Leukemia inhibitory factor protects photoreceptor cone cells against oxidative damage through activating JAK/STAT3 signaling
Introduction
Age-related macular degeneration (AMD) is a retinal degenerative disease, which is known to be a leading cause of severe visual impairment (1). The retinal photic injury of AMD is typically associated with excessive light exposure and the subsequent induction of oxidative stress (2,3). Upon exposure to light, photochemical damage to the retina is initiated, resulting in increases in reactive oxygen species (ROS) and decreased levels of superoxide dismutase (SOD), which can then progress into the pathology of AMD (4-6). The degeneration of retinal pigment epithelial (RPE) cells is considered to be the originating factor contributing to AMD (7). This subsequently promotes the degeneration of photoreceptor cells and thus, leads to vision loss (8). Furthermore, accumulating evidence has suggested that oxidative stress can induce cell death in photoreceptors and RPE cells due to the generation of ROS, such as hydrogen peroxide (H2O2) and superoxide (O2−) (9,10). Indeed, phototoxicity in the retina has been demonstrated to exert significant damage to photoreceptors (11,12).
Leukemia inhibitory factor (LIF) is a member of the interleukin 6 (IL-6) cytokine family; all members have their own receptors but share the common transmembrane receptor subunit gp130. Activated gp130 induces signaling through the Janus kinase (JAK) and signal transducer and activator of transcription (STAT) pathway, the mitogen-activated protein kinase (MAPK) pathway, and the PI3K pathway (13,14). Among these signaling events, JAK/STAT3 pathway had been considered to be the most important, which could activate the upregulation of several genes important for neuroprotection. In the early 1990s, the neuroprotective effect of LIF was recognized in cultured neuronal cells and a variety of animal models, and this effect was reported to occur in the retina following light damage (15,16). For example, the expression levels of LIF in the retina were found to be increased following the exposure to excessive light (17), whereas the lack of LIF signaling led to the increased death of photoreceptors in a light-induced LIF-/- mice model (18). Other studies have reported that the intravitreal injection of recombinant LIF could protect photoreceptors against light damage, in which the protective effects occurred through the LIFR/gp130 receptor complex and the subsequent activation of the JAK/STAT3 signaling pathway (19). These findings suggest that LIF may be a potential treatment for retinal degeneration.
In vertebrates, the well-organized structure of the retina includes seven neuronal cell types and one glial cell type. Approximately 70% of the cells in the retina are photoreceptors, which can be classified into two types: rods and cones (20). In addition to the neuronal cells in the retina, retinal glia cells, also known as Müller cells, are also present. Under numerous conditions of pathogenic stimuli, Müller cells become activated and respond to injury by producing multiple survival or inflammatory factors (21). Among these, LIF is a prominent protein that promotes photoreceptor survival (22). Previous studies have revealed that cones are less susceptible to light damage compared with rods, but cones may also die following a delayed response due to a bystander effect that may include reduced metabolic support (23,24). Due to rods being more common than cones, with ~100 million rod cells compared with ~7 million cone cells, the mechanisms behind light-induced damage in rods has been well investigated; however, accumulating evidence indicates that light-induced damage also affects cones, detailed mechanisms of which remain largely unclear. Therefore, the current study aimed to evaluate the protective effect of LIF against oxidative damage in photoreceptor cone cells and to clarify the possible mechanisms which underlie it.
We present the following article in accordance with the ARRIVE reporting checklist (available at http://dx.doi.org/10.21037/atm-20-8040).
Methods
Animals studies
A total of 45 BALB/c mice (age, 5–6 weeks; weight, 15–18 g) were obtained from the Shanghai Laboratory Animal Center. The mice were divided into 5 groups: (I) a normal control group (n=15), (II) a vehicle control [phosphate-buffered saline (PBS)] group without light damage (n=15), (III) a LIF treatment group without light damage (n=15), (IV) a vehicle control (PBS) group with light damage (n=15); and (V) a LIF treatment group with light damage (n=15). The animals were housed at The First Affiliated Hospital of Zhengzhou University at 25 °C in a 12-h light–dark cycle. All procedures were in accordance with the National Institutes of Health Guide for the Care and Use of Laboratory Animals. Ethical approval was obtained from the Ethics Committee of Zhengzhou University.
The mice were anesthetized with an intraperitoneal injection of 10 mg/g if xylazine and 70 mg/g of ketamine (Pfizer Inc., New York, NY, USA), and subsequently injected with 1 µL of LIF solution (0.4 g/L; Abcam, Cambridge, UK) or PBS into the vitreous chamber using a 36-gauge needle. For each mouse, the right eye was injected with LIF, whereas the left eye was injected with PBS and served as the control. For light exposure, mice were adapted in the dark for 12 h prior to being exposed to 5,000 lux bright light for 4 h from 8:00 pm to 12:00 am. All mice were allowed to recover for 24 h in the dark following light exposure and were then housed in normal lighting for 7 days before analysis by electroretinography (ERG), immunofluorescence, and western blotting.
Electroretinography (ERG)
Flash ERGs were recorded with an Espion E2 ERG system (Diagnosys, Lowell, MA, USA). Briefly, 5 mice/group were dark-adapted overnight and prepared for ERG recording in dim red light. Each mouse was anesthetized under dim red light, and pupils were dilated with one drop of 10% phenylephrine (Pfizer Inc.). The body temperature of the mice was maintained close to 37 °C with a water-heating pad. Animals were placed inside a Ganzfeld illuminating sphere with a gold electrode placed on the cornea. The reference electrode was hooked in the mouth and a ground wire was attached to the tail. After light adaptation (white light; 50 cd/m2) for 10 min, the function of whole cone cells, M-cone cells, and S-cone cells were detected; the parameters used are listed in Table 1. The amplitude of the a-wave was measured from the baseline to the a-wave trough. The amplitude of the b-wave was measured from the trough of the a-wave to the peak of the b-wave.
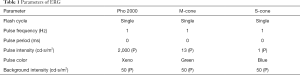
Full table
Morphologic analysis
Mice were sacrificed by displacement of air with 100% CO2 with the eyes being marked on the superior side (n=5/group). The eyes were subsequently enucleated and fixed overnight in PerFix (20% isopropanol, 2% trichloroacetic acid, 4% paraformaldehyde and 2% zinc chloride) and then placed in 70% ethanol. The eyes were then embedded in paraffin, and 5-µm sagittal sections were cut through the optic disc. To assess the effect of the treatment on the retinal cells, the paraffin-embedded sections were rehydrated, blocked, and incubated with a rabbit anti-mouse M-cone or S-cone polyclonal antibody (Santa Cruz Biotechnology, Inc., Dallas, TX, USA). Following the primary antibody incubation, sections were incubated with Alexa Fluor 594-conjugated goat anti-rabbit IgG secondary antibody (Molecular Probes; Thermo Fisher Scientific, Inc., USA); for the negative control, the primary antibody was replaced with PBS.
Reverse transcription-quantitative polymerase chain reaction (RT-qPCR)
Mice (n=5) were sacrificed with exposure to CO2, and the retina of the eyes were harvested and preserved in liquid nitrogen for subsequent experimentation. Total RNA was extracted from the retina using TRIzol® reagent (Thermo Fisher Scientific, Inc.), according to the manufacturer’s protocol. Total RNA was reverse transcribed into cDNA using an RT kit (Thermo Fisher Scientific, Inc.). qPCR was subsequently performed using a SYBR® Green Premix Ex TaqTM Real-time PCR kit (Takara Bio USA, Inc., Shiga, Japan) and an ABI 7500 thermocycler (Applied Biosystems; Thermo Fisher Scientific, Inc.). The following thermocycling conditions were used for the qPCR: initial denaturation at 95 °C for 10 min; followed by 40 cycles of denaturation at 95 °C for 20 sec, annellation at 63 °C for 20 sec, and extension at 72 °C for 20 s. Expression levels were quantified using the 2−ΔΔCq method and GAPDH was used as the internal loading control.
Cell culture and H2O2 treatment
The murine photoreceptor-derived 661W cell line was purchased from the American Type Culture Collection. The cells were cultured in Dulbecco’s Modified Eagle Medium (DMEM) (Merck KGaA, St. Louis, MO, USA), supplemented with 10% fetal bovine serum (FBS; Merck KGaA), 100 U/mL of penicillin, and 50 U/mL of streptomycin, and maintained in a 5% CO2-humidified incubator at 37 °C. For H2O2 exposure, 661W cells were serum-starved for 1 h prior to treatment with H2O2. For drug treatment, 661W cells were pretreated with 5 ng/mL LIF for 1 h prior to treatment with 1 mM H2O2, with or without pretreatment with 50 µM STAT3 inhibitor S3I201 (Merck KGaA) for 1 h prior to the treatment with LIF and H2O2. Cell viability; apoptosis; the levels of oxidative stress, including ROS, malondialdehyde (MDA), SOD, catalase (CAT), and glutathione peroxidase (GPx); and the phosphorylation of extracellular signal-regulated kinases (ERK)1/2, AKT and STAT3; were subsequently determined.
Cell viability assay
Following the treatment of cells, 10 µL of MTT solution (5 mg/mL) was added to each well of the 96-well plate. Following incubation for 4 h, the medium was removed and 150 µL of dimethyl sulfoxide (DMSO) was added. The absorbance value at 570 nm was read using a microplate reader (Omega Bio-Tek, Inc., Norcross, GA, USA).
Western blotting
Membranes were incubated with the following antibodies purchased from Cell Signaling Technology, Inc: anti-Bcl-2 (cat. no. 3498), anti-Bax (cat. no. 2772), anti-cleaved caspase 3 (cat. no. 9664), anti-phosphorylated (phospho)-p44/42 MAPK (ERK1/2; Thr202/Tyr204; cat. no. 4370), anti-p44/42 MAPK (137F5; cat. no. 4695), anti-phospho-STAT3 (Tyr705; cat. no. 9145), anti-STAT3 (79D7; cat. no. 4904), anti-phospho-AKT (Ser473; cat. no. 4060), anti-AKT (cat. no. 9272), and anti-GAPDH (cat. no. 5174). Following the primary antibody incubation, a horseradish peroxidase-conjugated immunoglobin G secondary antibody was subsequently incubated with the membrane. Protein expression levels were quantified using ImageJ software (National Institutes of Health). Following the normalization to GAPDH, the average band intensity value obtained from the untreated samples was used to calculate the fold change in protein expression levels for the treated samples.
Oxidative stress assay
Cells were treated as described above, and the production of intracellular ROS, the content of MDA, and the activity of SOD was determined according to the manufacturer's protocol (Cell Biolabs, Inc., San Diego, CA, USA). The activities of CAT and GPx were determined according to the manufacturer’s protocol (Cayman Chemical Company, Ann Arbor, MI, USA).
Apoptosis analysis
Apoptosis analysis was performed using a cell death detection ELISAplus kit (Roche Diagnostics GmbH, Basel, Switzerland), according to the manufacturer’s protocol. The optical density (OD405 nm) was determined using a microplate reader (Bio-Rad Laboratories, Inc., Hercules, CA, USA).
Statistical analysis
Statistical analysis was performed using SPSS version 22.0 software (IBM Corp., Armonk, NY, USA) and GraphPad Prism version 5.0 software (GraphPad Software, Inc., San Diego, CA, USA). Data are presented as the mean ± SD. Statistical differences were determined using a Student’s t-test. A P value <0.05 was considered to indicate a statistically significant difference.
Results
Neuroprotective effect of LIF against light damage in the retina
To determine whether or not the intravitreal injection of LIF prior to light damage protected photoreceptor function and morphology, a light-induced damage mouse model was established. To analyze the function of cone cells, the b-wave amplitude was measured using ERG, which determines the functional integrity of the cone cells in response to light. Under stimulation with white, green, and blue light, the b-wave amplitudes of all groups exhibited consistent trends. The eyes from the PBS and LIF-treated groups demonstrated no significant changes in the b-wave amplitudes compared with the normal controls (P>0.05; Figure 1). Upon light-induced damage, the b-wave amplitudes in eyes treated with PBS were significantly decreased compared with the PBS controls (P<0.05; Figure 1). Conversely, following the exposure to light damage, the b-wave amplitudes in eyes treated with LIF were significantly increased compared with eyes treated with PBS (P<0.05; Figure 1). These data suggested that the intravitreous injection of PBS had no significant effect on the cone cells, but the light-induced damage that affected the function of cone cells may be protected by LIF treatment.
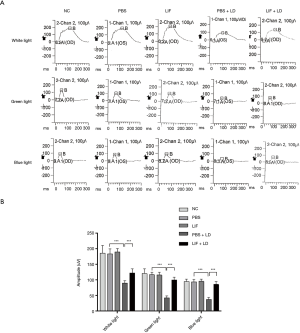
Morphological changes of cone cells in the retina following LIF treatment
In the retina, although cone cells are less sensitive to light compared with rod cells, they can detect color. There are two types of pigment in mice: M-cones that absorb green light, and S-cones that absorb blue light. The extracellular segments of M-cones and S-cones in the PBS and LIF groups were tapered and arranged in sequence, with no significant changes observed in the quantity and morphology (Figure 2); however, the quantity of M-cones and S-cones in the eyes exposed to light damage were significantly reduced, especially in the eyes treated with PBS. In the eyes treated with PBS followed by the exposure to light damage, M-cones and S-cones were observed to be clustered in spots or fragments. This morphological change could be partially blocked following the pretreatment of the eyes with LIF. In conclusion, the intravitreous injection of PBS or LIF had no significant effect on the morphology of cone cells, but the induction of light altered both the quantity and morphology of cone cells, with the LIF pretreatment being observed to partially protect cone cells from light-induced damage.
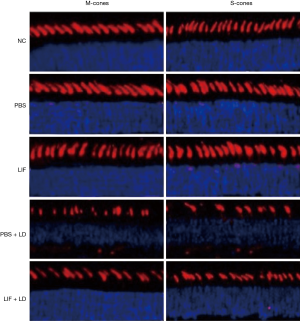
Protective effect of LIF in the retina was associated with STAT3 activation
LIF can activate gp130 and phosphorylate downstream signaling pathways, such as JAK/STAT3, MAPK/ERK, or PI3K/AKT. To determine whether the activation of these signaling proteins participated in the protective effect of LIF against light-induced retinal damage, phosphorylated STAT3 on Tyr705, ERK1/2 and AKT on Ser473 expression levels in the retina of each group were analyzed. LIF markedly increased the phosphorylation of STAT3 compared with the normal controls or the PBS group (Figure 3). Eyes that were exposed to light damage following PBS treatment had significantly increased phosphorylation levels of STAT3, ERK1/2, and AKT Ser473 compared with the PBS group (P<0.05). When compared with the eyes exposed to light damage followed by PBS treatment, LIF pretreatment increased the phosphorylation of STAT3 significantly (6.5±2.9 vs. 14.7±3.2 fold, respectively; Figure 3). As for p-ERK1/2 and p-AKT Ser473, no significant differences were found between the LIF and PBS pretreatment following light-induced damage. In conclusion, these findings suggested that STAT3 activation may contribute to the protective effect of LIF against light-induced retinal damage.
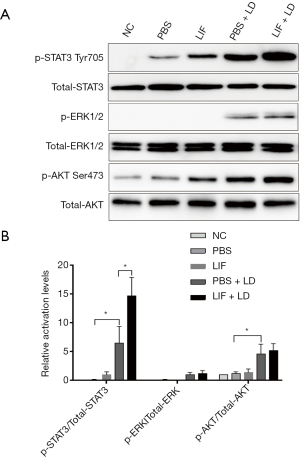
Protective effect of LIF in the retina was related to the altered expression levels of apoptosis and proliferation-related genes
Based on the above findings, it was hypothesized that the JAK/STAT3 signaling pathway may be strongly activated in the retina following LIF pretreatment prior to light exposure. In fact, the activation of the JAK/STAT3 signaling pathway may be involved in several cellular functions, including differentiation regulation, cell cycle arrest, and apoptosis. To investigate the complex protective mechanism of LIF against light-induced oxidative damage, the expression levels of the following genes were detected in the retina: antioxidant genes, SOD1, CAT and GPx1; major genes involved in the JAK/STAT3 pathway, STAT3, SOCS3 and JAK3; apoptosis-related genes, BCL2A1D, ATF3, and DDIT4; and proliferation-related genes, GADD45A, GZMM, and PARP1. Prior to the stimulation of light damage, LIF pretreatment was observed to increase the expression levels of the antioxidant genes SOD1, CAT and GPx1, along with STAT3, SOCS3, and JAK3 (Figure 4), which confirmed the participation of the JAK/STAT3 signaling pathway in the protective effect of LIF against light-induced oxidative damage. Furthermore, increased expression levels of the anti-apoptotic genes BCL2A1D and ATF3, and the pro-proliferative genes, GADD45A and PARP12, along with the decreased expression levels of the pro-apoptotic gene DDIT4 and the anti-proliferative gene GZMM, were found in the retinas pretreated with LIF prior to light-induced damage. Altogether, these findings suggested that LIF may exert its protective effect against light damage through increasing the expression levels of anti-apoptotic and pro-proliferative genes and decreasing the expression levels of pro-apoptotic and anti-proliferative genes.
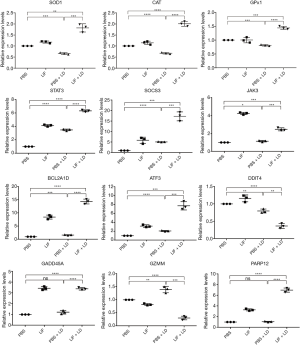
LIF protected 661W cells against H2O2-induced apoptosis
To further study the protective mechanism of LIF in cone cells, 661W cells and an H2O2-induced oxidative damage model was used. To identify the role of LIF in H2O2-induced cell damage, cell viability was first examined. The treatment of 661W cells with 1 mM H2O2 for 12 h led to an ~50% loss in cell viability (Figure 5A). By contrast, treatment with 5 ng/mL LIF followed by 1 mM H2O2 led to increased cell survival in 661W cells (P<0.05). Cell apoptosis was subsequently detected, which indicated that while H2O2 stimulation induced cell apoptosis, LIF treatment markedly decreased the levels of H2O2-induced cell apoptosis (P<0.05) (Figure 5B). Furthermore, LIF pretreatment increased the expression levels of the anti-apoptotic protein Bcl-2, and decreased the expression levels of the pro-apoptotic protein BAX, the downstream protein cleaved caspase 3, and the ratio of BAX/Bcl-2 following H2O2 stimulation in 661W cells (Figure 5C-E). Collectively, these findings suggested that LIF may alleviate H2O2-induced apoptosis in 661W cells.
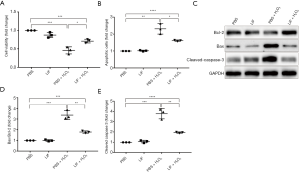
LIF inhibited oxidative stress in H2O2-stimulated 661W cells
Oxidative stress serves an important role in the H2O2-induced apoptosis of 661W cells. To investigate the potential role of LIF in H2O2-induced oxidative stress, the changes to the oxidative index (ROS, MDA, SOD, CAT, and GPx levels) in H2O2-stimulated 661W cells were evaluated. H2O2 stimulation significantly increased the production of ROS, whereas LIF treatment diminished the increased production of ROS observed in response to H2O2 (Figure 6A). Notably, LIF treatment also attenuated H2O2-induced MDA content (Figure 6B). In addition, the activities of the antioxidant enzyme SOD, CAT and GPx were evaluated; in response to H2O2 treatment, there was significantly decreased activity; however, this decrease in activity was blocked by LIF treatment (Figure 6C,D,E). Taken together, these data indicated that LIF may exert an antioxidant effect, which may protect against H2O2-induced oxidative damage.
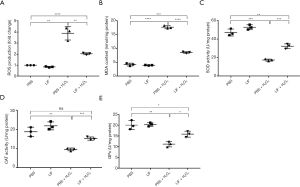
LIF exerted a protective effect against H2O2-induced damage through targeting the STAT3 signaling pathway
To further investigate the potential protective mechanism of LIF against oxidative damage in H2O2-stimulated 661W cells, phosphorylated (p)-STAT3 Tyr705, p-ERK1/2, and p-AKT Ser473 expression levels were detected. Compared with H2O2-treated cells, the expression levels of p-STAT3 Tyr705 were significantly increased in 661W cells treated with 5 ng/mL LIF followed by 1 mM H2O2 (P<0.05; Figure 7A,B). No significant changes were observed in the activation of ERK1/2 and AKT Ser473 compared with H2O2-treated cells (P>0.05). Subsequently, to confirm the protective role of LIF-induced STAT3 against oxidative damage, 661W cells were pre-incubated with the STAT3 inhibitor S3I201 for 1 h prior to treatment with LIF and H2O2. It was observed that the administration of S3I201 markedly abrogated the protective effects of LIF on H2O2-triggered apoptosis (Figure 7C,D). In addition, the inhibition of STAT3 significantly eliminated the beneficial effects of LIF over ROS production in response to H2O2 (Figure 7E). These findings suggested that LIF may exert protective effects against H2O2-induced apoptosis and oxidative stress through targeting, at least in part, the STAT3 signaling pathway.
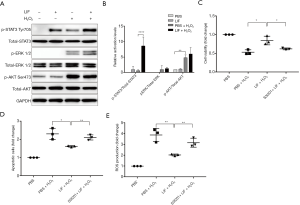
Discussion
Photoreceptor cells are sensory neurons in the eye that absorb light to stimulate vision, and damage to these photoreceptors can lead to permanent blindness (25). Upon exposure to prolonged periods of bright light, oxidative damage and subsequent cell death can occur in photoreceptors. Numerous studies have demonstrated that the exposure to excessive light leads to a significant increase in the expression levels of LIF, basic fibroblast growth factor (bFGF) and ciliary neurotrophic factor (CNTF) (26,27). In fact, some studies have also reported that the exogenous injection of these factors can protect photoreceptors from light-induced damage (28,29); however, it remains relatively unclear whether LIF can protect photoreceptor cone cells from light damage.
In the present study, an in vivo light-damage model was created by placing mice under light stress 2 days post-injection. To determine the functional and morphological changes of cone cells in the retina, ERGs and immunofluorescent staining were performed. Photoreceptors can be divided into rods and cones; among the four different opsin molecules found in humans, one type is found in rods and is responsible for low-light vision, while the other three types are found in cones and provide color vision by responding to red (558 nm), green (531 nm), and blue (419 nm) wavelengths (30). Cone cells are often classified into long (L), medium (M) and short (S) wavelength cones; however, mice only have two types: an M cone to detect green wavelengths and an S cone for blue wavelengths (30). Therefore, the present study used white, green, and blue light stimulation for ERG examinations to detect the function of the whole cone, M cone, and S cone cells. The morphological changes in M- and S-cones were also detected using their respective antibodies. Results from the ERG demonstrated that compared with the eyes in the normal control group, the function of M- and S- cones in the eyes treated with the intravitreal injection of PBS followed by light-induced damage was reduced to 30%. By contrast, the intravitreal injection of LIF recovered the M- and S-cones to 80–90% of their normal function. The morphological changes that occurred in M- and S-cones demonstrated the same trend as the functional changes. These findings suggest that LIF treatment may protect both the function and morphology of cones cells from light-induced damage.
Light-induced oxidative damage is a major stressor that generally subverts life of aerobic species, key adaptive mechanisms to cope with oxidative damage are the expression of a multitude of genes, including those involved in cell survival. Importantly, it can also be sensed via changes in metabolite levels and generation of ROS by mitochondria. A previous study reported that a single intravitreal injection of LIF activated downstream signaling pathways, including STAT3, ERK1/2, and AKT (31); however, despite studies having investigated the activation of STAT3 and ERK1/2 in Müller cells, the activation in photoreceptors has yet to be elucidated (32). In the present study, it was observed that the intravitreal injection of LIF resulted in STAT3 activation in the retina. To further investigate its molecular mechanism, differentially expressed genes in the retina between the LIF and PBS groups subjected to light damage were detected using microarray analysis (data not shown), and the expression of apoptosis- and proliferation-related genes were validated by RT-qPCR. The observed increased mRNA expression levels of SOD, CAT, and GPx1 validated the occurrence of light-induced oxidative damage; the increased mRNA expression of STAT3, SOCS3, and JAK3 confirmed the LIF-induced protective role of the JAK/STAT3 pathway against light-induced oxidative damage; and the increased expression levels of the anti-apoptotic genes BCL2A1D and ATF3 and the pro-proliferative genes GADD45A and PARP12, alongside the decreased expression of the pro-apoptotic gene DDIT4 and the anti-proliferative gene GZMM, indicated that LIF-induced activation of the JAK/STAT3 signaling pathway may promote anti-apoptotic and pro-proliferative functions that protect the retina from oxidative damage.
To further confirm the protective effect of LIF against light damage in vitro, an oxidative damage cell model was used. Treatment with H2O2 is a commonly used strategy to study oxidative stress in a variety of cell lines (33). Hence, H2O2-induced 661W cone cells were used as an in vitro model to study the biological function of photoreceptors. The findings of the present study demonstrated that H2O2 could decrease the cell viability and increase apoptosis in 661W cells, while the addition of LIF treatment alleviated the H2O2-induced apoptosis. Previous studies have reported that LIF is involved in the apoptosis of astrocytes, cholangiocarcinoma cells, and podocytes (34-36); however, the relationship between LIF and apoptosis in cone cells has yet to be reported. To the best of our knowledge, there have been no other studies that have reported on the protective effect of LIF against apoptosis in cone cells; our study thus provides valuable and novel insight into the potential role of LIF in H2O2-induced apoptotic processes in cone cells.
Accumulating research has indicated that oxidative stress serves a crucial role in the pathogenesis of AMD (37). Oxidative stress is thought to be a major contributor to photoreceptor damage, which may eventually lead to a loss of vision (38). These processes are involved in the formation of ROS, and increasing evidence has demonstrated that oxidative stress-induced ROS production exceeds the limits of scavenging, which leads to an imbalance between the oxidative and antioxidant systems (39). As a prominent antioxidant enzyme, SOD can protect cells from oxidative damage (40). To investigate whether LIF participated in the oxidative stress process, the effects of LIF on oxidative stress were evaluated by determining the levels of ROS production, MDA content, and the activities of SOD, CAT and GPx. The results demonstrated that treatment with LIF reduced the H2O2-induced production of ROS and MDA content, indicating that LIF may exert antioxidant activity in cone cells in response to oxidative damage. Also, LIF treatment increased the activity of SOD, CAT, and GPx in order to avoid peroxidative damage. Altogether, we can surmise from these findings that LIF may reduce H2O2-induced oxidative damage through promoting antioxidant activities.
To validate the role of JAK/STAT3 signaling in the protective effect of LIF against oxidative damage in 661W cone cells, the phosphorylation of STAT3 in 661W cells under different treatments was detected; a significant increase in the activation of STAT3 was observed in H2O2-induced 661W cells pretreated with LIF. Subsequently, the use of the STAT3 inhibitor S3I201 to block the function of STAT3 inhibited the protective effects of LIF on H2O2-induced apoptosis and ROS production. Collectively, these findings suggest that LIF may prevent H2O2-induced apoptosis and oxidative stress through targeting STAT3 signaling in photoreceptor cone cells.
In conclusion, these findings indicate that LIF may protect cone cells against oxidative damage through its anti-apoptotic and antioxidant activity, by targeting, at least in part, the STAT3 signaling pathway. Inhibition of this pathway provides a potential therapeutic strategy to ameliorate retinal oxidative stress that occurs due to prolonged light exposure or other factors, and help prevent the progression of AMD.
Acknowledgments
Funding: This study was supported by grants provided by the National Natural Science Foundation of China (no. 81800830), the Key Research and Development and Promotion Project (Science and Technology) Program of Henan Province (no. 192102310077), and the Science and Technology Project pf The Health Planning Committee of Sichuan (no. 18PJ119).
Footnote
Reporting Checklist: The authors have completed the ARRIVE reporting checklist. Available at http://dx.doi.org/10.21037/atm-20-8040
Data Sharing Statement: Available at http://dx.doi.org/10.21037/atm-20-8040
Conflicts of Interest: All authors have completed the ICMJE uniform disclosure form (available at http://dx.doi.org/10.21037/atm-20-8040). The authors have no conflicts of interest to declare.
Ethical Statement: The authors are accountable for all aspects of the work in ensuring that questions related to the accuracy or integrity of any part of the work are appropriately investigated and resolved. All procedures were in accordance with the National Institutes of Health Guide for the Care and Use of Laboratory Animals. The current study was approved by the ethics committee of the First Affiliated Hospital of Zhengzhou University (Zhengzhou, China).
Open Access Statement: This is an Open Access article distributed in accordance with the Creative Commons Attribution-NonCommercial-NoDerivs 4.0 International License (CC BY-NC-ND 4.0), which permits the non-commercial replication and distribution of the article with the strict proviso that no changes or edits are made and the original work is properly cited (including links to both the formal publication through the relevant DOI and the license). See: https://creativecommons.org/licenses/by-nc-nd/4.0/.
References
- Hanus J, Zhao F, Wang S. Current therapeutic developments in atrophic age-related macular degeneration. Br J Ophthalmol 2016;100:122-7. [Crossref] [PubMed]
- Pawlowska E, Szczepanska J, Koskela A, et al. Dietary polyphenols in age-related macular degeneration: protection against oxidative stress and beyond. Oxid Med Cell Longev 2019;2019:9682318. [Crossref] [PubMed]
- Chiras D, Kitsos G, Petersen MB, et al. Oxidative stress in dry age-related macular degeneration and exfoliation syndrome. Crit Rev Clin Lab Sci 2015;52:12-27. [Crossref] [PubMed]
- Kaarniranta K, Pawlowska E, Szczepanska J, et al. Role of mitochondrial DNA damage in ROS-mediated pathogenesis of Age-Related Macular Degeneration (AMD). Int J Mol Sci 2019;20:2374. [Crossref] [PubMed]
- Dulull NK, Dias DA, Thrimawithana TR, et al. L-Sulforaphane confers protection against oxidative stress in an in vitro model of Age-Related Macular Degeneration. Curr Mol Pharmacol 2018;11:237-53. [Crossref] [PubMed]
- Khandhadia S, Lotery A. Oxidation and age-related macular degeneration: insights from molecular biology. Expert Rev Mol Med 2010;12:e34. [Crossref] [PubMed]
- Ao J, Wood JP, Chidlow G, et al. Retinal pigment epithelium in the pathogenesis of age-related macular degeneration and photobiomodulation as a potential therapy? Clin Exp Ophthalmol 2018;46:670-86. [Crossref] [PubMed]
- Bardak H, Uğuz AC, Bardak Y. Protective effects of melatonin and memantine in human retinal pigment epithelium (ARPE-19) cells against 2-ethylpyridine- induced oxidative stress: implications for age-related macular degeneration. Cutan Ocul Toxicol 2018;37:112-20. [Crossref] [PubMed]
- George AK, Singh M, Homme RP, et al. A hypothesis for treating inflammation and oxidative stress with hydrogen sulfide during age-related macular degeneration. Int J Ophthalmol 2018;11:881-7. [PubMed]
- Nowak JZ. Oxidative stress, polyunsaturated fatty acids-derived oxidation products and bisretinoids as potential inducers of CNS diseases: focus on age-related macular degeneration. Pharmacol Rep 2013;65:288-304. [Crossref] [PubMed]
- Jaadane I, Boulenguez P, Chahory S, et al. Retinal damage induced by commercial light emitting diodes (LEDs). Free Radic Biol Med 2015;84:373-84. [Crossref] [PubMed]
- Imai S, Inokuchi Y, Nakamura S, et al. Systemic sdministration of a free radical scavenger, edaravone, protects against light-induced photoreceptor degeneration in the mouse retina. Eur J Pharmacol 2010;642:77-85. [Crossref] [PubMed]
- Taga T. Gp130, a shared signal transducing receptor component for hematopoietic and neuropoietic cytokines. J Neurochem 1996;67:1-10. [Crossref] [PubMed]
- Kunisada K, Hirota H, Fujio Y, et al. Activation of JAK-STAT and MAP kinases by leukemia inhibitory factor through gp130 in cardiac myocytes. Circulation 1996;94:2626-32. [Crossref] [PubMed]
- Murphy M, Reid K, Hilton DJ, et al. Generration of sensory neurons is stimulated by leukemia inhibitory factor. Proc Natl Acad Sci U S A 1991;88:3498-501. [Crossref] [PubMed]
- Azari MF, Profyris C, Karnezis T, et al. Leukemia inhibitory factor arrests oligodendrocyte death and demyelination in spinal cord injury. J Neuropathol Exp Neurol 2006;65:914-29. [Crossref] [PubMed]
- Samardzija M, Wenzel A, Aufenberg S, et al. Differential role of Jak-STAT signaling in retinal degenerations. FASEB J 2006;20:2411-13. [Crossref] [PubMed]
- Bürgi S, Samardzija M, Grimm C. Endogenous leukemia inhibitory factor protects photoreceptor cells against light-induced degeneration. Mol Vis 2009;15:1631-7. [PubMed]
- Ueki Y, Wang J, Chollangi S, et al. STAT3 activation in photoreceptors by leukemia inhibitory factor is associated with protection from light damage. J Neurochem 2008;105:784-96. [Crossref] [PubMed]
- DuVal MG, Allison WT. Impacts of the retinal environment and photoreceptor and type on functional regeneration. Neural Regen Res 2017;12:376-9. [Crossref] [PubMed]
- Bringmann A, Reichenbach A. Role of Muller cells in retinal degenerations. Front Biosci 2001;6:E72-92. [Crossref] [PubMed]
- Joly S, Lange C, Thiersch M, et al. Leukemia inhibitory factor extends the lifespan of injured photoreceptors in vivo. J Neurosci 2008;28:13765-74. [Crossref] [PubMed]
- Okano K, Maeda A, Chen Y, et al. Retinal cone and rod photoreceptor cells exhibit differential susceptibility to light-induced damage. J Neurochem 2012;121:146-56. [Crossref] [PubMed]
- Li S, Lee J, Zhou Y, et al. Fatty acid transport protein 4 (FATP4) prevents light-induced degeneration of cone and rod photoreceptors by inhibiting RPE65 isomerase. J Neurosci 2013;33:3178-89. [Crossref] [PubMed]
- Wiley LA, Burnight ER, DeLuca AP, et al. cGMP production of patient-specific iPSCs and photoreceptor precursor cells to treat retinal degenerative blindness. Sci Rep 2016;6:30742. [Crossref] [PubMed]
- Joly S, Pernet V, Chemtob S, et al. Neuroprotection in the juvenile rat model of light-induced retinopathy: evidence suggesting a role for FGF-2 and CNTF. Invest Ophthalmol Vis Sci 2007;48:2311-20. [Crossref] [PubMed]
- Qin Z, Kidd AR 3rd, Thomas JL, et al. FGF signaling regulates rod photoreceptor cell maintenance and regeneration in zebrafish. Exp Eye Res 2011;93:726-34. [Crossref] [PubMed]
- LaVail MM, Faktorovich EG, Hepler JM, et al. Basic fibroblast growth factor protects photoreceptors from light-induced degeneration in albino rats. Ann N Y Acad Sci 1991;638:341-7. [Crossref] [PubMed]
- LaVail MM, Unoki K, Yasumura D, et al. Multiple growth factors, cytokines, and neurotrophins rescue photoreceptors from the damaging effects of constant light. Proc Natl Acad Sci U S A 1992;89:11249-53. [Crossref] [PubMed]
- Molday RS, Moritz OL. Photoreceptors at a glance. J Cell Sci 2015;128:4039-45. [Crossref] [PubMed]
- Ueki Y, Le YZ, Chollangi S, et al. Preconditioning-induced protection of photoreceptors requires activation of the signal-transducing receptor gp130 in photoreceptors. Proc Natl Acad Sci U S A 2009;106:21389-94. [Crossref] [PubMed]
- Pannicke T, Wagner L, Reichenbach A, et al. Electrophysiological characterization of Müller cells from the ischemic retina of mice deficient in the leukemia inhibitory factor. Neurosci Lett 2018;670:69-74. [Crossref] [PubMed]
- Sies H. Role of metabolic H2O2 generation: redox signaling and oxidative stress. J Biol Chem 2014;289:8735-41. [Crossref] [PubMed]
- Huo L, Fan Y, Wang H. Leukemia Inhibitory Factor Receptor Is Involved in Apoptosis in Rat Astrocytes Exposed to Oxygen-Glucose Deprivation. Biomed Res Int 2019;2019:1613820. [Crossref] [PubMed]
- Morton SD, Cadamuro M, Brivio S, et al. Leukemia inhibitory factor protects cholangiocarcinoma cells from drug-induced apoptosis via a PI3K/AKT- dependent Mcl-1 activation. Oncotarget 2015;6:26052-64. [Crossref] [PubMed]
- Xu J, Li Z, Xu P, et al. Protective effects of leukemia inhibitory factor against oxidative stress during high glucose-induced apoptosis in podocytes. Cell Stress Chaperones 2012;17:485-93. [Crossref] [PubMed]
- Fanjul-Moles ML, López-Riquelme GO. Relationship between Oxidative Stress, Circadian Rhythms, and AMD. Oxid Med Cell Longev 2016;2016:7420637. [Crossref] [PubMed]
- Lajko M, Cardona HJ, Taylor JM, et al. Photoreceptor oxidative stress in hyperoxia-induced proliferative retinopathy accelerates rd8 degeneration. PLoS One 2017;12:e0180384. [Crossref] [PubMed]
- Newsholme P, Cruzat VF, Keane KN, et al. Molecular mechanisms of ROS production and oxidative stress in diabetes. Biochem J 2016;473:4527-50. [Crossref] [PubMed]
- Poprac P, Jomova K, Simunkova M, et al. Targeting Free Radicals in Oxidative Stress-Related Human Diseases. Trends Pharmacol Sci 2017;38:592-607. [Crossref] [PubMed]
(English Language Editor: J. Gray)