ABSTRACT
Nitrogen abundance inhomogeneities are present among main‐sequence stars in globular clusters of the Milky Way. Since N‐rich cluster dwarfs are unlikely to have nucleosynthesized nitrogen within their own interiors, they presumably obtained their excess N from elsewhere. If the abundance inhomogeneities are due to the self‐enrichment of a globular cluster, then the earliest source of nitrogen could have been massive cluster OB stars and Wolf‐Rayet stars of type N. Several obstacles to WN stars' being a viable enrichment mode for globular clusters are discussed: (1) a W‐R phase may have been inhibited for metal‐poor OB stars, due to the low mass‐loss rates expected of their radiatively driven winds, and (2) unless globular clusters had top‐heavy stellar mass functions, their WN stars would have been too few in number to explain the amount of CNO‐processed material in their nitrogen‐rich, low‐mass stars. In contrast, it is pointed out that OB stars in their main‐sequence and supergiant phases of evolution might also eject CNO‐processed material even if they do not evolve through a WN phase, while W‐R stars have been discovered in some metal‐poor dwarf galaxies and starburst galaxies. In addition, low metallicities could favor the production of WN stars over WC stars, and this could explain why the abundance inhomogeneities in Milky Way globular clusters generally involve excesses of CNO‐processed material. One scenario is discussed in which globular clusters form at the interface between massive, colliding gas clouds. If such clouds have masses comparable to dwarf galaxies, with relative velocities characteristic of the Galactic halo, then the collision process could drive gas into an accreting protocluster at a rate that is sufficient to complete cluster formation within several million years. This timescale could allow nitrogen‐rich wind ejecta from WN or OB stars to be incorporated into later cluster star formation. The ram pressure associated with a colliding‐cloud environment might not only confine the stellar wind ejecta, but also funnel it into sites of active star formation within the protocluster. In this picture, globular clusters form as open systems with gas continuing to flow into them from a greater cloud‐merger environment while star formation is occurring. In such a circumstance, not only may cluster WN and OB stars have promoted enrichment, but wind ejecta from massive stars that are external to the protocluster may also have been acquired.
Export citation and abstract BibTeX RIS
1. INTRODUCTION
Differences in the abundances of the CNO elements are present among main‐sequence stars within a number of globular clusters (Hesser 1978; Hesser & Bell 1980; Bell et al. 1983; Briley et al. 1991, 1994, 2004a, 2004b; Cannon et al. 1998; Cohen 1999; Briley & Cohen 2001; Gratton et al. 2001; Harbeck et al. 2003; Da Costa et al. 2004; Carretta et al. 2004). Many main‐sequence stars in clusters such as 47 Tucanae and M71 have enhanced nitrogen abundances and exhibit strong CN bands in their spectra. These abundance inhomogeneities can be explained in several ways. If all of the stars in a cluster were born with identical abundances, then at a later time some may have acquired nitrogen‐enriched material, either by accretion of gas from an intracluster reservoir composed of stellar ejecta (D'Antona et al. 1983; Thoul et al. 2002) or by mass transfer from a binary companion (Bell et al. 1981; Campbell 1986; Denissenkov & Weiss 2001; Denissenkov 2004b). In such cases, the CN‐strong cluster stars acquired their abundance anomalies after their formation but prior to the red giant phase of evolution. An alternative is to set the enrichment event(s) even further back in time and to presume that CN‐strong stars formed from gas that had already been pre‐enriched in nitrogen. In one such scenario, proto–globular clusters brought about their own internal chemical evolution: high‐mass stars formed sufficiently early to eject enriched material into the intracluster gas medium before star formation was finished. This may be referred to as a "primordial enrichment" or a "self‐enrichment" scenario.
The chemical inhomogeneity of one globular cluster (GC) in particular, ω Centauri, involves elements heavier than silicon. As such, this object has provided some of the best evidence for heterogeneous internal enrichment by massive stars (e.g., Freeman & Rodgers 1975; Butler et al. 1978; Manduca & Bell 1978; Mallia & Pagel 1981; Cohen 1981; Gratton 1982; Brown & Wallerstein 1993; Norris & Da Costa 1995b; Norris et al. 1996; Suntzeff & Kraft 1996; Smith et al. 2000; Cunha et al. 2002; Origlia et al. 2003; Sollima et al. 2005). Although most other globular clusters of the Milky Way appear to be relatively homogeneous in the Fe‐peak elements (e.g., Suntzeff 1993), they are nonetheless typically heterogeneous in the abundances of C, N, O, Na, Mg, and Al (e.g., Kraft 1979, 1994; Freeman & Norris 1981; Smith 1987; Salaris et al. 2002; Gratton et al. 2004; and Denissenkov 2004a). Could these more typical abundance anomalies also be the products of inhomogeneous self‐enrichment induced by massive stars? Challenges for such an explanation are to identify the source of the nitrogen and other elements, such as sodium, that are particularly enhanced within CN‐strong stars, and to determine how these elements got incorporated into an episode of enriched star formation within a proto–globular cluster.
One source of nitrogen could be an initial cluster population of intermediate‐mass (∼3–8 M⊙) asymptotic giant branch (AGB) stars (e.g., Cottrell & Da Costa 1981; Denissenkov et al. 1998; Ventura et al. 2001, 2002; Yong et al. 2003; Ventura & D'Antona 2005a, 2005b). Such stars produce nitrogen via the CNO bicycle of hydrogen‐burning reactions, in addition to elements such as sodium via proton addition reactions within the CNO‐burning shell (Denisenkov & Denisenkova 1990; Langer et al. 1993; Cavallo et al. 1996). The degree to which they can account for the N‐Na‐Al element enhancements observed in CN‐strong cluster giants has been the subject of some debate (Denissenkov et al. 1997; Denissenkov & Herwig 2003; Herwig 2004; Fenner et al. 2004). Since the CNO bicycle reactions occur within stars of a wide range of masses, it seems reasonable to ask whether stars more massive than 8 M⊙ may have played a role in any internal enrichment of globular clusters.
Brown & Wallerstein (1993) and Wallerstein et al. (1987) discussed Wolf‐Rayet stars as possible sources for the very early enrichment of not only ω Cen, but also the bimodal‐CN globular clusters M4 and M13. Such stars could eject nitrogen‐ or carbon‐rich material into a protocluster via relatively massive winds before any intermediate‐mass AGB stars contributed. They may constitute the earliest available sources of nitrogen within a forming globular cluster.1
2. MASSIVE WN STARS AS ENRICHMENT SOURCES FOR GLOBULAR CLUSTERS
Wolf‐Rayet (W‐R) stars originate as high‐mass O stars. As a result of massive winds, the outer envelope of the O star is removed, exposing interior regions in which nitrogen has been produced via the CNO bicycle reactions. The star now enters the WN phase (Conti 1976; Abbott & Conti 1987). The nitrogen‐rich surface layers are now ejected by the stellar wind, and eventually a carbon‐rich zone is exposed where triple‐α helium burning has occurred. The star then enters the WC stage (e.g., Conti 1976; Maeder 1991; Lamers et al. 1991). While in the WN phase, many solar masses of nitrogen‐rich material can be ejected. The contribution of W‐R stars to the nitrogen enrichment of the Milky Way as a whole is considered to be much less than that from AGB stars (e.g., Liang et al. 2001; Meynet & Maeder 2002; Dray et al. 2003). However, if star formation within a proto–globular cluster ceases before intermediate‐mass stars enter the AGB phase, then WN stars may be a main option for cluster self‐enrichment in nitrogen.
However, a W‐R star enrichment scenario for globular clusters is expected to face a major obstacle. The mass‐loss rates of radiatively driven winds from massive stars (Abbott 1982) depend on wind opacity, and hence on metal abundance (Kudritzki & Puls 2000; Vink & de Koter 2005). On account of the low metallicities of proto–globular cluster (proto‐GC) O stars, mass‐loss rates may not have been high enough to remove sufficient amounts of their outer envelopes to expose the hydrogen‐burning region. Evolutionary synthesis models and observations indicate that over the metallicity range − 1.7<[Fe/H]< + 0.3, the relative number of W‐R stars to O stars decreases with decreasing metallicity (Maeder 1991; Maeder & Meynet 1994; Meynet 1995; Schaerer & Vacca 1998; Guseva et al. 2000). At the metallicity of the Small Magellanic Cloud, observations suggest that the minimum initial mass required to achieve a WN phase may be as high as 65 M⊙ (Massey 2003; Massey et al. 2003). Dray & Tout (2003) predict from stellar models that the lower limit to the initial mass of W‐R stars is ≥50 M⊙ at a metallicity of [Fe/H]< - 1.7, unless some form of mass loss in addition to radiatively driven winds can occur.
Despite such results, both WN‐ and WC‐type Wolf‐Rayet stars have been found in some low‐metallicity dwarf galaxies (Izotov et al. 2001), such as I Zw 18 (Izotov et al. 1997; Brown et al. 2002). This galaxy has a metallicity of 0.02 Z⊙, which is comparable to that of many globular clusters. Low‐metallicity O stars could become W‐R stars if they are members of binary systems and lose their outer hydrogen‐rich envelopes due to mass transfer. If such alternative avenues for substantial mass loss were available to massive proto‐GC stars, then WN enrichment might still have been viable. It is not known what the maximum mass was for stars forming within a metal‐poor globular cluster; if it was as high as 100 M⊙, say, then even single‐star progenitors may have been available to produce some WN stars within Milky Way globular clusters.
Among Local Group galaxies, the number ratio of WC/WN stars appears to broadly correlate with metallicity, although there is scatter (Maeder & Conti 1994; Massey 2003). This trend has been matched by stellar models that incorporate metallicity‐dependent mass‐loss rates (Maeder 1991; Maeder & Meynet 1994). A prevalence of WN stars relative to WC stars at low metallicities would be consistent with a W‐R star enrichment scenario for globular clusters, because cluster CN‐strong stars invariably owe their enhanced CN bands to high abundances of nitrogen rather than carbon.
Meynet (1993) used stellar models to study theoretically the evolution of massive stars through the W‐R phase following an instantaneous burst of star formation. His results for a metallicity of Z = 0.001 could be relevant to the incidence of W‐R stars within a proto–globular cluster. He finds that at such a low metallicity, the W‐R star phase in the evolution of a starburst lasts about 0.6 × 106 yr, much less than the 4 × 106 yr phase for a solar‐metallicity starburst. In addition, since only very massive stars evolve through a W‐R phase at the lower metallicity (assuming only single stars), the variety of W‐R subtypes that are formed is much more restricted than at solar abundance. The only phase of W‐R evolution that the metal‐poor massive stars pass through is the so‐called WNL phase, the "entrance stage in the WR phase." Thus, in Meynet's (1993) model, a starburst with a metallicity of Z = 0.001 would not produce WC stars. The WNL phase starts at a time of 2.8 × 106 yr after the burst of star formation, peaks at 3.0 × 106 yr, and is all over after 3.4 × 106 yr. Thus, if globular clusters did sustain enrichment by W‐R stars early in their history, then nitrogen rather than carbon enrichment would be anticipated. This phenomenon is also indicated by the models of Meynet & Maeder (2005), which show that a 40 M⊙ star with a metallicity of Z = 0.004 will explode as a Type II supernova before entering a WC phase. As noted in the previous paragraph, a preponderance of WN rather than WC stars having contributed to self‐enrichment of Milky Way GCs would be in accord with the abundance properties of cluster CN‐strong stars.
Wolf‐Rayet stars, including both WN and WC varieties, have been discovered in super star clusters of starburst galaxies (Schaerer et al. 1997; Johnson et al. 2000; Origlia et al. 2001; Chandar et al. 2004; Buckalew et al. 2005). One of the central star clusters of NGC 5253 is located near nitrogen‐rich regions, leading Schaerer et al. (1997) to suggest that the cluster WN stars are sources of this nitrogen enrichment. On the other hand, Kobulnicky & Skillman (1996, 1997) found no evidence for massive star clusters' having produced any nitrogen or oxygen enrichment of the interstellar medium in several starburst galaxies. Their data indicated no "localized chemical self‐enrichment ... from massive star evolution." Furthermore, they found that starburst galaxies with W‐R features in their integrated spectrum have the same N/O ratios and He abundances as galaxies that do not exhibit spectroscopic W‐R signatures. They concluded that whereas abundance enhancements "must occur on long timescales and global spatial scales, we take the absence of significant differences between W‐R and non–W‐R galaxies as evidence that W‐R stars are not a significant contributor to abundance fluctuations on timescales comparable to the [duration] of the H ii regions." If globular clusters of the Milky Way were able to sustain WN star enrichment, then super star clusters in "nearby" starburst galaxies may not provide an appropriate analog.
3. SOME LOGISTICAL CONCERNS
How much nitrogen is required to be manufactured by massive stars in a primordial enrichment scenario? A CN‐weak star with a nitrogen‐to‐iron ratio of [N/Fe] = 0.0 dex in a globular cluster with an iron abundance of [Fe/H] = -1.5 will have a nitrogen mass fraction of 3.45 × 10-5 [for a solar 14N mass fraction of Z(14 N) = 1.10 × 10-3]. A CN‐strong star in a globular cluster may have nitrogen enhancements of 1.0 dex relative to the CN‐weak stars, such that the nitrogen mass fraction will be 3.45 × 10-4. If we have a globular cluster with a total mass Mcl, in which there are equal numbers of CN‐strong and CN‐weak stars, then the total excess mass of nitrogen in the CN‐strong population relative to the CN‐weak population is 1/2(3.45 - 0.35) × 10-4 Mcl = 1.55 × 10-4 Mcl. This is the amount of nitrogen required to be produced by the enriching massive stars. According to the calculations of Kobulnicky & Skillman (1997), a starburst of mass 3 × 105 M⊙ with a metallicity of Z = 0.004 and a Scalo (1986) power‐law stellar mass function would produce ≈8 M⊙ of nitrogen, such that the mass of nitrogen produced per unit mass of stars formed is ≈ 2.7 × 10-5. This is about a factor of 5 too low to account for the nitrogen content of the CN‐strong stars in a globular cluster. This suggests that for a massive‐star enrichment scenario to be successful for GCs, a stellar mass function more heavily weighted to high‐mass stars may be necessary.
To get a further idea of the amount of N‐enriched material that might be ejected by metal‐poor WN stars, we refer to the calculations of Meynet & Maeder (2005). They have computed stellar models for metallicities of Z = 0.008 and 0.004, which would be applicable for globular clusters with the relatively high metallicities of [Fe/H] = -0.4 and −0.7, respectively. These metallicities are encompassed by the higher abundance peak of the bimodal metallicity distribution found among Milky Way globular clusters (e.g., Zinn 1985). Bimodal CN distributions and CN‐strong stars have been found in several clusters of this latter metallicity, including M71 and the archetypal 47 Tuc. Thus, the Meynet & Maeder (2005) models seem to be applicable to W‐R star enrichment of the disk or bulge population of globular clusters.
The Meynet & Maeder (2005) model of a 40 M⊙ star with metallicity Z = 0.008 and initial rotation speed of 300 km s−1 enters the WN phase when its mass has decreased to ∼28 M⊙, having first evolved through both the O main‐sequence and blue supergiant (BSG) phases. The model enters the WC phase when the mass reaches ≈18 M⊙. In this model, therefore, some 10 M⊙ of nitrogen‐enriched material would be ejected during the WN stage. The nitrogen enhancement at the surface of the model while in the WN phase is 1.1 dex greater than the original abundance. However, during much of the preceding BSG phase, the surface nitrogen abundance is still very high, having achieved an enhancement of 0.8 dex by the time that the star's mass has dropped from the initial 40 M⊙ to 35 M⊙. Consequently, while the star is in the BSG phase, it loses some 7 M⊙ of material that is enhanced by ≈0.8 dex in nitrogen. So in total, this 40 M⊙ star loses ≈17 M⊙ of material in which nitrogen is enhanced by factors of 0.8–1.1 dex. The Meynet & Maeder (2005) model with an initial mass of 40 M⊙ and metallicity Z = 0.004 attains a surface nitrogen enhancement of 0.8 dex when its mass has dropped to 36 M⊙ during the early BSG phase of evolution. It then arrives at the WN stage when the mass has dropped to ≈27 M⊙ (which is only 1 M⊙ different from the Z = 0.008 model at the equivalent stage of evolution). According to Figure 14 of Meynet & Maeder (2005), this lower metallicity model can eject about 14 M⊙ of material with a nitrogen enhancement of 0.8 dex or more. It explodes as a Type II supernova when its mass has dropped to 22 M⊙, before entering a WC phase of evolution.
To estimate the amount of N‐enriched material that might be ejected by a population of massive proto‐GC stars, we make a number of simplifying assumptions based on the Z = 0.004 models of Meynet & Maeder (2005). At this metallicity, Meynet & Maeder (2005) find that the minimum initial mass a star must have to enter a W‐R phase of any type is 32 M⊙. Meynet & Maeder (2005) computed an initial‐final mass relation for massive stars of a variety of metal abundances. At their lowest metallicity of Z = 0.004, stars with initial masses of 32 and 60 M⊙ have final masses of 19 and 29 M⊙, respectively. We assume that at this metallicity, such stars are still in a WN phase of evolution when the final mass is reached.
A cluster O star loses an envelope mass of mw via stellar wind before entering on the early BSG phase, in which the surface nitrogen abundance becomes enhanced by 0.7 dex. The BSG phase is followed by a WN phase. While in both of these states, the star is assumed to lose material via a stellar wind that is enhanced in nitrogen by 0.7 dex or more. The star eventually undergoes core collapse while still in the WN phase, when its mass is further reduced by winds to mcc. Thus, the amount of CNO‐processed material that is ejected during the combined BSG and WN phases is mCNO = m - mw - mcc. Taking the models of Meynet & Maeder (2005) as a guide, a 40 M⊙ star acquires a surface nitrogen enhancement of ≈0.7 dex when it has lost mw ≈ 4 M⊙. It enters the blue supergiant phase slightly before this point, when its mass reaches ≈37 M⊙. When the mass drops to mcc = 22 M⊙, it becomes a core‐collapse supernova. Thus, the amount of nitrogen‐enhanced material that is ejected is mCNO = 40 M⊙ − mw - mcc = 14 M⊙, as noted in a previous paragraph. In the general case of other Z = 0.004 stars with initial masses in the range of 32 to 60 M⊙, we take mw = 4 M⊙ to be a constant that is independent of the original stellar mass. We assume that mcc is dependent on the initial stellar mass, with values of 19, 22, and 29 M⊙ for initial masses of 32, 40, and 60 M⊙, respectively. Approximating these values with a linear initial‐final mass relation gives mcc = 8.0 + 0.35m, where the units are solar masses. Adopting mw = 4 M⊙ as a constant, the amount of CNO‐processed material ejected by a Z = 0.004 star of initial mass m is
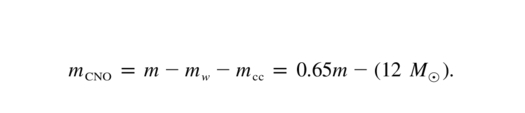
In the cases of stars with initial masses of 32, 40, and 60 M⊙, this relation estimates that the amount of CNO‐processed material ejected by WN/BSG winds is 8.8, 14, and 27 M⊙, respectively.
We suppose that massive stars formed as part of a first stellar generation within a proto–globular cluster. This first generation we also associate with the CN‐weak stars seen today in Milky Way globular clusters. The total mass of CNO‐processed material ejected by this generation of massive cluster stars is
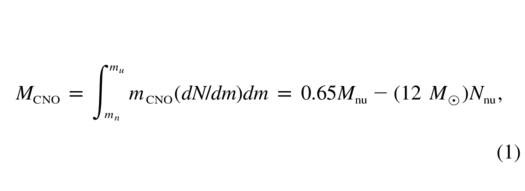
where (dN/dm) is the mass spectrum of the first stellar generation, dN being the number of stars with masses in the range m to m + dm, mu is the upper mass limit, mn is the lower limit to the initial mass of stars that evolve through a WN phase, Mnu is the total mass in "first generation" stars with initial masses between mn and mu, and Nnu is the original number of these stars. Both Mnu and Nnu will be proportional to the initial total mass M1 of the first stellar generation. The nitrogen‐rich ejecta from the first‐generation WN/BSG stars is assumed to be incorporated into a second generation of stars that form within the protocluster, the so‐called CN‐strong generation. The total mass of N‐enhanced gas ejected by first‐generation WN/BSG stars is then to be compared with the total mass in a typical population of CN‐strong stars from a globular cluster.
Cluster stars of both the first and second generations are assumed to have formed according to a stellar mass spectrum that was a power law of the form
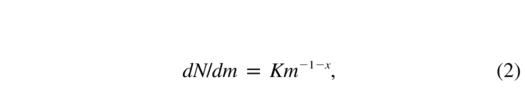
where K is a constant and the parameter x is taken to be a constant over the full range of stellar masses. The upper and lower limits to the mass function, mu and ml, as well as the value of x, are taken to be the same for both stellar generations. The mass of CNO‐processed material ejected from first‐generation WN/BSG stars now becomes
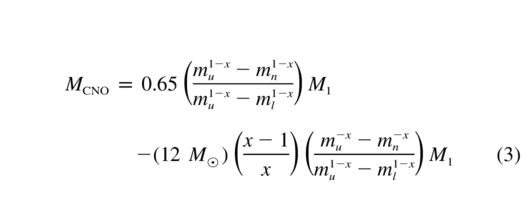
for x≠1,0.
We denote the total mass of second‐generation CN‐strong stars that are formed as M2. On the order of half of this mass must consist of enriched CNO‐processed ejecta if the observed abundances of CN‐strong stars are to be accounted for. This can be concluded from several facts. First, the nitrogen abundances of CN‐strong stars in a globular cluster can typically be ∼0.8–1.0 dex greater than in CN‐weak stars of the same magnitude in the same cluster (e.g., Da Costa & Cottrell 1980; Norris et al. 1981; Briley 1997; Briley & Cohen 2001; Cohen et al. 2002). Such enhancements are within 0.3 dex of the maximum enhancement that can be expected from the pure CNO‐processing of material with a Population II mix of C:N:O abundances. In addition, the CN‐strong stars often have carbon abundances that are some 0.3 dex less than that of CN‐weak stars of comparable magnitude (e.g., Da Costa & Cottrell 1980; Norris et al. 1981; Briley 1997). If the CN‐strong stars in a cluster consist of an admixture of primordial material having the same composition as the CN‐weak stars combined with CNO‐processed material that has a negligible carbon abundance, then both the nitrogen and carbon abundance patterns require the CN‐strong stars to be roughly half primordial material and half processed material. Therefore, if the mass MCNO of enriched material is distributed uniformly throughout the second‐generation stars, then the observed abundances of the CN‐strong stars require that MCNO/M2 ≈ 1/2.
The ratio between the number of CN‐strong and CN‐weak stars shows substantial variation among bimodal‐CN clusters (e.g., Norris 1987). In many clusters, CN‐strong stars are more numerous than CN‐weak stars, while in others it is the latter that are more common (e.g., Smith 2002). We consider here a cluster in which there are equal numbers of first‐generation CN‐weak stars and second‐generation CN‐strong stars. Combined with the assumption that the values of mu and ml were the same for both generations, we can then set M1 = M2 and obtain the mass ratio MCNO/M2. This ratio has been calculated for stellar mass limits of ml = 0.1 M⊙, mn = 32 M⊙, and mu = 60 M⊙, and is plotted as a function of x in Figure 1 (solid line). In order to have MCNO/M2∼ 1/2, a very extreme and potentially unlikely stellar mass function with x∼0 would be required.
Fig. 1.— Mass of nitrogen‐rich CNO‐processed ejecta MCNO from various first‐generation stars relative to the mass M2 of enriched second‐generation stars as a function of the power‐law exponent x of the stellar mass function. In all calculations, the present‐day main sequence is taken to be equally populated by first‐ and second‐generation stars as a function of mass. The upper and lower mass limits for first‐generation stars are mu = 60 M⊙ and ml = 0.1 M⊙ for all calculations. The same lower mass limit is adopted for the second‐generation stars; however, two different assumptions have been made about the upper limit for second‐generation stars: the solid line corresponds to mu = 60 M⊙, while the dashed and dotted lines are for mu = 0.8 M⊙. The solid and dashed lines correspond to enrichment by WN/BSG stars with initial masses of m≥32 M⊙. The dotted line is for the case of enrichment by AGB stars with masses in the range 3–7 M⊙.
One way to make a W‐R star enrichment scenario more viable would be to assume that the total mass incorporated into the CN‐strong population was less than that of the CN‐weak "primordial" population. As an alternative to assuming that M2 = M1, let us suppose that only low‐mass stars with m≤0.8 M⊙ formed as part of the CN‐strong population. If in addition the CN‐strong and CN‐weak stars are distributed in equal numbers along the present‐day main sequence (i.e., they have the same main‐sequence mass function), then the total mass M2 of second‐generation stars is equal to the total mass of first‐generation stars with masses less than 0.8 M⊙, so that
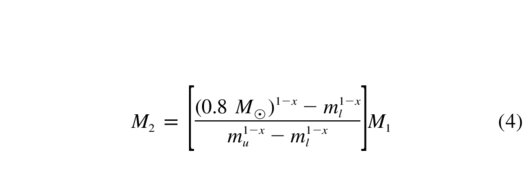
for x≠1. The ratio MCNO/M2 as a function of x is shown as the dashed curve in Figure 1. In order to obtain MCNO/M2 ≈ 1/2, a mass function with x ≈ 0.7 is now required. Whereas this is notably less extreme than for the situation discussed in the previous paragraph, the required mass function is nonetheless still flatter than either the Salpeter (1955) or Scalo (1986) function. On the other hand, it is not inconsistent with the main‐sequence mass functions observed in some, but not all, Milky Way globular clusters (e.g., Djorgovski et al. 1993). However, whether these observations might be relevant to the initial mass function of high‐mass stars that formed in globular clusters is far from clear.
Another way to advance the prospects for a W‐R star self‐enrichment scenario would be to increase the quantity of nitrogen‐enriched material expelled from O and W‐R stars. Larger amounts of nitrogen might be ejected if deep mixing occurs during the O main‐sequence and supergiant phases of evolution. In fact, a substantial fraction of both main‐sequence and supergiant OB stars do show CNO element peculiarities that are indicative of enhanced N/C and N/O ratios (e.g., Lyubimkov 1984; Smith & Howarth 1994; Walborn 2003; Walborn et al. 2004; Morrell et al. 2005; Crowther et al. 2006). Such discoveries indicate that many OB stars experience deep mixing of CNO‐processed material from their interiors to their surfaces, even during the main‐sequence phase of evolution (Langer 1992; Lyubimkov 1996). Denissenkov (1994) and Denissenkov et al. (1999) explored the possibility that deep mixing within the radiative envelope of massive main‐sequence O and B stars could be capable of bringing CNO‐processed material from the core to the stellar surface. Such deep mixing could enhance the amount of nitrogen‐rich material ejected by massive proto‐GC stars. In such circumstances, low‐metallicity high‐mass stars could eject significant amounts of CNO‐processed material without even going through a WN phase.
We conclude from the logistics of nitrogen production that a massive‐star self‐enrichment scenario for globular cluster abundance inhomogeneities is not without difficulties. Either a top‐heavy mass function may be required for the first stellar generation, or else nitrogen production per O star must be increased above what has been adopted in the above calculations (or both). One might ask whether cluster self‐enrichment by intermediate‐mass AGB stars would be a better solution to such logistical issues. If it is assumed that all mass ejected from 3–7 M⊙ stars consists of CNO‐processed material (an extreme assumption) and that all of these stars evolve into 1.0 M⊙ white dwarfs, then we can calculate MCNO for such first‐generation AGB stars using the stellar mass spectrum given by equation (2). The resulting MCNO/M2 ratio as a function of x is plotted as a dotted line in Figure 1, where it is again assumed that M2 is given by equation (4), corresponding to the most favorable circumstance considered here for self‐enrichment. In the AGB case, where all 3–7 M⊙ first‐generation stars are able to contribute to self‐enrichment, MCNO/M2 = 1/2 requires that x<0.85 (the upper limit on x resulting from our estimate of MCNO also being an upper limit). This is only slightly higher than the limit on x for the analogous case of O/WN star enrichment. The AGB star enrichment scenario therefore fares only slightly better than the O/WN star scenario in this respect. Such issues for AGB star self‐enrichment of globular clusters have previously been highlighted by Smith & Norris (1982) and D'Antona (2003). A similar situation appears to pertain to a primordial helium‐enrichment scenario for ω Cen (Bekki & Norris 2006).
4. ELEMENTS OTHER THAN NITROGEN
In this paper, we have concentrated on the nitrogen enhancements of the CN‐strong stars in globular clusters; but as noted above, these stars also have related inhomogeneities in other elements as well. Examples of typical element patterns are provided by the clusters NGC 6752 and M13. In both systems, the Na and Al abundances exhibit large spreads, and both elements are enhanced in the CN‐strong, N‐rich stars, with oxygen being depleted, relative to the CN‐weak stars (Norris et al. 1981; Cottrell & Da Costa 1981; Norris & Pilachowski 1985; Pilachowski et al. 1996; Kraft et al. 1992, 1993, 1997; Norris & Da Costa 1995a; Cavallo & Nagar 2000; Gratton et al. 2001; Grundahl et al. 2002; Yong et al. 2003; Cavallo et al. 2004; Carretta et al. 2005; Cohen & Meléndez 2005; Johnson et al. 2005); i.e., the stars in both clusters exhibit O‐N, O‐Na, and O‐Al anticorrelations, with accompanying N‐Na and N‐Al correlations. In M13, the spread in [Na/Fe] is ∼0.8–1.0 dex (Pilachowski et al. 1996; Kraft et al. 1997; Cavallo & Nagar 2000; Johnson et al. 2005), similar to the range found in NGC 6752 by Grundahl et al. (2002) and Yong et al. (2003). Grundahl et al. (2002), Yong et al. (2003, 2005), and Cavallo et al. (2004) measured a spread of ≥1.2 dex in Al in NGC 6752, comparable to the range in [Al/Fe] that Kraft et al. (1997), Cavallo & Nagar (2000), and Johnson et al. (2005) find at a given MV on the red giant branch of M13.
A broad [Mg/Fe]‐[Al/Fe] anticorrelation is seen in both M13 and NGC 6752 (Shetrone 1996a; Kraft et al. 1997; Grundahl et al. 2002; Yong et al. 2003), with a spread of ∼0.5–0.6 dex in [Mg/Fe] in M13 (Pilachowski et al. 1996; Shetrone 1996a; Kraft et al. 1997; Johnson et al. 2005) and ≈0.4 dex in NGC 6752 (Grundahl et al. 2002; Yong et al. 2003). The abundance pattern among the Mg isotopes is more complicated (Shetrone 1996b). In NGC 6752, Yong et al. (2003) found a significant star‐to‐star spread in the 24Mg:25Mg:26Mg ratios, with 24Mg and Al being mildly anticorrelated, while 26Mg and Al are correlated. There is little relation between 25Mg and Al. The fact that both 25Mg and 26Mg are enhanced relative to 24Mg compared to what is expected of metal‐poor supernovae led Yong et al. (2003) to suggest that AGB stars may have contributed to the Mg isotope abundances. Yong et al. (2006) find that the Mg‐Al isotope relations in M13 (see also Shetrone 1996b) are similar to those in NGC 6752.
Within the context of an early GC enrichment scenario, there has been considerable study of whether these N‐O‐Na‐Mg‐Al element inhomogeneities are the result of the nucleosynthetic activity of intermediate‐mass asymptotic giant branch (IM‐AGB) stars (e.g., Ventura et al. 2001, 2002; Ventura & D'Antona 2005a, 2005b; and other references cited in § 1), although this scenario is not without current problems (Denissenkov & Herwig 2003; Herwig 2004; Fenner et al. 2004). Could massive stars within globular clusters have contributed to the Na‐Mg‐Al inhomogeneities? Models of the nucleosynthesis within WN stars and their progenitors show that their winds will not only be enriched in 14N, but also in 23Na, because of the Ne‐Na cycle reactions in the convective core (Prantzos et al. 1986; Meynet et al. 2001). This could be consistent with the observation that CN‐strong stars are also enhanced in sodium.
Some relevant results on element nucleosynthesis within the hydrogen‐ and helium‐burning cores of very massive metal‐poor stars have come from the models of Meynet et al. (2006), who have also looked at the effects of rapid rotation on CNO‐element synthesis within such stars (see also Maeder & Meynet 2006). Their models of a rapidly rotating (vini = 800 km s−1) 60 M⊙ star with initial metallicity Z = 10-8 show that rotationally induced mixing can produce large surface enhancements in the CNO elements by the end of the core helium burning phase. These enhanced "metal" abundances lead to radiatively driven winds in the Meynet et al. (2006) models, and so they eject CNO‐processed elements into space. At low metallicities, unlike at solar abundance, they find that considerable amounts of primary 14N can be produced from the CNO‐processing of primary 12C that has been synthesized in the helium‐burning core.2 In their nonrotating model, the primary 13C and 14N enrichment of the stellar envelope is much less than in the rapidly rotating one, and the H‐burning shell is more convectively active in the rotating model. The amounts of 12C and 16O produced in the core are less sensitive to rotation, but Meynet et al. (2006) do find the 12C abundance to be "significantly smaller in the rotating model than in the non‐rotating one" at the end of the He‐burning phase, since 12C is partially converted to 16O.
Meynet et al. (2006) find that the amounts of 25Mg and 26Mg that are synthesized in their more rapidly rotating models are "greatly enhanced" relative to those of the nonrotating case. The production of these isotopes is enhanced as a by‐product of the diffusion of primary nitrogen into the He‐burning core in the rapidly rotating models, resulting in the production of 22Ne by α‐addition reactions. The 22Ne in turn produces 25Mg and 26Mg by further (α,n)‐ and (α,γ)‐reactions. Helium diffusion into the core at the end of the He‐burning phase can also enhance the 24Mg abundance, although this would need to be converted into the other Mg isotopes or Al in order to be consistent with the Mg‐Al anticorrelation observed in globular clusters. Meynet et al. (2006) find that neutrons generated by the 22Ne(α,n)25Mg reaction can be captured by iron‐peak elements to produce some amount of s‐process elements. In NGC 6752, Yong et al. (2005) find that variations in [Al/Fe] of up to 1.3 dex correlate with small spreads of ≈0.1 dex in some s‐process elements, such as Y, Zr, and Ba. While this might be attributed to IM‐AGB stars, the calculations of Meynet et al. (2006) suggest that synthesis in massive, rapidly rotating stars during the core helium burning phase could also be an option.
In a higher metallicity model that has Z = 10-5 and loses mass via a stellar wind, Meynet et al. (2006) find "strong enrichments in CNO elements" together with more moderate enhancements of 23Na and 27Al in the wind. Of particular relevance here is their finding that the "wind of rotating models is characterised by very low 12C/13C ratios, around 4–5 (close to the equilibrium value of the CN cycle) and by very high N/C (between about 3 and 40) and N/O ratios (between 1 and 36). Thus wind material presents the signature of heavily CNO‐processed material."
Nucleosynthesis of elements within the hydrogen‐ and helium‐burning cores of metal‐poor, rapidly rotating stars may therefore provide a scenario for the generation of globular cluster C‐N‐O and even Na‐Mg‐Al inhomogeneities by primordial enrichment. It may be able to mimic to some extent the effects of IM‐AGB star enrichment. A caveat to the above is that the Meynet et al. (2006) models are more metal‐poor than Milky Way globular clusters. It would be valuable to have studies of Na‐Mg‐Al nucleosynthesis for W‐R star models with metal abundances of Z∼0.002–0.0002.
Meynet et al. (2006) have emphasized the role of the initial rotation speed in determining the element patterns produced in massive stellar hydrogen‐ and helium‐burning cores. Their more rapidly rotating models seem to generate element enhancements that are qualitatively more consistent with globular cluster inhomogeneities than nonrotating models. There is a correlation between the incidence of CN‐strong stars in globular clusters and cluster ellipticity (Norris 1987; Smith 2002). If ellipticity reflects the bulk rotation of a globular cluster, this suggests that primordial enrichment was favored by a high angular momentum content. Could this be a by‐product of a larger fraction of massive stars that formed with preferentially fast rotation in clusters with high angular momentum?
The WN stars are observed to have enhanced helium/hydrogen ratios (e.g., Nugis & Niedzielski 1995). Hence, if they have driven globular cluster enrichment, then one might expect that the CN‐strong stars would have higher helium abundances than the CN‐weak stars. The possibility that stellar helium enhancements may be playing the role of a second parameter in governing the horizontal‐branch morphologies of some globular clusters has garnered attention in recent years (e.g., Lee et al. 2005). The highest metallicity population of stars in ω Cen may be enhanced in helium (Norris 2004; Piotto et al. 2005). The color distribution of main‐sequence stars in NGC 2808 has been interpreted in terms of a helium abundance spread (D'Antona et al. 2005). However, if a CN‐He correlation does prove to exist among stars within bimodal‐CN globular clusters, it does not necessarily provide verification of WN star enrichment, since the CNO bicycle of hydrogen burning will produce helium in any stellar interior.
Another element that appears to hold clues to the enrichment history of globular clusters is lithium. The abundance of Li has been studied in nine main‐sequence turnoff stars of NGC 6752 by Pasquini et al. (2005). They find that Li anticorrelates with N and Na while correlating with oxygen. As [N/Fe] ranges from +1.2 to +1.8 dex among their program stars, the lithium decreases by ∼0.4 dex. Pasquini et al. (2005) discuss how the observed trends are consistent with the N‐rich stars' being mixtures of "pristine" gas and CNO‐processed material, the later being depleted in lithium. They find that the Li‐N‐Na relations are in quantitative accord with the CNO‐processed material that has been synthesized within low‐metallicity IM‐AGB stars, as given by the models of Ventura et al. (2001, 2002). The nitrogen‐rich CNO‐processed material in the winds from BSG/WN stars would also presumably be depleted in lithium. Thus, at least at a qualitative level, an admixture of pristine plus BSG/WN material would be expected to show a Li‐Na anticorrelation relative to purely pristine material.
The difference in log N(Li) between CN‐strong and CN‐weak turnoff stars in NGC 6752 is similar to the difference in [C/Fe] between CN‐strong and CN‐weak giants of that cluster (Norris et al. 1981; Da Costa & Cottrell 1980); i.e., a factor of ≈0.3–0.4 dex. This is consistent with the former stars' being a roughly 1:1 mixture of pristine gas plus processed gas in which there is very little of either 12C or Li, due to the CNO process. As long as CN‐strong stars are admixtures of comparable amounts of pristine plus CNO‐processed material, they should still have a significant Li abundance, albeit one that is decreased below the primordial value. This point was also noted by Pasquini et al. (2005), who further emphasized that in the IM‐AGB star enrichment scenario, a "flat‐topped" initial stellar mass function is required, analogous to the W‐R star scenario in § 3.
Bonifacio et al. (2002) found that the lithium abundance among main‐sequence turnoff stars in NGC 6397 is uniform and equal to that of halo field dwarfs, despite the observation that the nitrogen abundance of these stars is enhanced. How this relates to the situation in NGC 6752 is unclear and awaits explanation.
The extent to which nucleosynthesis patterns of Li‐C‐N‐O‐Na‐Mg‐Al from rapidly rotating, massive, metal‐poor stars could imitate those of metal‐poor IM‐AGB stars seems worthy of further stellar modeling, such as that of Maeder & Meynet (2006). Even if it should prove that the IM‐AGB patterns provide the best fit for typical globular clusters, there may still have been a contribution from the winds of massive stars to some part of the chemical anomalies of GCs, possibly in cases of extreme nitrogen and/or helium enrichment, such as evinced by ω Cen (Norris 2004; Piotto et al. 2005) and NGC 2808 (D'Antona et al. 2005), and as discussed by Maeder & Meynet (2006).
5. WOLF‐RAYET STAR ENRICHMENT AND CONSTRAINTS ON GLOBULAR CLUSTER FORMATION TIMESCALES
A scenario of proto–globular cluster enrichment by first‐generation BSG/WN stars may place constraints on the timescale for star formation within a cluster. Since CN‐strong stars found in most globular clusters (excepting ω Cen and M22) are typically not enhanced relative to CN‐weak stars in the products of supernovae, it might be inferred that the period of CN‐strong star formation ceased before the first supernovae exploded. Indeed, it may have been these supernovae that shut down enriched star formation within a protocluster. It would then be necessary that BSG/WN ejecta get incorporated into the intracluster gas and into protostars prior to the explosion of the supernovae. The combined hydrogen‐ and helium‐burning phases of a 60 M⊙ star of metallicity Z = 0.001 take 4.1 Myr, according to the models of Schaller et al. (1992). At a metallicity of Z = 0.004, Meynet & Maeder (2005) find the equivalent time for a 60 M⊙ star to be 4.8 Myr if the rotation speed is 300 km s−1, and 5.5 Myr if it is 500 km s−1. In the case of a 120 M⊙ star with Z = 0.004 and a rotation speed of 300 km s−1, they find a time of 3.6 Myr. It is not known what the upper mass limit for globular cluster stars may have been, but if it was in the range of 60–120 M⊙, these models indicate that the earliest supernovae within a protocluster would have occurred some 4 Myr after the first massive stars formed. As noted above, in Meynet's (1993) metal‐poor (Z = 0.001), instantaneous‐starburst calculations, the first stars (of mass 120 M⊙) to enter the WNL phase do so at a time of 2.8 Myr after their formation, prior to the first supernovae. However, BSG stars can commence ejecting nitrogen‐enriched material via stellar winds before the start of this phase. Thus, there may be a period of ≈1 to 2 Myr in which CN‐strong stars could form in a globular cluster out of an internal gas reservoir enriched in the ejecta of BSG/WN stars. To form half of a 106 M⊙ globular cluster in such a period would require a star formation rate of 0.25 to 0.5 M⊙ yr−1.
Such star formation rates are relatively high but may not be unrealistic. Ashman (2003) concluded that "globular cluster formation occurs preferentially in regions in which star formation occurs at a high rate and efficiency." Elmegreen (2000) argued that clusters form on a free‐fall time. Taking this timescale for a spherical cloud of uniform density ρ to be
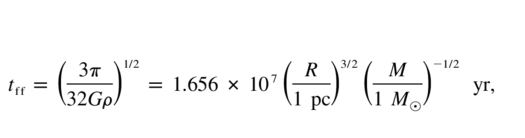
we find that for a cloud of mass M = 2 × 106 M⊙ and radius R = 10 pc, the free‐fall time is 7.4 × 105 yr. If a 106 M⊙ cluster were to form over such a time period, the resulting star formation rate of 1.4 M⊙ yr−1 would be even higher than implied by a WN star enrichment scenario. We conclude that if star formation endures for a period that is several times longer than tff, then even a high‐mass globular cluster could form before substantial numbers of supernovae exploded, while still leaving open the possibility that nitrogen‐rich wind ejecta from BSG/WN stars could be incorporated into an episode of CN‐strong star formation.
While a star formation rate on the order of 0.3 M⊙ yr−1 would not be unusual for the circumnuclear region of a starburst galaxy, it is much larger than that seen in the 30 Doradus giant H ii region, whose central cluster exhibits a star formation rate of ∼10−2 M⊙ yr−1 (Kennicutt 1998). However, it is comparable to the total star formation rates in many blue compact galaxies (Hopkins et al. 2002; Kong 2004), even in dwarf systems where the metallicity is relatively low (Fanelli et al. 1988; Hunt et al. 2005). We infer that proto–globular clusters would have been associated with significant starburst sources in the young Milky Way if a WN/BSG star enrichment hypothesis was applicable. This may accord with scenarios in which some globular clusters formed within dwarf galaxies (Zinnecker et al. 1988; Davies et al. 1998; Carraro & Lia 2000; Meylan et al. 2001; Hilker & Richtler 2000; Bekki & Freeman 2003). It could also accord with the Searle & Zinn (1978) scenario if their proposed GC progenitor clouds had masses that were comparable to dwarf galaxies, or with hierarchical construction of the Milky Way. Freeman (1993) has suggested that chemically enriched winds associated with the nuclei of dwarf elliptical galaxies could explain the chemical inhomogeneity in nitrogen and sodium observed within globular clusters.
If the first supernovae in a proto–globular cluster result from very high mass stars, then they may explode before some lower mass stars enter the WN phase. Thus, it may be that supernovae could truncate the ability of some lower mass WN stars to contribute to cluster nitrogen enrichment.3 If this were the case, then the logistics of the WN star enrichment scenario would be even more extreme than that presented in § 3. (For example, the calculations of Meynet & Maeder [2005] indicate that for 40 and 30 M⊙ stars of metallicity Z = 0.004, rotating at 300 km s−1, the core helium burning phase of evolution commences 5.7 and 7.1 Myr, respectively, after formation. Even if such stars were to go through a WN phase, they might still be in the O or BSG stages of evolution at the onset of supernova activity.) In such circumstances, a massive‐star enrichment picture for globular clusters may need to invoke nitrogen originating from WN stars exterior to a cluster, in addition to any internal contribution, as discussed in the next section.
6. MASSIVE‐STAR ENRICHMENT AND PROTOCLUSTER CLOUD COLLISIONS
One environment in which the chemical enrichment of globular clusters by massive stars might be particularly feasible is where proto‐GCs form within merging or colliding gas clouds. Young clusters seem to be a significant mode of star formation within merging galaxies (e.g., Ashman & Zepf 1993; Zepf & Ashman 1993; Schweizer 1997, 2003; Whitmore 2000). This suggests that colliding gas clouds may be sites of globular cluster formation. Collisions between massive gas clouds have been discussed as triggers for globular cluster formation by Murray & Lin (1993), Kumai et al. (1993), Fujimoto & Kumai (1997), Bekki et al. (2004), and Shigeyama & Tsujimoto (2004). Cloud collisions have also been implicated in the triggering of massive OB star formation and starbursts, and in star formation within metal‐poor protogalaxies (e.g., Struck‐Marcell 1982; Scoville et al. 1986; Olson & Kwan 1990; Habe & Ohta 1992; Elmegreen 1998; Sato et al. 2000; and Wang et al. 2004).
Cloud collisions could govern the star formation rate in a proto‐GC that forms at their interface by controlling the rate of gas influx. We make an order‐of‐magnitude estimate of the rates that might be achieved by supposing that two clouds of equal mass and radius collide with a relative velocity
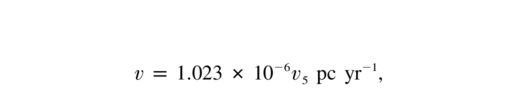
where v5 is the velocity in units of km s−1. If the collision has a small impact parameter, the duration of the collision will be on the order of
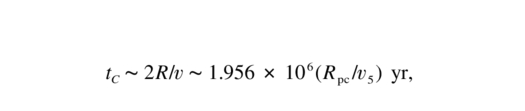
where Rpc is the radius of each cloud, in units of parsecs. We take each cloud to have a uniform density of
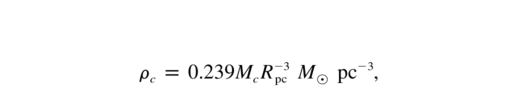
where Mc is the cloud mass, in units of M⊙. A globular cluster protocloud of radius rpc parsecs is assumed to form at the cloud collision interface. The projected area of the proto‐GC cloud is AGC = πr2pc. The rate of influx of mass into the protocluster region due to the cloud collision will be on the order of
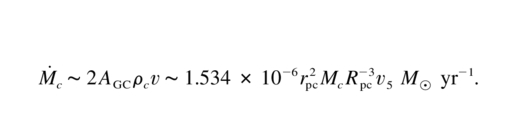
As an example, if we set Mc = 108 M⊙, Rpc = 100 pc, rpc = 5 pc, and v5 = 100 km s−1, then M ˙c∼0.38 M⊙ yr−1 and tC∼1.96 × 106 yr. If 50% of the inflowing gas is converted into stars, then the star formation rate is S∼0.19 M⊙ yr−1, and by the end of the collision, a protocluster of mass StC∼3.7 × 105 M⊙ may have accumulated. This is appropriate for a typical globular cluster of the Milky Way. The 2 Myr timescale set by the cloud collision could also allow nitrogen enrichment to be instigated by massive stars in the protocluster.
Since M ˙c scales as McR-3pc, a similar mass inflow rate applies for clouds with Mc = 107 M⊙ and Rpc ≈ 46.4 pc or Mc = 106 M⊙ and Rpc ≈ 21.5 pc. However, the mass of the cluster that is formed will scale as the collision time tC, which in turn scales as the cloud radius. So collisions between such clouds will give rise to clusters with smaller mass, but still on the order of 105 M⊙. Thus, for a range of colliding‐cloud masses and radii, we might anticipate cluster masses similar to those of the globular clusters of the Milky Way.
Colliding clouds could comprise a greater environment from which gas is funneled into any proto‐GC located near the cloud interface. The consequent influx of gas into the cluster region not only might sustain a high rate of star formation, but may also contribute to ram pressure confinement of the O and W‐R star winds. This may make the protocluster gas more resilient against disruption by these winds. The inflowing gas might entrain nitrogen‐rich wind material and bring it into star formation sites within the protocluster. The formation of a GC at a colliding‐cloud interface may also help to solve the nitrogen logistics problem identified in § 3. This scenario views a proto–globular cluster not as a closed system that is converting a single gas cloud into stars, but as an open system into which gas is being consistently input from an external environment. As such, the protocluster may be able to draw on nitrogen‐enriched material not only from its own WN/BSG stars, but also from any such stars that form outside the cluster and whose winds are swept up by the stream of gas constantly flowing into the cloud interface region. Bekki & Norris (2006) have proposed an external source for the helium enrichment of the massive cluster ω Cen, which they view as the remnant nucleus of a primordial dwarf galaxy.
Thus, a colliding‐cloud environment may support the production of an enriched second generation of stars within a proto–globular cluster. However, given the observational constraint that CN‐strong stars have essentially the same Fe‐peak element abundances as CN‐weak stars, it may be necessary to prevent the incorporation of supernova (SN) ejecta in the new stars being formed. As noted above, this may require that all star formation take place before the first cluster supernovae explode. The collision timescales calculated above seem consistent with this option if the inflowing gas can be rapidly turned into stars. Alternatively, the more energetic SN shells might expand out through certain regions of the protocluster where the gas densities or the ambient confining pressures are lowest, while in other regions, pristine gas may continue to be funneled in by the ongoing cloud collision.
Stellar evolution models suggest a further possibility. Perhaps any metal‐poor WN stars that did form within globular clusters were so massive that they did not experience normal Type II supernova events, but instead collapsed directly to black holes without a supernova‐inducing shock wave being launched. Figure 2 of Heger et al. (2003) indicates that very metal‐poor stars with initial masses in the range of 40–100 M⊙ may have evolved in this way (although other mechanisms can exist for exploding such stars as supernovae). In such a situation, WN star enrichment might not have been accompanied by Fe‐peak element or heavy α‐element enrichment until stars less massive than 40 M⊙ exploded as supernovae. If there were enough of these latter stars, their explosions may have shut down star formation within a protocluster and thereby brought about an end to the self‐enrichment epoch before significant numbers of low‐mass stars that were enhanced in elements such as calcium and iron could be formed.
Another option, with which we conclude this paper, is to allow that enrichment by supernovae did occur within globular clusters, and to incorporate WN or OB star winds into a more general self‐enrichment event.
7. SUPERNOVA SELF‐ENRICHMENT OF GLOBULAR CLUSTERS
Throughout the above discussions, we have made the tacit assumption that CN‐weak stars within a globular cluster have a chemical composition that is identical to that of the gas cloud or gas reservoir from which the protocluster first started to form. The similar abundances of elements heavier than silicon in both CN‐weak and CN‐strong stars then imply that neither group was enriched in ejecta from cluster supernovae. Cluster self‐enrichment in this context has been intended only as a means for enhancing a subpopulation of cluster stars in elements lighter than Si via the incorporation of wind material from massive WN and OB stars. However, the possibility might be considered that either all or some fraction of the heavy‐element content of globular clusters, including the Fe‐peak elements, resulted from self‐enrichment (e.g., Cayrel 1986). Morgan & Lake (1989), Brown et al. (1991, 1995), Parmentier et al. (1999), Parmentier & Gilmore (2001), Parmentier (2004), and Recchi & Danziger (2005) have explored various theoretical scenarios describing supernova‐induced metal enrichment of proto–globular clusters. In order for such models to be consistent with the uniform abundances of Fe‐peak and heavy α‐elements within Milky Way GCs, their extant low‐mass stars must have formed after the SN ejecta within each protocluster became largely homogenized.
It is possible that heterogeneous enrichment in WN and OB star winds could find a place in such scenarios. In fact, the very low metallicities of the Meynet et al. (2006) massive‐star models discussed in § 4 would make them more applicable to a supernova self‐enrichment scenario. However, cluster self‐enrichment in very heavy elements faces challenges from the observed similarity in the ratios of s‐process to r‐process elements as a function of [Fe/H] among cluster and field halo stars (James et al. 2004). By contrast, rapid enrichment by massive stars is expected to produce an r‐process–dominated abundance distribution, even in metal‐rich clusters (Truran 1988). This is not supported by the observations of James et al. (2004), whose [Ba/Eu] measurements "indicate clearly a growing contribution of the main s‐process to the chemical enrichment of GCs" with increasing [Fe/H]. On the other hand, inhomogeneous r‐process Eu abundances have been found in the globular cluster M15 (Sneden et al. 1997; Otsuki et al. 2006). These may possibly be due to self‐enrichment.
In a supernova self‐enrichment case, it would also be necessary to explain why the SN ejecta became more homogenized than the wind material. This might be attributed to either a greater dispersal of supernova ejecta or to the supernovae and nitrogen‐rich winds being contributed by stars of different masses. Perhaps the very high mass WN stars collapsed directly to black holes, whereas SNe were mainly produced by lower mass, 9–40 M⊙, stars (e.g., Heger et al. 2003). In such a circumstance, the nitrogen output from WN stars within a proto–globular cluster might have occurred first. The associated enrichment of the intracluster medium may have been inhomogeneous if the energies involved in WN star winds produced only limited dispersal. A more widespread enrichment of the intracluster gas that involved the Fe‐peak elements and most α‐elements may then have followed once Type II supernova activity occurred. This might have produced an intracluster gas medium throughout which many elements were smoothly distributed, but within which there were confined regions of nitrogen‐enhanced material. Following enrichment of the protocluster gas in this way, the low‐mass cluster stars may have formed. Alternatively, the homogeneous enrichment of protocluster gas in SN ejecta may have occurred first, followed by a heterogeneous influx of nitrogen‐rich gas from WN and OB stars exterior to the protocluster, such as might occur in a colliding‐cloud context.
Such enrichment histories for globular clusters are admittedly speculative; however, they may serve to illustrate how self‐enrichment models might be tailored to explain not only the homogeneity of the heavier metals, but also the heterogeneous abundances of elements lighter than silicon.
We thank the referee for a number of useful suggestions. The author is grateful for support from the National Science Foundation under grants AST 00‐98453 and AST 04‐06988.
Footnotes
- 1
Note added in proof: The winds of massive stars as sources of oxygen and sodium enrichment of globular clusters have recently been investigated by Prantzos & Charbonnel (2006).
- 2
According to Meynet et al. (2006), "in rotating models at solar metallicity, there is no primary nitrogen production due to the less efficient mixing at higher metallicity."
- 3
This difficulty could be mitigated to some extent by nitrogen ejection during the BSG phase of evolution.