The 3-dimensional printing for dental tissue regeneration: the state of the art and future challenges
- 1State Key Laboratory of Oral Diseases, West China Hospital of Stomatology, Sichuan University, Chengdu, China
- 2National Engineering Laboratory for Oral Regenerative Medicine, West China Hospital of Stomatology, Sichuan University, Chengdu, China
- 3Department of Pediatric Dentistry, West China School of Stomatology, Sichuan University, Chengdu, China
- 4Yunnan Key Laboratory of Stomatology, The Affiliated Hospital of Stomatology, School of Stomatology, Kunming Medical University, Kunming, China
Tooth loss or damage poses great threaten to oral and general health. While contemporary clinical treatments have enabled tooth restoration to a certain extent, achieving functional tooth regeneration remains a challenging task due to the intricate and hierarchically organized architecture of teeth. The past few decades have seen a rapid development of three-dimensional (3D) printing technology, which has provided new breakthroughs in the field of tissue engineering and regenerative dentistry. This review outlined the bioactive materials and stem/progenitor cells used in dental regeneration, summarized recent advancements in the application of 3D printing technology for tooth and tooth-supporting tissue regeneration, including dental pulp, dentin, periodontal ligament, alveolar bone and so on. It also discussed current obstacles and potential future directions, aiming to inspire innovative ideas and encourage further development in regenerative medicine.
1 Introduction
As an important craniofacial organ, teeth perform crucial functions including mastication and pronunciation. Loss or damaged tooth can be caused by trauma, bacterial infection, gene disease, or craniofacial cancer, posing great threaten to oral health even general health. Though there are already available treatments to remove damaged tissue and restore morphology and function of tooth to a certain extent, such as resin restoration for caries, root canal therapy for infected pulp, dental implants for edentulism, all of these clinical procedures have limited prognosis and fail to achieve full replacement of native tissue. Therefore, regenerative therapeutic strategies are urgently needed as alternative methods to create dental tissue substitutes with original structure and function (Cooper, 2009; Sallum et al., 2019; Siddiqui et al., 2022).
The natural tooth features a sophisticated and hierarchically organized architecture. Given that, the reproduction of complicated structure and integrated complex tissue remain challenging (Duailibi et al., 2006; Yildirim et al., 2011). Nevertheless, the advancement of biomaterials, further exploration of stem/progenitor cells, as well as innovative biofabrication technologies are believed to facilitate dental tissue functional regeneration.
Currently, three-dimensional (3D) printing technology, or additive manufacturing, have developed tremendously, providing new breakthrough for tissue/organ regeneration (Mota et al., 2020). As a rapid prototyping technology, 3D printing precisely deposit cells, materials, and bioactive agents into pre-defined locations in a “layer-by-layer” manner and construct intricated biomimetic structures (Murphy and Atala, 2014). Compared with conventional methods of tissue engineering, a 3D-printed scaffold holds many advantages, such like high fidelity, customized topographies, and superior production efficiency (Zhou et al., 2020; Vu et al., 2021). Up to now, numerous studies have focused on the application of 3D printing strategy in the field of regenerative dentistry (Ma et al., 2019).
The process workflow of 3D printing dental tissue is briefly illustrated in Figure 1. The first step is to create a 3D model containing the macroscopic and microscopic structure of the scaffold. The data for the model can be acquired by computed tomography (CT) scanning for post-processing or directly by computer design, also known as computer-aided-design (CAD). Based on the target tissue, suitable biomaterials and cells are selected and prepared, as well as other bioactive additions. Then, the developed inks (only biomaterial) or bioinks (incorporating with living cells) are loaded into the 3D printer. The common 3D printing techniques include extrusion-based printing, as well as several variants such like fused deposition modeling (FDM)and melt electrowritten (MEW), inkjet-based printing and laser-assisted printing (e.g., stereo lithography appearance, SLA; digital light processing, DLP, selective laser sintering, SLS) (Mota et al., 2020). These techniques have variable features, which should be taken into account for manufacturing (Table 1). For cell-free printing, cell seeding is commonly performed after scaffold fabrication has been completed. Eventually, 3D printed constructs are cultured in vitro or implanted in vivo for biological regeneration.
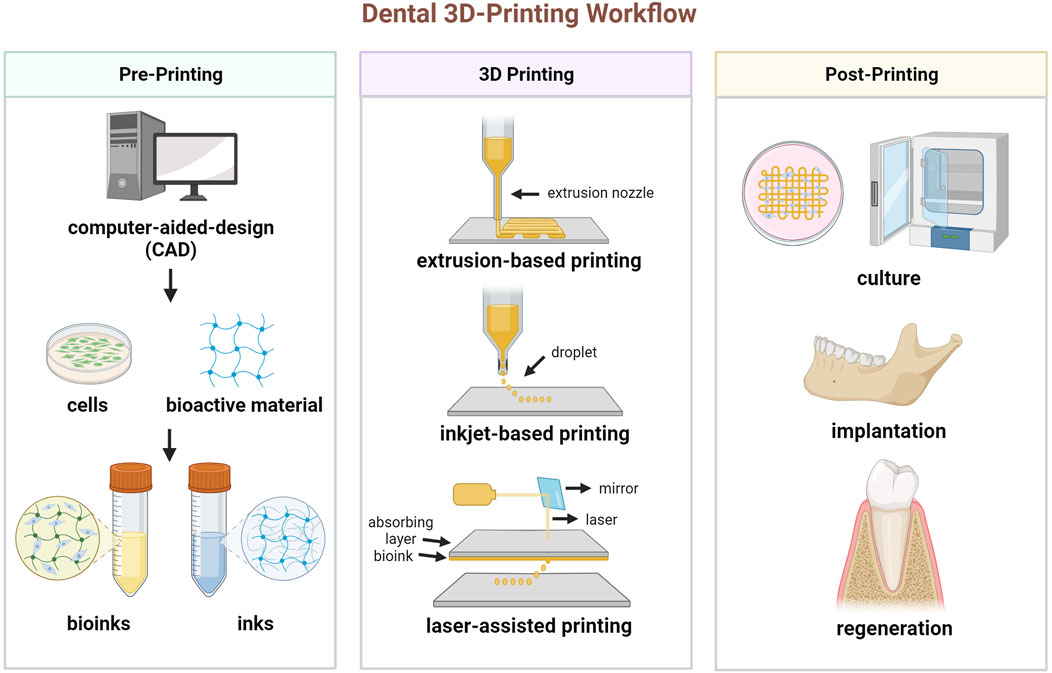
FIGURE 1. Schematic diagram of the process for 3D printing dental tissue. (Created with BioRender.com).
Even if 3D bioprinting still faces some hurdles, it has become an attractive and promising option to tooth and tooth-supporting tissue regeneration. In this review, we summarized the bioactive materials, cells, and recent progresses in the field of 3D printing for dental tissue, and then, we highlighted the challenges that have appeared in the tooth regeneration, and envision future directions for regenerative dentistry.
2 Biomaterials in 3D printing for dental tissue regeneration
Several types of bioactive material have been used to manufacture 3D-printed constructs for dental tissue regeneration, mainly including hydrogels, bioceramics, synthetic polymers and decellularized extracellular matrix (dECM). For mimicking the natural tissue microenvironment, hybrid bioactive ink or bioink are also developed (Table 2).
2.1 Natural hydrogel
2.1.1 Collagen
As the most abundant component in extracellular matrices (ECM), collagen is widely used as the scaffold material in tissue engineering (Wang Y. et al., 2023). Collagen contains arginine-glycine-aspartic acid (RGD)-motifs, which capable of mediate cell adhesion (Wu et al., 2021). Collagen is not only the main component of dental pulp and periodontium, but also the biomineralization matrix of dentin and bone. Thus, collagen-based bioink have been used for the reconstruction of dental pulp, periodontal ligament, and alveolar bone (Duarte Campos et al., 2020; Lee U.-L. et al., 2021; Li et al., 2022). Despite good biocompatibility, flexibility, and low immunogenicity, the poor mechanical property limit collagen’s use as bioinks. Incorporation with other hydrogel or organic/inorganic materials may be a feasible approach to improve mechanical stability and broaden the application boundaries of collagen-based bioink (Lee J. M. et al., 2021).
2.1.2 Chitosan
Derived from exoskeletons and shells of crustaceans, chitosan is abundant in nature and known as a widely used scaffold material in tissue engineering. Chitosan possesses high biocompatibility, hydrophilicity, and controllable biodegradability (Szulc and Lewandowska, 2022). Moreover, a broad spectrum of antibacterial properties makes it unique advantage for the dental application (Li Y. et al., 2020). Numerous studies have proven that 3D-printed chitosan-based scaffold can be employed for dental tissue regeneration due to its tunable physiochemical properties and favorable biological response (Suo et al., 2023). EzEldeen et al. (2021) explored the effects of chitosan source (animal vs. fungal), co-polymerization with gelatine, and crosslinking agent (3-glycidyloxyproply trimethoxysilane, GPTMS or genipin) on scaffold properties and biological response. However, the low mechanical property and sterilization strict the application of chitosan-based hydrogel in the bioprinting field (Lazaridou et al., 2022). Therefore, many attempts have been made to enhance the mechanical strength of chitosan by functional groups modification and co-crosslinking with other molecules (Liu et al., 2021; Salar Amoli et al., 2022).
2.1.3 Alginate
As a natural biopolymer, alginate attracted scientists’ focus for its water solubility and rapidly cross-linked by calcium ions at room temperature. Besides, alginate possesses proper mechanical property, tunable viscosity and acceptable printability, which make it suitable for bioprinting technique (Gao et al., 2021). However, the pure alginate lacks RGD motifs for cell attachment, and the enzyme that cleave alginate chain is absent in human body, leading to the uncontrollable biodegradation in vivo. Given that, alginate is often used with other materials to form composites for bioinks (Rastogi and Kandasubramanian, 2019; Yu et al., 2019).
2.1.4 Bioceramics
Bioceramic materials, including β-tricalcium phosphate (β-TCP), hydroxyapatite (HA), bioglass, calcium silicate (CS) and so on, have been well investigated as scaffold materials in traditional tissue engineering manufacturing for their excellent biocompatibility, favorable bioactivity and easy tailored characters (Baino et al., 2015). Owing to the intrinsic similar to the inorganic composition of bone and tooth, bioceramics are often used for the regeneration of hard tissues. But they also have disadvantages of low fracture resistance, low flexural resistance and wettability (Raveau and Jordana, 2020).
Typically, bioceramics often added as fillers to the polymer matrix to obtain ideal physicochemical and mechanical properties. A study conducted by Ahlfeld et al. (2023) indicated that varieties of mineral fillers, including CaCO3, SrCO3, strontium-modified hydroxyapatite (SrHAp) or tricalcium phosphates, integrated into a PLGA matrix can remarkably modify the degradation behavior of printed scaffold and an increase of the specific alkaline phosphatase activity were observed. Besides, mesoporous calcium silicate is also used in hard tissue regeneration as a component of biomaterial inks because of its ability to sustained release Si ions and other bioactive agents (Huang et al., 2018; Yeh et al., 2022). A bioactive strontium-doped calcium silicate (SrCS) scaffold released Sr and Si ions even after 6 months and enhanced secretion of osteogenic-related proteins (Wang et al., 2021).
Commonly, hydrogels are mixed with bioceramics particles for enhanced mechanical stability and adjustable biological properties. Tian et al. explored a novel bioink composed of sodium alginate (SA), gelatin (Gel), and nano-hydroxyapatite (na-HA). The SA/Gel/na-HA hydrogel exhibited shear-thinning behavior, optimized equilibrium swelling rate and compression modulus, as well as significantly improved the osteogenic differentiation of human periodontal ligament stem cells (hDPLSCs) (Tian et al., 2021).
2.1.5 GelMA
Gelatin methacryloyl (GelMA) is a gelatin derivative modified by methacrylamide and methacrylate groups. Resembling the native ECM, GelMA contains many RGD-sequences for cell attachment as well as matrix metalloproteinase (MMP)- degradable motifs for cell remolding (Yue et al., 2015). As a photo-crosslinked hydrogel, GelMA undergoes photoinitiated radical polymerization without chemical crosslinkers that may be detrimental to cells (Klotz et al., 2016). For its tunable mechanical characteristics and suitable biological properties, GelMA is a versatile matrix that can be vastly used in biomedical application (Sakr et al., 2022). Numerous studies have proven that GelMA-based scaffolds can promote cell viability and osteo/odontogenetic differentiation of dental stem cells, including DPSCs, PDLSCs and SCAPs (Ha et al., 2020; Buyuksungur et al., 2021; Zhu et al., 2023).
Not only served as cell delivery vehicles in bioprinting, GelMA also plays a key role in drug and growth factor delivery due to its biodegradable properties. Park et al. (2020) developed bone morphogenetic protein (BMP) peptide-tethering bioink by conjugating peptides to GelMA. The results showed that BMP-mimicking peptide remained in bioprinted construct for more than 50% after 3 weeks and robustly increased the expression of odontogenic-related genes of hDPSCs.
A study conducted by Choi et al. (2022) used two different types of MTA (ProRoot MTA and Endosem Zr) combined with GelMA and evaluated the biological effects on hDPSCs. Although 3D-printed MTA/GelMA scaffolds showed more calcium deposition, elevated expression of odontogenetic-related genes was not statistically significant. Lin et al. fabricated the composite scaffold with CS reinforced-GelMA bioink. The CS/GelMA bioink is capable of inducing odontogenic differentiation of hDPSCs. Another study showed that the addition of mesoporous bioactive glass nanoparticles significantly enhanced shape fidelity, surface roughness, and bioactivity (Mei et al., 2022).
2.1.6 Synthetic polymer
Synthetic polymers are another kind of vastly used materials in tissue engineering. They have the advantages of adjustable physical and chemical properties, which makes it suitable candidates for the dental tissue regeneration.
Polylactic acid (PLA) and poly(L-lactic-co-glycolic) acid (PLGA) are well-established polymers for their biosecurity approved by Food and Drug Administration (FDA) and excellent manufacturing ability. Moreover, PLGA possesses tunable degradation rate by different the ratio of lactic acid to glycolic acid, which can be employed as carries of biomedical substance (Danhier et al., 2012). However, both PLA and PLGA undergo hydrolytic degradation and generates acid degradation products that may trigger local inflammation in vivo, thus confine their application (Ramot et al., 2016; Li et al., 2017). Polycaprolactone (PCL) is also conventional scaffold material routinely used in 3D-printing and has desired biocompatibility and biodegradability. Nevertheless, the lack of cell-anchoring site and hydrophobic surface lead to the low bioactivity of synthetic polymers (Place et al., 2009). Various attempts have been made for the optimization of biological properties, including surface modification, biomaterial coating, introduction of functional groups and so on (Bow et al., 2021; Feng et al., 2021; Park et al., 2021).
Given that these traditional polymers often require organic solvent for dissolving in 3D printing process and cause solvent residue in the final construct, in recent years, waterborne biodegradable polyurethane (wPU) have shown great potential as 3D-printing materials (Hung et al., 2016). However, very few studies are found about PU application in the dental tissue regeneration. A study published recently employed PU scaffolds coated with boric acid. The wPU/boric acid scaffold can trigger the osteogenic differentiation of DPSCs without significantly reducing cell viability and proliferation (Çelebi-Saltik et al., 2023).
2.1.7 Decellularized extracellular matrix
dECM refers to the natural tissue with the removal of immunogenic cellular components while three-dimensional network of ECM and biological molecules were remained, including various proteins and polysaccharides. Compared with other hydrogels, dECM provides not only mechanical support and adhesion cites for cells, but also biological cues to impact cell behavior by cell-ECM interactions (Zhang et al., 2022).
Several studies have proven that dECM derived from dental tissue/cells hold the capacities of recruiting stem cells, promoting osteogenesis, and forming pulp-like tissue (Smith et al., 2012; Heng et al., 2016; Nowwarote et al., 2021; Tan et al., 2021). Due to the rising demand for biomimetic microenvironments of 3D printed constructs, dECM is gradually becoming a promising bioink material (Kim et al., 2022; Yang et al., 2023).
Treated dentin matrix (TDM), also termed as “demineralized dentin matrix” (DDM), is a kind of specific dECM that referred to dentin matrix after a certain degree of demineralization treatment. With exposed dentin tubules and collagen fibrils, TDM released abundant dentinogenetic-related biomolecules including COL-1, DSPP, DMP-1, TGF-β1, decorin, biglycan, and created an inductive microenvironment for dentin regeneration (Guo et al., 2009; Li et al., 2011). Athirasala et al. (2018) mixed the insoluble components of the DDM with alginate in 1:1 ratio, and then added extracted soluble dentin molecules, dramatically promoting hydrogel-encapsuled SCAPs differentiate into dentin. Besides, TDM particles are also used to form particle-based bioink and regenerate specific dental tissue or whole tooth (Huang et al., 2023).
3 The stem/progenitor cells used in the dentistry 3D printing
3.1 Dental pulp stem cells (DPSCs)
Dental pulp stem cells (DPSCs) was firstly isolated and identified by Shi and Gronthos in 2000 (Gronthos et al., 2000). As the mesenchymal stem cell, DPSCs possess self-renewal potential and multi-directional differentiation capacity, being capable of differentiating into odontoblasts, chondrocytes, adipocytes, and neural-like cells in vitro and regenerate dentin-pulp complex in vivo (Gronthos et al., 2002; Wei et al., 2007). The typical microscopic morphology of human DPSCs is shown in Figure 2. Compared with bone marrow-derived mesenchymal stem cells (BMMSCs), DPSCs express more odontoblast-related genes, such as ALP, DSPP, and DMP-1 (Yamada et al., 2006). Due to their wide sources and minimally invasive process (Ferrúa et al., 2017), DPSCs have already became a kind of ideal seeding cells for tooth tissue engineering (Anitua et al., 2018; Liu P. et al., 2022). DPSCs loaded 3D-printed PLA/HA scaffold exhibited higher degree of mineralization than the cell-free scaffold (Chen et al., 2021). Some studies have also used gene-modified dental pulp stem cells for 3D printing (Wang W. et al., 2023).
3.2 Stem cells from human exfoliated deciduous teeth (SHEDs)
In 2003, Miura firstly found that exfoliated human deciduous tooth contains a population of multipotent stem cells and named it stem cells from human exfoliated deciduous teeth (SHED) (Miura et al., 2003). Similar to DPSC, SHEDs are capable of differentiating into odontoblast, adipocytes and neural-like cells. Owing to the immaturity of SHEDs, several studies suggested that SHEDs are more proliferative than DPSCs and BMMSCs (Nakamura et al., 2009; Wang et al., 2012). In addition to the high proliferation rate and multilineage differentiation potential, the noninvasive harvesting procedure also make SHEDs a promising cell source for the regeneration of tooth, cartilage and neural tissue (Yang X. et al., 2019; Pereira et al., 2019; Mahdavi-Jouibari et al., 2023).
3.3 Periodontal ligament stem cells (PDLSCs)
Periodontal ligament stem cells (PDLSCs), firstly isolated from periodontal ligament by Seo in 2004, are progenitor cells of cementoblasts, periodontal ligament fibroblasts and alveolar bone osteoblasts. Seo also found that PDLSCs hold the potential to generate cementum/PDL-like structure in immunocompromised mice (Seo et al., 2004). As one of the well-established stem cells in the field of regenerative dentistry, PDLSCs have been considered as ideal seeding cells of 3D printed scaffold that are capable of regenerating periodontal tissue both in vivo and in vitro (Park et al., 2012; Lee et al., 2014; Daghrery et al., 2023).
3.4 Stem cells from apical papilla (SCAPs)
The stem cells from apical papilla (SCAPs), located on the exterior of the immature permanent tooth root foramen area, plays an important role in the root formation and development. In 2006, Sonoma collected human root apical papillae from young adults (18–20 years old) extracted third molars and isolated stem/progenitor cells that express the mesenchymal stem cells marker: STRO-1 (Sonoyama et al., 2006). It is demonstrated that SCAPs hold the capacity to regenerate dentin-like and pulp-like tissue in vivo (Sonoyama et al., 2006; Huang et al., 2010; Hilkens et al., 2017). Besides, SCAPs can also differentiate into cementoblasts in vitro, which hold the promise for periodontal regeneration (Ebadi et al., 2023).
3.5 Dental follicle stem cells (DFSCs)
The dental follicle is an ectomesenchyme-derived connective tissue that surrounds the enamel organ and dental papilla, playing a key role in periodontal tissue formation and tooth eruption (Zhang et al., 2019). Dental follicle stem cells (DFSCs) are mesenchymal stem cells isolated from dental follicle tissue and are considered as precursor cells of cementoblasts, osteoblasts and periodontal ligament cells (Morsczeck et al., 2005). The present researches have confirmed that DFSCs hold the capacity of regenerating bone, dentin, periodontal tissue and tooth root-like tissue in vivo (Guo et al., 2009; Guo et al., 2012; Yang H. et al., 2019; Meng et al., 2022). Though limited literatures reported the application of DFSCs in dental tissue bioprinting, it is gradually attracting more and more attention.
3.6 Others
In addition to the cells mentioned above, there also various kinds of stem/progenitor cells used for tooth and periodontal regeneration, such like bone marrow derived mesenchymal stem cells (BMSCs) (Golafshan et al., 2023; Miao et al., 2023), Hertwig’s epithelial root sheath (HERS) cells (Tang et al., 2022), dental papilla cells (DPCs), gingival fibroblast cells (GFs) (Wang et al., 2021; Liu et al., 2023) and induced pluripotent stem cells (iPSCs) (Kim et al., 2023).
4 3D printing for dental tissue regeneration
The natural tooth is composed of both hard tissue (dental enamel, dentin, cementum and alveolar bone) and soft tissue (dental pulp, periodontal ligament, gingiva). Tables 3, 4 summarized recent advancements in dental soft and hard tissue printing respectively. Notably, natural tissues and organs typically exhibit compartmentalized architecture with an intrinsic integration of diverse components, and the tooth follows suit. Therefore, Table 5 presents the applications of 3D printing/bioprinting in the regeneration of composite tissue, including the dentin-pulp complex, periodontal complex, as well as whole tooth and tooth root.
4.1 Soft tissue regeneration
4.1.1 Dental pulp regeneration
Surrounded by rigid dentin walls, dental pulp is a loose connective tissue composed of cells (odontoblasts, fibroblasts, DPSCs), collagen, nerves and blood vessels. Retaining vital pulp is of great importance for tooth nutrient, repairment, and sensory, especially for immature permanent teeth. When dental pulp infected, root canal therapy (RCT) remains the first-option in clinical practice. However, tooth after RCT is faced with problems such as persistent inflammation, discoloration, and increased fragility (Ricketts, 2001). While pulp revascularisation presents a promising clinical approach, it fails to reconstruct functional pulp-like tissue and leads to unexpected calcification (Chen et al., 2012). Therefore, stem cell-based regenerative endodontic therapy has attracted the worldwide attention.
To achieve cellular pulp regeneration, it is essential to create a microenvironment conducive to stem cell proliferation and differentiation. Hydrogel proves to be a fitting candidate owing to its resemblance to the natural ECM. Various hydrogel have been evaluated for pulp bioprinting, encompassing fibrin, hyaluronic acid, GelMA and so on (Han et al., 2019; Nejad et al., 2021; Cunha et al., 2023).
Pulp vascularization is one of the key objectives as well as challenges in functional pulp regeneration. Several strategies have been utilized including the sacrificial material method and co-culture with endothelial cells (Athirasala et al., 2017). Duarte Campos et al. (2020) injected collagen based bioink into prepared root canal via a handheld bioprinter. The results of immunofluorescence showed successful vascularization in the root canal without the shrink of bioink. However, these strategies have yet to demonstrate their feasibility in vivo.
Remarkably, in a recently published work, Qian et al. successfully fabricated DPSC-loaded GelMA microspheres via a DLP printer. These cell-loaded microspheres have improved stemness and higher multi-directional differentiation potential, including angiogenic, neurogenic, and odontogenic differentiation. The regeneration of vascularized and neutralized pulp-like tissue was demonstrated by subcutaneous transplantation in nude mice and in situ experiment in swine (Qian et al., 2023).
4.1.2 Periodontal ligament regeneration
Periodontal ligament (PDL) is a connective tissue that is mainly composed of collagen type I fibers and proteoglycans. Owing to the firm attachment between cementum and alveolar bone and specific oriented collagen fibers, it provides mechanical stability and attenuate the masticatory stress to protect tooth. Furthermore, PDL contains an abundance of blood vessels and nerve endings that provide nourishment to the cementum and alveolar bone. Utilizing mesenchymal stem cells present in the periodontium, repairment and remodeling of periodontal tissue are possible (Hurng et al., 2011). Hence, the PDL regeneration is an essential part of periodontal regeneration.
3D printing holds its intrinsic advantage to form highly-arranged collagen fibers because of the exist of micro-strands and precise control of cell position in the 3D network. It has been proven that compared with cell-seeding bulk collagen, the cell-laden collagen by bioprinting showed significantly more lang periodontal ligament-like soft tissue on the surface of titanium implants (Lee U.-L. et al., 2021).
To physically control the orientations of fiber bundles, so called “fiber-guiding scaffold,” has emerged and attracted increasing focus. Lin H.-H. et al. (2021) fabricated a collagen-based waveform microfibers and evaluated the microcrimped scaffold under shear load (6 dyne/cm2). Compared with straight microfibers, waveform microfibers exhibited enhanced cell spreading and adhesion, as well as upregulated expression of periostin (a key molecule for extracellular matrix assembly).
Kim and Park (2020) created microgroove patterns on the scaffold surfaces with different slice intervals. After 7 days culture, the μG-25 (25.40 µm intervals) showed the ability to organize aligned PDL cells while the μG-6 (6.35 µm intervals) leaded random organization. Similarly, Pilipchuk et al. (2016) employed 3D-printed scaffold combined with micropatterned film to achieve regeneration and integration of alveolar bone and PDL. Their conclusions support that optimized surface tomography induce specific orientations of PDL fibers in a more predictable manner.
4.1.3 Gingival regeneration
Gingiva is another key component of oral soft tissue, which is essential for oral structural integrity and dental aesthetics. Although autogenous gingival grafts have been widely used to reverse gingival recession and augment periodontal soft tissue, the lack of donor tissue sources, the complexity of the surgical procedures, and the postoperative pain and numbness still hamper a good prognosis (Yang M. et al., 2019). Therefore, the tissue engineering strategy seems to be a new option for regenerative oral soft tissue augmentation. Tang along with his team developed a kind of bioink composed of alginate, gelatin and injectable platelet-rich fibrin (i-PRF), which can release various repair-related growth factors. In vivo experiments, 3D-printed constructs with 50% i-PRF showed more collagen formation and host tissue infiltration compared to the group without. In their latest study, they bioprinted acellular dermal matrix (ADM)-based hydrogel composite and performed in vivo implantation in beagles, demonstrating that GF-loaded ADM scaffolds significantly promote keratinised gingival regeneration (Yi et al., 2022; Liu et al., 2023).
4.2 Hard tissue regeneration
4.2.1 Enamel regeneration
Dental enamel is the hardest tissue in the human body, providing external protection for the tooth against masticatory forces. As a kind of non-vascular, acellular, highly mineralized hard tissue, enamel is composed of substituted hydroxyapatite and organic macromolecules (Nanci, 2013). Since the enamel organ disappears at the end of the tooth germ development, there are no enamel epithelial cells in a mature tooth, resulting in the lack of enamel regeneration ability. Thus, development of alternative approaches to repair and regenerate enamel defects is much needed but remains challenging due to the highly-ordered hydroxyapatite structure. Zhao et al. (2022) used supergravity preparation technology and hydrothermal treatment to disperse the Hap nanorods in the hybrid resin-based composites (RBCs), developing a new bioactive ink. The printed tooth crown possesses multi-scale highly ordered structure and strong mechanical strength. Mohabatpour et al. (2022) combined carboxymethylcellulose (CMC) and alginate to create a new bioink that induced the differentiation of dental epithelial cells (HAT-7) to enamel-forming cells and promoted Ca and P deposition as well as matrix mineralization in vitro.
4.2.2 Dentin regeneration
Human dentin is composed of hydroxyapatite (70% by weight) and an organic matrix (35% by weight) of collagenous and noncollagenous proteins, such as dentin sialophosphoprotein (DSPP), dentin matrix protein-1 (DMP-1), osteopontin, and osteocalcin (Zhang et al., 2014). Dentin regeneration is one of the most explored parts in the field of dental tissue engineering. 3D printing is capable of forming customed macro structures and intricated interconnections. Compared with conventional cast-molded hydrogel, 3D-printed alginate/gelatin scaffold can better promote the cell adhesion, viability, and osteogenic/odontoblastic differentiation of hDPSCs (Yu et al., 2019). Besides, 3D printed Biodentine/PCL scaffold exhibited a good apatite-forming ability and induced hDPCs proliferation and differentiation (Ho et al., 2018).
Incorporation of biological cues also helps to induce the odontogenic differentiation of stem/progenitor cells, and then promote the secreting and mineralization of dentin matrix. The extracts of dentin matrix, both soluble and insoluble molecules, significantly enhanced odontogenic differentiation of SCAPs encapsulated in bioprinted hydrogels (Athirasala et al., 2018). Similarly, Han et al. (2021) mixed DDM particles with fibrinogen-gelatin to produce a DDM particle-based bio-ink, which enhanced the dentin-oriented differentiation of DPSCs. Besides, the study by Kim et al. indicated that supplemented with bone-derived dECM can accelerate the odontogenic differentiation of hDPSCs (Kim et al., 2022).
In addition to bioactive molecules, Yeh et al. (2022) fabricated a mesoporous calcium silicate/calcium sulfate (MSCS) scaffold and added quercetin, a novel bioactive molecule which can reduce inflammatory mediator and inhibit osteoclast activity, into the printed scaffold. The composite scaffold not only possessed better physicochemical and biological properties but also enhanced odontogenic and immuno-suppressive properties. Feng et al. (2021) coated a 5 nm thick titania layer via atomic layer deposition on the 3D printed PLA scaffold, and higher DPSCs plating efficiency and upregulated expression of osteocalcin compared with uncoated PLA scaffolds were observed.
4.2.3 Alveolar bone regeneration
Alveolar bone loss is often caused by periodontitis or peri-implant inflammation. Regeneration of alveolar bone is one of the goals of periodontal therapy, and it is also a prerequisite for implant restoration. Compared with other craniomaxillofacial bone constructs, alveolar bone scaffold design and manufacture as a tooth-supporting tissue remains a challenge due to the structural complexity and geometric adaptability of tooth-root surface.
Bioceramics play a crucial role in the bone tissue engineering. Qin et al. explored the effect of different pore dimensions (Ø 480, 600, and 720 μm) on mechanical properties, bioactive ion release, and bio-dissolution of bioceramic scaffolds. The 600 μm group exhibited maximal new bone formation in the rabbits’ mandibular bone defects model (Qin et al., 2022). In another study, Osteoink™, composed of hydroxyapatite and α-TCP, was utilized to develop calcium phosphate (CaP)-based bioceramic scaffolds for alveolar bone reconstruction. The printed scaffolds had a high degree of accuracy to fit the respective clinical defects for which they were designed (Anderson et al., 2022). A newly developed bioceramic material, ∼10% Mg-substituted wollastonite (Ca90%Mg10%SiO3; CSi-Mg10) was also used to fabricated customed scaffolds for reconstruction of alveolar bone defect, exhibited desired osteoconduction, osteoinductivity and adequate biodegradation (Shao et al., 2018).
GelMA is another well-established material for alveolar bone reconstruction by virtue of its analogue to extracellular matrix and adjustable physicochemical properties. 3D-printed PCL/GelMA hybrid scaffolds yielded cell viability, osteogenic differentiation of DPSCs and mineralization in vitro (Buyuksungur et al., 2021). Tang et al. (2022) combined HERS cells and dental papilla cells (DPCs) to mimic the micro-environment of cell-cell interaction. Plenty of mineralization texture were observed when transplanted into the rat’s alveolar sockets. GelMA/PEGDA hybrid hydrogel were fabricated by controlling the volume ratio of GelMA-to-PEGDA. The results indicated that the 4/1 GelMA/PEGDA composite hydrogel exhibited best performance in osteogenic differentiation and formed new bone tissue in vivo (Ma et al., 2017). Luo et al. (2022) developed GelMA/β-TCP hybrid scaffolds which showed good biocompatibility and mechanical properties, and stimulated osteogenesis of BMSCs.
Furthermore, the supplement of growth factors, peptides, and other bioactive substances undoubtedly endows 3D printed constructs with superior biological properties, such as antimicrobial peptide KSL-W (Li et al., 2022), recombinant human erythropoietin (rh-EPO) (Liu H. et al., 2022) and graphene oxide (Park et al., 2021).
4.3 Multi-tissue regeneration
4.3.1 Dentin-pulp complex regeneration
The dentin and dental pulp derived from dental papilla, which is formed during tooth germ development and participate in tooth activities together as the dentin–pulp complex (Abbass et al., 2020). Dentin offers durable shielding for the pulp, whereas the pulp supplies essential nutrients for the dentin and generates tertiary dentin as a response to caries, mechanical injury, or acid erosion. Therefore, the regeneration of vital dentin-pulp complex is the ideal target for endodontic medical treatment.
It has been widely studied that the fate of stem cells is closely associated with ECM characteristics (Liu et al., 2018). In this regard, Han et al. (2019) developed hDPSCs loaded bioink with different fibrinogen content to print dentin and pulp tissues (F20 for dentin, F5 for dental pulp) respectively. Bilayer scaffolds with region-specific induced soft and hard tissues are likewise seen as one of the viable strategies. Nejad et al. (2021) constructed PCL/45S5 bioglass (BG)-PCL/hyaluronic acid (HyA) scaffold and evaluated the physicochemical properties of the hybrid scaffold. The results showed that PCL/BG scaffolds had superior mechanical strength and surface roughness, and the PCL/HyA scaffolds possessed increased hydrophilicity for cell adhesion. Recently, Cunha et al. (2023) utilized 3D-printed microgels doped with dentin matrix molecules for direct dental pulp capping. Although both the microgel group supplemented with DMM particles and the MTA group showed the formation of organized pulp tissue, dentin-like tissue and new blood vessels, the presence of DMM helped to promote dentin bridge formation and exhibited more tertiary dentin deposition and attenuate less pulp necrosis.
4.3.2 Periodontal complex regeneration
Periodontitis is one of the most prevalent oral diseases in the world, resulting in periodontal attachment loss, alveolar bone resorption, even tooth loss (Kwon et al., 2021). The goal of periodontal treatment is to control the infection and reconstruct the functional tooth-supporting tissue. Guided tissue regeneration (GTR) is currently gold standard of periodontal tissue reestablishment by a barrier membrane to allow selective repopulation of PDL cells and bone, yet it still suffers from various limitations as well as clinical variability (Karring et al., 1993). The periodontal complex comprises multiple tissues, including periodontal ligament, cementum and alveolar bone, forming a “sandwich-like” structure. Herein, multiphasic scaffolds to mimic the cementum-PDL-alveolar bone complex has increasingly attracted the attention of researchers (Ivanovski et al., 2014).
In previous studies, Lee CH and his colleagues developed various growth factor-releasing scaffolds by loaded PLGA microspheres for periodontal tissue regeneration. They initially achieved integrated cementum-like tissue on the surface of dentin with high expression of cementum matrix protein 1 (CEMP1), an important marker of cementogenesis (Cho et al., 2016). Their another study used multiphase scaffolds for periodontium complex regeneration via spatiotemporal delivery of multiple bioactive cues, involving recombinant human amelogenin, CTGF and BMP-2. They also demonstrated the different microstrands spacing have an effect on the generation of integrated multiple tissues (Lee et al., 2014). Consistent with Lee’s studies, Daghrery et al. engineered PCL scaffold with F/Ca coating and revealed that aligned fibers strongly support ligamentogenesis and scaffold architecture with 500 μm strands spacing supports osteogenesis. The results of Masson’s trichrome staining indicated that the tissue-specific scaffold induced the regeneration of both soft and hard tissue in vivo (Daghrery et al., 2021; Daghrery et al., 2023). Furthermore, a novel composite hydrogel was designed as cell-laden bio-ink, encapsulating BMP-2 and PDGF that effectively facilitate alveolar bone regeneration and promote gingival healing respectively in the periodontal defect model of beagles (Miao et al., 2023). Ma et al. (2023) reported biomimetic periodontium patches (BPPs) by DLP technology. The BPPs featuring correctly oriented fibers and the assessment of clinically functional properties proved the regenerative periodontium can stand orthodontic migration force to achieve stable tooth movement.
Another important challenge to be solved is the interface integration. Soft-to-hard tissue interfaces have always been a tricky problem in tissue engineering for the difficulty to reproduce interface enthesis (Calejo et al., 2019). To overcome the lack of adhesion between newly formed PDL and bone, Blaudez et al. (2023) designed a decellularized construct consisting of consisting of PDL cell sheet and PCL scaffold for tissue-specific periodontal regeneration. Before decellularization, the biphasic construct was cultured for 24 h to allow cell sheet adhesion and enhance the cohesion between periodontium and bone part. Yao et al. (2022) introduced a transition region with 500-µm filament spacing, while the bone region was 250-μm filament spacing and the PDL region was consisted of aligned PCL filaments. The transition region separated the tissue-specific regeneration in the other two parts at the early time and promoted cellular cross-communication later between two regions. The insertion of ligamentous fibers towards the bone and calcium gradient were observed in the transition space. Similarly, Golafshan et al. (2023) fabricated a tri-layer scaffold in which PCL matrix with MgP was for bone, highly aligned PCL fibers were presented for PDL and random PCL fibers were printed to mimic the bone-to-PDL interface. The tri-layer scaffold showed enhanced mechanical stability and achieved coordinated periodontal tissue regeneration in the rodent model. Moreover, a recent study reported a biomimetic periodontal module with high architectural integrity. The module provided high-precision topographical cues and biochemical environment conducive to regulating cell behavior and achieving periodontal regeneration with the enthesis of the bone-ligament interface and well-aligned fibers in a beagle model (Yang et al., 2023).
4.3.3 Whole tooth/bio-root regeneration
Whole tooth regeneration with natural tooth morphology and function is the ultimate goal of dental regenerative medicine. Back in 2010, Kim et al. fabricated anatomically shaped human molar scaffolds and rat mandibular incisor scaffolds by 3D printing and performed orthotopic implantation of rat incisor scaffolds, combined with the delivery of SDF1 and BMP7. After 9 weeks, the histological results revealed regeneration of the PDL-like tissue and new bone at the interface between the scaffolds and native alveolar bone (Kim et al., 2010). While it is possible to replicate the morphology of natural teeth, grafts often struggle to form a strong enough periodontal bond with the host jawbone in a short period of time, which can compromise their ability to withstand substantial occlusal forces.
Tooth germ recombination appears to be another viable path to achieving whole tooth regeneration (Zhang and Yelick, 2021). Studies have been conducted to generate bioengineered tooth germs by the reconstruction of dental epithelial and mesenchymal cells (Ono et al., 2017). However, it is still tough to regenerate eruption-competent tooth germs hence the epithelial-mesenchymal transition during tooth development is yet to be completely unveiled and replicating spatiotemporal interactions between different cells remains challenging. 3D bioprinting technology is thought to offer new possibilities for bioengineered tooth germ due to its ability to precisely control microstructures at the cellular level.
Therefore, fabricating bioengineered roots, also known as “bio-root,” and then restoring with artificial crown seem to be a much more feasible approach. Bio-root, referred to the bioengineered tooth root, was firstly proposed by Sonoyama in 2006 and has demonstrated the ability to regenerate dentinal tubule-like and periodontal ligament-like structures in swine model (Wei et al., 2013). Up to recently, Huang et al. developed a personalized 3D-printed scaffold with PCL and TDM. Notably, the TDM scaffolds combined with DFSC sheets served as an analogue to natural tooth root and with satisfied angiogenic capacity in orthotopic transplantation in beagles (Huang et al., 2023).
Besides, a study by Chen et al. fabricated personalized bio-root by DLP printing of hydroxyapatite bioceramic. Bioceramic sintered at 1,250°C exhibited almost twice elastic modulus than natural decellularized dentine, which significantly enhanced the physicochemical properties of Hap. The nano-HAw (nano-hydroxyapatite whiskers) coating improved both mechanical property and surface hydrophilicity, and periodontal ligament-like enthesis formation in-situ transplantation in rat alveolar fossa (Chen et al., 2023).
5 Discussion
Although restorative treatment is still the mainstream treatment, regenerative treatment is gradually occupying a place in dentistry clinical practice. Notably, 3D printing technology is a promising solution in the field of regenerative medicine. 3D printing holds inherent advantages of construct geometric topologies that affect cell behavior and reproduce intricated microstructure to mimic the natural tissue/organ physiochemical and biological properties.
In general, the materials used for 3D printing-based tooth regeneration should conform the following characteristics: 1) bioactivity with stem/progenitor cells; 2) ease of processing; 3) proper mechanical properties that match the corresponding tissue; 4) ideal physical characteristics, such like porosity, surface roughness, wettability, and viscosity. The types of biomaterials commonly used in 3D dental printing are summarized above. Currently, composite materials are gaining increasingly focus for the single material fail to yield heterogeneous constructs. Besides, biological agents are integrated to the printed constructs for multifunctional biological effects, such like growth factors, mRNA, exosomes, and drugs (Zhou et al., 2018).
Dentoalveolar tissue exhibits compartmentalized architectures with structural integrations. Though studies referred to in this review has achieved regeneration of dental tissues to varying degrees, there are challenges need to be addressed, such as adequate vascularization, neuralization, and internal integration into the host tissue. Moreover, for the acellular hard tissues subjected to strong mechanical loads, the balance between prolonged mineralization and scaffold degradation is still a tackle problem. The regeneration of a single tissue cannot meet the needs of functional tooth reconstruction, herein, multi-tissue biofabrication of dental tissue has emerged as a prominent area of investigation. The utilization of multiphasic scaffolds with designed tissue-specific organization for detin-pulp and periodontal complex have been discussed above. However, scaffolds fabricated in this way usually lack interphasic cohesion and lead to compromised stability of printed constructs.
To better mimic natural tooth tissues and achieve functional tooth reconstruction, further research should be undertaken in several areas. One is to develop novel 3D printing technique with higher printing resolution. Up to now, extrusion-based 3D printing is the most used printing technique in the field of tissue engineering by virtue of easy access to material and low cost, but the printing precision is not comparable to laser-assisted printing. Secondly, the regulation of stem cell fate by the biomechanical properties of the extracellular matrix needs to be further explored, which will inspire the cell-matrix interaction in the printing architecture. The emerging 4D printing is capable of creating scaffolds that can create controlled dynamic cellular microenvironment, providing a potential tool for recapturing the natural process of tooth genesis and restoration (Wang et al., 2022). Another direction of development lies in immunomodulation for 3D printed constructs. The biomaterial scaffolds often trigger the host immune rejection in vivo, leading to the chronic inflammation and eventually regeneration failure (Whitaker et al., 2021). Only a few studies have reported the immune effects of 3D printed dental substitutes, including modulation of inflammatory factors and macrophage polarization.
Whilst the laboratory studies about 3D printing for dental tissue are encouraging, they are yet to be translated into clinical practice. There have only been a limited number of studies which have reported on the clinical evaluation of 3D-printed constructs in the human body. To bridge the gap between laboratory and clinical applications, further safety and function tests are urgently required. In conclusion, 3D printing technology displays promising translational and therapeutic potential for the regeneration of dental tissue, which lays a strong foundation for regenerative medicine.
Author contributions
FZ: Writing–original draft, Conceptualization, Investigation, Visualization. ZZ: Writing–review and editing, Project administration. WG: Funding acquisition, Supervision, Writing–review and editing.
Funding
The authors declare financial support was received for the research, authorship, and/or publication of this article. This work was supported by the Nature Science Foundation of China (82270958) and the Major Science and Technology Projects in Yunnan Province (202302AA310038).
Conflict of interest
The authors declare that the research was conducted in the absence of any commercial or financial relationships that could be construed as a potential conflict of interest.
Publisher’s note
All claims expressed in this article are solely those of the authors and do not necessarily represent those of their affiliated organizations, or those of the publisher, the editors and the reviewers. Any product that may be evaluated in this article, or claim that may be made by its manufacturer, is not guaranteed or endorsed by the publisher.
References
Abbass, M. M. S., El-Rashidy, A. A., Sadek, K. M., Moshy, S. E., Radwan, I. A., Rady, D., et al. (2020). Hydrogels and dentin-pulp complex regeneration: from the benchtop to clinical translation. Polymers-basel 12, 2935. doi:10.3390/polym12122935
Ahlfeld, T., Lode, A., Placht, A.-M., Fecht, T., Wolfram, T., Grom, S., et al. (2023). A comparative analysis of 3D printed scaffolds consisting of poly(lactic-co-glycolic) acid and different bioactive mineral fillers: aspects of degradation and cytocompatibility. Biomater. Sci. 11, 5590–5604. doi:10.1039/d2bm02071h
Anderson, M., Dubey, N., Bogie, K., Cao, C., Li, J., Lerchbacker, J., et al. (2022). Three-dimensional printing of clinical scale and personalized calcium phosphate scaffolds for alveolar bone reconstruction. Dent. Mater 38, 529–539. doi:10.1016/j.dental.2021.12.141
Anitua, E., Troya, M., and Zalduendo, M. (2018). Progress in the use of dental pulp stem cells in regenerative medicine. Cytotherapy 20, 479–498. doi:10.1016/j.jcyt.2017.12.011
Athirasala, A., Lins, F., Tahayeri, A., Hinds, M., Smith, A. J., Sedgley, C., et al. (2017). A novel strategy to engineer pre-vascularized full-length dental pulp-like tissue constructs. Sci. Rep. 7, 3323. doi:10.1038/s41598-017-02532-3
Athirasala, A., Tahayeri, A., Thrivikraman, G., França, C. M., Monteiro, N., Tran, V., et al. (2018). A dentin-derived hydrogel bioink for 3D bioprinting of cell laden scaffolds for regenerative dentistry. Biofabrication 10, 024101. doi:10.1088/1758-5090/aa9b4e
Baino, F., Novajra, G., and Vitale-Brovarone, C. (2015). Bioceramics and scaffolds: a winning combination for tissue engineering. Front. Bioeng. Biotechnol. 3, 202. doi:10.3389/fbioe.2015.00202
Blaudez, F., Ivanovski, S., and Vaquette, C. (2023). Harnessing the native extracellular matrix for periodontal regeneration using a melt electrowritten biphasic scaffold. J. Funct. Biomater. 14, 479. doi:10.3390/jfb14090479
Bow, A. J., Masi, T. J., and Dhar, M. S. (2021). Etched 3D-printed polycaprolactone constructs functionalized with reduced graphene oxide for enhanced attachment of dental pulp-derived stem cells. Pharmaceutics 13, 2146. doi:10.3390/pharmaceutics13122146
Buyuksungur, S., Hasirci, V., and Hasirci, N. (2021). 3D printed hybrid bone constructs of PCL and dental pulp stem cells loaded GelMA. J. Biomed. Mater Res. A 109, 2425–2437. doi:10.1002/jbm.a.37235
Calejo, I., Costa-Almeida, R., Reis, R. L., and Gomes, M. E. (2019). Enthesis tissue engineering: biological requirements meet at the interface. Tissue Eng. Part B Rev. 25, 330–356. doi:10.1089/ten.teb.2018.0383
Cao, S., Han, J., Sharma, N., Msallem, B., Jeong, W., Son, J., et al. (2020). In vitro mechanical and biological properties of 3D printed polymer composite and β-tricalcium phosphate scaffold on human dental pulp stem cells. Mater. (Basel) 13, 3057. doi:10.3390/ma13143057
Çelebi-Saltik, B., Babadag, S., Ballikaya, E., Pat, S., and Öteyaka, M. Ö. (2023). Osteogenic differentiation capacity of dental pulp stem cells on 3D printed polyurethane/boric acid scaffold. Biol. Trace Elem. Res. 23, 3781. doi:10.1007/s12011-023-03781-2
Chen, J., Gui, X., Qiu, T., Lv, Y., Fan, Y., Zhang, X., et al. (2023). DLP 3D printing of high-resolution root scaffold with bionic bioactivity and biomechanics for personalized bio-root regeneration. Biomater. Adv. 151, 213475. doi:10.1016/j.bioadv.2023.213475
Chen, M. Y.-H., Chen, K.-L., Chen, C.-A., Tayebaty, F., Rosenberg, P. A., and Lin, L. M. (2012). Responses of immature permanent teeth with infected necrotic pulp tissue and apical periodontitis/abscess to revascularization procedures. Int. Endod. J. 45, 294–305. doi:10.1111/j.1365-2591.2011.01978.x
Chen, R.-S., Hsu, S.-H., Chang, H.-H., and Chen, M.-H. (2021). Challenge tooth regeneration in adult dogs with dental pulp stem cells on 3D-printed hydroxyapatite/polylactic acid scaffolds. Cells-basel 10, 3277. doi:10.3390/cells10123277
Cho, H., Tarafder, S., Fogge, M., Kao, K., and Lee, C. H. (2016). Periodontal ligament stem/progenitor cells with protein-releasing scaffolds for cementum formation and integration on dentin surface. Connect. Tissue Res. 57, 488–495. doi:10.1080/03008207.2016.1191478
Choi, D., Qiu, M., Hwang, Y.-C., Oh, W.-M., Koh, J.-T., Park, C., et al. (2022). The effects of 3-dimensional bioprinting calcium silicate cement/methacrylated gelatin scaffold on the proliferation and differentiation of human dental pulp stem cells. Mater. (Basel) 15, 2170. doi:10.3390/ma15062170
Cooper, L. F. (2009). The current and future treatment of edentulism. J. Prosthodont 18, 116–122. doi:10.1111/j.1532-849X.2009.00441.x
Cunha, D., Souza, N., Moreira, M., Rodrigues, N., Silva, P., Franca, C., et al. (2023). 3D-printed microgels supplemented with dentin matrix molecules as a novel biomaterial for direct pulp capping. Clin. Oral Invest. 27, 1215–1225. doi:10.1007/s00784-022-04735-z
Daghrery, A., Ferreira, J. A., de Souza Araújo, I. J., Clarkson, B. H., Eckert, G. J., Bhaduri, S. B., et al. (2021). A highly ordered, nanostructured fluorinated CaP-coated melt electrowritten scaffold for periodontal tissue regeneration. Adv. Healthc. Mater 10, e2101152. doi:10.1002/adhm.202101152
Daghrery, A., Ferreira, J. A., Xu, J., Golafshan, N., Kaigler, D., Bhaduri, S. B., et al. (2023). Tissue-specific melt electrowritten polymeric scaffolds for coordinated regeneration of soft and hard periodontal tissues. Bioact. Mater 19, 268–281. doi:10.1016/j.bioactmat.2022.04.013
Danhier, F., Ansorena, E., Silva, J. M., Coco, R., Le Breton, A., and Préat, V. (2012). PLGA-based nanoparticles: an overview of biomedical applications. J. Control Release 161, 505–522. doi:10.1016/j.jconrel.2012.01.043
Duailibi, S. E., Duailibi, M. T., Vacanti, J. P., and Yelick, P. C. (2006). Prospects for tooth regeneration. Periodontol. 2000 41, 177–187. doi:10.1111/j.1600-0757.2006.00165.x
Duarte Campos, D. F., Zhang, S., Kreimendahl, F., Köpf, M., Fischer, H., Vogt, M., et al. (2020). Hand-held bioprinting for de novo vascular formation applicable to dental pulp regeneration. Connect. Tissue Res. 61, 205–215. doi:10.1080/03008207.2019.1640217
Ebadi, M., Miresmaeili, A., Rajabi, S., Shojaei, S., and Farhadi, S. (2023). Isolation and characterization of apical papilla cells from root end of human third molar and their differentiation into cementoblast cells: an in vitro study. Biol. Proced. Online 25, 2. doi:10.1186/s12575-023-00190-6
EzEldeen, M., Loos, J., Mousavi Nejad, Z., Cristaldi, M., Murgia, D., Braem, A., et al. (2021). 3D-printing-assisted fabrication of chitosan scaffolds from different sources and cross-linkers for dental tissue engineering. Eur. Cells Mater 41, 485–501. doi:10.22203/eCM.v041a31
Fahimipour, F., Dashtimoghadam, E., Rasoulianboroujeni, M., Yazdimamaghani, M., Khoshroo, K., Tahriri, M., et al. (2018). Collagenous matrix supported by a 3D-printed scaffold for osteogenic differentiation of dental pulp cells. Dent. Mater 34, 209–220. doi:10.1016/j.dental.2017.10.001
Feng, K.-C., Li, J., Wang, L., Chuang, Y.-C., Liu, H., Pinkas-Sarafova, A., et al. (2021). Combination of 3D printing and ALD for dentin fabrication from dental pulp stem cell culture. ACS Appl. Bio Mater 4, 7422–7430. doi:10.1021/acsabm.1c00577
Ferrúa, C. P., Centeno, E. G. Z., da Rosa, L. C., do Amaral, C. C., Severo, R. F., Sarkis-Onofre, R., et al. (2017). How has dental pulp stem cells isolation been conducted? A scoping review. Braz Oral Res. 31, e87. doi:10.1590/1807-3107BOR-2017.vol31.0087
Gao, G., Kim, B. S., Jang, J., and Cho, D.-W. (2019). Recent strategies in extrusion-based three-dimensional cell printing toward organ biofabrication. ACS Biomater. Sci. Eng. 5, 1150–1169. doi:10.1021/acsbiomaterials.8b00691
Gao, Q., Kim, B.-S., and Gao, G. (2021). Advanced strategies for 3D bioprinting of tissue and organ analogs using alginate hydrogel bioinks. Mar. Drugs 19, 708. doi:10.3390/md19120708
Golafshan, N., Castilho, M., Daghrery, A., Alehosseini, M., van de Kemp, T., Krikonis, K., et al. (2023). Composite graded melt electrowritten scaffolds for regeneration of the periodontal ligament-to-bone interface. ACS Appl. Mater Interfaces 15, 12735–12749. doi:10.1021/acsami.2c21256
Gronthos, S., Brahim, J., Li, W., Fisher, L. W., Cherman, N., Boyde, A., et al. (2002). Stem cell properties of human dental pulp stem cells. J. Dent. Res. 81, 531–535. doi:10.1177/154405910208100806
Gronthos, S., Mankani, M., Brahim, J., Robey, P. G., and Shi, S. (2000). Postnatal human dental pulp stem cells (DPSCs) in vitro and in vivo. Proc. Natl. Acad. Sci. U. S. A. 97, 13625–13630. doi:10.1073/pnas.240309797
Guo, W., Gong, K., Shi, H., Zhu, G., He, Y., Ding, B., et al. (2012). Dental follicle cells and treated dentin matrix scaffold for tissue engineering the tooth root. Biomaterials 33, 1291–1302. doi:10.1016/j.biomaterials.2011.09.068
Guo, W., He, Y., Zhang, X., Lu, W., Wang, C., Yu, H., et al. (2009). The use of dentin matrix scaffold and dental follicle cells for dentin regeneration. Biomaterials 30, 6708–6723. doi:10.1016/j.biomaterials.2009.08.034
Ha, M., Athirasala, A., Tahayeri, A., Menezes, P. P., and Bertassoni, L. E. (2020). Micropatterned hydrogels and cell alignment enhance the odontogenic potential of stem cells from apical papilla in-vitro. Dent. Mater 36, 88–96. doi:10.1016/j.dental.2019.10.013
Han, J., Jeong, W., Kim, M.-K., Nam, S.-H., Park, E.-K., and Kang, H.-W. (2021). Demineralized dentin matrix particle-based bio-ink for patient-specific shaped 3D dental tissue regeneration. Polymers-basel 13, 1294. doi:10.3390/polym13081294
Han, J., Kim, D. S., Jang, H., Kim, H.-R., and Kang, H.-W. (2019). Bioprinting of three-dimensional dentin-pulp complex with local differentiation of human dental pulp stem cells. J. Tissue Eng. 10, 204173141984584. doi:10.1177/2041731419845849
Heng, B. C., Zhu, S., Xu, J., Yuan, C., Gong, T., and Zhang, C. (2016). Effects of decellularized matrices derived from periodontal ligament stem cells and SHED on the adhesion, proliferation and osteogenic differentiation of human dental pulp stem cells in vitro. Tissue Cell 48, 133–143. doi:10.1016/j.tice.2015.12.004
Hilkens, P., Bronckaers, A., Ratajczak, J., Gervois, P., Wolfs, E., and Lambrichts, I. (2017). The angiogenic potential of DPSCs and SCAPs in an in vivo model of dental pulp regeneration. Stem Cells Int. 2017, 1–14. doi:10.1155/2017/2582080
Ho, C.-C., Fang, H.-Y., Wang, B., Huang, T.-H., and Shie, M.-Y. (2018). The effects of Biodentine/polycaprolactone three-dimensional-scaffold with odontogenesis properties on human dental pulp cells. Int. Endod. J. 51 (4), e291–e300. doi:10.1111/iej.12799
Huang, G. T.-J., Yamaza, T., Shea, L. D., Djouad, F., Kuhn, N. Z., Tuan, R. S., et al. (2010). Stem/progenitor cell-mediated de novo regeneration of dental pulp with newly deposited continuous layer of dentin in an in vivo model. Tissue Eng. Part A 16, 605–615. doi:10.1089/ten.TEA.2009.0518
Huang, K.-H., Chen, Y.-W., Wang, C.-Y., Lin, Y.-H., Wu, Y.-H. A., Shie, M.-Y., et al. (2018). Enhanced capability of bone morphogenetic protein 2-loaded mesoporous calcium silicate scaffolds to induce odontogenic differentiation of human dental pulp cells. J. Endodont 44, 1677–1685. doi:10.1016/j.joen.2018.08.008
Huang, Y., Zhang, Z., Bi, F., Tang, H., Chen, J., Huo, F., et al. (2023). Personalized 3D-printed scaffolds with multiple bioactivities for bioroot regeneration. Adv. Healthc. Mater 12, e2300625. doi:10.1002/adhm.202300625
Hung, K.-C., Tseng, C.-S., Dai, L.-G., and Hsu, S. (2016). Water-based polyurethane 3D printed scaffolds with controlled release function for customized cartilage tissue engineering. Biomaterials 83, 156–168. doi:10.1016/j.biomaterials.2016.01.019
Hurng, J. M., Kurylo, M. P., Marshall, G. W., Webb, S. M., Ryder, M. I., and Ho, S. P. (2011). Discontinuities in the human bone-PDL-cementum complex. Biomaterials 32, 7106–7117. doi:10.1016/j.biomaterials.2011.06.021
Ivanovski, S., Vaquette, C., Gronthos, S., Hutmacher, D. W., and Bartold, P. M. (2014). Multiphasic scaffolds for periodontal tissue engineering. J. Dent. Res. 93, 1212–1221. doi:10.1177/0022034514544301
Karring, T., Nyman, S., Gottlow, J., and Laurell, L. (1993). Development of the biological concept of guided tissue regeneration--animal and human studies. Periodontol. 2000 1, 26–35. doi:10.1111/j.1600-0757.1993.tb00204.x
Kim, D., Lee, H., Lee, G.-H., Hoang, T.-H., Kim, H.-R., and Kim, G. H. (2022). Fabrication of bone-derived decellularized extracellular matrix/ceramic-based biocomposites and their osteo/odontogenic differentiation ability for dentin regeneration. Bioeng. Transl. Med. 7, e10317. doi:10.1002/btm2.10317
Kim, E.-J., Kim, K.-H., Kim, H.-Y., Lee, D.-J., Li, S., Ngoc Han, M., et al. (2023). Harnessing the dental cells derived from human induced pluripotent stem cells for hard tissue engineering. J. Adv. Res. S2090-1232 (23), 00228. doi:10.1016/j.jare.2023.08.012
Kim, K., Lee, C. H., Kim, B. K., and Mao, J. J. (2010). Anatomically shaped tooth and periodontal regeneration by cell homing. J. Dent. Res. 89, 842–847. doi:10.1177/0022034510370803
Kim, M. G., and Park, C. H. (2020). The topographical optimization of 3D microgroove pattern intervals for ligamentous cell orientations: in vitro. Int. J. Mol. Sci. 21, 9358. doi:10.3390/ijms21249358
Klotz, B. J., Gawlitta, D., Rosenberg, A. J. W. P., Malda, J., and Melchels, F. P. W. (2016). Gelatin-methacryloyl hydrogels: towards biofabrication-based tissue repair. Trends Biotechnol. 34, 394–407. doi:10.1016/j.tibtech.2016.01.002
Kwon, T., Lamster, I. B., and Levin, L. (2021). Current concepts in the management of periodontitis. Int. Dent. J. 71, 462–476. doi:10.1111/idj.12630
Lazaridou, M., Bikiaris, D. N., and Lamprou, D. A. (2022). 3D bioprinted chitosan-based hydrogel scaffolds in tissue engineering and localised drug delivery. Pharmaceutics 14, 1978. doi:10.3390/pharmaceutics14091978
Lee, C. H., Hajibandeh, J., Suzuki, T., Fan, A., Shang, P., and Mao, J. J. (2014). Three-dimensional printed multiphase scaffolds for regeneration of periodontium complex. Tissue Eng. Part A 20, 1342–1351. doi:10.1089/ten.TEA.2013.0386
Lee, J. M., Suen, S. K. Q., Ng, W. L., Ma, W. C., and Yeong, W. Y. (2021a). Bioprinting of collagen: considerations, potentials, and applications. Macromol. Biosci. 21, e2000280. doi:10.1002/mabi.202000280
Lee, U.-L., Yun, S., Cao, H.-L., Ahn, G., Shim, J.-H., Woo, S.-H., et al. (2021b). Bioprinting on 3D printed titanium scaffolds for periodontal ligament regeneration. Cells-basel 10, 1337. doi:10.3390/cells10061337
Li, C., Xu, X., Gao, J., Zhang, X., Chen, Y., Li, R., et al. (2022). 3D printed scaffold for repairing bone defects in apical periodontitis. BMC Oral Health 22, 327. doi:10.1186/s12903-022-02362-4
Li, R., Guo, W., Yang, B., Guo, L., Sheng, L., Chen, G., et al. (2011). Human treated dentin matrix as a natural scaffold for complete human dentin tissue regeneration. Biomaterials 32, 4525–4538. doi:10.1016/j.biomaterials.2011.03.008
Li, X., Liu, B., Pei, B., Chen, J., Zhou, D., Peng, J., et al. (2020a). Inkjet bioprinting of biomaterials. Chem. Rev. 120, 10793–10833. doi:10.1021/acs.chemrev.0c00008
Li, Y., Chi, Y.-Q., Yu, C.-H., Xie, Y., Xia, M.-Y., Zhang, C.-L., et al. (2020b). Drug-free and non-crosslinked chitosan scaffolds with efficient antibacterial activity against both Gram-negative and Gram-positive bacteria. Carbohydr. Polym. 241, 116386. doi:10.1016/j.carbpol.2020.116386
Li, Y., Na, R., Wang, X., Liu, H., Zhao, L., Sun, X., et al. (2017). Fabrication of antimicrobial peptide-loaded PLGA/chitosan composite microspheres for long-acting bacterial resistance. Molecules 22, 1637. doi:10.3390/molecules22101637
Lin, H.-H., Chao, P.-H. G., Tai, W.-C., and Chang, P.-C. (2021a). 3D-Printed collagen-based waveform microfibrous scaffold for periodontal ligament reconstruction. Int. J. Mol. Sci. 22, 7725. doi:10.3390/ijms22147725
Lin, Y.-T., Hsu, T.-T., Liu, Y.-W., Kao, C.-T., and Huang, T.-H. (2021b). Bidirectional differentiation of human-derived stem cells induced by biomimetic calcium silicate-reinforced gelatin methacrylate bioink for odontogenic regeneration. Biomedicines 9, 929. doi:10.3390/biomedicines9080929
Liu, F., Li, W., Liu, H., Yuan, T., Yang, Y., Zhou, W., et al. (2021). Preparation of 3D printed chitosan/polyvinyl alcohol double network hydrogel scaffolds. Macromol. Biosci. 21, e2000398. doi:10.1002/mabi.202000398
Liu, H., Wang, C., Sun, X., Zhan, C., Li, Z., Qiu, L., et al. (2022a). Silk fibroin/collagen/hydroxyapatite scaffolds obtained by 3D printing technology and loaded with recombinant human erythropoietin in the reconstruction of alveolar bone defects. ACS Biomater. Sci. Eng. 8, 5245–5256. doi:10.1021/acsbiomaterials.2c00690
Liu, N., Zhou, M., Zhang, Q., Zhang, T., Tian, T., Ma, Q., et al. (2018). Stiffness regulates the proliferation and osteogenic/odontogenic differentiation of human dental pulp stem cells via the WNT signalling pathway. Cell Prolif. 51, e12435. doi:10.1111/cpr.12435
Liu, P., Li, Q., Yang, Q., Zhang, S., Yi, K., Zhang, G., et al. (2023). Evaluation of the effect of 3D-bioprinted gingival fibroblast-encapsulated ADM scaffolds on keratinized gingival augmentation. J. Periodontal Res. 58, 564–574. doi:10.1111/jre.13126
Liu, P., Zhang, Y., Ma, Y., Tan, S., Ren, B., Liu, S., et al. (2022b). Application of dental pulp stem cells in oral maxillofacial tissue engineering. Int. J. Med. Sci. 19, 310–320. doi:10.7150/ijms.68494
Luo, D., Chen, B., and Chen, Y. (2022). Stem cells-loaded 3D-printed scaffolds for the reconstruction of alveolar cleft. Front. Bioeng. Biotechnol. 10, 939199. doi:10.3389/fbioe.2022.939199
Ma, Y., Ji, Y., Zhong, T., Wan, W., Yang, Q., Li, A., et al. (2017). Bioprinting-based PDLSC-ECM screening for in vivo repair of alveolar bone defect using cell-laden, injectable and photocrosslinkable hydrogels. ACS Biomater. Sci. Eng. 3, 3534–3545. doi:10.1021/acsbiomaterials.7b00601
Ma, Y., Xie, L., Yang, B., and Tian, W. (2019). Three-dimensional printing biotechnology for the regeneration of the tooth and tooth-supporting tissues. Biotechnol. Bioeng. 116, 452–468. doi:10.1002/bit.26882
Ma, Y., Yang, X., Chen, Y., Zhang, J., Gai, K., Chen, J., et al. (2023). Biomimetic peridontium patches for functional periodontal regeneration. Adv. Healthc. Mater 12, e2202169. doi:10.1002/adhm.202202169
Mahdavi-Jouibari, F., Parseh, B., Kazeminejad, E., and Khosravi, A. (2023). Hopes and opportunities of stem cells from human exfoliated deciduous teeth (SHED) in cartilage tissue regeneration. Front. Bioeng. Biotechnol. 11, 1021024. doi:10.3389/fbioe.2023.1021024
Mei, N., Wu, Y., Chen, B., Zhuang, T., Yu, X., Sui, B., et al. (2022). 3D-printed mesoporous bioactive glass/GelMA biomimetic scaffolds for osteogenic/cementogenic differentiation of periodontal ligament cells. Front. Bioeng. Biotech. 10, 950970. doi:10.3389/fbioe.2022.950970
Meng, Z., Liu, J., Feng, Z., Guo, S., Wang, M., Wang, Z., et al. (2022). N-acetylcysteine regulates dental follicle stem cell osteogenesis and alveolar bone repair via ROS scavenging. Stem Cell Res. Ther. 13, 466. doi:10.1186/s13287-022-03161-y
Miao, G., Liang, L., Li, W., Ma, C., Pan, Y., Zhao, H., et al. (2023). 3D bioprinting of a bioactive composite scaffold for cell delivery in periodontal tissue regeneration. Biomolecules 13, 1062. doi:10.3390/biom13071062
Miura, M., Gronthos, S., Zhao, M., Lu, B., Fisher, L. W., Robey, P. G., et al. (2003). SHED: stem cells from human exfoliated deciduous teeth. P Natl. Acad. Sci. U. S. A. 100, 5807–5812. doi:10.1073/pnas.0937635100
Mohabatpour, F., Duan, X., Yazdanpanah, Z., Tabil, X. L., Lobanova, L., Zhu, N., et al. (2022). Bioprinting of alginate-carboxymethyl chitosan scaffolds for enamel tissue engineeringin vitro. Biofabrication 15, 015022. doi:10.1088/1758-5090/acab35
Morsczeck, C., Götz, W., Schierholz, J., Zeilhofer, F., Kühn, U., Möhl, C., et al. (2005). Isolation of precursor cells (PCs) from human dental follicle of wisdom teeth. Matrix Biol. 24, 155–165. doi:10.1016/j.matbio.2004.12.004
Mota, C., Camarero-Espinosa, S., Baker, M. B., Wieringa, P., and Moroni, L. (2020). Bioprinting: from tissue and organ development to in vitro models. Chem. Rev. 120, 10547–10607. doi:10.1021/acs.chemrev.9b00789
Murphy, S. V., and Atala, A. (2014). 3D bioprinting of tissues and organs. Nat. Biotechnol. 32, 773–785. doi:10.1038/nbt.2958
Nakamura, S., Yamada, Y., Katagiri, W., Sugito, T., Ito, K., and Ueda, M. (2009). Stem cell proliferation pathways comparison between human exfoliated deciduous teeth and dental pulp stem cells by gene expression profile from promising dental pulp. J. Endod. 35, 1536–1542. doi:10.1016/j.joen.2009.07.024
Nanci A. (2013). “Chapter 7 - enamel: composition, formation, and structure,” Ten cate’s oral histology. eighth edition (St. Louis (MO): Mosby), 122–164. doi:10.1016/B978-0-323-07846-7.00007-0
Nejad, Z. M., Zamanian, A., Saeidifar, M., Vanaei, H. R., and Amoli, M. S. (2021). 3D bioprinting of polycaprolactone-based scaffolds for pulp-dentin regeneration: investigation of physicochemical and biological behavior. Polymers 13, 4442. doi:10.3390/polym13244442
Nowwarote, N., Petit, S., Ferre, F. C., Dingli, F., Laigle, V., Loew, D., et al. (2021). Extracellular matrix derived from dental pulp stem cells promotes mineralization. Front. Bioeng. Biotechnol. 9, 740712. doi:10.3389/fbioe.2021.740712
Ono, M., Oshima, M., Ogawa, M., Sonoyama, W., Hara, E. S., Oida, Y., et al. (2017). Practical whole-tooth restoration utilizing autologous bioengineered tooth germ transplantation in a postnatal canine model. Sci. Rep. 7, 44522. doi:10.1038/srep44522
Park, C. H., Rios, H. F., Jin, Q., Sugai, J. V., Padial-Molina, M., Taut, A. D., et al. (2012). Tissue engineering bone-ligament complexes using fiber-guiding scaffolds. Biomaterials 33, 137–145. doi:10.1016/j.biomaterials.2011.09.057
Park, J., Park, S., Kim, J. E., Jang, K.-J., Seonwoo, H., and Chung, J. H. (2021). Enhanced osteogenic differentiation of periodontal ligament stem cells using a graphene oxide-coated poly(ε-caprolactone) scaffold. Polym. (Basel) 13, 797. doi:10.3390/polym13050797
Park, J. H., Gillispie, G. J., Copus, J. S., Zhang, W., Atala, A., Yoo, J. J., et al. (2020). The effect of BMP-mimetic peptide tethering bioinks on the differentiation of dental pulp stem cells (DPSCs) in 3D bioprinted dental constructs. Biofabrication 12, 035029. doi:10.1088/1758-5090/ab9492
Pereira, L. V., Bento, R. F., Cruz, D. B., Marchi, C., Salomone, R., Oiticicca, J., et al. (2019). Stem cells from human exfoliated deciduous teeth (SHED) differentiate in vivo and promote facial nerve regeneration. Cell Transpl. 28, 55–64. doi:10.1177/0963689718809090
Pilipchuk, S. P., Monje, A., Jiao, Y., Hao, J., Kruger, L., Flanagan, C. L., et al. (2016). Integration of 3D printed and micropatterned polycaprolactone scaffolds for guidance of oriented collagenous tissue formation in vivo. Adv. Healthc. Mater 5, 676–687. doi:10.1002/adhm.201500758
Place, E. S., George, J. H., Williams, C. K., and Stevens, M. M. (2009). Synthetic polymer scaffolds for tissue engineering. Chem. Soc. Rev. 38, 1139–1151. doi:10.1039/b811392k
Qian, Y., Gong, J., Lu, K., Hong, Y., Zhu, Z., Zhang, J., et al. (2023). DLP printed hDPSC-loaded GelMA microsphere regenerates dental pulp and repairs spinal cord. Biomaterials 299, 122137. doi:10.1016/j.biomaterials.2023.122137
Qin, H., Wei, Y., Han, J., Jiang, X., Yang, X., Wu, Y., et al. (2022). 3D printed bioceramic scaffolds: adjusting pore dimension is beneficial for mandibular bone defects repair. J. Tissue Eng. Regen. M. 16, 409–421. doi:10.1002/term.3287
Quan, H., Zhang, T., Xu, H., Luo, S., Nie, J., and Zhu, X. (2020). Photo-curing 3D printing technique and its challenges. Bioact. Mater 5, 110–115. doi:10.1016/j.bioactmat.2019.12.003
Ramot, Y., Haim-Zada, M., Domb, A. J., and Nyska, A. (2016). Biocompatibility and safety of PLA and its copolymers. Adv. Drug Deliv. Rev. 107, 153–162. doi:10.1016/j.addr.2016.03.012
Rastogi, P., and Kandasubramanian, B. (2019). Review of alginate-based hydrogel bioprinting for application in tissue engineering. Biofabrication 11, 042001. doi:10.1088/1758-5090/ab331e
Raveau, S., and Jordana, F. (2020). Tissue engineering and three-dimensional printing in periodontal regeneration: a literature review. J. Clin. Med. 9, 4008. doi:10.3390/jcm9124008
Ricketts, D. (2001). Management of the deep carious lesion and the vital pulp dentine complex. Br. Dent. J. 191, 606–610. doi:10.1038/sj.bdj.4801246
Sakr, M. A., Sakthivel, K., Hossain, T., Shin, S. R., Siddiqua, S., Kim, J., et al. (2022). Recent trends in gelatin methacryloyl nanocomposite hydrogels for tissue engineering. J. Biomed. Mater Res. A 110, 708–724. doi:10.1002/jbm.a.37310
Salar Amoli, M., Anand, R., EzEldeen, M., Amorim, P. A., Geris, L., Jacobs, R., et al. (2022). The development of a 3D printable chitosan-based copolymer with tunable properties for dentoalveolar regeneration. Carbohyd Polym. 289, 119441. doi:10.1016/j.carbpol.2022.119441
Sallum, E. A., Ribeiro, F. V., Ruiz, K. S., and Sallum, A. W. (2019). Experimental and clinical studies on regenerative periodontal therapy. Periodontol. 2000 79, 22–55. doi:10.1111/prd.12246
Seo, B.-M., Miura, M., Gronthos, S., Bartold, P. M., Batouli, S., Brahim, J., et al. (2004). Investigation of multipotent postnatal stem cells from human periodontal ligament. Lancet 364, 149–155. doi:10.1016/S0140-6736(04)16627-0
Shao, H., Sun, M., Zhang, F., Liu, A., He, Y., Fu, J., et al. (2018). Custom repair of mandibular bone defects with 3D printed bioceramic scaffolds. J. Dent. Res. 97, 68–76. doi:10.1177/0022034517734846
Siddiqui, Z., Acevedo-Jake, A. M., Griffith, A., Kadincesme, N., Dabek, K., Hindi, D., et al. (2022). Cells and material-based strategies for regenerative endodontics. Bioact. Mater 14, 234–249. doi:10.1016/j.bioactmat.2021.11.015
Smith, J. G., Smith, A. J., Shelton, R. M., and Cooper, P. R. (2012). Recruitment of dental pulp cells by dentine and pulp extracellular matrix components. Exp. Cell Res. 318, 2397–2406. doi:10.1016/j.yexcr.2012.07.008
Sonoyama, W., Liu, Y., Fang, D., Yamaza, T., Seo, B.-M., Zhang, C., et al. (2006). Mesenchymal stem cell-mediated functional tooth regeneration in swine. PLoS One 1, e79. doi:10.1371/journal.pone.0000079
Suo, L., Wu, H., Wang, P., Xue, Z., Gao, J., and Shen, J. (2023). The improvement of periodontal tissue regeneration using a 3D-printed carbon nanotube/chitosan/sodium alginate composite scaffold. J. Biomed. Mater Res. B Appl. Biomater. 111, 73–84. doi:10.1002/jbm.b.35133
Szulc, M., and Lewandowska, K. (2022). Biomaterials based on chitosan and its derivatives and their potential in tissue engineering and other biomedical applications-A review. Molecules 28, 247. doi:10.3390/molecules28010247
Tan, Q., Cao, Y., Zheng, X., Peng, M., Huang, E., and Wang, J. (2021). BMP4-regulated human dental pulp stromal cells promote pulp-like tissue regeneration in a decellularized dental pulp matrix scaffold. Odontology 109, 895–903. doi:10.1007/s10266-021-00620-5
Tang, H., Bi, F., Chen, G., Zhang, S., Huang, Y., Chen, J., et al. (2022). 3D-bioprinted recombination structure of Hertwig’s epithelial root sheath cells and dental papilla cells for alveolar bone regeneration. Int. J. Bioprinting 8, 512. doi:10.18063/ijb.v8i3.512
Tian, Y., Liu, M., Liu, Y., Shi, C., Wang, Y., Liu, T., et al. (2021). The performance of 3D bioscaffolding based on a human periodontal ligament stem cell printing technique. J. Biomed. Mater Res. A 109, 1209–1219. doi:10.1002/jbm.a.37114
Vu, A. A., Burke, D. A., Bandyopadhyay, A., and Bose, S. (2021). Effects of surface area and topography on 3D printed tricalcium phosphate scaffolds for bone grafting applications. Addit. Manuf. 39, 101870. doi:10.1016/j.addma.2021.101870
Wang, C.-Y., Chiu, Y.-C., Lee, A. K.-X., Lin, Y.-A., Lin, P.-Y., and Shie, M.-Y. (2021). Biofabrication of gingival fibroblast cell-laden collagen/strontium-doped calcium silicate 3D-printed Bi-layered scaffold for osteoporotic periodontal regeneration. Biomedicines 9, 431. doi:10.3390/biomedicines9040431
Wang, W., Zhu, Y., Li, J., Geng, T., Jia, J., Wang, X., et al. (2023a). Bioprinting EphrinB2-modified dental pulp stem cells with enhanced osteogenic capacity for alveolar bone engineering. Tissue Eng. Part A 29, 244–255. doi:10.1089/ten.TEA.2022.0180
Wang, X., Sha, X.-J., Li, G.-H., Yang, F.-S., Ji, K., Wen, L.-Y., et al. (2012). Comparative characterization of stem cells from human exfoliated deciduous teeth and dental pulp stem cells. Arch. Oral Biol. 57, 1231–1240. doi:10.1016/j.archoralbio.2012.02.014
Wang, Y., Cui, H., Esworthy, T., Mei, D., Wang, Y., and Zhang, L. G. (2022). Emerging 4D printing strategies for next-generation tissue regeneration and medical devices. Adv. Mater 34, e2109198. doi:10.1002/adma.202109198
Wang, Y., Wang, Z., and Dong, Y. (2023b). Collagen-based biomaterials for tissue engineering. ACS Biomater. Sci. Eng. 9, 1132–1150. doi:10.1021/acsbiomaterials.2c00730
Wei, F., Song, T., Ding, G., Xu, J., Liu, Y., Liu, D., et al. (2013). Functional tooth restoration by allogeneic mesenchymal stem cell-based bio-root regeneration in swine. Stem Cells Dev. 22, 1752–1762. doi:10.1089/scd.2012.0688
Wei, X., Ling, J., Wu, L., Liu, L., and Xiao, Y. (2007). Expression of mineralization markers in dental pulp cells. J. Endod. 33, 703–708. doi:10.1016/j.joen.2007.02.009
Whitaker, R., Hernaez-Estrada, B., Hernandez, R. M., Santos-Vizcaino, E., and Spiller, K. L. (2021). Immunomodulatory biomaterials for tissue repair. Chem. Rev. 121, 11305–11335. doi:10.1021/acs.chemrev.0c00895
Wu, D. T., Munguia-Lopez, J. G., Cho, Y. W., Ma, X., Song, V., Zhu, Z., et al. (2021). Polymeric scaffolds for dental, oral, and craniofacial regenerative medicine. Molecules 26, 7043. doi:10.3390/molecules26227043
Wu, Y., Azmi, D. F. B., Rosa, V., Fawzy, A. S., Fuh, J. Y. H., Wong, Y. S., et al. (2016). Fabrication of dentin-like scaffolds through combined 3D printing and bio-mineralisation. Cogent Eng. 3, 1222777. doi:10.1080/23311916.2016.1222777
Yamada, Y., Fujimoto, A., Ito, A., Yoshimi, R., and Ueda, M. (2006). Cluster analysis and gene expression profiles: a cDNA microarray system-based comparison between human dental pulp stem cells (hDPSCs) and human mesenchymal stem cells (hMSCs) for tissue engineering cell therapy. Biomaterials 27, 3766–3781. doi:10.1016/j.biomaterials.2006.02.009
Yang, H., Li, J., Hu, Y., Sun, J., Guo, W., Li, H., et al. (2019a). Treated dentin matrix particles combined with dental follicle cell sheet stimulate periodontal regeneration. Dent. Mater 35, 1238–1253. doi:10.1016/j.dental.2019.05.016
Yang, M., Guo, Z., Li, T., Li, J., Chen, L., Wang, J., et al. (2019b). Synergetic effect of chemical and topological signals of gingival regeneration scaffold on the behavior of human gingival fibroblasts. J. Biomed. Mater Res. A 107, 1875–1885. doi:10.1002/jbm.a.36708
Yang, X., Ma, Y., Guo, W., Yang, B., and Tian, W. (2019c). Stem cells from human exfoliated deciduous teeth as an alternative cell source in bio-root regeneration. Theranostics 9, 2694–2711. doi:10.7150/thno.31801
Yang, X., Ma, Y., Wang, X., Yuan, S., Huo, F., Yi, G., et al. (2023). A 3D-bioprinted functional module based on decellularized extracellular matrix bioink for periodontal regeneration. Adv. Sci. (Weinh) 10, e2205041. doi:10.1002/advs.202205041
Yao, Y., Raymond, J. E., Kauffmann, F., Maekawa, S., Sugai, J. V., Lahann, J., et al. (2022). Multicompartmental scaffolds for coordinated periodontal tissue engineering. J. Dent. Res. 101, 1457–1466. doi:10.1177/00220345221099823
Yeh, C.-L., Bhorade, R., Hsu, T.-T., Chen, C.-Y., and Lin, C.-P. (2022). Mechanical assessment and odontogenic behavior of a 3D-printed mesoporous calcium silicate/calcium sulfate/poly-ε-caprolactone composite scaffold. J. Formos. Med. Assoc. 121, 510–518. doi:10.1016/j.jfma.2021.06.025
Yi, K., Li, Q., Lian, X., Wang, Y., and Tang, Z. (2022). Utilizing 3D bioprinted platelet-rich fibrin-based materials to promote the regeneration of oral soft tissue. Regen. Biomater. 9, rbac021. doi:10.1093/rb/rbac021
Yildirim, S., Fu, S. Y., Kim, K., Zhou, H., Lee, C. H., Li, A., et al. (2011). Tooth regeneration: a revolution in stomatology and evolution in regenerative medicine. Int. J. Oral Sci. 3, 107–116. doi:10.4248/IJOS11042
Yu, H., Zhang, X., Song, W., Pan, T., Wang, H., Ning, T., et al. (2019). Effects of 3-dimensional bioprinting alginate/gelatin hydrogel scaffold extract on proliferation and differentiation of human dental pulp stem cells. J. Endodont 45, 706–715. doi:10.1016/j.joen.2019.03.004
Yue, K., Trujillo-de Santiago, G., Alvarez, M. M., Tamayol, A., Annabi, N., and Khademhosseini, A. (2015). Synthesis, properties, and biomedical applications of gelatin methacryloyl (GelMA) hydrogels. Biomaterials 73, 254–271. doi:10.1016/j.biomaterials.2015.08.045
Zhang, J., Ding, H., Liu, X., Sheng, Y., Liu, X., and Jiang, C. (2019). Dental follicle stem cells: tissue engineering and immunomodulation. Stem Cells Dev. 28, 986–994. doi:10.1089/scd.2019.0012
Zhang, W., and Yelick, P. C. (2021). Tooth repair and regeneration: potential of dental stem cells. Trends Mol. Med. 27, 501–511. doi:10.1016/j.molmed.2021.02.005
Zhang, X., Chen, X., Hong, H., Hu, R., Liu, J., and Liu, C. (2022). Decellularized extracellular matrix scaffolds: recent trends and emerging strategies in tissue engineering. Bioact. Mater 10, 15–31. doi:10.1016/j.bioactmat.2021.09.014
Zhang, Y.-R., Du, W., Zhou, X.-D., and Yu, H.-Y. (2014). Review of research on the mechanical properties of the human tooth. Int. J. Oral Sci. 6, 61–69. doi:10.1038/ijos.2014.21
Zhao, M., Yang, D., Fan, S., Yao, X., Wang, J., Zhu, M., et al. (2022). 3D-Printed strong dental crown with multi-scale ordered architecture, high-precision, and bioactivity. Adv. Sci. (Weinh) 9, e2104001. doi:10.1002/advs.202104001
Zhou, F., Hong, Y., Liang, R., Zhang, X., Liao, Y., Jiang, D., et al. (2020). Rapid printing of bio-inspired 3D tissue constructs for skin regeneration. Biomaterials 258, 120287. doi:10.1016/j.biomaterials.2020.120287
Zhou, Z., Yao, Q., Li, L., Zhang, X., Wei, B., Yuan, L., et al. (2018). Antimicrobial activity of 3D-printed poly(ε-caprolactone) (PCL) composite scaffolds presenting vancomycin-loaded polylactic acid-glycolic acid (PLGA) microspheres. Med. Sci. Monit. 24, 6934–6945. doi:10.12659/MSM.911770
Keywords: 3D printing, regenerative dentistry, bioprinting, biomaterial, dentin-pulp complex, periodontal regeneration, tissue engineering
Citation: Zhao F, Zhang Z and Guo W (2024) The 3-dimensional printing for dental tissue regeneration: the state of the art and future challenges. Front. Bioeng. Biotechnol. 12:1356580. doi: 10.3389/fbioe.2024.1356580
Received: 15 December 2023; Accepted: 06 February 2024;
Published: 22 February 2024.
Edited by:
Mona Kamal Marei, Alexandria University, EgyptReviewed by:
Pravin D. Potdar, Consultant, Mumbai, IndiaJingang Xiao, Southwest Medical University, China
Copyright © 2024 Zhao, Zhang and Guo. This is an open-access article distributed under the terms of the Creative Commons Attribution License (CC BY). The use, distribution or reproduction in other forums is permitted, provided the original author(s) and the copyright owner(s) are credited and that the original publication in this journal is cited, in accordance with accepted academic practice. No use, distribution or reproduction is permitted which does not comply with these terms.
*Correspondence: Weihua Guo, guoweihua943019@163.com