 103854. doi:10.1016/j.jddst.2022.103854
Barbosa, A. I., Lima, S. A. C., and Reis, S. (2019). Development of methotrexate loaded fucoidan/chitosan nanoparticles with anti-inflammatory potential and enhanced skin permeation. Int. J. Biol. Macromol. 124, 1115–1122. doi:10.1016/j.ijbiomac.2018.12.014
Basu, T., Singh, S., and Pal, B. (2018). Fe3O4 @ PLGA-PEG nanocomposite for improved delivery of methotrexate in cancer treatment. ChemistrySelect 3 (29), 8522–8528. doi:10.1002/slct.201801769
Bethesda (MD): National Library of Medicine (US) (2022). N.C.f.B.I.-P.C.S.f.C., Methotrexate. https://pubchem.ncbi.nlm.nih.gov/compound/Methotrexate National Library of Medicine National Center for Biotechnology Information, [cited 2022 June 5]. https://pubchem.ncbi.nlm.nih.gov/compound/Methotrexate ( https://pubchem.ncbi.nlm.nih.gov/compound/Methotrexate). [cited 2022 June 5]. Available from: https://pubchem.ncbi.nlm.nih.gov/compound/Methotrexate.
Bhattacharya, S. (2021). Methotrexate-loaded polymeric lipid hybrid nanoparticles (PLHNPs): A reliable drug delivery system for the treatment of glioblastoma. J. Exp. Nanosci. 16 (1), 344–367. doi:10.1080/17458080.2021.1983172
Blanco, E., Shen, H., and Ferrari, M. (2015). Principles of nanoparticle design for overcoming biological barriers to drug delivery. Nat. Biotechnol. 33 (9), 941–951. doi:10.1038/nbt.3330
Borse, S., Murthy, Z., and Kailasa, S. K. (2023). Fabrication of water-soluble blue emitting molybdenum nanoclusters for sensitive detection of cancer drug methotrexate. J. Photochem. Photobiol. A Chem. 435, 114323. doi:10.1016/j.jphotochem.2022.114323
Bruckmann, F. D. S., Rossato Viana, A., Tonel, M. Z., Fagan, S. B., Garcia, W. J. d. S., Oliveira, A. H. d., et al. (2022). Influence of magnetite incorporation into chitosan on the adsorption of the methotrexate and in vitro cytotoxicity. Environ. Sci. Pollut. Res. Int. 29, 70413–70434. doi:10.1007/s11356-022-20786-x
Chakraborty, M., Dasgupta, S., Bose, P., Misra, A., Kumar Mandal, T., Mitra, M., et al. (2011). Layered double hydroxide: Inorganic organic conjugate nanocarrier for methotrexate. J. Phys. Chem. Solids 72 (6), 779–783. doi:10.1016/j.jpcs.2011.03.012
Chang, J., Yu, B., Saltzman, W. M., and Girardi, M. (2023). Nanoparticles as a therapeutic delivery system for skin cancer prevention and treatment. JID Innov. 3, 100197. doi:10.1016/j.xjidi.2023.100197
Chen, J., Yang, X., Huang, L., Lai, H., Gan, C., and Luo, X. (2018). Development of dual-drug-loaded stealth nanocarriers for targeted and synergistic anti-lung cancer efficacy. Drug Deliv. 25 (1), 1932–1942. doi:10.1080/10717544.2018.1477856
Cirillo, G., Vittorio, O., Kunhardt, D., Valli, E., Voli, F., Farfalla, A., et al. (2019). Combining carbon nanotubes and chitosan for the vectorization of methotrexate to lung cancer cells. Materials 12 (18), 2889. doi:10.3390/ma12182889
Colciago, R. R., Fischetti, I., Giandini, C., La Rocca, E., Rancati T., T., Rejas Mateo, A., et al. (2023). Overview of the synergistic use of radiotherapy and immunotherapy in cancer treatment: Current challenges and scopes of improvement. Expert Rev. Anticancer Ther. 23, 135–145. doi:10.1080/14737140.2023.2173175
Connie, Z. Y., and Ariya, P. A. (2015). Co-adsorption of gaseous benzene, toluene, ethylbenzene, m-xylene (BTEX) and SO2 on recyclable Fe3O4 nanoparticles at 0–101% relative humidities. J. Environ. Sci. 31, 164–174. doi:10.1016/j.jes.2014.10.019
Coutinho, A. J., Costa Lima, S. A., Afonso, C. M., and Reis, S. (2020). Mucoadhesive and pH responsive fucoidan-chitosan nanoparticles for the oral delivery of methotrexate. Int. J. Biol. Macromol. 158, 180–188. doi:10.1016/j.ijbiomac.2020.04.233
Curcio, M., Mauro, L., Naimo, G. D., Amantea, D., Cirillo, G., Tavano, L., et al. (2018). Facile synthesis of pH-responsive polymersomes based on lipidized PEG for intracellular co-delivery of curcumin and methotrexate. Colloids Surfaces B Biointerfaces 167, 568–576. doi:10.1016/j.colsurfb.2018.04.057
Dai, Y., and Zhang, X. (2018). Dual stimuli-responsive supramolecular polymeric nanoparticles based on poly (α-cyclodextrin) and acetal-modified β-cyclodextrin-azobenzene. J. Polym. Res. 25 (4), 1–6.
Danafar, H., Hossein Taromchi, A., Rakhshbahar, A., Sharafi, A., Hasani, V., Tafvizi, S., et al. (2022). Co-delivery of methotrexate and curcumin with mPEG-PCL polymeric nanoparticles and evaluation of toxicity effect on MCF7 breast cancer cell line. Inorg. Chem. Commun. 142, 109715. doi:10.1016/j.inoche.2022.109715
Davaran, S., Fazeli, H., Ghamkhari, A., Rahimi, F., Molavi, O., Anzabi, M., et al. (2018). Synthesis and characterization of novel P(HEMA-LA-MADQUAT) micelles for co-delivery of methotrexate and Chrysin in combination cancer chemotherapy. J. Biomater. Sci. Polym. Ed. 29 (11), 1265–1286. doi:10.1080/09205063.2018.1456026
Dou, J., Mi, Y., Daneshmand, S., and Heidari Majd, M. (2022). The effect of magnetic nanoparticles containing hyaluronic acid and methotrexate on the expression of genes involved in apoptosis and metastasis in A549 lung cancer cell lines. Arabian J. Chem. 15 (12), 104307. doi:10.1016/j.arabjc.2022.104307
Ekinci, M., Koksal-Karayildirim, C., and Ilem-Ozdemir, D. (2023). Radiolabeled methotrexate loaded chitosan nanoparticles as imaging probe for breast cancer: Biodistribution in tumor-bearing mice. J. Drug Deliv. Sci. Technol. 80, 104146. doi:10.1016/j.jddst.2022.104146
Ezra Manicum, A.-L., Sargazi, S., Razzaq, S., Kumar, G. V., Rahdar, A., Er, S., et al. (2022). Nano-immunotherapeutic strategies for targeted RNA delivery: Emphasizing the role of monocyte/macrophages as nanovehicles to treat glioblastoma multiforme. J. Drug Deliv. Sci. Technol. 71, 103288. doi:10.1016/j.jddst.2022.103288
Faria, R., Sousa, Â., Neves, A. R., Queiroz, J. A., and Costa, D. (2019). Methotrexate-plasmid DNA polyplexes for cancer therapy: Characterization, cancer cell targeting ability and tuned in vitro transfection. J. Mol. Liq. 292, 111391. doi:10.1016/j.molliq.2019.111391
Farzanfar, J., Farjadian, F., Roointan, A., Mohammadi-Samani, S., and Tayebi, L. (2021). Assessment of pH Responsive delivery of methotrexate based on PHEMA-st-PEG-DA nanohydrogels. Macromol. Res. 29 (1), 54–61. doi:10.1007/s13233-021-9007-6
Fatima, I., Rahdar, A., Sargazi, S., Barani, M., Hassanisaadi, M., and Thakur, V. K. (2021). Quantum dots: Synthesis, antibody conjugation, and HER2-receptor targeting for breast cancer therapy. J. Funct. Biomaterials 12 (4), 75. doi:10.3390/jfb12040075
Ferreira, M., Barreiros, L., Segundo, M. A., Torres, T., Selores, M., Costa Lima, S. A., et al. (2017). Topical co-delivery of methotrexate and etanercept using lipid nanoparticles: A targeted approach for psoriasis management. Colloids Surfaces B Biointerfaces 159, 23–29. doi:10.1016/j.colsurfb.2017.07.080
Gao, C., Liu, T., Dang, Y., Yu, Z., Wang, W., Guo, J., et al. (2014). pH/redox responsive core cross-linked nanoparticles from thiolated carboxymethyl chitosan for in vitro release study of methotrexate. Carbohydr. Polym. 111, 964–970. doi:10.1016/j.carbpol.2014.05.012
Garg, N. K., Singh, B., Jain, A., Nirbhavane, P., Sharma, R., Tyagi, R. K., et al. (2016). Fucose decorated solid-lipid nanocarriers mediate efficient delivery of methotrexate in breast cancer therapeutics. Colloids Surfaces B Biointerfaces 146, 114–126. doi:10.1016/j.colsurfb.2016.05.051
Garg, N. K., Tyagi, R. K., Sharma, G., Jain, A., Singh, B., Jain, S., et al. (2017). Functionalized lipid-polymer hybrid nanoparticles mediated codelivery of methotrexate and aceclofenac: A synergistic effect in breast cancer with improved pharmacokinetics attributes. Mol. Pharm. 14 (6), 1883–1897. doi:10.1021/acs.molpharmaceut.6b01148
Gharebaghi, F., Dalali, N., Ahmadi, E., and Danafar, H. (2017). Preparation of wormlike polymeric nanoparticles coated with silica for delivery of methotrexate and evaluation of anticancer activity against MCF7 cells. J. biomaterials Appl. 31 (9), 1305–1316. doi:10.1177/0885328217698063
Ghazimoradi, M., Tarlani, A., Alemi, A., Hamishehkar, H., and Ghorbani, M. (2023). pH-responsive, magnetic-luminescent core/shell carriers for co-delivery of anticancer drugs (MTX & DOX) for breast cancer treatment. J. Alloys Compd. 936, 168257. doi:10.1016/j.jallcom.2022.168257
Ghumman, S. A., Mahmood, A., Noreen, S., Aslam, A., Ijaz, B., Amanat, A., et al. (2023). Chitosan-linseed mucilage polyelectrolyte complex nanoparticles of methotrexate: In vitro cytotoxic efficacy and toxicological studies. Arabian J. Chem. 16 (2), 104463. doi:10.1016/j.arabjc.2022.104463
Giri, B. R., Yang, H. S., Song, I. S., Choi, H. G., Cho, J. H., and Kim, D. W. (2022). Alternative methotrexate oral formulation: Enhanced aqueous solubility, bioavailability, photostability, and permeability. Pharmaceutics 14 (10), 2073. doi:10.3390/pharmaceutics14102073
González-Fernández, Y., Zalacain, M., Imbuluzqueta, E., Sierrasesumaga, L., Patiño-García, A., and Blanco-Prieto, M. J. (2015). Lipid nanoparticles enhance the efficacy of chemotherapy in primary and metastatic human osteosarcoma cells. J. Drug Deliv. Sci. Technol. 30, 435–442. doi:10.1016/j.jddst.2015.08.004
Gorjikhah, F., Azizi Jalalian, F., Salehi, R., Panahi, Y., Hasanzadeh, A., Alizadeh, E., et al. (2017). Preparation and characterization of PLGA-β-CD polymeric nanoparticles containing methotrexate and evaluation of their effects on T47D cell line. Artif. cells, nanomedicine, Biotechnol. 45 (3), 432–440. doi:10.3109/21691401.2016.1160915
Guo, Y., Chu, M., Zhao, S., Liu, H., Otieno, B. O., et al. (2014). Chitosan-g-TPGS nanoparticles for anticancer drug delivery and overcoming multidrug resistance. Mol. Pharm. 11 (1), 59–70. doi:10.1021/mp400514t
Guðmundsdóttir, J. S., Fredheim, E. G., Koumans, C. I., Hegstad, J., Tang, P. C., Andersson, D. I., et al. (2021). The chemotherapeutic drug methotrexate selects for antibiotic resistance. EBioMedicine 74, 103742. doi:10.1016/j.ebiom.2021.103742
Hadi, H., Safari, R., and Shamlouei, H. R. (2023). Synthesis and experimental/theoretical evaluation of β-CD/MTX nanostructure for use in targeted drug delivery systems. Chem. Pap. 77 (1), 63–74. doi:10.1007/s11696-022-02459-8
Hanna, D. H., Hamed, A. A., and Saad, G. R. (2023). Synthesis and characterization of poly (3-hydroxybutyrate)/chitosan-graft poly (acrylic acid) conjugate hyaluronate for targeted delivery of methotrexate drug to colon cancer cells. Int. J. Biol. Macromol. 240, 124396. doi:10.1016/j.ijbiomac.2023.124396
Hao, F., Lee, R., Yang, C., Zhong, L., Sun, Y., Dong, S., et al. (2019). Targeted Co-delivery of siRNA and methotrexate for tumor therapy via mixed micelles. Pharmaceutics 11 (2), 92. doi:10.3390/pharmaceutics11020092
Hassanisaadi, M., Bonjar, G. H. S., Rahdar, A., Pandey, S., Hosseinipour, A., and Abdolshahi, R. (2021). Environmentally safe biosynthesis of gold nanoparticles using plant water extracts. Nanomaterials 11 (8), 2033. doi:10.3390/nano11082033
He, C., Cheng, Y., Li, D., Gong, Y., Liu, J., et al. (2015). PLK1shRNA and doxorubicin co-loaded thermosensitive PLGA-PEG-PLGA hydrogels for localized and combined treatment of human osteosarcoma. J. Control Release 213, e18. doi:10.1016/j.jconrel.2015.05.026
Hou, M., Gao, Y. E., Shi, X., Bai, S., Ma, X., Li, B., et al. (2018). Methotrexate-based amphiphilic prodrug nanoaggregates for co-administration of multiple therapeutics and synergistic cancer therapy. Acta Biomater. 77, 228–239. doi:10.1016/j.actbio.2018.07.014
Jang, J.-H., Jeong, S.-H., and Lee, Y.-B. (2019). Preparation and in vitro/in vivo characterization of polymeric nanoparticles containing methotrexate to improve lymphatic delivery. Int. J. Mol. Sci. 20 (13), 3312. doi:10.3390/ijms20133312
Javanbakht, S., Shadi, M., Mohammadian, R., Shaabani, A., Ghorbani, M., Rabiee, G., et al. (2020). Preparation of Fe3O4@SiO2@Tannic acid double core-shell magnetic nanoparticles via the Ugi multicomponent reaction strategy as a pH-responsive co-delivery of doxorubicin and methotrexate. Mater. Chem. Phys. 247, 122857. doi:10.1016/j.matchemphys.2020.122857
Jin, B. Z., Dong, X. Q., Xu, X., and Zhang, F. H. (2018). Development and in vitro evaluation of mucoadhesive patches of methotrexate for targeted delivery in oral cancer. Oncol. Lett. 15 (2), 2541–2549. doi:10.3892/ol.2017.7613
Joshi, M., Kumar, P., Kumar, R., Sharma, G., Singh, B., Katare, O. P., et al. (2017). Aminated carbon-based “cargo vehicles” for improved delivery of methotrexate to breast cancer cells. Mater. Sci. Eng. C 75, 1376–1388. doi:10.1016/j.msec.2017.03.057
Kakkar, D., Dumoga, S., Kumar, R., Chuttani, K., and Mishra, A. K. (2015). PEGylated solid lipid nanoparticles: Design, methotrexate loading and biological evaluation in animal models. MedChemComm 6 (8), 1452–1463. doi:10.1039/c5md00104h
Khalili, L., Dehghan, G., Hosseinpour Feizi, M. A., Sheibani, N., and Hamishekar, H. (2021). Development of an albumin decorated lipid-polymer hybrid nanoparticle for simultaneous delivery of methotrexate and conferone to cancer cells. Int. J. Pharm. 599, 120421. doi:10.1016/j.ijpharm.2021.120421
Khan, I., Khan, M., Umar, M. N., and Oh, D. (2015). Nanobiotechnology and its applications in drug delivery system: A review. IET Nanobiotechnol 9 (6), 396–400. doi:10.1049/iet-nbt.2014.0062
Khatibi, Z., Kazemi, N. M., and Khaleghi, S. (2022). Targeted and biocompatible NMOF as efficient nanocomposite for delivery of methotrexate to colon cancer cells. J. Drug Deliv. Sci. Technol. 73, 103441. doi:10.1016/j.jddst.2022.103441
Kim, J.-H., Umemura, M., Eguchi, H., and Ishikawa, Y. (2022). Methotrexate-transferrin-functionalized Fe (Salen)-Polypyrrole nanocomposites for targeted photo-/magneto-thermal cancer treatments. J. Compos. Sci. 6 (5), 136. doi:10.3390/jcs6050136
Komarova, N. L., and Wodarz, D. (2005). Drug resistance in cancer: Principles of emergence and prevention. Proc. Natl. Acad. Sci. U. S. A. 102 (27), 9714–9719. doi:10.1073/pnas.0501870102
Kubo, T., Tachibana, K., Naito, T., Mukai, S., Akiyoshi, K., Balachandran, J., et al. (2018). Magnetic field stimuli-sensitive drug release using a magnetic thermal seed coated with thermal-responsive molecularly imprinted polymer. ACS Biomaterials Sci. Eng. 5 (2), 759–767. doi:10.1021/acsbiomaterials.8b01401
Kumar, R., Srivastava, V. R., Mahapatra, S., Dkhar, D. S., Kumari, R., Prerna, K., et al. (2023). Drug encapsulated lipid-polymeric nanohybrid as a chemo-therapeutic platform of cancer. Nanotheranostics 7 (2), 167–175. doi:10.7150/ntno.81173
Kumar, R., Varshney, N., Mahapatra, S., Mahto, S. K., Dubey, V. K., and Chandra, P. (2022). Design and development of lactoferrin conjugated lipid-polymer nano-bio-hybrid for cancer theranostics. Mater. Today Commun. 31, 103548. doi:10.1016/j.mtcomm.2022.103548
Li, M., Neoh, K. G., Wang, R., Zong, B. Y., Tan, J. Y., and Kang, E. T. (2013). Methotrexate-conjugated and hyperbranched polyglycerol-grafted Fe3O4 magnetic nanoparticles for targeted anticancer effects. Eur. J. Pharm. Sci. 48 (1-2), 111–120. doi:10.1016/j.ejps.2012.10.008
Li, Q., Ohulchanskyy, T. Y., Liu, R., Koynov, K., Wu, D., Best, A., et al. (2010). Photoluminescent carbon dots as biocompatible nanoprobes for targeting cancer cells in vitro. J. Phys. Chem. C 114 (28), 12062–12068. doi:10.1021/jp911539r
Li, Y., Chen, S., Chang, X., He, F., and Zhuo, R. (2019). Efficient Co-delivery of doxorubicin and methotrexate by pH-sensitive dual-functional nanomicelles for enhanced synergistic antitumor efficacy. ACS Appl. Bio Mater. 2 (5), 2271–2279. doi:10.1021/acsabm.9b00230
Li, Y., Gao, G. H., and Lee, D. S. (2013). Stimulus-sensitive polymeric nanoparticles and their applications as drug and gene carriers. Adv. Healthc. Mater. 2 (3), 388–417. doi:10.1002/adhm.201200313
Li, Y., Lin, J., Wu, H., Chang, Y., Yuan, C., Liu, C., et al. (2015). Orthogonally functionalized nanoscale micelles for active targeted codelivery of methotrexate and mitomycin C with synergistic anticancer effect. Mol. Pharm. 12 (3), 769–782. doi:10.1021/mp5006068
Liang, Z., Gao, J., Yin, Z. Z., Li, J., Cai, W., and Kong, Y. (2023). A sequential delivery system based on MoS2 nanoflower doped chitosan/oxidized dextran hydrogels for colon cancer treatment. Int. J. Biol. Macromol. 233, 123616. doi:10.1016/j.ijbiomac.2023.123616
Lima, S. A. C., and Reis, S. (2015). Temperature-responsive polymeric nanospheres containing methotrexate and gold nanoparticles: A multi-drug system for theranostic in rheumatoid arthritis. Colloids Surfaces B Biointerfaces 133, 378–387. doi:10.1016/j.colsurfb.2015.04.048
Lin, J., Li, Y., Li, Y., Cui, F., Yu, F., Wu, H., et al. (2015). Self-targeted, bacillus-shaped, and controlled-release methotrexate prodrug polymeric nanoparticles for intratumoral administration with improved therapeutic efficacy in tumor-bearing mice. J. Mater. Chem. B 3 (39), 7707–7717. doi:10.1039/c5tb00724k
Lin, Y.-K., Huang, Z. R., Zhuo, R. Z., and Fang, J. Y. (2010). Combination of calcipotriol and methotrexate in nanostructured lipid carriers for topical delivery. Int. J. nanomedicine 5, 117–128. doi:10.2147/ijn.s9155
Lodhi, M. S., Khalid, F., Khan, M. T., Samra, Z. Q., Muhammad, S., Zhang, Y. J., et al. (2022). A novel method of magnetic nanoparticles functionalized with anti-folate receptor antibody and methotrexate for antibody mediated targeted drug delivery. Molecules 27 (1), 261. doi:10.3390/molecules27010261
Macedo, L. B., Nogueira-Librelotto, D. R., de Vargas, J., Scheeren, L. E., Vinardell, M. P., and Rolim, C. M. B. (2019). Poly (ɛ-Caprolactone) nanoparticles with pH-responsive behavior improved the in vitro antitumor activity of methotrexate. AAPS PharmSciTech 20 (5), 165–212. doi:10.1208/s12249-019-1372-5
Madani, F., Esnaashari, S. S., Bergonzi, M. C., Webster, T. J., Younes, H. M., Khosravani, M., et al. (2020). Paclitaxel/methotrexate co-loaded PLGA nanoparticles in glioblastoma treatment: Formulation development and in vitro antitumor activity evaluation. Life Sci. 256, 117943. doi:10.1016/j.lfs.2020.117943
Mahmoodzadeh, F., Jannat, B., and Ghorbani, M. (2019). Chitosan-based nanomicelle as a novel platform for targeted delivery of methotrexate. Int. J. Biol. Macromol. 126, 517–524. doi:10.1016/j.ijbiomac.2018.12.223
Mahoutforoush, A., Solouk, A., Hamishehkar, H., Haghbin Nazarpak, M., and Abbaspour-Ravasjani, S. (2021). Novel decorated nanostructured lipid carrier for simultaneous active targeting of three anti-cancer agents. Life Sci. 279, 119576. doi:10.1016/j.lfs.2021.119576
Mansour, A., Mahmoud, M. Y., Bakr, A. F., Ghoniem, M. G., Adam, F. A., and El-Sherbiny, I. M. (2022). Dual-enhanced pluronic nanoformulated methotrexate-based treatment approach for breast cancer: Development and evaluation of in vitro and in vivo efficiency. Pharmaceutics 14 (12), 2668. doi:10.3390/pharmaceutics14122668
Massadeh, S., Alaamery, M., Al-Qatanani, S., Alarifi, S., Bawazeer, S., and Alyafee, Y. (2016). Synthesis of protein-coated biocompatible methotrexate-loaded PLA-PEG-PLA nanoparticles for breast cancer treatment. Nano Rev. Exp. 7 (1), 31996. doi:10.3402/nano.v7.31996
Massaro, M., Poma, P., Cavallaro, G., García-Villén, F., Lazzara, G., Notarbartolo, M., et al. (2022). Prodrug based on halloysite delivery systems to improve the antitumor ability of methotrexate in leukemia cell lines. Colloids Surfaces B Biointerfaces 213, 112385. doi:10.1016/j.colsurfb.2022.112385
Meng, F., Yun, Z., Yan, G., Wang, G., and Lin, C. (2021). Engineering of anticancer drugs entrapped polymeric nanoparticles for the treatment of colorectal cancer therapy. Process Biochem. 111, 36–42. doi:10.1016/j.procbio.2021.09.013
Meng, Q. Y., Cong, H., Hu, H., and Xu, F. J. (2020). Rational design and latest advances of codelivery systems for cancer therapy. Mater. Today Bio 7, 100056. doi:10.1016/j.mtbio.2020.100056
Mizrahy, S., Goldsmith, M., Leviatan-Ben-Arye, S., Kisin-Finfer, E., Redy, O., Srinivasan, S., et al. (2014). Tumor targeting profiling of hyaluronan-coated lipid based-nanoparticles. Nanoscale 6 (7), 3742–3752. doi:10.1039/c3nr06102g
Mokhtar, S., Khattab, S. N., Elkhodairy, K. A., Teleb, M., Bekhit, A. A., Elzoghby, A. O., et al. (2022). Methotrexate-lactoferrin targeted exemestane cubosomes for synergistic breast cancer therapy. Front. Chem. 10, 847573. doi:10.3389/fchem.2022.847573
Moradi, S., Najjar, R., Hamishehkar, H., and Lotfi, A. (2022). Triple-responsive drug nanocarrier: Magnetic core-shell nanoparticles of Fe3O4@ poly (N-isopropylacrylamide)-grafted-chitosan, synthesis and in vitro cytotoxicity evaluation against human lung and breast cancer cells. J. Drug Deliv. Sci. Technol. 72, 103426. doi:10.1016/j.jddst.2022.103426
Moshikur, R. M., Ali, M. K., Wakabayashi, R., Moniruzzaman, M., and Goto, M. (2021). Methotrexate-based ionic liquid as a potent anticancer drug for oral delivery: In vivo pharmacokinetics, biodistribution, and antitumor efficacy. Int. J. Pharm. 608, 121129. doi:10.1016/j.ijpharm.2021.121129
Mousazadeh, N., Gharbavi, M., Rashidzadeh, H., Nosrati, H., Danafar, H., and Johari, B. (2022). Anticancer evaluation of methotrexate and curcumin-coencapsulated niosomes against colorectal cancer cell lines. Nanomedicine 17 (4), 201–217. doi:10.2217/nnm-2021-0334
Muangsopa, P., Chansaenpak, K., Kampaengsri, S., Saetiew, J., Noisa, P., Meemon, P., et al. (2023). Hybrid cyanine/methotrexate nanoparticles for synergistic PDT/chemotherapy of breast cancer. ACS Appl. Bio Mater. 6, 603–614. doi:10.1021/acsabm.2c00893
Muhammad, Z., Raza, A., Ghafoor, S., Naeem, A., Naz, S. S., Riaz, S., et al. (2016). PEG capped methotrexate silver nanoparticles for efficient anticancer activity and biocompatibility. Eur. J. Pharm. Sci. 91, 251–255. doi:10.1016/j.ejps.2016.04.029
Mujokoro, B., Madani, F., Esnaashari, S. S., Khosravani, M., and Adabi, M. (2020). Combination and Co-delivery of methotrexate and curcumin: Preparation and in vitro cytotoxic investigation on glioma cells. J. Pharm. Innovation 15 (4), 617–626. doi:10.1007/s12247-019-09406-3
Mukesh, U., Kulkarni, V., Tushar, R., and Murthy, R. S. R. (2009). Methotrexate loaded self stabilized calcium phosphate nanoparticles: A novel inorganic carrier for intracellular drug delivery. J. Biomed. Nanotechnol. 5 (1), 99–105. doi:10.1166/jbn.2009.026
Muntoni, E., Martina, K., Marini, E., Giorgis, M., Lazzarato, L., Salaroglio, I., et al. (2019). Methotrexate-loaded solid lipid nanoparticles: Protein functionalization to improve brain biodistribution. Pharmaceutics 11 (2), 65. doi:10.3390/pharmaceutics11020065
Murawala, P., Tirmale, A., Shiras, A., and Prasad, B. (2014). In situ synthesized BSA capped gold nanoparticles: Effective carrier of anticancer drug methotrexate to MCF-7 breast cancer cells. Mater. Sci. Eng. C 34, 158–167. doi:10.1016/j.msec.2013.09.004
Muthukumar, T., Prabhavathi, S., Chamundeeswari, M., and Sastry, T. P. (2014). Bio-modified carbon nanoparticles loaded with methotrexate possible carrier for anticancer drug delivery. Mater. Sci. Eng. C 36, 14–19. doi:10.1016/j.msec.2013.11.046
Naderi, Z., Azizian, J., Moniri, E., and Farhadyar, N. (2020). Synthesis and characterization of carboxymethyl cellulose/β-cyclodextrin/chitosan hydrogels and investigating the effect of magnetic nanoparticles (Fe3O4) on a novel carrier for a controlled release of methotrexate as drug delivery. J. Inorg. Organomet. Polym. Mater. 30 (4), 1339–1351. doi:10.1007/s10904-019-01301-1
Narayanan, P., Anitha, A. K., Ajayakumar, N., and Kumar, K. S. (2022). Poly-lysine dendritic nanocarrier to target epidermal growth factor receptor overexpressed breast cancer for methotrexate delivery. Materials 15 (3), 800. doi:10.3390/ma15030800
Naveen, N. R., Kurakula, M., and Gowthami, B. (2020). Process optimization by response surface methodology for preparation and evaluation of methotrexate loaded chitosan nanoparticles. Mater. Today Proc. 33, 2716–2724. doi:10.1016/j.matpr.2020.01.491
Nezhad-Mokhtari, P., Ghorbani, M., and Mahmoodzadeh, F. (2019). Smart co-delivery of 6-mercaptopurine and methotrexate using disulphide-based PEGylated-nanogels for effective treatment of breast cancer. New J. Chem. 43 (30), 12159–12167. doi:10.1039/c9nj02470k
Nosrati, H., Abhari, F., Charmi, J., Davaran, S., and Danafar, H. (2019). Multifunctional nanoparticles from albumin for stimuli-responsive efficient dual drug delivery. Bioorg. Chem. 88, 102959. doi:10.1016/j.bioorg.2019.102959
Nozohouri, S., Salehi, R., Ghanbarzadeh, S., Adibkia, K., and Hamishehkar, H. (2019). A multilayer hollow nanocarrier for pulmonary co-drug delivery of methotrexate and doxorubicin in the form of dry powder inhalation formulation. Mater. Sci. Eng. C 99, 752–761. doi:10.1016/j.msec.2019.02.009
Ong, Y. S., Bañobre-López, M., Costa Lima, S. A., and Reis, S. (2020). A multifunctional nanomedicine platform for co-delivery of methotrexate and mild hyperthermia towards breast cancer therapy. Mater. Sci. Eng. C 116, 111255. doi:10.1016/j.msec.2020.111255
Pathania, K., Sah, S. P., Salunke, D. B., Jain, M., Yadav, A. K., Yadav, V. G., et al. (2023). Green synthesis of lignin-based nanoparticles as a bio-carrier for targeted delivery in cancer therapy. Int. J. Biol. Macromol. 229, 684–695. doi:10.1016/j.ijbiomac.2022.12.323
Pointer, K. B., Pitroda, S. P., and Weichselbaum, R. R. (2022). Radiotherapy and immunotherapy: Open questions and future strategies. Trends Cancer 8 (1), 9–20. doi:10.1016/j.trecan.2021.10.003
Pooresmaeil, M., and Namazi, H. (2022). D-mannose functionalized MgAl-LDH/Fe-MOF nanocomposite as a new intelligent nanoplatform for MTX and DOX co-drug delivery. Int. J. Pharm. 625, 122112. doi:10.1016/j.ijpharm.2022.122112
Pourmadadi, Mehrab, Eshaghi, M. M., Rahmani, E., Ajalli, N., Bakhshi, S., Mirkhaef, H., et al. (2022). Cisplatin-loaded nanoformulations for cancer therapy: A comprehensive review. J. Drug Deliv. Sci. Technol. 77, 103928. doi:10.1016/j.jddst.2022.103928
Qi, S.-S., Sun, J. H., Yu, H. H., and Yu, S. Q. (2017). Co-delivery nanoparticles of anti-cancer drugs for improving chemotherapy efficacy. Drug Deliv. 24 (1), 1909–1926. doi:10.1080/10717544.2017.1410256
Rahimi, M., Safa, K. D., and Salehi, R. (2017). Co-delivery of doxorubicin and methotrexate by dendritic chitosan-g-mPEG as a magnetic nanocarrier for multi-drug delivery in combination chemotherapy. Polym. Chem. 8 (47), 7333–7350. doi:10.1039/c7py01701d
Ram Prasad, S., Jayakrishnan, A., and Sampath Kumar, T. S. (2019). Hydroxyapatite-poly(vinyl alcohol) core-shell nanoparticles for dual delivery of methotrexate and gemcitabine for bone cancer treatment. J. Drug Deliv. Sci. Technol. 51, 629–638. doi:10.1016/j.jddst.2019.03.041
Rashidzadeh, H., Ramazani, A., Tabatabaei Rezaei, S. J., Danafar, H., Rahmani, S., Veisi, H., et al. (2023). Targeted co-delivery of methotrexate and chloroquine via a pH/enzyme-responsive biocompatible polymeric nanohydrogel for colorectal cancer treatment. J. Biomaterials Sci., 1–19. Polymer Edition (just-accepted). doi:10.1080/09205063.2023.2187986
Rathee, J., Kanwar, R., Kumari, L., Pawar, S. V., Sharma, S., Ali, M. E., et al. (2022). Development of nanostructured lipid carriers as a promising tool for methotrexate delivery: Physicochemical and in vitro evaluation. J. Biomol. Struct. Dyn. 41, 2747–2758. doi:10.1080/07391102.2022.2037465
Ruckmani, K., Sivakumar, M., and Ganeshkumar, P. (2006). Methotrexate loaded solid lipid nanoparticles (SLN) for effective treatment of carcinoma. J. Nanosci. Nanotechnol. 6 (9-10), 2991–2995. doi:10.1166/jnn.2006.457
Rui, Q., Yin, Z., Cai, W., Li, J., Wu, D., and Kong, Y. (2022). Hyaluronic acid encapsulated aminated mesoporous silica nanoparticles for pH-responsive delivery of methotrexate and release kinetics. Bull. Korean Chem. Soc. 43 (5), 650–657. doi:10.1002/bkcs.12499
Samani, R. K., Maghsoudinia, F., Mehradnia, F., Hejazi, S. H., Saeb, M., Sobhani, T., et al. (2023). Ultrasound-guided chemoradiotherapy of breast cancer using smart methotrexate-loaded perfluorohexane nanodroplets. Nanomedicine Nanotechnol. Biol. Med. 48, 102643. doi:10.1016/j.nano.2022.102643
Saraei, M., Sarvari, R., Massoumi, B., and Agbolaghi, S. (2019). Co-delivery of methotrexate and doxorubicin via nanocarriers of star-like poly(DMAEMA-block-HEMA-block-AAc) terpolymers. Polym. Int. 68 (10), 1795–1803. doi:10.1002/pi.5890
Seo, D.-H., Jeong, Y. I., Kim, D. G., Jang, M. J., Jang, M. K., and Nah, J. W. (2009). Methotrexate-incorporated polymeric nanoparticles of methoxy poly (ethylene glycol)-grafted chitosan. Colloids Surfaces B Biointerfaces 69 (2), 157–163. doi:10.1016/j.colsurfb.2008.10.020
Shadmani, N., Makvandi, P., Parsa, M., Azadi, A., Nedaei, K., Mozafari, N., et al. (2023). Enhancing methotrexate delivery in the brain by mesoporous silica nanoparticles functionalized with cell-penetrating peptide using in vivo and ex vivo monitoring. Mol. Pharm. 20, 1531–1548. doi:10.1021/acs.molpharmaceut.2c00755
Shahrousvand, M., Hajikhani, M., Nazari, L., Aghelinejad, A., Irani, M., et al. (2022). Preparation of colloidal nanoparticles PVA-PHEMA from hydrolysis of copolymers of PVAc-PHEMA as anticancer drug carriers. Nanotechnology 33 (27), 275603. doi:10.1088/1361-6528/ac6089
Shakeran, Z., Keyhanfar, M., Varshosaz, J., and Sutherland, D. S. (2021). Biodegradable nanocarriers based on chitosan-modified mesoporous silica nanoparticles for delivery of methotrexate for application in breast cancer treatment. Mater. Sci. Eng. C 118, 111526. doi:10.1016/j.msec.2020.111526
Shakeran, Z., Varshosaz, J., Keyhanfar, M., Mohammad-Beigi, H., Rahimi, K., and Sutherland, D. S. (2022). Co-delivery of STAT3 siRNA and methotrexate in breast cancer cells. Artif. Cells, Nanomedicine, Biotechnol. 50 (1), 29–39. doi:10.1080/21691401.2022.2030746
Sheikh, A., Shadab, M. D., and Kesharwani, P. (2022). Aptamer grafted nanoparticle as targeted therapeutic tool for the treatment of breast cancer. Biomed. Pharmacother. 146, 112530. doi:10.1016/j.biopha.2021.112530
Shilpi, S., Upadhaye, S., Shivvedi, R., Gurnany, E., Chimaniya, P., Singh, A., et al. (2019). Chondroitin sulphate mediated targeted delivery of methotrexate and aceclofenac to the joints for effective management of rheumatoid arthritis. Asian J. Pharm. Pharmacol. 5 (3), 495–502. doi:10.31024/ajpp.2019.5.3.10
Solignac, J., et al. (2021). Successful treatment with adapted high dose methotrexate in a hemodialysis patient with primary central nervous system lymphoma: 100 mg/m2 seems sufficient. Nefrologia.
Sung, H., Ferlay, J., Siegel, R. L., Laversanne, M., Soerjomataram, I., Jemal, A., et al. (2021). Global cancer statistics 2020: GLOBOCAN estimates of incidence and mortality worldwide for 36 cancers in 185 countries. CA A Cancer J. Clin. 71 (3), 209–249. doi:10.3322/caac.21660
Surve, C., Banerjee, A., Chakraborty, R., Kumar, D., Butti, R., et al. (2022). Antiproliferative and apoptotic potential of methotrexate lipid nanoparticles in a murine breast cancer model. Nanomedicine 17 (11), 753–764. doi:10.2217/nnm-2021-0446
Taran, Z., Yektaniroumand Digehsaraei, S., Salouti, M., Amini, B., Mahmazi, S., and Kalantari, M. (2023). Methotrexate loaded in alginate beads for controlled drug release against breast cancer. Gene 851, 146941. doi:10.1016/j.gene.2022.146941
Teixeira, M., Carbone, C., and Souto, E. (2017). Beyond liposomes: Recent advances on lipid based nanostructures for poorly soluble/poorly permeable drug delivery. Prog. lipid Res. 68, 1–11. doi:10.1016/j.plipres.2017.07.001
Torchilin, V. P. (2001). Structure and design of polymeric surfactant-based drug delivery systems. J. Control Release 73 (2-3), 137–172. doi:10.1016/s0168-3659(01)00299-1
Tripathi, P., Kumar, A., Jain, P. K., and Patel, J. R. (2018). Carbomer gel bearing methotrexate loaded lipid nanocontainers shows improved topical delivery intended for effective management of psoriasis. Int. J. Biol. Macromol. 120, 1322–1334. doi:10.1016/j.ijbiomac.2018.08.136
Vakilinezhad, M. A., Amini, A., Dara, T., and Alipour, S. (2019). Methotrexate and curcumin co-encapsulated PLGA nanoparticles as a potential breast cancer therapeutic system: In vitro and in vivo evaluation. Colloids Surf. B Biointerfaces 184, 110515. doi:10.1016/j.colsurfb.2019.110515
Vasil’kov, A., Voronova, A., Batsalova, T., Moten, D., Naumkin, A., Shtykova, E., et al. (2023). Evolution of gold and iron oxide nanoparticles in conjugates with methotrexate: Synthesis and anticancer effects. Materials 16 (8), 3238. doi:10.3390/ma16083238
Wang, Y., Yang, X., Yang, J., Chen, R., Wu, J., et al. (2011). Self-assembled nanoparticles of methotrexate conjugated O-carboxymethyl chitosan: Preparation, characterization and drug release behavior in vitro. Carbohydr. Polym. 86 (4), 1665–1670. doi:10.1016/j.carbpol.2011.06.080
Wu, J., Wang, X., Zhu, B., He, Q., Zhang, Y., and Jiang, W. (2022). Synthesis and characterization of magnetic polymeric nanocomposites for pH-sensitive controlled release of methotrexate. J. Biomaterials Sci. Polym. Ed. 33 (16), 2067–2080. doi:10.1080/09205063.2022.2093053
Wu, S., Yang, X., and Yang, X. (2022). Methotrexate and 10-hydroxycamptothecine loaded pullulan nanoparticles with the targeting property for efficient cancer therapy. Mater. Technol. 37, 2777–2784. doi:10.1080/10667857.2022.2075079
Xavier, C. P., Belisario, D. C., Rebelo, R., Assaraf, Y. G., Giovannetti, E., Kopecka, J., et al. (2022). The role of extracellular vesicles in the transfer of drug resistance competences to cancer cells. Drug Resist. Updat. 62, 100833. doi:10.1016/j.drup.2022.100833
Xie, B., Jin, L., Luo, Z., Yu, J., Shi, S., Zhang, Z., et al. (2015). An injectable thermosensitive polymeric hydrogel for sustained release of Avastin® to treat posterior segment disease. Int. J. Pharm. 490 (1-2), 375–383. doi:10.1016/j.ijpharm.2015.05.071
Xu, F., Zhong, H., Chang, Y., Jin, H., Zhang, M., et al. (2018). Targeting death receptors for drug-resistant cancer therapy: Codelivery of pTRAIL and monensin using dual-targeting and stimuli-responsive self-assembling nanocomposites. Biomaterials 158, 56–73. doi:10.1016/j.biomaterials.2017.12.018
Xu, W., Zheng, S., Sun, H., Li, Z., Xi, R., Luo, D., et al. (2022). Green-step fabrication of gliadin/sodium caseinate nanogels for methotrexate release, cytotoxicity and cell phagocytosis. J. Drug Deliv. Sci. Technol. 67, 103028. doi:10.1016/j.jddst.2021.103028
Xu, Z. P., Zeng, Q. H., Lu, G. Q., and Yu, A. B. (2006). Inorganic nanoparticles as carriers for efficient cellular delivery. Chem. Eng. Sci. 61 (3), 1027–1040. doi:10.1016/j.ces.2005.06.019
Yang, M., Yu, L., Guo, R., Dong, A., Lin, C., and Zhang, J. (2018). A modular coassembly approach to all-in-one multifunctional nanoplatform for synergistic codelivery of doxorubicin and curcumin. Nanomater. (Basel) 8 (3), 167. doi:10.3390/nano8030167
Yang, X., Ren, H., and Fu, J. (2022). Combinations of radiotherapy with immunotherapy in cervical cancer. J. Cancer 13 (5), 1480–1489. doi:10.7150/jca.65074
Yin, X., Ran, S., Cheng, H., Zhang, M., Sun, W., Wan, Y., et al. (2022). Polydopamine-modified ZIF-8 nanoparticles as a drug carrier for combined chemo-photothermal osteosarcoma therapy. Colloids Surfaces B Biointerfaces 216, 112507. doi:10.1016/j.colsurfb.2022.112507
Yoosefi, S. (2022). Novel biodegradable molecularly imprinted polymer nanoparticles for drug delivery of methotrexate anti-cancer; synthesis, characterization and cellular studies. DARU Journal of Pharmaceutical Sciences, 1–14.
Yu, S., Wang, Y., He, P., Shao, B., Liu, F., Xiang, Z., et al. (2022). Effective combinations of immunotherapy and radiotherapy for cancer treatment. Front. Oncol. 12, 231. doi:10.3389/fonc.2022.809304
Yun, K., Guo, J., Zhu, R., Wang, T., Zhang, X., Pan, H., et al. (2022). Design of ROS-responsive hyaluronic acid–methotrexate conjugates for synergistic chemo-photothermal therapy for cancer. Mol. Pharm. 19 (9), 3323–3335. doi:10.1021/acs.molpharmaceut.2c00472
Zhang, D., Jiang, L., and Liu, C. (2021). A convergent synthetic platform for polymeric nanoparticle for the treatment of combination colorectal cancer therapy. J. Biomaterials Sci. Polym. Ed. 32 (14), 1835–1848. doi:10.1080/09205063.2021.1941556
Zhang, D., Kong, Y. Y., Sun, J. H., Huo, S. J., Zhou, M., Gui, Y. L., et al. (2017). Co-delivery nanoparticles with characteristics of intracellular precision release drugs for overcoming multidrug resistance. Int. J. Nanomedicine 12, 2081–2108. doi:10.2147/ijn.s128790
Zhao, J., Zhao, M., Yu, C., Zhang, X., Liu, J., Cheng, X., et al. (2017). Multifunctional folate receptor-targeting and pH-responsive nanocarriers loaded with methotrexate for treatment of rheumatoid arthritis. Int. J. nanomedicine 12, 6735–6746. doi:10.2147/ijn.s140992
Zhao, Z., Hua, Z., Luo, X., Li, Y., Yu, L., Li, M., et al. (2022). Application and pharmacological mechanism of methotrexate in rheumatoid arthritis. Biomed. Pharmacother. 150, 113074. doi:10.1016/j.biopha.2022.113074
Zheng, D., Zhao, J., Li, Y., Zhu, L., Jin, M., Wang, L., et al. (2021). Self-assembled pH-sensitive nanoparticles based on Ganoderma lucidum polysaccharide-methotrexate conjugates for the Co-delivery of anti-tumor drugs. ACS Biomater. Sci. Eng. 7 (8), 3764–3773. doi:10.1021/acsbiomaterials.1c00663
Zhou, W., Deng, Y., Zhang, C., Liu, Z., Zhang, J., zhou, L., et al. (2022). Current status of therapeutic drug monitoring for methotrexate, imatinib, paclitaxel in China. Clin. Biochem. 104, 44–50. doi:10.1016/j.clinbiochem.2022.03.005
Ziyaee, S., Malekzadeh, R., Ghorbani, M., Nasiri Motlagh, B., Asghariazar, V., and Mortezazadeh, T. (2023). Preparation of MnO2@ poly-(DMAEMA-co-IA)-conjugated methotrexate nano-complex for MRI and radiotherapy of breast cancer application. Magnetic Reson. Mater. Phys. Biol. Med., 1–17. doi:10.1007/s10334-023-01091-1
Keywords: cancer therapy, methotrexate, nanoparticles, immune system, drug delivery
Citation: Mukhtar M, Ezra Manicum A-L, Shojaei Barjouei M, Eshaghi Malekshah R, Behzadmehr R, Rahdar A, Ghotekar S and Baino F (2023) Nanocarriers for methotrexate delivery/codelivery in the frame of cancer diagnostics and treatment: a review. Front. Front. Biomater. Sci. 2:1200670. doi: 10.3389/fbiom.2023.1200670
Received: 05 April 2023; Accepted: 19 May 2023;
Published: 05 June 2023.
Edited by:
Iman Yazdi, LiquiGlide, United StatesReviewed by:
Arul Prakash Francis, Saveetha Dental College and Hospitals, IndiaSajeesh Thampi, Lehigh University, United States
Copyright © 2023 Mukhtar, Ezra Manicum, Shojaei Barjouei, Eshaghi Malekshah, Behzadmehr, Rahdar, Ghotekar and Baino. This is an open-access article distributed under the terms of the Creative Commons Attribution License (CC BY). The use, distribution or reproduction in other forums is permitted, provided the original author(s) and the copyright owner(s) are credited and that the original publication in this journal is cited, in accordance with accepted academic practice. No use, distribution or reproduction is permitted which does not comply with these terms.
*Correspondence: Abbas Rahdar, a.rahdar@uoz.ac.ir; Suresh Ghotekar, ghotekarsuresh7@gmail.com; Francesco Baino, francesco.baino@polito.it