Mitochondrial dysfunction resulting from the down-regulation of bone morphogenetic protein 5 may cause microtia
Introduction
Microtia is one of the common congenital craniofacial and extracranial anomalies in children, and has an incidence rate of 0.83/10,000–17.40/10,000 (1-7). The clinical manifestations of microtia range in severity from mild structural abnormalities to the complete absence of ears (anotia). Clinical observations and previous reports have shown that microtia is mostly sporadic but cases of familial aggregation have also been reported. According to reports, the incidence of microtia multiple case family (MMCF) is between 2.9% and 33.3% (2,3,8,9). Microtia is associated with aural atresia and conduction deafness, with approximately 90% of patients with microtia suffering conductive hearing loss on the affected side (10-12). Hearing loss may have a negative impact on an individual’s speech development and communication ability (1-3), and may even prove life-threatening in daily life. Although many treatments exist for hearing loss, they produce different results (13). The stigma associated with microtia and the burden of undergoing multiple surgeries negatively impact the psychological state of patients, which especially affects their social life (4-7,14). Moreover, the considerable medical costs associated with treating this disease and its associated health issues place a burden not only on the patient’s family but on the public health system. Therefore, early diagnosis and prevention of microtia are of great significance.
Existing data indicate that the occurrence of microtia is affected by many factors including environmental and genetic causes (8). Although the etiology of microtia has not been confirmed, genetic variants have been identified as an important risk factor for the disease (9). As a member of the bone morphogenetic protein family, bone morphogenetic protein 5 (BMP5) is associated with bone and cartilage morphogenesis, soft tissue connection, and limb development in humans (10). The homozygous recessive mutation of BMP5 in mice can cause defective cartilage to affect the entire bone structure, thereby shortening the outer ear and causing it to wrinkle slightly (4). Previous studies have found that activation of BMP5 and Wnt5a causes SMADs to enter the nucleus and initiate cell proliferation and differentiation procedures (15). The ligands of the BMP family bind to various transforming growth factor (TGF)-β receptors, resulting in the recruitment and activation of SMAD family transcription factors that regulate gene expression. Exogenous TGF-β3 treatment attenuated mitochondria-mediated apoptosis resulted from serum deprivation under hypoxic conditions in adipose-derived stem cells (ADSCs), via the upregulation of poly ADP-ribose polymerase (PARP) (16). Thus, we considered whether mitochondria play an important role in the process of BMP5-regulated cell proliferation and differentiation.
The mitochondrial system is perhaps the most sophisticated and dynamic responsive eukaryotic sensing system. It participates in the regulation of Ca2+ homeostasis, cytochrome c release, reactive oxygen species (ROS) generation, and cellular signal transduction (11-13,17-19). Mitochondrial dysfunction is observed in many human diseases, including inherited genetic disorders, degenerative diseases, inflammation, and cancer. Mitochondrial dynamics are altered by physiological aging, which affects stem cell homeostasis through coordinated changes in BMP orthologue stemness signaling, niche contact, and cellular metabolism (20). Moreover, the existing literature indicates a close relationship between cell differentiation and mitochondrial function. Establishment of the mitochondrial network is the prerequisite for stem cells to differentiate into a functional phenotype (21,22). A previous study showed that interference with the expression of BMP and activin membrane-binding inhibitor (BAMBI) can promote the production of ROS in the cytoplasm and mitochondria along with the DNA binding activity of C/EBPβ, to ultimately promote adipocyte differentiation and adipogenesis (23). Collectively, these data suggest that mitochondria may play a role in BMP5-regulated cell proliferation and differentiation. Enhanced understanding of the interplay between BMP5, mitochondrial function, and cellular function integrity could provide novel directions for the etiological study and prevention of microtia. Although some studies have mentioned that BMP5 may be involved in the occurrence of microtia (24,25), the research on its specific mechanism is still not in-depth. Moreover, this study is the first to prove that lipid metabolism disorders may cause microtia. In this study, we investigated the relationship of BMP5 and mitochondrial function by establishing 293T and ADSC models of low BMP5 expression through transfection with small interfering RNA (siRNA) in an effort to demonstrate the relationship of BMP5 and mitochondrial function in microtia development. We present the following article in accordance with the MDAR reporting checklist (available at http://dx.doi.org/10.21037/atm-21-831).
Methods
Clinical specimens
Three patients with microtia and 3 otitis patients with normal pinna were enrolled into the study. Surgically resected auricular cartilage tissue specimens were obtained from Sun Yat-sen Memorial Hospital of Sun Yat-sen University. The study was conducted in accordance with the Declaration of Helsinki (as revised in 2013). Written informed consent was obtained from all the subjects and their parents involved in this study for participation in and publication of this study, and all procedures were approved by the Ethical Committee of Sun Yat-sen Memorial Hospital of Sun Yat-sen University (No.: 2019-KY-024) and were following the Declaration of Beijing.
Immunohistochemical staining of auricular cartilage
BMP5 expression was detected in the auricular cartilage of healthy people and microtia patients using an immunohistochemical staining method with specific antibodies.
Cell culture
ADSCs (OriCell, USA) were cultured in human adipose-derived mesenchymal stem cell medium (OriCell, USA) with 10% fetal bovine serum (FBS; OriCell, USA), 1% penicillin, and 1% L-glutamine at 37 °C with 5% CO2. Culture of 293T cells was done in Dulbecco’s Modified Eagle Medium (DMEM; Gibco, USA) containing 10% FBS (Gibco, USA) at 37 °C in a 5% CO2 incubator.
Cell transfection
ADSCs and 293T cells were cultured to ~60–70% confluence in culture medium without penicillin or streptomycin. BMP5 siRNA-1 (sense: GCAGCUGAAUUCCGGAUAUTT; antisense: AUAUCCGGAAUUCAGCUGCTT), BMP5 siRNA-2 (sense: CCUCGUCGCAUACAGUUAUTT; antisense: AUAACUGUAUGCGACGAGGTT), BMP5 siRNA-3(sense: GCUGGGUUCUAGUGGGUUATT; antisense: UAACCCACUAGAACCCAGCTT) and the NC siRNA (Shanghai, China). ADSCs and 293T cells were transfected with siRNA using a Lipofectamine 3000 Transfection Reagent Kit (Beyotime, China) according to the manufacturer’s instructions.
Colony formation assay
Cells were seeded in 6-well plates at 1,000/mL per well. The culture media were not changed during the experiment. Colonies were fixed with 4% paraformaldehyde and stained with 0.5% crystal violet after a week of culture. Colonies with 20–40 cells were counted.
Transwell assay
Cells (1×105 cells/well) were cultured in a Transwell chamber (BD Biosciences, USA) containing FBS-free medium in the upper chamber and 10% FBS in the lower chamber at 37 °C with 5% CO2. After 24 hours of culture, 4% paraformaldehyde was used to fix the cells that had migrated to the lower chamber, and 0.5% crystal violet was used to stain the cells for 15 minutes. The cells were photographed and counted under a microscope.
Cell viability assay
Cell Counting Kit (CCK)-8 kits (DOJINDO, Japan) were used to detect cell viability. Cells were seeded in 96-well plates at 37 °C for 24 hours. After treatment for 2 hours, the absorbance was measured at 450 nm with a microplate reader (Labsystems Dragon, Finland).
Measurement of mitochondrial transmembrane potential
The mitochondrial membrane potential was estimated by monitoring fluorescence using JC-1 (Yeasen Biotechnology, Shanghai). After treatment with specific siRMA, cells were incubated with 1 µg/mL JC-1 for 20 minutes at 37 °C in the dark. Finally, an Olympus BX63 microscope (Olympus, Japan) was used to take the fluorescence images.
Detection of ROS
Cells were cultured in medium with 0.5 mM H2O2 for 24 hours. Following that, they were covered with 0.5 mM dihydroethidium and incubated in a light-protected humidified chamber at 37 °C for 3 minutes. Images were obtained with a fluorescence microscope (Olympus BX63, Olympus, Japan).
Adenosine triphosphate (ATP) measurement
ATP was measured using ATP assay kits (Beyotime, Shanghai, China). After being diluted with dilution buffer, ATP detection reagent was added to a 96-well plate. After homogenization followed by centrifugation at 12,000 g at 4 °C for 5 minutes, the samples were added into the wells and mixed with the detection solution. Then, the levels of ATP were measured with a SpectraMax M5 microplate reader (Molecular Devices, San Jose, CA, USA). The ATP content was normalized to the ATP protein content on the basis of the standard curve.
RNA extraction and quantitative real-time PCR
After treatment for 48 hours with specific siRNA, TRIzol TM reagent (ThermoFisher Scientific, China) was used to extract total RNA from cells, which was then reverse-transcribed with Takara RNA PCR kits (Takara, Tokyo, Japan). Quantitative real-time PCR (qPCR) was performed on an ABI Prism 7500 Sequence Detection System (Applied Biosystems, USA) using SYBR Green PCR supermixes (Biorad, Hercules, California, USA). The relative expression of the gene of interest was calculated with the 2-ΔΔt method.
Western blotting
Cells were lysed in lysis buffer (ThermoPlus, USA) with a protease inhibitor cocktail (Kangcheng, Shanghai, China). Protein samples were separated through sodium dodecyl sulfate-polyacrylamide gel electrophoresis (SDS-PAGE) and transferred onto polyvinylidene difluoride (PVDF) membranes (Millipore, Burlington, MA, USA). After being blocked with 5% BSA, the membranes were incubated with primary antibodies to BMP5 (Abclonal, China, Cat#: A8401), VDAC1 (Abclonal, China, Cat#: A19707), CYCS (Abcam, USA, Cat#: ab133504), CPT2 (Affinity, China, Cat#: DF7089), DGAT2 (Affinity, China, Cat#: DF9442), and GAPDH (Abclonal, China, Cat#: AC001) for 12 hours at 4 °C. After further incubation with secondary antibodies (1:3,000) at room temperature for 1 hour, the immunoreactive bands were detected by enhanced chemiluminescence (Millipore, Burlington, MA, USA) and analyzed in ImageJ (NIH, USA). GAPDH served as the internal control.
Statistical analysis
Statistical analyses were performed using SPSS 18.0 (IBM Co., USA) and GraphPad Prism 7.0 (GraphPad Software, USA). Data were analyzed by unpaired Student’s t-test. All data were expressed as the mean ± standard error of the mean (SEM) and were obtained from three independent experiments. For all statistical analyses, a P value of <0.05 was considered statistically significant.
Results
BMP5 expression was down-regulated in auricular cartilage tissues from microtia patients
To evaluate the relationship between BMP5 expression and microtia, we measured the levels of BMP5 expression in auricular cartilage tissue specimens obtained from 3 microtia patients with varying degrees of minor pinna deformity and 3 otitis patients with normal pinna. Immunohistochemical (IHC) analysis demonstrated that microtia auricular cartilage tissues were negative or weakly stained for BMP5. Representative examples of BMP5 immunostaining are shown in Figure 1A. We further quantified BMP5 expression by calculating the number of stained cells, stained area, and dyeing degree, and found that compared with the normal tissues, the microtia auricular cartilage tissues showed decreases of 31.5% (Figure 1B), 63.9% (Figure 1C), and 79.7% (Figure 1D), respectively. These results indicated that BMP5, which was down-regulated in microtia auricular cartilage tissue, might be a potential genetic risk factor for microtia.
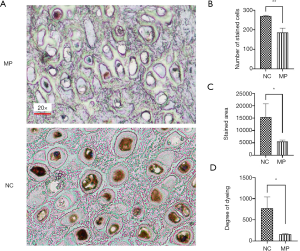
Down-regulation of BMP5 inhibited cellular function in vitro
To explore the biological effects of reduced BMP5 expression in the 293T cells and ADSCs, control cells were treated with BMP5-specific siRNA in 3 different sequences. The silencing potency of the siRNAs specific to BMP5 was verified by real-time PCR and western blotting. The results demonstrated that at 48 hours after transfection with the second and third BMP5 siRNA sequences (siBMP5-2 and siBMP5-3), BMP5 mRNA expression in 293T cells and ADSCs was down-regulated by more than 40% (Figure 2A,B). After 72 hours of transfection, the efficiency of knock-down of BMP5 protein in the siBMP5-2 and siBMP5-3 ADSCs was about 48%, while the knock-down efficiency in siBMP5-2 and siBMP5-3 293T cells was 44% and 55%, respectively (Figure 2C,D). These results showed that the second and third BMP5 siRNA sequences performed well in down-regulating BMP5 expression and that the BMP5 expression levels of BMP5 siRNA-transfected control cells were comparable to those of microtia auricular cartilage tissue.
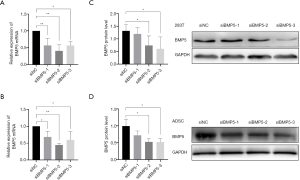
Subsequently, the activity of cells treated with siRNAs specific to BMP5 was examined by performing colony formation, Transwell migration, and CCK8 assays (Figure 3). As shown in Figure 3A and B, the treated 293T cells exhibited a significant decrease in the number of colonies formed (a 51% decrement in siBMP5-2 group and 55% decrement in siBMP5-3 group). After 48 hours of transfection, compared to the non-sense siRNA-treated cells, far fewer BMP5 siRNA-treated 293T cells were observed to have migrated through the Transwell membranes (an approximate 60% decrement in both siBMP5 groups, Figure 3C and F). Compared to non-sense siRNA-treated cells, 293T cells with BMP5 silencing showed lower cytoactivity according to the results of CCK-8 assay, with a decrease of approximately 30% in relative absorbance (Figure 3D). It was further confirmed that the cell viability of ADSCs had decreased following the down-regulation of BMP5 (Figure 3E). These findings verified that the down-regulation of BMP5 inhibited cellular function in vitro, as shown by reductions in cell proliferation, mobility, and cytoactivity.
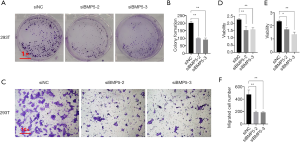
BMP5 down-regulation probably affected cellular lipid metabolism
To further investigate cellular vulnerabilities caused by BMP5 deficiency, genome-wide expression profiling was conducted. To identify the pathways responsible for the sensitivity of 293T-siRNA cells to 293T-NC cells, a pathway analysis of genes that showed a more than 2-fold increase or decrease in expression in 293T-siRNA cells but not in 293T-NC cells after treatment with BMP5-siRNA was performed (Figure 4). Among these genes, pathways related to fatty acid degradation and glycerolipid metabolism were significantly inhibited (P<0.001). Notably, several key genes of lipid metabolism associated with mitochondria, including CPT1A, CPT2, and DGAT2, were markedly down-regulated in 293T-siBMP5 cells, but not in 293T-siNC cells. These results indicated that mitochondria might play an important role in the process of cellular function regulation following BMP5 down-regulation.
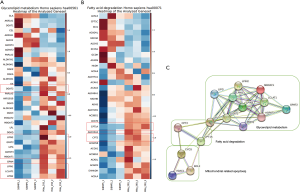
BMP5 silencing altered the function of mitochondria
The above findings suggested that the functional integrity of mitochondria was related to the regulation of cellular function. Therefore, we next investigated the mitochondrial function profiles between control and BMP5 siRNA-treated cells (Figure 5). The mitochondrial membrane potential, which was examined using JC-1 staining, was diminished (Figure 5A,B) in BMP5 siRNA-treated cells, which showed bright green fluorescence. Further characterization of mitochondrial structural proteins suggested that the levels of cytochrome C (CYCS) and voltage-dependent anion-selective channel protein 1 (VDAC1) were increased in BMP5 siRNA-treated 293T cells, which was confirmed by western blotting (CYCS, a 36% and 34% increment in siBMP5-2 and siBMP5-3 group respectively; and VDAC1, a 49% and 37% increment in siBMP5-2 and siBMP5-3 group respectively; Figure 5E,F,G). The destruction of the mitochondrial membrane resulted in mitochondrial dysfunction. Flow cytometry indicated that the ROS neutralization capacity, which was examined using dihydroethidium, was down-regulated by 10% in BMP5-transduced 293T cells (Figure 5D). Fluorescence microscopy also confirmed ROS elevation in ADSCs treated with BMP5 siRNA (Figure 5C,H). Also, compared to the control cells, 293T cells treated with BMP5 siRNA contained less ATP at the basal level (a 30% and 20% decrement in siBMP5-2 and siBMP5-3 group respectively, Figure 5I). Collectively, the presented results suggested that the down-regulation of BMP5 induces the destruction of mitochondrial functional integrity in 293T cells and ADSCs.
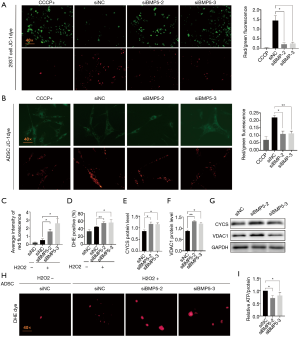
Down-regulation of BMP5 attenuated mitochondrial lipid oxidation and triglyceride synthesis
According to the genome-wide expression analysis of cells with BMP5 down-regulation, some key enzymes in fat oxidation were affected by the expression levels of BMP5. We monitored the expression of enzymes responsible for the transportation of fatty acids to mitochondria and oxidation by performing real-time PCR and Western blotting. The expression of carnitine O-palmitoyltransferase 2 (CPT2) was significantly reduced by BMP5 down-regulation at both the mRNA and the protein level (Figure 6A,B,C). CPT2 catalyze one of the key step in the transportation of long-chain acetyl coenzyme A (acyl-CoA) into mitochondria for subsequent oxidation. Furthermore, the expression of diacylglycerol acyltransferase 2 (DGAT2), a key protein that catalyzes the synthesis of triglycerides (TGs), was also significantly downregulated (Figure 6D,E,F,G), which indicated the dysfunction of TG synthesis.
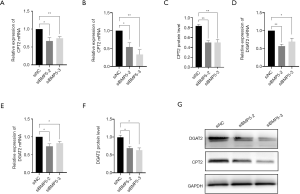
Discussion
In recent years, members of the BMP signaling pathway, including BMP5, have been reported to be associated with stem cell maintenance and self-renewal capacity in a number of organisms (26). Homozygous recessive mutations of BMP5 in mice can result in shortened, due to damage to the cartilage framework, which affects the external ear, causing a slightly wrinkled appearance (27). However, the question of how BMP5 regulates the cellular functions which lead to cartilage defects in patients with microtia remained unanswered.
In this study, we established an in vitro model of BMP5 down-regulation to clarify four key issues: (I) the impact of BMP5 expression on cellular function in vitro; (II) changes in genome-wide expression following BMP5 down-regulation; (III) the phenotypic variation of mitochondria in cells with BMP5 down-regulation; and, most importantly, (IV) alterations in the specific pathways related to mitochondrial function.
We demonstrated that BMP5 was decreased in the auricular cartilage tissues of microtia patients and that BMP5 expression was reduced in 293T cells and ADSCs following transfection with BMP5-specific siRNA, which led to the inhibition of cell proliferation, mobility, and cytoactivity. These novel findings indicate the mechanisms underlying an important role of BMP5 in the biogenetic process of microtia.
Existing data showed that intact mitochondrial function is important for the maintenance of progenitor cell function (28). Recent studies on mitochondrial dynamics have indicated that cellular aging is influenced by the fission and fusion of mitochondria through the regulation of the cell cycle, mitochondrial metabolism, redox signaling, and apoptosis (29,30). However, BMP5 down-regulation has not previously been reported to be associated with mitochondrial dysregulation. Our finding that BMP5 down-regulation in 293T and ADSCs has an association with mitochondrial dysfunction is consistent with the outcomes of cellular function inhibition in these cells. In the BMP5 down-regulation model of 293T cells, changes of mitochondrial membrane potential and mitochondrial structural proteins were observed, suggesting that BMP5 attenuates mitochondrial function. A loss of mitochondrial membrane potential and the formation of mitochondrial permeability transition pores are signs of mitochondrial dysfunction (31). Voltage-dependent anion channels (VDACs) are the most abundant proteins in the mitochondrial outer membrane and regulate Ca2+ influx, metabolism, inflammasome activation, and cell death (31-35). VDAC1 (the most abundant of the 3 VDAC isoforms) was found to be increased in cells with BMP5 down-regulation, and it might be a contributor to the permeability of mitochondrial outer membrane and the increase in the release rate of CYCS. Previous studies have demonstrated that mitochondrial ROS acts as a second messenger to activate various downstream signaling cascades (such as PKC and PI3K), and then continues to inhibit the formation of mitochondrial permeability transition pores (36,37). However, the excessive release of ROS can have harmful effects on mitochondrial components (such as mitochondrial DNA, mitochondrial membrane and respiratory chain protein, and nuclear DNA), resulting in impaired mitochondrial function (38). Cells with less BMP5 expression exhibited higher levels of oxidative stress induced by ROS overproduction, which was a critical factor in the disruption of mitochondrial function and the resulting in cell death signaling.
Following BMP5 transduction, we found that mitochondrial lipid oxidation and TG synthesis were inhibited. Previous research has demonstrated BMP signaling to be a crucial molecular link in the coordination of mitochondrial dynamics and lipid catabolism (39). Several key molecules regulate lipid metabolism associated with mitochondria, the dysregulation of which alters mitochondrial function (35,40). As illustrated by western blot, BMP5 down-regulation dramatically impaired the expression levels of DGAT2 and CPT2, which were the master regulators of TG synthesis and long-chain acyl-CoA transportation of the subsequent oxidation in mitochondria. Together, these results confirmed that the contribution of reduced BMP5 expression to mitochondrial dysfunction in vitro is closely related to lipid metabolism, especially in aspect of long-chain acyl-CoA oxidation and TG synthesis. However, the change of triglyceride content and the acyl-CoA accumulation in BMP5 down-regulated cells remained to be further analyzed.
The results of this study suggested that the down-regulation of BMP5 led to inefficiency in mitochondrial function, which subsequently impaired the integrity of cellular functions, especially in the processes of long-chain acyl-CoA oxidation and TG synthesis. However, the impact of BMP5 expression levels during the biogenic process of microtia in vivo demands further investigation.
Conclusions
Taken together, our findings have revealed for the first time that down-regulation of BMP5 affects glycerolipid metabolism and fatty acid degradation, resulting in mitochondrial dysfunction, reduced ATP production, changes in cellular function (Figure 7), and, eventually, microtia. This work provides supporting evidence of an important role of BMP5 down-regulation in mitochondrial metabolism and sheds new light on the mechanisms underlying the pathogenesis of microtia.
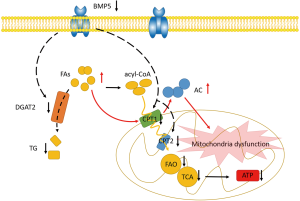
Acknowledgments
Funding: This work was supported by grants from the National Natural Science Foundation of Guangdong (No. 2019A1515010902), the Science and Technology Program of Guangzhou (No. 201903010075), the National Key R&D Program of China (2020YFB020003), GD Frontier & Key Tech Innovation Program (2018B030337001 and 2019B020228001), the National Natural Science Foundation of China (81801132, and 81971190).
Footnote
Reporting Checklist: The authors have completed the MDAR reporting checklist. Available at http://dx.doi.org/10.21037/atm-21-831
Data Sharing Statement: Available at http://dx.doi.org/10.21037/atm-21-831
Conflicts of Interest: All authors have completed the ICMJE uniform disclosure form (available at http://dx.doi.org/10.21037/atm-21-831). The authors have no conflicts of interest to declare.
Ethical Statement: The authors are accountable for all aspects of the work in ensuring that questions related to the accuracy or integrity of any part of the work are appropriately investigated and resolved. The study was conducted in accordance with the Declaration of Helsinki (as revised in 2013). Written informed consent was obtained from all the subjects and their parents involved in this study for participation in and publication of this study, and all procedures were approved by the Ethical Committee of Sun Yat-sen Memorial Hospital of Sun Yat-sen University (No.: 2019-KY-024) and were following the Declaration of Beijing.
Open Access Statement: This is an Open Access article distributed in accordance with the Creative Commons Attribution-NonCommercial-NoDerivs 4.0 International License (CC BY-NC-ND 4.0), which permits the non-commercial replication and distribution of the article with the strict proviso that no changes or edits are made and the original work is properly cited (including links to both the formal publication through the relevant DOI and the license). See: https://creativecommons.org/licenses/by-nc-nd/4.0/.
References
- Du JM, Zhuang HX, Chai JK, et al. Psychological status of congenital microtia patients and relative influential factors: analysis of 410 cases. Zhonghua Yi Xue Za Zhi 2007;87:383-7. [PubMed]
- Castilla EE, Orioli IM. Prevalence rates of microtia in South America. Int J Epidemiol 1986;15:364-8. [Crossref] [PubMed]
- Mastroiacovo P, Corchia C, Botto LD, et al. Epidemiology and genetics of microtia-anotia: a registry based study on over one million births. J Med Genet 1995;32:453-7. [Crossref] [PubMed]
- Shaw GM, Carmichael SL, Kaidarova Z, et al. Epidemiologic characteristics of anotia and microtia in California, 1989-1997. Birth Defects Res A Clin Mol Teratol 2004;70:472-5. [Crossref] [PubMed]
- Harris J, Kallen B, Robert E. The epidemiology of anotia and microtia. J Med Genet 1996;33:809-13. [Crossref] [PubMed]
- Canfield MA, Langlois PH, Nguyen LM, et al. Epidemiologic features and clinical subgroups of anotia/microtia in Texas. Birth Defects Res A Clin Mol Teratol 2009;85:905-13. [Crossref] [PubMed]
- Forrester MB, Merz RD. Descriptive epidemiology of anotia and microtia, Hawaii, 1986-2002. Congenit Anom (Kyoto) 2005;45:119-24. [Crossref] [PubMed]
- Llano-Rivas I, Gonzalez-del AA, Del CV, et al. Microtia: a clinical and genetic study at the National Institute of Pe-diatrics in Mexico City. Arch Med Res 1999;30:120-4. [Crossref] [PubMed]
- Zhang HS, Chen SJ, Zeng HC, et al. Characteristics of 43 multiple auricular deformity case families and auricle morphology in 463 microtia patients in South China. Ann Transl Med 2020;8:496. [Crossref] [PubMed]
- Suutarla S, Rautio J, Ritvanen A, et al. Microtia in Finland: comparison of characteristics in different populations. Int J Pediatr Otorhinolaryngol 2007;71:1211-7. [Crossref] [PubMed]
- Calzolari F, Garani G, Sensi A, et al. Clinical and radiological evaluation in children with microtia. Br J Audiol 1999;33:303-12. [Crossref] [PubMed]
- Ishimoto S, Ito K, Karino S, et al. Hearing levels in patients with microtia: correlation with temporal bone malfor-mation. Laryngoscope 2007;117:461-5. [Crossref] [PubMed]
- Lin J, Chen S, Zhang H, et al. Application of Implantable Hearing Aids and Bone Conduction Implant System in patients with bilateral congenital deformation of the external and middle ear. Int J Pediatr Otorhinolaryngol 2019;119:89-95. [Crossref] [PubMed]
- Kaye CI, Rollnick BR, Hauck WW, et al. Microtia and associated anomalies: statistical analysis. Am J Med Genet 1989;34:574-8. [Crossref] [PubMed]
- Zuzarte-Luís V, Montero JA, Rodriguez-Leon J, et al. A new role for BMP5 during limb development acting through the synergic activation of Smad and MAPK pathways. Dev Biol 2004;272:39-52. [Crossref] [PubMed]
- Wu F, Ye H, Lin J, et al. TGF-beta3 reduces apoptosis in ischemia-induced adipose-derived stem cells by enhancing DNA repair. Exp Ther Med 2018;15:4400-8. [PubMed]
- Blajszczak C, Bonini MG. Mitochondria targeting by environmental stressors: Implications for redox cellular sig-naling. Toxicology 2017;391:84-9. [Crossref] [PubMed]
- Zhang H, Chang Z, Mehmood K, et al. Nano Copper Induces Apoptosis in PK-15 Cells via a Mitochondria-Mediated Pathway. Biol Trace Elem Res 2018;181:62-70. [Crossref] [PubMed]
- Castora FJ. Mitochondrial function and abnormalities implicated in the pathogenesis of ASD. Prog Neuropsychopharmacol Biol Psychiatry 2019;92:83-108. [Crossref] [PubMed]
- Ahmed AS, Sheng MH, Wasnik S, et al. Effect of aging on stem cells. World J Exp Med 2017;7:1-10. [Crossref] [PubMed]
- Chung S, Dzeja PP, Faustino RS, et al. Mitochondrial oxidative metabolism is required for the cardiac differentiation of stem cells. Nat Clin Pract Cardiovasc Med 2007;4 Suppl 1:S60-S67. [Crossref] [PubMed]
- Pereira SL, Graos M, Rodrigues AS, et al. Inhibition of mitochondrial complex III blocks neuronal differentiation and maintains embryonic stem cell pluripotency. PLoS One 2013;8:e82095. [Crossref] [PubMed]
- Chen X, Zhao C, Xu Y, et al. Adipose-specific BMP and Activin Membrane-Bound Inhibitor (BAMBI) Deletion Promotes Adipogenesis by Accelerating ROS Production. J Biol Chem 2020. Epub ahead of print. [Crossref]
- Cohen A, Mulas R, Seri M, et al. Meier‐Gorlin syndrome (ear–patella–short stature syndrome) in an Italian patient: Clinical evaluation and analysis of possible candidate genes. Am J Med Genet 2002;107:48-51. [Crossref] [PubMed]
- Zhang QG, Zhang J, Yu P, et al. Environmental and genetic factors associated with congenital microtia: a case-control study in Jiangsu, China, 2004 to 2007. Plast Reconstr Surg 2009;124:1157-64. [Crossref] [PubMed]
- Tremblay M, Viala S, Shafer ME, et al. Regulation of stem/progenitor cell maintenance by BMP5 in prostate homeostasis and cancer initiation. Elife 2020;9:e54542. [Crossref] [PubMed]
- Minoux M, Kratochwil CF, Ducret S, et al. Mouse Hoxa2 mutations provide a model for microtia and auricle du-plication. Development 2013;140:4386-97. [Crossref] [PubMed]
- Liu J, Cao L, Chen J, et al. Bmi1 regulates mitochondrial function and the DNA damage response pathway. Nature 2009;459:387-92. [Crossref] [PubMed]
- Seo AY, Joseph AM, Dutta D, et al. New insights into the role of mitochondria in aging: mitochondrial dynamics and more. J Cell Sci 2010;123:2533-42. [Crossref] [PubMed]
- Elgass K, Pakay J, Ryan MT, et al. Recent advances into the understanding of mitochondrial fission. Biochim Biophys Acta 2013;1833:150-61. [Crossref] [PubMed]
- Chi L, Wang N, Yang W, et al. Protection of Myocardial Ischemia-Reperfusion by Therapeutic Hypercapnia: a Mechanism Involving Improvements in Mitochondrial Biogenesis and Function. J Cardiovasc Transl Res 2019;12:467-77. [Crossref] [PubMed]
- Zhou R, Yazdi AS, Menu P, et al. A role for mitochondria in NLRP3 inflammasome activation. Nature 2011;469:221-5. [Crossref] [PubMed]
- Shoshan-Barmatz V, Krelin Y, Shteinfer-Kuzmine A. VDAC1 functions in Ca(2+) homeostasis and cell life and death in health and disease. Cell Calcium 2018;69:81-100. [Crossref] [PubMed]
- Cheng EH, Sheiko TV, Fisher JK, et al. VDAC2 inhibits BAK activation and mitochondrial apoptosis. SCience 2003;301:513-7. [Crossref] [PubMed]
- Divakaruni AS, Hsieh WY, Minarrieta L, et al. Etomoxir Inhibits Macrophage Polarization by Disrupting CoA Homeostasis. Cell Metab 2018;28:490-503.e7. [Crossref] [PubMed]
- Cohen MV, Yang XM, Downey JM. The pH hypothesis of postconditioning: staccato reperfusion reintroduces oxygen and perpetuates myocardial acidosis. Circulation 2007;115:1895-903. [Crossref] [PubMed]
- Qiao X, Xu J, Yang QJ, et al. Transient acidosis during early reperfusion attenuates myocardium ischemia reperfusion injury via PI3k-Akt-eNOS signaling pathway. Oxid Med Cell Longev 2013;2013:126083. [Crossref] [PubMed]
- Shi T, Dansen TB. Reactive Oxygen Species Induced p53 Activation: DNA Damage, Redox Signaling, or Both? Antioxid Redox Signal 2020;33:839-59. [Crossref] [PubMed]
- Yu Y, Mutlu AS, Liu H, et al. High-throughput screens using photo-highlighting discover BMP signaling in mitochondrial lipid oxidation. Nat Commun 2017;8:865. [Crossref] [PubMed]
- Stone SJ, Myers HM, Watkins SM, et al. Lipopenia and skin barrier abnormalities in DGAT2-deficient mice. J Biol Chem 2004;279:11767-76. [Crossref] [PubMed]
(English Language Editor: J. Reynolds)