Abstract
Pancreatic cancer is a highly invasive disease with low survival rates. The high death rates associated with pancreatic cancer are due to multiple factors including late stage diagnosis, multi-drug resistance, invasive nature and restricted access of the therapeutic moiety to the cancer cells due to the stroma. Smart multifunctional nanocarriers that deliver the therapeutic agent in to the cancer tissue as well as enable imaging of the tissue represent an emerging paradigm in cancer therapy. Accurate and reliable detection of cancerous lesions in pancreas is essential for designing appropriate therapeutic strategy to annihilate the highly aggressive pancreatic cancer. A combination of imaging modalities can enhance the reliability of cancer detection. In this context, we report here a hybrid iron oxide-gold nanoparticle with dual contrast enhancing ability for both magnetic resonance imaging (MRI) and micro-computed tomography (micro-CT) that is co-encapsulated with the nucleotide analogue gemcitabine in a chitosan matrix. The theranostic system displayed enhanced cytotoxicity against PanC-1 pancreatic cancer cells when compared to normal cells over 48 h due to differences in cell internalization. The iron oxide-gold hybrid enabled visualization of the theranostic nanoparticle by MRI as well as micro-CT. Further, the magnetocaloric effect of the iron oxide enabled faster release of the chemotherapeutic agent as well as augmented the cytotoxicity by inducing hyperthermia. This system holds promise for further exploration as an integrated diagnostic and therapeutic platform for pancreatic cancer.
Export citation and abstract BibTeX RIS
1. Introduction
Pancreatic cancer is ranked as the seventh most fatal cancer in the world [1]. It remains mostly asymptomatic during the early phases and about 80% of people are diagnosed only after distant metastases occurs [1, 2]. The current standard intervention for treating pancreatic cancer is surgery, but most cancers are not completely resectable. Hence surgery is used in combination with adjuvant therapy like chemotherapy and radiotherapy [3, 4]. Additional challenges in pancreatic cancer therapy arise due to the formation of a stromal barrier that restricts drug permeability, rapid transition from epithelial-to-mesenchymal phenotype leading to faster spread of the disease, and emergence of multi-drug resistant types rendering chemotherapy ineffective [5]. Hence, concerted efforts to design strategies for improvement of the drug uptake in pancreatic cancer cells are underway in the past decade. Commonly used chemotherapy drugs for pancreatic cancer include gemcitabine, 5-fluorouracil, oxaliplatin, irinotecan, leucovorin, Abraxane (nab-Paclitaxel), cisplatin, oxaliplatin, capecitabine, and docetaxel [6]. Among these, the hydrophilic gemcitabine, a nucleoside analogue that inhibits DNA synthesis and blocks the cell cycle at the G1/S phase [7], and used as a first line drug for treatment of advanced pancreatic cancer, was found to be ineffective due to development of higher degree of resistance as a result of reduced intracellular uptake [8]. Combination therapy with Folfirinox, a combination comprising 5-fluorouracil, leucovorin, irinotecan and oxaliplatin, or gemcitabine and Abraxane had shown improvement in survival duration but toxicity issues and high mortality rates still persist [8].
Nanotechnology-driven drug delivery is emerging as a promising solution to overcome several limitations associated with conventional chemotherapy. Nanoparticles are small enough to extravasate and access the cancer tissue. The leaky vasculature in the tumor also facilitates the entry of nanoparticles. Further, introduction of magnetic stimuli responsive elements in the nanocarrier will facilitate improved cellular uptake upon application of an external magnetic field thereby promoting 'on-demand' release of the drug [9]. Different types of nanocarriers have been explored as delivery vehicles for chemotherapeutic agents [10]. These include liposomes, dendrimers natural polymers like fucoidan, fibroin, chitosan [11], and synthetic polymers such as poly(lactide-co-glycolic acid) and poly(caprolactone) apart from inorganic carriers like mesoporous silica [12]. The cationic polymer chitosan derived from crustacean shells and made of D-glucosamine and N-acetyl-D-glucosamine repeating units has been investigated for drug delivery applications owing to its high cell internalization properties and amenability to functionalization [13].
In the context of diagnosis of pancreatic cancer, imaging techniques like micro-computed tomography (micro-CT), magnetic resonance imaging (MRI), ultrasonography, positron emission tomography and magnetic resonance cholangiopancreatography have been commonly used for detection of lesions, tumorous growth and for determining the stage and resectability of the tumor as well as for visual assessment of the effect of therapy [14]. However, accurate diagnosis of pancreatic cancer is difficult due to shadowing effects mediated by gas bubbles and challenges in achieving high levels of spatial resolution. In this context, multi-modal imaging has been proposed as a superior alternative to conventional mono-modal imaging. A combination of micro-CT and MRI has been suggested as a tool for early diagnosis as these techniques in combination can offer spatial resolution that can enable one to distinctly analyze the morphological parameters to distinguish different types of pancreatic masses and ductal anomalies [15]. Use of contrast enhancers for both micro-CT and MRI has been successfully demonstrated to improve their sensitivity and resolution. Both positive (gadolinium chelates) and negative (iron oxide) contrast agents have been used as MRI contrast enhancers to clearly delineate morphological features of tissues [16, 17]. Micro-CT contrast agents have been traditionally used for hard tissues but use of contrast agents like gold nanoparticles have facilitated imaging of soft tissues with high resolution [18]. Integration of two different contrast agents to form a hybrid contrast system enables multi-modal imaging through MRI and micro-CT for precise and rapid diagnosis of the disease [19]. Different combinations of nanoparticles have been explored for multi-modal imaging of pancreatic cancer. These include manganese doped quantum dots that enable imaging by both near infrared (NIR) and MRI, desferroxamine linked with NIR dye for MRI and NIR imaging [20], gold nanoparticle-gadolinium-Cy7 conjugate targeted with an anti-glypican antibody for simultaneous MRI, NIR and fluorescence imaging [21], and iron oxide-gold nanocomposites for MRI–NIR imaging of pancreatic cancer [21]. Constant efforts to improve imaging sensitivity and spatial resolution across multiple imaging modalities have spurred research on development of composite contrast agents. Their immobilization in a nanocarrier can further enhance its residence time and target-specificity without compromising their imaging capabilities.
Co-encapsulation of a therapeutic agent along with the imaging moiety in the nanocarrier confers multi-functionality with both imaging and therapeutic capabilities to the system. Such carriers are known as 'nanotheranostic' systems and have garnered much attention in recent years due to their multiple capabilities to monitor the disease site, accumulation of the drug in the target site as well as monitor the treatment efficacy real-time [22]. Few nanoparticle formulations like irinotecan liposome (Onivyde®) and albumin-conjugated paclitaxel (Abraxane®) have also been administered though their impact on the survival appear to be minimal [23]. Several theranostic carriers have been explored for improved therapy and diagnosis of pancreatic cancer [24]. These include magnetic iron oxide nanoparticles containing gemcitabine and the N-terminal peptide sequence specific for urokinase plasminogen activator receptor [25], and indocyanine green-loaded polymeric nanoparticles [26]. Co-entrapment of gemcitabine and miR-21i, an inhibitor of miR-21 responsible for proliferation of pancreatic cancer cells in gold nanoparticle-polyamidoamine dendrimer constructs that respond to ultrasound [27], and gemcitabine and fluorescein loaded mesoporous SBA-15 silica [28] have also been explored for pancreatic cancer therapy.
In the present study, a theranostic nanocarrier fabricated using chitosan was used for co-encapsulating gemcitabine and an iron-gold composite contrast agent for both MRI and micro-CT. This combination of carrier and cargo explored has not been explored earlier for pancreatic cancer and offers dual imaging and dual therapeutic modalities for superior therapeutic and diagnostic outcomes.The superparamagnetic form of iron serves to induce hyperthermia, which not only aids cell death but also can enhance the release of the chemotherapeutic agent gemcitabine to pancreatic cancer cells thereby augmenting the cytotoxic effects.We believe that encapsulation of gemcitabine in the nanocarrier will improve its cell accumulation and efficacy, which can be effectively monitored using the co-encapsulated dual imaging contrast agent.
2. Materials and methods
2.1. Synthesis of Fe@Au nanoparticles (Fe@Au Nps)
Fe@Au nanoparticles were synthesized using a previously reported reverse micelle method [29]. Briefly, Au precursor was coated on Fe nanoparticles using cetyltrimethylammonium bromide (CTAB, Merck, India) as surfactant, 1-butanol (Merck, India) as co-surfactant and n-heptane (Merck, India) as the oil phase. Initially, the reduction of Fe2+ using NaBH4 (Merck, India) was performed by addition of 0.5 M FeSO4 (Merck, India) aqueous solution to 1.0 M of NaBH4 in the reverse micelle solution. The resultant mixture was continuously stirred at room temperature for 1 h. To achieve Au coating on the iron, the precursor HAuCl4 (0.4 M, Sigma-Aldrich, USA) was prepared as a reverse micelle solution and NaBH4 (1.6 M) solution was added immediately into the above solution and the resultant dark solution was allowed to stir overnight at room temperature. Then, the sample solution was washed using methanol (Merck, India) and chloroform (Merck, India) in the ratio of 1:1 to remove the unreacted molecules. The product was vacuum-dried and used for further analysis.
2.2. Synthesis of chitosan nanoparticles (CNps)
Ionic gelation method was used for synthesizing chitosan nanoparticles [13, 30]. The chitosan precursor (Everest Biotech, Bangalore) was dissolved in 10% acetic acid (Merck, India) at a concentration of 1 mg ml−1. Then, the precursor solution was cross-linked using 0.1% sodium tripolyphosphate (STPP, Merck, India) added in drops. The resultant opalescent suspension was stirred continuously for 2 h and then centrifuged. The pelletized nanoparticles were freeze-dried using lyophilizer (Alpha 1-4S, Martin Christ GmbH, Germany) and used for further experiments.
2.3. Synthesis of gemcitabine-loaded chitosan nanoparticles (CGNps)
Stock solution of gemcitabine (1 mg ml−1) in water (TCI Chemicals, India) was used to prepare various concentrations and added to the chitosan solution (1 mg ml−1) with constant stirring followed by drop-wise addition of STPP solution for cross-linking. The chitosan:drug ratio was varied as 1:1, 1:2, 1:3, 2:1 and 3:1. The obtained nanoparticle suspension was stirred for 2 h and then centrifuged at 12 000 rpm and 10 °C for 25 min. The pellet was re-suspended in water in order to remove excessive amounts of STPP and the unencapsulated gemcitabine. The pellet was then freeze-dried for further analyses.
2.4. Synthesis of Fe@Au loaded chitosan nanoparticles (CFe@Au Nps)
Different concentrations of Fe@Au nanoparticles were dispersed in chitosan solution (1 mg ml−1) and sonicated using a probe sonicator (Vibra Cell, Sonics, USA) at 20% amplitude for 45 min to obtain a homogenous dispersion of Fe@Au nanoparticles. The chitosan:Fe@Au ratios were varied as 1:1, 1:2, 2:1, 3:1 and 4:1. Then, the sample mixture was stirred at room temperature for 2 h following which STPP was added. The reaction mixture was further stirred for 2 h and centrifuged. The resultant pellet was then freeze-dried for future analyses.
2.5. Synthesis of Fe@Au/gemcitabine loaded chitosan nanoparticles (CGFe@Au Nps)
For preparing CGFe@Au nanoparticles, Fe@Au nanoparticles were dispersed in chitosan solution (1 mg ml−1) by sonication. Different concentrations of gemcitabine drug were introduced into the above mixture, which was stirred overnight. The chitosan:gemcitabine:Fe@Au ratios were varied as 3:1:1 and 3:2:1. To this mixture, STPP was added followed by centrifugation, and the resultant pellet was freeze-dried.
2.6. Encapsulation efficiency (EE) of drug
The amount of gemcitabine encapsulated in chitosan and in CGFe@Au nanotheranostic system was determined using UV–Visible spectrophotometry (Lambda 25, Perkin Elmer, USA). Drug-loaded nanoparticles were washed with distilled water to remove the unencapsulated gemcitabine from the surface of the nanocarrier. The free gemcitabine in the supernatant was determined by recording the absorbance in a UV–visible spectrophotometer. The EE was calculated using the following formula [13]:
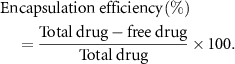
Different drug to carrier ratios were optimized for achieving maximum encapsulation of the drug.
2.7. In vitro drug release studies
The release of gemcitabine from chitosan nanoparticles and CGFe@Au was evaluated in various pH (5.5, 7.4 and 9.2) and also in the presence of protein. One milliliter of drug-loaded nanoparticles was transferred into a dialysis bag (HiMedia, USA), which was immersed in 3 ml buffer, with constant stirring and maintained at 37 °C. At pre-determined time intervals, 3 ml solution was withdrawn and replaced with fresh buffer. The release profile of the drug was measured using UV-visible spectrophotometry at 271 nm [13].
2.8. Physico–chemical characterization
The surface morphological characterization for blank chitosan nanoparticles and gemcitabine loaded chitosan nanoparticles was performed using field emission scanning electron microscopy (JSM 6701F, JEOL, Japan). The samples were fixed on a brass stub and a thin layer of gold was sputter-coated. The samples were then imaged at an acceleration voltage of 3 kV. The ultrafine structure of Fe@Au, CFe@Au and CGFe@Au nanoparticles was observed using field emission transmission electron microscopy (JEM 2100F, JEOL, Japan). FTIR spectrum for the sample was recorded between the wavenumbers 4000 and 400 cm−1 with a resolution of 4 cm−1 and averaging 50 scans using FTIR spectrometer (Spectrum 100, Perkin Elmer, USA). The hydrodynamic size and zeta potential of the nanoparticles were determined by the dynamic light scattering technique using zeta sizer (ZS nano, Malvern Instruments, UK). Thermal analysis of the samples was performed using differential scanning calorimetry (Q20, TA Instruments, USA) and thermogravimetric analysis (TGA) (SDT650, TA Instruments, USA).
2.9. Phantom agar gel assay
Suspensions of CGFe@Au of 0.01–1 mg ml−1 concentrations were prepared by adding 1% (w/v) of agar solution (Merck, India) and the warm mixture was mixed thoroughly and cooled to room temperature. Transverse T1 and T2 weighted spin echo images were acquired using MRI using a 1.5 T scanner (Philips Internal 1.5 Tesla DRW_R1.5, Germany) and the phantom containing agar gels were positioned at the iso-centric position of the magnet. T1 weighted images were obtained using a spin-echo pulse sequence with a slice thickness of 4 mm, constant TE (echo time) of 20 ms and variable TR (repetition time) of 100, 200, 300, 500, 600, 800, 1000, 1500, 2000, 3000, 4000 and 5000 ms. T2 weighted images were acquired using a fixed TR of 5000 ms and variable TE of 20, 40, 60, 80, 100, 120, 140, 160, 180, 200, 220, 240, 260, 280, 300, 320, 380 and 400 ms [31, 32].
2.10. Hyperthermia
Magnetic hyperthermia efficiency of the samples Fe@Au, CFe@Au and CGFe@Au was quantified by measuring the increase in temperature using fiber optic probe in the magnetic hyperthermia unit (MH07-KW, MSI Automation, USA). The fiber optic probe was placed inside a 2 ml Eppendorf tube containing the sample at a concentration of 3 mg ml−1 and different RF frequencies of 350, 390, 440, and 480 kHz were applied [33].
2.11. Micro-CT imaging
Micro-CT images for Fe@Au, CFe@Au and CGFe@Au were captured using micro-CT (Skyscan1176, Bruker, Germany). Nanoparticles of different concentrations (5, 10, 25, 50, 75 mg ml−1) were suspended in a tube containing 1% agarose solidified at room temperature. To determine the contrast enhancement by gold-containing nanoparticles, a voltage of 80 kV, current of 278 μA and Cu 0.1 mm filter was used.
2.12. In vivo micro-CT imaging
The animal studies were conducted at the Central Animal Facility, SASTRA Deemed University, with all protocols approved by the Institutional Animal Ethical Committee (407/SASTRA/IAEC/RPP). All animals were housed in individually ventilated sterile cages and fed with sterilized standard rodent diet. BalB/c mice were anesthetized through an intraperitoneal injection of ketamine and xylazine. Subsequently, 1.25 mg ml−1 of Fe@Au Nps and CGFe@Au Nps solution were intravenously injected via the tail vein. The micro-CT images for control and after the administration of contrast agent were acquired with the x-ray attenuation of pancreas and blood pool to evaluate the prolonged presence of CGFe@Au.
2.13. In vitro cytotoxicity assay
For the evaluation of the effectiveness of the CGFe@Au, in vitro cytotoxicity assay was performed for three concentrations of 5 µM, 50 µM and 100 µM of the free drug and drug-loaded carrier, Fe@Au, CFe@Au and CGFe@Au Nps in PanC1 cells, (NCCS, Pune). The cells were seeded at a density of 1 × 104 cells/well and maintained in Dulbecco's modified Eagle's medium (DMEM) (Gibco, USA) supplemented with 10% fetal bovine serum (Gibco, USA), 1% penicillin G and streptomycin (Gibco, USA) at 37 °C in 5% carbondioxide. The cell viability after 48 h of dosing was determined using standard protocols [13]. The MTS cell viability assay was performed to evaluate the cell damage induced by the nanoparticles. Similar studies were also carried out using H9c2 (NCCS, Pune), a normal myoblast cell line for concentrations ranging from 1 nM to 1 mM of the CGFe@Au nanoparticles to understand the response of normal cells to the developed hybrid theranostic nanocarrier. The concentration of the nanoparticles added was with respect to the drug concentrations.
3. Statistical analysis
Two way ANOVA was used to calculate the statistical significance between the experimental data of two groups and p < 0.05 was considered to be significant.
4. Results and discussion
4.1. Material characterization
The hybrid contrast agent for multimodal imaging (Fe@Au) was developed using iron and gold precursors. The morphology and composition of the hybrid contrast agent was evaluated using high resolution transmission electron microscopy (HR-TEM), energy dispersive analysis of x-rays (EDAX), x-ray diffraction (XRD) and x-ray photo-electron spectroscopy (XPS) and the results are presented in figure 1.
Figure 1. Characterization of Fe@Au Nps. Schematic illustration of Fe@Au (A); Transmission electron microscopy (TEM) images (B), Top inset: High magnification image of Fe@Au (scale bar represents 0.5 nm), Bottom inset: Lattice fringes of Fe@Au (scale bar represents 0.2 nm); Energy dispersive analysis of X-ray spectrum (C); Selected Area Electron Diffraction Pattern (D); X-ray diffraction (XRD) patterns (E); X-ray Photoelectron Spectrum (Survey Scan) of Fe@Au (F); elemental scans for Fe (a), Au (b) & O (c).
Download figure:
Standard image High-resolution imageIt is observed that the particles exhibit a spherical morphology with a narrow size distribution between 12-20 nm. The high magnification transmission electron micrographs reveal two distinct lattice fringes arising due to the iron oxide and gold phase (figure 1(B), bottom inset). The HRTEM also reveals that gold nanostructures are found on the surface of the iron oxide nanoparticles (figure 1(B)). The SAED pattern reveals the lattice planes (111), (200), (110), (220) and (311) corresponding to Fe and Au, and also at (205) that corresponds to Fe2O3 plane (figure 1(D)) [34]. The zeta potential values for the hybrid particles were found to be −16.7 ± 2.07 mV. The EDAX spectrum of the hybrid nanoparticles reveals the presence of iron and gold in the sample (figure 1(C)). The relative peak intensities of the two elements from the spectrum give an atomic percentage ratio of 1:3.75 (Au:Fe). The inductively coupled plasma-optical emission spectroscopy results also confirm the presence of the two elements iron and gold in the sample (data not shown). The concentrations of gold and iron were found to be 38.9 and 464.4 ppm respectively
The x-ray photoelectron spectrum for the hybrid imaging nanoparticle is shown in figure 1(F). The survey scan reveals the presence of iron, gold and oxygen in the sample. The elemental scan of the individual elements reveals their oxidation state and probable binding states. The binding energies for gold were recorded at 83.34 eV and 87.04 eV that are typical for elemental gold and gold nanoparticles respectively (figures 1(F) and (b)) [35]. The elemental scan for iron shows binding energy peaks at 710 eV and 725 eV that arise due to Fe2O3 while the satellite peaks appearing at 719 eV and 731 eV are characteristic of Fe3+ state (figures 1(F) and (a)) [36, 37]. The oxygen scan shows peaks corresponding to the binding energy 530.7 eV and 532.2 eV that are usually associated with oxides (figures 1(F) and (c)) [36, 37]. Figure 1(E) shows the XRD pattern of the hybrid imaging agent. The appearance of peaks at 2θ values of 38.18, 44.38, 64.57 and 77.59 correspond to the (111), (200), (220) and (311) planes respectively of Au (JCPDS 65-2870) confirming the presence of elemental gold in the hybrid sample [38]. The less intense peaks at 27.70, 32.48, 35.32 and 64.00 may be attributed to the (203), (205), (206) and (500) planes respectively of the Fe2O3 phase (JCPDS 25-1402). Interestingly, the peaks at 44 and 64.5 also correspond to the (110) and (200) planes of elemental iron (JCPDS 85-1410). Thus it is likely that the surface of the elemental iron at the core is oxidized to Fe2O3 that is further decorated by the gold nanoparticles. XPS being a surface technique was able to detect the iron oxide phase. Thus based on the characterization techniques, the structure of the iron-gold hybrid imaging agent (Fe–Au) is depicted in figure 1(A).
Chitosan nanoparticles were prepared by ionic gelation method and the morphology of the blank particles is shown in figure 2(A). Nearly monodisperse spherical particles between the sizes 15- 20 nm were observed. The zeta potential of the blank chitosan nanoparticles was found to be +28.3 ± 0.33 mV. The cationic amino groups confer the positive zeta potential which is in agreement with previous reports on chitosan. The EE of gemcitabine in chitosan was studied at different carrier:drug ratios and the results are presented in figure 2(C).
Figure 2. Scanning electron micrograph of blank chitosan nanoparticles (A) and Gemcitabine loaded chitosan nanoparticles (B); Encapsulation Efficiency of Gemcitabine loaded with chitosan nanoparticles at different chitosan: gemcitabine ratios (C). Values are expressed as mean+/-S.D. n=3. Transmission electron micrograph of CFe@Au Nps at 3:1(scale bar represents 10nm) (D) and CGFe@Au Nps at optimized condition 3:1:1(E) (scale bar represents 5nm). Top inset: High magnification image of Fe@Au Nps, Bottom inset: Lattice fringes. FTIR spectra (F), Differential scanning calorimetry profiles (G), thermal degradation curves (H) recorded for chitosan nanoparticles, gemcitabine loaded chitosan (CGNps), CFe@Au and CGFe@Au nanoparticles. Gemcitabine release profiles from drug loaded chitosan (CGNps) (I) and CGFe@Au Nps (J) at physiological conditions (pH 7.4), acidic conditions (pH 5.5), alkaline conditions (9.2) and in protein containing medium.
Download figure:
Standard image High-resolution imageIt is observed that the EE increased when the drug concentration was increased from carrier:drug ratio of 1:1 to 1:2. The EE did not significantly change when the carrier:drug ratio was changed to 1:3 or 2:1 and remained invariant at 75%. However, when the carrier concentrations were increased further to 3:1 carrier:drug ratio, a small but perceptible decrease was noted. This may be attributed to the hydrophobic character of the aromatic ring of gemcitabine that retards its distribution in the highly charged chitosan matrix as the carrier concentration is increased. As the carrier is intended to also co-encapsulate the hybrid imaging moiety, the carrier:drug ratio of 2:1 was chosen for further trials. The morphology of the drug-loaded carrier (2:1 carrier:drug ratio) presented in figure 2(B) shows the formation of spherical mono-disperse particles in the size range of 30–45 nm. The increase in size of the nanoparticles on drug encapsulation is due to the intercalation of the drug between the polymer chains.
The introduction of the hybrid imaging moiety in chitosan nanoparticles (CFe@Au) was optimized at different carrier:hybrid ratios. At ratios 1:1, 1:2 and 2:1 of the carrier and cargo, distorted structures were obtained whereas spherical nanoparticles were observed at carrier: hybrid ratios of 3:1 and 4:1 (supplementary figures S1(A) and (B) (available online at stacks.iop.org/BMM/16/055016/mmedia)). The 3:1 ratio exhibited higher entrapment efficiency of 58.75 ± 3.49% when compared with the 4:1 system (table 1) and hence was chosen for further trials. The size of CFe@Au nanoparticles was found to be 20 to 40 nm (figure 2(D)).
Table 1. Encapsulation efficiencies of gemcitabine and Fe@Au at various carrier drug to hybrid contrast agent ratios. Values are expressed as mean ± S.D. n = 3.
Carrier | Drug | Fe@Au | % Encapsulation of drug | %Entrapment of Fe@Au |
---|---|---|---|---|
3 | 0 | 1 | — | 58.75 ± 3.45 |
4 | 0 | 1 | — | 54.32 ± 2.10 |
3 | 1 | 1 | 91.20 ± 2.06 | 38.00 ± 3.20 |
3 | 2 | 1 | 96.24 ± 0.11 | 40.00 ± 3.20 |
The co-encapsulation of gemcitabine and the hybrid imaging moiety (CGFe@Au) was carried out at carrier:drug:hybrid ratios of 3:1:1 and 3:2:1. The incubation time was found to influence the EE with 31% gemcitabine encapsulated in the 3:1:1 ratio (figure 2(E)) after 2 h, which saturated to a maximum of 91.20 ± 2.06% after 24 h. The amount of the hybrid imaging moiety entrapped in chitosan at this ratio was 38%. Interestingly, the amount of gemcitabine encapsulated in the chitosan matrix in the presence of the hybrid imaging agent was significantly higher than when encapsulated independently. This can be attributed to the creation of voids due to the intercalation of the inorganic particles that facilitate greater encapsulation of the drug. When the drug ratio was increased to 3:2:1, the spherical structure was distorted though the EE of both drug and hybrid showed an increase (supplementary figure S1). When the carrier content was decreased to 2:1:1 ratio, the drug EE dropped to 68%. Therefore, based on the EE and morphology, the carrier: drug: hybrid ratio of 3:1:1 was chosen for further trials (table 1).
The morphology of the optimized carrier is shown in figure 2(E) and the size was found to range between 60 and 70 nm indicating that the co-encapsulation of the hybrid and drug increases the size. The zeta potential of the drug-loaded chitosan decreased to 20.6 ± 1.32 mV suggesting that the introduction of the drug disrupts the surface bound charges. Encapsulation of the hybrid in chitosan reduced the zeta potential from 28.3 ± 0.33 mV to 12.46 ± 0.32 mV (data not shown). This is due to the neutralization of the positive charges of chitosan polymer chains by the negatively charged hybrid. The co-encapsulation of gemcitabine and the hybrid in chitosan decreased the zeta potential further to 9.5 ± 0.63 mV due to a combination of the entrapment of negatively charged hybrid as well as disruption of the surface charges by gemcitabine.
The FTIR spectra recorded for the blank chitosan, free gemcitabine, gemcitabine-loaded chitosan, Fe@Au-loaded chitosan and the theranostic CGFe@Au are shown in figure 2(F). The blank chitosan shows C–H stretching vibrations at 2945 cm−1 that appears at 2955 cm−1 in the FTIR spectrum of drug-loaded chitosan, and at 2931 cm−1and 2948 cm−1 in the Fe@Au-loaded chitosan and CGFe@Au-encapsulated carrier respectively. The amide carbonyl stretch is observed at 1636 cm−1, 1634 cm−1, 1636 cm−1and 1637 cm−1 in the blank, gemcitabine-loaded, Fe@Au-loaded and CGFe@Au theranostic chitosan carrier respectively. The presence of gemcitabine in the carrier is indicated by the appearance of the aromatic ring bending vibrations at 891 cm−1 and 897 cm−1 in the gemcitabine-loaded carrier and CGFe@Au theranostic system respectively. The iron-oxygen bond vibration is discernible at 584 cm−1 and 587 cm−1 in the Fe@Au-loaded and CGFe@Au theranostic carriers.
The differential scanning calorimetry profile (figure 2(G)) of blank chitosan reveals a glass transition occurring at 113 °C while the melting point was recorded at 211 °C. The glass transition temperature exhibited a slight shift to 116 °C while the melting temperature displayed a negative shift to 204 °C upon loading with gemcitabine. This suggests that the intercalation of the drug molecules between the polymer chains disrupts the inter-chain associations thereby reducing the melting point. When chitosan was used for encapsulating Fe@Au, both the glass transition and melting temperatures of chitosan were positively shifted to 156 °C and 270 °C respectively. This indicates that the entrapment of the Fe@Au particles confers rigidity to the matrix there by restricting the polymer chain movement. The glass transition and melting temperatures of the CGFe@Au theranostic particles were further shifted to 171 °C and 276 °C respectively (figure 2(G)). This implies that the stiffening of the chitosan matrix by Fe@Au is augmented by the aromatic rings of the drug resulting in the positive shift in the glass transition and melting points of the polymer. The thermogravimetric profiles of blank chitosan, Fe@Au-loaded carrier and CGFe@Au shown in (figure 2(H)) reveal that the blank carrier exhibits negligible residue at 950 °C indicating the presence of organic groups that are completely degraded at higher temperatures. The drug-loaded carrier also leaves behind negligible residue indicating its organic nature. The degradation however starts at higher temperature (400 °C) indicating the presence of aromatic moieties that tend to degrade at higher temperatures when compared to the aliphatic moieties in the polymer. The Fe@Au-loaded carrier exhibits a residue of 32% and the CGFe@Au had a residue of 17% confirming the presence of inorganic content in these particles.
The XPS scan for blank chitosan shows peaks for carbon, oxygen and nitrogen (data not shown) which also appear in the survey scans for Fe@Au-loaded chitosan and CGFe@Au (supplementary figures S2(A) and (B)) confirming the presence of the organic polymer. In addition, peaks for iron and gold are found in the spectra of the Fe@Au-loaded and CGFe@Au confirming the encapsulation. The nitrogen elemental scan for CGFe@Au shows peaks corresponding to 399.41 eV and 401.74 eV (supplementary figure S2(B)) while the nitrogen from amine groups of chitosan gives peaks at the same binding energies. This is in accordance with earlier reports on chitosan nanoparticles [39].
4.2. Drug release from chitosan carriers
Figures 2(I) and 2(J) depict the release profiles of gemcitabine in different media from gemcitabine-loaded chitosan and CGFe@Au respectively. It is observed that in the gemcitabine-loaded carrier, the release of the drug is fastest at pH 5.5 where nearly complete release was observed within 5 h. The release at pH 7.4 was more sustained with about 60% of the drug released in the first hour as a burst due to the drug molecules located in the periphery of the chitosan matrix. Complete release was achieved over 19 h. The faster release in acidic pH is due to the higher swelling exhibited by the chitosan polymer resulting in voids that offer a low energy pathway for the diffusion of the drug [30, 40]. The release of gemcitabine at pH 9.2 was slower and complete release occurred over 32 h. The burst release of about 60% occurred in a little over 2 h. At alkaline pH, the –NH3 + groups of chitosan are deprotonated which can interact with the hydroxyl groups of the drug through hydrogen bonds that tend to retard the drug release [40, 41]. A similar release profile of gemcitabine was observed from the chitosan carrier in the presence of proteins at pH 7.4. Chitosan surface favors adsorption of proteins that blocks the paths for drug diffusion, which explains the slow release from chitosan in presence of proteins. Interestingly, the release of gemcitabine from CGFe@Au was found to be faster with the release at pH 5.5 completed within 2 h and the release at pH 7.4 and 9.2 completed in 9 h and 12 h respectively. In the presence of protein, the release occurred slowly over 16 h. This difference in release rates can be explained by the intercalation of Fe@Au between the polymer chains that creates voids and reduces the inter-chain interactions, which facilitates water-mediated diffusion of the drug molecules.
The release profiles were fit with different kinetic models to understand the most dominant mechanism(s) governing the drug release and the regression coefficients are tabulated in table 2 [42].
Table 2. Kinetic models for drug release gemcitabine-loaded chitosan and CGFe@Au.
Regression co-efficient (R2) | ||||||||
---|---|---|---|---|---|---|---|---|
Gemcitabine loaded chitosan | CGFe@Au | |||||||
Kinetic model | pH 7.4 | pH 5.5 | pH 9.2 | Protein | pH 7.4 | pH 5.5 | pH 9.2 | Protein |
Zero order | 0.8361 | 0.9416 | 0.8483 | 0.8642 | 0.8820 | 0.9266 | 0.8065 | 0.9098 |
First order | 0.9512 | 0.9970 | 0.9732 | 0.9608 | 0.9960 | 0.9999 | 0.9350 | 0.9824 |
Higuchi | 0.9446 | 0.9987 | 0.9568 | 0.9639 | 0.9716 | 0.9962 | 0.9234 | 0.9954 |
Korsmeyer–Peppas | 0.9971 | 1.0000 | 0.9907 | 0.9969 | 0.9874 | 0.9980 | 0.9897 | 0.9853 |
Hixson–Crowell | 0.9321 | 0.9859 | 0.9602 | 0.9482 | 0.9895 | 0.9972 | 0.9897 | 0.9770 |
Weibull | 0.9916 | 0.9975 | 0.9938 | 0.9912 | 0.9996 | 0.999 | 0.9957 | 0.9943 |
It is seen that the release profiles from gemcitabine-loaded chitosan in all release media are in agreement with the Weibull model that describes the release from a matrix-based system. The Korsmeyer–Peppas model that corresponds to release due to diffusion mechanism also correlates well with the release profiles in all conditions [41]. The release profile at pH 5.5 agrees well with the first order kinetics that generally describes the release of a water-soluble molecule from porous matrices. It is likely that the swelling of the polymer chains in acidic pH results in voids that govern the first order release. In addition, the release at pH 5.5 also correlates with the Higuchi diffusion model that describes the diffusion of a water-soluble molecule as well as the Hixson–Crowell kinetics that describes the release accompanied by surface erosion resulting in reduction in the size of the carrier [43]. It is evident that the acidic pH will also mediate the hydrolysis of the glycosidic linkages in the polymer resulting in a size reduction. When gemcitabine was co-encapsulated with the inorganic Fe@Au, the Weibull and Korsmeyer-Peppas kinetics correlate with the release in all conditions. In addition, the Hixson-Crowell model also correlates with the drug release in all conditions. This is due to the voids created by incorporation of the solid imaging agent that facilitate diffusion of water into the matrix which not only promotes drug diffusion but also the hydrolysis of the glycosidic chains resulting in dimension changes. For the same reason, the first order kinetics and Higuchi diffusion are in agreement with the release profiles from CGFe@Au at all conditions except at pH 9.2 where the hydrogen bonding between the drug and polymer chains continue to interfere with the drug release.
4.3. Functional characterization of the nanocarrier
The encapsulation of Fe@Au should enable both MRI as well as micro computed tomography (MicroCT) with better contrast enhancement. In addition, the chemotherapeutic agent should confer cytotoxicity to the cancer cells. For MRI contrast enhancement, the magnetic properties of Fe@Au before and after encapsulation in the chitosan carrier need to be characterized. Figure 3 depicts the magnetization curves obtained for unencapsulated and encapsulated Fe@Au along with the curve for CGFe@Au recorded at 300 K.
Figure 3. Magnetization curvesof Fe@Au (A), CFe@Au (B) and CGFe@Au (C). T2-Weighted magnetic resonance of Fe@Au, CFe@Au and CGFe@Au (D), Temperature change produced at different alternating magnetic fields for Fe@Au, CFe@Au and CGFe@Aunanoparticles (E), Transverse relaxivity obtained for different concentration of the theranostic carrier at 300K (F), Comparison of µCT contrast of different nanoparticles (Fe@Au, CFe@Au and CGFe@Au) dispersed in agar (G) and the variation of their signal intensity values with concentration (H), drug release from the theranostic carrier at pH 7.4 in presence and absence of alternating magnetic field (H)
Download figure:
Standard image High-resolution imageThe magnetic properties calculated from the magnetization profile for Fe@Au, CFe@Au and CGFe@Au are summarized in table 3.
Table 3. Magnetic properties of the nano-particles calculated from hysteresis loops.
Saturation magnetization (memu g−1) | Coercivity (G) | Relaxivity (M−1 s−1) | |||||||
---|---|---|---|---|---|---|---|---|---|
Sample | 300 K | 20 K | 100 K | 300 K | 20 K | 100 K | r2 | r1 | r2/r1 |
Fe@Au | 58.46 | 82.01 | 73.51 | 20.30 | 150.60 | 63.50 | 5.290 | 0.0480 | 110.28 |
CFe@Au | 7.72 | 23.79 | 15.13 | 1.29 | 72.88 | 9.34 | 2.189 | 0.0413 | 52.96 |
CGFe@Au | 7.10 | 22.09 | 13.09 | 0.65 | 71.19 | 8.92 | 2.647 | 0.2540 | 10.42 |
It is seen that the saturation magnetization is the highest for the unencapsulated Fe@Au while it reduces by nearly eight times when encapsulated in the chitosan matrix (table 3). This is due to the masking of the hybrid to the applied magnetic field as well as the restriction in orientation of the magnetic dipoles by the polymer chains. The gold decorated surface also partly contributes to the overall reduction in the saturation magnetization. Incorporation of the gemcitabine does not further alter this value significantly. The commercially used contrasts agent Ocean® was reported to exhibit r1 = 23.5 ± 0.9 and r2 = 93.8 ± 1.9 with a r2/r1 of 3.99 while FeREXTM® had been reported to show r1 = 33.1 ± 1.3 and r2 = 160.1 ± 6.6 with a r2/r1 of 4.84 [44]. The r2/r1 values of CGFe@Au are over two times higher than the commercially available agents and hence could be employed for imaging applications. A similar trend is observed in the coercivity values that saw a reduction at 300 K from 20.30 G for the unencapsulated Fe@Au to 1.29 G for CFe@Au. A further slight reduction to 0.65 G was recorded for CGFe@Au. As coercivity represents the resistance to demagnetization, lower values indicate soft magnetic materials that get demagnetized quickly on removal of the external magnetic field [45]. Lower coercivity values auger well for biomedical applications where remnant hysteresis may cause undesirable effects [46].
Figures 3(A), 3(B) & 3(C) show the saturation magnetization values recorded at different applied radio frequencies in an alternating magnetic field. It is seen that the unencapsulated Fe@Au exhibits an increase in the temperature with increasing applied magnetic field. This is in conformity with earlier reports on iron oxide nanoparticles where the increase in temperature has been attributed to the Neel relaxation of the magnetic nanoparticles. The encapsulation of Fe@Au in chitosan results in a reduction in the heating rate due to the masking of the particles to the applied frequency by the polymer chains. The co-encapsulation of Fe@Au with gemcitabine further reduces the heating rate as the organic content in this system is very high. It is seen from the figure 3(E) that at 480 kHz, a 10 °C increase in temperature was brought about by 3 mg ml−1 Fe@Au in 17 s while it takes 165 s and 724 s to achieve the same temperature increase in the CFe@Au and CGFe@Au respectively. Hyperthermia can lead to cytotoxicity between the temperature ranges of 42 °C to 46 °C beyond which thermal ablation can occur that will damage normal tissues. Moreover, the high Curie temperatures associated with iron (1043 K), Fe2O3 (948 K) and Fe3O4 (858 K) make it difficult to regulate the homogenous distribution of heat within the tumor tissue resulting in necrosis of the surrounding healthy tissues and hence are impractical for clinical hyperthermia. Further, low magnetization values such as those recorded for the encapsulated particles in this study, necessitate use of higher applied field or high concentrations of the nanoparticles, both of which can lead to collateral damage to normal tissues. Therefore, use of this agent to bring about hyperthermia-mediated cell death in cancer tissue may not be a practical application. However, the magnetocaloric effect of CGFe@Au can be utilized to bring about temperature-induced fluidization of the polymer chains to accelerate drug release. Figure 3(I) compares the release of gemcitabine from CGFe@Au after 15 min in the absence or presence of an alternating magnetic field. It is observed that 67% drug release occurred within 15 min when the system was subjected to hyperthermia when compared to 7% in the absence of the magnetocaloric effect. The temperature-induced accelerated drug release can result in better cytotoxicity against cancer cells.
The ability of Fe@Au to enhance the contrast in MRI was investigated using a phantom agar assay. As iron and its oxides are negative contrast agents and therefore alter the spin-spin relaxation, the T2 weighted images and corresponding relaxivity rate (r2) was obtained and are presented in figure 3(F). A dose-dependent reduction in the signal intensity is observed for the unencapsulated, encapsulated and co-encapsulated Fe@Au (figure 3(D), table 3). The reduction in signal intensity is higher for the unencapsulated Fe@Au when compared to its encapsulated counterparts. This is on expected lines as the chitosan chains tend to shield Fe@Au from the applied magnetic field and hence a reduction in the magnitude of negative contrast is observed. However, CGFe@Au shows a better negative contrast than CFe@Au. This can be attributed to the greater amount of water molecules occupying the voids created by the co-encapsulation of Fe@Au and gemcitabine. The water in the chitosan matrix has better proximity to the contrast agent as well as has restricted rotation due to its confinement within the polymer chains thereby resulting in better contrast according to the Solomon–Bloembergen–Morgan theory. This is also reflected in the relaxivity rates (r2 and r1) tabulated in table 3.
MicroCT is a non-invasive, high resolution imaging technique that was traditionally employed for bone imaging but in recent years, has garnered considerable interest towards soft tissue imaging. The ability of the hybrid to enhance the contrast in microCT was evaluated using an agar gel matrix embedded with different concentrations of the free, encapsulated and co-encapsulated hybrid imaging agent (figure 3(H)). Gold nanoparticles are excellent microCT contrast agents and have been reported to possess better x-ray attenuation property than iodinated compounds. The gold decorated iron oxide hybrid nanoparticles exhibit a concentration-dependent enhancement in the x-ray attenuation as observed from the intensity of the particles dispersed in agar gel (figure 3(G)) as well as in the linearity in the plot of x-ray attenuation in Hounsfield units (HU) versus concentration of the contrast agent (figure 3(H)). A similar trend is observed for the encapsulated and co-encapsulated Fe@Au. However, the magnitude of attenuation is maximum for the unencapsulated Fe@Au at all concentrations followed by the CFe@Au and CGFe@Au. This reduction maybe due to the masking of Fe@Au by the polymer chains. Interestingly, at lower concentrations (5 mg ml−1), the reduction in the magnitude of attenuation in CGFe@Au when compared to the unencapsulated Fe@Au was 86%. But, at the highest concentration (75 mg ml−1), the decrease in the magnitude of attenuation in CGFe@Au was 17% in comparison to the unencapsulated hybrid. Multiple factors can influence the x-ray attenuation in micro-CT. These include the thickness and the x-ray attenuation coefficient that varies for different materials. The higher effective cross-sectional area exposed to the x-rays in the case of free Fe@Au when compared with the aggregated Fe@Au in the encapsulated form may be a major contributor to the observed differences in the attenuation magnitude[47].
The ability of the nanoparticles to be visualized by microCT in in vivo conditions was performed in immune competent BalB/c mice. Micro-CT images acquired from animals 1.45 h after a tail vein injection with 1.25 mg ml−1 of either unencapsulated Fe@Au or CGFe@Au are shown in (supplementary figure S3). Both the free and encapsulated Fe@Au are visible in the animal indicating that the gold decorated particles can be used for imaging tissues. It is observed that the intensity of the free Fe@Au is higher than the co-encapsulated carrier which is on expected lines as the Fe@Au will be masked by the polymer chains and drug molecules in agreement with the results obtained in the in vivo phantom agar assays. Another interesting phenomenon that is discernible from the images is that the encapsulation of the chitosan carrier alters the distribution and localization of the Fe@Au. The unencapsulated Fe@Au shows greater accumulation in the right lobe of the liver and parts of the intestine while the chitosan carrier redirects the contrast agent to the left lobe of the liver and lower intestines. Recent research has revealed that the different lobes of the liver have heterogeneous expression of enzymes that can indicate differential response to molecules and xenobiotics. Several studies have indicated higher levels of asymmetric dimethylarginine, cytochromes CYP1A, CYP2C, CYP2D and cytochrome p450 reductase, an enzyme involved in electron transport, in the right lobe of liver. Cytochrome p450 reductase has been implicated in xenobiotic transport as well as in enhancing the expression of heme oxygenase, an enzyme that is activated in response to oxidative stress and catalyzes the transformation of heme to biliverdin. Though investigations on the differential expression of proteins in the liver lobes are under active investigation, their implications are yet to be thoroughly deciphered. The results from the present study show that the free Fe@Au accumulates in the right lobe of the liver due to the iron oxide that resembles the iron core in heme resulting in its sequestration in the right lobe of the liver that is rich in cytochrome p450 reductase and heme oxygenase. When the imaging agent is encapsulated in chitosan, it is masked by the polymer chains and hence is sequestered in the left lobe of the liver where the microsomal enzymes will act on the carrier. The CGFe@Au system also displays a distinct accumulation in the caecum region of the gastro-intestinal tract unlike its unencapsulated counterpart, which is distributed in the ileum and duodenum. This may be due to differences in the pH of the gastro-intestinal tract where the caecum displays greater acidic nature than the ileal and duodenal compartments [48]. It is also evident from the microCT images that the non-targeted Fe@Au both in the unencapsulated and encapsulated form displays a hepato-biliary mode of elimination. Similar accumulation has been reported for ormosil-based organic-inorganic hybrid imaging agent.
4.4. In vitro cytotoxicity evaluation
Cytotoxicity evaluation of CGFe@Au was carried out in two pancreatic cell lines—PanC1 (figure 4) and MiaPaCa2 (Data not shown). Three concentrations of gemcitabine namely 5 μM, 50 μM and 100 μM were chosen for the study based on the cell viabilities obtained during preliminary studies with cultures of PanC1. The IC50 for free drug was determined to be 1489 μM and that for gemcitabine-loaded chitosan was found to be 68 μM indicating a 22-fold decrease in the IC50 upon encapsulation (supplementary figure S4). Cell viability was expressed relative to untreated cells. It is observed that the PanC1 cell line treated with free gemcitabine does not exhibit significant reduction in cell viability at any time point. This may be attributed to activation of molecular mechanisms limiting the cellular uptake of the drug leading to drug resistance while in case of drug-loaded nanoparticles (CGNps) and CGFe@Au-treated cells, a progressive decrease in cell viability was observed when compared to their unencapsulated counterparts which can be attributed to the interaction of positively charged chitosan nanoparticles with the cell membrane improving cell internalization. The encapsulated drug is released into the cells, which disrupts the proliferation and survival signaling network. The free Fe@Au did show a significant reduction in the PanC1 while CFe@Au exhibited a slight improvement in the cytotoxicity. This may be due to the enhanced carrier-mediated internalization that enables the manifestation of anticancer properties of Fe@Au through growth inhibition and mitochondria-mediated autophagy in the cancer cells. Toxicity of iron-gold nanoparticles was demonstrated earlier in colorectal cancer by Ya-Na Wu et al [49].
Figure 4. In vitro cytotoxicity studies determined by MTS assay in PanC-1 cells. Statistical significance was tested between days (* = p < 0.001), n = 3.
Download figure:
Standard image High-resolution image4.5. Effect of the co-encapsulated carrier on the viability of normal cells
Figure 5 shows the effect of CGFe@Au over a wide range of concentrations from 1 nM to 1 mM on H9c2 cells, a myoblast cell line commonly employed for drug cytotoxicity studies. No significant reduction in the cell viability was observed even at 1 mM concentration after both 24 h and 48 h of treatment. The cell viability remained between 90% – 110% at all concentrations indicating that the chitosan formulation is not toxic to normal cells. Most normal cells differ from cancer cells in their membrane composition which consequently influences the permeability characteristics. Generally, cancer cells are more fluid and permeable than normal cells and this can affect their ability to internalize nanoparticles, which could partly account for the difference in response of normal and cancer cells towards CGFe@Au.
Figure 5. In-vitro cytotoxicity assay of CGFe@Au nanoparticles on normal cells (H9c2). Values are expressed as mean ± S.D. (n = 3).
Download figure:
Standard image High-resolution imageThe difference in internalization of the nanocariier observed between normal and cancer cells bodes well for pancreatic cancer therapy. Apart from the preferential accumulation in the cancer cells, the CGFe@Au nanoparticles containing Fe that confers magnetic responsive nature to the nanocarrier can be magnetically guided to the cancer tissue by application of an external magnetic field. The leaky vasculature present in cancer tissue also facilitates better accumulation of the nanocarrier through the enhanced permeation and retention (EPR) effect. Upon reaching the cancer tissue, the cationic chitosan carrier will facilitate better interaction with the negatively charged permeable cancer cell membrane resulting in superior internalization. The therapeutic agent is then released, either via enzyme activity, or through changes in physiological conditions, leading to better therapeutic effects against the cancer cells. Our results also suggest that the toxicity and undesirable accumulation of the nanoparticles can be avoided by tuning the particle shape, size and surface chemistry of nanoparticles, which also influence their biodistribution patterns and clearance mechanisms. Our results indicate that the chitosan nanocarrier exhibits greater cytotoxicity towards cancer cells when compared to normal tissues owing to superior internalization in cancer cells. Further, the enhanced permeation and retention properties exhibited by the cancer tissue can also facilitate better accumulation of the nanoparticles in the cancer cells thereby minimizing adverse effects to normal tissues. Chitosan is biodegradable and Fe@Au did not exhibit cytotoxicity suggesting that the hepato-biliary mode of elimination of the CGFe@Au observed from the micro-CT experiments may not cause undesirable side effects.
5. Concluding remarks
The present study reports the successful synthesis and characterization of gold-coated iron nanoparticles (Fe@Au) and its successful encapsulation in chitosan nanoparticles along with gemcitabine. This multifunctional nanoparticle allows effective T2-weighted MRI imaging and excellent CT imaging contrast. The multi-modal imaging provides better spatio-temporal information of the tumour tissue.The combined effect of co-encapsulated gemcitabine and dual modal imaging hybrid (CGFe@Au) nanotheranostic system exhibited sustained drug release and higher toxicity against PanC1 cells when compared to free gemcitabine. We also show that the nanoparticles are capable of inducing magnetocaloric effect that augmented rate of drug release. In addition, the CGFe@Au nanoparticles exhibited no obvious toxicity to normal cells. Our study reveal that image guided synergetic therapy can be achieved along with a better activity of gemcitabine drug when co-encapsulated in chitosan nanocarrier. Therefore, the nanotheranostic (CGFe@Au) system based on this combination could be further explored for treatment of pancreatic cancer.
Acknowledgments
The authors wish to thank Nano Mission, Department of Science & Technology (SR/NM/NS-1095/2012), and FIST, Department of Science & Technology (SR/FST/LSI-622/2014 & SR/FST/LSI-058/2010) for funding. The authors also acknowledge SASTRA Deemed University for financial & infrastructural support.
Data availability statement
All data that support the findings of this study are included within the article (and any supplementary files).