- 1Advanced Cellular Therapy Laboratory, Fundação Hemocentro de Ribeirão Preto, São Paulo, Brazil
- 2Cell Therapy Laboratory, Fundação Hemocentro de Ribeirão Preto, São Paulo, Brazil
- 3Department of Medical Images, Hematology and Clinical Oncology, Ribeirão Preto Medical School of University of São Paulo, Ribeirão Preto, Brazil
CAR-T cell therapies have been recognized as one of the most advanced and efficient strategies to treat patients with hematologic malignancies. However, similar results have not been observed for the treatment of solid tumors. One of the explanations is the fact that tumors have extremely hostile microenvironments for the infiltration and effector activity of T-cells, mainly due to the presence of highly suppressive cytokines, hypoxia, and reactive oxygen species. Taking advantage of cytokines functionally, new fourth-generation CAR constructs have been developed to target tumor cells and additionally release cytokines that can contribute to the cytotoxicity of T-cells. The manufacturing process, including the use of cytokines in the expansion and differentiation of T cells, is also discussed. Finally, the clinical aspects and the influence of cytokines on the clinical condition of patients, such as cytokine release syndrome, who receive treatment with CAR-T cells are addressed. Therefore, this review aims to highlight how important cytokines are as one of the major players of cell therapy.
Introduction
CAR-T cell therapy has recently emerged as a promising treatment for hematological malignancies (1, 2). Its effectiveness depends on an entire formation process, from construct engineering and manufacturing to clinical response. During these steps, cytokines perform distinct roles that directly impact the efficiency of this therapeutic resource once they are closed related to the regulation of inflammatory pathways. In addition, cytokines have an important contribution to the development of the suppressor tumor microenvironment (TME) given opportunities to design new strategies and alternatives for TME immunomodulation. For this purpose, new CAR vectors of fourth generation have being designed to incorporate, in the addition of CAR construct, other genes able to express pro-inflammatory cytokines that might favor the efficacy of this therapies (2, 3).
In the manufacturing process of CAR-T cells, cytokines have a key role in the activation, expansion of immune cells and in the selection/polarization in subpopulations, such as memory T cells. Memory T cells potentially might impact patient outcome due to their superior persistence, resistance to apoptosis, and inferior predisposition to the development of exhaustion (4).
After the infusion of CAR-T cells, patients potentially experiment a cytokines storm condition, called as Cytokine Release Syndrome (CRS) (5). In terms of clinical response, despite the outstanding performance of CAR-T cells for hematological neoplasm treatment, their successful application in solid tumors still presents several challenges. The selection of potential tumor-specific target antigens and tumor stroma provide a physical barrier to the CAR-T cells infiltration to the TME (2, 6), in addition to the immunosuppressive TME, a well-known down-regulating mechanism of the immune cells antitumor response (7). Among the mechanisms of immunosuppression and tumor immunoescape are the secretion of regulatory cytokines, recruitment and polarization of regulatory T cells, myeloid suppressor cells (e.g., TAM, TAN, MDSC), and expression of immune checkpoints that lead to T-cell anergy and depletion of essential amino acids for the proliferation of specific lymphocytes (8, 9).
This review aims to critically discuss the cytokines as an important player in the context of a highly complex scenario of CAR-T cell therapy for cancer focused on discussing the implications of cytokines functionalities in the design and engineering of new CAR constructs, manufacturing protocols and in the clinical response.
The role of cytokines in the maintenance of the immunosuppressive phenotype and the impact on the CAR-T cells function
Tumors are complex tissues that contain, in addition to tumor cells, endothelial cells, pericytes, inflammatory cells, fibroblasts, as well as acellular components, such as cytokines, lipid mediators and extracellular matrix. Within the TME, different elements are capable of inducing angiogenesis, promote the survival and proliferation of tumor cells and inactivate T cells antitumor responses through various mechanisms (10).
Tumor-infiltrating inflammatory cells are attracted to TME up cytokines secreted by tumor cells (11). In the first instance, they constitute an important initial line of defense against the tumor, through the generation of reactive oxygen species, secretion of pro-inflammatory cytokines, and mediating cytotoxicity. However, this pro-inflammatory environment is counterbalanced with the development of a chronic inflammation into the TME, leading the local tissue microenvironment to change into a growth-promoting state (12, 13). Cytokines have a prominent role in this scenario, once they are able to modulate the antitumor responses, cell transformation and malignancy proliferation due to the chronic inflammation (14).
Despite being a genetically modified T cells, CAR-T cells undergo the same process of turning off responses as non-genetically modified cells. Taking advantage of cytokines function, the TME cells impact negatively the proliferation, differentiation, activation, migration and survival of immunological cells which constitute a hostile microenvironment; variables closely correlated with the biology of immunological CAR-T cell therapy (15).
IL-2 and TGF-β
Some cytokines induce patterns response pro-tumor such as TGF-β, secreted by two major types of immunosuppressive cells of the TME, the myeloid-derived suppressor cells (MDSCs) and regulatory T cells (Tregs) (16).
Based on the TGF-β activity, the differentiation of Tregs (CD25+Foxp3+) impact the response of T cells and the neutralization of their activities is strongly implicated with the anti-tumor immune response (17). Tregs limit the expansion of effector T cells in the TME by capturing IL-2, as they have a high affinity trimeric receptor to this cytokine (7). Moreover, in vitro experiments showed that TGF-β suppressed the proliferation of human T cells through inhibition of the IL-2 receptor (18) and it reduces the production of IL-2 itself by inhibiting the activity of the IL-2 promoter. In vivo, TGF-β promoted downregulation of CD8+ and CD4+ T cell expansion (19) and cytotoxic T cells activity by several mechanisms, such as inhibition of the expression of perforin, granzyme and INF-y (20, 21). In mice, the deficiency of the production of TGF-β1 and TGF-β receptor in T cells, correlated with greater proliferation and activation of T cells subtypes helper 1 (Th1), as well as the production of Th2 cytokines (22, 23).
The close relationship of TGF-β with the IL-2 has important manufacturing and clinical implications, once most strategies for CAR-T cell processing are the IL-2 based manufacturing protocols. IL-2 is an important cytokine that can promote a relatively mature phenotype, with low expression of CD62L, CCR7, CD27 and CD28, which correlated with a reduced blood persistence in vivo and terminal effector T cells differentiation and proliferation (19).
To overcome the action of TGF cytokine’s immunosuppressive actions of the TME, the use of mesothelin-targeted chimeric antigen receptor T cell therapy together with an oncolytic adenovirus (rAd.sT) expressing sTGFβRIIFc (soluble transforming growth factor beta receptor II-Fc fusion protein) demonstrated enhanced anti-tumor responses against breast cancer tumor cells (24). Besides, Kloss and colleagues generated dominant-negative TGF-βRII-modified CAR-T cells specific for prostate-specific membrane antigen (PSMA), that exhibited superior level of proliferation, cytokine secretion, resistance to dysfunctionality related to chronic inflammation, long-term in vivo persistence, and the induction of tumor eradication in aggressive human prostate cancer mouse models (25).
Other studies have shown that the inhibition of the endogenous TGF-β receptor (TGFBR2) signal in CAR-T cells enhanced the anti-tumor function of ROR1-specific CAR-T cells against triple-negative breast cancer by reducing Treg conversion and also prevented the exhaustion of CAR-T cells (26, 27).
Potential strategies to enhance clinical response of CAR-T cell therapy might be targeting TME cells involved in the immunosuppression or combined cytokines production that could positively impact antitumor responses of immune cells, including the genetic modified ones, such as CAR-T cells.
IL-4
IL-4 is involved in the differentiation of naive CD4 T cells. It can favor tumor growth by inhibiting tumor-directed Th1-polarized effector and inducing Th2 cells, which have been associated with less antitumor activity than IFN-ℽ producing CD4 T cells (Th1). Additionally, several cancer types express IL-4 and the IL-4R, which suggests a role in tumor progression cancer (28).
However, modified cytokine receptors, including dominant negative receptor (DNR) and inverted cytokine receptor (ICR), can redirect this immunosuppressive cytokine in the TME towards pro-inflammatory response. Base on the knowledge that IL-7 is implicated with the proliferation and diversity of effector T cells in the TME with consequent superior antitumor effect, some strategies have been to merge the IL-7 receptor endodomain to the IL-4 receptor exodomain. This approach might be able, in the clinics, to promote a positive effect on cell expansion by the action of the IL-7 receptor endodomain after the recognition of IL-4 present in the TME (29).
IFN- ℽ, a dual cytokine
IFN-ℽ is a potent proinflammatory agent that plays an important role in cellular immunity and anti-tumor responses due to its cytostatic, antiproliferative, and pro-apoptotic effect on tumor cells, in addition to stimulating pro-inflammatory macrophages and inducing apoptosis of Tregs (30). The main sources of IFN-ℽ are innate immunity cells, NK and NKT cells, and adaptive immunity cells, CD4 and CD8 T cells (10). Despite all the afore mentioned antitumor effects, IFN-ℽ can contribute to the tumor’s escape from immune surveillance. It has been reported that in some tumors, such as NSCLC (31) and ovarian cancer (32), IFN-ℽ can induce the expression of PD-L1 and IDO. Models of hepatoma, mammary adenocarcinoma and melanoma showed that chronic exposure to low doses of IFN-ℽ leads to tumor growth and induces the expression of molecules, such as PD-L1, PD-L2, CTLA-4 and Foxp3 (33), which are implicated to the dysfunction of T cell activity. Another way that IFN-ℽ can contribute to tumor progression is by generating genetic instability in cancer cells, which could lead to the alteration of tumor antigens and immune escape (34). Taking advantage of this knowledge, Zhang et al. (31) demonstrated that the IL-6/IFN-γ double knockdown CAR-T cells reduce the release of multiple cytokines from PBMCs in vitro, showing a promise strategy to be translated to the clinics.
IL-6
Interleukin 6 is described as an important cytokine in tumor initiation and progression. Many studies reveal that high concentrations of IL-6 in the serum of patients with different types of cancer (such as gastric, melanoma, colorectal, pancreatic, among others) is related to poor prognosis of patients (35). The primary sources of IL-6 are tumor cells, MDSC, TAM, fibroblasts and CD8+ T cells. The effects of IL-6 can be direct on tumor cells, as well as indirectly through the promotion of angiogenesis and the modulation of stromal cells (36). Directly, IL-6 binds to its receptor on tumor cells and activates the STAT3 signaling pathway, which will lead to tumor progression through cyclin D1 and the proto-oncogene c-myc, an important regulator of the progression of G1 to S phase of the cell cycle (37). On the your side, STAT3 induces the anti-apoptotic proteins bcl-2, bcl-XL and survivin (38), which contribute to the survival of tumor cells. IL-6 also promotes the angiogenesis and metastasis that contribute to the expression of matrix metalloproteinase 2 (MMP-2) (39), expression of VEGF and bFGF (40) by tumor cells and also favor metastasis events by attracting metastatic cells outside the primary site of the tumor (41).
An interesting in vivo study demonstrated that ssCART-19 cells with shRNA-IL-6 gene knockdown resulted in increased interferon-gamma (IFN-γ), tumor necrosis factor (TNF), interleukin-2 (IL-2), and IL-17A and decreased IL-10 and IL-6 levels. ssCART-19 inhibited the proliferation of Raji-Luc cells in tumor-bearing NSG mice, and reduced the incidence of lymphomas in the liver, kidneys, and spleen. The ssCART-19 DNA was mainly concentrated in the liver within 3 hours, and was widely distributed in most of the organs/tissues for 4 weeks after administration, demonstrating that ssCART-19 with shRNA-IL-6 gene knockdown prolongs the survival time of tumor-bearing mice with a potential inferior risk of immunotoxicity and tumorigenicity (42).
It becomes increasingly important to better understand and take advantage of cytokines in the tumor’s biogenesis to modify the hostile TME and improve the therapeutic effect of CAR-T cells. In Figure 1 it is shown the cytokines dynamics in the hostile TME.
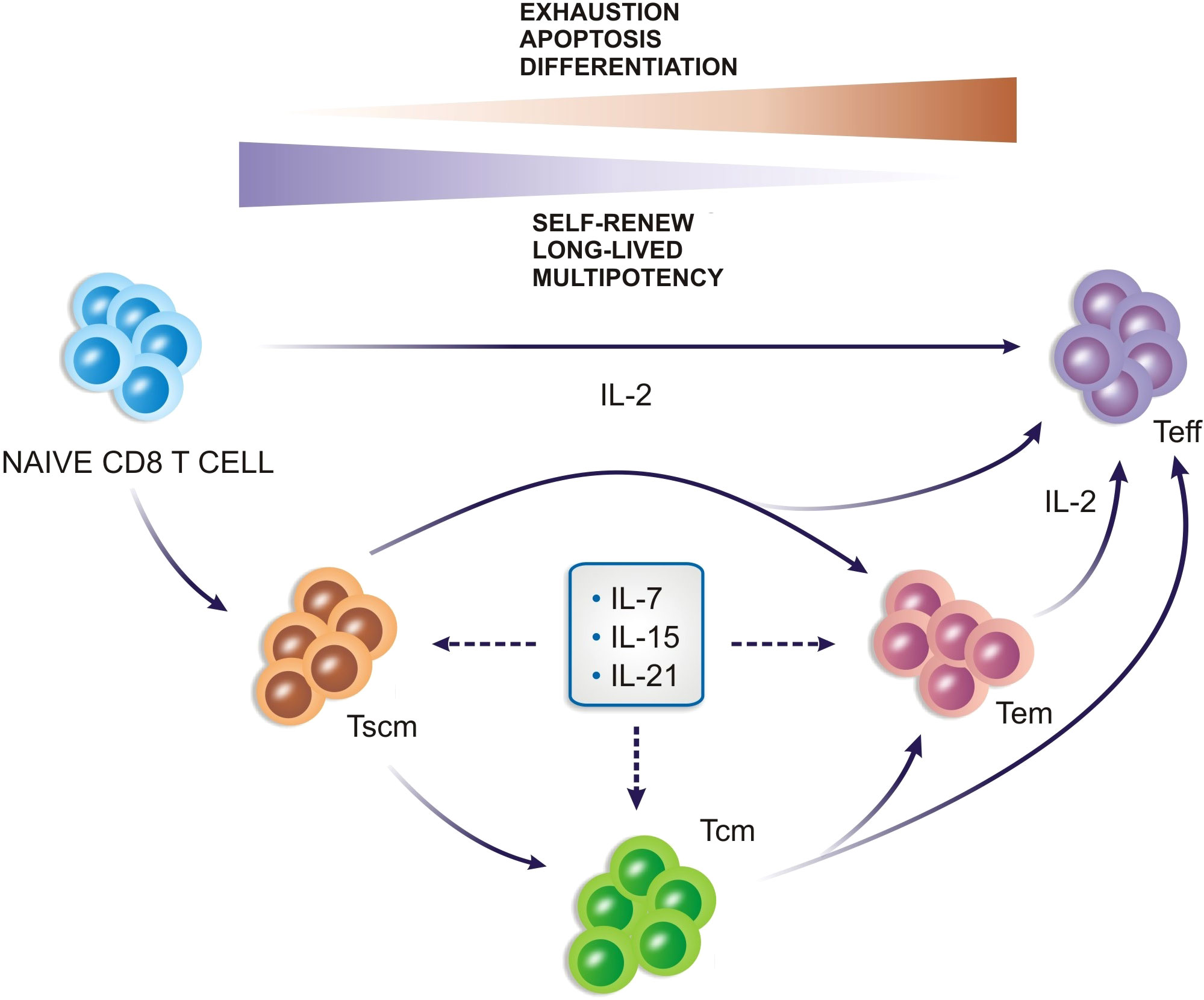
Figure 1 The cytokines dynamics in the tumor microenvironment and their role in the maintenance of the immunosuppressive phenotype. The microenvironment becomes hostile to the proliferation and effector functions of T cells and CAR-T cells.
T-cells redirected for universal cytokine killing
Given the importance of cytokines in the biology of immunological therapies, including CAR-T cells, numerous studies have looked to modulate the profile of cytokines intended to enhance the effectiveness of these cells against diverse types of tumors (43–45).
Recently, a new generation of CAR T-cells was developed, “T-cells redirected for antigen-unrestricted cytokine-initiated killing” (TRUCKs), with the aim to associate the cytotoxicity of CAR T-cells with the delivery in situ of cytokines, aiming to circumvent the tumor microenvironment and modulating the immune system (43–46).
These CAR-T cells are modified with an (inducible) cytokine production cassette, such that the release of the cytokine is conditioned to the recognition of the antigen by the T cell and its activation (3, 47). This process requires the transfer of two transgenes (CAR and cytokine), which require integration into different genomic sites to avoid transactivation of the inducible cassette by the constitutive CAR promoter (3, 48).
Initially designed by Chmielewski et al., the study used the carcinoembryonic antigen (CEA)-specific CAR with the additional expression of IL-12, in vitro and in vivo models, demonstrated that IL-12-secreting CAR-T cells produced a greater pro-inflammatory response, improving T cell activation, modulated the tumor’s immune and vascular environment, and recruited additional immune cells, such as antitumor macrophages to the tumor microenvironment, significantly improving the antitumor response (46).
Previously, some approaches were tested with the aim to use IL-12 in the clinical setting. The use of IL-12 was implicated with systemic toxicity events and not feasible in some types of metastatic tumors. Alternatively, the to deliver IL-12 locally to the tumor lesion was also explored with no encourages results (1, 2, 6, 7). Compared with those strategies, CAR and iIL-12–modified T cells have the advantage to deliver IL-12 in a targeted tissue, and with satisfactory results, demonstrating to be a very promising approach (46).
In ovarian cancer which has a high immunosuppressive tumor environment (8, 49), the use of Muc16ecto specific CAR T cells modified to secrete IL-12 against ovarian cancer cells demonstrated by Koneru and colleagues (50) greater proliferation and increased IFN-γ secretion compared to CAR-T cells without IL-12. In vivo, the same study showed higher survival, prolonged persistence, and superior systemic IFN-γ, demonstrating greater antitumor efficacy of these cells (50). Subsequently, the same group extended their previous work using a syngeneic model of murine ovarian peritoneal carcinomatosis to characterize the mechanisms of these IL-12 secreting T CAR cells. Their data suggested that secreting CAR-T cells mediated tumor-associated macrophage depletion and resisted the inhibition induced by endogenous PD-L1, demonstrating that CAR IL-12 T cells were able to overcome these barriers by exercising a more efficient response. In addition, they have also demonstrated a potential acceptable profile of the toxicity of this therapy (50).
Currently, a panel of cytokines including IL-12, IL-7, IL-15, IL-18, IL-22, and IL-23 are being investigated. The IL-18 investigation came up with interesting results. Previous studies have shown that, in some models, higher levels of IL-18, in the absence of Th1 cytokines, promoted tumor progression (51) favored the occurrence of metastasis, and suppressed the function of NK cells by positive regulation of PD-1 (52, 53). On the other hand, other studies demonstrate that IL-18 supports an anti-tumor response of Tc1 cells by positive regulation of T-bet and suppression of Tc2 cells (54, 55). Recently, analyzing refractory pancreatic and lung tumors, Chmielewski and colleagues (56) demonstrated that IL-18 can polarize CAR T cells towards T-bethighFoxOlow effectors producing an acute inflammatory response. These cells (CAR-T/IL-18) induced a general change in the profile of immune cells associated with the tumor, increasing the number of CD206-M1 macrophages and NKG2D+ NK cells, and decreasing Tregs, suppressive CD103+ DCs, and M2 macrophages, besides prevented exhaustion of CAR T cells in the long term. They conclude that the association of IL-18 with CAR T cells are promisor strategy for the treatment of advanced solid cancer, as these cells demonstrated to induce acute inflammatory response and alter the balance of pro- and anti-inflammatory cells in the TME (56).
IL-23 is another cytokine that does not have an entirely clear role in tumor progression, however, in the context of immunotherapy, the association of this cytokine with CAR-T cells has also shown to be promising. MA et al. developed a strategy to couple the release and activity of IL-23 with the activation of T cells through the supplementation of T cells with the subunit IL-12β p40. The T cells that expressed p40 (p40-Td cells) produced IL-23 after activation, inducing a greater proliferation and survival of these T cells. This work demonstrated that the integration of p40 in CAR-T cells was able to increase its antitumor activity in xenograft models and syngeneic mice. This approach was also shown an acceptable safety profile, because the IL-23 produced by p40-Td cells act mainly through an autocrine mechanism with inferior effect on non-tumor cells (57).
Investigating the IL-22 functionality in a head and neck squamous cell carcinoma (HNSCC) model, a comparison of a second-generation CAR T cells with fourth-generation CAR-T cells merged with this cytokine (55) was done. In vitro data demonstrated that IL-22 positively regulates central memory and effective memory T cells in CD3+ T cells, resulting in higher rates of proliferation and survival of these cells. In vivo, the CAR-MUC1-IL-22 T cells were able to progressively decrease the size of tumors more efficiently than second-generation cells. Investigators attributed this effect to the inferior persistence of CAR-MUC1 T cells, while IL-22 can facilitate the differentiation of CAR-T cells into a TCM phenotype and improve CAR-MUC1-IL-22 T cells activity.
Chen, et al. (58) investigated a construct for the NB GD2 antigen with IL-15 (GD2.CAR.15) and without (GD2.CAR) in a xenogeneic model of neuroblastoma in vitro and in vivo. The construct with IL-15 secretion induced increased memory T cells with stem cell phenotypes, in addition to lower expression of PD-1. In vivo, they also tested the continue reexposure of GD2.CAR to tumor cells and the results further highlighted the role of IL-15 to promote superior survival of CAR-T cells with greater antitumor activity (58).
On your side, Lanitis et al. used a bicistronic vector with the coexpression of murine IL-15 and CAR in T cells. They observed that in non-activated IL-15 secreting CAR-T, there were low levels of IL-15 in the culture supernatant, but after antigenic stimulation there was a significant increase, proving that the secretion of IL-15 is conditioned to cell activation CAR-T by the antigen. The functionality of co-expressed IL-15 was demonstrated by increased proliferation and survival when compared to second-generation CAR-T cells, in addition to also promoting a TCM cell phenotype, inferior expression of PD-1 and superior cell activity after the antigenic challenge. They also evaluated the efficacy of 4G-CAR-T cells in vivo in a B16 melanoma tumor model and found that 4G-CAR-T cells showed greater persistence and greater efficacy against tumor cells when compared to CAR-T cells without IL-15 secretion (59).
Adopting another approach, Batra et al. (60) tested the cytokines IL-15 and IL-21 associated with CAR-T cells separately and together in a model of hepatocellular carcinoma (HCC). They showed that CAR-T cells that coexpress IL-15 and/or IL-21 were effective against tumor cells. CAR-T cells with the combined expression of IL-15 and IL-21 showed a less differentiated profile and longer survival in repeated exposures to tumor cells, in addition to maintaining the expression of T-cell factor-1 (TCF-1), an important factor for the development and survival of T cells. In vivo experiments observed that these cells (CAR-T/IL-15/IlL-21) showed a larger expansion and persistence, resulting in greater control of tumor burden and survival of animals when compared to only CAR-T cells and CAR-T cells with the secretion of isolated cytokines. These results demonstrated the superiority of the efficiency of the approach with the coexpression of IL-15 and IL-21 in the preclinical scenario, given the data needed to launch a phase I clinical trial that will recruit patients with liver tumors (NCT02932956 and NCT02905188) (60).
Recently, Duan et al. (61) working with fourth-generation CAR-T cells targeting BCMA and IL-7 and CCL19 expression (CAR-T BCMA-7×19) demonstrated that these cells had a greater capacity for expansion, differentiation, migration, and cytotoxicity. They are currently conducting a clinical trial in humans for Refractory/Recurrent Multiple Myeloma therapy using these BCMA-7×19 CAR-T cells (ClinicalTrials.gov Identifier: NCT03778346). Preliminary data of this clinical trial demonstrated that two patients receiving the treatment achieved a good response with low levels of CRS, and responses lasting more than 12 months (61).
Diverse factors impact the persistence and effectiveness of CAR T-cells, such as the presence of immunosuppressive cytokines, loss of antigen expression, T cellular exhaustion or dysfunctionality, and the variability of TME of different cancer subtypes. Cytokines functions have been seen as a great player that might give opportunities to be explored to circumvent these obstacles. In general, the association of CAR-T cells with cytokines might modulate the tumor microenvironment to privilege a pro-inflammatory profile and to recruit other cells of the immune system that traduce in superior persistence and effectiveness of CAR T cells with an acceptable safety profile (Table 1).
In summary, the TRUCKs approach demonstrates a substantial translational potential, and currently, is already being explored in clinical protocols, summarized in Table 2.
The role of cytokines in the CAR-T cells manufacturing process
The CAR-T cell manufacturing platform comprises several steps where the cytokines have a central role in the quality and functionality of the final product. Firstly, the isolation and enrichment of the T cells. CD3+ T cells are commonly obtained from peripheral blood mononuclear cells (PBMCs), harvested from leukapheresis, and further separated by lymphocyte separation medium centrifugation by manually or automated systems. Secondly, the enrichment of specific subsets of T cells could be achieved by using magnetic microbeads such as CD3+, CD4+, CD8+, CD25+, being also used for the selection or depletion of specific T cell types within the PBMCs enabling T cell expansion and administration of the final cell product with a defined CD4:CD8 ratio (47, 62). Thirdly, the gene transfer system is normally done through viral vectors transferring the corresponding genetic information into the T cells mediating CAR expression on the T cell surface. Virus-based gene delivery systems are commonly used, and they can achieve high transduction efficiency rates. Finally, CAR-T cells are expanded by ex vivo culture methods and the final cell product is subjected to end-of-process formulation and cryopreservation (47, 62). Quality control testing is performed during the production as well as for the final cryopreserved product (63). These steps of CAR-T cell manufacturing are described in Figure 2.
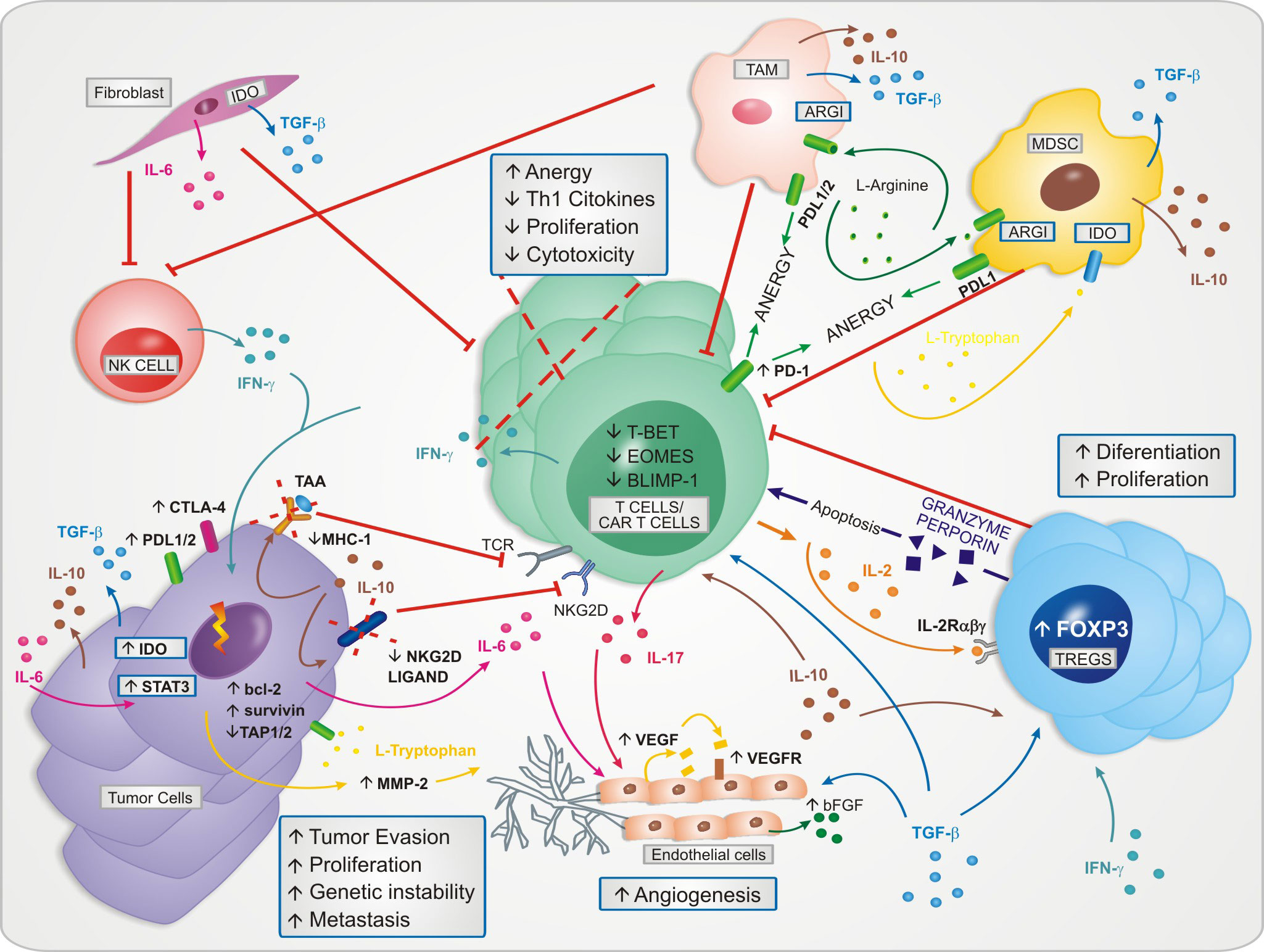
Figure 2 A representative scheme of CAR-T cell manufacturing platform. The definition of equipment, steps and protocols used depend on the patient, the type of tumor and clinical setting.
Cytokines play an important role on the activation, expansion and quality of T cells for CAR-T cells manufacturing. T cells are cultured in specific mediums generally supplemented with important cytokines such as IL-2, IL-7, IL15, and IL-21, influencing the composition, quality, and phenotype of the adoptively transferred T cells. The two most used strategies for CAR-T cell production are based on either IL-2 or IL-7, with or without IL-15 (64).
As mentioned before, IL-2 drives terminal effector T cells differentiation and proliferation by upregulating perforin, granzyme B and IFN-γ and suppressing the memory cell marker, such as BCL6 and IL7RA (65, 66). IL-2 is an important cytokine to T cell culture; however, it can promote a relatively mature phenotype, with low expression of CD62L, CCR7, CD27 and CD28, which correlated with a reduced blood persistence in vivo (67). In addition, it is known that IL-2 favors the expansion of regulatory T cells that can inhibit the anti-tumor activity of the CAR-T cells (4, 68).
The receptors for cytokines of the γ-chain family, such as IL-7, IL-15, and IL-21, have a common CD132 or γ-chain and can reduce the CAR-T cells terminal differentiation and increase the frequency of memory stem cells, yielding improved in vivo persistence (69).
It was reported that supplementation of IL-15 alone can lead to reduced exhaustion marker expression, an increase of anti-apoptotic properties providing similar performance in stimulating CAR-T cell expansion, persistence in vivo and tumor-lysis functions in vitro. IL-21 is another cytokine implicated with CAR-T cells expansion memory phenotype, however, potentially correlated with inferior expansion when compared with IL-2 processing (70).
IL-7/IL-15 have shown an enhancement in activation and ex vivo proliferation, with inferior exhaustion markers when compared to IL-2 (84). Additionally, it was reported that a combination of IL-7/IL-15 promotes the survival and maintenance of less differentiated T cells, while IL-15 and IL-21 seem better suited for in vivo administration after CAR-T cell infusion and triggering differentiation in memory cells. CAR-T cells that were exposed to IL-2 and IL-15 secreted more proinflammatory cytokines and presented stronger tumor-lysis ability in vitro (70).
In summary, the CAR-T cell manufacturing protocols have to be supplemented with specific cytokines during ex vivo production. Currently, studies mainly rely on IL-2, IL-7, IL-15, and IL-21 Figure 3. The optimal cytokine composition, as well as the role of other cytokines for CAR-T cell generation, is not clearly defined yet (70), mainly because it depends on the type of final product defined according to the patient, tumor, and clinical scenario.
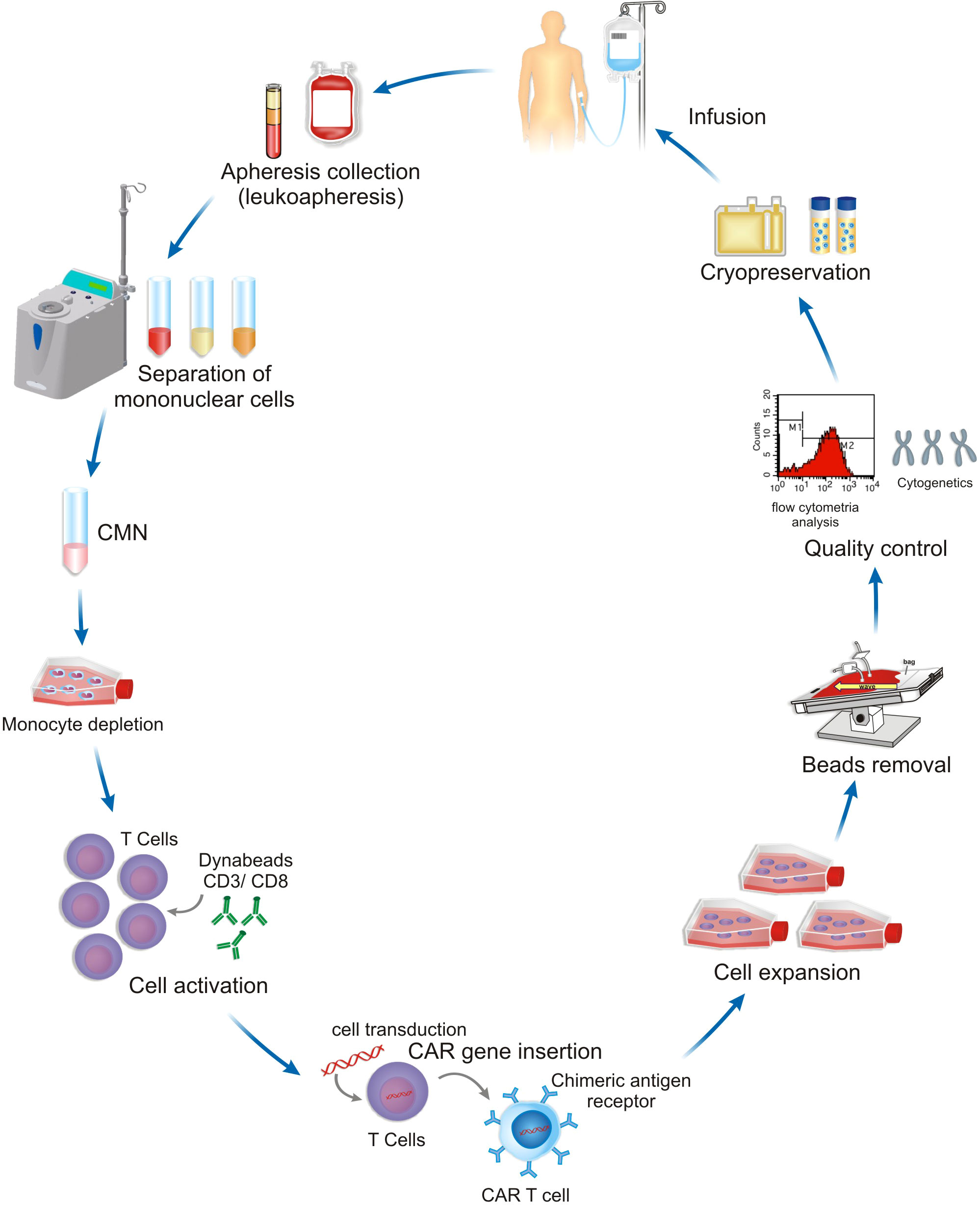
Figure 3 Cytokines play an important role in the generation of CD8+ T cells and can drive memory cell profiles.
Cytokine release syndrome
Although the use of CAR T cells is still considered an innovative treatment, several clinical complications after infused cells have been well identified. The CRS is the most prominent side effect that is not clearly elucidated (71, 72). According to first clinical trials, up to 90% of patients treated with CAR-T will develop CRS, and half of the patients progressed to grade 3–4 or even cause death. CRS is characterized by the increase of serum cytokines levels, inflammatory markers, and generalized activation of the immune system then infusion effector cells (72–80).
Usually, CRS occurs between the first and the fourteenth day after the infusion with a wide expression of clinical manifestations. Fortunately, most patients will take a self-limited clinical course. However, few patients might require the use of anti-IL 6 specific treatment, such as tocilizumab, or high doses of steroids and the need of intensive care unit (73, 81). Initial clinical manifestation may include fever, myalgia, fatigue and might progress into hypoxia, capillary leakage, important organ dysfunction that implicate with a life-threatening condition (5, 67).
CAR-T cells have an important role in the genesis of the cytokine storm, however other immunological cells are also implicated, such as macrophages and endothelial cells (67, 72–74, 82). It was observed increases in F4/80int-loLy6Cint-hi macrophages, which, following administration of mCD40L CD19 CAR T-cells engineered to further engage macrophages, led to markedly increased macrophage numbers, CRS symptoms, and mortality. IL-6 was predominantly produced by these macrophages, and blockade with anti-murine IL-6R antibody or with anakinra. Macrophages are also implicated with another mechanism in the genesis of CRS, the inducible nitric oxide synthase (iNOS) and nitric oxide production pathway. In addition to IL-6, other cytokines are involved in CRS such IL-1, IFN-γ, TNFα, IL-8 and IL-10, GM-SCM, also showing the importance of the cytokines in the clinical setting, as shown in the Figure 4 (67, 72–74, 82).
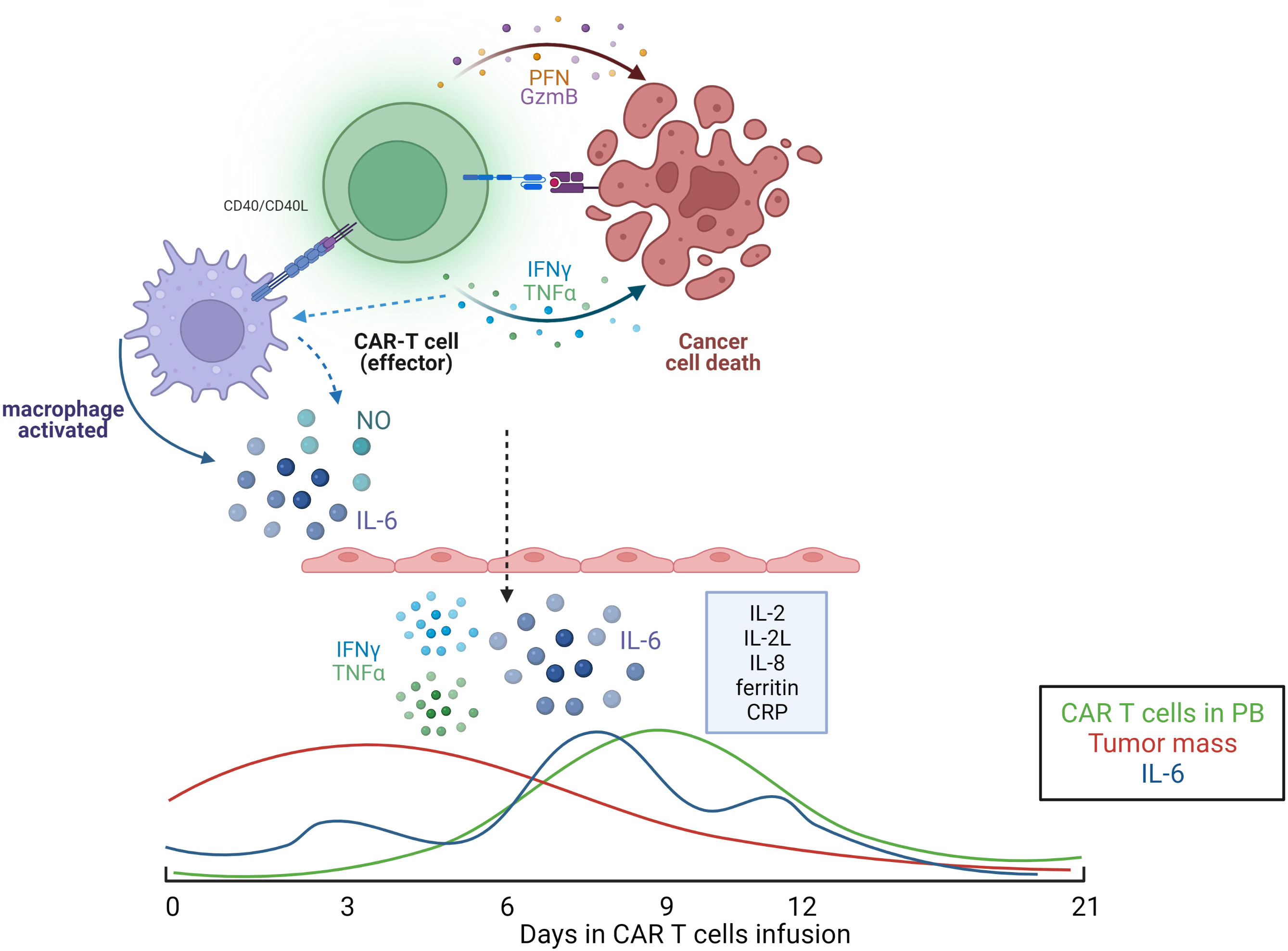
Figure 4 Illustrative model of cytokine secretion mechanism commonly observed in patients receiving CAR-T cell therapy. Below, kinetics of released factors involved in CRS. Illustration was created using BioRender.
Several recent studies have looked at alternative CAR T-cell strategies to improve the safety profile, such as engineering CAR T-cells with suicide genes, ON- and OFF- switches, AND/OR logic gating, or various inhibitory domains. However, these strategies directly limit CAR T-cell function (82). A possible alternative to circumvent the effects of IL-6 on CRS and preserve the antitumor activity of CAR-T cell was addressed in a study that developed a CD19 CAR T cell with a membrane-bound scFv targeting IL-6 constitutively expressed on its surface. In this approach, CAR-T cells might function as an IL-6 scavenger (83).
Another proposed mechanism by which CAR T-cells activate macrophages is direct activation through secretion of granulocyte macrophage colony-stimulating factor (GM-CSF) and GM–CSF is specifically upregulated in a CAR-dependent manner (82). This condition has led to speculation that GM–CSF may play a key role in monocyte activation, resulting in CRS association with CAR-T cell therapy. In order to reduce the release of GM-CFS, CRISPR/Cas9-mediated knockout of GM–CSF in CAR-T cells and consequently lower GM-CFS release in TME was observed in addition to preservation of the anti-tumor effect in the cell (82).
Conclusions
CAR-T cell therapy has been recognized as a breakthrough treatment for patients with hematological malignant diseases. However, their application on the solid tumors remains limited. In this setting, cytokines have been demonstrated to have important whole related to the complex procedure that involves CAR-T cell therapy, since the preclinical development, the harvest of T cells until the infusion and clinical management. Special highlights should be done to the manufacturing process where cytokines activities have crucial impact on the quality of CAR-T cell final product.
Author contributions
CS, AC, SC, NM, NB, FH and RG-C: wrote the manuscript. CS designed Figure 1. SC and TF designed Figure 2. FH and SC designed Figure 3. NM designed Figure 4. CS, PA, MO and RG-C reviewed the article. All authors contributed to the article and approved the submitted version.
Funding
This article is supported by the ASH Research Global Award (2018) and the following Brazilian research agencies: CNPq (grant #442686/2020-0); CRFS has postdoctoral fellowships from FAPESP (grant# 2020/10804-3); AC (CAPES grant # 88887.597999/2021-00) and FH (CNPq grant #88887.600222/2021-00); PA and NB have CNPq technical fellowship grant#380594/2022-6 and grant# 380029/2022-7 respectively.
Acknowledgments
We would like to thank Mrs. Sandra Navarro Bresciani for support in the design of the figures. Figure 4 was created using BioRender.com.
Conflict of interest
The authors declare that the research was conducted in the absence of any commercial or financial relationships that could be construed as a potential conflict of interest.
Publisher’s note
All claims expressed in this article are solely those of the authors and do not necessarily represent those of their affiliated organizations, or those of the publisher, the editors and the reviewers. Any product that may be evaluated in this article, or claim that may be made by its manufacturer, is not guaranteed or endorsed by the publisher.
References
1. Cao J, Wang G, Cheng H, Wei C, Qi K, Sang W, et al. Potent anti-leukemia activities of humanized CD19-targeted chimeric antigen receptor T (CAR-T) cells in patients with relapsed/refractory acute lymphoblastic leukemia. Am J Hematol (2018) 93(7):851–8. doi: 10.1002/ajh.25108
2. Fraietta JA, Lacey SF, Orlando EJ, Pruteanu-Malinici I, Gohil M, Lundh S, et al. Determinants of response and resistance to CD19 chimeric antigen receptor (CAR) T cell therapy of chronic lymphocytic leukemia. Nat Med (2018) 24(5):563–71. doi: 10.1038/s41591-018-0010-1
3. Chmielewski M, Abken H. TRUCKs: The fourth generation of CARs. Expert Opin Biol Ther (2015) 15:1145–54. doi: 10.1517/14712598.2015.1046430
4. Hinrichs CS, Spolski R, Paulos CM, Gattinoni L, Kerstann KW, Palmer DC, et al. IL-2 and IL-21 confer opposing differentiation programs to CD8+ T cells for adoptive immunotherapy. Blood (2008) 111(11):5326–33. doi: 10.1182/blood-2007-09-113050
5. Yan Z, Zhang H, Cao J, Zhang C, Liu H, Huang H, et al. Characteristics and risk factors of cytokine release syndrome in chimeric antigen receptor T cell treatment. Front Immunol (2021) 12. doi: 10.3389/fimmu.2021.611366
6. Whilding LM, Parente-Pereira AC, Zabinski T, Davies DM, Petrovic RMG, Kao YV, et al. Targeting of aberrant αvβ6 integrin expression in solid tumors using chimeric antigen receptor-engineered T cells. Mol Ther (2017) 25(1):259–73. doi: 10.1016/j.ymthe.2016.10.012
7. Zhang Y, Guan XY, Jiang P. Cytokine and chemokine signals of T-cell exclusion in tumors. Front Immunol (2020) 11. doi: 10.3389/fimmu.2020.594609
8. Kusmartsev S, Gabrilovich DI. Immature myeloid cells and cancer-associated immune suppression. Cancer Immunol Immunother (2002) 51(6):293–8. doi: 10.1007/s00262-002-0280-8
9. Sevko A, Umansky V. Myeloid-derived suppressor cells interact with tumors in terms of myelopoiesis, tumorigenesis and immunosuppression: Thick as thieves. J Cancer (2013) 4(1):3–11. doi: 10.7150/jca.5047
10. Burke JD, Young HA. IFN-Γ: A cytokine at the right time, is in the right place. Semin Immunol (2019) 43:1–8. doi: 10.1016/j.smim.2019.05.002
12. Meireson A, Devos M, Brochez L. IDO expression in cancer: Different compartment, different functionality? Front Immunol (2020) 11(September):1–17. doi: 10.3389/fimmu.2020.531491
13. Barashi N, Weiss ID, Wald O, Wald H, Beider K, Abraham M, et al. Inflammation-induced hepatocellular carcinoma is dependent on CCR5 in mice. Hepatology (2013) 58(3):1021–30. doi: 10.1002/hep.26403
14. Puerari F, Bosio B, Heyen G. Energy efficiency optimisation in different plant solutions for methanol production from biomass gasification. Chem Eng Trans (2014) 37:301–6. doi: 10.3303/CET1437051
15. Landskron G, de la Fuente M, Thuwajit P, Thuwajit C, Hermoso MA. Chronic inflammation and cytokines in the tumor microenvironment. J Immunol Res (2014) 2014:1–19. doi: 10.1155/2014/149185
16. Liu G, Rui W, Zhao X, Lin X. Enhancing CAR-T cell efficacy in solid tumors by targeting the tumor microenvironment. Cell Mol Immunol (2021) 18:1085–95. doi: 10.1038/s41423-021-00655-2
17. Zou W. Immunosuppressive networks in the tumour environment and their therapeutic relevance. Nat Rev Cancer (2005) 5(4):263–74. doi: 10.1038/nrc1586
18. Kehrl BYJH, Wakefield LM, Roberts AB, Jakowlew S, Alvarez-mon M, Derynck RIK, et al. Production of transforming growth factor beta by human T lymphocytes and its potential role in the regulation of T cell growth. J Exp Med (1986) 163:1037–50. doi: 10.1084/jem.163.5.1037
19. Gorelik L, Flavell RA. Abrogation of TGFβ signaling in T cells leads to spontaneous T cell differentiation and autoimmune disease. Immunity (2000) 12(2):171–81. doi: 10.1016/S1074-7613(00)80170-3
20. Zhu Y, Ju S, Chen E, Dai S, Li C, Morel P, et al. T-Bet and eomesodermin are required for T cell-mediated antitumor immune responses. J Immunol (2010) 185(6):3174–83. doi: 10.4049/jimmunol.1000749
21. Pearce EL, Mullen AC, Martins GA, Krawczyk CM, Hutchins AS, Zediak VP, et al. Control of effector CD8+ T cell function by the transcription factor eomesodermin. Science (2003) 302:1041–43. doi: 10.1126/science.1090148
22. Gorelik L, Constant S, Flavell RA. Mechanism of transforming growth factor β-induced inhibition of T helper type 1 differentiation. J Exp Med (2002) 195(11):1499–505. doi: 10.1084/jem.20012076
23. Lucas PJ, Kim SJ, Melby SJ, Gress RE. Disruption of T cell homeostasis in mice expressing a T cell-specific dominant negative transforming growth factor β II receptor. J Exp Med (2000) 191(7):1187–96. doi: 10.1084/jem.191.7.1187
24. Li Y, Xiao F, Zhang A, Zhang D, Nie W, Xu T, et al. Oncolytic adenovirus targeting TGF-β enhances anti-tumor responses of mesothelin-targeted chimeric antigen receptor T cell therapy against breast cancer. Cell Immunol (2020) 1:348. doi: 10.1016/j.cellimm.2020.104041
25. Kloss CC, Lee J, Zhang A, Chen F, Melenhorst JJ, Lacey SF, et al. Dominant-negative TGF-β receptor enhances PSMA-targeted human CAR T cell proliferation and augments prostate cancer eradication. Mol Ther (2018) 26(7):1855–66. doi: 10.1016/j.ymthe.2018.05.003
26. Stüber T, Monjezi R, Wallstabe L, Kühnemundt J, Nietzer SL, Dandekar G, et al. Inhibition of TGF- β- receptor signaling augments the antitumor function of ROR1-specific CAR T-cells against triple-negative breast cancer. J Immunother Cancer (2020) 8(1):1–7. doi: 10.1136/jitc-2020-000676
27. Tang N, Cheng C, Zhang X, Qiao M, Li N, Mu W, et al. TGF-β inhibition via CRISPR promotes the long-term efficacy of CAR T cells against solid tumors. JCI Insight (2020) 5:1–17. doi: 10.1172/jci.insight.133977
28. Bell M, Gottschalk S. Engineered cytokine signaling to improve CAR T cell effector function. Front Immunol (2021) 12(June):1–16. doi: 10.3389/fimmu.2021.684642
29. Mohammed S, Sukumaran S, Bajgain P, Watanabe N, Heslop HE, Rooney CM, et al. Improving chimeric antigen receptor-modified T cell function by reversing the immunosuppressive tumor microenvironment of pancreatic cancer. Mol Ther (2017) 25(1):249–58. doi: 10.1016/j.ymthe.2016.10.016
30. Jorgovanovic D, Song M, Wang L, Zhang Y. Roles of IFN-γin tumor progression and regression: A review. biomark Res (2020) 8(1):1–16. doi: 10.1186/s40364-020-00228-x
31. Zhang H, Lv X, Kong Q, Tan Y. IL-6/IFN-γ double knockdown CAR-T cells reduce the release of multiple cytokines from PBMCs in vitro. Hum Vaccines Immunother (2022) 18(1):1–14. doi: 10.1080/21645515.2021.2016005
32. Abiko K, Matsumura N, Hamanishi J, Horikawa N, Murakami R, Yamaguchi K, et al. IFN-γ from lymphocytes induces PD-L1 expression and promotes progression of ovarian cancer. Br J Cancer (2015) 112(9):1501–9. doi: 10.1038/bjc.2015.101
33. He YF, Wang XH, Zhang GM, Chen HT, Zhang H, Feng ZH. Sustained low-level expression of interferon-γ promotes tumor development: Potential insights in tumor prevention and tumor immunotherapy. Cancer Immunol Immunother (2005) 54(9):891–7. doi: 10.1007/s00262-004-0654-1
34. Takeda K, Nakayama M, Hayakawa Y, Kojima Y, Ikeda H, Imai N, et al. IFN-γ is required for cytotoxic T cell-dependent cancer genome immunoediting. Nat Commun (2017) 8:1–13. doi: 10.1038/ncomms14607
35. Lippitz BE. Cytokine patterns in patients with cancer: A systematic review. Lancet Oncol (2013) 14(6):e218–28. doi: 10.1016/S1470-2045(12)70582-X
36. Fisher DT, Appenheimer MM, Evans SS. The two faces of IL-6 in the tumor microenvironment. Semin Immunol (2014) 26(1):38–47. doi: 10.1016/j.smim.2014.01.008
37. Kiuchi N, Nakajima K, Ichiba M, Fukada T, Narimatsu M, Mizuno K, et al. STAT3 is required for the gp130-mediated full activation of the c-myc gene. J Exp Med (1999) 189(1):63–73. doi: 10.1084/jem.189.1.63
38. Hirano T, Ishihara K, Hibi M. Roles of STAT3 in mediating the cell growth, differentiation and survival signals relayed through the IL-6 family of cytokine receptors. Oncogene (2000) 19(21):2548–56. doi: 10.1038/sj.onc.1203551
39. Xie TX, Wei D, Liu M, Gao AC, Ali-Osman F, Sawaya R, et al. Stat3 activation regulates the expression of matrix metalloproteinase-2 and tumor invasion and metastasis. Oncogene (2004) 23(20):3550–60. doi: 10.1038/sj.onc.1207383
40. Wei LH, Kuo ML, Chen CA, Chou CH, Lai KB, Lee CN, et al. Interleukin-6 promotes cervical tumor growth by VEGF-dependent angiogenesis via a STAT3 pathway. Oncogene (2003) 22(10):1517–27. doi: 10.1038/sj.onc.1206226
41. Kim MY, Oskarsson T, Acharyya S, Nguyen DX, Zhang XHF, Norton L, et al. Tumor self-seeding by circulating cancer cells. Cell (2009) 139(7):1315–26. doi: 10.1016/j.cell.2009.11.025
42. Wen H, Huo G, Hou T, Qu Z, Sun J, Yu Z, et al. Preclinical efficacy and safety evaluation of interleukin-6-knockdown CAR-T cells targeting at CD19. Ann Transl Med (2021) 9(23):1713–3. doi: 10.21037/atm-21-3372
43. Singh N, Perazzelli J, Grupp SA, Barrett DM. Early memory phenotypes drive T cell proliferation in patients with pediatric malignancies. Sci Transl Med (2016) 8(320):1–10. doi: 10.1126/scitranslmed.aad5222
44. Frigault MJ, Maus MV, Dietrich J, Martinez-Lage M, Leick M, Choi BD, et al. Tisagenlecleucel CAR T-cell therapy in secondary CNS lymphoma. Blood (2019) 134(11):860–6. doi: 10.1182/blood.2019001694
45. Brudno JN, Lam N, Vanasse D, Shen Yw, Rose JJ, Rossi J, et al. Safety and feasibility of anti-CD19 CAR T cells with fully human binding domains in patients with b-cell lymphoma. Nat Med (2020) 26(2):270–80. doi: 10.1038/s41591-019-0737-3
46. Chmielewski M, Kopecky C, Hombach AA, Abken H. IL-12 release by engineered T cells expressing chimeric antigen receptors can effectively muster an antigen-independent macrophage response on tumor cells that have shut down tumor antigen expression. Cancer Res (2011) 71(17):5697–706. doi: 10.1158/0008-5472.CAN-11-0103
47. Fesnak A, Lin CY, Siegel DL, Maus MV. CAR-T cell therapies from the transfusion medicine perspective. Transfusion Med Rev (2016) 30:139–45. doi: 10.1016/j.tmrv.2016.03.001
48. Zhang L, Kerkar SP, Yu Z, Zheng Z, Yang S, Restifo NP, et al. Improving adoptive T cell therapy by targeting and controlling IL-12 expression to the tumor environment. Mol Ther (2011) 19(4):751–9. doi: 10.1038/mt.2010.313/nature06264
49. Zhao X, Ye F, Chen L, Lu W, Xie X. Human epithelial ovarian carcinoma cell-derived cytokines cooperatively induce activated CD4+CD25-CD45RA+ naïve T cells to express forkhead box protein 3 and exhibit suppressive ability in vitro. Cancer Sci (2009) 100(11):2143–51. doi: 10.1111/j.1349-7006.2009.01286.x
50. Yeku OO, Purdon TJ, Koneru M, Spriggs D, Brentjens RJ. Armored CAR T cells enhance antitumor efficacy and overcome the tumor microenvironment. Sci Rep (2017) 7(1):1–14. doi: 10.1038/s41598-017-10940-8
51. Vidal-Vanaclocha F, Mendoza L, Telleria N, Salado C, Valcárcel M, Gallot N, et al. Clinical and experimental approaches to the pathophysiology of interleukin-18 in cancer progression. Cancer Metastasis Rev (2006) 25(3):417–34. doi: 10.1007/s10555-006-9013-3
52. Dinarello CA. The paradox of pro-inflammatory cytokines in cancer. Cancer Metastasis Rev (2006) 25(3):307–13. doi: 10.1007/s10555-006-9000-8
53. Terme M, Ullrich E, Aymeric L, Meinhardt K, Desbois M, Delahaye N, et al. IL-18 induces PD-1-dependent immunosuppression in cancer. Cancer Res (2011) 71(16):5393–9. doi: 10.1158/0008-5472.CAN-11-0993
54. Colak S, ten Dijke P. Targeting TGF-$β$ signaling in cancer. Trends Cancer (2017) 3(1):56–71. doi: 10.1016/j.trecan.2016.11.008
55. Mei Z, Zhang K, Lam AKY, Huang J, Qiu F, Qiao B, et al. MUC1 as a target for CAR-T therapy in head and neck squamous cell carinoma. Cancer Med (2020) 9(2):640–52. doi: 10.1002/cam4.2733
56. Chmielewski M, Abken H. CAR T cells releasing IL-18 convert to T-bethigh FoxO1low effectors that exhibit augmented activity against advanced solid tumors. Cell Rep (2017) 21(11):3205–19. doi: 10.1016/j.celrep.2017.11.063
57. Ma X, Shou P, Smith C, Chen Y, Du H, Sun C, et al. Interleukin-23 engineering improves CAR T cell function in solid tumors. Nat Biotechnol (2020) 38(4):448–59. doi: 10.1038/s41587-019-0398-2
58. Chen Y, Sun C, Landoni E, Metelitsa L, Dotti G, Savoldo B. Eradication of neuroblastoma by T cells redirected with an optimized GD2-specific chimeric antigen receptor and interleukin-15. Clin Cancer Res (2019) 25:2915–24. doi: 10.1158/1078-0432.CCR-18-1811
59. Lanitis E, Rota G, Kosti P, Ronet C, Spill A, Seijo B, et al. Optimized gene engineering of murine CAR-T cells reveals the beneficial effects of IL-15 coexpression. J Exp Med (2021) 218(2):1–19. doi: 10.1084/jem.20192203
60. Batra SA, Rathi P, Guo L, Courtney AN, Fleurence J, Balzeau J, et al. Glypican-3-specific CAR T cells coexpressing IL15 and IL21 have superior expansion and antitumor activity against hepatocellular carcinoma. Cancer Immunol Res (2020) 8(3):309–20. doi: 10.1158/2326-6066.CIR-19-0293
61. Duan D, Wang K, Wei C, Feng D, Liu Y, He Q, et al. The BCMA-targeted fourth-generation CAR-T cells secreting IL-7 and CCL19 for therapy of Refractory/Recurrent multiple myeloma. Front Immunol (2021) 12(March):1–11. doi: 10.3389/fimmu.2021.609421
62. Wang X, Rivière I. Clinical manufacturing of CAR T cells: Foundation of a promising therapy. Mol Ther - Oncol (2016) 3(February):16015. doi: 10.1038/mto.2016.15
63. Gee AP. GMP CAR-T cell production. Best Pract Res Clin Haematol (2018) 31(2):126–34. doi: 10.1016/j.beha.2018.01.002
64. Stock S, Schmitt M, Sellner L. Optimizing manufacturing protocols of chimeric antigen receptor t cells for improved anticancer immunotherapy. Int J Mol Sci (2019) 20(24):1–21. doi: 10.3390/ijms20246223
65. Liao W, Lin JX, Leonard WJ. Interleukin-2 at the crossroads of effector responses, tolerance, and immunotherapy. Immunity (2013) 38:13–25. doi: 10.1016/j.immuni.2013.01.004
66. Pipkin ME, Sacks JA, Cruz-Guilloty F, Lichtenheld MG, Bevan MJ, Rao A. Interleukin-2 and inflammation induce distinct transcriptional programs that promote the differentiation of effector cytolytic T cells. Immunity (2010) 32(1):79–90. doi: 10.1016/j.immuni.2009.11.012
67. Huang R, Li X, He Y, Zhu W, Gao L, Liu Y, et al. Recent advances in CAR-T cell engineering. J Hematol Oncol (2020) 13(1):1–19. doi: 10.1186/s13045-020-00910-5
68. Malek TR, Castro I. Interleukin-2 receptor signaling: At the interface between tolerance and immunity. Immunity (2010) 33(2):153–65. doi: 10.1016/j.immuni.2010.08.004
69. Zhang H, Zhao P, Huang H. Engineering better chimeric antigen receptor T cells. Exp Hematol Oncol (2020) 9(1):1–13. doi: 10.1186/s40164-020-00190-2
70. Xu XJ, Song DG, Poussin M, Ye Q, Sharma P, Rodríguez-García A, et al. Multiparameter comparative analysis reveals differential impacts of various cytokines on CART cell phenotype and function ex vivo and in vivo. Oncotarget (2016) 7(50):82354–68. doi: 10.18632/oncotarget.10510
71. Brudno JN, Kochenderfer JN. Toxicities of chimeric antigen receptor T cells: Recognition and management. Blood (2016) 127:3321–30. doi: 10.1182/blood-2016-04-703751
72. Frey N, Porter D. Cytokine release syndrome with chimeric antigen receptor T cell therapy. Biol Blood Marrow Transplant (2019) 25:e123–7. doi: 10.1016/j.bbmt.2018.12.756
73. Hao Z, Li R, Meng L, Han Z, Hong Z. Macrophage, the potential key mediator in CAR-T related CRS. Exp Hematol Oncol (2020) 9:1–12. doi: 10.1186/s40164-020-00171-5
74. Garfall AL, Maus MV, Hwang W-T, Lacey SF, Mahnke YD, Melenhorst JJ, et al. Chimeric antigen receptor T cells against CD19 for multiple myeloma. N Engl J Med (2015) 373(11):1040–7. doi: 10.1056/NEJMoa1504542
75. Maude SL, Laetsch TW, Buechner J, Rives S, Boyer M, Bittencourt H, et al. Tisagenlecleucel in children and young adults with b-cell lymphoblastic leukemia. N Engl J Med (2018) 378(5):439–48. doi: 10.1056/NEJMoa1709866
76. Neelapu SS, Locke FL, Bartlett NL, Lekakis LJ, Miklos DB, Jacobson CA, et al. Axicabtagene ciloleucel CAR T-cell therapy in refractory Large b-cell lymphoma. N Engl J Med (2017) 377(26):2531–44. doi: 10.1056/NEJMoa1707447
77. Locke FL, Miklos DB, Jacobson CA, Perales M-A, Kersten M-J, Oluwole OO, et al. Axicabtagene ciloleucel as second-line therapy for Large b-cell lymphoma. N Engl J Med (2022) 386(7):640–54. doi: 10.1056/NEJMoa2116133
78. Maude SL, Frey N, Shaw PA, Aplenc R, Barrett DM, Bunin NJ, et al. Chimeric antigen receptor T cells for sustained remissions in leukemia. N Engl J Med (2014) 371(16):1507–17. doi: 10.1056/NEJMoa1407222
79. June CH, Sadelain M. Chimeric antigen receptor therapy. N Engl J Med (2018) 379(1):64–73. doi: 10.1056/NEJMra1706169
80. Park JH, Rivière I, Gonen M, Wang X, Sénéchal B, Curran KJ, et al. Long-term follow-up of CD19 CAR therapy in acute lymphoblastic leukemia. N Engl J Med (2018) 378(5):449–59. doi: 10.1056/NEJMoa1709919
81. Tan AHJ, Vinanica N, Campana D. Chimeric antigen receptor-T cells with cytokine neutralizing capacity. Blood Adv (2020) 4(7):1419–31. doi: 10.1182/bloodadvances.2019001287
82. Lindo L, Wilkinson LH, Hay KA. Befriending the hostile tumor microenvironment in CAR T-cell therapy. Front Immunol (2021) 11. doi: 10.3389/fimmu.2020.618387
Keywords: CAR-T cells, chimeric antigen receptor, cytokines, immunomodulation, cell therapy, cancer microenvironment, immunotherapy
Citation: Silveira CRF, Corveloni AC, Caruso SR, Macêdo NA, Brussolo NM, Haddad F, Fernandes TR, de Andrade PV, Orellana MD and Guerino-Cunha RL (2022) Cytokines as an important player in the context of CAR-T cell therapy for cancer: Their role in tumor immunomodulation, manufacture, and clinical implications. Front. Immunol. 13:947648. doi: 10.3389/fimmu.2022.947648
Received: 19 May 2022; Accepted: 23 August 2022;
Published: 12 September 2022.
Edited by:
Cleni Mara Marzocchi-Machado, University of São Paulo, BrazilReviewed by:
Philippe Lewalle, Jules Bordet Institute, BelgiumSaverio Alberti, University of Messina, Italy
Copyright © 2022 Silveira, Corveloni, Caruso, Macêdo, Brussolo, Haddad, Fernandes, de Andrade, Orellana and Guerino-Cunha. This is an open-access article distributed under the terms of the Creative Commons Attribution License (CC BY). The use, distribution or reproduction in other forums is permitted, provided the original author(s) and the copyright owner(s) are credited and that the original publication in this journal is cited, in accordance with accepted academic practice. No use, distribution or reproduction is permitted which does not comply with these terms.
*Correspondence: Renato Luiz Guerino-Cunha, rlgc@usp.br
†These authors have contributed equally to this work