ABSTRACT
Molecular oxygen (O2) and carbon dioxide (CO2) ices were irradiated with energetic D+2 ions to simulate the exposure of oxygen-bearing solar system ices to magnetospheric H+2 and H+ ions and energetic protons from the solar wind. The experiments provided evidence on the incorporation of the implanted deuterium and inherent formation of D2-water (D2O) as well as D2-carbonic acid (D2CO3). In the molecular oxygen ices, the temporal profiles inferred that D2-water formation followed successive deuterium atom addition to atomic oxygen via a D-hydroxyl radical intermediate in the matrix. In the carbon dioxide ices, D2-carbonic acid was likely formed via successive deuterium atom reaction with cyclic carbon trioxide. These chemical processes have specific relevance to water formation on outer solar system bodies, such as the icy moons of Jupiter and Saturn, as well as possible implications for the formation of water on the lunar surface.
Export citation and abstract BibTeX RIS
1. INTRODUCTION
Ices of varying molecular compositions have been observed on the surfaces of satellite bodies surrounding the outer planets of the solar system as well as on the surfaces of Kuiper Belt objects. Condensed water (H2O) is the most abundant species pertaining to these ices, having been detected on satellites of Jupiter, Saturn, Uranus, and Neptune, and as a primary component of cometary matter (Hudson & Moore 2001). Even though water is the dominant component of these ices, molecular species such as oxygen (O2) and carbon dioxide (CO2) have also been detected. Solid carbon dioxide is harbored on the surfaces of the Galilean satellites Europa, Callisto, and Ganymede (Showman & Malhotra 1999; Cooper et al. 2001) and on the Saturnian moons Enceladus, Dione, Hyperion, Iapetus, and Phoebe (Brown et al. 2006; Cruikshank et al. 2010). The more elusive oxygen molecule has been identified on the Jovian moons Callisto (Spencer & Calvin 2002) and Ganymede (Spencer et al. 1995). The species is detected by its 577 nm visible absorption band (Calvin & Spencer 1997), which is active due to an interacting pair of oxygen molecules. This infers that condensed-phase molecular oxygen is stable at the 90–150 K surface temperatures of these bodies and are most likely accumulated in pores, defects, and ion tracks within the icy matrix (Johnson & Jesser 1997). Here, molecular oxygen is a product of the continual irradiation of water ice on these surfaces (Spencer & Calvin 2002; Zheng et al. 2006a, 2006b; Cooper et al. 2008). As molecular oxygen displays weak absorption in the infrared region, the presence of the species can be inferred by the detection of ozone (O3) in such environments (Kasting & Catling 2003). As ozone is a by-product of the radiolytic processing of oxygen, the identification of ozone on the icy surfaces of Ganymede (Noll et al. 1996), Rhea, and Dione (Noll et al. 1997) indicates that molecular oxygen is present in sufficient abundances to act as a precursor for ozone formation on these satellite bodies.
These solar system environments are continually exposed to an array of irradiation sources such as galactic cosmic rays (GCRs; 87% H+, 12% He2+, and electrons) and solar wind particles (photons, electrons, and ions; 96% H+, 4% He2+, and a few heavier nuclei; Johnson 1990). Orbiting bodies of the Jovian and Saturnian systems are additionally exposed to heavy ions of energies of a few keV per amu, energetic neutrals and electrons, all trapped in the extended magnetospheres of these planets. In the context of the present experiments, we are particularly interested in the reactions propagated by hydrogen atoms (H) in these surface environments. For instance, H atoms can be implanted in oxygen-bearing ices by collisions with plasma neutrals (H, OH, H2O) and ions (H+, OH+, H2O+, H3O+) trapped in Saturn's magnetosphere. The abundance of these species in Saturn's magnetosphere is replenished by the water ejecta from Enceladus (Young et al. 2005). The stopping of energetic irradiation by the solid surface can create cascades of knock-on particles consisting of the atoms of the target material, i.e., mostly hydrogen, oxygen, nitrogen, and carbon atoms.
Exposure of pure oxygen ices to 100 keV protons (H+) (Baragiola et al. 1999; Fama et al. 2002) identified the formation of ozone via radiolytic energy transfer. However, water was not detected as the ice thickness was insufficient to bring the protons to rest in the sample. Miyauchi et al. (2008) investigated oxygen ice exposed to hydrogen atoms sourced from a microwave-induced plasma (70 K: ∼ 6 × 10−3 eV) to detect the formation of water and hydrogen peroxide (H2O2) in the solid state. The study found that water was primarily produced via the hydrogen peroxide intermediate at the vacuum–surface interface, as the low-energy hydrogen atoms displayed limited penetration into the oxygen surface. Subsequent studies involving "cold" hydrogen atom exposure to molecular oxygen films (∼15 monolayers; Cuppen et al. 2010; Ioppolo et al. 2010) also showed that water could be formed through a hydrogen peroxide intermediate, and that the diffusion of the hydrogen atoms through the surface is dependent on the temperature of the ice. However, reaction pathways leading to water formation in proton-irradiated low-temperature solids do not necessarily require a hydrogen peroxide intermediate for completion. Hiraoka et al. (1998) showed that water can be produced by irradiating a 12 K, plasma activated, nitrous oxide (N2O) matrix with hydrogen atoms sourced from a discharge tube. With oxygen atoms liberated within the sample matrix, a reaction mechanism involving the successive hydrogenation of atomic oxygen was proposed to proceed without activation barriers. Kinetic analysis monitoring the increase in water abundance over the irradiation period determined that this reaction pathway was highly efficient. In addition to these studies, irradiation of 9 K water–oxygen mixtures with 0.8 MeV H+ (Cooper et al. 2006, 2008) has uncovered stabilized radical species such as HO2 and HO3, as well as molecular hydrogen peroxide (H2O2).
Switching attention to carbon dioxide condensates, the irradiation of water and carbon dioxide ice mixtures with energetic ions, electrons, and UV photons has been performed to investigate the processes of carbonic acid (H2CO3) production, which is stable in low-temperature matrices (Moore & Khanna 1991; DelloRusso et al. 1993; Gerakines et al. 2000; Wu et al. 2003; Zheng & Kaiser 2007). The possibility of carbonic acid residing in solar system and interstellar ices containing carbon dioxide has important biological implications, particularly as a potential reactant for higher-order molecules such as oxalic acid. Pristine carbon dioxide ices have also been observed to form carbonic acid through keV proton implantation (Brucato et al. 1997; Garozzo et al. 2008), although in smaller yields compared to the irradiated mixtures containing water. In addition, studies involving energetic keV electron bombardment of pure carbon dioxide ices have reported a host of irradiation products, including carbon monoxide (CO), cyclic carbon trioxide (c-CO3), ozone (O3) (Bennett et al. 2004), dicarbon monoxide (CCO), and carbon suboxide (C3O2) (Bennett et al. 2010). These experiments featured detailed kinetic analyses which untangled the formation routes for each species at 10 K. Further, the processes leading to cyclic, oxygen-rich species carbon tetraoxide (CO4; Jamieson et al. 2007a), carbon pentaoxide (CO5; Jamieson et al. 2007b), and carbon hexaoxide (CO6; Jamieson et al. 2008) were also proposed from electron-irradiated carbon dioxide condensates.
In the present study, we simulate the interaction of molecular oxygen and carbon dioxide ices exposed to energetic H+2 ions. For example, in the case of Saturn's outer magnetosphere, molecular hydrogen is released from Titan's exosphere where it is ionized by energetic particle interaction to form H+2 (Cui et al. 2008; Sittler et al. 2010). To increase our ability to detect these species, which are likely to be formed in very low abundances, we will irradiate our samples with deuterated molecular hydrogen ions (D+2). Therefore, products such as D2-water will be produced in amounts sufficient to be detected above the molecular density of contaminant species in our experimental chamber, which is evacuated to ultrahigh vacuum (UHV; 2 × 105 molecules cm−3). Here, we use online Fourier transform infrared spectroscopy (FTIR) to analyze the solid state and quadrupole mass spectrometry (QMS) to detect the gas-phase species released during irradiation or the subsequent annealing period of the condensate. The application of both techniques will allow us to not only qualitatively detect species within the ice matrix as they are formed, but also perform quantitative studies, including the elucidation of reaction mechanisms based on kinetic schemes. This approach has been firmly established in our group over the last few years to extract the formation pathways of astrobiologically important molecules such as glycolaldehyde (Bennett & Kaiser 2007) and glycine (Holtom et al. 2005). In the present study, we hope to provide new evidence pertaining to water and carbonic acid production routes in oxygen-bearing ices exposed to energetic irradiation.
2. EXPERIMENTAL DETAILS
The irradiation of oxygen and carbon dioxide condensates with D+2 was performed in a stainless steel chamber which was evacuated to UHV conditions ((8.5 ± 0.5) × 10−11 torr). Within the chamber, a 31.75 × 31.75 mm2 silver mono-crystal substrate was attached to the cold head of a closed-cycle helium refrigerator (CTI-Cryogenics CP-1020). Measured by a Lakeshore DT-470 silicon diode sensor, the substrate reached a temperature of 11.0 ± 0.5 K which was maintained throughout the irradiation period. BOC Gases Grade 5.0 oxygen and carbon dioxide were deposited in separate onto the cold substrate via a glass capillary array. Here, a base pressure of 1.0 × 10−8 torr was used during the condensation to deposit the gases over 20 minutes to produce ice samples of appropriate thicknesses. In addition, the deposition gases were monitored for contaminants using a Balzer QMG 420 QMS operating in residual gas analyzer mode (electron impact energy of 100 eV at a 0.3 mA emission current). Once solidified, a Nicolet 6700 FTIR was used to record a spectrum of the 6000–500 cm−1 mid-infrared (mid-IR) region for thickness verification. Each spectrum (resolution 2 cm−1) is compiled from 196 individual scans collected over 2.0 minutes. The oxygen and carbon dioxide ice thicknesses could then be determined using their infrared spectra absorption bands (Tables 1 and 2) by procedures outlined in Bennett & Kaiser (2005) for pure oxygen ice and Bennett et al. (2004) for pure carbon dioxide ice, which yielded an oxygen condensate of 2.0 ± 0.6 μm thickness and a carbon dioxide condensate of 0.37 ± 0.12 μm thickness.
Table 1. Absorption Band Positions and Assignments from Figure 1
O2 Ice | After Irradiation | 140 K | Assignmenta | Characterization |
---|---|---|---|---|
(cm−1) | at 12 K (cm−1) | (cm−1) | ||
2230–2702 | ν1/ν3(D2O) b | DO stretching | ||
2106 | ν1 + ν3(O3) | Combination band | ||
1740 | ... | ... | ||
1702 | ... | ... | ||
1620 | νL + ν2 (D2O) | Combination band | ||
1612 | νL(O2) | Lattice mode | ||
1550 | ν1(O2) | OO stretching | ||
1302 | 1302 | ν2(D2O)b | DOD bending | |
1105 | ν1(O3) | Symmetric stretch | ||
1036 | ν3(O3) | Asymmetric stretch | ||
702 | ν2(O3) | Bending |
Notes. aBrewer & Wang (1972). bZheng et al. (2007a).
Download table as: ASCIITypeset image
Table 2. Absorption Band Positions and Assignments from Figure 2
CO2 Ice | After Irradiation | 120 K [200 K] | Assignmenta | Characterization |
---|---|---|---|---|
(cm−1) | at 12 K (cm−1) | (cm−1) | ||
3709 | ν1 + ν3(CO2) | Combination band | ||
3600 | 2ν2 + ν3(CO2) | Combination band | ||
2730–2300 | ν(D2O)b | D–O stretching | ||
2961 | D–O stretching | |||
[2262–2110] | ν(D2CO3)b | D–O stretching | ||
2414 | νL(CO2) | Lattice mode | ||
2339 | 2345 | ν3(CO2) | Asymmetric stretch | |
2284 | ν3(13CO2) | Isotope peak | ||
2142 | ν1(CO) | Stretching | ||
2092 | ν1(13CO) | Isotope peak | ||
2045 | ν1(CO3) | Stretching | ||
1876 | Fermi(CO3) | Fermi resonance | ||
1709 [1720] | ν(D2CO3)b | C=O stretching | ||
1433 [1486] | ν(D2CO3)b | C–OD asymmetric stretch | ||
1385 | Fermi(CO2) | Fermi resonance | ||
1330 | δ(D2CO3) b | C–OD in-plane bend | ||
1040 | ν3(O3) | Asymmetric stretch | ||
1025 | ν(D2CO3)b | C–OD symmetric stretch | ||
819 | δ(D2CO3)b | CO3 out-of-plane bend | ||
654, 659 | 674 | ν2(CO2) | In-plane/out-of-plane bend | |
638 | ν2(13CO2) | Isotope peak |
Notes. aGerakines et al. (1995). bHage et al. (1996).
Download table as: ASCIITypeset image
The ices were then irradiated isothermally at 11 K by 5 keV molecular deuterium ions (D+2). The ions were produced by feeding 5.0 × 10−4 torr of high-purity molecular deuterium gas (D2: 99.8%; Icon Isotopes) into an ion source (SPECS IQE 12/38). After removal of D+ and D3+ particles from the beam by a Wien mass filter (0.12 T), a monoenergetic 5 keV beam of D+2 was focused onto the target. Here, the total D+2 current was measured to be 840 ± 200 nA, irradiating a cross-sectional area of 0.50 ± 0.06 cm2. Exposure periods for the oxygen (2 hr) and carbon dioxide (10 hr) ices were dependent on the time necessary to reach saturation of the product abundances detected by online FTIR analysis. Once product peaks in the FTIR were observed to stabilize, the ion source was switched off and the irradiated ice was left to stand over a 30 minute period. The ice samples were then heated to 300 K (at a rate of 0.5 K minute−1) using the temperature control unit (Lakeshore 331). This enabled the identification of all volatile species in the irradiated ice composition at their specific sublimation points under UHV conditions.
Within their detailed overview of molecular ion irradiation processes, Sigmund et al. (1996) summarize that "implant profiles of keV diatomic molecular ions are, by and large, equivalent with those of monoatomic ion beams at twice the fluence and half the energy." There are, as the authors also acknowledge, considerable limitations to this approximation (Arista 2000). However, as nonlinear effects are observed to increase with molecular ion mass, it can be reasonably approximated that for small 5 keV D+2 ions impinging on a solid target, the molecular ion is dissociated upon impact forming a D+ ion and a D atom, each possessing 2.5 keV of translational energy. Therefore, the maximum penetration depth (Table 3) for the ions was approximated by calculating the projected range of 2.5 keV D+ ions in the sample ices using the SRIM Monte Carlo program (Ziegler et al. 1985). For oxygen ice of density 1.54 g cm−3, the stopping range for D+ was calculated to be 79 ± 4 nm, while for carbon dioxide ice of density 1.70 g cm−3, a maximum penetration depth of 67 ± 3 nm was calculated. In both cases, the maximum stopping ranges were less than the thickness of the ices. This infers that the kinetic energy of the impinging ion is completely transferred to the sample. It follows that the average energy supplied to each molecule in the ice sample over the D+2 irradiation period, or the average dose, is calculated to be (1.4 ± 0.3) × 103 eV molecule−1 for the oxygen ice and (13.6 ± 2.7) × 103 eV molecule−1 for the carbon dioxide ice. As the strength of the diatomic bond in molecular oxygen is 5.1 eV and the strength of a carbon–oxygen double bond in carbon dioxide is 8.1 eV, the magnitude of energy absorbed by the individual molecules of the sample ices can initiate bond-breaking processes.
Table 3. Experimental Irradiation Parameters
Ice | Density | Maximum Penetration | Exposure | Energy | Dosage |
---|---|---|---|---|---|
(g cm−3) | Depth (nm) | Time (s) | (× 1020 eV) | (× 103 eV molecule−1) | |
O2 | 1.545 | 79 ± 4 | 7,200 | 1.6 ± 0.1 | 1.4 ± 0.3 |
CO2 | 1.702 | 67 ± 3 | 36,000 | 10.7 ± 0.3 | 13.6 ± 2.7 |
Download table as: ASCIITypeset image
3. RESULTS
3.1. Qualitative Analysis
3.1.1. Infrared Spectroscopy
Figure 1(a) shows the 3000–500 cm−1 (3.3–20.0 μm) mid-IR spectrum obtained from the pure oxygen condensate and the spectrum recorded immediately after 10 hr of exposure to D+2 at 11 K. Band positions and assignments are compiled in Table 1. Here, the 1550 cm−1 (6.5 μm) absorption band assigned to the molecular oxygen ν1(O2) stretching mode, which becomes IR active in an amorphous solid composition (Freiman & Jodl 2004), is observed both before and after irradiation. This band, in addition to the broad absorption feature centered at 1612 cm−1 (6.2 μm) and assigned to the νL(O2) lattice mode, indicates the sample is predominantly amorphous oxygen ice interspersed with regions of crystalline material. The irradiation of the sample with D+2 produces molecular ozone (O3 X1A1). The positions of three fundamental bands are used for the assignment of ozone; the intense ν3(O3) asymmetric stretching band at 1036 cm−1 (9.7 μm), the ν1(O3) symmetric stretching band at 1105 cm−1 (9.0 μm), and the ν2(O3) bending vibration at 702 cm−1 (14.2 μm), which correlate well with previous studies (Brewer & Wang 1972). In addition, the ν1+ν3(O3) combination band at 2106 cm−1 (4.7 μm) is also clearly identified. These absorption features are observed to disappear during the controlled heating of the condensate, with molecular oxygen subliming at ∼35 K and ozone subliming at ∼60 K.
Figure 1. Mid-IR spectra of (a) pristine molecular oxygen ice surface and after D+2 irradiation at 11 K and (b) D2-water remaining between 140 and 200 K. Absorption band positions and assignments are presented in Table 1.
Download figure:
Standard image High-resolution imageDepicted in Figure 1(b) is the mid-IR spectra recorded at 140, 170, and 200 K during the annealing period of the oxygen ice following irradiation. Here, polar ice mixtures remain on the substrate due to their higher sublimation temperatures (i.e., Tsub(D2O) ∼ 180 K). The broad feature between 2702 cm−1 (3.7 μm) and 2230 cm−1 (4.5 μm) is assigned to the diffuse absorption patterns of ν3(D2O) asymmetric and ν1(D2O) symmetric vibrations in an amorphous composition (Zheng et al. 2007a). D2-water can be further characterized by the weak absorption band at 1620 cm−1 (6.2 μm) being assigned to the νL+ν2(D2O) combination band. The ν2(D2O) bending mode is expected to result in an absorption band at ∼1200 cm−1 (8.3 μm); however, we can only tentatively assign this vibration mode to a diffuse absorption feature centered at 1302 cm−1 (7.7 μm). Absorption features at 1702 cm−1 (5.9 μm) and 1740 cm−1 (5.7 μm) remain unassigned after a thorough search of all modes associated with deuterium oxides (e.g., D2O2 or D2O3). Radical species such as DO, DO2, and DO3 can be ruled out as they are not stable in the condensed phase at these temperatures.
We now consider Figure 2(a) which displays the 4000–500 cm−1 (2.5–20.0 μm) mid-IR spectrum for the pristine carbon dioxide ice at 11 K and the spectrum obtained after 2 hr of D+2 irradiation. Band positions and assignments are compiled in Table 2. The linear, ground-state carbon dioxide molecule (CO2 X1Σg+) is assigned ν2(CO2) in- and out-of-plane bending fundamentals at 654 cm−1 (15.3 μm) and 659 cm−1 (15.2 μm), and its intense ν3(CO2) asymmetric stretching band at 2339 cm−1 (4.3 μm), which are in agreement with the literature values (Gerakines et al. 1995). In addition, the 2ν2+ν3(CO2) and ν1+ν3(CO2) combination bands are located at 3600 cm−1 (2.8 μm) and 3709 cm−1 (2.7 μm), respectively, while the prominent lattice mode ν3+νL(CO2) absorption feature is identified at 2414 cm−1 (4.1 μm). The absorption bands located at 638 cm−1 (15.7 μm) and 2284 cm−1 (4.4 μm) can be assigned to the ν2(13CO2) and ν3(13CO2) modes of the 13CO2 isotopic analog. Finally, the feature at 1385 cm−1 (7.2 μm) can be assigned to a Fermi-resonance mode of the carbon dioxide molecule. Exposure to fast D+2 ions produces a number of new absorption features which are displayed in the spectrum collected after irradiation. Carbon monoxide is identified by its intense ν1(CO) stretching fundamental positioned at 2142 cm−1 (4.7 μm) and the redshifted ν1(13CO) mode of its isotopic analog at 2092 cm−1 (4.8 μm) (Gerakines et al. 1995). Formation of cyclic carbon trioxide (c-CO3) is confirmed by its 2045 cm−1 (4.9 μm) ν1(C=O) stretching fundamental and its Fermi-resonance band at 1876 cm−1 (5.3 μm), which is induced by vibrational mixing of the ν1(C=O) mode and the weak absorption of the ν5(C–O) stretching mode (not identified at ∼970 cm−1 (10.3 μm)). The Fermi-resonance band supports the product carbon trioxide as the cyclic structural isomer, as the absorption mode can be activated in the symmetric C2v molecule (Bennett et al. 2004). In addition, ozone was also observed to be formed via the assignment of its ν3(O3) asymmetric stretching vibration positioned at 1040 cm−1 (9.6 μm).
Figure 2. Mid-IR spectra of (a) pristine carbon dioxide ice surface and after D+2 irradiation at 11 K and (b) D2-water and D2-carbonic acid remaining between 140 and 240 K. Absorption band positions and assignments are presented in Table 2.
Download figure:
Standard image High-resolution imageFigure 2(b) shows the 2800–700 cm−1 (3.6–14.3 μm) mid-IR spectra collected at 120, 160, 200, and 240 K during the annealing period of the D+2-irradiated carbon dioxide ice. An array of absorption features are observed in the spectra after the carbon dioxide condensate (Tsub(CO2) ∼ 95 K) and primary irradiation products carbon monoxide (Tsub(CO) ∼ 65 K), ozone (Tsub(O3) ∼ 50 K), and carbon trioxide (Tdecomp(CO3) ∼ 90 K) have sublimed or thermally decomposed. At 120 K, a broad and diffuse absorption feature in the 2730–2300 cm−1 (3.7–4.3 μm) region is likely to originate from the overlapping asymmetric (ν3) and symmetric (ν1) D–O stretching modes of amorphous D2-water ice, previously seen in the D+2-irradiated oxygen spectra. This is supported by the rapid reduction in absorption intensity of the band at ∼160 K, correlating with the sublimation temperature of D2-water. However, after all D2-water is completely sublimed at 200 K, two broad absorption features centered at 2262 cm−1 (4.4 μm) and 2110 cm−1 (4.7 μm) remain in the D–O stretching region. In addition, intense absorption signatures located at 1720 cm−1 (5.8 μm) and 1486 cm−1 (6.7 μm) are still clearly identified, blueshifted from their respective 1709 cm−1 (5.9 μm) and 1433 cm−1 (7.0 μm) position at 120 K. Finally, low-intensity absorption bands are observed at 1330 cm−1 (7.5 μm), 1025 cm−1 (9.8 μm), and 819 cm−1 (12.2 μm). After consideration of all possible analogs of deuterium–carbon–oxygen compounds, including D2-formaldehyde (D2CO) and D2-formic acid (DCOOD) as carriers for the obvious ν(C=O) stretching absorption at 1720 cm−1, the absorption spectrum at 200 K best resembles that of α- phase D2-carbonic acid (D2CO3) previously assigned in the literature (DelloRusso et al. 1993; Hage et al. 1993, 1996). Further evidence is provided by the complete disappearance of the bands by 240 K, corresponding with the 210–256 K sublimation range expected for carbonic acid (H2CO3) (Zheng & Kaiser 2007). The complete assignment of the absorption bands to vibrational modes of D2-carbonic acid can be observed in Table 2.
In summary, the online FTIR analysis of D+2-irradiated molecular oxygen (O2) condensates identified the formation of ozone (O3) and D2-water (D2O), while the exposed carbon dioxide (CO2) ices also displayed absorption features attributed to ozone and D2-water, in addition to bands assigned to carbon monoxide (CO), cyclic carbon trioxide (c-CO3), and D2-carbonic acid (D2CO3).
3.1.2. Mass Spectrometry
It is useful to correlate the results of the solid-state FTIR analysis to the abundance of gaseous species evolved as the substrate is annealed to 300 K. Initially, the controlled heating promotes the diffusion of reactive species in the condensates. However, at higher temperatures, the heating induces the sublimation of the ice matrix (molecular oxygen or carbon dioxide) together with any molecular products formed during the ice irradiation at their inherent sublimation temperatures. For the present set of experiments, mass-to-charge (m/z) signals are detected as the molecules sublime from the irradiated ices and are ionized by the QMS. Correlating with the gas-phase abundance of a species, these ion currents are presented as a function of substrate temperature (Figure 3: line format) and are compared to the blank ice samples (Figure 3: dotted format).
Figure 3. Ion counts for gas-phase species produced during the controlled heating of (a) molecular oxygen ice and (b) carbon dioxide ice after D+2 irradiation at 11 K. The ion counts for pristine oxygen and carbon dioxide ice samples are depicted in dotted format.
Download figure:
Standard image High-resolution imageThe ion currents produced from the annealed oxygen ice sample, after D+2 ion exposure, are displayed in Figure 3(a). Here, implanted deuterium molecules (D+2: m/z = 4) are observed to be released from the ice between 12 and 20 K. This indicates that the irradiating D+2 ions predominantly undergo dissociation and neutralization upon impact to produce D atoms. Once these D atoms have dissipated their energy to the matrix, they then combine to reform deuterium molecules (D2). It follows that the molecular oxygen condensate (O2+: m/z = 32) starts to sublime at 25 K, releasing a significant amount of entrapped molecular D2 with it. Molecular ozone (O3+: m/z = 48) is retained on the substrate before subliming between 53 and 70 K (Bennett & Kaiser 2005). Finally, between 145 and 160 K, an ion current for D2-water (D2O+: m/z = 20) is observed as it leaves the substrate at its expected sublimation temperature of ∼150 K (Zheng et al. 2007a). It should be noted that the gas-phase D2-hydrogen peroxide (D2O2+: m/z = 36) was searched for but could not be identified above the current threshold of 10−13 A.
Figure 3(b) displays the detected ion currents from annealed carbon dioxide ice irradiated with D+2 ions. The sublimation of the carbon dioxide matrix (CO+2: m/z = 44) is observed to occur between 75 and 95 K. Implanted deuterium molecules (D+2: m/z = 4) sublime in the 12–25 K temperature range correlating with the irradiated oxygen condensates. The ion current for carbon monoxide (CO+: m/z = 28) increases at 40 K and displays a prolonged sublimation profile up to the release of the carbon dioxide matrix at 75 K, which infers a slow diffusion of carbon monoxide through the condensate before release. Subliming between 150 and 175 K, D2-water (D2O+: m/z = 20) is clearly observed, over one order of magnitude above the detection limit of the QMS. Concerning the other irradiation products previously identified in the solid state, there was no detection of the ozone (O3+: m/z = 48) subliming at ∼60 K indicating it was only formed in trace abundances. Cyclic carbon trioxide (c-CO3) undergoes thermal decomposition at ∼90 K (Kaiser & Mebel 2008) and is therefore not detected in the gas phase. In addition, D2-formaldehyde (D2CO+: m/z = 32) shares its mass-to-charge signal with the prominent O2+ signal, which prevents the species from being verified in the gas phase.
3.2. Quantitative Analysis
3.2.1. Oxygen Ice
The irradiation of oxygen ice with D+2 ions produced ozone and D2-water as molecular irradiation products, both identified by their characteristic infrared absorption bands in the solid state. First, the oxygen molecules must be dissociated by energetic particle impact to produce atomic oxygen as a reaction intermediate for the formation of ozone (Cosby 1993). This leads to a decrease in the molecular oxygen abundance in the ice. However, the large error associated with calculating the column density of the oxygen molecules from its weak ν1(O2) absorption band (Bennett & Kaiser 2005), combined with the low yield for oxygen molecule destruction in the sample, meant the temporal abundance of molecular oxygen could not be developed over the irradiation period. However, the initial column density of molecular oxygen was approximately measured to be ∼1 × 1018 molecules cm−2. The reaction of a suprathermal oxygen atom with an adjacent oxygen molecule results in the formation of ozone, which increases its abundance (molecules cm−2) in the oxygen matrix over the exposure period (Figure 4). The temporal column densities for ozone were calculated by using an integral absorption coefficient of 1.5 × 10−17 cm molecule−1 (Adler-Golden et al. 1985) for the ν3(O3) asymmetric stretching fundamental at 1045 cm−1. It can be observed in Figure 4 that, after a period of ozone formation (∼2.0 × 103 s), the abundance of ozone in the condensate reaches a maximum column density of (9.5 ± 1.5) × 1015 molecules cm−2. Finally, the abundance of D2-water formed in the condensate can be monitored in the same way, after converting the integrated area of the broad 2702–2230 cm−1 absorption region to a column density using a the sum of the ν1(D2O) and ν3(D2O) absorption coefficients, combining to 1.4 × 10−16 cm molecule−1 (Bergren et al. 1978). There are multiple possible pathways for D2-water production, including deuterium atom attachment to molecular or atomic oxygen to form DO2 or DO radical intermediates. Subsequent radical reactions with deuterium atoms result in D2-water production, inferring that its abundance is not completely dependent on the destruction rate of molecular oxygen. In Figure 4, we see that the column density of D2-water is still increasing toward the end of the irradiation period (6.0 × 103 s), as more deuterium is implanted in the condensate for reaction.
Figure 4. Temporal column densities (error of 15%) for ozone and D2-water during the irradiation of oxygen ice with D+2 ions. Inferred temporal column density for D-hydroxyl (OD) intermediate depicted as a dashed line.
Download figure:
Standard image High-resolution image3.2.2. Carbon Dioxide
FTIR spectroscopy of carbon dioxide ice irradiated with 5 keV D+2 resulted in the detection of solid-state carbon monoxide, carbon trioxide, ozone, D2-water, and D2-carbonic acid. The initial column density of carbon dioxide was calculated to be (2.4 ± 0.4) × 1018 molecules cm−2 by the species infrared absorption intensity. The exposure of carbon dioxide to energetic ion impacts induces the target molecules to undergo homolytic bond cleavage, resulting in the production of carbon monoxide and atomic oxygen. The subsequent increase in abundance of carbon monoxide is monitored by its ν1(CO) stretching absorption band, which after being converted to the species column density using an integrated absorption coefficient of 1.1 × 10−17 cm molecule−1 (Gerakines et al. 1995), can be observed in Figure 5. Here, the abundance of carbon monoxide appears to reach a maximum column density of (3.0 ± 0.5) × 1016 molecules cm−2 over the exposure period. In addition, the suprathermal oxygen atom can react with an adjacent carbon dioxide molecule to form cyclic carbon trioxide. The production of the species is traced by its ν1(CO3) fundamental and, after conversion using an integral absorption coefficient of 8.9 × 10−17 cm molecule−1 (Bennett et al. 2004), the resultant temporal column density plot is displayed in Figure 5. It is observed that the species is formed rapidly in the matrix once irradiation commences, reaching a maximum abundance of (3.9 ± 0.6) × 1014 molecules cm−2 after ∼1 hr of exposure. However, following this time period, the abundance of carbon trioxide decreases in an exponential fashion, reduced to a column density of (2.3 ± 0.3) × 1014 molecules cm−2 after irradiation is halted at t = 10 hr.
Figure 5. Temporal column densities (error of 15%) calculated for carbon dioxide, carbon monoxide, cyclic carbon trioxide, and ozone during the irradiation of carbon dioxide ice with D+2 ions. Substrate temperature (red: line format) and extrapolated sublimation points (blue: dotted format) are also displayed for each species.
Download figure:
Standard image High-resolution imageAn alternate fate for newly formed atomic oxygen in the condensate is to commence successive radical recombination reactions to produce ozone, by way of an oxygen molecule intermediate which is undetectable in the solid-state infrared analysis. The production of ozone is similarly observed by converting the band intensity of its ν3(O3) fundamental to an ozone column density, using the same 1.53 × 10−17 cm molecule−1 integral absorption coefficient previously used for the oxygen ice analysis. The resultant temporal profile for ozone abundance is depicted in Figure 5. Here, the ozone column density reaches a threshold value of (4.0 ± 0.6) × 1015 molecules cm−2 after ∼1 hr of irradiation, before decreasing slightly in abundance to (3.6 ± 0.5) × 1015 molecules cm−2 toward the completion of the experiment. With ozone displaying a similar temporal profile to carbon trioxide, both species are likely to play a role as reaction intermediates in the irradiated ice system. This is inferred as they appear to be consumed over the prolonged irradiation period.
Of the host of possible deuterated molecules that could be produced in the condensate, only D2-water and D2-carbonic acid could be verified by infrared analysis. Unfortunately, time-resolved column densities for D2-water could not be calculated at 11 K due to its ν1(D2O) and ν3(D2O) absorption region being dominated by the strong ν3(CO2) absorption band of the host carbon dioxide. However, temporal abundances could be determined for D2-carbonic acid using the species carbonyl stretching band at ∼1720 cm−1 and applying an integrated absorption coefficient of (11 ± 1) × 10−17 cm molecule−1 (Gerakines et al. 2000). As displayed in Figure 6, the species steadily increases in abundance over the irradiation period, reaching a final column density of (1.0 ± 0.2) × 1015 molecules cm−2 after the 10 hr exposure, which does not appear to be a threshold value. Construction of reaction mechanisms to correlate with the observed irradiation products and associated abundance profiles will follow in the discussion section, where we hope to untangle the radiolytic processes proceeding in these ices.
Figure 6. Temporal column density (error of 15%) calculated for D2-carbonic acid formed during the irradiation of carbon dioxide ice with D+2 ions. Substrate temperature (red: line format) and extrapolated sublimation points (blue: dotted format) are also displayed.
Download figure:
Standard image High-resolution image4. DISCUSSION
4.1. Oxygen Ice
The irradiation of a solid surface initiates chemical processing through particle–target interaction. In the case of fast ions colliding with a low-temperature condensate, energy is transferred from the ions to the surface by inelastic electronic interactions (Se) or by direct "knock-on" processes, where the implant ejects an atom by elastic nuclear collision (Sn). Both regimes act to generate a cascade of suprathermal electrons and atoms which are expelled from the track of the impinging particle. For 5 keV D+2 ions colliding with an oxygen ice surface at 11 K, these processes induce the unimolecular decomposition of the internally excited oxygen molecules via homolytic bond rupture (Reaction (1)—term symbols omitted for clarity), producing oxygen atoms that are not in thermal equilibrium with the surrounding matrix. This is assumed to proceed via first-order kinetics in line with previous studies of solid oxygen irradiation (Bennett & Kaiser 2005). Unfortunately, the resultant decrease in molecular oxygen column density could not be monitored during the exposure period to verify this inference:

The suprathermal oxygen atoms possess sufficient mobility to react with an adjacent oxygen molecule in the matrix to produce an ozone molecule (Reaction (2)). The bimolecular reaction is defined to proceed at a rate k (molecules−1 cm2 s−1). Now we assume that the unimolecular decomposition reaction (Reaction (1)) proceeds much faster than the bimolecular reaction (Reaction (2)), making k the rate-determining step for ozone production displayed in Figure 4. Therefore, after defining [O2]0 as the starting abundance of molecular oxygen which is converted to ozone over time, t, we fit the temporal evolution of ozone, [O3]t, to a simple pseudo-first-order kinetic profile to establish its formation rate in the condensate at 11 K (Equation (3)):


Figure 4 depicts the optimized kinetic fit for the profile, where [O2]0 = (9.5 ± 1.5) × 1015 molecules cm−2 and k = (3.5 ± 0.4) × 10−3 molecules−1 cm2 s−1. The formation rate for ozone induced by 5 keV D+2 is faster than the k = (7.9 ± 0.3) × 10−4 molecules−1 cm2 s−1 rate calculated for 5 keV electrons by Bennett & Kaiser (2005), who also adopted a similar pseudo-first-order kinetic analysis for irradiated oxygen ice at 10 K. This discrepancy is likely due to the 5 keV D+2 irradiation inducing oxygen atom "knock-on" particles in the condensate through nuclear (Sn) particle–target stopping interactions in addition to energy lost electronic (Se) interactions. This is opposed to the 5 keV electron irradiation that transfers its kinetic energy exclusively through electronic interactions.
The observation of D2-water in the solid state indicates additional chemical processes are proceeding in the oxygen ice matrix. There are a number of possible pathways that can be proposed to account for its formation. Unfortunately, the production of likely reaction intermediates such as the DO radical could not be detected, due to their absorption bands overlapping with other species (i.e., D2O). However, we consider that an implanted deuterium atom can participate in a number of reactions involving either oxygen atoms or oxygen molecules to generate D2-water. Previous studies (Miyauchi et al. 2008; Cuppen et al. 2010; Ioppolo et al. 2010) involving the reaction of cold (70–273 K) hydrogen atoms with oxygen at 10 K favored water formation at the vacuum–surface interface through the following pathway (Reactions (4)–(6). This diffusion-limited pathway involves the formation of the hydroperoxy (HO2) radical and molecular hydrogen peroxide (H2O2) intermediates, both of which have also been identified in low-temperature ice mixtures containing water and molecular oxygen after electron irradiation (Zheng et al. 2007b):



As we do not observe any D2-hydrogen peroxide in the solid state by FTIR, or desorbed from the surface during the annealing period by the QMS, we suggest D2-water is formed by an alternate reaction pathway. As the high-energy collisions between the impinging particles and the condensate can efficiently dissociate the molecular oxygen precursor, as opposed to cold hydrogen atoms, we favor a reaction mechanism that involves sequential deuterium atom addition reactions to atomic oxygen (Reactions (7) and (8); Hiraoka et al. 1998). For the associated reactions involving the hydrogenation of atomic oxygen, these pathways have been calculated to proceed without activation barriers (Cuppen & Herbst 2007):


Assuming that D2-water is produced from successive deuterium addition pathways shown above, we can fit its temporal abundance, [D2O]t (molecules cm−2), to a pseudo-first-order kinetic model with two sequential steps (A→B→C) over the exposure time, t (s) using Equation (9) (Steinfeld et al. 1989). Here, we define [A]0 as the starting atomic oxygen abundance from Reaction (7), reacting with a deuterium atom at a unimolecular rate k1 (s−1) to form the DO radical intermediate defined as [B]. It follows that [B] undergoes a second successive deuterium addition (Reaction (8)) to form [C], the D2-water product, at rate k2 (s−1):

The kinetic fit depicted in Figure 4 yields a total oxygen atom consumption of [A]0 = 3.1 × 1015 molecules cm−2 in forming DO radicals, with the reaction proceeding at a rate k1 = 8.0 ± 1.2 × 10−6 s−1. Subsequently, the newly formed DO radicals react with additional deuterium atoms to form the D2-water product at a rate k2 = 6.0 ± 0.9 × 10−4 s−1. These kinetic parameters were then used to compile a temporal profile for the column density of the DO radical intermediate, [DO]t, which is superimposed on the D2-water plot in Figure 4 (dashed line), using Equation (10). This indicates a maximum DO column density of 3.0 × 1014 radicals cm−2 at t ≈ 1 hr:

4.2. Carbon Dioxide Ice
The bombardment of solid carbon dioxide with 5 keV D+2 ions initiates non-equilibrium chemical processes within the surface through both electronic and nuclear particle–target interactions, as detailed above for irradiated molecular oxygen. These processes induce homolytic bond cleavage of carbon dioxide molecules to yield carbon monoxide and suprathermal oxygen atoms. The decomposition of carbon dioxide is thought to follow a similar first-order kinetic model to that applied in a previous study involving electron irradiation (Bennett et al. 2004). This would account for the observed decrease in column density of the carbon dioxide precursor and the associated increase in carbon monoxide column density displayed in Figure 5 for the present experiments. Furthermore, the liberated oxygen atoms are expected to undergo subsequent radical addition reactions with host carbon dioxide molecules to form cyclic carbon trioxide, and recombination reactions with other newly formed oxygen atoms to generate molecular oxygen and ozone in the local ice matrix. Cyclic carbon trioxide and ozone were identified in the condensate by their absorption bands, which were converted to their temporal abundances depicted in Figure 5.
The specific pathways leading to the formation of irradiation products carbon monoxide, carbon trioxide, and ozone are assumed to be similar to those proposed in earlier work involving the exposure of carbon dioxide ice to energetic electrons (Bennett et al. 2004). In addition, successive deuterium attachment to atomic oxygen is expected to result in the D2-water observed to form in the system, following the same reaction pathway as constructed above for the D+2 irradiation of oxygen ice. The absence of D2-formaldehyde (D2CO) in both the online FTIR analysis of the irradiated ice and subsequent sublimation process by mass spectroscopy is in agreement with many previous studies investigating irradiated carbon dioxide and water ice mixtures which did not detect formaldehyde (H2CO) in the solid state (Brucato et al. 1997; Gerakines et al. 2000; Wu et al. 2003; Zheng & Kaiser 2007). Exhaustive efforts to fit the temporal profiles in Figure 5 to a kinetic model were unsuccessful. It is interesting to note that in this study, carbon trioxide shows a rapid increase in column density immediately after ion exposure, only to be followed by an exponential-like decay in abundance over the remaining irradiation period (Figure 5). Such a temporal profile is commonly associated with a reaction intermediate species. This is opposed to the electron-irradiation experiment performed by Bennett et al. (2004), where the exponential growth profile fitted to the carbon trioxide abundance implied that the species was a final product.
A possible pathway that includes cyclic carbon trioxide as a reaction intermediate is proposed for the formation of D2-carbonic acid. This process could be driven by an increase in the implanted deuterium atom concentration within the matrix over the irradiation time. We propose that this reaction proceeds according to a sequential pathway shown in Reaction (11). Here, cyclic carbon trioxide is formed in the first reaction by the addition of a suprathermal oxygen atom to a carbon dioxide molecule. It follows that two reactive deuterium atoms can successively attach to the strained oxygen ring of the cyclic carbon trioxide molecule to successively form the two D-hydroxyl groups of the D2-carbonic acid species. We suggest this mechanism as it involves the least number of sequential steps from the carbon dioxide precursor to the D2-carbonic acid product. In addition, the pathway conserves the C=O carbonyl bond from carbon dioxide precursor to the carbon trioxide intermediate to, finally, the product D2-carbonic acid molecule:
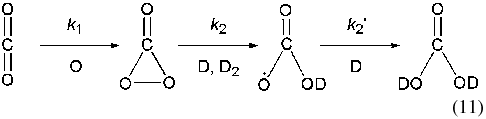
Validation for the proposed mechanism may be provided by comparing the total abundance of carbon trioxide to the D2-carbonic acid abundance in the condensate over the exposure period (3.6 × 104 s). The maximum carbon trioxide column density (∼(4.5 ± 0.5) × 1014 molecules cm−2) is reached quickly after exposure before falling away to ∼(2.5 ± 0.5) × 1014 molecules cm−2 at the end of the irradiation period—a total decrease of (2.0 ± 0.5) × 1014 molecules cm−2 in the condensate. However, the total abundance of D2-carbonic acid formed during exposure is measured to be (1.0 ± 0.2) × 1015 molecules cm−2. This implies that the production of D2-carbonic acid from the carbon trioxide intermediate, depicted as k2 and k2` (both in molecules−1 cm2 s−1), occurs at a faster rate than the replenishment of the carbon trioxide abundance, k1 (molecules−1 cm2 s−1); regenerated as carbon dioxide reacts with suprathermal oxygen atoms over continual irradiation with D+2 ions. However, due to the complexity of the overall reaction scheme, which must also take into account the depletion of oxygen atoms in the condensate due to molecular oxygen and ozone formation, the associated rates for these steps in Reaction (11) could not be calculated.
Further evidence is provided during the controlled heating of the condensate from 11 to 300 K, the sublimation temperatures of the individual condensate molecules can be observed in the temporal abundances displayed in Figure 5. The carbon dioxide matrix rapidly sublimes from the substrate between 85 and 100 K. This follows the initial decrease in carbon monoxide and ozone column density as the irradiation products start to sublime at 38 and 65 K, respectively. Cyclic carbon trioxide displays the slowest depletion rate during the annealing of the solid, as instead of subliming to the gas phase, the species undergoes thermal decomposition between 35 and 110 K (Kaiser & Mebel 2008). It is interesting to note that this temperature range corresponds to a rapid increase in column density of the D2-carbonic acid species (Figure 6) to a maximum (2.6 ± 0.4) × 1015 molecules cm−2 at a substrate temperature of 90 K. However, a direct correlation between the destruction of carbon trioxide and D2-carbonic acid during the annealing of the sample cannot be confirmed. This is due to physical properties of the remaining solid, including the density and ice morphology, being completely altered after the sublimation of the carbon dioxide matrix. These changes can also be a cause for the sharp increase in the observed D2–carbonic acid column density, as opposed to carbon trioxide conversion, particularly as nascent D+ or D+2 ions would have reacted or sublimed from the sample at these elevated temperatures.
5. ASTROPHYSICAL AND PLANETARY IMPLICATIONS
In summary, the implantation of protons into cold, oxygen-bearing condensates—as simulated in the present study by the irradiation of molecular oxygen and carbon dioxide ices by 5 keV D+2 ions—is of considerable importance in the context of icy solar system surfaces. The results of the present study show the ability of fast deuterium atoms (formed by neutralization of their implanted ions) and their nascent ions to transfer their kinetic energy to the solid sample through multiple particle–target interactions. These collisions induce bond dissociation of the target molecules which ultimately yield product species such as ozone from the irradiated molecular oxygen ice and ozone, carbon monoxide, and cyclic carbon trioxide from the irradiated carbon dioxide ice. These products were identified during the present experiments by FTIR in the solid condensates and in the gas phase by QMS once the species had desorbed from the heated substrate. In addition, the deuterium atoms can undergo reaction with reactive species in the matrix once they have dissipated all their kinetic energy, forming D2-water in the oxygen ice sample and D2-water and D2-carbonic acid in the carbon dioxide sample.
Solid-state oxygen and carbon dioxide, having been identified on the predominantly water ice surfaces of the moons of Jupiter (McCord et al. 1998) and Saturn (Filacchione et al. 2010), are continually bombarded with energetic particles. To locate a source for the D+2 ions used to irradiate the icy samples during the present study, it is observed that Jupiter and Saturn maintain an isotopic D/H ratio of ∼2 × 10−5 (Owen & Encrenaz 2003) in their molecular composition, corresponding to the isotopic ratio detected in interstellar media and in solar nebulae. Therefore, in relation to the molecular hydrogen molecules which have thermally escaped from the extended atmospheres of Jupiter (Radioti et al. 2005) and Saturn (Shemansky et al. 2009), D2 molecules are particularly scarce (keep in mind that most deuterium would be found in partially deuterated HD molecules). However, icy bodies with hydrogen residing in their atmospheres, such as Europa (Smyth & Marconi 2006) and Titan (Cui et al. 2008), display an enriched stellar ice D/H ratio of ∼2 × 10−4 (Esposito et al. 2004), meaning a higher proportion of D2 molecules are expected to escape from these satellite's tenuous atmospheres. Here, the combined component of D2 molecules liberated from gas giants and their satellite atmospheres are readily ionized to D+2 and D+ via photoionization or electron impact processes (Liu & Shemansky 2004), with the charged species contributing to the hot plasmas suspended within the magnetospheres of both planets. In addition, fast protons from the solar wind also maintain a solar nebula D/H ratio of ∼2 × 10−5, providing another source for D+ which can be implanted on icy outer solar system bodies along with the magnetospheric particles described above. It can therefore be reasoned that energetic D+2 and D+ ions, even as a small fraction of the total solar system hydrogen abundance, still contribute to surface radiolysis processes. It follows that deuterated analogs of the reactive intermediates and molecular products formed via hydrogen implantation are expected to be produced in low yields within planetary ices.
The present experiments have shown that D2-water formation is feasible in pure oxygen condensate via exposure to 5 keV D+2 ion irradiation. Assuming that the impinging particle is dissociated and neutralized upon impact with the surface (Sigmund et al. 1996), we propose that D2-water is produced by the successive attachment of deuterium atoms to a suprathermal oxygen atom. This mechanism has been previously suggested to occur in ice samples containing liberated oxygen atoms which are exposed to a source of hydrogen atoms (Hiraoka et al. 1998). As the D-hydroperoxy (DO2) radical or the D2-hydrogen peroxide (D2O2) molecule is not readily identified in the solid state during irradiation in the present study, sequential deuterium attachment directly to the molecular oxygen precursor, as proposed in similar experiments using "cold" hydrogen atoms, does not appear to proceed with higher-energy regimes (presumably due to low abundances of atomic oxygen being generated by low-energy collisions). The generation of water from molecular oxygen could be of particularly relevance to Jupiter's largest moon Ganymede. Here, condensed oxygen molecules have been detected on the body's relatively warm (∼100 K) surface. As shown previously, the species can be converted to water via reaction with hydrogen atoms which are observed to be an abundant species (1.5 × 104 ions cm−3 (Barth et al. 1997)) in the moons contained atmosphere. Although these interactions may have a limited influence on the abundance of water (the dominant component of outer solar system ices such as Ganymede's), such processes are an important consideration when modeling for the amount of molecular oxygen and ozone harbored within these environments.
Furthermore, in regards to the Earth moon's frigid polar regions, it can be speculated that energetic hydrogen ions emanating from the solar wind could undergo similar implantation processes (Starukhina 2006) and possibly react with oxygen inside the silicates (Colaprete et al. 2010). The detection of absorbed lunar water (with recent data pointing toward a 5.6% ± 2.9% mass abundance in a south polar crater; Colaprete et al. 2010) has caused postulations that the hydrogenation of silicate based minerals is the source of the water abundance observed on the moon surface (Clark 2009). Our studies suggest that implanted protons—after neutralization—react with oxygen atoms released as knock on particles from these silicates, which could be a channel for water production in these regions. Here, the synthesis of water involves hydroxyl radicals (OH) only if sufficient protons are implanted and neutralized, where hydroxyl radicals can recombine with atomic hydrogen to form water. In case of insufficient hydrogen concentrations, the synthesis is "stuck" with the formation of the hydroxyl radicals (OH). Therefore, the "detection" of "water" in the south polar crater (Colaprete et al. 2010) could be either water (H2O) or hydroxyl (OH) radicals as the detection method employed in the in situ study cannot discriminate between these species.
In addition to water being efficiently formed from the irradiation of molecular oxygen ices with hydrogen ions and atoms, we demonstrate that carbon dioxide ice exposed to energetic D+2 ions yields D2-carbonic acid. We have presented experimental evidence that this reaction pathway most likely involves a successive deuterium atom addition to cyclic carbon trioxide, which is a species commonly detected in electron-irradiated carbon dioxide (Bennett et al. 2004). As carbon dioxide has been detected on many outer solar system surfaces, the analogous chemical processing of these ices with H+2 or H+ ions to form carbonic acid is of particular importance to abiotic chemistry. Solid carbonic acid, which is unstable under ambient conditions, could react with co-deposited species in the icy environment such as ammonia (NH3) to form high-order products through acid–base chemistry. If produced in sufficient quantities within the ice, two neighboring carbonic acid molecules could react to form oxalic acid, which is a biologically significant molecule on Earth.
C.P.E. and R.I.K. thank the Air Force Office of Scientific Research (A9550-09-1-0177) for support. C.J.B. and B.M.J. thank the National Aeronautics Space Administration (NASA Astrobiology Institute under Cooperative Agreement no. NNA09DA77A issued through the Office of Space Science) for funding.