Abstract
Carbon dioxide (CO2) is one of the primary greenhouse gases that contribute to climate change. Consequently, emission reduction technologies will be needed to reduce CO2 atmospheric concentration. Microalgae may have an important role in this context. They are photosynthetic microorganisms that are able to fix atmospheric CO2 using solar energy with efficiency ten times higher than terrestrial plants. The objectives of this study were: (i) to analyse the effect of light supply on the growth of Chlorella vulgaris and Pseudokirchneriella subcapitata; (ii) to assess the atmospheric CO2 capture by these microalgae; and (iii) to determine the parameters of the Monod model that describe the influence of irradiance on the growth of the selected microalgae. Both microalgae presented higher growth rates with high irradiance values and discontinuous light supply. The continuous supply of light at the highest irradiance value was not beneficial for C. vulgaris due to photooxidation. Additionally, C. vulgaris achieved the highest CO2 fixation rate with the value of 0.305 g-CO2 L−1 d−1. The parameters of the Monod model demonstrated that C. vulgaris can achieve higher specific growth rates (and higher CO2 fixation rates) if cultivated under higher irradiances than the studied values. The presented results showed that microalgal culture is a promising strategy for CO2 capture from atmosphere.
Similar content being viewed by others
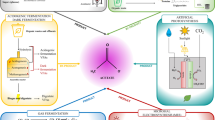
1 Introduction
A recent study identified some important planetary boundaries that must not be transgressed to avoid unacceptable environmental changes (Rockstrom et al. 2009). The continuous increase of atmospheric concentrations of greenhouse gases (mainly CO2) has been associated to perturbations on the climate (Rockstrom et al. 2009; Singh and Ahluwalia 2013). Consequently, several nations have recognized the need to shift to a low-carbon economy (Dovi et al. 2009; Shepherd et al. 2009; Pires et al. 2011). However, the progress in CO2 mitigation has been very slow and, even if CO2 emissions were immediately cut to zero, climate change would continue in the future due to long residence time of this greenhouse gas in atmosphere (Allen et al. 2009; Keith 2009; Shepherd et al. 2009; Moss et al. 2010; McLaren 2012). The application of geoengineering methods would be needed, which are divided in two groups (Pielke 2009; Shepherd et al. 2009; McLaren 2011): (i) carbon dioxide removal from atmosphere; and (ii) solar radiation management—reflexion of a small percentage of sun’s light and heat back into space. The first methodologies are preferable than the last ones, as they are able to return the climate system to its natural state (Singh and Ahluwalia 2013). Carbon dioxide removal methods include (Shepherd et al. 2009): (i) land use management (to protect land carbon sinks); (ii) the use of biomass (result of photosynthetic conversion of CO2) as carbon neutral energy source; (iii) enhancement of natural weathering processes to capture atmospheric CO2; (iv) direct engineered capture (physicochemical processes); and (v) enhancement of oceanic CO2 uptake. These methods may allow future reductions of atmospheric CO2 concentrations, reason to also be called negative emission technologies (NETs) (Keith 2009; Lemoine et al. 2012).
Currently, photosynthesis is the only practical form of air CO2 capture. With the constant increase of atmospheric CO2 concentrations, the enhancement of natural sinks can have a strong impact in the reduction of atmospheric concentrations (DuPont 2013). The largest carbon sink in the planet is the algae floating in the ocean that converts CO2 into biomass. Currently, it is estimated that they are responsible for capture of 12 Gt-CO2 yr−1 (Singh and Ahluwalia 2013). Afforestation and bioenergy with carbon capture and sequestration (BECCS) can have an important role in the atmospheric CO2 capture. Afforestation aims to increase biomass production, while BECCS aims to produce bioenergy followed by the capture of released CO2 (Obersteiner et al. 2001; Keith 2009; Lemoine et al. 2012). The main disadvantage of these biological methods is the requirement for land (Keith et al. 2006). Aiming to reduce the land use requirements, microalgal culture can be applied. These photosynthetic microorganisms use solar energy with efficiency ten times greater than terrestrial plants (Murakami and Ikenouchi 1997; Pires et al. 2012; Singh and Ahluwalia 2013). They are responsible for about 50 % of the world oxygen production (Chapman 2013; Singh and Ahluwalia 2013). Moreover, contrary to physicochemical processes for CO2 capture (absorption, adsorption, membrane separation and cryogenic distillation), microalgal culture have a final product (their biomass) with several applications (Chanakya et al. 2013; Chapman 2013; DuPont 2013; Gonçalves et al. 2013; Sing et al. 2013): (i) bioenergy production; (ii) food and feed production; (iii) pharmaceuticals; and (iv) cosmetics.
The process variables that could influence the success of microalgal cultivation are light distribution and saturation, temperature, pH, salinity, nutrient qualitative and quantitative profiles, dissolved oxygen concentration and presence of toxic elements (heavy metals) (Singh and Ahluwalia 2013). Light supply is one of the most important variables that influence the growth kinetics of microalgae. Thus, this study aims: (i) to analyse the influence of irradiance and light/dark ratio on the growth of Chlorella vulgaris and Pseudokirchneriella subcapitata; (ii) to assess the atmospheric CO2 capture by microalgae; and (iii) to determine the parameters of the Monod model that describe the influence of irradiance on microalgal growth. As far as it is known, there has been no previous research study focusing the analysis of light supply effect on CO2 capture from atmosphere by microalgae.
2 Materials and methods
2.1 Microorganisms and culture medium
Stock solutions of the freshwater green algae Chlorella vulgaris and Pseudokirchneriella subcapitata were prepared by previously described methods with the following composition (per liter) (OECD 2011): 12 mg MgCl2 · 6H2O, 18 mg CaCl2 · 2H2O, 15 mg MgSO4 · 7H2O, 1.6 mg KH2PO4, 0.08 mg FeCl3 · 6H2O, 0.1 mg Na2EDTA · 2H2O, 0.185 mg H3BO3, 0.415 mg MnCl2 · 4H2O, 3 μg ZnCl2, 1.5 μg CoCl2 · 6H2O, 0.01 μg CuCl2 · 2H2O, 7 μg Na2MoO4 · 2H2O, and 50 mg NaHCO3. Nitrogen was supplied in the form of NaNO3 for C. vulgaris, and in the form of NH4Cl for P. subcapitata (15 mg L−1). These algae strains were selected to compare their growth rate in the determined experimental conditions for selection of the best one for future research work. The cells were incubated in 500 mL flasks at room temperature, under continuous fluorescent light with an irradiance of 72 μE m−2 s−1 at the surface of the flasks. Agitation was obtained by bubbling filtrated (0.2 μm, Orange Scientific GyroDisc CA-PC) atmospheric air (flow rate of 1.5 L min−1) in the bottom of the flasks.
2.2 Experimental setup
Experiments were performed in 500 mL flasks (VWR, Germany) operating in batch with a working volume of 450 mL. Cells were cultivated for 12 days using the growth medium described above. The experimental conditions were the following: initial biomass concentration of 0.05–0.08 g L−1 (Taştan et al. 2013), room temperature (22 ± 1 ºC), and continuous aeration with the injection of atmospheric air in the bottom of the flasks. The assays were carried out under different light irradiance values: 36, 72, 96, and 126 μE m−2 s−1. For each irradiance value, different light cycles were evaluated: 10:14, 14:10, and 24:0 (light:dark). All the experiments were performed in triplicates.
2.3 Analytical methods
Irradiance was monitored using a light meter (IsoTech Lux-1335). Duplicate samples were collected at 24 h intervals and biomass concentration was determined by measuring optical density at 683 nm (OD683) (Kwon et al. 2005), using a V-1200 spectrophotometer provided by VWR company (Portugal). Each sample was diluted to give an OD683 in the range of 0.1–1.0 (assuming that the biomass concentration is linearly correlated with OD683). The relationship between optical density and the dry cell weight of C. vulgaris and P. subcapitata was previously determined. In different microalgal growth stages, simultaneous evaluation of OD683 and biomass concentration were performed and the linear relationships are given by linear regression: y = 1.8415x (R 2 = 0.9974) and y = 2.7318x (R 2 = 0.9928), respectively. The value y is the OD683 and the value x is the biomass concentration in g L−1. The pH of the cultures was also determined everyday using a HI 8424 pH meter (HANNA Instruments, USA).
2.4 Kinetic parameters
Cell concentration values were used to determine specific growth rates (μ, d−1), maximum biomass concentration (Xmax, g L−1), and maximum biomass productivities (Pmax, g L−1 d−1) of each microorganism. Specific growth rates were calculated by exponential regression during the logarithmic phase (Bailey and Ollis 1986). Biomass productivities (P) were calculated from the variation in biomass concentration (g L−1) within a cultivation time (d), according to the following equation:
where X 1 and X 0 were the biomass concentration (g L−1) on days t 1 and t 0, respectively. CO2 fixation rate (RC) was calculated based on the relationship with microalgal carbon content (CC) and biomass productivities (Jacob-Lopes et al. 2009), represented by:
Considering the typical molecular formula of microalgal biomass, CO0.48H1.83N0.11P0.01, each gram of microalgal biomass is equivalent to about 1.88 g of captured CO2 (Chisti 2007; Wang et al. 2008; Jacob-Lopes et al. 2009).
Growth rate values for different irradiance values (I) were then used to determine the kinetic parameters μ max and K I , according to the mathematical Monod model (Fergola et al. 2007), expressed by:
where μ max is the maximum specific growth rate and K I is the half saturation constant. This model was fitted to the experimental data (irradiance versus specific growth rates) using a non-linear minimization function (NonLinearRegress) of the software package Mathematica (Wolfram Mathematica 8). These parameters were chosen to minimize the χ 2 function given by the sum of squared residuals \( \sum {_ie_i^2} \).
2.5 Statistical analysis
For each parameter tested, the average and the standard deviation were calculated. The statistical significance of the results was evaluated using the Student’s paired t-test to investigate whether the differences between the controls and the actual tests could be considered significant. Additionally, 3-way factorial design was applied to evaluate if the three factors (algal species, light/dark ratio and irradiance) or their interaction were significant for Xmax, μ and Pmax. All statistical tests were carried out at a significance level of 0.05, using the statistical software SPSS 17.0 (SPSS Inc., Chicago, IL, USA).
3 Results and discussion
3.1 Biomass growth and productivity
Microalgal cultures were performed under photoautotrophic conditions, converting inorganic carbon into biomass by photosynthesis. Figure 1 presents the growth curves of C. vulgaris and P. subcapitata for different light conditions at room temperature and aerated with CO2 at atmospheric concentration, showing their different growth stages. For almost all cultures, it was observed the lack of an adaptation phase. The exponential phase started before completing the first day of culture. However, for a light/dark ratio of 10:14 and low light irradiance values, the adaptation phase was observed with both microalgal species. Generally, the stationary phase occurred at the seventh day of culture. Similar behaviour was observed by Jacob-Lopes et al. (2009), when analysed the effect of light cycles on cultures of the cyanobacterium Aphanothece microscopica Nägeli.
Table 1 shows the main kinetic parameters (μ; Xmax; and Pmax) for cultures of C. vulgaris and P. subcapitata. From t-test (p < 0.05), the maximum value for specific growth rate was achieved with C. vulgaris with constant supply of light energy (light/dark ratio of 24:0) with irradiance of 96 μE m−2 s−1 (0.738 d−1), which value was not statistically different (p > 0.05) from the ones obtained with the same light/dark ratio and irradiance of 126 μE m−2 s−1, and with the same irradiance value and the light/dark ratio of 14:10. Regarding the maximum biomass concentration, it occurred with the same microalga for the light/dark ratio of 14:10 and irradiance of 126 μE m−2 s−1 (0.821 g L−1, representing 10 times more the initial concentration of the culture). This kinetic parameter did not present significant differences varying the irradiance, but it was significantly different for other light/dark ratios. The achievement of the highest value in a discontinuous light supply (already observed in Fig. 1 for both microalgae) may be related with possible photooxidation (Molina et al. 2001; Chisti 2008). The oxygen generated by photosynthesis may accumulate in culture medium, reaching values that in combination with intense light can damage microalgal cells. During the dark period, microalgae do not perform photosynthesis and the oxygen may be released from the culture by the constant aeration. On the other hand, the cells get energy by oxidizing the compounds produced during the light period. Consequently, the O2 concentration in the culture decreases and the microalgae could repair the photo-induced damage (Merchuk et al. 1998; Carvalho et al. 2011). Taking into account the maximum biomass productivity, the highest value was obtained for the light/dark ratio of 14:10 and irradiance of 36 μE m−2 s−1 (0.162 g L−1 d−1) that did not statistically differ from the values obtained with other light/dark ratios (maintaining the irradiance value) and other irradiances (maintaining the light/dark ratio).
Three-way factorial design was applied using as main effects: (i) algal species with two levels (C. vulgaris and P. subcapitata); (ii) light/dark ratio with three levels (10:14, 14:10 and 24:0); and (iii) irradiance with four levels (36, 72, 96, and 126 μE m−2 s−1). Regarding specific growth rate, algal specie (p = 0.0064), light/dark ratio (p = 0.0002) and irradiance (p = 0.0036) were considered statistically significant, as well as the interactions between light/dark ratio with algal specie (p = 0.0271) and irradiance (p = 0.0461). However, concerning Xmax and Pmax, only the main effects were considered statistically significant: (i) algal species (p = 0.0039 and p = 0.0023, respectively); (ii) light/dark ratio (p = 0.0114 and p = 0.0333, respectively); and (iii) irradiance (p = 0.0048 and p = 0.0217, respectively).
3.2 Carbon sequestration
The determination of carbon sequestration rate was performed using an empirical chemical formula for microalgae proposed by Chisti (2007). This assumption was considered to avoid the elemental characterization of biomass for each experiment (24 experiments, excluding the replicates), as the chemical composition depends on microalgal species and culture conditions. Moreover, other authors have already applied this relationship to determine the CO2 capture by microalgae from the produced biomass (Wang et al. 2008; Jacob-Lopes et al. 2009). In this study, the maximum fixation rate was calculated based on the maximum productivity, achieving a value of 0.305 g L−1 d−1 for C. vulgaris, which is the same order of magnitude as values obtained in other research studies that aerated microalgal cultures with enriched CO2 streams (Jin et al. 2006; Jacob-Lopes et al. 2009; Tang et al. 2011; Yeh and Chang 2011).
The experimental results showed that microalgal culture is a promising methodology to integrate BECCS technology, contributing to negative carbon dioxide emissions. Capturing CO2 from atmosphere represents a cost reduction in microalgal cultures, as it is obtained for free and in any location (land use). However, to implement this technology in industrial scale, research based on the design of photobioreactors should be performed to reduce the energy required in the process, improving their sustainability. Renewable energy sources (solar and wind) could be coupled in microalgal cultivation to reduce the energetic dependence.
3.3 Monod model
The influence of irradiance on microalgal growth was modelled by Monod function (Eq. 3). Table 2 shows the model parameters that characterize each microalga growth with continuous illumination obtained by the non-linear minimization function. C. vulgaris presented higher maximum specific growth rate and half saturation constant than P. subcapitata. The half constant of 124.112 μE m−2 s−1 determined for C. vulgaris is almost equal to the maximum irradiance value applied in this study (126 μE m−2 s−1), which means that future studies with this microalga could be performed with higher light irradiance values to achieve higher growth rates and, consequently, CO2 removal efficiencies.
4 Conclusions
Chlorella vulgaris and Pseudokirchneriella subcapitata presented higher growth rates under high irradiances and discontinuous light supply. Based on the Monod model, C. vulgaris can significantly increase its growth rate (to almost double) if the culture is performed under higher irradiance. Regarding the CO2 capture from atmosphere, even under sub-optimal culture conditions, C. vulgaris achieved fixation rates (up to 0.305 g L−1 d−1) comparable to the ones obtained by other species from CO2 enriched streams. Thus, microalgal cultures showed to be a promising technology for capturing CO2 from atmosphere.
References
Allen MR, Frame DJ, Huntingford C, Jones CD, Lowe JA, Meinshausen M, Meinshausen N (2009) Warming caused by cumulative carbon emissions towards the trillionth tonne. Nature 458(7242):1163–1166
Bailey JE, Ollis DF (eds) (1986) Biochemical engineering fundamentals, 2nd edn. McGraw-Hill, Singapore
Carvalho AP, Silva SO, Baptista JM, Malcata FX (2011) Light requirements in microalgal photobioreactors: an overview of biophotonic aspects. Appl Microbiol Biot 89(5):1275–1288
Chanakya HN, Mahapatra DM, Sarada R, Abitha R (2013) Algal biofuel production and mitigation potential in India. Mitig Adapt Strat Gl 18(1):113–136
Chapman RL (2013) Algae: the world’s most important “plants”-an introduction. Mitig Adapt Strat Gl 18(1):5–12
Chisti Y (2007) Biodiesel from microalgae. Biotechnol Adv 25(3):294–306
Chisti Y (2008) Biodiesel from microalgae beats bioethanol. Trends Biotechnol 26(3):126–131
Dovi VG, Friedler F, Huisingh D, Klemes JJ (2009) Cleaner energy for sustainable future. J Clean Prod 17(10):889–895
DuPont A (2013) Best practices for the sustainable production of algae-based biofuel in China. Mitig Adapt Strat Gl 18(1):97–111
Fergola P, Cerasuolo M, Pollio A, Pinto G, DellaGreca M (2007) Allelopathy and competition between Chorella vulgaris and Pseudokirchneriella subcapitata: experiments and mathematical model. Ecol Model 208(2–4):205–214
Gonçalves AL, Pires JCM, Simões M (2013) Biodiesel from microalgal oil extraction. In: Lichtfouse E, Schwarzbauer J, Robert D (eds) Environmental chemistry for a sustainable world, vol 3. Accepted for Publication. Springer
Jacob-Lopes E, Scoparo CHG, Lacerda LMCF, Franco TT (2009) Effect of light cycles (night/day) on CO2 fixation and biomass production by microalgae in photobioreactors. Chem Eng Process 48(1):306–310
Jin HF, Lim BR, Lee K (2006) Influence of nitrate feeding on carbon dioxide fixation by microalgae. J Environ Sci Heal A 41(12):2813–2824
Keith DW (2009) Why capture CO2 from the atmosphere? Science 325(5948):1654–1655
Keith DW, Ha-Duong M, Stolaroff JK (2006) Climate strategy with CO2 capture from the air. Clim Chang 74(1–3):17–45
Kwon B, Park N, Cho J (2005) Effect of algae on fouling and efficiency of UF membranes. Desalination 179(1–3):203–214
Lemoine DM, Fuss S, Szolgayova J, Obersteiner M, Kammen DM (2012) The influence of negative emission technologies and technology policies on the optimal climate mitigation portfolio. Clim Chang 113:141–162
McLaren D (2011) Negatonnes—an initial assessment of the potential for negative emission techniques to contribute safely and fairly to meeting carbon budgets in the 21st Century. http://www.foe.co.uk/resource/reports/negatonnes.pdf. Cited December 2012
McLaren D (2012) A comparative global assessment of potential negative emissions technologies. Process Saf Environ 90(6):489–500
Merchuk JC, Ronen M, Giris S, Arad S (1998) Light/dark cycles in the growth of the red microalga Porphyridium Sp. Biotechnol Bioeng 59(6):705–713
Molina E, Fernandez J, Acien FG, Chisti Y (2001) Tubular photobioreactor design for algal cultures. J Biotechnol 92(2):113–131
Moss RH, Edmonds JA, Hibbard KA, Manning MR, Rose SK, van Vuuren DP, Carter TR, Emori S, Kainuma M, Kram T, Meehl GA, Mitchell JFB, Nakicenovic N, Riahi K, Smith SJ, Stouffer RJ, Thomson AM, Weyant JP, Wilbanks TJ (2010) The next generation of scenarios for climate change research and assessment. Nature 463(7282):747–756
Murakami M, Ikenouchi M (1997) The biological CO2 fixation and utilization project by RITE.2. Screening and breeding of microalgae with high capability in fixing CO2. Energ Convers Manag 38:493–497
Obersteiner M, Azar C, Kauppi P, Mollersten K, Moreira J, Nilsson S, Read P, Riahi K, Schlamadinger B, Yamagata Y, Yan J, van Ypersele JP (2001) Managing climate risk. Science 294(5543):786–787
OECD (2011) Freshwater alga and cyanobacteria, growth inhibition test, test guideline 201. Cited December 2012
Pielke RA (2009) An idealized assessment of the economics of air capture of carbon dioxide in mitigation policy. Environ Sci Policy 12(3):216–225
Pires JCM, Martins FG, Alvim-Ferraz MCM, Simoes M (2011) Recent developments on carbon capture and storage: an overview. Chem Eng Res Des 89(9A):1446–1460
Pires JCM, Alvim-Ferraz MCM, Martins FG, Simões M (2012) Carbon dioxide capture from flue gases using microalgae: engineering aspects and biorefinery concept. Renew Sust Energ Rev 16:3043–3053
Rockstrom J, Steffen W, Noone K, Persson A, Chapin FS, Lambin EF, Lenton TM, Scheffer M, Folke C, Schellnhuber HJ, Nykvist B, de Wit CA, Hughes T, van der Leeuw S, Rodhe H, Sorlin S, Snyder PK, Costanza R, Svedin U, Falkenmark M, Karlberg L, Corell RW, Fabry VJ, Hansen J, Walker B, Liverman D, Richardson K, Crutzen P, Foley JA (2009) A safe operating space for humanity. Nature 461(7263):472–475
Shepherd J, Caldiera K, Cox P, Haigh J, Keith D, Launder B, Mace G, MacKerron G, Pyle J, Rayner S, Redgwell C, Watson A (2009) Geoengineering the climate: science, governance and uncertainty. Cited December 2012
Sing SF, Isdepsky A, Borowitzka MA, Moheimani NR (2013) Production of biofuels from microalgae. Mitig Adapt Strat Gl 18(1):47–72
Singh UB, Ahluwalia AS (2013) Microalgae: a promising tool for carbon sequestration. Mitig Adapt Strat Gl 18(1):73–95
Tang DH, Han W, Li PL, Miao XL, Zhong JJ (2011) CO2 biofixation and fatty acid composition of Scenedesmus obliquus and Chlorella pyrenoidosa in response to different CO2 levels. Bioresour Technol 102(3):3071–3076
Taştan BE, Duygu E, İlbaş M, Dönmez G (2013) Utilization of LPG and gasoline engine exhaust emissions by microalgae. J Hazard Mater 246–247:173–180
Wang B, Li YQ, Wu N, Lan CQ (2008) CO2 bio-mitigation using microalgae. Appl Microbiol Biot 79(5):707–718
Yeh KL, Chang JS (2011) Nitrogen starvation strategies and photobioreactor design for enhancing lipid production of a newly isolated microalga Chlorella vulgaris ESP-31: implications for biofuels. Biotechnol J 6(11):1358–1366
Acknowledgments
A.L. Gonçalves and J.C.M. Pires are grateful to Foundation for Science and Technology (FCT), POPH-QREN and FSE for their fellowships SFRH/BD/88799/2012 and SFRH/BPD/66721/2009, respectively.
Author information
Authors and Affiliations
Corresponding author
Rights and permissions
About this article
Cite this article
Pires, J.C.M., Gonçalves, A.L., Martins, F.G. et al. Effect of light supply on CO2 capture from atmosphere by Chlorella vulgaris and Pseudokirchneriella subcapitata . Mitig Adapt Strateg Glob Change 19, 1109–1117 (2014). https://doi.org/10.1007/s11027-013-9463-1
Received:
Accepted:
Published:
Issue Date:
DOI: https://doi.org/10.1007/s11027-013-9463-1