Additive manufacturing technologies in the oral implant clinic: A review of current applications and progress
- State Key Laboratory of Military Stomatology, National Clinical Research Center for Oral Diseases, Shaanxi Engineering Research Center for Dental Materials and Advanced Manufacture, Department of Oral Implants, School of Stomatology, The Fourth Military Medical University, Xi’an, Shaanxi, China
Additive manufacturing (AM) technologies can enable the direct fabrication of customized physical objects with complex shapes, based on computer-aided design models. This technology is changing the digital manufacturing industry and has become a subject of considerable interest in digital implant dentistry. Personalized dentistry implant treatments for individual patients can be achieved through Additive manufacturing. Herein, we review the applications of Additive manufacturing technologies in oral implantology, including implant surgery, and implant and restoration products, such as surgical guides for implantation, custom titanium meshes for bone augmentation, personalized or non-personalized dental implants, custom trays, implant casts, and implant-support frameworks, among others. In addition, this review also focuses on Additive manufacturing technologies commonly used in oral implantology. Stereolithography, digital light processing, and fused deposition modeling are often used to construct surgical guides and implant casts, whereas direct metal laser sintering, selective laser melting, and electron beam melting can be applied to fabricate dental implants, personalized titanium meshes, and denture frameworks. Moreover, it is sometimes required to combine Additive manufacturing technology with milling and other cutting and finishing techniques to ensure that the product is suitable for its final application.
1 Introduction
In recent years, digital technologies, such as computer-aided design/computer-aided manufacture (CAD/CAM), digital intraoral scanners, and additive manufacturing (AM) technologies, have been successfully applied in implant dentistry (Dawood et al., 2015), providing new clinical and technical methods for surgical oral implant operations and restoration manufacture. Digitalization shows a steady development trend in the field of dentistry. As a typical digital manufacturing technology, AM can connect disease diagnosis, treatment planning, and production processes through data flow, forming a fully digital process for dental product processing (Salmi, 2021). Digital workflows not only greatly improve the safety of implant placement and the convenience of manufacturing restoration, but also reduce labor intensity for dentists and provide a satisfactory medical experience for patients (Barazanchi et al., 2017).
Additive manufacturing (AM) technologies, also known as three-dimensional (3D) printing technologies, are rapid prototyping technologies that have gradually become alternative methods for generating products from CAD files in dentistry (Abduo et al., 2014). Previously, numerical control processing technology (NC technology, also known as subtractive machining technology) was typically used. In the industry, NC refers to the use of turning, milling, grinding, and other approaches to remove material from a specific solid object to form a desired shape. As the processed objects in stomatology NC machining are specific oral materials, milling and grinding techniques are often adopted according to the characteristics of the material used and precision manufacturing requirements (Abduo et al., 2014). NC is a mature processing technology that achieves high precision using a wide range of materials and can be used to directly process almost all commonly used stomatological materials. In addition, NC is the first choice for batch fabrication (Petrick and Simpson, 2015); however, the disadvantages of NC include the material waste associated with this technology, which leads to high production costs. Moreover, particularly complex dental auxiliary therapy devices (such as root-analogue implants, personalized titanium meshes, etc.) are difficult to achieve using NC (Dawood et al., 2015).
AM is a processing technology based on discrete stacking forms. The underlying principle involves transformation of a 3D digital model into continuous superposition of a two-dimensional sheet model through a discrete process, where a computer program controls the stacking of materials in order, layer by layer. The most remarkable features of AM are that it overcomes the limitations of subtractive machining technology and can be used to mass produce a variety of products with complex morphology in a short time. In addition, in principle, AM consumes a minimum amount of raw materials, and unformed raw materials (resin liquid, metal powder, etc.) can be reused, which greatly reduces costs (Kessler et al., 2020; Tian et al., 2021).
Overall, these two manufacturing methods (NC and AM) each have advantages and disadvantages, and AM is not conclusively superior to subtractive manufacturing; in most cases, the techniques present complementary advantages (Alammar et al., 2022). In implant dentistry, applications of and research into AM are becoming increasingly extensive, ranging from fabrication of surgical guides and dental implants to dental casts and implant frameworks, among other items. The use of AM has led to a progression of implant dentistry applications from traditional, purely empirical methods to more accurate digital medicine (Schweiger et al., 2021; Huang et al., 2022).
Several reviews on the applications of AM in dentistry have been published (Barazanchi et al., 2017; Revilla-Leon and Ozcan, 2019; Tian et al., 2021); however, few have focused on the application of this technology in oral implantology. Moreover, the materials reviewed have been limited to polymers, and the types of applications covered were not comprehensive (Revilla-León et al., 2020). In this review, we discuss the AM technologies commonly used in oral implantology and their applications. In addition, we compare the accuracy of different AM technologies and describe the clinical applications of products fabricated by AM.
The aim of this review is to provide readers with information on recent progress in the application of AM in oral implantology. As a result, we focus on the following questions: Which AM technologies are commonly used in implant dentistry? What are the applications of AM technologies in implant dentistry? What are the benefits of the application of AM technologies in implant dentistry?
2 Additive manufacturing technologies commonly used in implant dentistry
AM refers to a class of manufacturing processes in which parts are built by stacking layers of material on one another. There are seven 3D printing categories in the American Society for Testing and Materials classification standard: vat photopolymerization (VPP), powder bed fusion (PBF), material jetting, binder jetting, material extrusion (MEX), sheet lamination, and directed energy deposition. Although there are various AM processes, not all of them are used in implant dentistry. Technologies frequently adopted in implant dentistry practice include VPP [stereolithography (SLA), digital light processing (DLP), etc.], PBF [selective laser melting (SLM), selective laser sintering (SLS), etc.], and MEX (fused deposition modeling, etc.) (Schweiger et al., 2021). Parts are built directly from digital 3D models created using CAD software. CAD models are converted into many thin layers, and the fabrication facility uses this geometric data to build each layer in turn until the part is completed (Bozkurt and Karayel, 2021). Given this approach, AM is often referred to as layered manufacturing, direct digital manufacturing, or physical free-form manufacturing (Turkyilmaz and Wilkins, 2021).
Each 3D printing technology is based on the principle of the “additive” method, with the main differences among them being the molding methods and materials used. Appropriate 3D printing technologies should be selected according to the application purpose (Figure 1) (Jawahar and Maragathavalli, 2019; Schweiger et al., 2021).
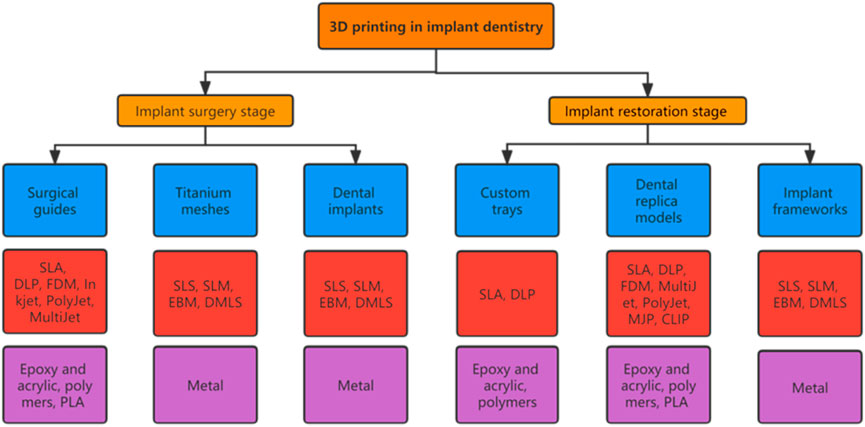
FIGURE 1. Applications of 3D printing technologies commonly used in implant dentistry. Chart showing the applications (blue boxes), primary 3D printing technologies (red boxes), and materials needed (purple boxes) (Dawood et al., 2015; Khorsandi et al., 2021; Tian et al., 2021).
2.1 Vat photopolymerization
2.1.1 Stereolithography
SLA is a well-known 3D printing technology that has been used for almost 30 years (Turkyilmaz and Wilkins, 2021), and is primarily applied to print surgical guides, dental model replicas, custom trays, and provisional restorations (Anadioti et al., 2018; Khorsandi et al., 2021; Schweiger et al., 2021). SLA uses a high-intensity ultraviolet light source, which applies the wavelength and heat of light to polymerize and selectively solidify liquid resin for lamination. In this process, to better integrate new layers with previous layers, the polymerization reaction of each layer is usually not fully completed under the direct light source, but further light processing is rather performed after the printing is completed (Pagac et al., 2021). Finally, the support structures added automatically by the printer require manual removal (Revilla-León et al., 2020).
2.1.2 Digital light processing
DLP, as a VPP technology, has attracted wide attention. A major area of DLP printer application is the digital manufacture of dental models (Park et al., 2021). Differences between DLP and SLA include the type of light source and the way the light source is controlled to selectively illuminate and cure the resin. In SLA, the light source is a laser, while DLP uses a projector, similar to a movie projection device, that illuminates the entire shape of the printed object at the surface of the liquid (Pagac et al., 2021; Son et al., 2021). In theory, DLP printing of an object takes less time, because each layer does not require a step-by-step laser scan; however, most DLP devices do not have the high resolution that SLA laser beams can provide (Revilla-León et al., 2020). Therefore, DLP is advantageous for rapid printing of larger parts with fewer details, while SLA is superior for printing accurate parts with intricate details (Huang et al., 2022).
2.1.3 Continuous liquid interface production (CLIP)
CLIP is a variant of DLP considered to be a vat-polymerization technology (Alammar et al., 2022), conducted using a liquid resin cylinder, a bottom-up construction platform, an ultraviolet lamp, an oxygen-permeable window, and a projector. The projector displays a continuous, extremely thin cross section of an object, using ultraviolet light from below (Pagac et al., 2021). Ultraviolet rays harden the liquid in cross section in a cylinder of liquid resin. Simultaneously, a lift pulls the formed objects out of the resin tank (Alammar et al., 2022). The key to CLIP printing is the presence of a window for oxygen and ultraviolet light to pass through at the bottom of the resin cylinder. As oxygen hinders the curing process, resin at the bottom of the cylinder continuously forms a “dead zone” that does not cure. This “dead zone” is very thin, such that ultraviolet light can pass through and solidify the resin above, which does not come into contact with oxygen. As there is no resin stuck to the bottom of the cylinder, the printing speed is very fast, because printing does not occur in the air but in the resin (printing in the air reduces curing speed, due to the presence of oxygen) (Alammar et al., 2022).
2.2 Powder bed fusion
PBF is the metal-printing technology most commonly used in dentistry. Ti and Cr-Co alloys are preferred metal materials in biomedical applications, primarily because of their mechanical properties, biocompatibility, thermal, magnetic, and electrical conductivity, and general high temperature resistance (Revilla-León et al., 2017). PBF includes SLS, direct metal laser sintering (DMLS), SLM, and electron beam melting (EBM). These techniques typically use high-powered lasers or electron beams to melt small particles, such as plastics, metals, ceramics, or glass, in powder form (Velasquez-Garcia and Kornbluth, 2021). The powder is usually preheated to a temperature below the melting point of the material before printing begins. The energy source is then controlled by the printer, enabling it to selectively melt powder on the surface of the powder bed. After melting one layer, the powder bed reduces by the height of one layer, and a new powder layer is then laid on top with a roller, subsequently completing printing of the new layer (Revilla-Leon et al., 2019a).
Most non-metallic materials printed by PBF do not require support structures, because the model is always fully wrapped and supported by green powder; however, metallic materials may require support structures to assist in rapidly transferring heat away from the part while reducing expansion during printing. PBF printers can build three-dimensional geometries, such as fine lattices, which are valuable for making prostheses to promote bone ingrowth; hence, PBF technology is widely used in medical fields, including orthopedics and for dental implants, among other applications (Mangano et al., 2014a). In addition, personalized titanium mesh and implant frameworks can be fabricated using this technology (Sumida et al., 2015; Barbin et al., 2020).
The main speed-limiting steps of PBF are the thermal cycle of the machine and post-processing of parts (Attarilar et al., 2021). Most PBF machines need to be warmed up to a certain temperature to start printing, and after printing, cooling is required before the printed parts can be removed from the machine. The post-processing procedures required are also highly dependent on the type of technology and materials used. For example, metal materials require processes such as thermal hardening and residual stress relaxation (Sing et al., 2016). Metal-printed parts may also require subsequent milling with a computer numerical control (CNC) lathe, to achieve a smooth finish, after they have been removed from the printer platform (Bordin et al., 2017).
At present, in the PBF category, SLM makes comprehensive use of cutting-edge technologies, such as new material, laser, and computer technologies, which have great potential for further future development (Ansari et al., 2019). Dental implants printed by SLM have higher density and strength, as well as sufficient dimensional accuracy (Chen et al., 2014), which can be attributed to the SLM forming principle. SLS binds metal or non-metallic powders with high melting points by melting a metal or binder with a low melting point (Jawahar and Maragathavalli, 2019), while SLM uses a high power laser with a small spot to melt metal powder rapidly and completely, and requires higher laser power density than that used for SLS (Revilla-León et al., 2017). In addition, although SLM parts have good mechanical strength, they may have high internal stresses caused by the thermal gradients induced during processing, necessitating additional heat treatment.
EBM is also a powder bed melting technology. The principles of fabrication using EBM and SLM are similar, but the heat sources differ (Velasquez-Garcia and Kornbluth, 2021). Compared with technologies that use lasers as the energy source, EBM has many advantages, such as high energy utilization, no reflection, high power density, and convenient focusing, which can be used to make implants (Raheem et al., 2021).
2.3 Material extrusion
The MEX process is also termed fused filament fabrication (FFF) (Bozkurt and Karayel, 2021). The MEX family process, fused deposition modeling (FDM), is the most common type of printing used in medical or dental equipment; however, its printing resolution is inferior to that of SLA (Huang et al., 2017; Oberoi et al., 2018). FDM can handle a variety of materials, such as foundry wax, polyamide (commonly known as nylon), acrylonitrile butadiene styrene plastic, polylactic acid (PLA) plastic, low melting point metals, and ceramics (Lin et al., 2019). In FDM, the thermoplastic material is heated, melted, and extruded uniformly from a nozzle to generate filaments. Simultaneously, the nozzle moves along a specific path, operated by an NC system according to the continuous thin layer data planned by the slicing software, for filling. After cooling, the filamentous material is bonded layer by layer to form a thin cross-section, and finally the layers are superimposed to form a three-dimensional entity (Torabi et al., 2015).
2.4 Material jetting
In 2000, Objet (Israel) applied for a patent for PolyJet technology (now owned by Stratasys). PolyJet sprays a liquid photopolymer layer onto the construction tray and immediately solidifies it using ultraviolet light. Compared with SLA, the PolyJet laser spot diameter is 0.06–0.10 mm, allowing much higher printing accuracy than that achieved by SLA, and facilitating fabrication of smooth and precision parts. In addition, due to its high-speed raster construction process, PolyJet can achieve fast printing, and does not require secondary curing. It has been demonstrated that implant guides made using PolyJet are more accurate than those fabricated using SLA technology (Tappa and Jammalamadaka, 2018; Patpatiya et al., 2022).
3 Research status on additive manufacturing applications in implant dentistry
The accuracy of surgical implant placement is greatly improved by surgical guides constructed by 3D printing technology. Customized titanium mesh improves the accuracy of bone augmentation, and 3D printing technology can even be used to prepare personalized implants to meet individual patient needs (Revilla-León et al., 2020). During implant restoration, 3D printing technologies can replace certain manual operations, such as the manufacture of custom trays, working casts, etc., helping to reduce human error. Suitable tray and accurate dental models are key to restoration. There is evidence that 3D printed custom trays and models are sufficiently accurate, which significantly impacts the success of the final restoration. Additionally, implant-supported frameworks generated by CNC cutting are very popular, but this process results in a waste of materials, whereas implant frameworks fabricated by 3D printing effectively avoid this problem. The emergence of 3D printing has undeniably aroused the interest of many dentists and accelerated the clinical development of implantology. Some examples of the application of 3D printing technologies in dentistry are presented in Figure 2.
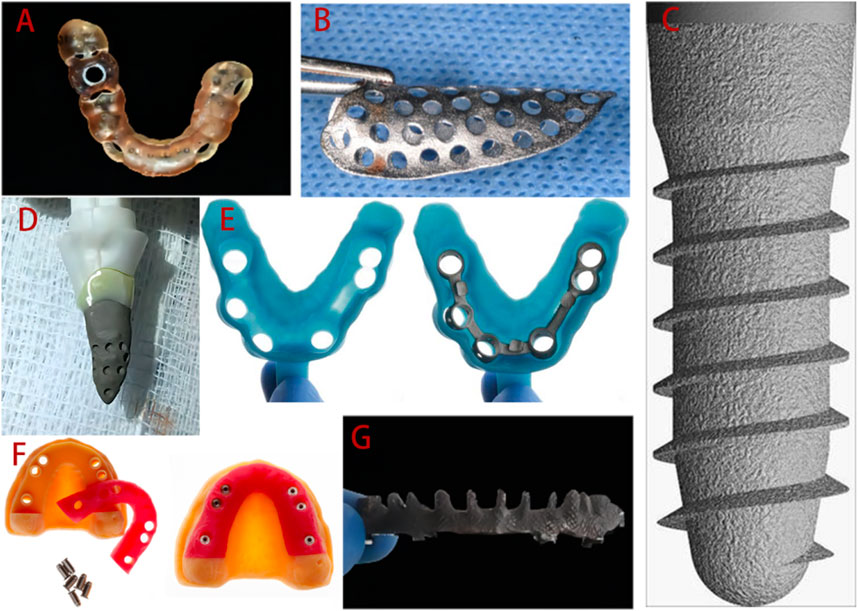
FIGURE 2. Applications of 3D printing technologies in implant dentistry clinical practice. (A) 3D printed surgical guide. (B) Personalized titanium mesh. (C) Standard implant. (D) Customized root-analogue implant. (E) Custom tray. (F) Implant models. (G) Implant framework. Adopted from (Mangano et al., 2012b; Revilla-Leon et al., 2017; Revilla-Leon et al., 2018a; Westover, 2019; Olea Vielba et al., 2020; Cucchi et al., 2021; Herschdorfer et al., 2021).
3.1 Applications of additive manufacturing in the surgical stage of implant treatment
3.1.1 Surgical guides
3D printed surgical guides have been used for more than 10 years, and the digital workflow of the guides is as follows. Virtual planning and design is conducted using computer software and digital workflows for planning and manufacturing, based on data obtained from 3D imaging, and these plans are then transmitted through 3D printed surgical guides (Rouzé L'Alzit et al., 2022; Unsal et al., 2020; Zhang et al., 2020). At present, SLA is the most widely used approach, due its economical nature and speed. Some newer technologies, such as PolyJet, can also be used to prepare guides (Henprasert et al., 2020). The guide manufacturing process is illustrated in Figure 3, taking tooth-supported guides as an example (Sedov et al., 2021).
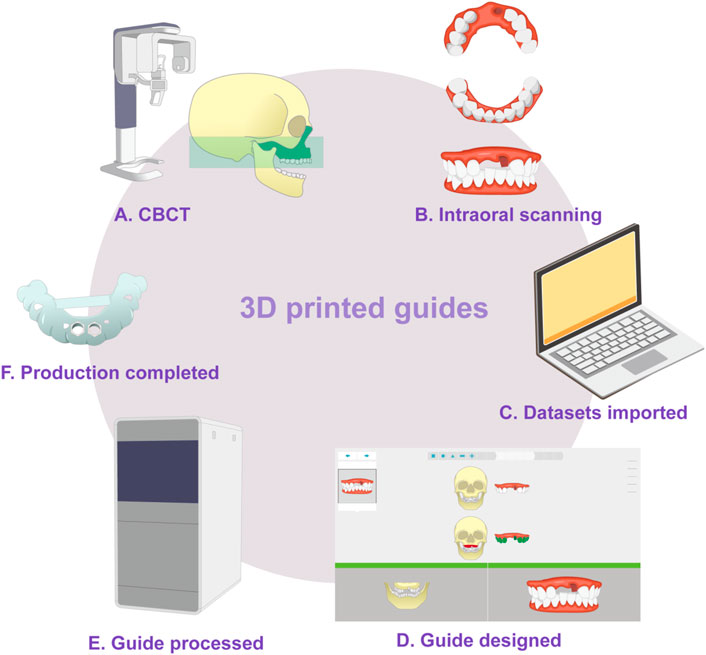
FIGURE 3. Schematic diagram of guide production. (A) Cone-beam computed tomography is used to generate 3D data from the teeth and jaws. (B) Three-dimensional information from the teeth and surrounding soft tissue is obtained by intraoral optical impression or scanned from a traditional plaster model. (C) In the guide design software, the two datasets are imported in turn and then matched, checked, and confirmed. (D) The guide is designed. (E) 3D printing is used to fabricate the guide. (F) The final guide is completed.
The production of guides involves multiple, closely-linked steps, including data collection and integration, design of the implanting plan and guide structure, and manufacturing, and errors in previous steps will accumulate in the final guides (Putra et al., 2022; Rouzé L'Alzit et al., 2022). Factors influencing template accuracy can be roughly divided into three categories: 1) System error: that is, errors generated during CBCT scanning and data conversion, which cannot be controlled by humans; 2) Manufacturing error: related to the type of 3D printer (Zhang et al., 2022) (Table 1), selection of printing materials, use of supporting structures, slicing method and software types (Cho et al., 2021; Elliott et al., 2022; D. D; Rubayo, et al., 2021); and 3) Other factors.
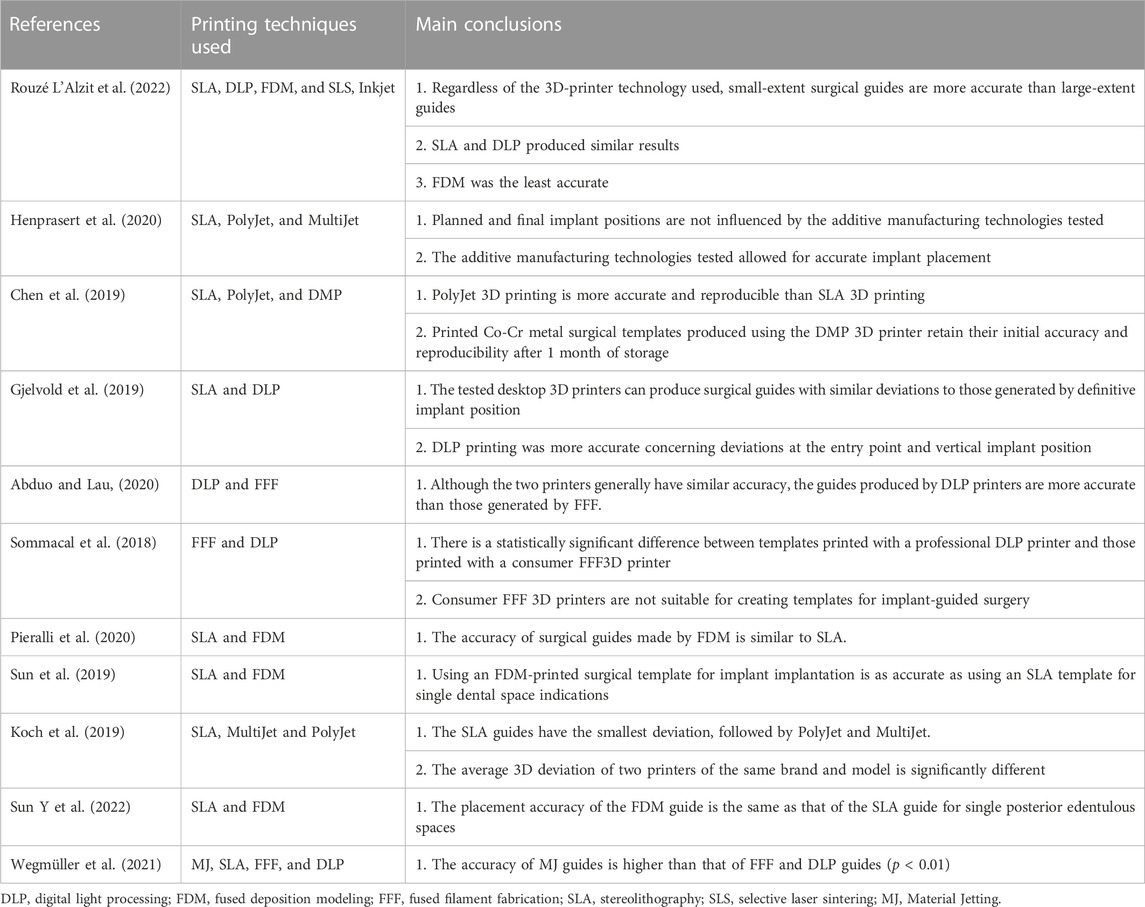
TABLE 1. Accuracy of different 3D printing techniques for fabrication of surgical guides for use in dental implantology.
To reduce surgical complications caused by problems with 3D printed surgical guide production, it is vital to understand the limitations of 3D printing technology. Research has demonstrated that 50 µm layer printing provides better overall guide dimensions than 100 µm layer printing (Dalal et al., 2020). Further, printing angulation can influence the intaglio surface, as well as tube deviations (Dalal et al., 2020). Rubayo et al. showed that 0- and 45-degree build angles produced the most accurate surgical templates, while a 90-degree build angle generated the least accurate surgical templates (D. D. Rubayo, et al., 2021). Tahir et al. evaluated the effect of different printing directions on the placement accuracy of implant surgical templates made by DLP. The results showed that the horizontally printed templates showed excellent accuracy (Tahir and Abduo, 2022).
Regarding other factors that influence template accuracy, Zhou et al. (Zhou et al., 2018) conducted a comprehensive comparison of various clinical factors and concluded that guide accuracy may be affected by guide position (maxilla or mandible), guide fixation (fixation screw or not) (Pessoa et al., 2022), type of guide (total or partial) (Mangano et al., 2018; Lou et al., 2021; Fotopoulos et al., 2022; Gargallo-Albiol et al., 2022), flap approach (open flap or flapless), differences in implant system (Zhu et al., 2021), high temperature sterilization (Marei et al., 2019; Kirschner et al., 2022) and support mode (tooth-supported, mucosa-supported or mixed-supported) (Pan et al., 2022). In addition, Henprasert et al. concluded that there was no significant difference in accuracy between 3D-printed and milled guides, but found that the former had the advantages of high efficiency and reduced material waste (Henprasert et al., 2020). Mukai et al. compared the repeatability and accuracy of two surgical guides obtained using 3D printing and milling methods (Mukai et al., 2021) by overlaying images and the results revealed no significant differences in average mismatch between the two groups in terms of trueness (p = 0.529) or precision (p = 0.3021), indicating that both milling and printing manufacturing methods are suitable for guided surgery.
3.1.2 Customized titanium mesh
The development of customized titanium mesh generated by 3D printing technology, with the aim of solving the shortcomings of traditional titanium mesh, has become a focus in GBR research and application (Xie et al., 2020). Based on patient CBCT three-dimensional jaw data, the ideal alveolar bone is virtually designed using CAD software, according to the shape of the dental arch and the expected implant position (Manzano Romero et al., 2021). Then, the matching customized titanium mesh is designed directly on the reconstructed alveolar bone model. Finally, customized titanium mesh is manufactured using SLM or EBM (Figure 4) (Xie et al., 2020; Cucchi et al., 2019; Seiler et al., 2018).
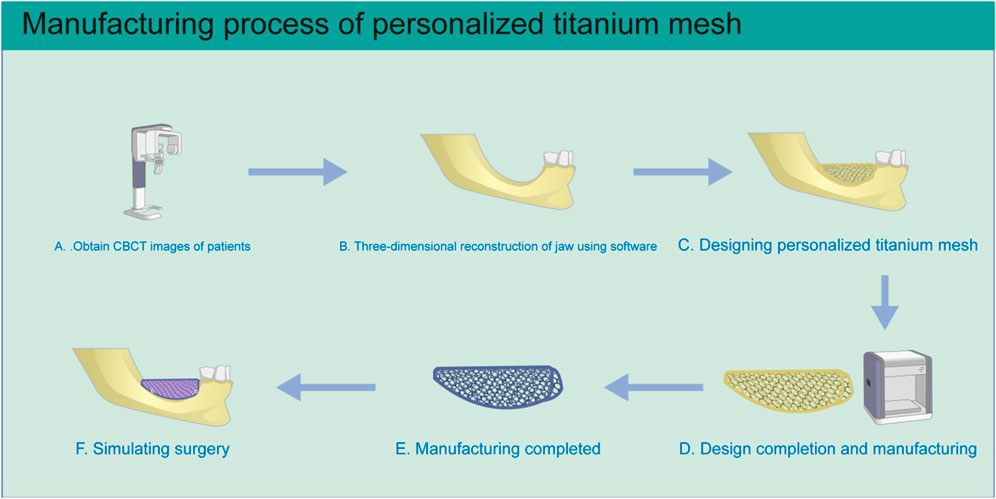
FIGURE 4. Schematic diagram of personalized titanium mesh production. (A) Three-dimensional CBCT image data of the patient’s jaws is obtained. (B) The three-dimensional jaw structure is reconstructed. (C) Personalized titanium mesh is designed according to the condition of alveolar bone defect. (D) After the design is completed, the file is imported into the 3D printer for manufacturing. (E) After printing is completed, the titanium mesh is treated by ultrasonic cleaning, sandblasting, and acid etching. (F) The situation is simulated during the operation.
There are many advantages of 3D printed customized titanium mesh (Seiler et al., 2018; Hartmann et al., 2019), as follows.
• The cell structure, pore size, and thickness of 3D printed titanium mesh can be adjusted according to requirements (Jung et al., 2014).
• The scope of 3D printed titanium mesh is limited to the area of the bone defect rather than extending into areas with sufficient bone mass, avoiding overstretching of soft tissue. In the second stage of surgery, the doctor only needs to remove the titanium mesh through the alveolar crest incision, avoiding the need to turn the flap again.
• The use of customized titanium mesh can reduce the operation time, thus shortening exposure to general anesthesia, decreasing blood loss, decreasing wound exposure time, and simplifying the surgical procedure.
• Personalized titanium mesh has lower exposure rates, as confirmed by a meta-analysis conducted by Zhou et al. (Zhou et al., 2021). Titanium mesh avoids nerves and blood vessels in the initial design, which is of great significance in improving the accuracy of GBR.
Guidelines for designing 3D printed customized titanium meshes have been published (Sagheb et al., 2017; Hartmann and Seiler, 2020; Li L et al., 2021) and include.
• The bone mass around the implant, the shape of alveolar bone, and the condition of soft tissues are factors that should be considered in the design.
• The distance between the boundary of the titanium mesh and adjacent teeth, nerves, blood vessels and other important structures should be ≥2 mm.
• The position and number of nail holes should be determined according to the retention needs. Nail hole diameter can be designed according to that of the titanium nail to be used.
In terms of the accuracy of 3D printed titanium mesh, Sumida et al. (Sumida et al., 2015) confirmed that the maximum error between customized titanium mesh and CAD data is <300 μm, indicating that it has sufficient accuracy for GBR. Regarding mechanical properties, the average tensile strength and test stress of titanium mesh with a thickness of 0.5 mm and a pore size of 1 mm printed by SLM are 627 ± 41 and 541 ± 26 MPa, respectively, while the average elongation strength and micro-Vickers hardness are 7.2% ± 2.8% and 203 ± 5 HV, respectively, demonstrating that titanium mesh has good mechanical properties. Otawa et al. (Otawa et al., 2015) tested the accuracy of SLM titanium mesh. The results show that dimensional accuracy, pore structure accuracy and the error between CAD design and the scanned real product by overlapped images are tolerable, and the maximum error and average error are 292 μm and 139 μm, respectively.
In term of indications, customized CAD/CAM titanium mesh can be used in bone augmentation surgery for horizontal and vertical bone defects, particularly for cases with large area, complex, combined horizontal and vertical bone defects. Some clinical studies have focused on the bone augmentation effect of CAD/CAM titanium mesh as shown in Table 2. It is worth noting that many clinical studies have shown that customized titanium mesh cannot completely avoid the problem of titanium mesh exposure, but exposure does not necessarily affect the final bone augmentation effect (Zhou et al., 2021; Ciocca et al., 2018a; Hartmann and Seiler, 2020). From the perspective of design, some scholars have demonstrated this by optimizing the design of customized titanium mesh thickness and pore size and other parameters and making the shape of customized titanium mesh smooth, so as to reduce the possibility of exposure. In addition, some scholars suggest using fibrin rich in platelets or collagen membrane to cover titanium mesh to reduce the exposure rate (Cucchi et al., 2021; Sagheb et al., 2017).
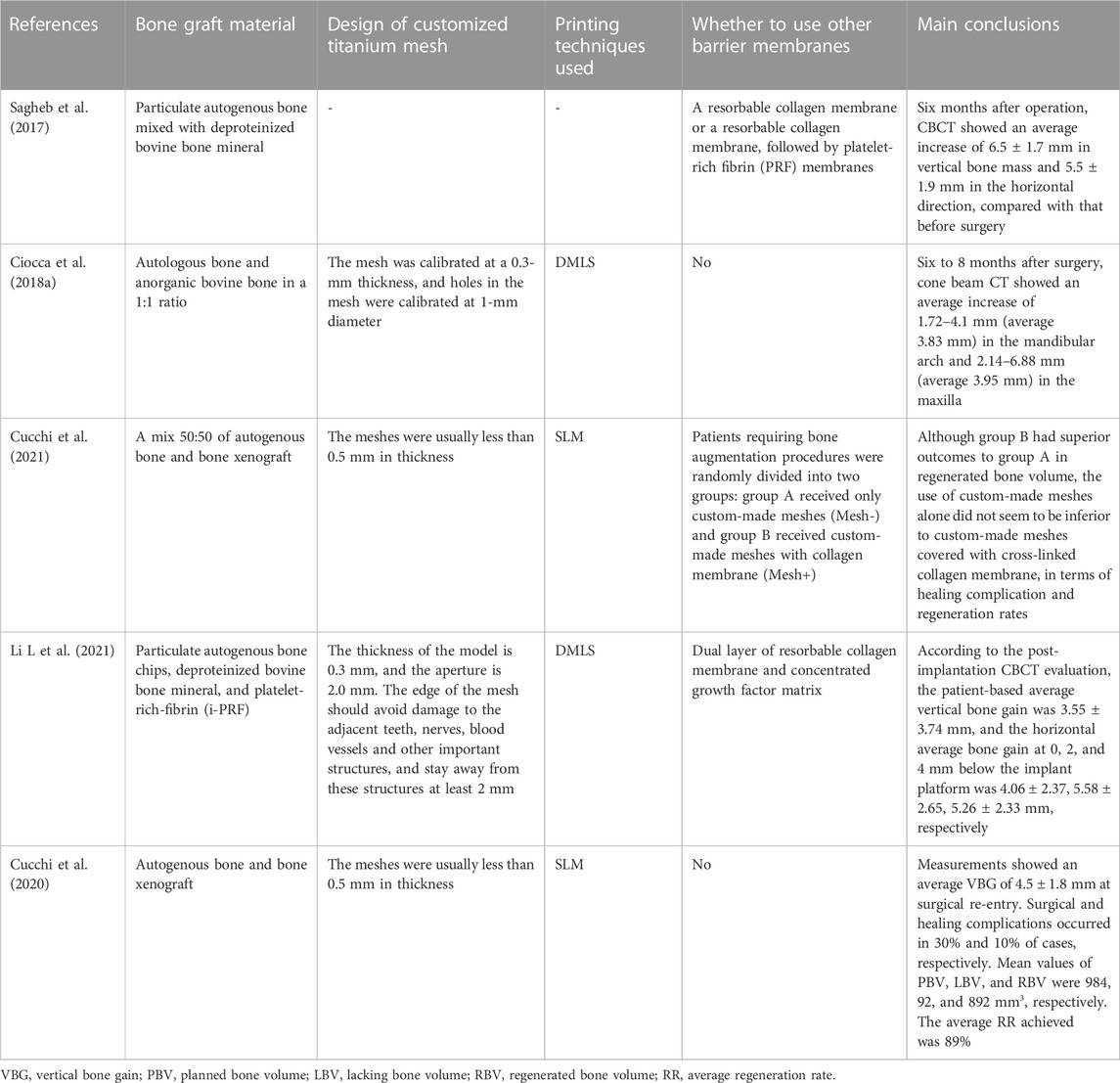
TABLE 2. Different studies used 3D printing titanium mesh to obtain the effect of bone augmentation.
In fact, there is another way AM can help GBR, as used by Li et al. (Li S et al., 2021). By collecting intraoral scanning and DICOM (digital imaging and communications in medicine) data from patients, the implant position can be digitally designed, and the alveolar bone digitally augmented around the ideal implant position. This process provides superior precision and efficiency relative to traditional GBR procedures, as reflected in the preoperative virtual bone augmentation design, with an increment of 0.5 mm beyond the contour of the labial bone arch to compensate for possible bone resorption during bone healing. After 3D printing of the reconstructed alveolar bone model, the titanium mesh was trimmed and prefabricated on the alveolar bone model. The preformed personalized titanium mesh has lower technical sensitivity and better plasticity than 3D printed titanium mesh (Xie et al., 2020). Indeed, the customized titanium mesh made by both methods increases the possibility of customized bone regeneration (Seiler et al., 2018).
3.1.3 Dental implants
Interconnected pore structures are conducive to the transport of nutrients and increase surface roughness, which facilitates new bone formation and osseointegration (Chen et al., 2020; Yu et al., 2020). Moreover, porous structures reduce implant stiffness and generate an implant elastic modulus similar to that of the human jaw, thereby reducing stress shielding effects and allowing implants to be retained and function in the jaw for long periods of time (Wally et al., 2019). Titanium implants with uniform micron-scale porous structure can be produced by 3D printing (Mangano et al., 2014b). SLM and EBM are typically used to prepare dental implants, and implant materials are mainly titanium and titanium alloys, although some scholars have attempted to use zirconia (Pillai et al., 2021). Further, some researchers have proposed the use of titanium for the root portion and zirconium in the abutment portion to form one-piece implants that can achieve optimal osseointegration and ideal soft tissue attachment (Westover, 2019).
Using the characteristics of the complex geometric components formed by 3D printing, implants simulating the natural root can be customized. Personalized implants can be completely consistent with the shape of the patient’s extraction socket. In immediate implant application, this approach can achieve high initial stability and reproduce the perfect gingival profile of natural teeth (Figliuzzi et al., 2012). In addition, personalized implants have similar stress conduction and distribution characteristics to natural teeth (Moin et al., 2013).
To obtain personalized implants, teeth and jaw data are collected by computed tomography or CBCT before surgery, and a three-dimensional model of the teeth reconstructed using software. Then, a CAD model of the implant is generated, exported to an STL file, and transferred to specialist reverse software. The surface of the model is smoothed to generate a regular surface, and then transferred to CAD software for abutment design. After completion, an STL file of the implant and abutment is obtained and imported into the printer for processing and manufacture (F. G. Mangano F. G. et al., 2013; Westover, 2019). Due to deviations in precision, some researchers have incremented implant CAD models by dimension percentages (0%, 5%, and 10%) for clinical applications (F. G. Mangano F. G. et al., 2013). Another technique involves laser scanning of the root to construct the final implant after extraction, and design of macro-retainers on the implant surface to increase its stability following placement (Dantas et al., 2021).
Another class of implants, referred to as patient-matched implants, are less personalized than 3D printed root-analogue implants; for example, some scholars have used 3D printing to generate narrow-diameter implants for patients with insufficient alveolar bone width (F. Mangano et al., 2013b). In addition, 3D printing is also used in the manufacture of non-customized dental implants (similar to current commercial implants). The Italian “Tixos” implant, which is similar to a traditional implant, is produced by DMLS technology and has a variety of sizes to choose from (Mangano et al., 2012a). Furthermore, implants produced by DMLS have high fatigue strength and good corrosion resistance (Yang et al., 2020). Gehrke et al. confirmed that the mean fracture strength of DMLS implants with diameter 3.5 mm and length 16 mm can reach >1200 N (Gehrke et al., 2018): However, for two-stage dental implants, it is difficult to achieve a tight connection between a 3D printed implant and the abutment due to a lack of surface accuracy, but a tight connection can be achieved by machining of the connection structure in the 3D printing implant platform (Tunchel et al., 2016). The predicted survival rates of 3D printed implants confirmed in clinical studies are presented in Table 3.
Poly ether ether ketone (PEEK) 3D printed implants have also attracted attention (Basgul et al., 2021b; Sun C et al., 2022). PEEK is an aromatic polymer with characteristics of corrosion resistance, high temperature resistance, non-cytotoxicity, X-ray transmission, chemical stability, and good biological safety (Najeeb et al., 2016; Panayotov et al., 2016; Basgul et al., 2021a). Compared with titanium alloy, the elastic coefficient of PEEK is relatively low and closer to that of human cortical bone, which helps to reduce stress shielding effects at the bone-implant interface, thereby minimizing implant loosening and peri-implant bone loss (Han et al., 2022). Although it has many advantages, the main challenge for PEEK as a dental implant material is that it is a bioinert material with low surface energy; Therefore, appropriate strategies should be developed to improve the biological activity of PEEK and realize its potential benefits (Jung et al., 2019; Basgul et al., 2021b).
FDM and SLS 3D printing technologies can be used to print PEEK implants, and the former is most commonly used (Schmidt et al., 2007; Baek et al., 2022; Basgul & Spece et al., 2021). The molecular structure of PEEK does not change at high temperature; thus, no toxic substances are produced in the printing process (Prechtel et al., 2020; Shilov et al., 2022). Further, 3D printed PEEK implants have many advantages, including free design, interconnected porous structures and specific surface topography (Shishkovsky et al., 2013; Torstrick et al., 2018; Yuan et al., 2018). Han et al. systematically analyzed the biological activity of PEEK implants printed by FDM, including surface roughness, wettability, cell adhesion, metabolic activity, and proliferation (Han et al., 2019a). They found that, compared with the sandblasted surface of traditional molded or milled PEEK implants, the special surface morphology and porous structure of 3D printed PEEK implants played an important role in stimulating bioactive potential.
In addition, to improve the bioactivity of 3D printed PEEK implants, Han et al. used plasma surface treatment technology to introduce Ar or O2 functional groups into the surface of 3D printed PEEK, which significantly improved surface hydrophilicity and changed surface morphology and roughness (Han et al., 2022). Plasma-treated PEEK induced adhesion, metabolic activity, proliferation, and osteogenic differentiation of SAOS-2 cells in vitro. Su et al. developed a sulfonation strategy to create uniform micropores on PEEK lattice scaffolds fabricated by FFF (Su et al., 2020). The suitable lattice structure sulfonation time was 30–45 s, and the mean size of formed micropores was 0.19 ± 0.07 μm. Compared with the untreated PEEK scaffold, the micropore structure on the FFF printed PEEK lattice scaffold significantly improved cell attachment, spreading, proliferation, and bone-specific differentiation of MC3T3-E1 cells. More importantly, the existence of micropores on the lattice scaffold promoted the attachment of new soft tissue to PEEK implants.
In addition to biocompatibility studies, other investigations have been dedicated to improving the mechanical strength of PEEK (Chen et al., 2022). Numerous factors can influence the biomechanical qualities of 3D printed PEEK implants, including the size/shape of the test sample, printing temperature, printing speed, layer thickness, component orientation, nozzle diameter, and raster angle (Arif et al., 2018; Basgul & Spece et al., 2021;Basgul & Thieringer et al., 2021; Prechtel et al., 2020; Moby et al., 2022). PEEK formed by FFF has sufficient tensile, bending, and fracture strength (Arif et al., 2018), but the fatigue properties of these implants require further evaluation (Fabris et al., 2022). Sonaye et al. studied the printing parameter set of PEEK implants produced by FFF technology (Sonaye et al., 2022) and showed that the best printing parameters for PEEK implants are: nozzle temperature, 450°C; bedplate temperature, 150°C; chamber temperature, 90°C; layer thickness, 0.1 mm; and printing speed, 30 mm/s. The use of optimized process parameters resulted in implants with excellent fatigue performance. Further, reinforcement of PEEK with various materials, such as glass fiber, carbon fiber, and silicate based bioceramics, improves its mechanical strength to a certain extent (Han et al., 2019b; Petersmann et al., 2020; Taymour et al., 2022).
In summary, the excellent overall properties of PEEK mean that it has considerable prospects for application as dental implant material. Combined with 3D printing, customized and graded porous PEEK implants can be manufactured quickly, resulting in implants with better performance than those generated by traditional manufacturing processes. However, 3D printing of PEEK implants is mostly at the laboratory research stage, and wide application of PEEK implants in the clinic requires more supportive evidence from basic research and clinical trials.
3.2 Applications of additive manufacturing in the restoration stage of implant therapy
3.2.1 Custom trays
Three-dimensional printing technology has been applied to fabricate custom trays for implants (Kim et al., 2019b). Revilla-León et al. and Piedra et al. described an implant impression technique for a complete arch, digitally designing metal splint structured and custom trays, which were generated using DMLS and DLP technology, respectively (Revilla-León and Özcan, 2017; Piedra and Revilla-Leon, 2018). Good matching between the metal splint structure and the impression copings makes it simple to locate in the patient’s mouth. Compared with manual individual trays, 3D printed custom trays have many advantages: First, custom trays have sufficient extension range and more uniform 3D impression material space between the splinting structure and the custom tray (Sun et al., 2017), providing more accurate oral tissue records; and second, they reduce the time required to fix the impression rod using materials such as resin, shorten clinical operation duration, and avoid the inaccuracies in definitive models caused by resin polymerization shrinkage (Kim et al., 2019a; Revilla-Leon and Ozcan, 2019).
Research confirms that 3D printed custom trays are stronger than traditional custom trays (Liu et al., 2019), which can be attributed to the parameters set in the manufacturing process. With increasing printing layer thickness, the tensile bond strength of trays first increases and then decreases, reaching a peak at 0.4 mm thickness, and printing time decreases sharply. Although bending and tensile strength decrease, dimensional printing accuracy remains constant from 0.1 to 0.4 mm, and then decreases at 0.5 mm, demonstrating that moderate layer thickness provides the best performance for 3D printing of custom trays (Liu et al., 2021).
To meet clinical requirements, tray materials should have both sufficient rigidity and dimensional stability and provide sufficient retention of impression materials. Xu et al. evaluated the bonding strength between three 3D printed custom tray materials (SLA, DLP, and FFF) and three elastomeric impression/adhesive systems [vinyl siloxane ether (VSXE), vinyl polysiloxane (VPS), and polyether (PE)] using the peel test (Xu et al., 2020). The results showed that the three 3D printing tray materials have good chemical compatibility with adhesives such as VSXE, VPS, and PE, and that 3D printing tray materials can provide sufficient clinical bonding strength with elastic impression/adhesive systems; when severe impression removal resistance is detected, it is recommended to use both PLA and VPS.
The studies described above confirmed that 3D printed custom trays have sufficient strength, hardness, and bonding strength with impression materials. Hence, 3D printing implant impression techniques could provide alternatives to conventional impression techniques for implant restoration.
3.2.2 Implant models
Materializing digital impressions is among the earliest applications of 3D printing in dentistry (Revilla-Leon and Ozcan, 2019). The definitive implant model must ensure accurate implant location and relationship with adjacent teeth. Compared with traditional plaster models, 3D printed models have the advantages of being lightweight, resistant to damage, and having high finish, good wear resistance, and avoiding the inaccurate position of the analogue, which may be caused by artificial fixation of the implant analogue on the impression (Monaco et al., 2018; Tian et al., 2021). Moreover, 3D printed models overcome the disadvantages of digital models, as the physical model facilitates simple evaluation of the occlusal condition and interproximal contact (Buda et al., 2018).
Printed model inaccuracy results from accumulation of distortions caused by the acquisition method, parameters determined by the design software, and the printing process (Tian et al., 2021), which may be affected by many factors, such as scanner selection and the digitization process, among others. Papaspyridakos et al. (Papaspyridakos et al., 2020) proposed that the deviation of a printed model for use in implant restoration should ideally be <100 μm and should not exceed 150 μm.
Maria et al. (Maria et al., 2021) measured the physical positions of implants in a master model and analogs in printed resin models using a coordinate measuring machine. Three analog implant systems for 3D printed resin models [Straumann (ST), Core3DCentres (CD) and Medentika (MD)] were tested. Mean 3D linear distortion for ST (−155.7 ± 60.6 μm), CD (124.9 ± 65.0 μm), and MD (−92.9 ± 48.0 μm) differed significantly (p < 0.01), confirming that the implant analog system has a significant effect on the accuracy of analogs in 3D printed models. Mean absolute angular distortion did not differ significantly between ST (0.57° ± 0.48°) and CD (0.41° ± 0.27°), while both differed significantly from MD (2.11° ± 1.14°). Print orientation had a significant effect on 3D linear distortion, but no discernible trend could be found. Michelinakis et al. pointed out that, when choosing a semi-digital method, the cumulative deviation of the model in the 3D printing process may lead to a deviation in the position of the implant analogue, which depends on the printing technique and materials used (Table 4) (Michelinakis et al., 2021). In addition, Yousef et al. (Yousef et al., 2021) suggested that restoration be conducted as soon as possible after the model is printed, as the 3D printed model will deform during long-term storage.
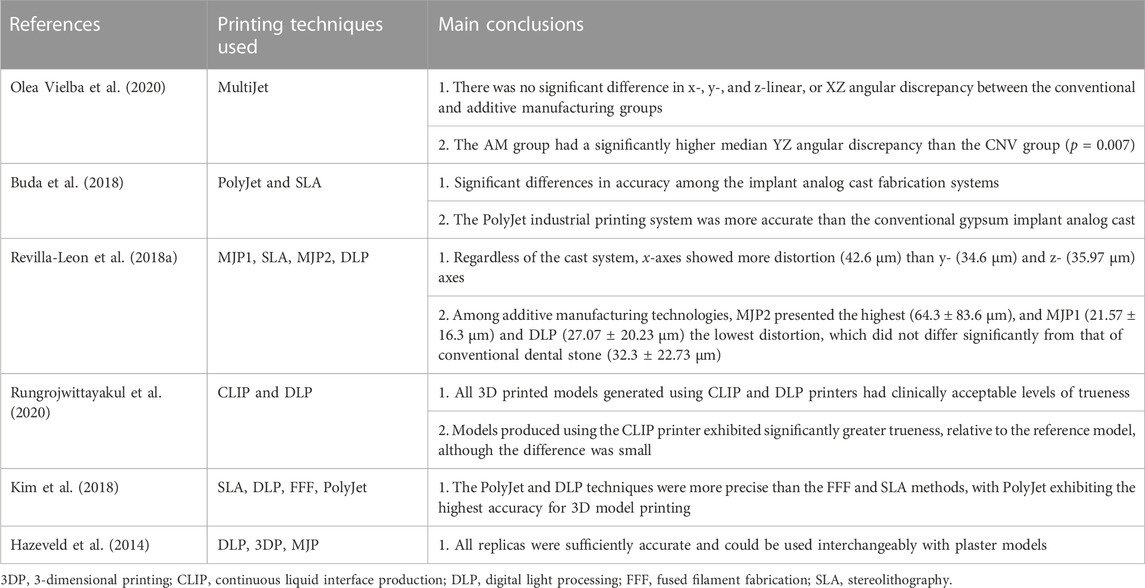
TABLE 4. Accuracy of different 3D printing techniques for fabrication of implant models for use in dental implantology.
3.2.3 Implant frameworks
CAD/CAM technologies are widely used to design and manufacture frameworks for implant-supported prostheses (Kapos and Evans, 2014). After completing CAD of implant-supported frameworks, the virtual design is transformed into a physical object by addition or subtraction manufacturing (Revilla-Leon et al., 2019b). Compared with traditional casting technology, subtractive technologies reduce some clinical steps and eliminate certain human errors, but result in waste of materials and may fail to reflect the finer details of frameworks (Svanborg et al., 2018). Metal AM processes, such as SLM or EBM, are also used to fabricate implant frameworks, and effectively reduce material waste (Barbin et al., 2020). The mechanical properties of 3D printed implant frameworks can be comparable to, or even better than, those of traditional casting (Revilla-Leon et al., 2021). In addition, the shape of retainers on frameworks can be freely designed to increase the adhesion between the framework and the resin material.
The accuracy of the fit between implant frameworks and the underlying structures is an extremely important factor in avoiding biological and technical complications (Svanborg et al., 2018), which affect the long-term clinical success of implant-supported restorations. In an in vitro study, Revilla-Leon et al. evaluated discrepancies in the manufacture of titanium frameworks for implant-supported complete-arch prostheses manufactured using SLM and EBM(Revilla-Leon et al., 2018a). First, titanium frameworks for implant-supported complete-arch prostheses were designed using dental software. Then, frameworks were fabricated using SLM or EBM technology. The manufactured titanium frameworks and framework STL files were fitted and superimposed using a coordinate measuring machine. The results showed that there were no significant differences between SLM and EBM in the x and y-axes of implant frameworks, while the z-axis varied. The 3D discrepancies of all comparisons ranged from 60 ± 18 μm to 69 ± 30 μm, and the differences were not statistically significant. The discrepancy in the y-axis was largest (37–56 μm), followed by the x (16–44 μm) and z (6–11 μm) axes. Revilla-Leon et al. also compared the discrepancy at the implant abutment-prosthesis interface of complete-arch cobalt-chromium implant frameworks fabricated by additive and subtractive technologies before and after ceramic veneering in a separate study (Revilla-Leon et al., 2021). No significant differences were detected between the CNC and AM groups, except that the AM group presented a significantly higher discrepancy on the x-axis compared with the CNC group. Abu and Onoral. (2021) fabricated 3-unit Co-Cr frameworks with three indirect (conventional technique, polymethyl methacrylate milling, SLA) and two direct (SLM and soft alloy milling) methods. The mean vertical marginal discrepancy value of the SLM group (74.2 ± 20.5 μm) was significantly lower than those of all other groups (p < 0.05), demonstrating superior fitting accuracy. Barbin et al. (2020) evaluated the influence of milling, SLM, and EBM on full-arch fixed dental prostheses (FAFDPs) manufacture, in terms of marginal FAFDP misfits, prosthetic screw stability, and strain and stress on implant-supported systems, among other parameters. Compared with SLM and EBM frameworks, milled frameworks had the highest average marginal match, but the deviation of the former was within a clinically acceptable range. Ceramic veneer had no significant effect on the average marginal misfit values of any manufacturing process, while spark erosion reduced mean marginal misfit values for SLM and EBM titanium frameworks. On screw stability analysis, milled frameworks showed higher mean screw-loosening values, but after chewing simulations, none of the frameworks exhibited screw loosening.
In a recent systematic review (Thakur et al., 2021), the marginal fit and accuracy of complete-arch implant-supported frameworks, implant-retained fixed partial dentures, single implant crowns, and interim implant-retained restorations fabricated using AM and subtractive manufacturing methods were compared. The results showed that there was no significant difference in the marginal fit of single implant crowns or complete-arch implant frameworks between the two fabrication methods. For implant-supported fixed partial dentures, AM was superior to subtractive milling, but both digital fabrication methods produced implant-supported superstructures with clinically acceptable marginal fit.
The characteristic superficial texture of the layer-by-layer buildup in AM technologies results in a rough metal surface. A strong correlation between the roughness values on mating surfaces and implant-prosthodontic discrepancy has been reported. To achieve acceptable implant prosthodontic discrepancy, some scholars have combined 3D printing technologies and subtractive processing, electropolishing, sandblasting, or other post-processing techniques (Revilla-Leon et al., 2019b; Revilla-Leon et al., 2021). Ciocca et al. (2018b) compared the trueness and precision of frameworks manufactured with an SLM/milling hybrid technique (SLM/m) and conventional milling. The maximum misfit for the milled group was 20–35 μm, while there was no significant difference between SLM and SLM/m, with errors of 8–16 μm and 9–22 μm, respectively. Moreover, irrespective of the manufacture method, the trueness of titanium framework misfit was affected by framework span.
4 Discussion
The focus of this review was to provide an overview of AM technologies commonly used in implant dentistry and their application in the surgical and restoration stages of implant therapy. In addition, the accuracy of different 3D printing techniques for fabrication of printed parts is summarized in Table 2 and Table 4. The growing interest in 3D printing technologies clearly shows their potential impact on the future of implant dentistry (Nikoyan and Patel, 2020). With their advantages of high material utilization, ability to form complex geometric structures, and production of personalized products, 3D printing technologies have become an alternative method to generate components from CAD files. The various materials used in implant dentistry require the application of different AM techniques. Metal materials, such as personalized titanium meshes and titanium dental implants, can be manufactured using DMLS/SLM/EBM, whereas polymer materials, such as surgical guides and implant models, are fabricated using SLA, DLP, and PolyJet techniques.
Resin material products (surgical guides and implant models, etc.) produced by VPP technology have been widely studied and applied; however, there are specific areas that warrant further investigation. In particular, the numbers of patients and observation time in clinical studies of personalized titanium mesh generated by 3D printing have been limited to date; hence, the therapeutic effects of this approach require further study and verification. In future research, to ensure mechanical strength, continued optimization of personalized titanium mesh parameters, such as thickness, pore diameter, and shape, is required. In addition, the effects of combining personalized titanium mesh with absorbable collagen membrane, concentrated growth factor membrane, or other bone augmentation techniques warrant further exploration. Furthermore, 3D printing to manufacture dental implants has only recently been introduced; thus, more research and clinical studies are needed to understand the long-term safety and clinical efficacy of 3D printed implants. Future work will include study of the mechanical properties and structural characteristics, as well as printing process optimization of 3D printed dental implants, to achieve improved accuracy and performance (Oliveira and Reis, 2019). Finally, although it may seem that all oral implant medical devices can be made using 3D printers, a single technology may not be able to meet all patient needs. For some applications, 3D printing technology needs to be combined with milling or other cutting/finishing techniques, to generate a product that can achieve the final application goal (de Oliveira Campos et al., 2020).
Author contributions
SH and HW were involved in study design, literature research, data analysis, and writing the manuscript. HW was also involved in project administration, writing-review and editing, and conceptualization. DL was involved in conceptualization, study design, supervision, submission of the manuscript, and obtaining funding.
Funding
This work was supported by grants from The National Key Research and Development Program of China (2017YFB1104100).
Conflict of interest
The authors declare that the research was conducted in the absence of any commercial or financial relationships that could be construed as a potential conflict of interest.
Publisher’s note
All claims expressed in this article are solely those of the authors and do not necessarily represent those of their affiliated organizations, or those of the publisher, the editors and the reviewers. Any product that may be evaluated in this article, or claim that may be made by its manufacturer, is not guaranteed or endorsed by the publisher.
References
Abduo, J., and Lau, D. (2020). Effect of manufacturing technique on the accuracy of surgical guides for static Computer-Aided implant surgery. Int. J. Oral Maxillofac. Implants 35 (5), 931–938. doi:10.11607/jomi.8186
Abduo, J., Lyons, K., and Bennamoun, M. (2014). Trends in computer-aided manufacturing in prosthodontics: A review of the available streams. Int. J. Dent. 2014, 1–15. doi:10.1155/2014/783948
Abu, G. A., and Onoral, O. (2021). An assessment of the passivity of the fit of multiunit screw-retained implant frameworks manufactured by using additive and subtractive technologies. J. Prosthet. Dent. [Epub ahead of print]. doi:10.1016/j.prosdent.2021.06.022
Alammar, A., Kois, J. C., Revilla León, M., and Att, W. (2022). Additive manufacturing technologies: Current status and future perspectives. J. Prosthodont. 31, 4–12. doi:10.1111/jopr.13477
Anadioti, E., Kane, B., and Soulas, E. (2018). Current and emerging applications of 3D printing in restorative dentistry. Curr. Oral Health Rep. 5 (2), 133–139. doi:10.1007/s40496-018-0181-3
Ansari, M. J., Nguyen, D., and Park, H. S. (2019). Investigation of SLM process in terms of temperature distribution and melting pool size: Modeling and experimental approaches. Materials 12 (8), 1272. doi:10.3390/ma12081272
Arif, M. F., Kumar, S., Varadarajan, K. M., and Cantwell, W. J. (2018). Performance of biocompatible PEEK processed by fused deposition additive manufacturing. Mater. Des. 146, 249–259. doi:10.1016/j.matdes.2018.03.015
Attarilar, S., Ebrahimi, M., Djavanroodi, F., Fu, Y., Wang, L., and Yang, J. (2021). 3D printing technologies in metallic implants: A thematic review on the techniques and procedures. Int. J. Bioprint 7 (1), 306. doi:10.18063/ijb.v7i1.306
Baek, I., Kwon, O., Lim, C., Park, K. Y., and Bae, C. (2022). 3D PEEK objects fabricated by fused filament fabrication (FFF). Materials 15 (3), 898. doi:10.3390/ma15030898
Barazanchi, A., Li, K. C., Al-Amleh, B., Lyons, K., and Waddell, J. N. (2017). Additive technology: Update on current materials and applications in dentistry. J. Prosthodont. 26 (2), 156–163. doi:10.1111/jopr.12510
Barbin, T., Velôso, D. V., Del Rio Silva, L., Borges, G. A., Presotto, A. G. C., Barão, V. A. R., et al. (2020). 3D metal printing in dentistry: An in vitro biomechanical comparative study of two additive manufacturing technologies for full-arch implant-supported prostheses. J. Mech. Behav. Biomed. 108, 103821. doi:10.1016/j.jmbbm.2020.103821
Basgul, C., Spece, H., Sharma, N., Thieringer, F. M., and Kurtz, S. M. (2021a). Structure, properties, and bioactivity of3D printedPAEKs for implant applications: A systematic review. J. Biomed. Mater. Res. Part B Appl. Biomaterials 109 (11), 1924–1941. doi:10.1002/jbm.b.34845
Basgul, C., Thieringer, F. M., and Kurtz, S. M. (2021b). Heat transfer-based non-isothermal healing model for the interfacial bonding strength of fused filament fabricated polyetheretherketone. Addit. Manuf. 46, 102097. doi:10.1016/j.addma.2021.102097
Bordin, A., Sartori, S., Bruschi, S., and Ghiotti, A. (2017). Experimental investigation on the feasibility of dry and cryogenic machining as sustainable strategies when turning Ti6Al4V produced by Additive Manufacturing. J. Clean. Prod. 142, 4142–4151. doi:10.1016/j.jclepro.2016.09.209
Bozkurt, Y., and Karayel, E. (2021). 3D printing technology; Methods, biomedical applications, future opportunities and trends. J. Mater. Res. Technol. 14, 1430–1450. doi:10.1016/j.jmrt.2021.07.050
Buda, M., Bratos, M., and Sorensen, J. A. (2018). Accuracy of 3-dimensional computer-aided manufactured single-tooth implant definitive casts. J. Prosthet. Dent. 120 (6), 913–918. doi:10.1016/j.prosdent.2018.02.011
Chen, J., Xiao, Z., Yangpeng, S., Deng, F., and Zhiguang, Z. (2020). Production of inter-connective porous dental implants by computer-aided design and metal three-dimensional printing. J. Biomater. Appl. 34 (9), 1227–1238. doi:10.1177/0885328219899523
Chen, J., Zhang, Z., Chen, X., Zhang, C., Zhang, G., and Xu, Z. (2014). Design and manufacture of customized dental implants by using reverse engineering and selective laser melting technology. J. Prosthet. Dent. 112 (5), 1088–1095.e1. doi:10.1016/j.prosdent.2014.04.026
Chen, L., Lin, W., Polido, W. D., Eckert, G. J., and Morton, D. (2019). Accuracy, reproducibility, and dimensional stability of additively manufactured surgical templates. J. Prosthet. Dent. 122 (3), 309–314. doi:10.1016/j.prosdent.2019.02.007
Chen, W., Zhang, X., Tan, D., Xu, P., Yang, B., Shi, K., et al. (2022). Improvement in mechanical properties of 3D-printed PEEK structure by nonsolvent vapor annealing. Macromol. Rapid Comm. 43 (7), 2100874. doi:10.1002/marc.202100874
Cho, J. Y., Kim, S. B., and Ryu, J. (2021). The accuracy of a partially guided system using an in-office 3D-printed surgical guide for implant placement. Int. J. Comput. Dent. 24 (1), 19–27.
Ciocca, L., Lizio, G., Baldissara, P., Sambuco, A., Scotti, R., and Corinaldesi, G. (2018a). Prosthetically CAD-CAM-Guided bone augmentation of atrophic jaws using customized titanium mesh: Preliminary results of an open prospective study. J. Oral Implantol. 44 (2), 131–137. doi:10.1563/aaid-joi-D-17-00125
Ciocca, L., Meneghello, R., Savio, G., Scheda, L., Monaco, C., Gatto, M. R., et al. (2018b). Manufacturing of metal frameworks for full-arch dental restoration on implants: A comparison between milling and a novel hybrid technology. J. Prosthodont. 28 (5), 556–563. doi:10.1111/jopr.13067
Cucchi, A., Bianchi, A., Calamai, P., Rinaldi, L., Mangano, F., Vignudelli, E., et al. (2020). Clinical and volumetric outcomes after vertical ridge augmentation using computer-aided-design/computer-aided manufacturing (CAD/CAM) customized titanium meshes: A pilot study. BMC Oral Health 20 (1), 219. doi:10.1186/s12903-020-01205-4
Cucchi, A., Giavatto, M. A., Giannatiempo, J., Lizio, G., and Corinaldesi, G. (2019). Custom-Made titanium mesh for maxillary bone augmentation with immediate implants and delayed loading. J. oral Implant. 45 (1), 59–64. doi:10.1563/aaid-joi-D-18-00141
Cucchi, A., Vignudelli, E., Franceschi, D., Randellini, E., Lizio, G., Fiorino, A., et al. (2021). Vertical and horizontal ridge augmentation using customized CAD/CAM titanium mesh with versus without resorbable membranes. A randomized clinical trial. Clin. Oral Implan. Res. 32 (12), 1411–1424. doi:10.1111/clr.13841
Dalal, N., Ammoun, R., Abdulmajeed, A. A., Deeb, G. R., and Bencharit, S. (2020). Intaglio surface dimension and guide tube deviations of implant surgical guides influenced by printing layer thickness and angulation setting. J. Prosthodont. 29 (2), 161–165. doi:10.1111/jopr.13138
Dantas, T., Madeira, S., Gasik, M., Vaz, P., and Silva, F. (2021). Customized root-analogue implants: A review on outcomes from clinical trials and case reports. Materials 14 (9), 2296. doi:10.3390/ma14092296
Dawood, A., Marti, B. M., Sauret-Jackson, V., and Darwood, A. (2015). 3D printing in dentistry. Brit. Dent. J. 219 (11), 521–529. doi:10.1038/sj.bdj.2015.914
de Oliveira Campos, F., Araujo, A. C., Jardini Munhoz, A. L., and Kapoor, S. G. (2020). The influence of additive manufacturing on the micromilling machinability of Ti6Al4V: A comparison of SLM and commercial workpieces. J. Manuf. Process. 60, 299–307. doi:10.1016/j.jmapro.2020.10.006
Elliott, T., Hamilton, A., Griseto, N., and Gallucci, G. O. (2022). Additively manufactured surgical implant guides: A review. J. Prosthodont. 31 (S1), 38–46. doi:10.1111/jopr.13476
Fabris, D., Moura, J. P. A., Fredel, M. C., Souza, J. C. M., Silva, F. S., and Henriques, B. (2022). Biomechanical analyses of one-piece dental implants composed of titanium, zirconia,PEEK,CFR-PEEK, orGFR-PEEK: Stresses, strains, and bone remodeling prediction by the finite element method. J. Biomed. Mater. Res. Part B Appl. Biomaterials 110 (1), 79–88. doi:10.1002/jbm.b.34890
Figliuzzi, M., Mangano, F., and Mangano, C. (2012). A novel root analogue dental implant using CT scan and CAD/CAM: Selective laser melting technology. Int. J. Oral Max. Surg. 41 (7), 858–862. doi:10.1016/j.ijom.2012.01.014
Fotopoulos, I., Lillis, T., Panagiotidou, E., Kapagiannidis, I., Nazaroglou, I., and Dabarakis, N. (2022). Accuracy of dental implant placement with 3D-printed surgical templates by using Implant Studio and MGUIDE. An observational study. Int. J. Comput. Dent. 25 (3), 249–256. doi:10.3290/j.ijcd.b2599735
Gargallo-Albiol, J., Zilleruelo-Pozo, M. J., Lucas-Taulé, E., Muñoz-Peñalver, J., Paternostro-Betancourt, D., and Hernandez-Alfaro, F. (2022). Accuracy of static fully guided implant placement in the posterior area of partially edentulous jaws: A cohort prospective study. Clin. Oral Invest. 26 (3), 2783–2791. doi:10.1007/s00784-021-04254-3
Gehrke, S. A., Pérez-Díaz, L., and Dedavid, B. A. (2018). Quasi-static strength and fractography analysis of two dental implants manufactured by direct metal laser sintering. Clin. Implant Dent. R. 20 (3), 368–374. doi:10.1111/cid.12590
Gjelvold, B., Mahmood, D., and Wennerberg, A. (2019). Accuracy of surgical guides from 2 different desktop 3D printers for computed tomography-guided surgery. J. Prosthet. Dent. 121 (3), 498–503. doi:10.1016/j.prosdent.2018.08.009
Han, X., Sharma, N., Spintzyk, S., Zhou, Y., Xu, Z., Thieringer, F. M., et al. (2022). Tailoring the biologic responses of 3D printed PEEK medical implants by plasma functionalization. Dent. Mat. 38 (7), 1083–1098. doi:10.1016/j.dental.2022.04.026
Han, X., Sharma, N., Xu, Z., Scheideler, L., Geis-Gerstorfer, J., Rupp, F., et al. (2019a). An in vitro study of osteoblast response on Fused-Filament fabrication 3D printed PEEK for dental and Cranio-Maxillofacial implants. J. Clin. Med. 8 (6), 771. doi:10.3390/jcm8060771
Han, X., Yang, D., Yang, C., Spintzyk, S., Scheideler, L., Li, P., et al. (2019b). Carbon fiber reinforced PEEK composites based on 3D-Printing technology for orthopedic and dental applications. J. Clin. Med. 8 (2), 240. doi:10.3390/jcm8020240
Hartmann, A., Hildebrandt, H., Schmohl, J. U., and Kämmerer, P. W. (2019). Evaluation of risk parameters in bone regeneration using a customized titanium mesh: Results of a clinical study. Implant Dent. 28, 543–550. Publish Ahead of Print. doi:10.1097/ID.0000000000000933
Hartmann, A., and Seiler, M. (2020). Minimizing risk of customized titanium mesh exposures – A retrospective analysis. BMC Oral Health 20 (1), 36. doi:10.1186/s12903-020-1023-y
Hazeveld, A., Huddleston Slater, J. J. R., and Ren, Y. (2014). Accuracy and reproducibility of dental replica models reconstructed by different rapid prototyping techniques. Am. J. Orthod. Dentofac. 145 (1), 108–115. doi:10.1016/j.ajodo.2013.05.011
Henprasert, P., Dawson, D. V., El Kerdani, T., Song, X., Couso Queiruga, E., and Holloway, J. A. (2020). Comparison of the accuracy of implant position using surgical guides fabricated by additive and subtractive techniques. J. Prosthodont. 29 (6), 534–541. doi:10.1111/jopr.13161
Herschdorfer, L., Negreiros, W. M., Gallucci, G. O., and Hamilton, A. (2021). Comparison of the accuracy of implants placed with CAD-CAM surgical templates manufactured with various 3D printers: An in vitro study. J. Prosthet. Dent. 125 (6), 905–910. doi:10.1016/j.prosdent.2020.03.017
Huang, G., Wu, L., Hu, J., Zhou, X., He, F., Wan, L., et al. (2022). Main applications and recent research progresses of additive manufacturing in dentistry. Biomed. Res. Int. 2022, 1–26. doi:10.1155/2022/5530188
Huang, Y., Zhang, X., Gao, G., Yonezawa, T., and Cui, X. (2017). 3D bioprinting and the current applications in tissue engineering. Biotechnol. J. 12 (8), 1600734. doi:10.1002/biot.201600734
Jawahar, A., and Maragathavalli, G. (2019). Applications of 3D printing in dentistry – A review. J. Pharm. Sci. Res. 11 (5), 1670–1675.
Jung, G., Jeon, J., Hwang, K., and Park, C. (2014). Preliminary evaluation of a three-dimensional, customized, and preformed titanium mesh in peri-implant alveolar bone regeneration. J. Korean Assoc. Oral Maxillofac. Surg. 40 (4), 181. doi:10.5125/jkaoms.2014.40.4.181
Jung, H., Jang, T., Lee, J. E., Park, S. J., Son, Y., and Park, S. (2019). Enhanced bioactivity of titanium-coated polyetheretherketone implants created by a high-temperature 3D printing process. Biofabrication 11 (4), 045014. doi:10.1088/1758-5090/ab376b
Kapos, T., and Evans, C. (2014). CAD/CAM technology for implant abutments, crowns, and superstructures. Int. J. oral Maxillofac. implants 29, 117–136. doi:10.11607/jomi.2014suppl.g2.3
Kessler, A., Hickel, R., and Reymus, M. (2020). 3D printing in dentistry—State of the art. Oper. Dent. 45 (1), 30–40. doi:10.2341/18-229-L
Khorsandi, D., Fahimipour, A., Abasian, P., Saber, S. S., Seyedi, M., Ghanavati, S., et al. (2021). 3D and 4D printing in dentistry and maxillofacial surgery: Printing techniques, materials, and applications. Acta Biomater. 122, 26–49. doi:10.1016/j.actbio.2020.12.044
Kim, J. E., Kwon, D. H., Kim, J. H., and Shim, J. S. (2019a). A digital implant custom tray fabrication method using the design process for simulating the position of the impression copings and 3D printing technology. J. Prosthet. Dent. 121 (4), 566–570. doi:10.1016/j.prosdent.2018.07.005
Kim, J. E., Park, J. H., Kim, J. H., and Shim, J. S. (2019b). Computer-based implant planning involving a prefabricated custom tray with alumina landmark structures. J. Prosthet. Dent. 121 (3), 373–377. doi:10.1016/j.prosdent.2018.06.002
Kim, S., Shin, Y., Jung, H., Hwang, C., Baik, H., and Cha, J. (2018). Precision and trueness of dental models manufactured with different 3-dimensional printing techniques. Am. J. Orthod. Dentofac. 153 (1), 144–153. doi:10.1016/j.ajodo.2017.05.025
Kirschner, A., David, S., Brunello, G., Keilig, L., Drescher, D., Bourauel, C., et al. (2022). Impact of steam autoclaving on the mechanical properties of 3D-Printed resins used for insertion guides in orthodontics and implant dentistry. Appl. Sci. 12 (12), 6195. doi:10.3390/app12126195
Koch, G. K., James, B., Gallucci, G. O., and Hamilton, A. (2019). Surgical template fabrication using Cost-Effective 3D printers. Int. J. Prosthodont. 32 (1), 97–100. doi:10.11607/ijp.5975
Li, L., Wang, C., Li, X., Fu, G., Chen, D., and Huang, Y. (2021). Research on the dimensional accuracy of customized bone augmentation combined with3D -printing individualized titanium mesh: A retrospective case series study. Clin. Implant Dent. R. 23 (1), 5–18. doi:10.1111/cid.12966
Li, S., Zhang, T., Zhou, M., Zhang, X., Gao, Y., and Cai, X. (2021). A novel digital and visualized guided bone regeneration procedure and digital precise bone augmentation: A case series. Clin. Implant Dent. R. 23 (1), 19–30. doi:10.1111/cid.12959
Lin, L., Fang, Y., Liao, Y., Chen, G., Gao, C., and Zhu, P. (2019). 3D printing and digital processing techniques in dentistry: A review of literature. Adv. Eng. Mat. 21 (6), 1801013. doi:10.1002/adem.201801013
Liu, Y., Bai, W., Cheng, X., Tian, J., Wei, D., Sun, Y., et al. (2021). Effects of printing layer thickness on mechanical properties of 3D-printed custom trays. J. Prosthet. Dent. 126 (5), 671.e1–671.e7. doi:10.1016/j.prosdent.2020.08.025
Liu, Y., Di, P., Zhao, Y., Hao, Q., Tian, J., and Cui, H. (2019). Accuracy of multi-implant impressions using 3D-printing custom trays and splinting versus conventional techniques for complete arches. Int. J. Oral Maxillofac. Implants 34 (4), 1007–1014. doi:10.11607/jomi.7049
Lou, F., Rao, P., Zhang, M., Luo, S., Lu, S., and Xiao, J. (2021). Accuracy evaluation of partially guided and fully guided templates applied to implant surgery of anterior teeth: A randomized controlled trial. Clin. Implant Dent. R. 23 (1), 117–130. doi:10.1111/cid.12980
Mangano, C., Mangano, F. G., Shibli, J. A., Ricci, M., Perrotti, V., D'Avila, S., et al. (2012a). Immediate loading of mandibular overdentures supported by unsplinted direct laser Metal-Forming implants: Results from a 1-Year prospective study. J. Periodontol. 83 (1), 70–78. doi:10.1902/jop.2011.110079
Mangano, C., Mangano, F., Shibli, J. A., Luongo, G., De Franco, M., Briguglio, F., et al. (2012b). Prospective clinical evaluation of 201 direct laser metal forming implants: Results from a 1-year multicenter study. Laser. Med. Sci. 27 (1), 181–189. doi:10.1007/s10103-011-0904-3
Mangano, F., Chambrone, L., van Noort, R., Miller, C., Hatton, P., and Mangano, C. (2014a2014). Direct metal laser sintering titanium dental implants: A review of the current literature. Int. J. Biomaterials 2014, 1–11. doi:10.1155/2014/461534
Mangano, F. G., Caprioglio, A., Levrini, L., Farronato, D., Zecca, P. A., and Mangano, C. (2015). Immediate loading of mandibular overdentures supported by one-piece, direct metal laser sintering mini-implants: A short-term prospective clinical study. J. Periodontol. 86 (2), 192–200. doi:10.1902/jop.2014.140343
Mangano, F. G., De Franco, M., Caprioglio, A., Macchi, A., Piattelli, A., and Mangano, C. (2013a). Immediate, non-submerged, root-analogue direct laser metal sintering (dlms) implants: A 1-year prospective study on 15 patients. Laser. Med. Sci. 29, 1321–1328. doi:10.1007/s10103-013-1299-0
Mangano, F., Hauschild, U., and Admakin, O. (2018). Full in-office guided surgery with open selective tooth-supported templates: A prospective clinical study on 20 patients. Int. J. Env. Res. Pub. He. 15 (11), 2361. doi:10.3390/ijerph15112361
Mangano, F., Luongo, F., Shibli, J. A., Anil, S., and Mangano, C. (2014b). Maxillary overdentures supported by four splinted direct metal laser sintering implants: A 3-year prospective clinical study. Int. J. Dent. 2014, 1–9. doi:10.1155/2014/252343
Mangano, F., Pozzi-Taubert, S., Zecca, P. A., Luongo, G., Sammons, R. L., and Mangano, C. (2013b). Immediate restoration of fixed partial prostheses supported by One-Piece Narrow-Diameter selective laser sintering implants. Implant Dent. 22 (4), 388–393. doi:10.1097/ID.0b013e31829afa9d
Manzano Romero, P., Vellone, V., Maffia, F., and Cicero, G. (2021). Customized surgical protocols for guided bone regeneration using 3D printing technology: A retrospective clinical trial. J. Craniofac. Surg. 32 (2), e198–e202. doi:10.1097/SCS.0000000000007081
Marei, H. F., Alshaia, A., Alarifi, S., Almasoud, N., and Abdelhady, A. (2019). Effect of steam heat sterilization on the accuracy of 3D printed surgical guides. Implant Dent. 28 (4), 372–377. doi:10.1097/ID.0000000000000908
Maria, R., Tan, M. Y., Wong, K. M., Lee, B. C. H., Chia, V. A. P., and Tan, K. B. C. (2021). Accuracy of implant analogs in 3D printed resin models. J. Prosthodont. 30 (1), 57–64. doi:10.1111/jopr.13217
Michelinakis, G., Apostolakis, D., Kamposiora, P., Papavasiliou, G., and Özcan, M. (2021). The direct digital workflow in fixed implant prosthodontics: A narrative review. BMC Oral Health 21 (1), 37. doi:10.1186/s12903-021-01398-2
Moby, V., Dupagne, L., Fouquet, V., Attal, J., François, P., and Dursun, E. (2022). Mechanical properties of fused deposition modeling of polyetheretherketone (PEEK) and interest for dental restorations: A systematic review. Materials 15 (19), 6801. doi:10.3390/ma15196801
Moin, D. A., Hassan, B., Mercelis, P., and Wismeijer, D. (2013). Designing a novel dental root analogue implant using cone beam computed tomography and CAD/CAM technology. Clin. Oral Implan. Res. 24, 25–27. doi:10.1111/j.1600-0501.2011.02359.x
Monaco, C., Scheda, L., Ciocca, L., and Zucchelli, G. (2018). The prototype concept in a full digital implant workflow. J. Am. Dent. Assoc. 149 (10), 918–923. doi:10.1016/j.adaj.2018.04.026
Mukai, S., Mukai, E., Santos-Junior, J. A., Shibli, J. A., Faveri, M., and Giro, G. (2021). Assessment of the reproducibility and precision of milling and 3D printing surgical guides. BMC Oral Health 21 (1), 1. doi:10.1186/s12903-020-01362-6
Najeeb, S., Zafar, M. S., Khurshid, Z., and Siddiqui, F. (2016). Applications of polyetheretherketone (PEEK) in oral implantology and prosthodontics. J. Prosthodont. Res. 60 (1), 12–19. doi:10.1016/j.jpor.2015.10.001
Nikoyan, L., and Patel, R. (2020). Intraoral scanner, Three-Dimensional imaging, and Three-Dimensional printing in the dental office. Dent. Clin. N. Am. 64 (2), 365–378. doi:10.1016/j.cden.2019.12.004
Oberoi, G., Nitsch, S., Edelmayer, M., Janjić, K., Müller, A. S., and Agis, H. (2018). 3D printing—Encompassing the facets of dentistry. Front. Bioeng. Biotechnol. 6, 172. doi:10.3389/fbioe.2018.00172
Olea Vielba, M., Jareño García, D., Methani, M. M., Martinez Klemm, I., and Revilla León, M. (2020). Accuracy of the implant replica positions on the complete edentulous additive manufactured cast. J. Prosthodont. 29 (9), 780–786. doi:10.1111/jopr.13179
Oliveira, T. T., and Reis, A. C. (2019). Fabrication of dental implants by the additive manufacturing method: A systematic review. J. Prosthet. Dent. 122 (3), 270–274. doi:10.1016/j.prosdent.2019.01.018
Otawa, N., Sumida, T., Kitagaki, H., Sasaki, K., Fujibayashi, S., Takemoto, M., et al. (2015). Custom-made titanium devices as membranes for bone augmentation in implant treatment: Modeling accuracy of titanium products constructed with selective laser melting. J. Craniomaxillofac Surg. 43 (7), 1289–1295. doi:10.1016/j.jcms.2015.05.006
Pagac, M., Hajnys, J., Ma, Q., Jancar, L., Jansa, J., Stefek, P., et al. (2021). A review of vat photopolymerization technology: Materials, applications, challenges, and future trends of 3D printing. Polymers-Basel. 13 (4), 598. doi:10.3390/polym13040598
Pan, Y., Tu, Y., Wang, T., Liang, J., and Lin, H. (2022). Clinical study of precision analysis and deviation control of a domestic guide plate-assisted edentulous implant surgery. J. Stomatol. Oral Maxillofac. Surg. 2022, 101328. doi:10.1016/j.jormas.2022.11.004
Panayotov, I. V., Orti, V., Cuisinier, F., and Yachouh, J. (2016). Polyetheretherketone (PEEK) for medical applications. J. Mater. Sci. Mater. Med. 27 (7), 118. doi:10.1007/s10856-016-5731-4
Papaspyridakos, P., Chen, Y. W., Alshawaf, B., Kang, K., Finkelman, M., Chronopoulos, V., et al. (2020). Digital workflow: In vitro accuracy of 3D printed casts generated from complete-arch digital implant scans. J. Prosthet. Dent. 124 (5), 589–593. doi:10.1016/j.prosdent.2019.10.029
Park, J. M., Jeon, J., Koak, J. Y., Kim, S. K., and Heo, S. J. (2021). Dimensional accuracy and surface characteristics of 3D-printed dental casts. J. Prosthet. Dent. 126 (3), 427–437. doi:10.1016/j.prosdent.2020.07.008
Patpatiya, P., Chaudhary, K., Shastri, A., and Sharma, S. (2022). A review on polyjet 3D printing of polymers and multi-material structures. Proc. Institution Mech. Eng. Part C J. Mech. Eng. Sci. 236 (14), 7899–7926. doi:10.1177/09544062221079506
Pessoa, R., Siqueira, R., Li, J., Saleh, I., Meneghetti, P., Bezerra, F., et al. (2022). The impact of surgical guide fixation and implant location on accuracy of static computer-assisted implant surgery. J. Prosthodont. 31 (2), 155–164. doi:10.1111/jopr.13371
Petersmann, S., Spoerk, M., Van De Steene, W., Üçal, M., Wiener, J., Pinter, G., et al. (2020). Mechanical properties of polymeric implant materials produced by extrusion-based additive manufacturing. J. Mech. Behav. Biomed. 104, 103611. doi:10.1016/j.jmbbm.2019.103611
Petrick, I. J., and Simpson, T. W. (2015). 3D printing disrupts manufacturing: How economies of one create new rules of competition. Res. Technol. Manage. 56 (6), 12–16. doi:10.5437/08956308X5606193
Piedra, C. W., and Revilla-Leon, M. (2018). Digital workflow for the design and additively manufacture of a splinted framework and custom tray for the impression of multiple implants: A dental technique. J. Prosthet. Dent. 120 (6), 805–811. doi:10.1016/j.prosdent.2018.02.003
Pieralli, S., Spies, B. C., Hromadnik, V., Nicic, R., Beuer, F., and Wesemann, C. (2020). How accurate is oral implant installation using surgical guides printed from a degradable and Steam-Sterilized biopolymer? J. Clin. Med. 9 (8), 2322. doi:10.3390/jcm9082322
Pillai, S., Upadhyay, A., Khayambashi, P., Farooq, I., Sabri, H., Tarar, M., et al. (2021). Dental 3D-printing: Transferring art from the laboratories to the clinics. Polymers-Basel 13 (1), 157. doi:10.3390/polym13010157
Prechtel, A., Reymus, M., Edelhoff, D., Hickel, R., and Stawarczyk, B. (2020). Comparison of various 3D printed and milled PAEK materials: Effect of printing direction and artificial aging on Martens parameters. Dent. Mat. 36 (2), 197–209. doi:10.1016/j.dental.2019.11.017
Putra, R. H., Yoda, N., Astuti, E. R., and Sasaki, K. (2022). The accuracy of implant placement with computer-guided surgery in partially edentulous patients and possible influencing factors: A systematic review and meta-analysis. J. Prosthodont. Res. 66 (1), 29–39. doi:10.2186/jpr.JPR_D_20_00184
Raheem, A. A., Hameed, P., Whenish, R., Elsen, R. S., Aswin, G., Jaiswal, A. K., et al. (2021). A review on development of bio-inspired implants using 3D printing. Biomimetics 6 (4), 65. doi:10.3390/biomimetics6040065
Revilla-Leon, M., Ceballos, L., Martinez-Klemm, I., and Ozcan, M. (2018a). Discrepancy of complete-arch titanium frameworks manufactured using selective laser melting and electron beam melting additive manufacturing technologies. J. Prosthet. Dent. 120 (6), 942–947. doi:10.1016/j.prosdent.2018.02.010
Revilla-Leon, M., Ceballos, L., and Ozcan, M. (2019a). Implant-prosthodontic discrepancy of complete-arch cobalt-chromium implant frameworks manufactured through selective laser melting additive manufacturing technology using a coordinate measuring machine. Int. J. Oral Maxillofac. Implants 34 (3), 698–707. doi:10.11607/jomi.6739
Revilla-Leon, M., Gonzalez-Martin, O., Perez, L. J., Sanchez-Rubio, J. L., and Ozcan, M. (2018b). Position accuracy of implant analogs on 3D printed polymer versus conventional dental stone casts measured using a coordinate measuring machine. J. Prosthodont. 27 (6), 560–567. doi:10.1111/jopr.12708
Revilla-Leon, M., Meyer, M. J., and Ozcan, M. (2019b). Metal additive manufacturing technologies: Literature review of current status and prosthodontic applications. Int. J. Comput. Dent. 22 (1), 55–67.
Revilla-León, M., and Özcan, M. (2017). Additive manufacturing technologies used for 3D metal printing in dentistry. Curr. Oral Health Rep. 4 (3), 201–208. doi:10.1007/s40496-017-0152-0
Revilla-Leon, M., and Ozcan, M. (2019). Additive manufacturing technologies used for processing polymers: Current status and potential application in prosthetic dentistry. J. Prosthodont. 28 (2), 146–158. doi:10.1111/jopr.12801
Revilla-León, M., Sadeghpour, M., and Özcan, M. (2020). An update on applications of 3D printing technologies used for processing polymers used in implant dentistry. Odontology 108 (3), 331–338. doi:10.1007/s10266-019-00441-7
Revilla-Leon, M., Sanchez-Rubio, J. L., Oteo-Calatayud, J., and Ozcan, M. (2017). Impression technique for a complete-arch prosthesis with multiple implants using additive manufacturing technologies. J. Prosthet. Dent. 117 (6), 714–720. doi:10.1016/j.prosdent.2016.08.036
Revilla-Leon, M., Sanchez-Rubio, J. L., Perez-Lopez, J., Rubenstein, J., and Ozcan, M. (2021). Discrepancy at the implant abutment-prosthesis interface of complete-arch cobalt-chromium implant frameworks fabricated by additive and subtractive technologies before and after ceramic veneering. J. Prosthet. Dent. 125 (5), 795–803. doi:10.1016/j.prosdent.2020.03.018
Rouzé L'Alzit, F., Cade, R., Naveau, A., Babilotte, J., Meglioli, M., and Catros, S. (2022). Accuracy of commercial 3D printers for the fabrication of surgical guides in dental implantology. J. Dent. 117, 103909. doi:10.1016/j.jdent.2021.103909
Rubayo, D. D., Phasuk, K., Vickery, J. M., Morton, D., and Lin, W. S. (2021). Influences of build angle on the accuracy, printing time, and material consumption of additively manufactured surgical templates. J. Prosthet. Dent. 126 (5), 658–663. doi:10.1016/j.prosdent.2020.09.012
Rungrojwittayakul, O., Kan, J. Y., Shiozaki, K., Swamidass, R. S., Goodacre, B. J., Goodacre, C. J., et al. (2020). Accuracy of 3D printed models created by two technologies of printers with different designs of model base. J. Prosthodont. 29 (2), 124–128. doi:10.1111/jopr.13107
Sagheb, K., Schiegnitz, E., Moergel, M., Walter, C., Al-Nawas, B., and Wagner, W. (2017). Clinical outcome of alveolar ridge augmentation with individualized CAD-CAM-produced titanium mesh. Int. J. Implant Dent. 3 (1), 36. doi:10.1186/s40729-017-0097-z
Salmi, M. (2021). Additive manufacturing processes in medical applications. Materials 14 (1), 191. doi:10.3390/ma14010191
Schmidt, M., Pohle, D., and Rechtenwald, T. (2007). Selective laser sintering of PEEK. CIRP Ann. 56 (1), 205–208. doi:10.1016/j.cirp.2007.05.097
Schweiger, J., Edelhoff, D., and Güth, J. (2021). 3D printing in digital prosthetic dentistry: An overview of recent developments in additive manufacturing. J. Clin. Med. 10 (9), 2010. doi:10.3390/jcm10092010
Sedov, Y. G., Avanesov, A. M., Saleev, R. A., Saleeva, G. T., and Yarulina, Z. I. (2021). A classification of surgical guides application for dental implantation. Stomatologiya 100 (1), 84. doi:10.17116/stomat202110001184
Seiler, M., Kammerer, P. W., Peetz, M., and Hartmann, A. (2018). Customized lattice structure in reconstruction of three-dimensional alveolar defects. Int. J. Comput. Dent. 21 (3), 261–267.
Shilov, S. Y., Rozhkova, Y. A., Markova, L. N., Tashkinov, M. A., Vindokurov, I. V., and Silberschmidt, V. V. (2022). Biocompatibility of 3D-Printed PLA, PEEK and PETG: Adhesion of bone marrow and peritoneal lavage cells. Polymers-Basel 14 (19), 3958. doi:10.3390/polym14193958
Shishkovsky, I., Volchkov, S., Veiko, V. P., and Vartanyan, T. A. (2013). “Influence of the laser assisted fabricated 3D porous scaffolds from bioceramoplasts of micron and nano sizes on culture of MMSC,” in Proc. SPIE 9065, Fundamentals of Laser-Assisted Micro- and Nanotechnologies 2013, St. Petersburg. Russia, June 24-28. 2013. Paper presented at the.
Sing, S. L., An, J., Yeong, W. Y., and Wiria, F. E. (2016). Laser and electron-beam powder-bed additive manufacturing of metallic implants: A review on processes, materials and designs. J. Orthop. Res. 34 (3), 369–385. doi:10.1002/jor.23075
Sommacal, B., Savic, M., Filippi, A., Kühl, S., and Thieringer, F. M. (2018). Evaluation of two 3D printers for guided implant surgery. Int. J. oral Maxillofac. implants 33 (4), 743–746. doi:10.11607/jomi.6074
Son, K., Lee, J., and Lee, K. (2021). Comparison of intaglio surface trueness of interim dental crowns fabricated with SLA 3D printing, DLP 3D printing, and milling technologies. Healthcare 9 (8), 983. doi:10.3390/healthcare9080983
Sonaye, S. Y., Bokam, V. K., Saini, A., Nayak, V. V., Witek, L., Coelho, P. G., et al. (2022). Patient-specific 3D printed Poly-ether-ether-ketone (PEEK) dental implant system. J. Mech. Behav. Biomed. 136, 105510. doi:10.1016/j.jmbbm.2022.105510
Su, Y., He, J., Jiang, N., Zhang, H., Wang, L., Liu, X., et al. (2020). Additively-manufactured poly-ether-ether-ketone (PEEK) lattice scaffolds with uniform microporous architectures for enhanced cellular response and soft tissue adhesion. Mater. Des. 191, 108671. doi:10.1016/j.matdes.2020.108671
Sumida, T., Otawa, N., Kamata, Y. U., Kamakura, S., Mtsushita, T., Kitagaki, H., et al. (2015). Custom-made titanium devices as membranes for bone augmentation in implant treatment: Clinical application and the comparison with conventional titanium mesh. J. Cranio Maxill. Surg. 43 (10), 2183–2188. doi:10.1016/j.jcms.2015.10.020
Sun, C., Kang, J., Yang, C., Zheng, J., Su, Y., Dong, E., et al. (2022). Additive manufactured polyether-ether-ketone implants for orthopaedic applications: A narrative review. Biomater. Transl. 3 (2), 116–133. doi:10.12336/biomatertransl.2022.02.001
Sun, Y., Chen, H., Li, H., Deng, K., Zhao, T., Wang, Y., et al. (2017). Clinical evaluation of final impressions from three-dimensional printed custom trays. Sci. Rep.-UK 7 (1), 14958. doi:10.1038/s41598-017-14005-8
Sun, Y., Ding, Q., Tang, L., Zhang, L., Sun, Y. C., and Xie, Q. F. (2019). Accuracy of a chairside fused deposition modeling 3D-printed single-tooth surgical template for implant placement: An in vitro comparison with a light cured template. J. Cranio Maxill. Surg. 47 (8), 1216–1221. doi:10.1016/j.jcms.2019.03.019
Sun, Y., Ding, Q., Yuan, F., Zhang, L., Sun, Y., and Xie, Q. (2022). Accuracy of a chairside, fused deposition modeling three-dimensional-printed, single tooth surgical guide for implant placement: A randomized controlled clinical trial. Clin. Oral Implan. Res. 33 (10), 1000–1009. doi:10.1111/clr.13981
Svanborg, P., Eliasson, A., Stenport, V., Sahlgrenska, A., Göteborgs, U., Gothenburg, U., et al. (2018). Additively manufactured titanium and Cobalt-Chromium implant frameworks: Fit and effect of ceramic veneering. Int. J. oral Maxillofac. implants 33 (3), 590–596. doi:10.11607/jomi.6028
Tahir, N., and Abduo, J. (2022). An in vitro evaluation of the effect of 3D printing orientation on the accuracy of implant surgical templates fabricated by desktop printer. J. Prosthodont. 31, 791–798. doi:10.1111/jopr.13485
Tappa, K., and Jammalamadaka, U. (2018). Novel biomaterials used in medical 3D printing techniques. J. Funct. Biomaterials 9 (1), 17. doi:10.3390/jfb9010017
Taymour, N., Fahmy, A. E., Gepreel, M. A. H., Kandil, S., and El-Fattah, A. A. (2022). Improved mechanical properties and bioactivity of silicate based bioceramics reinforced poly(ether-ether-ketone) nanocomposites for prosthetic dental implantology. Polymers-Basel. 14 (8), 1632. doi:10.3390/polym14081632
Thakur, J., Parlani, S., Shivakumar, S., and Jajoo, K. (2021). Accuracy of marginal fit of an implant-supported framework fabricated by 3D printing versus subtractive manufacturing technique: A systematic review and meta-analysis. J. Prosthet. Dent. [Epub ahead of print]. doi:10.1016/j.prosdent.2021.05.010
Tian, Y., Chen, C., Xu, X., Wang, J., Hou, X., Li, K., et al. (2021). A review of 3D printing in dentistry: Technologies, affecting factors, and applications. Scanning 2021, 1–19. doi:10.1155/2021/9950131
Torabi, K., Farjood, E., and Hamedani, S. (2015). Rapid prototyping technologies and their applications in prosthodontics, a review of literature. J. Dent. (Shiraz). 16 (1), 1–9.
Torstrick, F. B., Lin, A. S. P., Potter, D., Safranski, D. L., Sulchek, T. A., Gall, K., et al. (2018). Porous PEEK improves the bone-implant interface compared to plasma-sprayed titanium coating on PEEK. Biomaterials 185, 106–116. doi:10.1016/j.biomaterials.2018.09.009
Tunchel, S., Blay, A., Kolerman, R., Mijiritsky, E., and Shibli, J. A. (2016). 3D printing/additive manufacturing single titanium dental implants: A prospective multicenter study with 3 years of follow-up. Int. J. Dent. 2016, 1–9. doi:10.1155/2016/8590971
Turkyilmaz, I., and Wilkins, G. N. (2021). 3D printing in dentistry – exploring the new horizons. J. Dent. Sci. 16 (3), 1037–1038. doi:10.1016/j.jds.2021.04.004
Unsal, G., Turkyilmaz, I., and Lakhia, S. (2020). Advantages and limitations of implant surgery with CAD/CAM surgical guides: A literature review. J. Clin. Exp. Dent. 12, e409–e417. doi:10.4317/jced.55871
Velasquez-Garcia, L. F., and Kornbluth, Y. (2021). Biomedical applications of metal 3D printing. Annu. Rev. Biomed. Eng. 23, 307–338. doi:10.1146/annurev-bioeng-082020-032402
Wally, Z. J., Haque, A. M., Feteira, A., Claeyssens, F., Goodall, R., and Reilly, G. C. (2019). Selective laser melting processed Ti6Al4V lattices with graded porosities for dental applications. J. Mech. Behav. Biomed. 90, 20–29. doi:10.1016/j.jmbbm.2018.08.047
Wegmüller, L., Halbeisen, F., Sharma, N., Kühl, S., and Thieringer, F. M. (2021). Consumer vs. High-end 3D printers for guided implant surgery—An in vitro accuracy assessment study of different 3D printing technologies. J. Clin. Med. 10 (21), 4894. doi:10.3390/jcm10214894
Westover, B. (2019). Three-Dimensional Custom-Root replicate tooth dental implants. Oral Maxil. Surg. Clin. 31 (3), 489–496. doi:10.1016/j.coms.2019.03.010
Xie, Y., Li, S., Zhang, T., Wang, C., and Cai, X. (2020). Titanium mesh for bone augmentation in oral implantology: Current application and progress. Int. J. Oral Sci. 12 (1), 37. doi:10.1038/s41368-020-00107-z
Xu, Y., Huettig, F., Schille, C., Schweizer, E., Geis-Gerstorfer, J., and Spintzyk, S. (2020). Peel bond strength between 3D printing tray materials and elastomeric impression/adhesive systems: A laboratory study. Dent. Mat. 36 (7), e241–e254. doi:10.1016/j.dental.2020.04.015
Yang, Z., Xu, Y., Sisson, R. D., and Liang, J. (2020). Factors influencing the corrosion behavior of direct metal laser sintered Ti-6Al-4V for biomedical applications. J. Mat. Eng. Perform. 29 (6), 3831–3839. doi:10.1007/s11665-020-04904-9
Yousef, H., Harris, B. T., Elathamna, E. N., Morton, D., and Lin, W. S. (2021). Effect of additive manufacturing process and storage condition on the dimensional accuracy and stability of 3D-printed dental casts. J. Prosthet. Dent. 128, 1041–1046. doi:10.1016/j.prosdent.2021.02.028
Yu, T., Gao, H., Liu, T., Huang, Y., and Wang, C. (2020). Effects of immediately static loading on osteointegration and osteogenesis around 3D-printed porous implant: A histological and biomechanical study. Mater. Sci. Eng. C 108, 110406. doi:10.1016/j.msec.2019.110406
Yuan, B., Cheng, Q., Zhao, R., Zhu, X., Yang, X., Yang, X., et al. (2018). Comparison of osteointegration property between PEKK and PEEK: Effects of surface structure and chemistry. Biomaterials 170, 116–126. doi:10.1016/j.biomaterials.2018.04.014
Zhang, C., Yuan, Y., and Chen, J. (2022). Material extrusion based fabrication of surgical implant template and accuracy analysis. Materials 15 (5), 1738. doi:10.3390/ma15051738
Zhang, F., Gao, X., Ye, Z. Y., Xu, D. Q., and Ding, X. (2020). The clinical accuracy of the implant digital surgical guide: A meta-analysis. Am. J. Dent. 33 (6), 296–304.
Zhou, L., Su, Y., Wang, J., Wang, J., Wang, X., and Liu, Q. (2021). Effect of exposure rates with customized versus conventional titanium mesh on guided bone regeneration: Systematic review and meta-analysis. J. Oral Implantol. 48, 339–346. doi:10.1563/aaid-joi-D-20-00200
Zhou, W., Liu, Z., Song, L., Kuo, C. L., and Shafer, D. M. (2018). Clinical factors affecting the accuracy of guided implant Surgery-A systematic review and meta-analysis. J. Evid. Based Dent. Pract. 18 (1), 28–40. doi:10.1016/j.jebdp.2017.07.007
Keywords: additive manufacturing technologies, 3D printing, oral implantology, surgical guides, customized titanium meshes, dental implants, custom trays, implant models
Citation: Huang S, Wei H and Li D (2023) Additive manufacturing technologies in the oral implant clinic: A review of current applications and progress. Front. Bioeng. Biotechnol. 11:1100155. doi: 10.3389/fbioe.2023.1100155
Received: 16 November 2022; Accepted: 11 January 2023;
Published: 20 January 2023.
Edited by:
Xing Wang, Shanxi Medical University, ChinaReviewed by:
Yunfeng Liu, Zhejiang University of Technology, ChinaTing Jiao, Shanghai Jiao Tong University, China
Copyright © 2023 Huang, Wei and Li. This is an open-access article distributed under the terms of the Creative Commons Attribution License (CC BY). The use, distribution or reproduction in other forums is permitted, provided the original author(s) and the copyright owner(s) are credited and that the original publication in this journal is cited, in accordance with accepted academic practice. No use, distribution or reproduction is permitted which does not comply with these terms.
*Correspondence: Hongbo Wei, weihongbo101@126.com; Dehua Li, lidehua@fmmu.edu.cn