Abstract
Key message
Rose has 19 MLO genes. Of these, RhMLO1 and RhMLO2 were shown to be required for powdery mildew infection, which suggests their potential as susceptibility targets towards disease resistance.
Abstract
Powdery mildew, caused by Podosphaera pannosa, is one of the most serious and widespread fungal diseases for roses, especially in greenhouse-grown cut roses. It has been shown that certain MLO genes are involved in powdery mildew susceptibility and that loss of function in these genes in various crops leads to broad-spectrum, long-lasting resistance against this fungal disease. For this reason, these MLO genes are called susceptibility genes. We carried out a genome-wide identification of the MLO gene family in the Rosa chinensis genome, and screened for allelic variants among 22 accessions from seven different Rosa species using re-sequencing and transcriptome data. We identified 19 MLO genes in rose, of which four are candidate genes for functional homologs in clade V, which is the clade containing all dicot MLO susceptibility genes. We detected a total of 198 different allelic variants in the set of Rosa species and accessions, corresponding to 5–15 different alleles for each of the genes. Some diploid Rosa species shared alleles with tetraploid rose cultivars, consistent with the notion that diploid species have contributed to the formation of tetraploid roses. Among the four RhMLO genes in clade V, we demonstrated using expression study, virus-induced gene silencing as well as transient RNAi silencing that two of them, RhMLO1 and RhMLO2, are required for infection by P. pannosa and suggest their potential as susceptibility targets for powdery mildew resistance breeding in rose.
Similar content being viewed by others
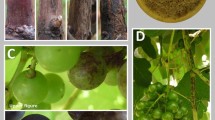
Introduction
Rose is one of the most important ornamental plants worldwide, holding great economic and cultural value. It was domesticated both in China and in Europe several thousand years ago for its application in gardens, use in medical treatment and usage for fragrance (Debener and Byrne 2014). Today, roses are widespread and are sold as the most popular garden plants or cut flowers with various colours, growth types and long-lasting flowering period. One of the reasons for this wide variation is the crossing of different rose species by a large number of breeders for a long period of time. Because of this activity, wild species with varying ploidy levels have contributed to the genome of tetraploid cut and garden roses (Koopman et al. 2008; Dubois and Sakr 2012; Zhang et al. 2013; Vukosavljev et al. 2013; Smulders et al. 2019).
Powdery mildew causes significant economic losses of agricultural crops as well as ornamental plants. In rose it is caused by Podosphaera pannosa. It was estimated that up to 40% of the pesticides applied on rose are used to control this disease (Debener and Byrne 2014). In order to reduce the use of pesticides, an alternative to overcome this problem can be an introgression program focusing on dominant plant resistance genes (R-genes); however, it can be difficult to transfer these genes into cultivated species due to interspecific crossability barriers (Fu et al. 2009). Furthermore, R-gene-mediated race-specific resistance may be easily overcome by new races of the pathogen in a short period (Pavan et al. 2010).
Recently, impairing plant susceptibility genes (S genes), which are plant genes contributing to susceptibility to pathogens, has been proposed as a novel breeding strategy (Pavan et al. 2010). The MLO (Mildew resistance Locus O) gene is a remarkable example of an S gene. It was first observed in barley that mutations in a particular MLO gene confer resistance to Blumeria graminis f. sp. Hordei (Jørgensen 1992; Piffanellii et al. 2004). As a typical plant S gene, the presence of the barley MLO gene is required for the powdery mildew infection. When the function of barley MLO gene is lost, local callose deposition occurs in the epidermal cells, causing the cell wall to thicken, so that the fungus fails to penetrate the epidermal cells of the plant to form a haustorium and obtain nutrients (Wolter et al. 1993). As a consequence, the plant acquires resistance to powdery mildew (Consonni et al. 2006; Eichmann and Huckelhoven 2008; Elliott et al. 2002; Kusch and Panstruga 2017). Loss of barley MLO gene function produced resistance to all known physiological races of barley powdery mildew, and mlo has been used in barley breeding since 1979 without being overcome by the pathogen (Büschges et al. 1997; Jørgensen 1992; Lyngkjaer et al. 2000). Following barley, natural mlo mutants have been identified in tomato, pea, melon and cucumber (Bai et al. 2008; Berg et al. 2015; Humphry et al. 2011; Nie et al. 2015; Cheng et al. 2015; Pavan et al. 2013). The pea mlo mutant (er1) has been worldwide used in breeding for powdery mildew resistance since it was first reported in 1948 (Harland 1948). These examples show that mlo-based resistance can be identified in various plant species and is effective to a broad-spectrum powdery mildew species. In a number of plant species, a reduced susceptibility to powdery mildew was generated by the knockdown of particular MLO genes, i.e. in apple and grape by RNAi (Pessina et al. 2016a, b) and in pepper by virus-induced gene silencing (VIGS) (Zheng et al. 2013).
The susceptibility MLO gene family encodes proteins that belong to a plant-specific membrane protein family containing seven-transmembrane helices (Devoto et al. 2003; Kim et al. 2002; Panstruga, 2005). Up to 20 MLO homologs have been identified in several plant species. Phylogenetically, MLO isoforms experimentally shown to be functional as a S gene for powdery mildew are grouped together in one clade, clade IV for monocots and clade V for dicots (Zheng et al. 2016). In rose, only four MLO genes isolated from Rosa multiflora have been reported (Kaufmann et al. 2012), two of which (RhMLO1 and RhMLO2) were up-regulated in the rose-powdery mildew pathogen interaction (Qiu et al. 2015a). An antisense RhMLO1 transgenic rose line showed postponed conidia development upon powdery mildew infection, but as the fragment used for RNAi covered a region that is also present in RhMLO2, specificity to RhMLO1 silencing remained unclear (Qiu et al. 2015b).
At present, next-generation sequencing is extensively used to accelerate genetic, genomic, transcriptomic and epigenetic analysis. It becomes convenient and cheap to generate sequences from a large variety of samples. A reference genome and resequenced genomes of several related species or accessions enable identification of candidate genes and alleles prior to validation of the functional allele (Smulders et al. 2019, 2020). This has been recently done for MLO genes in various species, including soybean (Deshmukh et al. 2014), Cicer arietinum and Medicago truncatula (Deshmukh et al. 2017), Populus trichocarpa (Filiz and Vatansever 2018), pumpkin (Win et al. 2018), lentil (Polanco et al. 2018), bitter gourd (Chen et al. 2021), for the promotor region of MLO genes in melon and other species (Andolfo et al. 2019), and for other susceptibility genes (Pirrello et al. 2021). In rose overviews have been generated for WRKY genes (Liu et al. 2019), NBS-LRR genes (Lopez Arias et al. 2020), the disease resistance gene family Rdr1 (Menz et al. 2020), AP2/ERF transcription factors (Li et al. 2021), and S-RNases and F-box genes involved in self-incompatibility (Vieira et al. 2021). These inventories may be used as a basis to find or generate loss-of-function alleles of relevant MLO genes.
In this study, we identified putative RhMLO orthologs in the R. chinensis cv. ‘Old Blush’ (OB) genome sequence. In order to analyse the allelic variation of these RhMLO genes, we also explored resequencing data of a set of seven wild rose species (Hibrand Saint-Oyant et al. 2018) and transcriptome data of cut and garden roses (Dubois and Sakr 2012; Koning-Boucoiran et al. 2015). In addition, we used two different approaches for a functional analysis of the four clade V MLO homologs in rose: a transient knockdown approach through VIGS, followed by inoculation with powdery mildew as well as a transient RNAi silencing in petals and subsequent mildew inoculation. These two approaches used different strains of powdery mildew and different rose varieties. We show that, in addition to RhMLO1, RhMLO2 also acts as a susceptibility factor to powdery mildew, while RhMLO3 and RhMLO4 seem not to be involved or only with a minor effect.
Materials and methods
Identification of Rosa chinensis ‘Old Blush’ homologs of MLO genes
We refer here to all MLO genes from the genus Rosa as RhMLO genes, following the naming convention set by Kaufmann et al. (2012), but we add a reference to the Rosa species in which they were found. The protein sequences of four RhMLOs from Rosa multiflora (RhMLO1-4 in Kaufmann et al. 2012; GenBank accession numbers JX847131-JX847134) and all MLO genes used by Pessina et al. (2014), namely 18 FvMLOs from Fragaria vesca, 19 PpMLOs from Prunus persica, 21 MdMLOs from Malus domestica as well as 15 AtMLOs from A. thaliana http://www.arabidopsis.org/, were used for the identification of MLO gene members in the rose reference genome sequence of Rosa chinensis. All protein sequences of Rosa chinensis ‘Old Blush’ (Hibrand Saint-Oyant et al. 2018) were downloaded from GDR (Genome Database for Rosaceae, Jung et al. (2019); https://www.rosaceae.org/). Using BLASTp the best hits were identified against this set of MLO genes from different plant species. We used an e-value of 1e−5 as minimum, and report all e-values in Supplementary Excel File S1.
Based on the recently released high-quality F. vesca genome v4.0.a1 (Edger et al. 2018) and the updated gene annotation v4.0.a2 (Li et al. 2019), we replaced FvMLO11 from v1.0 (Shulaev et al. 2010) by FvH4_1g11630.1 because the protein sequence of FvMLO11 hits two different sequences named FvH4_1g11630.1 and FvH4_1g11620.1 with 100% (545 aa alignment) and 97.8% (413 aa alignment) identity, respectively, in v4.0.a1. FvH4_1g11630.1 has a gene structure consisting of 14 exons, which code for a protein with seven transmembrane domains, corresponding to the other characterized MLO genes (which have 12–15 exons), while FvH4_1g11620.1 only has 11 exons and the predicted protein lacks a typical transmembrane domain. FvH4_1g11620.1 may therefore represent an assembly error. The position and number of transmembrane domains were predicted using HMMER (https://www.ebi.ac.uk/Tools/hmmer/).
Bioinformatic analysis of MLO gene family in rose
The chromosomal location and predicted intron/exon structure of each RhMLO gene were extracted from the available genomic information of Rosa chinensis genome HapOB2 v1.0 in GDR. We visualized the chromosomal location and intron/exon structure by MapChart 2.32 and online tool GSDS 2.0 (http://gsds.cbi.pku.edu.cn/), respectively.
A phylogenetic analysis was performed for the RhMLO proteins obtained from the BLAST search. For this, next to the MLO sequences obtained from rose, apple, strawberry, peach and Arabidopsis, we also included a series of MLO homologs that have been functionally associated with powdery mildew susceptibility: HvMLO (Z83834), OsMLO2 (AF384030), TaMLO1_A1b (AX063298), TaMLO_B1 (AF361932), SlMLO1 (AAX77013), CaMLO2 (AFH68055), PsMLO1 (FJ463618), MtMLO1 (HQ446457), LjMLO1 (AAX77015), VvMLO6 (Genoscope ID: GSVIVT00018217001; http://www.genoscope.cns.fr/externe/GenomeBrowser/Vitis/entry_ggb.html), VvMLO7 (Genoscope ID: GSVIVT00018219001), CsMLO1 (Cucurbit Genomics Database ID: Csa5M623470.1; http://cucurbitgenomics.org/organism/2) and NtMLO1 (AIT98396) (Buschges et al. 1997; Elliott et al. 2002; Bai et al. 2008; Feechan et al. 2008; Winterhagen et al. 2008; Humphry et al. 2011; Zheng et al. 2013; Berg et al. 2015; Nie et al. 2015; Pessina et al. 2016b).
MLO protein sequences were aligned first with Clustalx 1.83 and then further aligned by Clustalw in MEGA7. Sequences were trimmed at the C and N terminal parts. Different amino acid substitution models were tested, and a Poisson model with uniform rates was chosen as most suitable. Then, the alignment was used to generate phylogenetic trees in MEGA7 using ML (maximum likelihood) and UPGMA (unweighted pair group method with arithmetic mean) methods with a bootstrap value of 1000.
Expression pattern of RhMLO genes
To determine the expression pattern of RhMLO genes identified, their full-length protein sequences were used in a tBLASTn analysis of the ROSAseq transcriptome database https://lipm-browsers.toulouse.inra.fr/plants/R.chinensis (Dubois and Sakr 2012) with the parameters: e-value 1e-10, identity 90% or more. As next-generation sequencing often yields sequences to only parts of the gene, more than one cluster of target sequences in transcriptome database could match each RhMLO, in such case we used the hit with the highest score.
Identification of alleles of RhMLO genes
Allelic variation across candidate RhMLO genes was analysed in assembled contigs of twenty-two resequenced Rosa accessions of the diploid rose species R. persica, R. moschata, R. xanthina spontanea, R. chinensis var. spontanea, R. laevigata, R. minutifolia alba and R. rugosa (Hibrand Saint-Oyant et al. 2018; assemblies unpublished). In addition, transcriptome data from earlier studies (Dubois and Sakr 2012; Koning-Boucoiran et al. 2015) were also used. The origin of all sequence information used is listed in Table 1.
For the seven resequenced rose species (accessions No. 2–8 in Table 1), we used the predicted protein sequences. Sequences with an amino acid identity higher than 75% in a BLASTp to the 19 RhMLOs in the R. chinensis genome sequence were selected and checked manually, because in some cases two or more partial sequences from a single accession matched the query sequence, which may represent different parts of the same MLO gene. In such cases, we named these short sequences-a, -b and -c. We have tentatively assumed they were from the same allele, unless there was evidence for two alleles in that accession. Subsequently, the corresponding DNA sequences were retrieved from the resequencing database.
For diploid and tetraploid accessions No. 9 to 22 (Table 1) only RNA-seq data were available. The 19 RhMLO genes from R. chinensis cv. ‘Old Blush’ identified were used (without introns) in a tBLASTn analysis with a cut-off at 90% identity, and the longest sequences were kept.
All DNA sequences were aligned using Seqman Pro and MegAlign (DNASTAR) and alignments were corrected manually. Alleles of the same gene were distinguished from different genes manually, based on SNPs in the coding region. Criteria used to assign alleles to a single gene: alleles from the same gene should be > 90% and preferably > 95% similar, different genes have a different location in the assembled genome, maximum two alleles per locus/gene in a diploid, four in a tetraploid genotype. This produced a non-redundant list of candidate genes. It was not necessary to assume that any of the Rosa species had more than 19 genes.
Structural analysis of RhMLO1-RhMLO19
A multiple sequence alignment was made to highlight transmembrane regions and conserved domains using Clustalx 1.83 software. The online tool HMMER (https://www.ebi.ac.uk/Tools/hmmer/) was used to identify transmembrane domains. The gene structures of RhMLO1-RhMLO19 were analysed through the online Gene Structure Display Server (http://gsds.cbi.pku.edu.cn/).
Functional analyses of clade V RhMLO genes using VIGS
To test whether the four MLO genes (RhMLO1-4) from clade V act as susceptibility gene for powdery mildew, a VIGS experiment was conducted for these genes. For this, rose plantlets (Rosa hybrida ‘Samantha’) were propagated in tissue culture on Murashige and Skoog (MS) medium supplemented with 3% (w/v) sucrose, 1.0 mg/l 6-benzylaminopurine (6-BA), 0.05 mg/L naphthalene acetic acid (NAA), and 1.0 mg/L GA3 for 60 d. Healthy, strong plantlets were transferred to rooting medium comprising half-strength MS medium supplemented with 3% sucrose and 0.1 mg/L NAA for 30 days.
Vector construction for VIGS
For construction of the VIGS vectors, 349-, 279-, and 398-bp fragments from the coding sequence region of RhMLO1, RhMLO2, and RhMLO3/4 (the fragment is from a region in which RhMLO3 and RhMLO4 are identical) were PCR amplified using primers RhMLO1-VIGS-F and RhMLO1-VIGS-R, RhMLO2-VIGS-F and RhMLO2-VIGS-R, RhMLO3/4-VIGS-F and RhMLO3/4-VIGS-R, respectively (Supplementary Table S1). The generated fragments were inserted into the pTRV2 vector as described previously (Liu et al. 2002). The pTRV2, pTRV2-RhMLO1, pTRV2-RhMLO2, and pTRV2-RhMLO3/4 constructs were transformed into Agrobacterium tumefaciens strain GV3101. The transformed A. tumefaciens strains were cultured in Luria–Bertani (LB) medium supplemented with 10 mM MES, 20 μM acetosyringone, 25 μg/ml rifampicin, and 50 μg/ml kanamycin. The cultures were collected and resuspended in infiltration buffer (10 mM MES, 10 mM MgCl2, and 200 μM acetosyringone, pH 5.6) to a final OD600 of 1.5. Mixtures of cultures containing an equal ratio (v/v) of pTRV1 (Liu et al. 2002) and each recombinant pTRV2 construct were placed in the dark for 3–5 h at room temperature and were then used for vacuum infiltration.
Preparation and analysis of infiltrated plants
Rose plantlets were placed in deionized water for 2 days and then immersed in the bacterial suspension and infiltrated under a vacuum at 0.7 MPa twice. Plantlets infiltrated with an A. tumefaciens strain containing the empty virus were used as controls (TRV-00). After infiltration, the plantlets were washed in water five times and in deionized water twice and then placed in the dark at 8 °C for 3 d. The plantlets were transplanted into pots and grown at 23 ± 2 ℃ for 16 h in the light and 8 h in the dark. At 30 days post-vacuum infiltration, RNA was isolated from infiltrated plantlets using the OMEGA Plant RNA Kit (OMEGA, China) to determine the expression of the target gene (RhMLO1, RhMLO2, or RhMLO3/4 combined). The R. hybrida ubiquitin gene RhUBI2 (Supplementary Table S1) was used as an internal control. RT-qPCR reactions (10 μl volume) were performed using the KAPA SYBR FAST Universal RT-qPCR kit (Takara, China) in the ABI Real-time PCR System (Thermo Fisher Scientific). Cycling conditions were as follows: 3 min of denaturation at 95 °C, followed by 40 cycles of 95 °C for 3 s, 60 °C for 30 s, and 72 °C for 30 s. Each reaction was performed in triplicate, and products were verified by melting curve analysis. The transcript levels of genes were determined using the 2−ΔΔCT method. The plants with down-regulated target gene expression were retained for further pathogen assays.
Powdery mildew infection assays
The P. pannosa strain CAU8311, causing powdery mildew in rose, was cultivated on living rose leaves in an illumination incubator. Infected rose leaves were collected in sterilized water and thoroughly vibrated using a vortex mixer to separate P. pannosa from the leaves. The P. pannosa spore suspension was adjusted to a final concentration of 3 × 105 conidia/ml in sterilized water for foliar application. Approximately 30-day-old infiltrated rose plantlets were inoculated with P. pannosa. The plants were transferred into the illumination incubator at 16ºC in the dark for 24 h and then at 22/16 °C, with a 16/8-h light/dark cycle. To test powdery mildew resistance of the infiltrated plants, the average number of visible P. pannosa colonies that formed on each plant leaves was evaluated at 7, 10, 13, 16 and 25 days post-inoculation (dpi). A Student’s t test was used for statistical analysis. Differences were considered statistically significant at p < 0.05 (*) or p < 0.01 (**). For fungal biomass analysis of infiltrated plants, at 25 days post-inoculation total DNA was isolated from whole plant leaves and the fungal biomass was determined by RT-qPCR of the P. pannosa internal transcribed spacer (ITS) relative to the rose Actin5 gene RhActin5, using primers ITS-F and ITS-R, RhActin5-F and RhActin5-R, respectively (Supplementary Table S1). The RT-qPCR reaction was performed in an ABI Real-time PCR System (Thermo Fisher Scientific) with cycling conditions: 3 min at 95 °C, followed by 40 cycles of 95 °C for 3 s, 60 °C for 30 s, and 72 °C for 30 s.
Functional analyses of clade V RhMLO genes using transient RNAi
Vector construction for the transient RNAi in petals
As a basis for the RNAi experiments the vector p9U10-RNAi (DNA-cloning service, Hamburg, Germany) was used. In this vector, double stranded RNA is generated by cloning the target fragment between two 35S promoters in reverse orientation (Schmidt et al. 2012). The vector was linearized via BamHI/HindIII double digest and gel-purified to remove a dummy fragment from the GUS gene cloned between the two 35S promoters. Fragments from the most variable last exon of the four RhMLO genes (283 bp for RhMLO1, 291 bp for RhMLO2, and 311 bp for RhMLO3/4, Supplementary Figure S3) were amplified via proof reading PCR with overhangs specific to the BamHI/HindIII restriction site of the vector. Cloning into the vector was then performed using the In-Fusion™ HD Cloning Plus Kit (Takara Bio, Mountain View, USA). Plasmids were transformed into E. coli DH10B by electroporation, and after isolation of plasmids, the sequences were checked via custom Sanger sequencing (GATC, Cologne, Germany). Plasmids with correctly inserted MLO fragments were then electroporated into Agrobacterium tumefaciens strain GV3101 via electroporation.
Transient RNAi silencing in rose petals
Fresh streaks of the Agrobacterium strains harbouring either GUS (negative control), RhMLO1, RhMLO2 or RhMLO3/4 RNAi constructs were precultured in 10 ml YEP medium overnight. From these cultures Erlenmeyer flasks with 25 ml each were inoculated with 200 µl of the overnight culture and incubated with shaking at 28 °C overnight. From this culture a third culture was inoculated the next day so that the starting OD600 was 0.2 and then grown until an OD600 of 1.0 to 1.5 was reached. The final cultures were centrifuged for 1 h at 3500 rpm, and the pellet was resuspended in infiltration buffer (2.13 g/l MES, 2.03 g/l MgCl2·6H2O, pH 5.6) at an OD600 of 0.5. Prior to the infiltrations the suspension of each MLO construct was mixed in equal amounts with the suspension of a strain harbouring an empty pRedU10-35 s construct expressing dsRed under the Arabidopsis Ubiquitin promoter (the same culture conditions were used). Prior to the infiltration petals of the variety Pariser Charme were harvested from unopened floral buds and placed on wet filter paper in translucent plastic boxes. A small incision was placed at the base of the petal through which the suspension was infiltrated into the petal with a 1-ml syringe without needle. The boxes were incubated at 20 °C for 6 days before they were inoculated with conidia of rose powdery mildew sampled from infected greenhouse plants.
Only those areas of the infiltrated petals were sampled for either qPCR or for the disease assay that expressed the dsRed signal approx. 6 days after infiltration.
qPCR of infiltrated petals
For qPCR 100 mg of dsRed positive parts of the petals were excised and frozen in liquid nitrogen. RNA was extracted using the RNeasy® Plant Mini Kit (Qiagen, Germany) according to manufacturer’s instructions, but using DDT instead of beta-mercaptoethanol. DNA was digested using the DNA-free™ DNA Removal Kit (Ambion, USA). For cDNA synthesis, the High-Capacity cDNA Reverse Transcription Kit (Applied Biosystems USA) was used. Quantitative real-time PCR was conducted using the Takyon Rox SYBR Master-Mix dTTP Blue kit (Eurogentec, Belgium) (10 µl reaction volume) and cycling conditions: 95 °C 1 min, followed by 40 cycles of 95 °C 10 and 59°-64 °C 60 s (StepOnePlus cycler from Applied Biosystems, USA). Each infiltration was repeated four times, and in each experiment, three petals were sampled and combined into one RNA pool. Each reaction was performed in triplicate, and the melting curve was used for product verification. As internal controls, the genes UBC and SAND were used.
The obtained data were used for primer efficiency calculation of each reaction using LinRegPCR software (Version 2016.1, Ramakers et al. 2003). Based on this, the RQ value was determined using R (version 3.2.4, R Core Team 2016). Data were log-transformed and on the basis of a linear model, significant differences in mean comparison and ANOVA analysis to the control were determined (α = 0.05). The p values of the mean comparison were adjusted using the Dunnett test.
Infection assay on rose petals
Powdery mildew conidia were obtained from naturally infected greenhouse plants by washing them off infected leaves in distilled water with 0.05% Tween 20. The concentration of conidial suspensions was determined in counting a sample with a Fuchs-Rosenthal chamber and adjusted to a concentration of 500,000 conidia/ml. Suspensions were sprayed onto petals using an atomizer followed by immediately drying the liquid in the airstream of a fume hood. After drying of petal surfaces was complete, vitality of a suspension kept in an Eppendorf tube was checked by staining with phenosafranin. Samples were taken 3 dpi by cutting out those parts of the petal that displayed dsRed fluorescence. Samples were fixed on filter paper soaked in ethanol/acetic acid (3:1) with the inoculated side up. Fixed samples were washed 3 × in PBS buffer avoiding contact of the inoculated area with the buffer and then stained with the WGA-AlexaFlour488 stain (Invitrogen, Carlsbad, USA) at 10 µg/ml in PBS. Microscopy was done with a Zeiss AxioScope A1 (Zeiss, Jena, Germany) with the filter set 38 (excitation: 493 nm, emission: 520 nm) at a magnification of 100 × and 200x. Four independent experiments at different dates using independently prepared inoculum were conducted, each comprising 6 (experiment1) or 8 (experiments 2–4) petals. All germinated spores were counted on the excised area of the petal, and the fraction of those which formed a mycelium was determined for each petal per experiment. An ANOVA was computed in R based on a generalized linear model, assuming a Poisson distribution for the ratio of colonies formed in relation to the number of germinated conidia. This was followed by a Dunnett post hoc test for significance in pairwise comparisons between the GUS control and the RNAi constructs.
Results
In silico identification of the rose RhMLO gene family
A blast search for MLO homologs of the R. chinensis genome sequence with F. vesca, P. persica, M. domestica and A. thaliana MLO gene models produced 19 significant matches in rose (Table 2, Supplementary Excel File S1). We named them RhMLO1-19 (tentative names for RhMLO5-19). Generally, for each of the MLO genes from the other species there was one highly similar counterpart gene in rose, except for RhMLO3 and RhMLO4, both on chromosome 1, which have their highest similarity both to FvMLO12, although below 90% similarity. The two genes are only 86.8% similar to each other, and their gene structure is also different.
In addition, one of the matched sequences from R. chinensis, RC4G0104600, encoded a protein of 321 amino acids, which is much shorter than all MLO homologs reported in, for example, the genomes of Arabidopsis and grapevine (Devoto et al. 2003; Feechan et al. 2008). The adjacent annotated sequence, RC4G0104700, is also a part of an MLO gene. We have merged them in order to recreate a full-length MLO gene, called RhMLO19. Details on genomic localization, length of the predicted proteins, and the level of similarity to other known MLO homologs in this study are provided in Table 2.
The physical position of RhMLO1-RhMLO19 across all seven R. chinensis chromosomes is depicted in Fig. 1. We found four cases of adjacent genes (RhMLO3 and RhMLO4, RhMLO2 and RhMLO7, RhMLO1 and RhMLO14, RhMLO12 and RhMLO17). Except for RhMLO3 and RhMLO4 the pairs of adjacent genes were, however, not from the same clade (see below).
As reported previously, the orthologous relationship between rose and strawberry is very close (Shulaev et al. 2010; Hibrand Saint-Oyant et al. 2018). We made a synteny analysis between R. chinensis ‘Old Blush’ and F. vesca chromosomal regions containing MLO homologs. The locations of 15 of the 19 RhMLO homologs (Table 2) are in accordance with known syntenic relations of the chromosomes. RhMLO5 on chromosome 2 showed homology to both FvMLO6 and FvMLO7 on chromosome 3, which is unexpected as rose linkage group 5 is syntenic to F. vesca linkage group 3. In addition, the chromosome locations of RhMLO1, RhMLO13 and RhMLO19, located, respectively, on chromosome 5, 3 and 4, are homologous to FvMLOs on chromosome 6, 7 and 5, respectively. This is surprising as rose linkage group 3, 4 and 5 are syntenic to F. vesca linkage group 6, 4, and 3, respectively (Vukosavljev et al. 2016; Hibrand Saint-Oyant et al. 2018).
Phylogenetic relations of the rose RhMLO gene family
A phylogenetic analysis was performed to establish the relationship between the RhMLO proteins and the MLO proteins of several other plant species (Arabidopsis thaliana), peach (Prunus persica), strawberry (Fragaria vesca), apple (Malus domestica), tomato (Solanum lycopersicum), pepper (Capsicum annuum), cucumber (Cucumis sativus), tobacco (Nicotiana tabacum), pea (Pisum sativum), barrelclover (Medicago truncatula), lotus (Lotus japonicus), grapevine (Vitis vinifera), barley (Hordeum vulgare), wheat (Triticum aestivum) and rice (Oryza sativa). The UPGMA (Fig. 2) and ML (not shown) trees had similar topology. The UPGMA tree contains eight different clades named from I to VIII following the classification of previous studies in Arabidopsis and Rosaceae MLO protein families (Feechan et al. 2008; Pessina et al. 2014). Four RhMLO homologs (RhMLO1, RhMLO2, RhMLO3 and RhMLO4) were found in Clade V, which includes all dicot MLO proteins shown to be required for powdery mildew susceptibility (Consonni et al. 2006; Bai et al. 2008; Humphry et al. 2011; Zheng et al. 2013; Berg et al. 2015; Nie et al. 2015; Qiu et al. 2015a, b; Fujimura et al. 2016; Pessina et al. 2016a, b). Two homologs from rose (RhMLO17 and RhMLO19) were found in Clade IV, which contains the MLO proteins acting as susceptibility factor in monocots.
Phylogenetic tree of RhMLO genes. Phylogenetic relationships of predicted RhMLO amino acid sequences to MLO proteins of other pant species including several Rosaceae MLO (Pessina et al. 2014; Edger et al. 2018) and some dicot (Bai et al. 2008; Feechan et al. 2008; Winterhagen et al. 2008; Humphry et al. 2011; Zheng et al. 2013; Berg et al. 2015; Nie et al. 2015; Pessina et al. 2016b) and monocot MLO genes (Büschges et al. 1997; Elliott et al. 2002). 15 AtMLO amino acid sequences were obtained from The Arabidopsis Information Resource (TAIR, http://www.arabidopsis.org/). Displayed is only topology and cut-off value for the consensus tree to 50%. The 19 RhMLOs are highlighted in blue. Proteins that have previously been functionally characterized as susceptibility genes are marked by red triangles. Numbers at each node represent bootstrap support values (1000 replications)
Structural organization of RhMLO genes
All RhMLO genes had seven transmembrane domains, except for RhMLO9, RhMLO13 and RhMLO19 that have six (Table 3). In all proteins except RhMLO6 we detected a conserved calmodulin-binding site (CaMBD). Furthermore, in some proteins (RhMLO1, RhMLO2, RhMLO10, RhMLO17 and RhMLO19) the conserved domain II (D/E-F-S/T-F) was detected in the highly polymorphic C terminal part as previously found by Panstruga (2005) (Supplementary Figure S1). Most MLO genes consist of 13–15 exons except RhMLO6 (11 exons) and RhMLO8 (1 exon) (Fig. 3). The single exon structure of RhMLO8 is an exception within the MLO family in rose, but its homologs in apple and peach also consist of a single exon.
Gene structure of 19 rose MLO genes in the R. chinensis genome sequence. Gene models (5′–3′) were derived from the GFF3 file at https://www.rosaceae.org/species/rosa/chinensis/genome_v1.0. The gene structure of RhMLO19 was based on the combination of RC4G0104700 and RC4G0104600
Expression patterns of RhMLO genes
Gene expression was found in various transcriptome datasets for 11 of the 19 RhMLO genes. A heatmap (Supplementary Figure S2) shows that RhMLO3 and RhMLO4 were detected at high levels in leaves under water stress, while RhMLO5, RhMLO10 and RhMLO15 accumulated in young roots. RhMLO8 was expressed in all floral samples, especially in floral meristem and early floral organ development.
Allelic variants in wild and cultivated roses
We produced an overview of the allelic variants of the MLO genes across the rose species based on both the available genome sequences and the RNAseq data. Alleles may go undetected in the RNAseq data if they are not expressed, or if read numbers are too low, so this analysis may underestimate the real allelic diversity.
After BLASTp or tBLASTn, a total of 300 different complete or partial sequences were detected across the 19 RhMLOs in the 22 rose accessions (Supplementary Table S2 & Supplementary Table S3). Because RhMLO19 has a high similarity (87.7%) to RhMLO17, the sequences that matched with RhMLO19 all matched with RhMLO17 as well (Supplementary File 1), and of these only DL_g32145.t1 had a higher similarity to RhMLO19 (93.8%) than to RhMLO17 (93.1%). For the sake of the diversity analysis, we have treated all these sequences as putative alleles of RhMLO17.
Among the 18 RhMLO genes with allele information, RhMLO1 had the highest number of sequences (23), while RhMLO4 had the lowest (6). After aligning the DNA sequences, we focused on the occurrence of SNPs among the sequences. For ease of visualisation, sequences were reduced to a concatenated sequence of all SNP positions. The length of these runs of SNPs found for the same RhMLO gene in different accessions is dependent on the actual length of sequences found. Therefore, we inserted ‘N’ to fill missing SNP positions in the assembled reads of a particular haplotype (Supplementary Table S4) and indicate the position for all SNPs and indels in Supplementary Excel File S2. Some short runs of SNPs were set aside because they were not unique for one haplotype (e.g. CCACCCG in RhMLO1 could be part of the longer haplotypes H2, H3, H7 or H8). Resolving these short runs will require generating longer sequence reads.
From the 300 sequences, a total of 198 different SNP haplotypes, further referred to as allelic variants or alleles, were found with an average of 10 alleles per gene. RhMLO8 and RhMLO18 showed the highest number of alleles (putatively 16 alleles). To find more information about the relationship among the rose accessions, the sharing of identical alleles deserves closer attention. Allele H1 in RhMLO1 was present in diploid species R. chinensis cv.‘Old Blush’ and R. chinensis var. spontanea, together with tetraploid garden roses AH and MF. This is consistent with the notion that R. chinensis var. spontanea is one of the ancestors of R. chinensis cv.‘Old Blush’ and that these diploid species from the section Chinenses have contributed to the makeup of tetraploid modern roses. The situation that a SNP haplotype was found in both a diploid species and a tetraploid cultivar occurred also for RhMLO2, RhMLO4, RhMLO5, RhMLO6, RhMLO7, RhMLO8, RhMLO9, RhMLO10 and RhMLO15 (Supplementary Table S4). RhMLO8 and RhMLO9 were found in all 22 Rosa accessions (Supplementary Table S4) and are thus best covered and most suitable to check for common haplotypes between diploid species and tetraploid cultivars.
Specific analysis of the RhMLO genes in clades IV and V
For RhMLO1, among the 271 detected SNPs, we observed 25 non-synonymous SNPs and two indels with an effect on the resulting amino acid sequence (alignment in Supplementary PDF S1). Some amino acid changes were caused by two or three sequential SNPs, such as Y34L (TAC → TTA) and I35C (ATA → TGT). One insertion (+ 105E) and one deletion (Q512-) were in non-conserved regions.
For RhMLO2, among 161 detected SNPs, we found 17 non-synonymous SNPs and two indels (Supplementary PDF S2). The first indel with a 36-bp deletion was in a conserved region (NAFQLAFFAWTW386-). The other 12-bp deletion (HTRD570-) was in non-conserved regions, but the amino acid asparagine (D) belongs to the C-terminal D/E-F-S/T-F tetra-peptide sequence, which is one of several motif characteristic of MLO orthologs.
For RhMLO3, among 337 detected SNPs, we found 39 non-synonymous SNPs and five indels (Supplementary PDF S3). Two insertions, namely + 134 T and + 464L, were in conserved regions. For RhMLO4, among 142 detected SNPs, we found 15 non-synonymous SNPs and no indels (Supplementary PDF S4). For RhMLO17, among 78 detected SNPs, we found 8 non-synonymous SNPs and one indel (Supplementary PDF S5). The only deletion (VVVGIS290-) was in a conserved region.
RhMLO1 gene silencing in rose using VIGS
To test the practicality of using VIGS to study the MLO gene function in rose for powdery mildew resistance, we set out at CAU in Beijing to suppress the expression of the endogenous RhMLO1 gene, which was suspected to play an important role in the susceptibility of rose towards powdery mildew (Qiu et al. 2016). To this end, the 349-bp RhMLO1 sequence was cloned and used. Compared to control plants treated with an empty TRV vector (TRV-00), plants infiltrated with TRV-RhMLO1 showed an increased resistance against powdery mildew, evidenced by decreased fungal growth (Fig. 4a, b) and biomass (Fig. 4c). Prior to the pathogen inoculation, RT-qPCR of independently infiltrated plants showed a significant decrease in the abundance of RhMLO1 transcripts in the leaves of plants treated with TRV-RhMLO1 at 30 days post-vacuum infiltration (Fig. 4d). The suppressed growth of powdery mildew on the VIGS-silencing rose plants resembled what could be seen on plants with stable RNAi down-regulation of endogenous rose RhMLO1 (Qiu et al. 2016). This further confirmed the involvement of RhMLO1 in powdery mildew susceptibility of rose. Importantly, these results also highlight the feasibility of using this VIGS method for studying the function of MLO genes and powdery mildew resistance in rose.
Silencing RhMLO1 in rose enhances resistance to powdery mildew. a Typical appearance of control (TRV-00) and TRV-RhMLO1-infiltrated rose plants upon inoculation with powdery mildew 25 days post-inoculation (dpi). b Quantification of powdery mildew symptoms in control (TRV-00) plants and TRV-RhMLO1-infiltrated plants, shown as the average number of fungal colonies on each rose leaf. c Fungal biomass determined by RT-qPCR in control (TRV-00) and three independent lines of TRV-RhMLO1-infiltrated rose plants. Bars represent P. pannosa ITS transcript levels relative to rose RhActin5 transcript levels for equilibration. Lines 1, 2, and 3 represent three independent TRV-RhMLO1-infiltrated plants. The samples were taken at 25 dpi. d RT-qPCR was performed to monitor expression of RhMLO1 in both control (TRV-00) and TRV-RhMLO1-infiltrated rose plants (TRV-RhMLO1–1, 1–2, and 1–3). The total RNA was isolated from infiltrated plantlets at 30 days post-vacuum infiltration, prior to powdery mildew inoculation. The rose ubiquitin gene RhUBI2 was used as an internal control. Asterisks indicate significant differences according to Student’s t test (*P < 0.05, **P < 0.01, ***P < 0.001)
RhMLO2 is involved in powdery mildew susceptibility
RhMLO2 is a close homolog of RhMLO1. As the function of RhMLO2 in powdery mildew infection in rose has not been previously reported, we assessed its role also by VIGS. At 25 days post-infection, fewer fungal colonies were observed in R. hybrida ‘Samantha’ plantlets treated with TRV-RhMLO2 (Fig. 5a) compared to control plants treated with the empty vector TRV-00. The symptom development was quantified by evaluating the average number of fungal colonies on each rose leaf (Fig. 5b). The abundance of the RhMLO2 transcript was substantially reduced in TRV-RhMLO2-treated plants (Fig. 5c), suggesting that RhMLO2 is also involved in susceptibility of rose towards powdery mildew.
Silencing RhMLO2 in rose enhanced resistance to powdery mildew. a Typical appearance of control plants and rose plants infiltrated with TRV-RhMLO2 upon inoculation with powdery mildew at 25 dpi. b Quantification of powdery mildew symptoms in TRV-RhMLO2-infiltrated and control plants. Quantification of symptom development is shown as an average number of fungal colonies on each rose leaf. c RT-qPCR was performed to monitor expression of RhMLO2 in both control (TRV-00) and TRV-RhMLO2-infiltrated plants. The total RNA was isolated from infiltrated plantlets at 30 days post-vacuum infiltration, prior to powdery mildew inoculation. The rose ubiquitin gene RhUBI2 was used as an internal control. Asterisks indicate significant differences according to Student’s t test (*P < 0.05; **P < 0.01; ***P < 0.001)
RhMLO3 and RhMLO4 are likely not required for powdery mildew infection in rose
We also assessed whether RhMLO3 and RhMLO4 are involved in rose powdery mildew susceptibility using VIGS. RhMLO3 and RhMLO4 share 95.5% sequence identity. Therefore, a 398-bp coding sequence shared by RhMLO3 and RhMLO4 was cloned into the vector pTRV2 to generate TRV-RhMLO3/4. The powdery mildew resistance of plants infiltrated with TRV-RhMLO3/4 was then assessed by quantifying the number of powdery mildew colonies per leaf following fungal inoculation. RT-qPCR confirmed that the abundance of the RhMLO3 and RhMLO4 transcripts was substantially reduced in the leaves of different independent plants infiltrated with TRV-RhMLO3/4 (Fig. 6c). However, there was no significant decrease in fungal colony numbers upon RhMLO3/4 silencing (Fig. 6a, b), suggesting that RhMLO3 and RhMLO4 may be not involved in powdery mildew resistance in rose.
Silencing RhMLO3/4 in rose does not affect resistance to powdery mildew. a Typical appearance of control (TRV-00) and TRV-RhMLO3/4-infiltrated rose plants upon inoculation with powdery mildew at 25 dpi. b Quantification of powdery mildew symptoms in control (TRV-00) plants and in different independent TRV-RhMLO3/4-infiltrated plants, shown as the average number of fungal colonies on each rose leaf. c RT-qPCR was performed to monitor expression of RhMLO3/4 in both control (TRV-00) and in different independent TRV-RhMLO3/4-infiltrated rose plants. The total RNA was isolated from infiltrated plantlets at 30 days post-vacuum infiltration, prior to powdery mildew inoculation. The rose ubiquitin gene RhUBI2 was used as an internal control. Asterisks indicate significant differences according to Student’s t test (*P < 0.05, **P < 0.01, ***P < 0.001)
RNAi silencing
To gain independent evidence for the role of RhMLO1-4 in the interaction with powdery mildew using another approach, we transiently silenced them by RNA interference in petals of the susceptible variety Pariser Charme at the LUH in Hannover. For this we infiltrated RNAi-constructs for RhMLO1, RhMLO2, RhMLO3/4 and GUS as a control, each mixed with a dsRed construct, into petals. Two days after infiltration petals were inoculated with powdery mildew. Six days after infiltration areas with a strong dsRed signal were excised and used for gene expression analysis via qPCR (Fig. 7). This was repeated four times independently, each time with fresh cultures of the infiltration constructs. For RhMLO1- and RhMLO3/4-RNAi constructs a partial but significant downregulation of the target RhMLO genes could be demonstrated in relation to the GUS negative control (Fig. 7), whereas the downregulation of RhMLO2 expression was not significant although it showed a lower average compared to the control (Figure S4). The respective non-target RhMLOs were not affected by the treatments.
Six to nine infiltrated petals were inoculated two days after infiltration for each infiltration experiment and, as for the gene expression analysis, only parts of the petals expressing dsRed were sampled six days after infiltration (four days after inoculation). Statistical analysis of all four experiments together with an ANOVA and a Dunnett test for post hoc analyses revealed a statistically significant reduction of the infection in case of the RNAi constructs for RhMLO1 as well as RhMLO2 but only a small, not significant reduction for RhMLO3/4, thereby confirming the VIGS experiments (Table 4).
Discussion
Genomic organization and evolution between Rosaceae MLO homologs
We identified 19 MLO homologs in the reference genome of rose through an in silico approach. This number is in line with other genome-wide studies in dicotyledonous species, which found 18 MLO homologs in the genome of strawberry, 19 in peach and 15 in Arabidopsis (Devoto et al. 2003; Pessina et al. 2014).
Consistent with the high level of synteny between the rose and woodland strawberry genomes, 15 probable orthologous relationships between MLO genes in these two closely related species are in syntenic positions, while four are not. Whether assembly errors play a role remains to be determined.
We found four cases of adjacent homologs. RhMLO3 and RhMLO4 may be the result of a recent gene duplication as both of them had their highest similarity to the same MLO gene in F. vesca (FvMLO12). If so, they have already diverged somewhat in their expression pattern, according to our analysis of the ROSAseq data. RhMLO1 on Chr. 5 is most similar to FvMLO1 (which might be FvH4_6g15610.1 in F. vesca Genome v4.0.a1) on Chr. 6, but these positions are not syntenous, while the adjacent gene RhMLO14 is in a syntenic position with FvMLO14 (which might be FvH4_3g32830.1 in F. vesca Genome v4.0.a1) on Chr. 3. It may be useful to screen these regions for microhomology, to differentiate putative translocations from assembly errors. For the other two pairs (RhMLO2 and RhMLO7, RhMLO12 and RhMLO17), the genes with the highest similarity in F. vesca are also adjacent to each other.
RhMLO homologs and susceptibility to powdery mildew
The expression patterns of MLO genes may indicate possible functions of these genes. Previously, Qiu et al. (2015a) found that the leaf expression levels of RhMLO1 and RhMLO2 increased more than two folds on treatment with various abiotic stimuli, while RhMLO3 and RhMLO4 decreased. Xiang et al. (2019) identified RhMLO genes from Rosa gigantea and Rosa longicuspis, respectively. The two genes which are homologs of RhMLO1/2 were drastically up-regulated upon powdery mildew inoculation, which is in agreement with observation of other studies showing up-regulated expression of the MLO S gene in different plant species (e.g. Zheng et al. 2013, 2016; Pessina et al. 2016a, b). The functionality as an S gene for powdery mildew in rose was confirmed for RhMLO1 and RhMLO2 by our VIGS experiments as well as by our experiments using transient RNAi. The two sets of experiments were conducted in two different laboratories using different rose genotypes, using different sources of powdery mildew inoculum, different methods for reducing gene expression, and independent biological replications of the experiments rather than technical replications of the measurements.
All results showed a significant effect except the transient RNAi-downregulation of RhMLO2, which, although showing a tendency for reduction, was statistically not significant due to the very low basic expression level of RhMLO2 and the large variation across the four independent experiments conducted. Nevertheless, the effects on the infection with powdery mildew were significant. As transient expression in rose petals is less effective than comparable experiments in leaves of Nicotiana benthamiana and given the significance of the functional effects in the infection assays in both VIGS and transient RNAi experiments, in our opinion the sets of experiments strongly support the function of both genes in the rose-powdery mildew interaction. The role of RhMLO2 in powdery mildew susceptibility is less pronounced than the role of RhMLO1.
In the experiments on silencing RhMLO1, the line with the highest RhMLO1 expression shows the lowest fungal biomass (Fig. 4b, c). Such an experimental outcome in a VIGS/pathogen assay is very common and generally accepted. Even by using a stable transgenic RNAi line of rose RhMLO1, Qiu et al. (2015b) found that their RhMLO1-RNAi line 2 had the highest RhMLO1 expression level with the lowest number of germinated conidia. The transient RNAi approach independently showed that RhMLO1 is a functional gene in powdery mildew susceptibility.
In contrast, the homologs of MLO3/4 in these species were down-regulated or moderately up-regulated by powdery mildew (Xiang et al. 2019). In our study, silencing of RhMLO3 and RhMLO4, whether performed by VIGS or by RNAi, did not change rose susceptibility to powdery mildew significantly. These results indicate that RhMLO3/4 may possess distinct biological functions in rose, even though they are grouped in clade V with MLO orthologs proven for their S gene function to powdery mildew. Alternatively, RhMLO3 and RhMLO4 may have a minor contribution to powdery mildew infection (as seen in the transient silencing experiments in petals), as it is the case for AtMLO6 and AtMLO12, which are additive to AtMLO2 (Consonni et al. 2016).
An alternative to the functional down-regulation of RhMLO1-4 by VIGS and RNAi is a CRISPR knockout of the genes (Geike et al. 2015), as this may give a more clear-cut phenotype. We have developed rose transformation systems but so far only for tetraploid roses and still with suboptimal efficiency. We have made a first attempt for genome editing of RhMLO genes in tetraploid rose but due to the low transformation efficiency only a few transgenics for RhMLO2 were generated, in which not all four alleles were edited (not shown). For RHMLO3 and -4, finding targets which are specific for one of the genes is as impossible as it is for the RNAi approach.
Possible biological functions of other RhMLO genes
RNA sequencing has been effectively used for the study of expression patterns and molecular networks in rose for petal development (Han et al. 2017) and disease resistance (Liu et al. 2018). Dubois and Sakr (2012) sampled all rose plant organs and tissues, and leaves also in response to biotic and abiotic stresses. This early study was broad in its sampling, but the sequencing depth was limited. Hence, although it is the broadest source of transcribed MLO genes in rose, the data are mostly relevant for presence of gene expression, not for absence. We found 11 of the 19 RhMLO genes in the transcriptome data of Dubois and Sakr (2012). There were two plant tissues with relatively high expression levels of MLO genes, namely leaves from water-stressed plants and white young roots. Especially RhMLO3 and RhMLO4 were highly expressed in leaves from water-stressed plants while RhMLO1 and RhMLO2 were not. These results further suggest that RhMLO3 and RhMLO4 may play a different role compared to RhMLO1 and RhMLO2 in rose.
Two homologs (RhMLO17 and RhMLO19) clustered with the functional MLO susceptibility homologs from monocots in clade IV, which also contains FvMLO17 from strawberry and PpMLO12 from peach as well as four monocot MLO homologs. Whereas the S gene function for powdery mildew has been found for the monocot MLO genes in Clade IV, this was not so for the MLO genes in dicots (Kusch and Panstruga 2017). Further, RhMLO17 and RhMLO19 were not found in the transcriptome data of Old Blush.
RhMLO9 and RhMLO11 are in clade I, along with AtMLO4 and AtMLO11 which are involved in root thigmomorphogenesis. RhMLO10 and RhMLO12 are grouped in clade II along with AtMLO7, which is expressed during pollen tube growth. The available expression data for rose in ROSAseq are insufficient to be able to infer possible functions of the rose genes. Further research will be needed to link tissue-specific gene expression levels at specific developmental stages to a particular (a)biotic stimulus in a variety of different rose genotypes.
Allelic variants of RhMLO genes
Almost all modern rose cultivars are tetraploid, and are presumed to have been arisen by hybridization of a number of wild species through a long breeding history (Smulders et al. 2011). The relationship among cultivated and wild material has been investigated in several studies using different molecular techniques, for instance with AFLP (Koopman et al. 2008), SSRs (Liorzou et al. 2016; Tan et al. 2017; Qi et al. 2018) and SNPs (Zhang et al. 2013; Heo et al. 2017; Yan et al. 2018). The appearance of next-generation sequencing technologies has made it cost-efficient to detect genome-wide polymorphisms (Craig et al. 2008; Smulders et al. 2019) and study the hybrid origin of tetraploid rose more thoroughly.
We identified the alleles of 19 RhMLOs in 22 diploid or tetraploid roses using re-sequencing data and transcriptome data, and determined the SNPs among all the alleles in the coding regions. In total, we found 198 alleles for 19 RhMLO genes, an average of 10 alleles per gene. We detected these alleles on average 1.5 times across the set of genotypes. The inferred alleles have to be confirmed by PCR amplification or cloning and sequencing, but the fact that almost half of them were found in two or more accessions or species, and a few in four accessions and species, indicates that at least part of the predictions are fairly robust. The sharing of haplotypes (e.g. allele H1 of RhMLO1) between diploid species and tetraploid cultivars may indicate that these diploid species or close relatives have been involved in the formation of tetraploid roses (Zhang et al. 2013). Further research is needed including more wild species to be sequenced and using long read sequencing at suitable read depth. This will likely overcome the issue of finding partial sequences which makes it difficult to align MLO alleles for SNPs and haplotype characterization.
Here, we have corrected sequence alignments and SNP discovery, and then defined alleles manually after aligning. Some programs and algorithms may improve the speed and veracity for data analysis if more genes and accessions are included, such as QualitySNPng (Nijveen et al. 2013) and SAMtools. Indels have been found in some alleles during the procedure of manual correction. Some of these may represent errors of sequencing and assembling, but genetic variation in the form of indels may be related to the functionality of the alleles (Appiano et al. 2015). We did not find any indels in RhMLO1 and RhMLO4 in coding regions. However, we did find two long deletions in RhMLO2. One of them was in a conserved region of the encoded protein while the other one was relevant to the C-terminal D/E-F-S/T-F tetra-peptide sequence, which is one of several motifs characteristic for MLO orthologs. Since RhMLO2 is a functional gene, these two long indels could pinpoint natural mutants. In addition, two insertions in RhMLO3 and one deletion in RhMLO17 were found. These findings indicate possible natural loss-of-function alleles of RhMLO genes in clade IV or V, which may be introgressed into commercial breeding programs.
Next to existing loss-of-function alleles, new ones may be generated using random mutagenesis (e.g. with ethyl methanesulfonate, EMS) or by targeted mutagenesis with CRISPR/Cas. The latter technology is very useful for targeted mutagenesis in polyploids (Smulders et al. 2019), as all alleles of a gene or even of multiple genes can be targeted at once. As mentioned, a complication is that transformation systems for tetraploid roses still have suboptimal efficiency. Genome editing may be used to modify elite rose cultivars, but also to increase the frequency of non-functional alleles in a breeding program, so that homozygosity at S gene loci may be achieved without affecting the genetic diversity of other genes (Schaart et al. 2021).
Conclusion
In this study, we have identified 19 MLO homologs in rose using the recently published rose genome of the diploid garden rose Old Blush. In an allelic diversity analysis of resequencing data of 22 Rosa accessions of eight species, 198 different alleles were observed across the 19 RhMLO genes. Some alleles are shared between diploid wild species and tetraploid varieties, in line with the contribution of various diploid rose species to the formation of tetraploid roses. Furthermore, VIGS and transient RNAi assays both implied that the two homologs in clade V, RhMLO1 and likely also RhMLO2, are required for infection by powdery mildew. Based on this, the task ahead is to verify the function of RhMLO2 through stable RNAi, or using gene editing. For future use, it will also be important to test them on different powdery mildew isolates. Altogether, our results provide possibilities and direction in studying MLO functionality in rose.
Data access
All data are presented in the Supplementary material.
References
Andolfo G, Iovieno P, Ricciardi L et al (2019) Evolutionary conservation of MLO gene promoter signatures. BMC Plant Biol 19:150. https://doi.org/10.1186/s12870-019-1749-3
Appiano M, Catalano D, Santillan Martinez MI, Lotti C, Zheng Z, Visser RGF, Ricciardi L, Bai Y, Pavan SNC (2015) Monocot and dicot MLO powdery mildew susceptibility factors are functionally conserved in spite of the evolution of class-specific molecular features. BMC Plant Biol 15:257. https://doi.org/10.1186/s12870-015-0639-6
Bai Y, Pavan S, Zheng Z, Zappel NF, Reinstadler A, Lotti C, De Giovanni C, Ricciardi L, Lindhout P, Visser RGF, Theres K, Panstruga R (2008) Naturally occurring broad-spectrum powdery mildew resistance in a Central American tomato accession is caused by loss of mlo function. Mol Plant Microbe Interact 21:30–39. https://doi.org/10.1094/MPMI-21-1-0030
Berg JA, Appiano M, Santillán Martínez M, Hermans FWK, Vriezen WH, Visser RGF, Bai Y, Schouten HJ (2015) A transposable element insertion in the susceptibility gene CsaMLO8 results in hypocotyl resistance to powdery mildew in cucumber. BMC Plant Biol 15:243. https://doi.org/10.1186/s12870-015-0635-x
Büschges R, Hollricher K, Panstruga R, Simons G, Wolter M, Frijters A, van Daelen R, van der Lee T, Diergaarde P, Groenendijk J, Topsch S, Vos P, Salamini F, Schulze-Lefert P (1997) The barley Mlo gene: a novel control element of plant pathogen resistance. Cell 88:695–705. https://doi.org/10.1016/s0092-8674(00)81912-1
Chen L, Liu J, Liu Z et al (2021) Genome-wide identification and expression analysis of the MLO gene family reveal a candidate gene associated with powdery mildew susceptibility in bitter gourd (Momordica charantia). Eur J Plant Pathol 159:163–178. https://doi.org/10.1007/s10658-020-02152-0
Cheng H, Kong W, Lü J, Li J (2015) Analysis of powdery mildew resistance in wild melon MLO mutants. Hortic Plant J 1:165–171. https://doi.org/10.16420/j.issn.2095-9885.2015-0036
Consonni C, Humphry ME, Hartmann HA, Livaja M, Durner J, Westphal L, Vogel J, Lipka V, Kemmerling B, Schulze-Lefert P, Somerville SC, Panstruga R (2006) Conserved requirement for a plant host cell protein in powdery mildew pathogenesis. Nat Genet 38:716–720. https://doi.org/10.1038/ng1806
Craig DW, Pearson JV, Szelinger S, Sekar A, Redman M, Corneveaux JJ, Pawlowski TL, Laub T, Nunn G, Stephan DA, Homer N, Huentelman MJ (2008) Identification of genetic variants using bar-coded multiplexed sequencing. Nat Methods 5:887–893. https://doi.org/10.1038/nmeth.1251
Debener T, Byrne DH (2014) Disease resistance breeding in rose: current status and potential of biotechnological tools. Plant Sci 228:107–117. https://doi.org/10.1016/j.plantsci.2014.04.005
Deshmukh R, Singh VK, Singh BD (2014) Comparative phylogenetic analysis of genome-wide Mlo gene family members from Glycine max and Arabidopsis thaliana. Mol Genet Genom 289:345–359. https://doi.org/10.1007/s00438-014-0811-y
Deshmukh R, Singh VK, Singh BD (2017) Mining the Cicer arietinum genome for the mildew locus O (Mlo) gene family and comparative evolutionary analysis of the Mlogenes from Medicago truncatula and some other plant species. J Plant Res 130:239–253. https://doi.org/10.1007/s10265-016-0868-2
Devoto A, Hartmann HA, Piffanelli P, Elliott C, Simmons C, Taramino G, Goh CS, Cohen FE, Emerson BC, Schulze-Lefert P, Panstruga R (2003) Molecular phylogeny and evolution of the plant-specific seven-transmembrane MLO family. J Mol Evol 56:77–88. https://doi.org/10.1007/s00239-002-2382-5
Dubois A, Sakr S (2012) Transcriptome database resource and gene expression atlas for the rose. BMC Genom 13:638–638. https://doi.org/10.1186/1471-2164-13-638
Edger PP, VanBuren R, Colle M, Poorten TJ, Wai CM, Niederhuth CE, Alger EI, Ou S, Acharya CB, Wang J, Callow P, McKain MR, Shi J, Collier C, Xiong Z, Mower JP, Slovin JP, Hytonen T, Jiang N, Childs KL, Knapp SJ (2018) Single-molecule sequencing and optical mapping yields an improved genome of woodland strawberry (Fragaria vesca) with chromosome-scale contiguity. Gigascience 7:1–7
Eichmann R, Huckelhoven R (2008) Accommodation of powdery mildew fungi in intact plant cells. J Plant Physiol 165:5–18
Elliott C, Fasong Zhou F, Spielmeyer W, Panstruga R, Schulzelefert P (2002) Functional conservation of wheat and rice Mlo Orthologs in defense modulation to the powdery mildew fungus. Mol Plant Microbe Interact 15:1069–1077
Feechan A, Jermakow AM, Torregrosa L, Panstrugar R, Dry IB (2008) Identification of grapevine MLO gene candidates involved in susceptibility to powdery mildew. Funct Plant Biol 35:1255–1266
Filiz E, Vatansever R (2018) Genome-wide identification of mildew resistance locus O (MLO) genes in tree model poplar (Populus trichocarpa): powdery mildew management in woody plants. Eur J Plant Pathol 152:95–109. https://doi.org/10.1007/s10658-018-1454-3
Fu XL, Lu YG, Liu XD, Li JQ (2009) Crossability barriers in the interspecific hybridization between Oryza sativa and O. meyeriana. J Integr Plant Biol 51:21–28
Fujimura T, Sato S, Tajima T, Arai M (2016) Powdery mildew resistance in the Japanese domestic tobacco cultivar Kokubu is associated with aberrant splicing of MLO orthologues. Plant Pathol 65:1358–1365
Geike J, Kaufmann H, Schürmann F, Debener T (2015) Targeted mutagenesis of MLO-homologous genes in the rose genome. Acta Hortic 1087:507–513. https://doi.org/10.17660/ActaHortic.2015.1087.68
Han Y, Wan H, Cheng T, Wang J, Yang W, Pan H, Zhang Q (2017) Comparative RNA-seq analysis of transcriptome dynamics during petal development in Rosa chinensis. Sci Rep 7:43382
Harland SC (1948) Inheritance of immunity to mildew in Peruvian forms of Pisum sativum. Heredity 2:263–269
Heo M-S, Han K, Kwon J-K, Kang B-C (2017) Development of SNP markers using genotyping-by-sequencing for cultivar identification in rose (Rosa hybrida). Hortic Environ Biotechnol 58:292–302
Hibrand Saint-Oyant L, Ruttink T, Hamama L, Kirov I, Lakhwani D, Zhou NN, Bourke PM, Daccord N, Leus L, Schulz D, Van de Geest H, Hesselink T, Van Laere K, Debray K, Balzergue S, Thouroude T, Chastellier A, Jeauffre J, Voisine L, Gaillard S, Borm TJA, Arens P, Voorrips RE, Maliepaard C, Neu E, Linde M, Le Paslier MC, Bérard A, Bounon R, Clotault J, Choisne N, Quesneville H, Kawamura K, Aubourg S, Sakr S, Smulders MJM, Schijlen E, Bucher E, Debener T, De Riek J, Foucher F (2018) A high-quality genome sequence of Rosa chinensis to elucidate ornamental traits. Nat Plants 4:473–484
Humphry M, Reinstädler A, Ivanov S, Bisseling T, Panstruga R (2011) Durable broad-spectrum powdery mildew resistance in pea er1 plants is conferred by natural loss-of-function mutations in PsMLO1. Mol Plant Pathol 12:866–878
Jørgensen IH (1992) Discovery, characterization and exploitation of Mlo powdery mildew resistance in barley. Euphytica 63:141–152. https://doi.org/10.1007/BF00023919
Jung S, Lee T, Cheng CH, Buble K, Zheng P, Yu J, Humann J, Ficklin SP, Gasic K, Scott K, Frank M, Ru S, Hough H, Evans K, Peace C, Olmstead M, DeVetter LW, McFerson J, Coe M, Wegrzyn JL, Staton ME, Abbott AG, Main D (2019) 15 years of GDR: new data and functionality in the genome database for rosaceae. Nucl Acids Res 47:D1137–D1145. https://doi.org/10.1093/nar/gky1000
Kaufmann H, Qiu X, Wehmeyer J, Debener T (2012) Isolation, molecular characterization, and mapping of four rose MLO orthologs. Front Plant Sci 3:244. https://doi.org/10.3389/fpls.2012.00244
Kim MC, Lee SH, Kim JK, Chun HJ, Choi MS, Chung WS, Moon BC, Kang CH, Park CY, Yoo JH, Kang YH, Koo SC, Koo YD, Jung JC, Kim ST, Schulze-Lefert P, Lee SY, Cho MJ (2002) Mlo, a modulator of plant defense and cell death, is a novel calmodulin-binding protein. Isolation and characterization of a rice Mlo homologue. J Biol Chem 277:19304–19314. https://doi.org/10.1074/jbc.M108478200
Koning-Boucoiran CF, Esselink GD, Vukosavljev M, van Westende WPC, Gitonga VW, Krens FA, Voorrips RE, van de Weg WE, Schulz D, Debener T, Maliepaard C, Arens P, Smulders MJM (2015) Using RNA-Seq to assemble a rose transcriptome with more than 13,000 full-length expressed genes and to develop the WagRhSNP 68k Axiom SNP array for rose (Rosa L.). Front Plant Sci 6:249. https://doi.org/10.3389/fpls.2015.00249
Koopman WJ, Wissemann V, De Cock K, Van Huylenbroeck J, De Riek J, Sabatino GJ, Visser D, Vosman B, Ritz CM, Maes B, Werlemark G, Nybom H, Debener T, Linde M, Smulders MJM (2008) AFLP markers as a tool to reconstruct complex relationships: a case study in Rosa (Rosaceae). Am J Bot 95:353–366. https://doi.org/10.3732/ajb.95.3.353
Kusch S, Panstruga R (2017) mlo-based resistance: an apparently universal “Weapon” to defeat powdery mildew disease. Plant Microbe Interact 30:179–189. https://doi.org/10.1094/MPMI-12-16-0255-CR
Li Y, Pi M, Gao Q, Liu Z, Kang C (2019) Updated annotation of the wild strawberry Fragaria vesca V4 genome. Hortic Res 6:61. https://doi.org/10.1038/s41438-019-0142-6
Li W, Geng Z, Zhang C, Wang K, Jiang X (2021) Whole-genome characterization of Rosa chinensis AP2/ERF transcription factors and analysis of negative regulator RcDREB2B in Arabidopsis. BMC Genom 22:90. https://doi.org/10.1186/s12864-021-07396-6
Liorzou M, Pernet A, Li S, Chastellier A, Thouroude T, Michel G, Malecot V, Gaillard S, Briee C, Foucher F, Oghina-Pavie C, Clotault J, Grapin A (2016) Nineteenth century French rose (Rosa sp.) germplasm shows a shift over time from a European to an Asian genetic background. J Exp Bot 67:4711–4725. https://doi.org/10.1093/jxb/erw269
Liu YL, Schiff M, Dinesh-Kumar SP (2002) Virus-induced gene silencing in tomato. Plant J 31:777–786
Liu X, Cao X, Shi S, Zhao N, Li D, Fang P, Chen X, Qi W, Zhang Z (2018) Comparative RNA-Seq analysis reveals a critical role for brassinosteroids in rose (Rosa hybrida) petal defense against Botrytis cinerea infection. BMC Genet 19:62. https://doi.org/10.1186/s12863-018-0668-x
Liu X, Li D, Zhang S, Xu Y, Zhang Z (2019) Genome-wide characterization of the rose (Rosa chinensis) WRKY family and role of RcWRKY41 in gray mold resistance. BMC Plant Biol 19:522. https://doi.org/10.1186/s12870-019-2139-6
Lopez Arias DC, Chastellier A, Thouroude T et al (2020) Characterization of black spot resistance in diploid roses with QTL detection, meta-analysis and candidate-gene identification. Theor Appl Genet 133:3299–3321. https://doi.org/10.1007/s00122-020-03670-5
Lyngkjaer MF, Newton AC, Atzema JL, Baker SJ (2000) The Barley mlo-gene: an important powdery mildew resistance source. Agronomie 20:745–756
Menz I, Lakhwani D, Clotault J, Linde M, Foucher F, Debener T (2020) Analysis of the Rdr1 gene family in different Rosaceae genomes reveals an origin of an R-gene cluster after the split of Rubeae within the Rosoideae subfamily. PLoS ONE 15:e0227428. https://doi.org/10.1371/journal.pone.0227428
Nie J, Wang Y, He H, Guo C, Zhu W, Pan J, Li D, Lian H, Pan J, Cai R (2015) Loss-of-function mutations in CsMLO1 confer durable powdery mildew resistance in cucumber (Cucumis sativus L.). Front Plant Sci 6:1155
Nijveen H, van Kaauwen M, Esselink DG, Hoegen B, Vosman B (2013) QualitySNPng: a user-friendly SNP detection and visualization tool. Nucl Acids Res 41:W587-590
Panstruga R (2005) Discovery of novel conserved peptide domains by ortholog comparison within plant multi-protein families. Plant Mol Biol 59:485–500
Pavan S, Jacobsen E, Visser RG, Bai Y (2010) Loss of susceptibility as a novel breeding strategy for durable and broad-spectrum resistance. Mol Breed 25:1–12
Pavan S, Schiavulli A, Appiano M, Miacola C, Visser RGF, Bai Y, Lotti C, Ricciardi L (2013) Identification of a complete set of functional markers for the selection of er1 powdery mildew resistance in Pisum sativum L. Mol Breed 31:247–253
Pessina S, Pavan S, Catalano D, Gallotta A, Visser RG, Bai Y, Malnoy M, Schouten HJ (2014) Characterization of the MLO gene family in Rosaceae and gene expression analysis in Malus domestica. BMC Genom 15:618
Pessina S, Angeli D, Martens S, Visser RG, Bai Y, Salamini F, Velasco R, Schouten HJ, Malnoy M (2016a) The knock-down of the expression of MdMLO19 reduces susceptibility to powdery mildew (Podosphaera leucotricha) in apple (Malus domestica). Plant Biotechnol J 14:2033–2044
Pessina S, Lenzi L, Perazzolli M, Campa M, Costa LD, Urso S, Valè G, Salamini F, Velasco R, Malnoy M (2016b) Knock down of MLO genes reduces susceptibility to powdery mildew in grapevine. Hortic Res 3:16016. https://doi.org/10.1038/hortres.2016.16
Piffanelli P, Ramsay L, Waugh R, Benabdelmouna A, D’Hont A, Hollricher K, Jørgensen JH, Schulze-Lefert P, Panstruga R (2004) A barley cultivation-associated polymorphism conveys resistance to powdery mildew. Nature 430:887–891. https://doi.org/10.1038/nature02781
Pirrello C, Zeilmaker T, Bianco L, Giacomelli L, Moser C, Vezzulli S (2021) Mining grapevine downy mildew susceptibility genes: a resource for genomics-based breeding and tailored gene editing. Biomolecules 11:181. https://doi.org/10.3390/biom11020181
Polanco C, Sáenz de Miera LE, Bett K, Pérez de la Vega M (2018) A genome-wide identification and comparative analysis of the lentil MLO genes. PLoS ONE 13:e0194945. https://doi.org/10.1371/journal.pone.0194945
Qi W, Chen X, Fang P, Shi S, Li J, Liu X, Cao X, Zhao N, Hao H, Li Y, Han Y, Zhang Z (2018) Genomic and transcriptomic sequencing of Rosa hybrida provides microsatellite markers for breeding, flower trait improvement and taxonomy studies. BMC Plant Biol 18:119
Qiu X, Jian H, Wang Q, Tang K, Bao M (2015a) Expression pattern analysis of Four Mlo Genes from Rose. J Am Soc Hortic Sci 140:333–338
Qiu X, Wang Q, Zhang H, Jian H, Zhou N, Ji C, Yan H, Bao M, Tang K (2015b) Antisense RhMLO1 gene transformation enhances resistance to the powdery mildew pathogen in Rosa multiflora. Plant Mol Biol Rep 33:1659–1665
Qiu X, Wang Q, Zhang H, Jian H, Zhou N, Ji C, Yan H, Bao M, Tang K (2016) Antisense RhMLO1 Gene Transformation Enhances Resistance to the Powdery Mildew Pathogen in Rosa multiflora. Plant Mol Biol Rep 33:1659–1665. https://doi.org/10.1007/s11105-015-0862-1
R Core Team (2016) R: A Language and Environment for Statistical Computing. R Foundation for Statistical Computing, Vienna, Austria. https://www.Rproject.org/
Ramakers C, Ruijter JM, Lekanne Deprez RH, Moorman AFM (2003) Assumption-free analysis of quantitative real-time polymerase chain reaction (PCR) data. Neurosci Lett 339:62–66. https://doi.org/10.1016/S0304-3940(02)01423-4
Schaart JG, Van de Wiel CCM, Smulders MJM (2021) Genome editing of polyploid crops: prospects, achievements and bottlenecks. Transgenic Res. https://doi.org/10.1007/s11248-021-00251-0
Schmidt N, Merker M, Becker D (2012) Novel high-throughput RNAi vectors for plant biotechnology. Plant Breed 131:453–456. https://doi.org/10.1111/j.1439-0523.2012.01953.x
Shulaev V, Sargent DJ, Crowhurst RN, Mockler TC, Folkerts O, Delcher AL et al (2010) The genome of woodland strawberry (Fragaria vesca). Nat Genet 43:109–116
Smulders MJM, Arens P, Koning-Boucoiran CFS, Gitonga VW, Krens FA, Atanassov A, Atanassov I, Rusanov KE, Bendahmane M, Dubois A, Ray-mond O, Caissard JC, Baudino S, Crespel L, Gudin S, Ricci SC, Kovatcheva N, Van Huylenbroeck J, Leus L, Wissemann V, Zimmermann H, Hensen I, Werlemark G, Nybom H (2011) Rosa. In: Kole C (ed) Wild crop relatives: genomic and breeding resources. Springer, Berlin Heidelberg, pp 243–275. https://doi.org/10.1007/978-3-642-21201-7_12
Smulders MJM, Arens P, Bourke PM, Debener T, Linde M, De Riek J, Leus L, Ruttink T, Baudino S, Hibrant Saint-Oyant L, Clotault J, Foucher F (2019) In the name of the rose: a roadmap for rose research in the genome era. Hortic Res 6:65. https://doi.org/10.1038/s41438-019-0156-0
Smulders MJM, Bourke PM, Tumino G, Voorrips RE, Maliepaard C, Arens P (2020) Using molecular markers in breeding: ornamentals catch up. Acta Hortic 1283:49–54. https://doi.org/10.17660/ActaHortic.2020.1283.8
Tan J, Wang J, Luo L, Yu C, Xu T, Wu Y, Cheng T, Wang J, Pan H, Zhang Q (2017) Genetic relationships and evolution of old Chinese garden roses based on SSRs and chromosome diversity. Sci Rep 7:15437. https://doi.org/10.1038/s41598-017-15815-6
Vieira J, Pimenta J, Gomes A et al (2021) The identification of the Rosa S-locus and implications on the evolution of the Rosaceae gametophytic self-incompatibility systems. Sci Rep 11:3710. https://doi.org/10.1038/s41598-021-83243-8
Vukosavljev M, Zhang J, Esselink GD, Van Westende WPC, Cox P, Visser RGF, Arens P, Smulders MJM (2013) Genetic diversity and differentiation in roses: a garden rose perspective. Sci Hortic 162:320–332. https://doi.org/10.1016/j.scienta.2013.08.015
Vukosavljev M, Arens P, Voorrips RE, Vant Westende WPC, Esselink GD, Bourke PM, Cox P, Van de Weg WE, Visser RGF, Maliepaard C, Smulders MJM (2016) High-density SNP-based genetic maps for the parents of an outcrossed and a selfed tetraploid garden rose cross, inferred from admixed progeny using the 68k rose SNP array. Hortic Res 3:16052. https://doi.org/10.1038/hortres.2016.52
Win KT, Zhang C, Lee S (2018) Genome-wide identification and description of MLO family genes in pumpkin (Cucurbita maxima Duch.). Hortic Environ Biotechnol 59:397–410. https://doi.org/10.1007/s13580-018-0036-9
Winterhagen P, Howard SF, Qiu W, Kovács LG (2008) Transcriptional up-regulation of grapevine MLO genes. Am J Enol Vitic 59:159–168
Wolter M, Hollricher K, Salamini F, Schulze-Lefert P (1993) The mlo resistance alleles to powdery mildew infection in barley trigger a developmentally controlled defence mimic phenotype. Mol Gen Genet 239:122–128
Xiang GS, Zhang H, Jian HY, Yan HJ, Wang QG, Zhou NN, Li SB, Tang KX, Qiu XQ (2019) De Novo assembly and characterization of the transcriptome of susceptible and resistant rose species in response to powdery mildew. Sci Hortic 257:108653
Yan M, Byrne DH, Klein PE, Yang J, Dong Q, Anderson N (2018) Genotyping-by-sequencing application on diploid rose and a resulting high-density SNP-based consensus map. Hortic Res 5:17
Zhang J, Esselink GD, Che D, Fougère-Danezan M, Arens P, Smulders MJM (2013) The diploid origins of allopolyploid rose species studied using single nucleotide polymorphism haplotypes flanking a microsatellite repeat. J Hortic Sci Biotechnol 88:85–92. https://doi.org/10.1080/14620316.2013.11512940
Zheng Z, Nonomura T, Appiano M, Pavan S, Matsuda Y, Toyoda H, Wolters AM, Visser RGF, Bai Y (2013) Loss of function in Mlo orthologs reduces susceptibility of pepper and tomato to powdery mildew disease caused by Leveillula taurica. PLoS ONE 8:e70723. https://doi.org/10.1371/journal.pone.0070723
Zheng Z, Appiano M, Pavan S, Bracuto V, Ricciardi L, Visser RGF, Wolters A-MA, Bai Y (2016) Genome-wide study of the tomato SlMLO gene family and its functional characterization in response to the powdery mildew fungus Oidium neolycopersici. Front Plant Sci 7:380. https://doi.org/10.3389/fpls.2016.00380
Acknowledgements
This work was supported by the National Natural Science Foundation of China (Grants Number 31501791) to Zhao Zhang, by a China Scholarship Council fellowship to Peihong Fang, and by TKI T&U (TU18142). We acknowledge Ms. Zhiyi Yu and Ms. Shuyao Deng for technical assistance and plant care.
Author information
Authors and Affiliations
Contributions
PA, MS, TD, YB and ZZ conceived the study. PF performed the bioinformatics analysis, produced and tested the VIGS plants, and wrote the first draft of the manuscript. XL supervised the VIGS work in the laboratory. XZ cloned MLO sequences from rose and constructed TRV vectors. ZZ supervised the VIGS experiments and wrote the VIGS part of the manuscript. DL, FF and JC produced the resequencing data, advised on the bioinformatics, and revised the manuscript. JG, HK and TD performed the RNAi experiments and analysed and described them. PA and MS supervised the bioinformatics analysis and revised the manuscript. ZZ, PA, YB, TD and MS performed the final editing. All authors approved the final version.
Corresponding authors
Ethics declarations
Conflict of interest
The authors declare that they have no conflict of interest.
Additional information
Communicated by Reinhard Toepfer.
Publisher's Note
Springer Nature remains neutral with regard to jurisdictional claims in published maps and institutional affiliations.
Supplementary Information
Below is the link to the electronic supplementary material.
Rights and permissions
Open Access This article is licensed under a Creative Commons Attribution 4.0 International License, which permits use, sharing, adaptation, distribution and reproduction in any medium or format, as long as you give appropriate credit to the original author(s) and the source, provide a link to the Creative Commons licence, and indicate if changes were made. The images or other third party material in this article are included in the article's Creative Commons licence, unless indicated otherwise in a credit line to the material. If material is not included in the article's Creative Commons licence and your intended use is not permitted by statutory regulation or exceeds the permitted use, you will need to obtain permission directly from the copyright holder. To view a copy of this licence, visit http://creativecommons.org/licenses/by/4.0/.
About this article
Cite this article
Fang, P., Arens, P., Liu, X. et al. Analysis of allelic variants of RhMLO genes in rose and functional studies on susceptibility to powdery mildew related to clade V homologs. Theor Appl Genet 134, 2495–2515 (2021). https://doi.org/10.1007/s00122-021-03838-7
Received:
Accepted:
Published:
Issue Date:
DOI: https://doi.org/10.1007/s00122-021-03838-7