Abstract
Single- and double-electron capture (SEC and DEC) processes occurring in O6+ and H2 collisions are investigated in a wide-energy domain ranging from 0.1 to 100 keV u−1. Total and partial cross sections are calculated using a three-center, two-active-electron, semiclassical nonperturbative approach. To date, our close-coupling description of the collision is the most elaborate one in terms of accounting for electron correlation, molecular structures, and active channels. Our results are, in general, in good agreement with the available experimental ones for both total and partial cross sections. The comparison between the present calculations and available experimental data suggests that about 70% of the autoionization double capture may contribute to the measured SEC cross sections through postcollisional autoionization, while the stabilization of 30% of doubly excited states via the autotransfer to Rydberg states mechanism contributes to the measured DEC ones. Furthermore, we extend the understanding of the electron-capture processes on this system to impact energies above 20 keV u−1 for which no data exists. Our work provides new data for these electronic processes, which will be helpful for modeling astrophysical X-ray emissions induced by charge exchange.
Export citation and abstract BibTeX RIS

Original content from this work may be used under the terms of the Creative Commons Attribution 4.0 licence. Any further distribution of this work must maintain attribution to the author(s) and the title of the work, journal citation and DOI.
1. Introduction
In atomic physics, charge exchange (or electron capture) is a fundamental process in which an ion takes one or more electrons from the target atom or molecule during collisions. The charge-exchange process was initially studied as a diagnostic for laboratory plasmas (Isler 1977; Hoekstra et al. 1998). Since the first discovery of X-ray emission from comet C/Hyakutake (Lisse et al. 1996) and its interpretation as the result of charge-exchange collisions between highly charged solar-wind oxygen ions and the cometary neutral atmosphere (Cravens 1997), the investigations on charge-exchange collisions have expanded considerably. Over the past decades, the presence and importance of charge-exchange X-ray emission have been confirmed in a broad range of astrophysical environments, particularly in the interfaces where solar-wind ions interact with neutrals in comets and planetary atmospheres (Hoekstra et al. 1998; Cravens 2000, 2002; Holmström et al. 2001; Beiersdorfer et al. 2003; Lallement 2004; Branduardi-Raymont et al. 2007; Robertson et al. 2009; Wargelin et al. 2014). In addition, charge exchange is also considered as a potential mechanism responsible for X-ray emission from stellar winds of supergiants (Pollock 2007), shock rims of supernova remnants (Katsuda et al. 2011; Cumbee et al. 2014), star-forming galaxies (Liu et al. 2012; Zhang et al. 2014), active galactic nuclei (Gu et al. 2017), etc.
Elaborate models (Cravens 1997; Gunell et al. 2005; Smith et al. 2012; Cumbee et al. 2014, 2017; Gu et al. 2016; Liang et al. 2021) have been developed to simulate the astrophysical X-ray emissions induced by charge exchange. In practice, detailed knowledge of charge-exchange cross sections for the relevant ion–neutral collisions are needed as input data. In the charge-exchange model of Smith et al. (2012), which does not include realistic electron-capture cross sections, the hydrogenic method was adopted to obtain the total cross sections, and the approximation of Janev & Winter (1985) was used to obtain the n ℓ-resolved cross sections. Cumbee et al. (2014, 2017) constructed a comprehensive model using the KRONOS 5 charge-exchange database, where the cross sections are primarily obtained via multichannel Landau–Zener calculations, supplemented by the results from more accurate quantum-mechanical molecular-orbital close-coupling, atomic-orbital close-coupling, and classical trajectory Monte Carlo methods when available. However, only single-electron capture processes are taken into account in the model. More recently, Liang et al. (2021) set up a charge-exchange model with the inclusion of double-electron capture processes, showing a nonnegligible contribution from the latter processes. The development of these sophisticated charge-exchange models presents an urgent requirement of accurate electron-capture cross sections for relevant ion–neutral collisions.
In the present work, we are interested in the most abundant solar wind-heavy ion O6+ (Schwadron & Cravens 2000) in collisions with the H2 molecule. The electronic processes can be broadly categorized into three classes; they are: (i) single-electron capture (SEC) processes,



(ii) double-electron capture (DEC) into bound states,

and (iii) autoionization double capture (ADC),

Experimentally, these electronic processes have been extensively studied in the past. Crandall et al. (1977, 1979) have measured total SEC cross sections in the energy range of 1–6 keV u−1 by using the Oak Ridge National Laboratory Penning ion gauge (ORNL-PIG) ion source, and the charge-state analysis from the electrostatic analyzer was used to determine the charge-exchange cross sections. Phaneuf et al. (1982) extended the SEC measurements to a wider energy region from 0.01 to 10 keV u−1 using the ORNL-PIG ion source for energies above 0.4 keV u−1 and the pulsed-laser ion source for lower energies; the time-of-flight technique permitted cross sections for different charge states to be measured simultaneously. Later on, by means of photon emission spectroscopy, Dijkkamp et al. (1985) and Lubinski et al. (2000) measured absolute state-resolved SEC cross sections for energies 0.94–7.5 keV u−1 and 0.007–1.3 keV u−1, respectively. In the former experiment, total SEC cross sections were also measured by means of charge-state analysis at 0.5–5 keV u−1. More recently, both total SEC and DEC cross sections were measured by Machacek et al. (2014) at 1.17 and 2.33 keV u−1 and Han et al. (2021) for energies ranging from 2.63 to 19.50 keV u−1. Though with different apparatuses, electron cyclotron resonance ion source was used in both experiments, and cross sections for ions in different charge states due to the electron-capture process were measured by applying an appropriate potential field to deflect the ions (see also Greenwood et al. 2001 and Zhang et al. 2018 for more details about the experiments). Despite extensive experimental studies on O6+ and H2 collisions, only very few data are available for state-resolved SEC processes limited in the energy range of 0.007–10 keV u−1 (Dijkkamp et al. 1985; Lubinski et al. 2000), and the DEC ones for energies within 1–20 keV u−1 (Machacek et al. 2014; Han et al. 2021). From the theoretical point of view, investigating this collision system remains challenging due to the strong coupling between various channels, the multicenter features of the H2 molecular target, and the role of the electronic correlation, notably in the important two-electron processes invoked just above. To our knowledge, no detailed theoretical investigation for O6+ and H2 collisions has been reported so far.
In this work, we study theoretically single- and double-electron capture processes in a wide-energy domain ranging from 0.1 to 100 keV u−1. We use a two-active-electron, three-center, semiclassical, asymptotic-state close-coupling (SCASCC) method with large basis sets, ensuring a controlled convergence of the cross sections and providing physical insight on this collision system. Total and state-resolved SEC, DEC, and ADC cross sections are presented and compared with available experimental results. In particular, the contributions of ADC processes to the SEC and DEC cross sections are analyzed in detail.
The organization of the paper is as follows. In Section 2, we briefly outline the SCASCC method used in the present calculations. In Section 3, we present a detailed analysis of the total and partial cross sections for SEC, DEC, and ADC processes, including direct comparisons with available experimental data. A brief conclusion follows in Section 4. Atomic units are used throughout, unless explicitly indicated otherwise.
2. Theoretical Methods
In the present work, the cross sections of the electronic processes occurring in O6+ and H2 collisions are calculated using a two-active-electron SCASCC method that has been previously described (e.g., in Sisourat et al. 2011; Gao et al. 2017, 2019, 2021; Zhang et al. 2020). Here we just outline the main features of the approach as they relate to the present calculations. The two-electron time-dependent Schrödinger equation (TDSE) is written as

with H the electronic Hamiltonian defined as

where
r
i
and are the position vector of the electrons with respect to the center of mass of the target and to the projectile nuclei, respectively. The relative projectile-target position
R
(t) defines the trajectory in the usual straight-line, constant velocity approximation
R
(t) =
b
+
v
t, where
b
and
v
are the impact parameter and velocity, respectively (see Figure 1). Note that our approach is based on the rovibrational sudden approximation: the nuclei of the molecular target are frozen (i.e., fixed
R
AB
). This assumption is valid in the energy range considered in this work since the electronic processes take place in a timescale much shorter (sub-femtosecond) than the vibrational motion of the molecular target (>10 fs).
Figure 1. Collision geometry: the impact parameter b and velocity v define the collision plane (xOz), where Φb is the azimuthal angle used to define the direction of b with respect to the x-axis (for convenience Φb = 0 in the present case). The center of mass of the diatomic molecule defines the origin of the reference frame. Two target nuclei A and B are separated by the internuclear vector R AB . The angles Θm and Φm define the molecular orientation. The position of the two electrons are denoted as r 1 and r 2, and r 12 = r 1 − r 2 is their relative position.
Download figure:
Standard image High-resolution imageThe term VT (VP ) in Equation (5) defines the electron–target (electron–projectile) nuclei interaction. For the present collision system, these terms are defined as
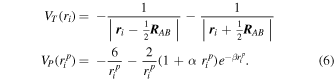
VP describes the interaction between an active electron and the O6+ ion in the frozen-core electron approximation in which the inner-shell electrons are assumed to be inactive. The variational parameters α = 5.074 and β = 10.148 were obtained through an optimization procedure in order to reproduce the O5+ doublet and O4+ singlet spectrum (see Table 1).
Table 1. Energies (EGTO, in au) of O5+ and O4+ States Obtained in This Work, Compared with Energies ENIST from NIST (Kramida et al. 2021) and Eref from Bachau et al. (1990)
O5+ | |||||||
---|---|---|---|---|---|---|---|
State | EGTO | ENIST | Δ | State | EGTO | ENIST | Δ |
1s22s2Se | −5.0746 | −5.0758 | −0.02% | 1s24p2Po | −1.1386 | −1.1419 | −0.29% |
1s22p2Po | −4.6108 | −4.6350 | −0.52% | 1s24d2De | −1.1192 | −1.1259 | −0.60% |
1s23s2Se | −2.1609 | −2.1595 | 0.06% | 1s24f2Fo | −1.1180 | −1.1251 | −0.63% |
1s23p2Po | −2.0325 | −2.0403 | −0.38% | 1s25s2Se | −0.7533 | −0.7532 | 0.01% |
1s23d2De | −1.9910 | −2.0018 | −0.54% | 1s25p2Po | −0.7259 | −0.7287 | −0.38% |
1s24s2Se | −1.1913 | −1.1906 | 0.06% | 1s25d2De | −0.7123 | −0.7206 | −1.14% |
O4+(bound) | O4+(autoionizing) | ||||||
State | EGTO | ENIST | Δ | State | EGTO | Eref | Δ |
1s22s2 1Se | −9.2556 | −9.2615 | −0.06% | 1s23s2 1Se | −3.9621 | −3.9654 | −0.08% |
1s22s2p1Po | −8.5026 | −8.5379 | −0.41% | 1s23s3d1De | −3.7880 | −3.7900 | −0.05% |
1s22p2 1De | −8.1552 | −8.2057 | −0.62% | 1s23s3p1Po | −3.7681 | −3.7823 | −0.38% |
1s22p2 1Se | −7.8948 | −7.9497 | −0.69% | 1s23p3d1Do | −3.6808 | −3.6663 | 0.40% |
1s22s3s1Se | −6.7023 | −6.7041 | −0.03% | 1s23p21De | −3.6464 | −3.6336 | 0.35% |
1s22s3p1Po | −6.6058 | −6.6151 | −0.14% | 1s23p2 1Se | −3.6158 | −3.6445 | −0.79% |
1s22s3d1De | −6.4554 | −6.4702 | −0.23% | 1s23p3d1Fo | −3.5253 | −3.5356 | −0.29% |
1s22p3s1Po | −6.2100 | −6.2339 | −0.38% | 1s23d3d1Ge | −3.4847 | −3.5154 | −0.87% |
1s22p3p1Pe | −6.1665 | −6.1965 | −0.48% | 1s23p3d1Po | −3.4541 | −3.4661 | −0.34% |
1s22p3d1Do | −6.0638 | −6.0965 | −0.54% | 1s23d2 1De | −3.4477 | −3.4737 | −0.75% |
1s22p3p1De | −6.0531 | −6.0849 | −0.52% | 1s23d2 1Se | −3.2955 | −3.3231 | −0.83% |
1s22p3p1Se | −6.0151 | −6.0373 | −0.37% | 1s23s4s1Se | −3.0915 | −3.0907 | 0.03% |
1s22p3d1Fo | −5.9789 | −6.0130 | −0.57% | 1s23s4p1Po | −3.0631 | −3.0698 | −0.22% |
1s22p3d1Po | −5.9507 | −5.9842 | −0.56% | 1s23s4d1De | −3.0138 | −3.0224 | −0.28% |
1s22s4s1Se | −5.9160 | −5.9277 | −0.20% | 1s23s4f1Fo | −2.9513 | −2.9780 | −0.90% |
1s22s4p1Po | −5.8911 | −5.8995 | −0.14% | 1s23p4p1Pe | −2.9421 | −2.9568 | −0.50% |
1s22s4d1Do | −5.8505 | −5.8612 | −0.18% | 1s23p4s1Po | −2.9420 | −2.9553 | −0.45% |
1s22s4f1Fo | −5.8210 | −5.8450 | −0.41% | 1s23d4s1De | −2.9348 | −2.9464 | −0.39% |
1s22s5s1Se | −5.6207 | ⋯ | ⋯ | 1s23p4d1Fo | −2.8928 | −2.9127 | −0.68% |
1s22s5p1Po | −5.5915 | −5.6052 | −0.24% | 1s23p4d1Do | −2.8892 | −2.9061 | −0.58% |
1s22s5d1De | −5.5209 | −5.5784 | −1.03% | 1s23p4d1Po | −2.8823 | −2.8995 | −0.59% |
1s22p4s1Po | −5.4784 | −5.5058 | −0.50% | 1s23d4p1Do | −2.8666 | −2.8808 | −0.49% |
1s22p4p1Pe | −5.4548 | −5.4816 | −0.49% | 1s23p4p1Se | −2.8662 | −2.8715 | −0.18% |
1s22p4d1Do | −5.4164 | −5.4440 | −0.51% | 1s23p4f1Fe | −2.8608 | −2.8838 | −0.80% |
1s22p4p1De | −5.4138 | −5.4439 | −0.55% | 1s23p4p1De | −2.8536 | −2.8648 | −0.39% |
1s22p4p1So | −5.3842 | −5.4368 | −0.97% | 1s23d4d1Fe | −2.8406 | −2.8591 | −0.65% |
1s22p4f1Ge | −5.3743 | −5.4071 | −0.61% | 1s23d4d1Pe | −2.8214 | −2.8375 | −0.57% |
1s22p4d1Fo | −5.3782 | −5.4016 | −0.43% | 1s23p4f1Ge | −2.8179 | −2.8466 | −1.01% |
1s22p4d1Po | −5.3684 | −5.4002 | −0.59% | 1s23d4f1Go | −2.8070 | −2.8418 | −1.22% |
1s22p5p1Pe | −5.1400 | −5.1673 | −0.53% | 1s23d4f1Fo | −2.7862 | −2.7719 | 0.52% |
1s22p5p1De | −5.1093 | −5.1497 | −0.78% | 1s23p4f1De | −2.7784 | −2.8131 | −1.23% |
1s22p5d1Do | −5.0847 | −5.1485 | −1.24% | 1s23d4f1Do | −2.7745 | −2.8042 | −1.06% |
1s22p5d1Fo | −5.0803 | −5.1316 | −1.00% | 1s23d4p1Po | −2.7724 | −2.7910 | −0.67% |
1s23d4d1De | −2.7535 | −2.7799 | −0.95% | ||||
1s23d4d1Ge | −2.7447 | −2.7853 | −1.46% | ||||
1s23s5s1Se | −2.7335 | −2.7438 | −0.37% | ||||
1s23s5p1Po | −2.7006 | −2.7104 | −0.36% | ||||
1s23d4d1Se | −2.6983 | −2.7130 | −0.54% | ||||
1s23s5d1De | −2.6548 | −2.6922 | −1.39% | ||||
1s23p5p1Pe | −2.5992 | −2.6041 | −0.19% | ||||
1s23p5d1Po | −2.5849 | −2.5716 | 0.52% | ||||
1s23p5d1Do | −2.5842 | −2.5807 | 0.13% | ||||
1s23p5p1Se | −2.5611 | −2.5712 | −0.39% | ||||
1s23p5d1Fo | −2.5505 | −2.5810 | −1.18% | ||||
1s23p5d1Do | −2.5430 | −2.5488 | −0.23% | ||||
1s23p5f1De | −2.5366 | −2.5412 | −0.18% | ||||
Note. The values of the energies are given relative to O5+ first ionization threshold ( 5.0758 au), which sets the zero of our scale. The percentage of deviation between the present energies and experimental ones is defined as Δ = ((EGTO − ENIST/ref)/ENIST/ref) × 100%.
Download table as: ASCIITypeset image
The TDSE, Equation (4), is solved by expanding the wave function onto a basis set composed of states of the isolated collision partners (i.e., asymptotic states):
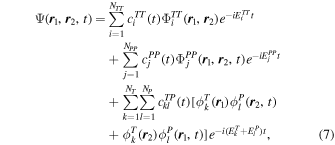
where the superscripts T and TT (P and PP) denote states, including pseudostates, and corresponding energies for which respectively one and two electrons are on the target (projectile). For both electrons, the projectile states contain plane-wave electron translation factors (ETFs), , ensuring Galilean invariance of the results. Note that for the collision system under consideration, the initial state is singlet so that only singlet one- and two-center states are considered in the expansion Equation (7). The insertion of Equation (7) into Equation (4) results in a system of first-order coupled differential equations, which can be written in matrix form as

where c(t) is the column vector of the time-dependent expansion coefficients, i.e., cTT , cPP , and cTP in Equation (7) and S and M are the overlap and coupling matrices, respectively. These equations are solved using the predictor-corrector variable time-step Adams–Bashford–Moulton method for a set of initial conditions: initial electronic state i, velocity v, impact parameter b, and molecular target orientation defined by (Θm , Φm ) (see Figure 1).
The probability of a transition i → f is given by the coefficient cf (≡cTT , cPP or cTP ) as

The corresponding cross sections for a given molecular geometry can be calculated from these probabilities as follows:

where the dependence upon Φm disappears after integration over b . The total cross sections (averaged over the degrees of freedom of the molecule) are then given by

where ρ(Θm ) and ρ(RAB ) are respectively the distribution of the molecular geometry over Θm and RAB . The former is assumed to be isotropic, i.e.,

The distribution ρ(RAB ) over the internuclear distance of the target is generally that corresponding to a given (e.g., ground) vibrational state or a superposition of vibrational states of the molecular target (Caillat et al. 2004). The cross sections should then be evaluated for different values of RAB and generally averaged over the initial vibrational state distribution. However, it has been shown that considering only the equilibrium distance value Req is sufficient to obtain accurate cross sections (Caillat et al. 2004, 2006; Sisourat et al. 2011; Rabadán et al. 2017; Zhang et al. 2020). In the following we therefore consider only a molecular target frozen at the equilibrium distance, the so-called Franck–Condon approximation (Errea et al. 1997). Thus, the ρ(RAB ) can be expressed as

with Req = 1.4 au for H2.
The total cross sections (Equation (11)) can be rewritten as

In order to reduce the computational costs, it is often chosen to evaluate the cross sections through an approximate averaging procedure using only three characteristic molecular orientations, namely (Θm , Φm ) = (0, 0), (π/2, 0), and (π/2, π/2):
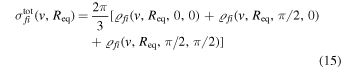
and

The approximation has been widely used and proven to give good estimates of the cross sections (see, e.g., Lühr & Saenz 2010; Sisourat et al. 2011; Zhang et al. 2020).
In the present calculations, the one- and two-electron states and pseudostates included in the expansion Equation (7) are expressed in terms of Gaussian-type orbitals (GTOs) and products of them. For the present collision system, a set of 70 GTOs (12 for l = 0; 8 × 3 for l = 1; 4 × 5 for l = 2; and 2 × 7 for l = 3) are used on the O6+ center, and 7 GTOs for l = 0 taken from Sisourat et al. (2011) are used for each center of H2. In the wave-function expansion, we have included 2479 singlet states and pseudostates in total: 38 TT (H2); 575 TP (H2 +, O5+); and 1866 PP (O4+) ones. In Table 1, we show the energies of the relevant O5+ and O4+ states compared with the corresponding available data from NIST (Kramida et al. 2021) and Bachau et al. (1990). The overall agreement between our calculated energies and available data is excellent, and at worst the deviation equals to −1.46% for the doubly excited autoionizing states (O4+(1s23d4d1Ge)).
The cross sections reported in the following have been compared with those from a smaller basis set on the O6+ center built from 53 GTOs (10 for l = 0; 7 × 3 for l = 1; 3 × 5 for l = 2; and 1 × 7 for l = 3). The total direct SEC (processes in Equation (1a)) cross sections from these two sets agree with each other within 10%. The cross sections for the dominant channels (SEC into O5+(1s23ℓ and 1s24ℓ)) from these two sets agree with each other within 30% at lower- and higher-impact energies, while they agree within about 20% in the intermediate-energy region. For the ADC processes, the cross sections differ by less than 3% at low energies, by less than 9% at intermediate energies, and by less than 20% at high-impact energies. For the DEC processes, the cross sections are more than 3 orders of magnitude smaller than the ADC ones. The convergence was found to be better than 50% at the lowest energy (0.1 keV u−1), to be about 30% at intermediate energies, and within 12% at high-impact energies.
3. Results and Discussions
3.1. Total SEC and DEC Cross Sections
In Figure 2, our total SEC cross sections for O6+ ion in collisions with H2 molecule are presented as a function of impact energy, together with available experimental data(Crandall et al. 1977, 1979; Phaneuf et al. 1982; Dijkkamp et al. 1985; Machacek et al. 2014; Han et al. 2021). The present results are also listed in Table 2. It is shown that the present results of direct SEC, i.e., including only the three processes listed in Equation (1a), decrease monotonically for increasing impact energies. Compared with the available experimental data, our results lie systematically lower than the experimental ones in the whole overlapping energy region. Before commenting on the discrepancy, we will compare our results with the existing data for two-electron processes. We display in Figure 3 the present DEC and ADC cross sections, compared with available experimental data for DEC (Crandall et al. 1977; Machacek et al. 2014; Han et al. 2021). The experimental results do not agree with each other, showing disagreement of a factor of 3 around 3 keV u−1. Our cross sections for the DEC process are more than 1 order of magnitude smaller than the experimental ones, while the present ADC results are about a factor of 3 larger than the experimental data.
Figure 2. Total SEC cross sections as a function of the impact energy. The present calculations for direct SEC to O5+ are denoted by the black solid line; the total SEC cross sections (direct SEC + 70%ADC) are denoted by the red dashed line. The experimental results denoted by symbols are from Crandall et al. (1977, 1979), Phaneuf et al. (1982), Dijkkamp et al. (1985), Machacek et al. (2014), and Han et al. (2021).
Download figure:
Standard image High-resolution imageFigure 3. Total DEC cross sections as a function of the impact energy. The present calculations for DEC into bound states are plotted as the black solid line; the total ADC cross sections are denoted by the red dashed line; and the total DEC cross sections (DEC into bound states +30%ADC) are depicted with the black dashed–dotted line. The experimental results denoted by symbols are from Crandall et al. (1977), Machacek et al. (2014), and Han et al. (2021).
Download figure:
Standard image High-resolution imageTable 2. Present Total and n ℓ-resolved SEC, DEC, and ADC Cross Sections (in Units of 10−16 cm2) for O6+ + H2 Collisions
Direct SEC | ||||||||
---|---|---|---|---|---|---|---|---|
E (keV u−1) | O5+(1s23s) | O5+(1s23p) | O5+(1s23d) | O5+(1s24s) | O5+(1s24p) | O5+(1s24d) | O5+(1s24f) | Total SEC |
0.10 | 0.0160 | 0.0526 | 0.1535 | 9.6127 | 24.3754 | 3.4349 | 2.5248 | 43.3512 |
0.20 | 0.0344 | 0.0898 | 0.2339 | 14.6673 | 13.2150 | 3.7049 | 4.6816 | 39.4840 |
0.40 | 0.0799 | 0.1175 | 0.1839 | 17.8953 | 9.6162 | 2.3984 | 5.8566 | 38.2961 |
0.60 | 0.0892 | 0.1449 | 0.1692 | 18.1693 | 8.9535 | 2.3636 | 5.9265 | 38.0161 |
1.00 | 0.1590 | 0.2082 | 0.2046 | 16.9163 | 7.7414 | 2.7683 | 6.6700 | 36.8370 |
1.56 | 0.1926 | 0.3080 | 0.2473 | 14.8537 | 7.1690 | 3.2604 | 7.5361 | 35.9066 |
2.63 | 0.2227 | 0.4738 | 0.3343 | 10.8433 | 6.6308 | 4.4566 | 8.4080 | 33.6321 |
4.00 | 0.2352 | 0.6716 | 0.3868 | 7.7306 | 6.2688 | 5.7376 | 9.0684 | 32.2791 |
6.00 | 0.2831 | 0.6122 | 0.5035 | 4.9464 | 5.2889 | 6.3282 | 10.2174 | 30.8031 |
9.00 | 0.2119 | 0.5480 | 0.5890 | 2.6611 | 3.7867 | 6.7511 | 11.4367 | 29.4548 |
12.00 | 0.1596 | 0.5040 | 0.5662 | 1.5721 | 2.7879 | 6.4309 | 11.8308 | 27.4867 |
15.00 | 0.1775 | 0.4648 | 0.5804 | 0.9777 | 2.1032 | 5.8730 | 11.6577 | 25.4244 |
19.50 | 0.2073 | 0.4724 | 0.6073 | 0.5480 | 1.4943 | 5.0388 | 10.7792 | 22.5032 |
25.00 | 0.2268 | 0.4975 | 0.6048 | 0.3098 | 1.0122 | 4.0400 | 9.3645 | 19.2025 |
50.00 | 0.1765 | 0.4609 | 0.5201 | 0.0894 | 0.2574 | 1.1261 | 4.6686 | 10.6768 |
72.25 | 0.0984 | 0.3358 | 0.5164 | 0.0436 | 0.1564 | 0.3406 | 2.4628 | 7.3190 |
100.00 | 0.0380 | 0.2030 | 0.4483 | 0.0165 | 0.1101 | 0.1414 | 1.2382 | 5.1876 |
DEC into Bound States | ADC | |||||||
E (keV u−1) | O4+(![]() | O4+(![]() | O4+(![]() | Total DEC | O4+(![]() | O4+(![]() | O4+(![]() | Total ADC |
0.10 | 0.0046 | 0.0114 | 0.0180 | 0.0351 | 4.7245 | 13.7343 | 1.0683 | 20.4485 |
0.20 | 0.0036 | 0.0099 | 0.0147 | 0.0297 | 5.8560 | 10.2587 | 0.9634 | 18.0987 |
0.40 | 0.0027 | 0.0086 | 0.0195 | 0.0313 | 6.4443 | 8.2578 | 0.9155 | 16.6945 |
0.60 | 0.0039 | 0.0081 | 0.0140 | 0.0274 | 6.7941 | 7.2339 | 0.7960 | 16.0875 |
1.00 | 0.0025 | 0.0080 | 0.0138 | 0.0254 | 6.5141 | 6.4484 | 0.9172 | 15.2058 |
1.56 | 0.0033 | 0.0100 | 0.0158 | 0.0301 | 6.2405 | 5.5603 | 0.8071 | 14.0299 |
2.63 | 0.0015 | 0.0077 | 0.0155 | 0.0250 | 5.8572 | 4.7677 | 0.8096 | 13.0394 |
4.00 | 0.0025 | 0.0110 | 0.0223 | 0.0360 | 5.0143 | 4.3449 | 0.8938 | 11.9547 |
6.00 | 0.0041 | 0.0147 | 0.0191 | 0.0379 | 3.9045 | 4.3832 | 1.0903 | 11.1342 |
9.00 | 0.0064 | 0.0166 | 0.0182 | 0.0412 | 2.9597 | 4.1733 | 1.3973 | 10.4397 |
12.00 | 0.0097 | 0.0207 | 0.0167 | 0.0472 | 2.4884 | 4.0649 | 1.7946 | 10.4928 |
15.00 | 0.0122 | 0.0290 | 0.0183 | 0.0595 | 2.1830 | 3.7056 | 2.0870 | 10.3975 |
19.50 | 0.0169 | 0.0398 | 0.0210 | 0.0778 | 2.1119 | 3.1998 | 2.2909 | 10.3152 |
25.00 | 0.0254 | 0.0444 | 0.0229 | 0.0930 | 2.2129 | 2.7897 | 2.2939 | 10.3466 |
50.00 | 0.0583 | 0.0481 | 0.0242 | 0.1324 | 1.8445 | 1.6305 | 0.9618 | 8.5183 |
72.25 | 0.0534 | 0.0431 | 0.0247 | 0.1249 | 0.8840 | 0.8684 | 0.3275 | 5.8566 |
100.00 | 0.0359 | 0.0327 | 0.0184 | 0.0925 | 0.3336 | 0.3450 | 0.1088 | 3.2052 |
Download table as: ASCIITypeset image
It should be noted that the doubly excited states populated by the ADC process were widely believed to completely autoionize (Roncin et al. 1993; Ali et al. 2016), in which only one electron remains on the projectile, thus contributing to the measured SEC cross sections (Machacek et al. 2014; Han et al. 2021). However, a number of experiments for multiply charged ions colliding with the neutral target have reported the stabilization of the doubly excited states populated by ADC, that both electrons could remain on the projectile with a rather high probability (Roncin et al. 1991; Gaboriaud et al. 1993; Martin et al. 1994, 1995; Chen et al. 1996; Hasan et al. 1999; Ali et al. 2016). Possible stabilization mechanisms were also proposed, including the two-step uncorrelated DEC followed by postcollisional autotransfer to Rydberg states (ATR; Bachau et al. 1992; Roncin et al. 1993), feeding doubly excited states with () from symmetric (
) or quasisymmetric (
) ones; the two-step correlated transfer excitation(Stolterfoht et al. 1986; Winter et al. 1987); the one-step correlated double-electron transfer (Chen & Lin 1993); and the two-step transfer excitation involving nucleus–electron interaction(Flechard et al. 1997). Therefore, the ADC processes listed in Equation (3) could also contribute to the measured DEC cross sections.
In Figure 2, we also present the results for the sum of the direct SEC cross sections and 70% of the ADC ones (dashed red line). Our results agree well with available experimental data in the overlapping energy region though they lie slightly lower than the one from Han et al. (2021) for energies above 6 keV u−1. On the other hand, it can be observed from Figure 3 that our results for the sum of DEC cross sections and 30% of the ADC ones (dashed–dotted black line) are in excellent agreement with the most recent measured DEC cross sections. The above comparison suggests that about 70% of the ADC may contribute to the measured SEC cross sections through postcollisional autoionization, while the stabilization of 30% of doubly excited states populated by ADC contributes to the measured DEC cross sections. The latter stabilization is mainly by way of the ATR mechanism since the symmetric () and quasisymmetric (
) doubly excited states are dominantly populated in ADC cross sections (see Figure 6(b)). It should be noted that, although our cross sections were obtained in the ab initio calculations, the contributions of 70% and 30% of ADC in the measured SEC and DEC cross sections were estimated from comparison with experimental measurements (Han et al. 2021).
3.2. nℓ-resolved SEC Cross Section
We now investigate n ℓ-resolved SEC cross sections. Such detailed information on the final-state distribution of captured electrons is of particular interest both in astrophysics and in plasma diagnostics research since it determines the characteristics of the emitted radiation.
Our calculated n ℓ-resolved SEC cross sections for the production of O5+(1s24ℓ) are presented in Figure 4, together with the available experimental results from Dijkkamp et al. (1985) and Lubinski et al. (2000). The present results are also displayed in Table 2. Our results show that electron capture to O5+(1s24f) is dominant above 3.5 keV u−1. Below this energy, electron capture to O5+(1s24s) takes over until about 0.2 keV u−1, at which O5+(1s24p) starts to dominate. Comparing our results with the experimental data, overall good agreements can be obtained, except for SEC into O5+(1s24d) below 0.2 keV u−1 and SEC into O5+(1s24p) at 7 keV u−1, where our results lie slightly higher than experimental data. Furthermore, we extend the cross sections to impact energies higher than 8 keV u−1, for which no data exists.
Figure 4. The 4ℓ-resolved SEC cross sections as a function of impact energy. The present results are shown by lines; the experimental data are from Dijkkamp et al. (1985; filled symbols) and Lubinski et al. (2000; open symbols).
Download figure:
Standard image High-resolution imageFigure 5 shows our calculated cross sections for SEC into O5+(1s23ℓ) in comparison with the only available experimental data from Dijkkamp et al. (1985) for the production of O5+(n = 3). The present results are also listed in Table 2. It is shown that SEC to O5+(1s23d) is the dominant capture channel for energies below 1 keV u−1 and above 10 keV u−1, while in between, the dominance of O5+(1s23p) channel is seen. As can be seen in Figure 5, our cross sections for the SEC into O5+(n = 3) are smaller than the only available experimental data by a factor of 2 or worse. We emphasize that the convergence of our calculations has been checked and is better than 20% for SEC into O5+(1s23ℓ). The validity of our results is also supported by the overall good agreements with available experimental data shown in Figure 4 for SEC into O5+(1s24ℓ) since the lower excited states are obviously better described than the higher excited states with our GTO basis set.
Figure 5. The 3ℓ-resolved SEC cross sections as a function of impact energy. The present results are shown by lines, and the only available experimental data for SEC into O5+(n = 3) from Dijkkamp et al. (1985) are denoted as solid red circles.
Download figure:
Standard image High-resolution imageNote finally that our calculations indicate that the SEC into O5+(1s23ℓ and 1s24ℓ) follows approximately the statistical ℓ distribution at high-impact energies, for which the electron is therefore mainly captured into subshells of the maximum ℓ.
3.3. Shell-resolved DEC and ADC Cross Sections
For completeness, Figures 6(a) and (b), as well as Table 2, show our calculated shell-resolved cross sections for DEC and ADC, respectively. The cross sections for DEC into bound states are more than 2 orders of magnitude smaller than the ADC ones. Our results show that DEC into higher excited states of O4+(
n ≥ 5) is preferred at low energies, while the productions of O4+(
) and O4+(
) become dominant for higher-impact energies. The present total DEC cross sections present a maximum around 60 keV u−1 and decrease about 3 times at low energies; this behavior is due to the increasing importance of the productions for symmetric O4+(
) and quasisymmetric O4+(
) doubly excited states in the two-electron capture processes, as shown in Figure 6(b).
Figure 6. Total and shell-resolved (a) DEC and (b) ADC cross sections as a function of the impact energy.
Download figure:
Standard image High-resolution imageFor the ADC process, it can be observed from Figure 6(b) that the productions for symmetric O4+() and quasisymmetric O4+(
) doubly excited states are of comparable importance and dominant in the whole considered energy region. Both of them display oscillatory energy-dependence structures but with opposite phases over the energy range 0.1–50 keV, which tends to indicate that they are strongly coupled. The cross sections for ADC to O4+(
) are about 1 order of magnitude smaller than the ones for ADC to O4+(
) and O4+(
) at lower energies, while they get of comparable importance for energies higher than 15 keV u−1. However, without any other data, it is clear that further experimental and theoretical efforts are required to draw definite conclusions.
4. Conclusions
In this paper, we have investigated one- and two-electron processes occurring in collisions of O6+ ions and H2 molecules over a wide collision energy domain ranging from 0.1 to 100 keV u−1. We used a two-active-electron, three-center, SCASCC method with a large basis set to reach controlled reasonable convergence. To date, our close-coupling description of the collision is the most elaborate one in terms of accounting for electron correlation, molecular structures, and active channels. For total SEC and DEC cross sections, our results are, in general, in good agreement with the experimental ones. The comparison between the present calculations and available experimental data suggests that about 70% of the ADC may contribute to the measured SEC cross sections through postcollisional autoionization, while the stabilization of 30% of doubly excited states populated by ADC via the ATR mechanism contributes to the measured DEC ones. For the n ℓ-resolved SEC cross sections, our results for SEC into dominant channels, i.e., O5+(1s24ℓ), are in good agreement with available experimental data. It is shown that the SEC into O5+(1s23ℓ and 1s24ℓ) follows approximately the statistical ℓ distribution at high-impact energies, for which the electron is therefore mainly captured into subshells of the maximum ℓ. Furthermore, we also provide the first set of shell-resolved DEC and ADC cross sections.
Our work provides new data of total and n ℓ-resolved SEC, DEC, and ADC cross sections for O6+ + H2 collisions, which are essential to improve the understanding of this relevant collision system and will be helpful for modeling astrophysical X-ray emissions induced by charge exchange.
This work is supported by the National Natural Science Foundation of China (grant No. 12004350), the startup project from Hangzhou Normal University, and the Key Laboratory of Computational Physics of Institute of Applied Physics and Computational Mathematics (grant No. 6142A05QN21001).