ABSTRACT
Supernova remnants (SNRs) interacting with dense molecular clouds (MCs) are proven to be bright γ-ray emitters by recent observations in the GeV–TeV band. We theoretically investigate the multiband radiative properties of the four middle-aged SNRs IC443, W51C, W28, and W44 with a time-dependent injection model. In the model, part of the SNR shell transports into a dense MC, with the other part of the shell evolving in a relatively tenuous interstellar medium. We find a broken power law with a break energy of ∼3–40 GeV that must be imposed to reproduce the observed multiwavelength spectra for the four remnants. The results indicate that the observed γ-ray spectra can be reproduced as a p–p interaction of the high-energy protons injected by the shell interacting with the MC with the dense matter, whereas the radio emission is produced via synchrotron radiation of the injected electrons from the other part of the shell for the four middle-aged SNRs.
Export citation and abstract BibTeX RIS
1. INTRODUCTION
Recently, GeV gamma-ray emissions from four middle-aged supernova remnants (SNRs) IC443, W51C, W28, and W44 interacting with molecular clouds (MCs) have been detected by the Fermi Large Area Telescope (LAT; Abdo et al. 2009, 2010a, 2010b, 2010c). From Fermi observations, the gamma-ray spectra of these SNRs indicate broken power-law forms with typical break energies of ∼3–10 GeV and different spectral indices above the break energy. In addition, three of these SNRs are also detected to be emitting TeV gamma-ray emission. These data provide an exciting opportunity to study the emission from the interaction of the SNR with the surrounding matter. Since MCs with high densities are efficient targets of cosmic-ray protons in the SNR–MC interaction system, the clouds illuminated by the protons accelerated in a nearby SNR could be bright gamma-ray sources in which GeV–TeV gamma rays mainly arise from the decay of π0 produced in inelastic collisions of the accelerated protons with MCs.
Two kinds of models have been proposed to describe gamma-ray emission in the SNR–MC interaction system. The first one assumes that gamma rays produced in MCs result from π0 decay in the inelastic collisions of the accelerated protons which have escaped from a nearby SNR with MCs and the spectrum of the accelerated protons has a broken power-law form due to the finite size of the emission region (Aharonian & Atoyan 1996; Torres et al. 2008; Gabici et al. 2009; Li & Chen 2010; Ohira et al. 2011). Ohira et al. (2011) applied the model to explain observed gamma-ray spectra in the GeV–TeV energy bands for these four middle-aged SNRs. They found that a runaway-cosmic-ray (CR) spectrum of these SNRs interacting with MCs could be the same, even though it leads to different gamma-ray spectra. On the other hand, Zhang & Fang (2008) proposed a model to explain the observed multiband nonthermal emission of IC443. In this model, an MC is located near the SNR between us and the SNR, a fraction f of the SNR shell evolves in the MC (f = 1 means that the whole SNR shell is surrounded by the MC) and the other part evolves in the ambient interstellar environment immediately after supernova explosion (the two parts of the shell evolve independently), and the shell evolves in a uniform medium, i.e., the densities both in the interstellar medium (ISM) and in the MC are constant for simplicity. Zhang & Fang (2008) found that the observed gamma rays are produced both via bremsstrahlung of the shell in the MC and via p–p interaction because the high-energy protons interact with the dense matter in the MC, whereas the radio emission from the rim of the SNR is produced via synchrotron radiation in the shell interacting with the ISM.
In the model of Zhang & Fang (2008, hereafter ZF08), a time-dependent nonthermal particle and photon spectra for both young and old shell-type SNRs by including the evolution of secondary e± produced via p–p interaction when high-energy protons collide with the ambient matter in an SNR (Zhang & Fang 2007; Fang & Zhang 2008) are calculated, where the volume-averaged production rates of the shock-accelerated electrons and protons are the same as those in Sturner et al. (1997), and the total amount of the kinetic energy contained in the injected particles has been completely converted into the kinetic energy of both the electrons and protons during the time up to the radiative stage. However, theoretical investigations show that (1) CRs accelerated at the shock in the partially ionized medium have a broken power-law spectrum, the spectrum above the break energy is steeper than that below the break energy because the damping of waves that resonantly scatter the CRs is significant (Malkov et al. 2011; Inoue et al. 2010), and (2) the accelerated particles at the shock reach their maximum energy near a Sedov stage (Caprioli et al. 2010), so that it is possible that both the electrons and protons obtain their highest kinetic energies during more or less the Sedov stage. Therefore, we make the following modifications to revise the model of Zhang & Fang (2008): (1) the volume-averaged production rates of the shocked-accelerated particles are assumed to have a broken power-law form with a break energy Eb and (2) the total amount of the kinetic energy contained in the injected particles has been completely converted into the kinetic energy of both the electrons and protons during the time tci = ξtSed with a parameter ξ > 10, where tSed is the time that the Sedov phase begins. We apply this revised model to these middle-aged SNRs and find out that our results can reproduce the multiband nonthermal spectra of these SNRs under reasonable parameter spaces.
This paper is organized as follows. In Section 2, we review briefly the model of Zhang & Fang (2008) and our modifications to this model. We apply the revised model to these middle-aged SNRs in Section 3. Finally, we give our conclusions and discussion in Section 4.
2. THE MODEL
2.1. Review of the ZF08 Model
In the ZF08 model, three assumptions are made: (1) an MC is located near the SNR between us and the SNR; (2) immediately after supernova explosion, a fraction f of the SNR shell evolves in the MC and other part (1 − f) in the ambient interstellar environment, moreover, the two parts of the shell evolve independently; and (3) the shell evolves in a uniform medium (the ambient ISM or MC). Note that f = 1 represents that the whole SNR shell is surrounded by the MC. Under these assumptions, the nonthermal photon spectra from these two parts for the SNR for which a part of the shell (f < 1) interacts with the MC are calculated on the frame of the time-dependent model given by Fang & Zhang (2008). However, the value of the factor f would be limited to f ≪ 1, otherwise the above assumptions will not be valid. Therefore, we change the condition f < 1 to f ≪ 1 or (1 − f) ≪ 1. In the ZF08 model, the temporal evolution of photon emission from the SNRs is modeled through three parts: the production of accelerated particles by a shock wave, the temporal evolution of particle energy distributions, and the production of photons.
For the production of accelerated particles, the analytical model of the shock dynamics of an SNR with an explosion energy E = E51 × 1051 erg expanding at a velocity v0 = v9/109 cm s−1 into a uniform ambient medium with density n0 is used, where n0 = μnISM and nISM is the hydrogen density in the local ISM, μ = 1.4 is the mean atomic weight of the ISM assuming 1 helium atom for every 10 hydrogen atoms. The SNR evolves through the free expansion stage which ends at t = tSed ≈ 2.1 × 102(E51/n0)1/3v95/3), the Sedov stage which ends at t = trad ≈ 4.0 × 104E4/1751n−9/170 yr, and the radiative stage in which the SNR begins to experience significant radiative cooling (Sturner et al. 1997). The shock velocity vs(t) corresponding to these stages is vs(t) = v0 for t < tSed, vs(t) = v0(t/tSed)−3/5 for tSed ⩽ t < trad, and vs(t) = v0(trad/tSed)−3/5(t/trad)−2/3 for t > trad. The shock radius is given by Rs(t) = ∫vs(t)dt.
The volume-averaged production rates of the shock-accelerated electrons and protons are assumed to be

where i = e, p, G(t) is a factor which relates to time, i.e., G(t) = Rs(tSed)/Rs(t) for t ⩽ trad and G(t) = 0 for t > trad, α is the spectral index, and Ee, max and Ep, max are the maximum energies of the accelerated electrons and protons, respectively. Factors Q0e and Q0p are used to normalize the particle spectra so that the total amount of kinetic energy contained in both the injected electrons and the injected protons is Epar = ηMejv20/2, where η ∼ 0.1 is the efficiency that the kinetic energy of the ejecta with initial mass Mej and initial velocity v0 is converted into the kinetic energy of both the electrons and the protons. In the estimate of Epar, a parameter Kep = Q0e/Q0p is introduced (see Zhang & Fang 2007 for details).
Assuming that an SNR interior is homogeneous, with a constant density nSNR = 4nISM and a magnetic field strength BSNR = 4BISM, ne(Ee, t) and np(Ee, t) are used to represent the differential densities of accelerated electrons and protons, respectively. The direction- and volume-averaged electron intensity Je(Ee, t) = (cβ/4π)ne(Ee, t) and proton intensity Jp(Ep, t) = (cβ/4π)np(Ep, t) at each moment during the SNR lifetime can be calculated by solving Fokker–Planck equations for both electrons and protons in energy space, which are given by (Zhang & Fang 2007)
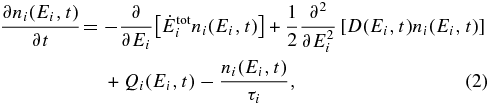
where i = e, p, the terms on the right-hand side in Equation (2) represent systematic energy losses, diffusion in energy space, the particle source function, and catastrophic energy loss. It should be noted that the source term for electrons includes the evolution of secondary e± produced via p–p interaction when high-energy protons collide with the ambient matter in the SNR, i.e.,

where

where μpp is an enhancement factor for collisions involving heavy nuclei in an SNR (Sturner et al. 1997) and and
are the differential cross section for electrons and positrons produced via p–p interaction, respectively (Kamae et al. 2006). We solve Equation (1) using a Crank–Nicholson finite difference scheme.
After obtaining the electron intensity Je(Ee, t) and the proton intensity Jp(Ep, t) at each moment during the SNR lifetime, we can calculate the photon emission from the SNR. The nonthermal radiation processes of the accelerated particles involved in an SNR are synchrotron radiation, bremsstrahlung, inverse Compton scattering (ICS) for leptons including electrons and positrons, and p–p interaction for protons; for the formulae of various radiation processes, see Zhang & Fang (2007).
In this model, model inputs include the distance d and the age T of the source, initial ejecta mass Mej, initial shock velocity v0, the maximum wavelength of magnetohydrodynamic (MHD) turbulence λmax, conversion efficiency η, electron/proton ratio Kep, the spectral index α, hydrogen density nISM and magnetic field strength BISM of the ISM, hydrogen density nMC and magnetic field strength BMC of the MC, and the fraction of the shell in the MC f. For each SNR, Equation (2) is respectively solved both with the parameters for the part of the shell evolving in the ISM and with those for the other part interacting with MCs, and then the multiband nonthermal spectra of the two parts can be calculated.
2.2. Revised Version of the ZF08 Model
As mentioned in Section 1, we make two modifications to revise the ZF08 model. The first modification concerns the volume-averaged production rates of the shocked-accelerated particles. Malkov et al. (2011) proposed a mechanism for the spectral break in the accelerated proton spectrum of an SNR. In this mechanism, the steepening of the energy spectrum of accelerated particles with exactly one power is produced by strong ion–neutral collisions in the surrounding remnant and the spectral break is caused by Alfvén wave evanescence leading to the fractional particle losses (Malkov et al. 2011). Following Malkov et al. (2011), therefore, we assume that the volume-averaged production rates of the shocked-accelerated particles have a broken power-law form with a break energy Eb, i.e.,
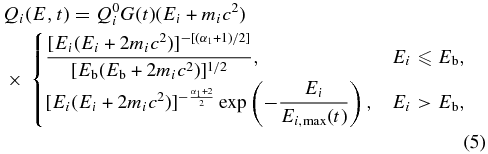
where Ei is the particle kinetic energy and α1 is the spectral index below the break energy Eb. Malkov et al. (2011) have shown that the break energy (or break momentum) depends on the magnetic field strength and ion density as well as on the frequency of ion–neutral collisions and given an approximate expression of the break momentum; however, the expression cannot make an accurate independent prediction of the position of the break in the gamma-ray emission region since the quantities in the expression are known poorly. As an example, therefore, they used the Fermi observations of the gamma-ray spectrum of SNR W44 (Abdo et al. 2010c) to determine the break momentum in the parent particle spectrum. Here, for a given SNR, spectral index α1 is constrained by observed radio spectral index αr and the break energy Eb is determined by the Fermi observations.
The second modification is the total amount of the kinetic energy contained in the injected particles that has been completely converted into the kinetic energy of both the electrons and protons during the time tci = ξtSed with a parameter ξ > 10, i.e.,
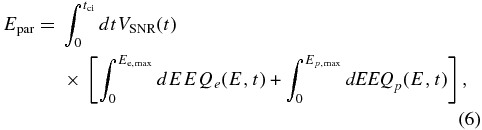
where VSNR(t) = 4πR3SNR(t)/3 is the SNR volume.
After making the above modifications, we can numerically calculate the isotropic intensities of the primary electrons, the primary protons, and the secondary e± pairs in the shell evolving in the ISM and in the shell interacting with the MC for a given SNR. The intensity is cut off at high energies by three mechanisms: the finite age of the SNR, energy-loss processes, and free escape from the shock region when the particles cannot be effectively scattered by MHD turbulence (Gaisser 1990; Reynolds 1996; Sturner et al. 1997). Particularly with MHD turbulence, a turbulent maximum wavelength is described as λmax = f0rL (f0 ∼ 10; see Zhang & Fang 2007); here, rL = Ei/eB is the particle gyroradius and B is the local magnetic field strength. Through these mechanisms, the maximum kinetic energies of electrons and protons, Ee, max and Ep, max, can be calculated with Equations (3)–(5) in Zhang & Fang (2007), which depend on the shock speed and age as well as on any of the above-mentioned loss processes.
In the revised model, we calculate nonthermal photon spectra by using the accelerated electron and proton intensities with a broken power-law particle injection. These photons can be produced by electron synchrotron radiation, bremsstrahlung, ICS, and neutral π0-decay gamma rays from the proton–proton interaction. The ambient soft photon fields of the ICS include the cosmic microwave background (CMB), Galactic IR emission from the warm dust, and the Galactic starlight field (Porter et al. 2008). The detailed process of the photon emission is shown in Fang & Zhang (2008). Based on Kamae et al. (2006), the gamma-ray spectrum of π0 decay is calculated with a new scaling factor of 1.85 for helium and other heavy nuclei (Mori 2009).
3. APPLICATIONS
In this section, we apply the model to four middle-aged SNRs IC443, W51C, W28, and W44 interacting with the surrounding MC. The model parameters used in our calculations for these SNRs are listed in Table 1, where the initial shock velocity v0, conversion efficiency η, the maximum wavelength of MHD turbulence λmax, local ISM density nISM, and MC hydrogen density nMC are fixed to be 109 cm s−1, 0.1, 5 × 1016 cm, and 0.1 cm−3 respectively for these SNRs. The comparisons of our calculating results with observed data are shown in Figures 1–4. In these figures, we indicate nonthermal photon spectra through various radiation mechanisms under the circumstances that the SNR shock interacts with the ISM: the dashed, dot-dashed, and dotted lines represent the spectra through synchrotron emission, ICS, and bremsstrahlung, respectively; double-dot-dashed lines express the spectra from π0-decay process; and thin solid lines represent the summation of all the above processes in the ISM. For the clarity of the figure, only the summation for all the above-mentioned radiations from the shock shell interacting with the MCs is shown (thick solid lines), whereas the individual radiative spectrum, such as synchrotron radiation, bremsstrahlung, ICS, and neutral π0-decay emission from the part interacting with the MCs, is not given in these figures.
Figure 1. Comparison of the predicted SEDs with the observed data for SNR IC443. The radio emission (from Erickson et al. 1985) is explained by synchrotron radiation from the SNR shock evolving in the ISM, while the gamma-ray emission is from the combinations of bremsstrahlung (dotted line), IC scattering (dot-dashed line), and π0-decay (double-dot-dashed line) owing to the SNR shock interacting with MC. Gamma-ray data are released by the EGRET (Hartman et al. 1999), Fermi LAT (Abdo et al. 2010a), MAGIC (Albert et al. 2007), and VERITAS (Acciari et al. 2009). Details of the model are described in the text.
Download figure:
Standard image High-resolution imageFigure 2. Comparison of the predicted SEDs with the observed data for SNR W51C. All the curves are stated to be the same as in the caption of Figure 1. The physical parameters are shown in Table 1. The multiband data are from the observations in radio (Moon & Koo 1994) and high-energy gamma rays (Abdo et al. 2009). Details of the models are described in the text.
Download figure:
Standard image High-resolution imageFigure 3. Comparison of the predicted SEDs with the observed data for SNR W28. The multiband data are from the observations in radio (Dubner et al. 2000) and high-energy gamma rays (Aharonian et al. 2008; Giuliani 2010; Abdo et al. 2010b). Details of the models are described in the text.
Download figure:
Standard image High-resolution imageFigure 4. Comparison of the predicted SEDs with the observed data for the SNR W44. The multiband data are from the observations in radio (Castelletti et al. 2007) and high-energy gamma rays (Abdo et al. 2010c). Details of the models are described in the text.
Download figure:
Standard image High-resolution imageTable 1. Model Parameters in Our Calculations
Model Parameter | IC443 | W51C | W28 | W44 |
---|---|---|---|---|
T (104 yr) | 3.0 | 3.5 | 4.0 | 2.0 |
d (kpc) | 1.5 | 6.0 | 2.0 | 3.0 |
Mej (M☉) | 1.5 | 6.0 | 3.0 | 6.0 |
BISM (μG) | 10 | 25 | 10 | 10 |
nMC (cm−3) | 50 | 100 | 100 | 100 |
BMC (μG) | 10 | 25 | 10 | 10 |
Kep | 0.012 | 0.012 | 0.01 | 0.01 |
ξ | 90 | 55 | 70 | 36 |
α1 | 1.7 | 1.5 | 1.7 | 2.0 |
Eb (GeV) | 40 | 10 | 3 | 10 |
f | 0.08 | 0.15 | 0.12 | 0.10 |
Notes. In our calculations, we assume the same values of the initial velocity v0, conversion factor η, the maximum wavelength of MHD turbulence λmax, and local ISM density nISM for these SNRs: v0 = 1.0 × 109 cm s−1, η = 0.1, λmax = 5.0 × 1016 cm, and nISM = 0.1 cm−3.
Download table as: ASCIITypeset image
SNR IC443 is a well-studied shell-type SNR with age 20–30 kyr (Bykov et al. 2008; Lee et al. 2008); it is located at a distance of 1.5 kpc (Gaensler et al. 2006) and listed as the core-collapse SNR G189.1+3.0 in Green's catalog (Green 2004). There is a complex composition of molecular and atomic clouds in the southern rim of the shell (Snell et al. 2005). The multiband data have been obtained at radio (Erickson et al. 1985), GeV gamma-ray (Esposito et al. 1996; Hartman et al. 1999; Abdo et al. 2010a), and VHE gamma-ray (Albert et al. 2007; Acciari et al. 2009) bands; specifically, the VHE gamma rays observed by MAGIC are correlated with an MC (Albert et al. 2007), and the total mass of the MC in the region is estimated to be ∼104 M☉ (Torres et al. 2003). We calculate the multiband spectral energy distribution (SED) of IC443 using our revised model and show the comparison of our results with observed radio data (Erickson et al. 1985), EGRET data (Hartman et al. 1999), Fermi LAT data (Abdo et al. 2010a), and VHE data detected by MAGIC (Albert et al. 2007) and VERITAS (Acciari et al. 2009) in Figure 1. The parameters involved in the calculation are shown in Table 1. In our calculation, we assume that the radio photons are produced by the synchrotron radiation of the accelerated electrons, so we can estimate the spectral index α1 = 1–2αr by using the observed radio spectral index, from the radio observations, αr = −0.36 ± 0.2 (e.g., Erickson et al. 1985), so α1 ≈ 1.7; on the other hand, we use the Fermi observations to estimate Eb and find Eb = 40 GeV. Therefore, we have the broken power-law form of the volume-averaged production rates of the shocked-accelerated particles with α1 = 1.7 and Eb = 40 GeV. With the parameter ξ = 90 listed in Table 1, we find that tci ≈ 41,270 years when the shell evolves in the ISM, and tci ≈ 5200 years for the shell interacting with the MC, indicating that the total amount of the kinetic energy contained in the injected particles could be more quickly converted into the kinetic energy of both the electrons and protons in the dense MC than that in the ISM. The soft photon fields of ICS include the CMB, Galactic IR emission from the warm dust, and the Galactic starlight field (two optical blackbody components); the temperatures and energy densities of these photon fields are 2.7, 25, 5000, and 10,000 K and UCMB = 2.5 × 10−7 MeV cm−3, UIR = 2.2 × 10−7 MeV cm−3, U5000 = 2.2 × 10−7 MeV cm−3, and U10, 000 = 2.2 × 10−7 MeV cm−3, respectively (Sturner et al. 1997). Moreover, Kep = 0.01 is used in our calculation which is approximately consistent with the CR composition observed at Earth (Abdo et al. 2010c). Note that gamma-ray emissivity for the bremsstrahlung and p–p interaction in the MC is proportional to f × nMC when the value of tci is fixed in the MC. Therefore, in order to produce the gamma-ray spectrum which can be comparable to the observed data, increasing nMC leads to decreasing f with a fixed tci. Here, f = 0.08 and nMC = 50 cm−3 are used, if we fix the value of tci in the MC, obviously a higher or lower value of nMC is possible to reproduce the observed data if a lower or higher value of f is used. From our calculation, the radio emission from the rim of the SNR is produced by the radiation from the shell evolving in the ISM, whereas the gamma rays detected by EGRET, Fermi LAT, MAGIC, and VERITAS are prominently from the π0 decay of the shell interacting with the MC.
SNR W51C (G49.2-0.7) is a radio-bright SNR with an estimated age of ∼3.5 × 104 yr at a distance of D ≃ 6 kpc (Koo et al. 2005). Koo & Moon (1997a, 1997b) have given evidence of MC-shock interaction. X-ray observations by ROSAT, ASCA, and Chandra indicate intense radio synchrotron emission in its shell and both shell-type and center-filled morphologies. An age of ∼3 × 104 yr and an explosion energy of ∼3.6 × 1051 erg for this SNR were estimated based on either the Sedov or the evaporative models (Koo et al. 1995). In the TeV gamma-ray band, an extended source HESS J1923+141 coincident with W51C has been found using HESS (Fiasson et al. 2009). In the GeV gamma-ray band, bright gamma-ray emission from W51C was observed (Abdo et al. 2009) and known to be interacting with an MC. The gamma-ray emission is spatially extended, broadly consistent with the radio and X-ray extent of W51C. Abdo et al. (2009) took into account constant particle injection over a period of ∼3 × 104 yr, and they found that the large luminosity of the shocked shell could be explained naturally by π0 decay with a large number of accelerated protons in dense environments. The initial mass of the ejecta for W51C is considered as ∼6 M☉. We calculate the multiband SED of W51C using our revised model and show the comparison of our results with observed radio data (Moon & Koo 1994), Fermi LAT data (Abdo et al. 2009), and VHE data detected by HESS (Fiasson et al. 2009) in Figure 2. In our calculation, α1 is constrained to be 1.5 by using αr ≈ −0.25 and Eb is constrained to 10 GeV by using the Fermi observations. The interstellar radiation fields for ICS include the CMB and two diluted blackbody components (IR and optical); the temperatures are shown as TIR = 2.7 K, TIR = 35 K, and TIR = 2900 K, corresponding to UCMB = 2.6 × 10−7 MeV cm−3, UIR = 9.0 × 10−7 MeV cm−3, and Uopt = 8.4 × 10−7 MeV cm−3, respectively (Porter et al. 2008; Abdo et al. 2009). We find that ξ = 55, which gives tci ≈ 40,039 years in the ISM and tci ≈ 4004 years in the dense MC with f = 0.15. From our calculation, the gamma ray is also explained as the contribution from the π0 decay of accelerated protons in dense MC. Correspondingly, the radio emission is from the shell interacting with the ISM.
SNR W28 (G6.4-0.1) has a mixed morphology characterized by a shell-like radio morphology and center-filled thermal X-ray emission, and located at a distance of ∼2 kpc; its age varies between 3.5 and 15 × 104 yr (Kaspi et al. 1993), here we take its age as 4.0 × 104 yr. Dubner et al. (2000) have shown that the shell-like radio radiation should be prominently from its northeastern region. The X-ray emission is from the northeast and southwest, and it also shows a limb-brightened shell morphology (Rho & Borkowski 2002). HESS observations of the W28 field have revealed four TeV gamma-ray sources coincident with MCs (Aharonian et al. 2008): HESS J1801−233 located along the northeastern boundary coincides with an MC interacting with W28, and other three sources, HESS J1800−240A, B, and C, located at the south of W28. Fermi LAT observed gamma-ray emission from two gamma-ray sources, 1FGL J1801.3−2322c and 1FGL J1801.3−2322c. The source 1FGL J1801.3−2322c is an extended source within the northeastern boundary of SNR W28, and extensively overlaps with the TeV gamma-ray source HESS J1801−233. Here we model the multiband emission from this region; the soft photon fields for ICS include the CMB, two infrared (TIR = 29, 490 K, UIR = 2.9 × 10−7, 5.3 × 10−8 MeV cm−3, respectively), and two optical components (TIR = 3600, 10,000 K, Uopt = 3.7 × 10−7, 1.3 × 10−7 MeV cm−3, respectively; Porter et al. 2008; Abdo et al. 2010b). In Figure 3, we show the comparison of our results with observed radio data (Dubner et al. 2000), Fermi LAT data (Abdo et al. 2010b), and VHE data detected by HESS (Aharonian et al. 2008). In order to reproduce the observed multiband spectrum, we obtain α1 = 1.7 from the observed radio spectral index αr ≈ −0.35 and Eb = 3 GeV from the Fermi observations. Moreover, ξ = 70 for this SNR, resulting in tci ≈ 40,446 years in the ISM and tci ≈ 4045 years in the MC environment.
SNR W44 (G34.7-0.4) is also a radio-bright SNR; over the age of the SNR (2 × 104 yr) with a mixed morphology, it is located in a complex region at a distance of ∼3 kpc. Castelletti et al. (2007) performed low-frequency observations of SNR W44 at 74 and 324 MHz using multiple configurations of the Very Large Array (VLA); the observation of W44 has shown a highly filamentary radio shell and centrally concentrated thermal X-ray emission, and there was no correlation found between the associated pulsar PSR B1853+01 and the surrounding SNR shell by analyzing the radio continuum spectrum. However, the brightest X-ray features located in the center region of the remnant are relative to low radio surface brightness (Rho & Petre 1998). The gamma-ray emission spatially associates with W44, and existing studies have shown that the SNR shock should be interacting with an external MC (Abdo et al. 2010c). We calculate the multiband SED of W44 using our revised model and show the comparison of our results with observed radio data (Castelletti et al. 2007) and Fermi LAT data (Abdo et al. 2010c) in Figure 4. The seed photons for ICS include infrared radiation with energy density of 9.3 × 10−7 MeV cm−3, optical radiation with 9.6 × 10−7 MeV cm−3, and the CMB with 2.6 × 10−7 MeV cm−3 as well as infrared photons from W44 itself with 6.9 × 10−7 MeV cm−3 (de Jager & Mastichiadis 1997; Abdo et al. 2010c). The model parameters are listed in Table 1. In order to reproduce the observed data, α1 = 2.0 since αr ≈ −0.5 and Eb = 10 GeV from the Fermi observations, i.e., a steep power-law injection above Eb is required. With ξ = 36 for this source, we have tci ≈ 26,207 years in the ISM and tci ≈ 2621 years in the MC environment.
4. SUMMARY AND DISCUSSION
In this paper, we have revised the model of nonthermal photon emission from a shell-type SNR with part of the shell interacting with an MC given by Zhang & Fang (2008) and then applied the model for four middle-aged SNRs IC443, W51C, W28, and W44. In this revised model, we assumed a broken power-law particle injection by assuming that the injected electrons have the same energy distribution as the protons, and that the total amount of the kinetic energy contained in the injected particles were completely converted into the kinetic energy of both the electrons and protons during the time ξtSed. The nonthermal photon emission consists of two components: one comes from the shell evolving in the ISM and another from the MC. The main conclusion is that the observed GeV–TeV gamma rays are produced both via the bremsstrahlung of the shell in the MC and via the p–p interaction because the high-energy protons interact with the ambient matter in the MC whereas the radio emissions from the rim of the SNRs are produced via synchrotron radiation in the shell interacting with the ISM.
In our calculations, we found that Kep ∼ 0.01 for these SNRs which is approximately consistent with the CR composition observed on Earth. Before the observations of Fermi LAT, the study of multiband (from radio to GeV–TeV bands) emission for the SNR interacting with MCs mainly focused on SNR IC443 since this SNR has been observed from the radio (Erickson et al. 1985) to GeV–TeV bands by EGRET (Hartman et al. 1999) and MAGIC (Albert et al. 2007). For example, Sturner et al. (1997) calculated the nonthermal spectra from IC443 in the ambient medium with nISM = 10 and nISM = 1 cm−3, respectively, by using a time-dependent SNR model with Kep = 0.625 and concluded that the synchrotron emission dominates in the radio band and the bremsstrahlung in the gamma-ray band (i.e., the contribution of the p–p collision to gamma rays is negligible). Bykov et al. (2000) proposed a model to describe the nonthermal emission from an SNR which is fully surrounded by the MC and applied to SNR IC443 and predicted that the spectrum has a sharp cutoff at ∼0.1 TeV which is not consistent with the observation. Zhang & Fang (2008) reproduced an observed multiband spectrum of SNR IC443 using the ZF08 model with the Kep = 0.1, which is larger than the observed value. For the other three SNRs, a simple model with a broken power-law particle injection can explain multiband nonthermal photon emissions (Abdo et al. 2009, 2010b, 2010c), where Kep ∼ 0.01 is used. For the model parameters, the maximum turbulence scale is the same for all four sources, the values (see Table 1) of the spectral index α1 of the broken power law can be estimated from the observed data in the radio band (αr = −0.36 ± 0.02 for IC443 (Erickson et al. 1985), αr ≈ −0.26 for W51C (Moon & Koo 1994), αr ≈ −0.35 for W28 (Dubner et al. 2000), and αr ≈ −0.5 for W44 (Castelletti et al. 2007)), and the break energy Eb can be constrained by the gamma-ray spectrum for a certain SNR (Abdo et al. 2009, 2010a, 2010b, 2010c). However, it is very difficult to accurately determine all physical parameters; future observation is expected to give more accurate model parameters.
The values of the factor f of the shell in the MC were adopted as 0.08, 0.15, 0.12, and 0.10 for SNRs IC443, W51C, W28, and W44, respectively, i.e., there is ∼10% of the SNR shell interacting with the MC for these four SNRs. When high-energy particles interact with the surrounding dense MC, two emission processes become very important: π0-decay gamma rays in p–p interaction and electron bremsstrahlung. The parameters nMC and f are certainly important to these two mechanisms, and f × nMC is proportional to the gamma-ray emissivity. A reasonable value of f × nMC should be given in order to reproduce gamma-ray spectra consistent with the observed data, thus increasing f can result in decreasing nMC on the condition that the value of tci is fixed in the MC and vice versa.
Finally, we would like to point out that although our model can reproduce the multiband spectral energy distributions from four middle-aged SNRs interacting with MCs, another kind of model that gamma rays produced in MCs results from π0 decay in the inelastic collisions of the accelerated protons which have escaped from a nearby SNR with MCs can also explain the GeV–TeV emission observed by Fermi LAT well (Torres et al. 2008; Gabici et al. 2009; Li & Chen 2010; Ohira et al. 2011). Therefore, further observed information are required to further understand the emission processes of these SNRs.
We thank the anonymous referee for very constructive comments that substantially improved the quality of this paper. This work is partially supported by the National Natural Science Foundation of China (NSFC 10778702, 10803005), a 973 Program (2009CB824800), the Foundation of Yunnan University (21132014, 2010YB043), and Yunnan Province under a grant 2009 OC.