Super-Resolution Imaging With Lanthanide Luminescent Nanocrystals: Progress and Prospect
- 1Department of Chemistry, Shanghai Key Laboratory of Molecular Catalysis and Innovative Materials, State Key Laboratory of Molecular Engineering of Polymers, iChem, Fudan University, Shanghai, China
- 2Molecular Neuroendocrinology Research Group, Institute of Physiology, Medical School, Centre for Neuroscience, Szentágothai Research Institute, University of Pécs, Pécs, Hungary
Stimulated emission depletion (STED) nanoscopy has overcome a serious diffraction barrier on the optical resolution and facilitated new discoveries on detailed nanostructures in cell biology. Traditional fluorescence probes employed in the super-resolution imaging approach include organic dyes and fluorescent proteins. However, some limitations of these probes, such as photobleaching, short emission wavelengths, and high saturation intensity, still hamper the promotion of optical resolution and bio-applications. Recently, lanthanide luminescent probes with unique optical properties of non-photobleaching and sharp emissions have been applied in super-resolution imaging. In this mini-review, we will introduce several different mechanisms for lanthanide ions to achieve super-resolution imaging based on an STED-like setup. Then, several lanthanide ions used in super-resolution imaging will be described in detail and discussed. Last but not least, we will emphasize the future challenges and outlooks in hope of advancing the next-generation lanthanide fluorescent probes for super-resolution optical imaging.
Introduction
It is often said that seeing is believing. This applies not only to our daily lives but certainly also to the academic research. For centuries, fluorescence microscopy has greatly facilitated our understanding on the spatial organization and interactions of the biological system (Misgeld et al., 2007; Klar et al., 2000). The main indicator to evaluate the performance of optical micro-imaging is the spatial resolution (Hell and Wichmann, 1994; Hell, 2004). However, as we learned in high school, the resolution of a visible light microscope is usually larger than 200 nm due to the diffraction limit theoretically proposed by Ernst Abbe in 1873, making many nanostructures, such as neurons and cytoskeletons in cells, unresolvable (Betzig et al., 2006). Facing the seemingly insurmountable challenge, several advanced and ingenious methods to break through the bottleneck of the optical resolution in microscopy have been invented so far (Gwosch et al., 2020; Berning et al., 2012; Rust et al., 2006; Sharonov and Hochstrasser, 2006; Bates et al., 2008), one of which is named as stimulated emission depletion (STED) microscopy honored by the 2014 Nobel Prize in Chemistry (Hell, 2015; Sahl et al., 2017). In this design (Figure 1A), two excitation laser beams are required: one laser beam with Gaussian intensity distribution is used to excite probes to generate fluorescence. Another donut laser beam, called the depletion laser, is employed to erase fluorescence signals around the focal spot through stimulated emission so as to reduce the size of the region that fluoresces. Then scanning the little light spot across samples will record a super-resolution image (Harke et al., 2008; Eggeling et al., 2009).
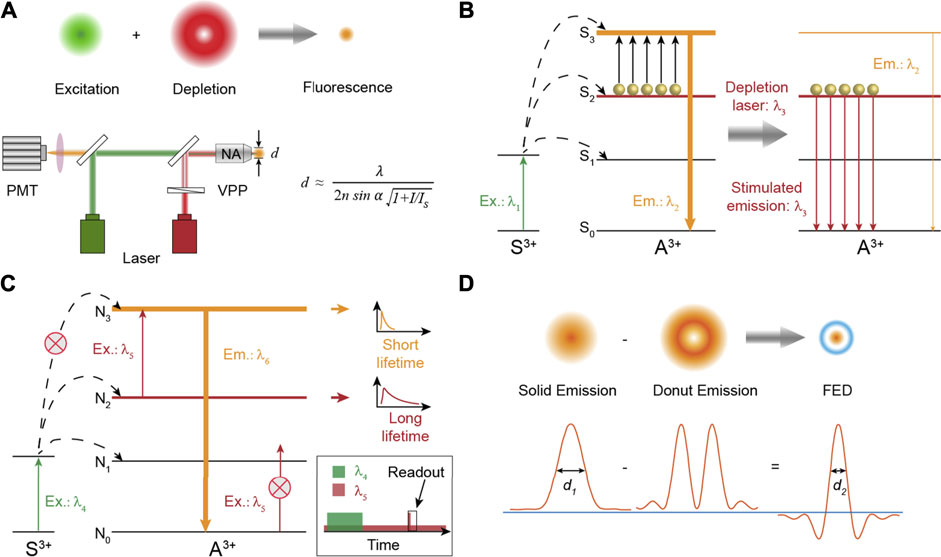
FIGURE 1. (A) Schematic diagram showing the principle of super-resolution imaging and the simplified setup of STED nanoscopy. VPP: vortex phase plate. The formula describes the resolution of STED nanoscopy. d is the diameter of the spot that fluoresces. (B) The energy transfer pathway of typical Tm3+-based nancrystals for super-resolution. Stimulated emission is triggered under a λ3 laser excitation to deplete the population of energy state S3. (C) The energy transfer pathway of the excited state absorption process to achieve super-resolution through separated time–domain detection. (D) Theoretical results of point spread functions obtained in fluorescence emission difference microscopy. The negative values caused by the intensity subtraction are set to zero to ensure a better spatial resolution.
Up until now, the super-resolution imaging of neurons, mitochondria, and cytoskeletons in live cells and live bodies has been realized by STED technology (Eggeling et al., 2009; Sahl et al., 2017; Carola et al., 2018; Masch et al., 2018; Rifka et al., 2018; Grimm et al., 2019; Stephan et al., 2019), in which organic dyes and fluorescent proteins (ATTOs, DAEs, 61CP, and so on) that have the fundamental transition property of stimulated emission are commonly used as fluorescent probes (Kolmakov et al., 2015; Butkevich et al., 2018; Uno et al., 2019). For biological samples, a feature resolution of 20 nm could be obtained by STED super-resolution imaging (Sahl et al., 2010). Despite great efforts, some annoying restrictions related to the current fluorescent probes still exist and hamper the improvement of spatial resolution of STED technology. Photobleaching or photostability of the most organic probes due to high power intensity (1–200 MW/cm2) of the depletion laser for the desired spatial resolution is a platitude but disturbing problem.7 In addition, the high excitation power density of the depletion laser could also cause overheating and phototoxic effects on biological specimens (Waldchen et al., 2015; Masch et al., 2018). Besides, the imaging depth of STED technology is often limited by the short excitation, depletion, and emission (<800 nm) wavelengths because of their strong photo scattering in bio-tissues (Kolmakov et al., 2015). In contrast to conventional biomarkers, quantum dots (QDs) with excellent photostability can also be utilized as standard fluorescent probes in STED microscopy with a resolution of 50 nm (Hanne et al., 2015). However, the excitation power density and excitation wavelengths in these reports still need to be optimized.
Besides the abovementioned fluorescent probes, lanthanide-based luminescent biological probes with near-infrared emissions have been proven to be superior for in vivo optical imaging and detection due to their unique optical properties, including non-photobleaching, sharp emissions, and large Stokes shifts (Wang et al., 2014; Zhang et al., 2019; Fan and Zhang, 2019; Zhong et al., 2019). So far, researchers have demonstrated the potential applications of several kinds of lanthanide-doped nanomaterials on super-resolution optical microscopy (Liu et al., 2017; Zhan et al., 2017; Huang et al., 2018; Krause et al., 2019; Dong et al., 2020). In review of the already reported results, here we first describe several mechanisms for super-resolution optical microscopy based on lanthanide nanostructures and a STED-like setup. Then, we present and analyze the development and applications of these inorganic fluorescent probes for optical micro-imaging. Last, the emphasis of the review is placed on the challenges and opportunities in advancing next-generation fluorescent probes for super-resolution optical imaging.
Designing a Scheme for Breaking Through the Diffraction Limit
Taking advantage of the STED setup, three disparate mechanisms are proposed to obtain a high spatial resolution of optical imaging. They are stimulated emission depletion fluorescence, excited state absorption, and fluorescence emission difference, all of which rely on emitting transition (switching) between two states, typically a fluorescent “on” state and a dark, nonfluorescent “off” state, allowing the limiting character of diffraction to be suppressed with or without data processing.
Stimulated Emission Depletion Fluorescence
Stimulated emission to deplete fluorescence for super-resolution imaging, experimentally realized by Stefan W. Hell in 1999 (Klar and Hell, 1999), is an efficient and widely used method now. For organic dyes, stimulated emission from a suitable laser excitation is a fundamental property of electron transition. However, due to the abundant energy levels of trivalent lanthanide ions, excitation under two-color fields will always cause enhanced luminescence intensity of lanthanide activators through excited state absorption (Zhou et al., 2013a; Chen et al., 2015; Zhang et al., 2016). Until the seminal paper published in 2017, Jin et al. reported that the conventional stimulated emission of Yb3+/Tm3+ co-doped nanocrystals could be triggered by an 808-nm laser illumination due to the population inversion of a metastable 3H4 level caused by intense cross-relaxation (CR) at high concentrations of Tm3+ ions (Liu et al., 2017). The stimulated emission mechanism can be simplified as shown in Figure 1B. Electrons on the energy level S2 will be populated to the S3 state to emit fluorescence with the wavelength at λ2 when the lanthanide materials are only excited at laser λ1. After the depletion laser λ3, matching the emission band of the S2 → S0 transition, is turned on simultaneously, electrons on the S2 state will be discharged to the ground state S0 through a stimulated emission process, which in turn greatly reduces the electron population of the energy level S3 and thus exhausts its fluorescence intensity. It should be noted that one crucial prerequisite is the population inversion of the S2 state relative to the S0 ground level to maximally alleviate the ground state absorption of S0 on the depletion laser λ3. In STED nanoscopy, the resolution obeys the formula (Wildanger et al., 2009):
where
Excited State Absorption
Benefiting from the abundant energy states of trivalent lanthanide ions, diversified excitations on lanthanide-doped materials can provide a desired luminescent behavior. It was reported that depleted fluorescence under two laser co-excitation could be realized through excited state absorption (ESA) as well (Kolesov et al., 2011; Wu et al., 2015). The schematic energy transfer pathway is depicted in Figure 1C. Electrons on ground state N0 are first pumped to excited state N2 through resonant energy transfer or direct self-absorption by a pulsed laser (λ4). After the pulse excitation is over, electrons still remain at N2 state for a short time due to the long luminescence lifetime of intermediate N2 state. Meanwhile, a donut-shaped laser λ5 matching the energy gap between N2 state and N3 is turned on all the time, which will consequently consume the electron population of N2 state and then result in elevated population of N3 state around the focal spot through the ESA process. Emission at λ6 from N3 state is much faster than that at λ5 from N2 state. Hence, in the region around the focal spot, electrons on N3 state are quickly depopulated to ground state N0, while in the central zone of the donut-shaped laser, most of the electrons still rest on N2 state. Finally, a short readout Gaussian pulse of λ5 laser is applied to generate the fluorescence signal from energy level N3 only in the finite central region. Therefore, a super-resolution image can be scanned through separated time–domain detection.
Fluorescence Emission Difference
Fluorescence emission difference (FED) microscopy, also termed as switching laser mode (SLAM) microscopy, as a derivative method of STED, was independently proposed in theory and experiments by Xu Liu and Yves De Koninck in 2013 (Dehez et al., 2013; Kuang et al., 2013). In this design and the corresponding STED-like setup, two laser beams with Gaussian and donut intensity distributions are applied to stimulate bio-probes to generate fluorescence with solid and donut luminescence intensity distributions, respectively. Then two different images are subsequently collected based on the scanning imaging system of a conventional confocal microscope (Wen et al., 2020). The final super-resolution FED microscopic image is constructed using a subtractive intensity method of the above two images (Figure 1D). Some inevitably negative intensity values introduced by the subtraction process can be excluded by setting the value at zero to improve the imaging resolution. It should be noted that almost all the fluorescence probes are suitable for the super-resolution method, in which the optical resolution does not rely on a fluorescence depletion property under simultaneous excitation of two lasers but the emission saturation property of fluorescence probes. Lower laser power density to achieve maximum emission intensity will decrease the size of the dark spot in the emission donut pattern, thereby improving the optical resolution.
Photon Avalanching Mechanism
Two-photon microscopy is a powerful tool for visualizing biological activities with deep-tissue imaging capability, but it is hard to resolve structures, such as cytoskeletons, with satisfactory spatial resolution (Helmchen and Denk, 2005). To address this issue, Artur Bednarkiewicz et al. proposed a novel concept that depends on using photon avalanching (PA) emission nanoparticles to explosively enhance the nonlinear relationship (S) between luminescence intensity and excitation power density (Bednarkiewicz et al., 2019). This technique utilizes the high S value (>50) to narrow the point spread function below 50 nm. Different from STED, this nonlinear laser scanning method for optical super-resolution exploits a single excitation laser, and the resolution increases according to the equation:
where d is the spatial resolution, λ is the excitation wavelength, NA is the numerical aperture of the oil immersion objective (typically NA = 1.4), and S is the degree of the nonlinear relationship between luminescence intensity and excitation power density. That is, to say, high nonlinearity will be beneficial for optical resolution. In a typical two-photon microscopic method, S value is about 2 (Ning et al., 2017). For lanthanide-doped nanoparticles, S can reach 5 (Chen et al., 2016). As to photon-avalanche nanoparticles, S can be adjusted to exceed 20, using which sub −70 nm spatial resolution can be realized (Lee et al., 2021).
Performance Evaluation of Lanthanide-Doped Nanoparticles for Super-Resolution Imaging
Actually, one important role that makes the optical nanoscopy so powerful in bio-imaging and bio-detection is the great development of multifarious fluorescence probes that permit higher photostability, lower photon scattering and bio-toxicity, and so on. Until now, among various fluorescence probes, a series of nano-compositions doped with lanthanide ions, including Tm3+, Er3+, Pr3+, Dy3+, and Eu3+, have been used as fluorescence emitters for optical super-resolution imaging based on the STED-like setup. Different lanthanide ions exhibit varying abilities to break through the diffraction limit by disparate energy transfer channels and solution designs.
Tm3+-Doped Nanocrystals for Super-Resolution Imaging
Activator Tm3+, often co-doped with sensitizer Yb3+, is a typically efficient blue-violet (475, 455, 360, 340 and 290 nm) light emitter under a continuous wave 980 nm laser excitation (Li et al., 2015; Wang et al., 2018). However, due to the much more intense cross-relaxation between Tm3+ ions than that between other activators (such as Er3+ and Ho3+), the optimal doping concentration of Tm3+ is relatively low, in the range of 0.2–1 mol% under excitation power density below 100 W/cm2 (Zhang et al., 2011). Thus, the upconversion luminescence intensity of Tm3+ is very weak because of the smaller number of activators (one of the main reasons). In 2013, Jin et al. found that 8% of Tm3+-doped nanocrystals at higher excitation irradiance (>106 W/cm2) could generate strong upconversion luminescence at 800 nm that is about 70 times brighter than that in low Tm3+-doping nanocrystals (Zhao et al., 2013a). The mechanism investigation indicates that the saturated electron population of 3H4 state at high excitation power density induces an efficient energy transfer from the 2F5/2 state of Yb3+ to 1D2 and 1G4 states of Tm3+ ions. Subsequently, taking advantage of the high (8%mol) Tm3+-doped nano-composition, Jin reported a lanthanide-based STED microscopy for imaging single upconversion nanocrystals. In this study, 980 nm laser with a Gaussian intensity distribution acts as an excitation laser for illuminating the upconversion luminescence of Tm3+. At high excitation power density, almost all the electrons on ground state 3H6 are excited to state 3H4, resulting in a sharp population inversion between 3H4 and 3H6 states. At the same time, an 808-nm laser, matching only the 3H4 to 3H6 transition, stimulates disturbance on the electrons of the 3H4 state to produce stimulated emission at 808 nm, thus largely reducing the population of the 3H4 energy level and those of the higher states (1D2 and 1G4) that strongly depend on the population of 3H4 (Figures 2A andB). Combining the unique optical property of NaYF4:Yb3+/8%Tm3+ nanocrystals under two laser simultaneous excitation with an STED technique, Jin realized optical super-resolution imaging of a single nanocrystal with a saturation intensity (means the depletion laser power density that halves fluorescence intensity) as low as 0.19 MW/cm2 and spatial resolution of 28 nm, 1/36th of the excitation wavelength (Figure 2C).26 This work brings a very valuable application of lanthanide fluorescence probes on super-resolution imaging because the lanthanide nanocrystals possess excellent photostability compared with conventional organic dyes used in STED microscopy.
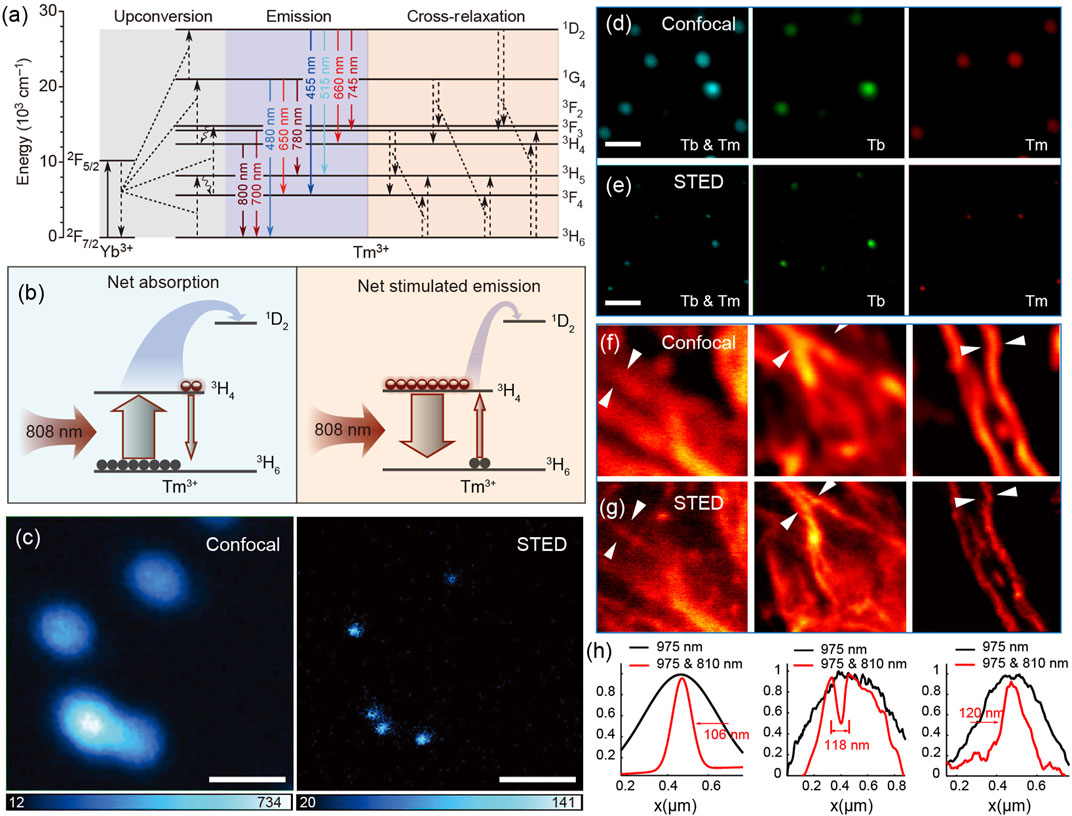
FIGURE 2. (A) Energy level diagram and energy transfer pathways of Yb3+/Tm3+ co-doped nanocrystals excited by a 980 nm laser. Solid arrows mean emissions; curved arrows mean energy transfer from Yb3+ to Tm3+ and typical cross-relaxation between Tm3+ ions. (B) Simplified diagrams describing net absorption (left) and net stimulated emission (right) between excited state 3H4 and ground state 3H6 of Tm3+ ions under a 980-nm laser excitation. (C) Optical imaging of 40 nm 8% Tm3+-doped nanocrystals based on confocal and STED microscopy, demonstrating the practicability of lanthanide-doped nanocrystals on super-resolution imaging. Adapted with permissions from the study by Liu et al. (2017) (scale bars, 500 nm). (D) Confocal and (E) super-resolution fluorescence images of NaGdF4:40%Yb3+,10% Tm3+@NaGdF4:15%Tb3+ nanocrystals with the emission wavelength at 455 and 547 nm (left), 547 nm (middle), and 455 nm (right). (F) Confocal and (G) super-resolution fluorescence images of the cellular cytoskeleton protein desmin conjugated with Tm3+-doped nanocrystals. (H) Line profiles taken from (F) and (G) quantitatively indicate the improvement of optical resolution of STED nanoscopy. Adapted with permissions from the study by Zhan et al. (2017).
Almost at the same time, Zhan independently reported a similar stimulated emission phenomenon of high (10% mol) Tm3+-doped nanocrystals excited simultaneously by 975 and 810 nm lasers (Zhan et al., 2017). In his article, more detailed and in-depth researches on bio-applications were carried out. First, using NaYF4:Yb3+/Tm3+ nanocrystals (blue emission) together with NaGdF4:Yb3+/Tm3+@NaGdF4:Tb3+ nanocrystals ( green emission) that originates from the energy of 1I6 (Tm3+) through an energy migration–mediated upconversion (EMU) process, the two-color super-resolution imaging was exhilaratingly achieved (Figures 2D,E). Second, the super-resolution imaging of immunostained HeLa cells using lanthanide nanocrystals was first demonstrated, indicating the real practicability of lanthanide nanocrystals on STED microscopy (Figures 2F–H).
However, because of the electric dipole forbidden nature of the 4f–4f transitions and the shielding effect of the outer electronic orbit in trivalent lanthanide ions, the luminescence lifetimes of lanthanide-doped nanocrystals are typically long, ranging from tens of microseconds to milliseconds (Lu et al., 2013; Tan et al., 2020). However, in the STED microscopic scanning imaging system, longer luminescence lifetimes will inevitably reduce imaging scanning speed and lengthen the acquisition times of a super-resolution image, which limits the applications of the lanthanide-based STED technique in investigations of fast dynamic life processes and 3D volume super-resolution imaging. To address this challenge, Zhan et al. subtly designed high Yb3+-doped nanocrystals NaYF4@NaYbF4:10%Tm3+ to enhance upconversion luminescence by intensifying the local energy supply from Yb3+ to Tm3+ and meanwhile shorten the 455-nm emission lifetime from 34.32 to 7.45 µs due to the increased energy transfer speed between Yb3+ and Tm3+ (Peng et al., 2019). Compared to the earlier reported nanocrystals (NaYF4:18%Yb3+/10%Tm3+) that show long luminescence at 455 nm, the newly developed nanocrystals (NaYF4@NaYbF4:10%Tm3+) used for fast scanning super-resolution microscopy with a speed of 10 µs per pixel exhibit less emission streaking and higher spatial resolution. This work provides the potential for lanthanide-based STED microscopy to record dynamic vital activities.
By view of the abundant and ladder-like energy levels in trivalent thulium ions, the co-illumination of different stimulation wavelengths on low Tm3+-doped nanocrystals can induce the depleted fluorescence as well. In 2017, we also reported the quenched upconversion luminescence of NaYF4:Yb3+/Tm3+ nanocrystals under simultaneous excitations of 980 and 1,550 nm lasers (Zhang et al., 2017). The depletion ratio of emission around 455 nm could reach 90% at a depletion laser power density lower than 100 W/cm2. Such high-depletion efficiency at a low irradiation energy flux portends an underlying superior performance on super-resolution imaging. Great efforts were conducted to evaluate the possible depletion mechanism, including cross-relaxation, thermal effect, and stimulated emission. Last, we demonstrated that stimulated emission was responsible for the quenched fluorescence phenomenon at two-color fields. However, it should be noted that excitation sources with the wavelength around 1,500 nm are unamiable for bio-imaging and detection due to strong water absorption around the photon wavelength at 1,500 nm.
As for bio-applications, the emission at 455 nm of Tm3+ is not suitable for deep tissue super-resolution imaging because the luminescence intensity of four-photon upconversion (455 nm) will be seriously attenuated through deep tissues, and photon scattering of short wavelengths in deep tissues can result in reduced resolution as well (Hong et al., 2014). Therefore, in 2018, Jin et al. proposed near-infrared (800 nm) emission saturation nanoscopy for deep tissue super-resolution imaging by the FED technique (Chen et al., 2018). In the study, they first revealed that 4% Tm3+-doped upconversion nanocrystals showed lower power density to realize a similar spatial resolution as that for the reported 8% Tm3+-doped nanocrystals. For the sake of deep tissue super-resolution imaging, the near-infrared emission around 800 nm of Tm3+ through the two-photon process was used. Their results demonstrated sub-50 nm optical resolution through an 88-µm liver tissue slice. Due to the obvious different saturation thresholds of 800 and 740 nm emissions from Tm3+-doped nanocrystals, donut-shaped and Gaussian-shaped emissions were simultaneously generated to enhance the spatial resolution (40 nm) and imaging quality through a method of Fourier domain heterochromatic fusion derived from the FED method (Chen et al., 2021). Actually, this is far from the limit of resolution and penetration depth. Investigations of longer near-infrared emissive probes (especially emission wavelengths longer than 1,000 nm) on deeper tissue super-resolution imaging still need to be pushed in the future.
Although the 800-nm luminescence emission used in super-resolution imaging has been reported, the power density of the excitation laser was as high as 5.5 MW/cm2 (Chen et al., 2018). In 2021, Lee experimentally reported optical super-resolution imaging using photon-avalanche nanoparticles (NaY0.92Tm0.08F4@NaY0.8Gd0.2F4) under a very low power density (Lee et al., 2021). In this design, 11,064 nm wavelength as excitation laser and 8% Tm3+-doped nanoparticles as fluorescent probes were used to obtain effective photon-avalanche due to the weak ground state absorption and intense excited state absorption. The degree of nonlinearity S can be larger than 20 under a power density below 10 kW/cm2, which is two orders of magnitude lower than that used in most STED nanoscopies (Xu et al., 2021). According to Equation 2, sub-70 nm optical resolution was experimentally achieved under a power density of 7.6 kW/cm2. Besides the low power density used in this work, the super-resolution imaging system is based on a scanning confocal microscopic system, which is easier to implement than the STED system.
Er3+-Doped Nanocrystals for Super-resolution Imaging
Apart from lanthanide Tm3+ for nanoscopic studies, Er3+ with typical green and red emissions can also be used in super-resolution imaging. As early as in 2015, Zhan et al. had already proposed the concept of lanthanide-doped nanocrystals used in STED nanoscopy (Wu et al., 2015). They developed a co-excitation system of 795 and 1,140 nm lasers for depleting the green emission of Er3+ions. Two processes, the ESA of the energy level 2H11/2 and 4S3/2 on 1,140 nm photon energy and energy transfer from the higher energy state (4G11/2) of Er3+ to Yb3+ions, synergistically contribute to the photo-induced depletion of green emissions. However, such a low depletion efficiency (maximum: 30%) cannot meet the practical requirements of super-resolution imaging in STED-like nanoscopy. Maybe, more efficient energy acceptors, such as organic dyes and quantum dots, will induce a higher fluorescence depletion performance.
Subsequently, to break through the annoying limit of depletion efficiency on imaging resolution, an FED technique was used for multiphoton super-resolution imaging based on red emission nanocrystals NaYF4:Nd3+/Yb3+/Er3+@NaYF4:Nd3+ with an 808-nm laser excitation. The spatial resolution in the design could reach 80 nm (Wu et al., 2017). Nonetheless, in FED imaging, two images at a solid and donut lasers excitation are scanned successively, which is time-consuming and suffers from sample jitters under high laser power density excitation. In view of this issue, core/multi-shell NaYF4:2%Er3+@NaYF4@NaYF4:20%Yb3+/2%Tm3+ nanocrystals with independent orthogonal excitation/emission luminescence at 808 and 940 nm laser excitation were developed and used as fluorescence probes in the two-detection-channel FED microscopy (Huang et al., 2018). Blue emissions of Tm3+ excited by a solid 940-nm laser and green emissions of Er3+ ions excited by a donut-shaped 808-nm laser could be collected synchronously, reducing the acquisition time in contrast to the conventional method of ordinal collection and ensuring the exactly identical position of the pixels in two images. After straightforward subtraction of the two images, a feature resolution of 54 nm was realized.
Er3+-doped nanocrystals, exhibiting intense emissions at both visible (540 and 654 nm) and near-infrared (1,525 nm) ranges, have been widely applied in diagnosis, therapy, and in vivo bio-imaging with high spatial resolution (Zhong et al., 2017; Wang et al., 2019; Zhang et al., 2019; Gao et al., 2019). However, an effective excitation/emission system to deplete the upconversion and downshifting luminescence intensity in Er3+-doped nanocrystals under two lasers irradiation has not been reported so far. For a better optical imaging resolution of in vivo STED nanoscopy, it is really desired to explore more feasible doping nanostructures of Er3+ ions.
Other Lanthanide Ion–Doped Nanocrystals for Super-Resolution Imaging
To the best of our knowledge, Pr3+ is the first lanthanide ion that is used in STED nanoscopy (Kolesov et al., 2011). In 2011, Kolesov described super-resolution imaging on ultraviolet emission nanocrystals (Pr: YAG) through an ESA mechanism (Figure 3A). Pr3+ had strong ground state absorption on 609 nm to populate its 1D2 state, while the excited state absorption from 1D2 to 4f5d (1) was only efficient at a wavelength of 532 nm rather than 609 nm. The population of 1D2 state in Pr3+ ions spatially located at the peripheral focus spot excited by a solid 609 nm pulsed laser could be consumed quickly through the ESA of the 1D2 energy level on a donut-shaped 532-nm continuous wave laser, resulting in a prior ultraviolet emission from 4f5d (1) of Pr3+ ions spatially around the focus spot. Because the luminescence lifetime of 4f5d (1) (18 ns) was much shorter than that of 1D2 (150–200 µs), the electron population of 1D2 state in the center of the focus spot was nearly unchanged after the 4f5d (1) state was absolutely depopulated. Finally, a short readout (20 ns) Gaussian pulse with a wavelength at 532 nm was used to generate ultraviolet emissions only at the dark spot of the donut laser. Based on the temporal control and STED equipment system, Roman Kolesov obtained sub-diffraction limit optical imaging on Pr: YAG nanocrystals with a spatial resolution of 50 nm that was restricted by the particle size (Figure 3B). The mechanism of ESA is very dependable and intriguing; however, it also requires a complicated optical system and precise synchronization control in temporal. A simpler optical system and rational design of fluorescence probes for super-resolution imaging based on the ESA mechanism still needs to be explored and developed.
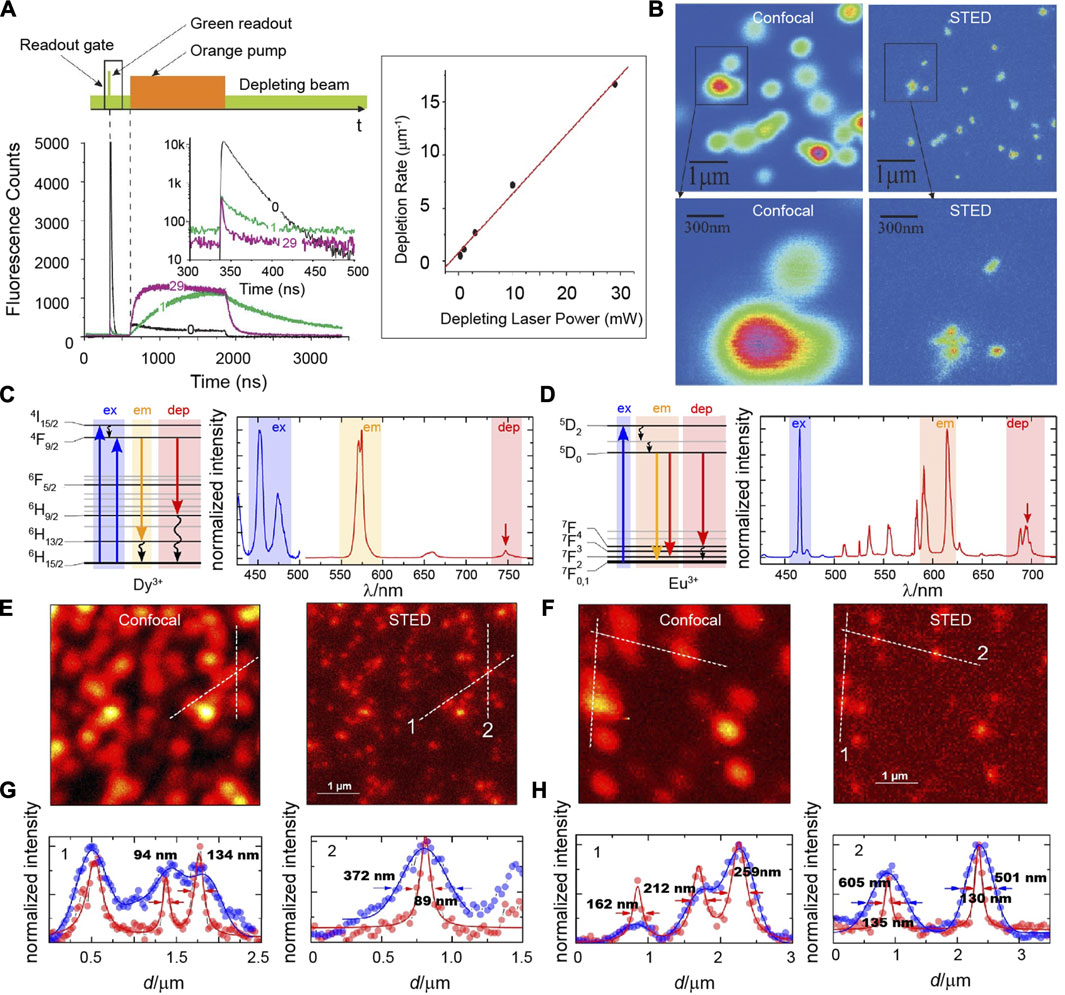
FIGURE 3. (A) Excitation sequence of pulsed and continuous wave lasers in time–domain (upper), the decay curves of upconversion luminescence at different times (lower) and the depletion rates at different laser powers. (B) Confocal (left) and STED (right) images of Pr: YAG fluorescent nanoparticles. Adapted with permissions from the study by Kolesov et al. (2011). Simplified energy-level diagram (left), excitation, and emission spectra (right) of (C) Dy3+ and (D) Eu3+ ions. Confocal (left) and STED (right) imaging of (E) NaYF4: Dy3+ and (F) NaYF4: Eu3+ nanocrystals at the same region. Intensity cross-section profiles of (G) NaYF4: Dy3+ and (H) NaYF4: Eu3+ nanocrystals of the dashed lines 1 and 2 in panels (E) and (F). Adapted with permissions from the study by Stephan et al. (2019).
The EMU process that mainly depends on the nanostructure NaGdF4:Yb3+/Tm3+@NaGdF4:X3+ is a promising energy transfer channel for the upconversion luminescence of those lanthanide activators without long-lived intermediary energy levels, such as Sm3+, Tb3+, Dy3+, and Eu3+ ions (Wang et al., 2011; Liang et al., 2019). Taking advantage of the mechanism and fluorescence depletion property of highly doped Tm3+ions, green emissions at 547 nm of Tb3+-doped EMU nanocrystals could be efficiently quenched and applied in STED super-resolution imaging (Zhan et al., 2017). This strategy can be readily extended to other lanthanide ions, including Eu3+, Dy3+, and Sm3+. However, no relevant further studies have been reported so far.
Recently, Stefan Krause had described a proof of the general lanthanide applicability on STED super-resolution imaging (Figures 3C–H) (Krause et al., 2019). A fluorescence intensity of 572 nm in NaYF4: Dy3+ nanocrystals directly excited by 449 nm, 452 nm, or 473 nm lasers could be exhausted by using a 748-nm laser co-excitation. A saturation intensity of 7.1 MW/cm2 for NaYF4: 3% Dy3+ nanocrystals was similar to what was measured for organic dyes, but it was obviously higher than those evaluated for Tm3+-based nanocrystals. As for NaYF4: Eu3+ nanocrystals, a 465-nm laser and a 695-nm laser were chosen to stimulate and deplete fluorescence around 600 nm, respectively. Similarly, the saturation intensity (3.3 MW/cm2) for NaYF4: 3% Eu3+ nanocrystals was so high that only a highly elevated depletion laser power density (222 MW/cm2) could guarantee an acceptable depletion efficiency and increased optical resolution.
To decrease the power density of the depletion laser, Liang et al. developed a novel lanthanide ion Nd3+-doped nanoparticle for STED nanoscopy. In the nanosystem, an 808-nm laser was used as an excitation source and a 1,064-nm laser was used as a depletion source to depopulate the excited state (4F3/2). Attributed to the long-lived metastable level (4F3/2, >50 µs) that is beneficial for population inversion, the saturation intensity was reduced to 19 kW/cm2, which enabled high-contrast deep-tissue imaging with about a 70-nm spatial resolution of subcellular structures. This work extends an important lanthanide ion in STED nanoscopy and will provide a great opportunity to achieve optical super-resolution using other lanthanide ions because the Nd3+ ion is a significant sensitizer to activate upconversion and downshifting luminescence of Er3+, Tm3+, Ho3+, and so on (Zhou et al., 2013b; Shen et al., 2013; Wang et al., 2013; Wang et al., 2014).
Challenges and Outlook
Great efforts and improvements of lanthanide-doped nanocrystals in super-resolution imaging have been recently made and achieved. Until now, lanthanide ions including Tm3+, Er3+, Tb3+, Eu3+, Pr3+, and Dy3+ have been reported for potential super-resolution imaging on nanocrystals themselves and the cytoskeletons of HeLa cancer cells (Table 1). Despite these endeavors, the related development is still in its infancy. Several crucial challenges and issues still exist, involving the high power density of the depletion laser, a long luminescence lifetime of lanthanide probes, big particle size of lanthanide nanocrystals, short emission wavelength, and superior mechanism design, all of which need considerable explorations and investments from research fields of chemistry, materials science, and optics.
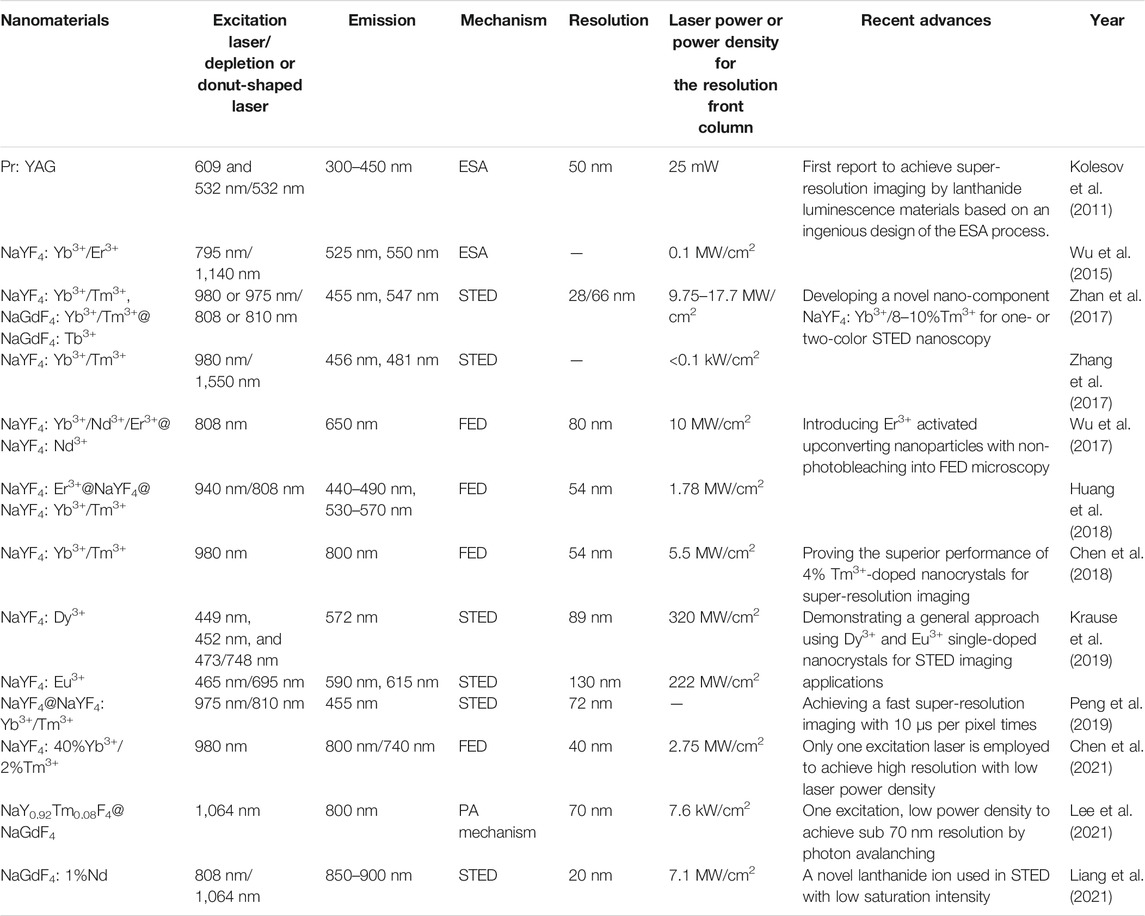
TABLE 1. Progress in the design and results of lanthanide-doped nanocrystals for super-resolution imaging.
For a long time, organic compounds and fluorescent proteins were the commonly used fluorophores for STED nanoscopy that require fluorescent probes to be cycled many times between emission “on” and “off” states. Therefore, the photostablity of fluorescent probes is very important. Since lanthanide-doped nanocrystals show excellent photostability without photobleaching (Wang and Liu, 2009), they have great advantages on micro-imaging that usually needs high laser power density. However, this does not mean that the high power density is tolerable because there exists an underlying over-heating effect on biological specimens when a required spatial resolution only occurring at a high power density of the depletion laser is required. The current power density of the depletion laser for satisfying the spatial resolution is as high as 10 MW/cm2 (Table 1). A significant approach to decrease the demanded power density is to elevate the depletion efficiency, so as to lower the energy flux for an equivalent resolution. According to Equation 1, it is obvious that
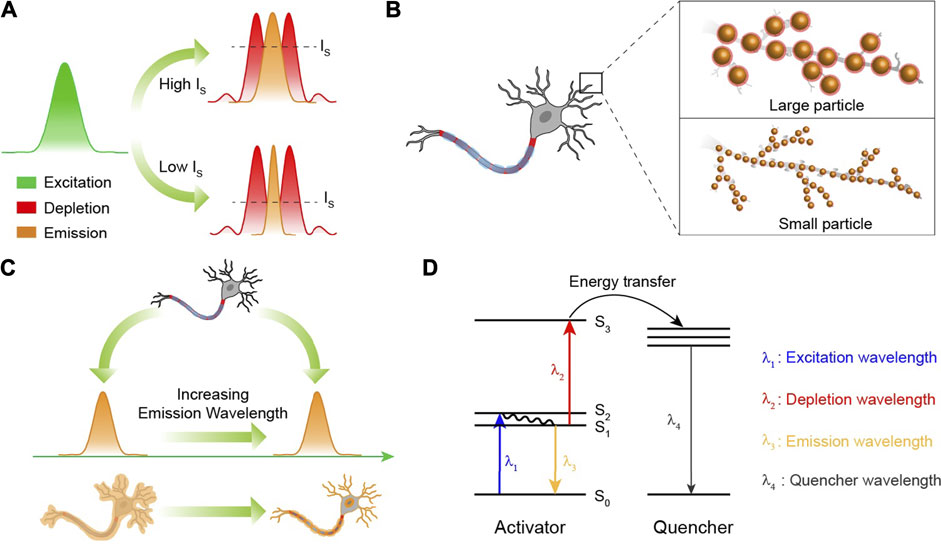
FIGURE 4. (A) The spatial region that fluoresces can be narrowed by reducing the saturation intensity (IS). Green, red, and yellow curves represent the areas of excitation, depletion, and emission, respectively. (B) The smaller nanoparticles that still possess a strong fluorescence intensity will ensure a higher spatial resolution than the larger ones. (C) In STED nanoscopy, the fluorescence probes with a longer emission wavelength will be favorable for in vivo super-resolution imaging due to their low photon scattering. (D) The excited state absorption mechanism for lanthanide ions in STED nanoscopy. λ1 is the wavelength of the excitation laser. λ2 is the wavelength of the depletion laser. λ3 is the emission wavelength. λ4 is the emission wavelength of the chosen quenchers. In the design, depletion laser, energy transfer from S3 of activator to quenchers and emissions from quenchers will continuously consume the population of S1state.
As is well known, STED nanoscopy is a point-by-point scanning imaging process. Hence, the imaging rates of STED nanoscopy usually depend on the pixel dwell times. Nevertheless, due to the shielding of the outer orbit in trivalent lanthanide ions, the luminescence lifetimes of lanthanide ions are uniquely long (>50 µs) (Zhao et al., 2013b; Lu et al., 2013; Wang et al., 2016), going against a quick frame capture. The imaging acquisition time is necessarily shortened when it comes to the dynamic imaging of certain biological activities. Therefore, effective approaches to greatly shorten the luminescence lifetime to a sub-microsecond level, and in the meantime reserve or enhance the emission intensity, is mightily desired to ensure brief pixel dwell times. Several methods including elevating the doping concentration of sensitizer Yb3+ and coupling gap plasmonic cavity are proposed (Su et al., 2017; Feng et al., 2019). So far, ultrabright spontaneous emission of Er3+ with ultrashort luminescence lifetimes (1.4 µs) has been realized through a finely designed nanocavity, where the nanostructures should be elaborately and specifically fabricated, leading to an inevitable obstacle for the freewheeling targeting distribution of the luminescence center on bio-specimens (Wu et al., 2019; Xu et al., 2021).
Imaginably, the size distribution of nanoparticles at some point will become a limiting factor because the spatial resolution of the present STED technique can reach below 30 nm (Gottfert et al., 2013; Kasper et al., 2010), indicating that a large nanoparticle (>30 nm) would restrain the improvement of the spatial resolution (Figure 4B). And for a better optical resolution, the size should be as small as possible, while fluorescence signals are still kept strong enough to be detected for super-resolution imaging. It is well known that nanocrystals based on the Gd3+ host exhibit ultrasmall particle sizes (<5 nm), yet accompanied by significantly quenched fluorescence intensity as well (Wong et al., 2011; Chen et al., 2019). Some efforts have been made to develop lanthanide-doped nanoparticles with bright upconversion luminescence at a single particle level. In 2018, NaYF4@NaYb0.92F4:Er0.08@NaYF4 nanoparticles exhibiting bright luminescence (upconversion quantum yield: 5.03 ± 0.60%) were reported by Steve Chu (Liu et al., 2018). Besides, an enhanced resolution (294.4 nm) of single nanoparticle (NaYF4: 60%Yb, 8%Er0.08@NaYF4) imaging was achieved due to its bright upconversion luminescence (Ma et al., 2020). However, a size distribution of more than 29 nm for these nanoparticles is unsatisfactory for optical super-resolution because a spatial resolution below 30 nm has been achieved through STED nanoscopy (Xu et al., 2021). It is still a great challenge to develop a general synthetic method or nano-architectural method to achieve bright fluorescence intensity from ultrasmall lanthanide-doped nanocrystals with a particle size less than 5 nm (Willets, 2013; Liu et al., 2021). In 2021, Gu et al. prepared NaGdF4: 1%Nd nanoparticles with 6.68 ± 0.8 nm size distribution to achieve STED super-resolution imaging (Liang et al., 2021), which is a considerable improvement in the particle size used in STED nanoscopy, and more efficient luminescence emission with smaller particle size of various lanthanide ion–doped nanoparticles is required in the future.
Here, we introduced and concluded the design and potential application of lanthanide-doped nanoparticles for optical super-resolution imaging. Present works mainly focus on the imaging of a single lanthanide-doped nanoparticle. The super-resolution imaging of cytoskeletons and intracellular microtubule structures based on lanthanide ions has been realized (Zhan et al., 2017; Liang et al., 2021). However, as can be seen, the bio-applications of super-resolution imaging based on lanthanide-doped nanoparticles developed slowly. One great challenge is that the conjugated efficiency of lanthanide-doped nanoparticles labeling the substructures of cells is low. Presently, conjugating these nanoparticles to subcellular structures by antibodies is a popular method.
Recently, scientists have demonstrated that fluorescence probes with second near-infrared (NIR II, 1,000 nm–1700 nm) emissions show superior tissue penetration and better imaging resolution because of reduced photon scattering and biological absorption at this wavelength region (Hong et al., 2014; Ding et al., 2018; Li et al., 2020; Zhao et al., 2021). The current emission wavelengths of lanthanide-doped nanocrystals used in STED-like nanoscopy are usually located at the visible region, which is not suitable for in vivo super-resolution imaging at a relatively deeper tissue. In 2018, an 800-nm emission from Tm3+-doped nanocrystals was used in STED-like nanoscopy to achieve super-resolution imaging through a 92-µm brain tissue slice, where only 11.3% of the fluorescence intensity at 455 nm was left due to the strong photon attenuation (Chen et al., 2018). So, developing a novel excitation system and lanthanide-doped nanocrystals with NIR II emissions that satisfy the requirement of in vivo super-resolution imaging beneath a deep tissue is also a significant research field (Figure 4C). It should be noted that in conversional optical and confocal microscopy, the imaging resolution principally relies on the emission wavelengths of fluorescence probes, while in STED-like nanoscopy, it is entirely different. According to Equation. 1, the spatial resolution is dependent on the wavelengths of depletion lasers rather than the emission wavelengths of fluorescence probes. So, prolonging the luminescence wavelength will not lead to the natural decrease of the imaging resolution.
FED microscopy, as a derivative design of the STED technique, breaks the limit that lanthanide ions applied in popular STED nanoscopy must have an efficient behavior of stimulated emission, meaning that almost all the fluorescent lanthanide ions can be used in FED microscopy. However, different from STED nanoscopy, the theoretical optical resolution in FED microscopy is intrinsically related to the emission wavelengths of lanthanide ions, resulting in increased difficulty in distinguishing adjacent lanthanide ions. Hence, a more complete data processing model is desired to promote the theoretical optical resolution of FED microscopy.
Novel solutions to achieve the efficient fluorescence depletion of targeted energy levels with lower
In summary, we introduced and analyzed a recent process of lanthanide-doped nanocrystals on super-resolution imaging. Exciting works in the multidisciplinary field including chemistry, nanomaterials, and optics have already established the scientific fundamentals and design solutions for higher spatial resolution and more practical applications. Under the inspiring achievements, great efforts on developing superior emission conditions of lanthanide ions that satisfy the demand of super-resolution imaging should be made in the future.
Author Contributions
HZ designed and wrote the first draft of the manuscript. All authors contributed in the revisions and approved to publish the final manuscript.
Funding
The work was sponsored by Shanghai Sailing Program of Science and Technology Commission of Shanghai Municipality (20YF1402200, 20490710600) and supported by the National Key R&D program of China (2017YF A0207303) and the National Natural Science Foundation of China (21725502, 21701027, 2210040480).
Conflict of Interest
The authors declare that the research was conducted in the absence of any commercial or financial relationships that could be construed as a potential conflict of interest.
Publisher’s Note
All claims expressed in this article are solely those of the authors and do not necessarily represent those of their affiliated organizations, or those of the publisher, the editors and the reviewers. Any product that may be evaluated in this article, or claim that may be made by its manufacturer, is not guaranteed or endorsed by the publisher.
References
Auzel, F. (2004). Upconversion and Anti-stokes Processes with F and D Ions in Solids. Chem. Rev. 104, 139–174. doi:10.1021/cr020357g
Bates, M., Blosser, T. R., and Zhuang, X. (2005). Short-Range Spectroscopic Ruler Based on a Single-Molecule Optical Switch. Phys. Rev. Lett. 94, 108101. doi:10.1103/PhysRevLett.94.108101
Bates, M., Huang, B., and Zhuang, X. (2008). Super-Resolution Microscopy by Nanoscale Localization of Photo-Switchable Fluorescent Probes. Curr. Opin. Chem. Biol. 12, 505–514. doi:10.1016/j.cbpa.2008.08.008
Bednarkiewicz, A., Chan, E. M., Kotulska, A., Marciniak, L., and Prorok, K. (2019). Photon Avalanche in Lanthanide Doped Nanoparticles for Biomedical Applications: Super-resolution Imaging. Nanoscale Horiz. 4, 881–889. doi:10.1039/c9nh00089e
Berning, S., Willig, K. I., Steffens, H., Dibaj, P., and Hell, S. W. (2012). Nanoscopy in a Living Mouse Brain. Science 335, 551. doi:10.1126/science.1215369
Betzig, E., Patterson, G. H., Sougrat, R., Lindwasser, O. W., Olenych, S., Bonifacino, J. S., et al. (2006). Imaging Intracellular Fluorescent Proteins at Nanometer Resolution. Science 313, 1642–1645. doi:10.1126/science.1127344
Butkevich, A. N., Ta, H., Ratz, M., Stoldt, S., Jakobs, S., Belov, V. N., et al. (2018). Two-Color 810 Nm Sted Nanoscopy of Living Cells with Endogenous Snap-Tagged Fusion Proteins. ACS Chem. Biol. 13, 475–480. doi:10.1021/acschembio.7b00616
Chen, B., Kong, W., Wang, N., Zhu, G., and Wang, F. (2019). Oleylamine-Mediated Synthesis of Small Naybf4 Nanoparticles with Tunable Size. Chem. Mater. 31, 4779–4786. doi:10.1021/acs.chemmater.9b01050
Chen, C., Liu, B., Liu, Y., Liao, J., Shan, X., Wang, F., et al. (2021). Heterochromatic Nonlinear Optical Responses in Upconversion Nanoparticles for Super‐Resolution Nanoscopy. Adv. Mater. 33, 2008847. doi:10.1002/adma.202008847
Chen, C., Wang, F., Wen, S., Su, Q. P., Wu, M. C. L., Liu, Y., et al. (2018). Multi-Photon Near-Infrared Emission Saturation Nanoscopy Using Upconversion Nanoparticles. Nat. Commun. 9, 3290. doi:10.1038/s41467-018-05842-w
Chen, X., Jin, L., Kong, W., Sun, T., Zhang, W., Liu, X., et al. (2016). Confining Energy Migration in Upconversion Nanoparticles towards Deep Ultraviolet Lasing. Nat. Commun. 7, 10304. doi:10.1038/ncomms10304
Chen, Z., Wu, G., Jia, H., Sharafudeen, K., Dai, W., Zhang, X., et al. (2015). Improved Up-Conversion Luminescence from Er3+:Laf3 Nanocrystals Embedded in Oxyfluoride Glass Ceramics via Simultaneous Triwavelength Excitation. J. Phys. Chem. C 119, 24056–24061. doi:10.1021/acs.jpcc.5b08103
Dehez, H., Piché, M., and De Koninck, Y. (2013). Resolution and Contrast Enhancement in Laser Scanning Microscopy Using Dark Beam Imaging. Opt. Express 21, 15912–15925. doi:10.1364/OE.21.015912
Ding, F., Zhan, Y., Lu, X., and Sun, Y. (2018). Recent Advances in Near-Infrared Ii Fluorophores for Multifunctional Biomedical Imaging. Chem. Sci. 9, 4370–4380. doi:10.1039/c8sc01153b
Dong, H., Sun, L.-D., and Yan, C.-H. (2020). Lanthanide-Doped Upconversion Nanoparticles for Super-resolution Microscopy. Front. Chem. 8, 619377. doi:10.3389/fchem.2020.619377
Eggeling, C., Ringemann, C., Medda, R., Schwarzmann, G., Sandhoff, K., Polyakova, S., et al. (2009). Direct Observation of the Nanoscale Dynamics of Membrane Lipids in a Living Cell. Nature 457, 1159–1162. doi:10.1038/nature07596
Fan, Y., and Zhang, F. (2019). A New Generation of NIR‐II Probes: Lanthanide‐Based Nanocrystals for Bioimaging and Biosensing. Adv. Opt. Mater. 7, 1801417. doi:10.1002/adom.201801417
Feng, Z., Hu, D., Liang, L., Xu, J., Cao, Y., Zhan, Q., et al. (2019). Laser‐Splashed Plasmonic Nanocrater for Ratiometric Upconversion Regulation and Encryption. Adv. Opt. Mater. 7, 1900610. doi:10.1002/adom.201900610
Gao, R., Xu, L., Hao, C., Xu, C., and Kuang, H. (2019). Circular Polarized Light Activated Chiral Satellite Nanoprobes for the Imaging and Analysis of Multiple Metal Ions in Living Cells. Angew. Chem. Int. Ed. 58, 3913–3917. doi:10.1002/anie.201814282
Göttfert, F., Wurm, C. A., Mueller, V., Berning, S., Cordes, V. C., Honigmann, A., et al. (2013). Coaligned Dual-Channel Sted Nanoscopy and Molecular Diffusion Analysis at 20 Nm Resolution. Biophysical J. 105, L01–L03. doi:10.1016/j.bpj.2013.05.029
Gregor, C., Gwosch, K. C., Sahl, S. J., and Hell, S. W. (2018). Strongly Enhanced Bacterial Bioluminescence with the Ilux Operon for Single-Cell Imaging. Proc. Natl. Acad. Sci. USA 115, 962–967. doi:10.1073/pnas.1715946115
Grimm, F., Nizamov, S., and Belov, V. N. (2019). Green‐Emitting Rhodamine Dyes for Vital Labeling of Cell Organelles Using STED Super‐Resolution Microscopy. ChemBioChem 20, 2248–2254. doi:10.1002/cbic.201900177
Gwosch, K. C., Pape, J. K., Balzarotti, F., Hoess, P., Ellenberg, J., Ries, J., et al. (2020). Minflux Nanoscopy Delivers 3d Multicolor Nanometer Resolution in Cells. Nat. Methods 17, 217–224. doi:10.1038/s41592-019-0688-0
Hanne, J., Falk, H. J., Görlitz, F., Hoyer, P., Engelhardt, J., Sahl, S. J., et al. (2015). Sted Nanoscopy with Fluorescent Quantum Dots. Nat. Commun. 6, 7127. doi:10.1038/ncomms8127
Harke, B., Keller, J., Ullal, C. K., Westphal, V., Schönle, A., and Hell, S. W. (2008). Resolution Scaling in Sted Microscopy. Opt. Express 16, 4154–4162. doi:10.1364/oe.16.004154
Hell, S. W. (2015). Nanoscopy with Focused Light. Annalen der Physik 527, 423–445. doi:10.1002/andp.201500805
Hell, S. W. (2004). Strategy for Far-Field Optical Imaging and Writing without Diffraction Limit. Phys. Lett. A 326, 140–145. doi:10.1016/j.physleta.2004.03.082
Hell, S. W., and Wichmann, J. (1994). Breaking the Diffraction Resolution Limit by Stimulated Emission: Stimulated-Emission-Depletion Fluorescence Microscopy. Opt. Lett. 19, 780–782. doi:10.1364/Ol.19.000780
Helmchen, F., and Denk, W. (2005). Deep Tissue Two-Photon Microscopy. Nat. Methods 2, 932–940. doi:10.1038/nmeth818
Hong, G., Diao, S., Chang, J., Antaris, A. L., Chen, C., Zhang, B., et al. (2014). Through-Skull Fluorescence Imaging of the Brain in a New Near-Infrared Window. Nat. Photon 8, 723–730. doi:10.1038/nphoton.2014.166
Hu, C., Wu, Z., Yang, X., Zhao, W., Ma, C., Chen, M., et al. (2020). Mute-Sim: Multiphoton Up-Conversion Time-Encoded Structured Illumination Microscopy. OSA Continuum 3, 594. doi:10.1364/osac.387129
Huang, B., Wang, W., Bates, M., and Zhuang, X. (2008). Three-Dimensional Super-resolution Imaging by Stochastic Optical Reconstruction Microscopy. Science 319, 810–813. doi:10.1126/science.1153529
Huang, B., Wu, Q., Peng, X., Yao, L., Peng, D., and Zhan, Q. (2018). One-Scan Fluorescence Emission Difference Nanoscopy Developed with Excitation Orthogonalized Upconversion Nanoparticles. Nanoscale 10, 21025–21030. doi:10.1039/C8NR07017B
Kasper, R., Harke, B., Forthmann, C., Tinnefeld, P., Hell, S. W., and Sauer, M. (2010). Single-Molecule Sted Microscopy with Photostable Organic Fluorophores. Small 6, 1379–1384. doi:10.1002/smll.201000203
Klar, T. A., and Hell, S. W. (1999). Subdiffraction Resolution in Far-Field Fluorescence Microscopy. Opt. Lett. 24, 954–956. doi:10.1364/ol.24.000954
Klar, T. A., Jakobs, S., Dyba, M., Egner, A., and Hell, S. W. (2000). Fluorescence Microscopy with Diffraction Resolution Barrier Broken by Stimulated Emission. Proc. Natl. Acad. Sci. 97, 8206–8210. doi:10.1073/pnas.97.15.8206
Kolesov, R., Reuter, R., Xia, K., Stöhr, R., Zappe, A., and Wrachtrup, J. (2011). Super-Resolution Upconversion Microscopy of Praseodymium-Doped Yttrium Aluminum Garnet Nanoparticles. Phys. Rev. B 84. doi:10.1103/PhysRevB.84.153413
Kolmakov, K., Hebisch, E., Wolfram, T., Nordwig, L. A., Wurm, C. A., Ta, H., et al. (2015). Far-Red Emitting Fluorescent Dyes for Optical Nanoscopy: Fluorinated Silicon-Rhodamines (Sirf Dyes) and Phosphorylated Oxazines. Chem. Eur. J. 21, 13344–13356. doi:10.1002/chem.201501394
Krause, S., Liisberg, M. B., Lahtinen, S., Soukka, T., and Vosch, T. (2019). Lanthanide-Doped Nanoparticles for Stimulated Emission Depletion Nanoscopy. ACS Appl. Nano Mater. 2, 5817–5823. doi:10.1021/acsanm.9b01272
Kuang, C., Li, S., Liu, W., Hao, X., Gu, Z., Wang, Y., et al. (2013). Breaking the Diffraction Barrier Using Fluorescence Emission Difference Microscopy. Sci. Rep. 3, 1441. doi:10.1038/srep01441
Lee, C., Xu, E. Z., Liu, Y., Teitelboim, A., Yao, K., Fernandez-Bravo, A., et al. (2021). Giant Nonlinear Optical Responses from Photon-Avalanching Nanoparticles. Nature 589, 230–235. doi:10.1038/s41586-020-03092-9
Li, B., Zhao, M., Feng, L., Dou, C., Ding, S., Zhou, G., et al. (2020). Organic Nir-Ii Molecule with Long Blood Half-Life for In Vivo Dynamic Vascular Imaging. Nat. Commun. 11, 3102. doi:10.1038/s41467-020-16924-z
Li, H., and Vaughan, J. C. (2018). Switchable Fluorophores for Single-Molecule Localization Microscopy. Chem. Rev. 118, 9412–9454. doi:10.1021/acs.chemrev.7b00767
Li, X., Zhang, F., and Zhao, D. (2015). Lab on Upconversion Nanoparticles: Optical Properties and Applications Engineering via Designed Nanostructure. Chem. Soc. Rev. 44, 1346–1378. doi:10.1039/c4cs00163j
Liang, L., Feng, Z., Zhang, Q., Cong, T. D., Wang, Y., Qin, X., et al. (2021). Continuous-Wave Near-Infrared Stimulated-Emission Depletion Microscopy Using Downshifting Lanthanide Nanoparticles. Nat. Nanotechnol. doi:10.1038/s41565-021-00927-y
Liang, L., Qin, X., Zheng, K., and Liu, X. (2019). Energy Flux Manipulation in Upconversion Nanosystems. Acc. Chem. Res. 52, 228–236. doi:10.1021/acs.accounts.8b00469
Liu, B., Chen, C., Di, X., Liao, J., Wen, S., Su, Q. P., et al. (2020). Upconversion Nonlinear Structured Illumination Microscopy. Nano Lett. 20, 4775–4781. doi:10.1021/acs.nanolett.0c00448
Liu, Q., Zhang, Y., Peng, C. S., Yang, T., Joubert, L.-M., and Chu, S. (2018). Single Upconversion Nanoparticle Imaging at Sub-10 W Cm−2 Irradiance. Nat. Photon 12, 548–553. doi:10.1038/s41566-018-0217-1
Liu, Y., Lu, Y., Yang, X., Zheng, X., Wen, S., Wang, F., et al. (2017). Amplified Stimulated Emission in Upconversion Nanoparticles for Super-resolution Nanoscopy. Nature 543, 229–233. doi:10.1038/nature21366
Liu, Y., Zhou, Z., Wang, F., Kewes, G., Wen, S., Burger, S., et al. (2021). Axial Localization and Tracking of Self-Interference Nanoparticles by Lateral Point Spread Functions. Nat. Commun. 12. doi:10.1038/s41467-021-22283-0
Lu, Y., Zhao, J., Zhang, R., Liu, Y., Liu, D., Goldys, E. M., et al. (2013). Tunable Lifetime Multiplexing Using Luminescent Nanocrystals. Nat. Photon 8, 32–36. doi:10.1038/nphoton.2013.322
Ma, C., Shan, C., Park, K., Mok, A. T., Antonick, P. J., and Yang, X. (2020). Enhancing the Generating and Collecting Efficiency of Single Particle Upconverting Luminescence at Low Power Excitation. Nanophotonics 9, 1993–2000. doi:10.1515/nanoph-2019-0526
Masch, J.-M., Steffens, H., Fischer, J., Engelhardt, J., Hubrich, J., Keller-Findeisen, J., et al. (2018). Robust Nanoscopy of a Synaptic Protein in Living Mice by Organic-Fluorophore Labeling. Proc. Natl. Acad. Sci. USA 115, E8047–E8056. doi:10.1073/pnas.1807104115
Misgeld, T., Kerschensteiner, M., Bareyre, F. M., Burgess, R. W., and Lichtman, J. W. (2007). Imaging Axonal Transport of Mitochondria In Vivo. Nat. Methods 4, 559–561. doi:10.1038/nmeth1055
Ning, P., Dong, P., Geng, Q., Bai, L., Ding, Y., Tian, X., et al. (2017). A Two-Photon Fluorescent Probe for Viscosity Imaging In Vivo. J. Mater. Chem. B 5, 2743–2749. doi:10.1039/c7tb00136c
Pei, P., Chen, Y., Sun, C., Fan, Y., Yang, Y., Liu, X., et al. (2021). X-Ray-Activated Persistent Luminescence Nanomaterials for Nir-Ii Imaging. Nat. Nanotechnol. doi:10.1038/s41565-021-00922-3
Peng, X., Huang, B., Pu, R., Liu, H., Zhang, T., Widengren, J., et al. (2019). Fast Upconversion Super-resolution Microscopy with 10 μs Per Pixel Dwell Times. Nanoscale 11, 1563–1569. doi:10.1039/c8nr08986h
Rifka, V., Li, X., Marko, P., Diana, R., Shoji, H., Frank, H., et al. (2018). Sted Nanoscopy of the Centrosome Linker Reveals a Cep68-Organized, Periodic Rootletin Network Anchored to a C-Nap1 Ring at Centrioles. Proc. Natl. Acad. Sci. USA 115, E2246–E2253. doi:10.1073/pnas.1814449115
Rust, M. J., Bates, M., and Zhuang, X. (2006). Sub-Diffraction-Limit Imaging by Stochastic Optical Reconstruction Microscopy (Storm). Nat. Methods 3, 793–796. doi:10.1038/nmeth929
Sahl, S. J., Hell, S. W., and Jakobs, S. (2017). Fluorescence Nanoscopy in Cell Biology. Nat. Rev. Mol. Cel Biol 18, 685–701. doi:10.1038/nrm.2017.71
Sahl, S. J., Leutenegger, M., Hilbert, M., Hell, S. W., and Eggeling, C. (2010). Fast Molecular Tracking Maps Nanoscale Dynamics of Plasma Membrane Lipids. Proc. Natl. Acad. Sci. 107, 6829–6834. doi:10.1073/pnas.0912894107
Sharonov, A., and Hochstrasser, R. M. (2006). Wide-Field Subdiffraction Imaging by Accumulated Binding of Diffusing Probes. Proc. Natl. Acad. Sci. 103, 18911–18916. doi:10.1073/pnas.0609643104
Shen, J., Chen, G., Vu, A.-M., Fan, W., Bilsel, O. S., Chang, C.-C., et al. (2013). Engineering the Upconversion Nanoparticle Excitation Wavelength: Cascade Sensitization of Tri-doped Upconversion Colloidal Nanoparticles at 800 Nm. Adv. Opt. Mater. 1, 644–650. doi:10.1002/adom.201300160
Stephan, T., Roesch, A., Riedel, D., and Jakobs, S. (2019). Live-Cell Sted Nanoscopy of Mitochondrial Cristae. Sci. Rep. 9, 12419. doi:10.1038/s41598-019-48838-2
Su, X., Sun, X., Wu, S., and Zhang, S. (2017). Manipulating the Emission Intensity and Lifetime of NaYF4:Yb3+,Er3+ Simultaneously by Embedding it into CdS Photonic Crystals. Nanoscale 9, 7666–7673. doi:10.1039/c7nr01172e
Sun, T., Li, Y., Ho, W. L., Zhu, Q., Chen, X., Jin, L., et al. (2019). Integrating Temporal and Spatial Control of Electronic Transitions for Bright Multiphoton Upconversion. Nat. Commun. 10, 1811. doi:10.1038/s41467-019-09850-2
Tan, M., Li, F., Wang, X., Fan, R., and Chen, G. (2020). Temporal Multilevel Luminescence Anticounterfeiting through Scattering Media. ACS Nano 14, 6532–6538. doi:10.1021/acsnano.9b08326
Uno, K., Bossi, M. L., Irie, M., Belov, V. N., and Hell, S. W. (2019). Reversibly Photoswitchable Fluorescent Diarylethenes Resistant against Photobleaching in Aqueous Solutions. J. Am. Chem. Soc. 141, 16471–16478. doi:10.1021/jacs.9b08748
Wäldchen, S., Lehmann, J., Klein, T., van de Linde, S., and Sauer, M. (2015). Light-Induced Cell Damage in Live-Cell Super-resolution Microscopy. Sci. Rep. 5, 15348. doi:10.1038/srep15348
Wang, B.-k., Barbiero, M., Zhang, Q.-m., and Gu, M. (2019b). Super-Resolution Optical Microscope: Principle, Instrumentation, and Application. Front. Inf Technol Electron. Eng 20, 608–630. doi:10.1631/fitee.1800449
Wang, F., Deng, R., Wang, J., Wang, Q., Han, Y., Zhu, H., et al. (2011). Tuning Upconversion through Energy Migration in Core-Shell Nanoparticles. Nat. Mater 10, 968–973. doi:10.1038/nmat3149
Wang, F., and Liu, X. (2009). Recent Advances in the Chemistry of Lanthanide-Doped Upconversion Nanocrystals. Chem. Soc. Rev. 38, 976–989. doi:10.1039/b809132n
Wang, R., Li, X., Zhou, L., and Zhang, F. (2014). Epitaxial Seeded Growth of Rare-Earth Nanocrystals with Efficient 800 Nm Near-Infrared to 1525 Nm Short-Wavelength Infrared Downconversion Photoluminescence for In Vivo Bioimaging. Angew. Chem. Int. Ed. 53, 12086–12090. doi:10.1002/anie.201407420
Wang, S., Liu, L., Fan, Y., El-Toni, A. M., Alhoshan, M. S., Li, D., et al. (2019a). In Vivo High-Resolution Ratiometric Fluorescence Imaging of Inflammation Using Nir-Ii Nanoprobes with 1550 Nm Emission. Nano Lett. 19, 2418–2427. doi:10.1021/acs.nanolett.8b05148
Wang, Y.-F., Liu, G.-Y., Sun, L.-D., Xiao, J.-W., Zhou, J.-C., and Yan, C.-H. (2013). Nd3+-Sensitized Upconversion Nanophosphors: Efficient In Vivo Bioimaging Probes with Minimized Heating Effect. ACS Nano 7, 7200–7206. doi:10.1021/nn402601d
Wang, Y., Deng, R., Xie, X., Huang, L., and Liu, X. (2016). Nonlinear Spectral and Lifetime Management in Upconversion Nanoparticles by Controlling Energy Distribution. Nanoscale 8, 6666–6673. doi:10.1039/c6nr00812g
Wang, Y., Song, S., Zhang, S., and Zhang, H. (2019c). Stimuli-Responsive Nanotheranostics Based on Lanthanide-Doped Upconversion Nanoparticles for Cancer Imaging and Therapy: Current Advances and Future Challenges. Nano Today 25, 38–67. doi:10.1016/j.nantod.2019.02.007
Wang, Y., Zheng, K., Song, S., Fan, D., Zhang, H., and Liu, X. (2018). Remote Manipulation of Upconversion Luminescence. Chem. Soc. Rev. 47, 6473–6485. doi:10.1039/c8cs00124c
Wen, S., Liu, Y., Wang, F., Lin, G., Zhou, J., Shi, B., et al. (2020). Nanorods with Multidimensional Optical Information beyond the Diffraction Limit. Nat. Commun. 11, 6047. doi:10.1038/s41467-020-19952-x
Wildanger, D., Medda, R., Kastrup, L., and Hell, S. W. (2009). A Compact Sted Microscope Providing 3d Nanoscale Resolution. J. Microsc. 236, 35–43. doi:10.1111/j.1365-2818.2009.03188.x
Willets, K. A. (2013). Super-Resolution Imaging of Interactions between Molecules and Plasmonic Nanostructures. Phys. Chem. Chem. Phys. 15, 5345–5354. doi:10.1039/c3cp43882a
Wong, H.-T., Vetrone, F., Naccache, R., Chan, H. L. W., Hao, J., and Capobianco, J. A. (2011). Water Dispersible Ultra-small Multifunctional Kgdf4:Tm3+, Yb3+ Nanoparticles with Near-Infrared to Near-Infrared Upconversion. J. Mater. Chem. 21, 16589. doi:10.1039/c1jm12796a
Wu, Q., Huang, B., Peng, X., He, S., and Zhan, Q. (2017). Non-Bleaching Fluorescence Emission Difference Microscopy Using Single 808-Nm Laser Excited Red Upconversion Emission. Opt. Express 25, 30885–30894. doi:10.1364/OE.25.030885
Wu, R., Zhan, Q., Liu, H., Wen, X., Wang, B., and He, S. (2015). Optical Depletion Mechanism of Upconverting Luminescence and its Potential for Multi-Photon Sted-like Microscopy. Opt. Express 23, 32401–32412. doi:10.1364/OE.23.032401
Wu, Y., Xu, J., Poh, E. T., Liang, L., Liu, H., Yang, J. K. W., et al. (2019). Upconversion Superburst with Sub-2 μs Lifetime. Nat. Nanotechnol. 14, 1110–1115. doi:10.1038/s41565-019-0560-5
Xu, J., Dong, Z., Asbahi, M., Wu, Y., Wang, H., Liang, L., et al. (2021b). Multiphoton Upconversion Enhanced by Deep Subwavelength Near-Field Confinement. Nano Lett. 21, 3044–3051. doi:10.1021/acs.nanolett.1c00232
Xu, Y., Xu, R., Wang, Z., Zhou, Y., Shen, Q., Ji, W., et al. (2021a). Recent Advances in Luminescent Materials for Super-resolution Imaging via Stimulated Emission Depletion Nanoscopy. Chem. Soc. Rev. 50, 667–690. doi:10.1039/d0cs00676a
Yan, L., Zhou, B., Song, N., Liu, X., Huang, J., Wang, T., et al. (2018). Self-sensitization Induced Upconversion of Er3+ in Core-Shell Nanoparticles. Nanoscale 10, 17949–17957. doi:10.1039/c8nr04816a
Zhan, Q., Liu, H., Wang, B., Wu, Q., Pu, R., Zhou, C., et al. (2017). Achieving High-Efficiency Emission Depletion Nanoscopy by Employing Cross Relaxation in Upconversion Nanoparticles. Nat. Commun. 8, 1058. doi:10.1038/s41467-017-01141-y
Zhang, H., Chen, Z.-H., Liu, X., and Zhang, F. (2020). A Mini-Review on Recent Progress of New Sensitizers for Luminescence of Lanthanide Doped Nanomaterials. Nano Res. 13, 1795–1809. doi:10.1007/s12274-020-2661-8
Zhang, H., Fan, Y., Pei, P., Sun, C., Lu, L., and Zhang, F. (2019a). Tm 3+ ‐Sensitized NIR‐II Fluorescent Nanocrystals for In Vivo Information Storage and Decoding. Angew. Chem. Int. Ed. 58, 10153–10157. doi:10.1002/anie.201903536
Zhang, H., Jia, T., Chen, L., Zhang, Y., Zhang, S., Feng, D., et al. (2017). Depleted Upconversion Luminescence in NaYF4:Yb3+,Tm3+ Nanoparticles via Simultaneous Two-Wavelength Excitation. Phys. Chem. Chem. Phys. 19, 17756–17764. doi:10.1039/c7cp00099e
Zhang, H., Jia, T., Shang, X., Zhang, S., Sun, Z., and Qiu, J. (2016). Mechanisms of the Blue Emission of NaYF4:Tm3+ Nanoparticles Excited by an 800 Nm Continuous Wave Laser. Phys. Chem. Chem. Phys. 18, 25905–25914. doi:10.1039/c6cp04413a
Zhang, H., Li, Y., Lin, Y., Huang, Y., and Duan, X. (2011). Composition Tuning the Upconversion Emission in Nayf4:Yb/Tm Hexaplate Nanocrystals. Nanoscale 3, 963–966. doi:10.1039/c0nr00823k
Zhang, Z., Jayakumar, M. K. G., Zheng, X., Shikha, S., Zhang, Y., Bansal, A., et al. (2019b). Upconversion Superballs for Programmable Photoactivation of Therapeutics. Nat. Commun. 10, 4586. doi:10.1038/s41467-019-12506-w
Zhao, J., Jin, D., Schartner, E. P., Lu, Y., Liu, Y., Zvyagin, A. V., et al. (2013a). Single-Nanocrystal Sensitivity Achieved by Enhanced Upconversion Luminescence. Nat. Nanotech 8, 729–734. doi:10.1038/nnano.2013.171
Zhao, J., Lu, Z., Yin, Y., McRae, C., Piper, J. A., Dawes, J. M., et al. (2013b). Upconversion Luminescence with Tunable Lifetime in Nayf4:Yb,Er Nanocrystals: Role of Nanocrystal Size. Nanoscale 5, 944–952. doi:10.1039/c2nr32482b
Zhao, M., Li, B., Wu, Y., He, H., Zhu, X., Zhang, H., et al. (2020). A Tumor‐Microenvironment‐Responsive Lanthanide-Cyanine FRET Sensor for NIR‐II Luminescence‐Lifetime In Situ Imaging of Hepatocellular Carcinoma. Adv. Mater. 32, 2001172. doi:10.1002/adma.202001172
Zhao, M., Li, B., Zhang, H., and Zhang, F. (2021). Activatable Fluorescence Sensors for In Vivo Bio-Detection in the Second Near-Infrared Window. Chem. Sci. 12, 3448–3459. doi:10.1039/d0sc04789a
Zhong, Y., Ma, Z., Wang, F., Wang, X., Yang, Y., Liu, Y., et al. (2019). In Vivo Molecular Imaging for Immunotherapy Using Ultra-bright Near-Infrared-Iib Rare-Earth Nanoparticles. Nat. Biotechnol. 37, 1322–1331. doi:10.1038/s41587-019-0262-4
Zhong, Y., Ma, Z., Zhu, S., Yue, J., Zhang, M., Antaris, A. L., et al. (2017). Boosting the Down-Shifting Luminescence of Rare-Earth Nanocrystals for Biological Imaging beyond 1500 Nm. Nat. Commun. 8, 737. doi:10.1038/s41467-017-00917-6
Zhou, J., Deng, J., Zhu, H., Chen, X., Teng, Y., Jia, H., et al. (2013a). Up-Conversion Luminescence in Laf3:Ho3+Via Two-Wavelength Excitation for Use in Solar Cells. J. Mater. Chem. C 1, 8023. doi:10.1039/c3tc31581a
Keywords: super-resolution imaging, lanthanide-doped nanocrystals, stimulated emission depletion nanoscopy, fluorescence emission difference nanoscopy, in vivo imaging
Citation: Zhang H, Zhao M, Ábrahám IM and Zhang F (2021) Super-Resolution Imaging With Lanthanide Luminescent Nanocrystals: Progress and Prospect. Front. Bioeng. Biotechnol. 9:692075. doi: 10.3389/fbioe.2021.692075
Received: 07 April 2021; Accepted: 16 August 2021;
Published: 30 September 2021.
Edited by:
Yao Sun, Central China Normal University, ChinaReviewed by:
Xusan Yang, Cornell University, United StatesDambarudhar Mohanta, Tezpur University, India
Copyright © 2021 Zhang, Zhao, Ábrahám and Zhang. This is an open-access article distributed under the terms of the Creative Commons Attribution License (CC BY). The use, distribution or reproduction in other forums is permitted, provided the original author(s) and the copyright owner(s) are credited and that the original publication in this journal is cited, in accordance with accepted academic practice. No use, distribution or reproduction is permitted which does not comply with these terms.
*Correspondence: Hongxin Zhang, zhanhonxin@163.com; Fan Zhang, zhang_fan@fudan.edu.cn