Advances in Skin Tissue Bioengineering and the Challenges of Clinical Translation
- 1Skin Engineering Laboratory, Adult Burns Centre, Royal Adelaide Hospital, Adelaide, SA, Australia
- 2Adult Burns Centre, Royal Adelaide Hospital, Adelaide, SA, Australia
- 3Faculty of Health and Medical Science, The University of Adelaide, Adelaide, SA, Australia
- 4Department of Surgery, University of Cincinnati, Cincinnati, OH, United States
Skin tissue bioengineering is an emerging field that brings together interdisciplinary teams to promote successful translation to clinical care. Extensive deep tissue injuries, such as large burns and other major skin loss conditions, are medical indications where bioengineered skin substitutes (that restore both dermal and epidermal tissues) are being studied as alternatives. These may not only reduce mortality but also lessen morbidity to improve quality of life and functional outcome compared with the current standards of care. A common objective of dermal-epidermal therapies is to reduce the time required to accomplish stable closure of wounds with minimal scar in patients with insufficient donor sites for autologous split-thickness skin grafts. However, no commercially-available product has yet fully satisfied this objective. Tissue engineered skin may include cells, biopolymer scaffolds and drugs, and requires regulatory review to demonstrate safety and efficacy. They must be scalable for manufacturing and distribution. The advancement of technology and the introduction of bioreactors and bio-printing for skin tissue engineering may facilitate clinical products' availability. This mini-review elucidates the reasons for the few available commercial skin substitutes. In addition, it provides insights into the challenges faced by surgeons and scientists to develop new therapies and deliver the results of translational research to improve patient care.
Introduction
The challenges of translational medicine are becoming more prevalent with developing new technologies as novel therapies for personalised medicine. One therapy where translational research is at the forefront is reducing the use of skin autografts for extensive full-thickness burns with laboratory-generated skin (1–7). The split-thickness meshed and expanded skin autograft has been the prevailing standard of care for burns surgeons for decades and remains the preferred method of wound closure due to its relatively high efficacy of stable wound closure (3, 8, 9). However, if the burn area massively exceeds the area of available donor site for skin autografts, the advantages of autologous engineered skin substitutes is compelling. To regenerate a substitute of uninjured human skin that definitively provides wound closure both anatomically and physiologically (6) is a common challenge for tissue engineers, and may involve polymer chemists, cellular and molecular biologists, surgeons, nurses, and therapists. A systematic review of clinical studies investigating autologous bilayered skin substitutes as epithelial stem cell niches after grafting, identified 16 potential studies and nine types of autologous skin substitutes over a 25-year period (10). Currently, only a small number of these are still available for therapeutic use, with no ideal substitute in the market. The current models have distinct attributes, for the majority, the scaffold type is a source or derivation of collagen (biologic) with autologous fibroblasts and keratinocytes. Another novel synthetic scaffold utilising a polyurethane (PUR) has also been used to generate a skin composite (composite cultured skin, CCS) and has reported its use for the treatment of a 95% total body surface area burn patient (11). However, these all remain deficient in pigmentation, hair, and other dermal appendages. The authors draw on combined experiences from taking the research bench to bedside. This review will describe the distinct models of bilayered tissue engineered products that have been used therapeutically, which there are few, but all address the same clinical challenges.
The Need for an Alternative to Skin Autografts for Extensive Full-Thickness Burns
Burns are a global health concern, especially for low to middle-income countries, accounting for over 95% of burn deaths (12). Burn injuries of all depths make up only a small proportion (1%) of trauma hospitalisations in Australia (13), but are one of the most costly, due to long hospital and rehabilitation stays (14, 15). In the United States, hospitalised burns cost over $1billion per year (16) and in high income countries the mean cost per 1% TBSA is US$4159.00 (17). These costs are significant, but the major indirect cost is the patient's lifelong scars and disfigurements. As the percentage of total body surface area (TBSA) burn and burn depth increases, the costs increase exponentially (14). Extensive, full-thickness burn injuries (>50% TBSA) usually require intensive care, multiple surgical procedures, physical and occupational therapy and psycho-social interventions to recover. Patients with these degrees of burn often die which obviates skin graft paucity (18). However, advances in burn care have led to increased survival rates due to early excision of eschar, temporary wound closure, advanced nutritional support, infection prevention, and improvements in critical care medicine (19–22). Although, burns in the elderly and those with coincident trauma such as inhalation injury, remain challenging.
In this patient population, temporary wound coverage provides time for utilisation of donor sites from superficially burned skin and re-harvesting to allow multiple procedures of skin autografting (23). Maximising wound coverage with available donor site involves thin, widely-meshed, or expanded (Meek-Wall technique) (24) skin autografts, resulting in poor functional and aesthetic outcomes. In addition, donor sites generated by split-thickness skin graft harvesting are extremely painful, may require opiate analgesia, limit mobilisation, and discourage compliance with physical therapy (25). Skin autografts, however, have properties that promote their continued widespread use for the closure of large, deep skin wounds (no rejection, vascular inosculation, high efficacy, long-term stability), but their correlation with increased morbidity, especially in the elderly, is a significant disadvantage, which motivates the search for alternatives (26, 27).
An ideal skin substitute should adhere, vascularise, and integrate quickly, contain both epidermal and dermal components, provide permanent and definitive wound closure, be autologous, resist infection, be easy to prepare, handle well, easy to apply, cost-effective, and resist mechanical shear forces (28). They should demonstrate high engraftment rates, restore natural pigmentation, and provide all skin appendages and sensory networks in uninjured skin. This list of qualities is comprehensive, and to simultaneously replicate these features in vivo requires complex engineering in the laboratory. Engineered skin fabrication is a specialised professional field with many aspects still to be elucidated and reduced to practise. A standardised universal classification system for “skin substitutes” was published by Davison-Kotler et al. in 2018 to encapsulate all adaptations (research and clinical) using a factorial design (29). Primary categories include acellular dermal substitutes, temporary skin substitutes, and permanent skin substitutes, further expanding into sub-categories (2). The many variations have been tabulated in former reviews and will not be detailed here (6, 7, 29–38). This review focuses on permanent, cellular, and mainly autologous products with dermal and epidermal components. It will explore a few commercially available products and some clinically used in extensive wounds (Table 1).
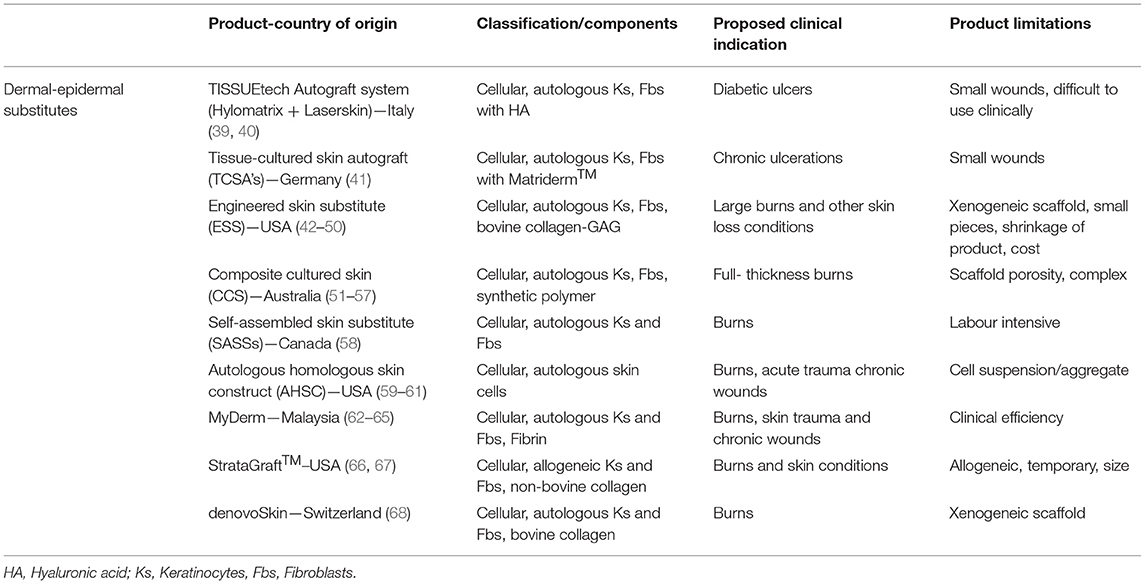
Table 1. Examples of clinically-available or investigative skin substitutes [adapted from Vig et al. (7), Boyce et al. (31)].
Large, Excised Wounds—Temporising the Wound Bed for Definitive Closure
The loss of the epidermis, and sufficient dermis to ensure loss of all the epidermal adnexa, requires rapid wound closure. The primary focus is on reducing inflammation and granulation, preventing infection and limiting contraction. The associated mortality and morbidity rates decrease with the successful implementation of the above and achieved by staged closure (69). Acellular dermal substitutes comprising a dermal and a pseudo-epidermal component have been widely used to achieve physiological closure. Their implementation has produced a paradigm shift in burn care (21, 70, 71). The dermal components may originate from decellularised human skin, biological polymers, or synthetic polymers. Their function is to temporarily close the excised wound to decrease fluid loss, allow integration and controlled granulation tissue invasion inducing a vascularised wound bed. Commercial examples include Integra® Dermal Regeneration Template, Pelnac, Terudermis, Hyalomatrix, and RenoSkin (69). These products and similar ones have limitations, including a risk of transmissible disease, loss from infection and high costs (5, 72). Despite regulatory approval for specific medical indications, most dermal substitutes have not achieved worldwide consensus as market leaders for large, deep dermal wounds. However, establishing a neo-dermis enhances structural stability and provides the time required for definitive epithelial wound closure, whether by serial grafting or by generating and applying autologous engineered skin.
The NovoSorb™ Biodegradable Temporising Matrix (BTM) is a synthetic scaffold that is currently in routine use for burns and complex wound repair (73–79). It is a scaffold that temporises the wound and biodegrades after integration and establishment of dermal elements (80). Furthermore, it resists infection, can be made in large sheets, is inexpensive to produce, easy to handle, and provides integration time (76–82). With the optimisation of a dermal replacement template and a major limitation addressed, i.e., acquisition of time for cellular growth, the prospective next step is the specification of a definitive wound closure alternative.
The Current State of Bioengineered Dermal-Epidermal Substitutes
Bioengineered skin substitutes involving dermal and epidermal components are the focus of this paper; however, epidermal replacements (cellular) require brief reference to appreciate the desirability of both components. A skin substitute is yet to be achieved that replaces the anatomy and physiology of uninjured skin or completely replaces all skin autograft properties- implying why an epidermal replacement alone will not replicate a meshed, or sheet, autograft. Cultured Epithelial Autografts (CEA's) have been used since 1986 (83), and other adaptations or iterations of keratinocyte suspensions [e.g., Epicel (84, 85), Cell Spray, RECELL® (86), BioSeed (87), Laserskin (39, 40)] have evolved. These are clinical adjuncts to therapies with traditional treatments of burn care to expedite reepithelialisation rate. Clinically applicable for small wounds (88), ulcers (87, 89–92), superficial burns (93) and skin graft donor sites; they have not been universally accepted by burns surgeons independently for deep large burns due to their limited expansion rate, mechanical fragility on handling, tendency to blister in vivo and vulnerability to shear after application (partly to deficiencies in basement membrane formation) (94). In addition, they are costly to produce, can take weeks to manufacture, and are epidermal derived replacements (95–100). Incorporating a substitute containing epidermal and dermal components is a logical progression toward regenerating a tissue more like uninjured skin (101).
A critical paracrine dialogue between fibroblasts and keratinocytes is essential for basement membrane synthesis, a beneficial feature for engineered skin substitutes (102–104). The basement membrane protects against shear by establishing a molecular bond that anchors the cellular epidermis to the extracellular matrix of the dermis. The most analogous to skin, and the most successful clinically to date, is an Engineered Skin Substitute (ESS) developed in Cincinnati, Ohio (105). Developed over the past 30 years, the ESS comprises autologous keratinocytes and fibroblasts in a bovine collagen-glycosaminoglycan (GAG) scaffold (42–50). The ESS model was the first to demonstrate stable closure of full-thickness burns by combination with Integra® Dermal Regeneration Template (106). In 2017, a report was published of ESS' clinical results in 16 subjects treated from 2007 to 2010. For patients with >50%, TBSA full-thickness burns, ESS's were able to reduce the need for harvesting donor skin grafts and reduce the mortality rate compared with data from similar patient populations reported in the National Burn Repository of the American Burn Association (107). The ESS results in a closed wound that has structural and functional similarities to native skin. However, this model also has limitations (lack of other cell types and adnexal structures, contraction of the collagen scaffold during ESS fabrication, relatively high cost and regulatory complexity); and is not commercially available. Pre-clinical studies have recently demonstrated the successful incorporation of melanocytes (108, 109), microvascular endothelial cells (110), and hair follicles (111) into the ESS model.
Bovine collagen is also used in denovoSkin™ (Cutiss AG, Zurich), which consists of a collagen hydrogel and human dermal fibroblasts and keratinocytes. It has been classified as an Advanced Therapy Medical Product (ATMP) and has received FDA and EMA Orphan status to treat burns in the US and EU (112, 113). It is currently undergoing clinical trial recruitment for adult and children burns, with an estimated completion date of 2023. However, the production of a dermal-epidermal equivalent with xenogeneic (non-human)-derived biologicals, such as bovine, rat, or porcine collagens or glycosaminoglycans (42) raises the potential for immune recognition and rejection and risk of prion transmission. A synthetic scaffold and autologous cell approach may reduce these risks.
Several matrices using fibroblasts alone to provide the biological extracellular matrix environment (114–117) have shown the generated skin's long-term stability in vitro (118). Through the Special Access Program in Canada, a Self-Assembled Skin Substitute (SASS) has shown clinical effectiveness, reporting a case series of 14 severely burned subjects (58). This substitute contains autologous fibroblasts and keratinocytes, forming a human biopolymer fibroblast scaffold with subsequent keratinocyte seeding. The constraining factor for this type of substitute, like some others, is the production time, with an average of 9 weeks from the initial biopsy (58). In addition, the SASSs post-transplantation displayed visible junctions between applications, re-iterating the need for a sizeable sheet that can be generated and transplanted with fewer anaesthetics.
Improved scalability has now been reported using a biodegradable polyurethane (PUR) as the scaffold for a dermal-epidermal alternative, known as a composite cultured skin (CCS) (51–57). The attributes for an “ideal engineered skin,” as mentioned previously (119), formulated the premise of combining an engineered-epidermis to a modified BTM dermal substitute. Compared to bovine collagen (a biologic), a synthetic biodegradable PUR showed lower toxicity and cytotoxicity, reduced immunogenic reaction, and minimal inflammatory response (51, 52, 120). The BTM-CCS provided a two-stage strategy, with the CCS as a definitive second stage wound closure material. The application of NovoSorb™ BTM, a temporising matrix, addresses one of the major limitations of available skin substitutes [i.e., time required for autologous cell expansion 3–5 weeks (38)]. The integration period enables the time required for cell isolation, expansion and bilayered construction (up to 7-weeks if needed) (11, 69). The CCS is a 1 mm thick PUR porous scaffold, populated with autologous fibroblasts in a fibrin network and layered with autologous keratinocytes (53, 54). Pre-clinical studies in a porcine model initially demonstrated the efficacy of small CCS, and later large pieces, generated in an automated bioreactor (54, 57). This custom-made novel bioreactor device has taken this from research to clinic (11, 57). The two-stage strategy of BTM-CCS has been used clinically in a 95% TBSA burn injury (covering 40% TBSA of original burn) (11). The patient not only survived but, at 1.5 years post-injury, required minimal contracture release in areas where autografts were applied and none to the CCS-applied areas. The result for CCS was a smooth, supple aesthetic appearance with varying pigmentation from primary epithelial engraftment. No delineation between junctions of CCSs can be observed. ROM and SOSS scores were comparable to sheet graft, but favourable over 1:3 meshed STSG and Meek.
The subcutaneous layer (the deepest layer of skin) is absent in many investigational and clinical substitutes. Polarity TE, a US company, produces an Autologous Homologous Skin Construct (AHSC). They claim that functional full-thickness skin can be regenerated by obtaining a full-thickness biopsy with immediate application (59). A retrospective, 15-patient post-AHSC application review case series was reported (60) for various wound types (burns, acute/traumatic injuries, and chronic wounds). It differs from the conventional dermal-epidermal substitute, in that it seems not to necessitate culture and is returned to the patient within days. These wounds were closed at 3 months post application; however, further studies are required to investigate and substantiate the claims of efficacy, especially in full-thickness, excised burns (61).
Several other dermal-epidermal constructs have been used clinically or gone to clinical trial pending commercialisation (Table 1). Some examples include, tissue cultured skin autograft (TCSAs using Matriderm™, Germany) (41), TISSUEtech Autograft system™ (using Hylomatrix, Anika Therapeutics Inc., Bedford) (121), and others using Allodermis (122, 123), Human plasma (124, 125), and Fibrin (MyDerm, Japan) (62–65). Another bi-layered product recently receiving (2021) FDA approval for adult deep partial-thickness burns is StrataGraft® (Mallinckrodt, USA) (66, 67). Although it is not autologous, this bilayered allogeneic product comprises murine collagen and allogeneic fibroblasts and keratinocytes, this acts indirectly on the autologous cells to assist with wound closure (66). This type of treatment is limited for deep full-thickness burns as it needs another source of autologous cells e.g., meshed graft or other skin appendages, to close the wound. However, it is readily available and “off-the-shelf” ready for immediate use, whereas typical bilayered autologous substitutes can take weeks to fabricate. As with any graft, there is potential for loss if there is no neovascularization. The majority of clinically available engineered skins are avascular; however, this is under investigation by researchers (126). The loss of graft can be due to an accumulation of blood (haematoma), fluid (seroma), contamination, or mechanical shear. The different skin models mentioned have varying pore sizes and can contribute to the success of the engraftment. The density of the dermal component (i.e., too small or large pores) can inhibit or promote vascularisation (57, 127). Shear of a substitute graft or blistering will also occur if there is loss or no basement membrane and reiterates the importance of cell-cell contact of the epidermal-dermal component in vitro culture. When this loss occurs, the wound heals by secondary reepithelialisation and healing is delayed. Although, a systematic review of bilayered skin substitutes showed wound healing rates for leg ulcers were comparable with the standard of care (RR 1.51, 95% 1.22–1.88) (128). A widely meshed STSG used for extensive wound coverage results in a weave-like pattern, producing a poor aesthetic result. In contrast, autologous engineered skin provides immediate coverage with a stratified epidermis that suppresses granulation tissue and arrests the scarring process. Producing a favourable smooth, pliable, even skin, with a reduction in pain and itch (55, 68, 107). Another major strength and benefit over skin autografts is the reduction of autologous donor skin and its associated morbidities. The diverse bilayered approaches mentioned all have their strengths and weaknesses, and in review, the ideal model may likely be combinations of biopolymer scaffolds and stem cells that can produce a functional, clinically safe and effective alternative (129, 130). Any of these tissue engineered products will face regulatory reviews and reimbursement requirements.
Clinical Challenges for Skin Substitutes
As cell-based therapeutic inventions, these products require approval by regulatory authorities to ensure high quality, safety and proven efficacy (131) (Therapeutic Goods Administration, TGA in Australia; Food and Drug Administration, FDA in the United States; European Medicines Agency (EMA), in the European Union, etc.). Several pre-clinical substitutes are being used through Special Access Programs (SAP) in designated countries. This scheme is a way of using non-licenced products to treat life-threatening injuries where other methods are not suitable, or non-existent. In the United States, the passage of the 21st Century Cures Act, in 2016 (31) and new agency programs will facilitate the clinical use of novel products and devices to treat patients at severe medical risk.
The generation of highly manipulated tissue-engineered products follows the standards for current Good Manufacturing Practices (cGMP) (132). They should ideally be free of any xenogeneic product (131) and include mandatory testing for microbiological assessment [sterility assurance level (SAL) of 10−6] and transportation validation to ensure that product integrity is maintained. Generating a clinically viable, and ethical, product suitable for market is a lengthy and labour intensive process, with high initial capital costs. These infrastructure costs, process complexity, and stringent quality control result in expensive products, making commercialisation less practical (133, 134) and are translational challenges a therapeutic product may encounter (Figure 1). The cost of such substitutes, however, should not be assessed directly by the cost per unit of production only (31, 135), but also indirectly by assessing overall hospital cost reductions concerning length of stay, the number of reconstructive surgeries post-major burn, patient outcome and aesthetics. Although, an experienced highly trained medical team, including specialised nurses and therapy protocols are required during the intense early stages of treatment until they become the prevailing standard of care.
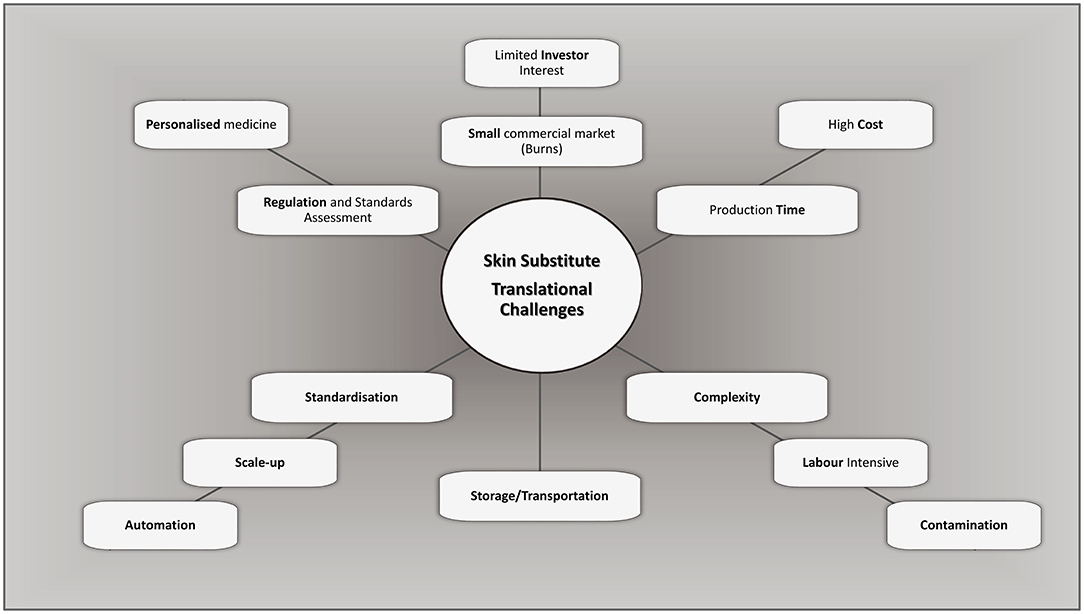
Figure 1. Challenges and considerations in bioengineering of bilayered skin substitutes. Adapted from Al-Himdani et al. (133).
The Future Opportunities of Skin Substitutes
The generation of laboratory-generated “skin substitutes,” irrespective of classification, have to date only partially addressed the requirements for achieving stable wound closure. They currently produce inadequate pigmentation (hypo- or hyperpigmentation), they lack vasculature, hair, glands, and none have replicated the results of unmeshed autograft or duplicated the anatomy and physiology of uninjured skin. Due to cost, regulatory restraints, and the significant scientific challenge to incorporate all skin features simultaneously (136–139). Approaches to the refinement of fabrication systems for skin substitutes will facilitate advanced models of engineered skin to reach their markets with a consequent decrease in costs. The requirement for scalability is a compelling demand for large burn injuries and can be met by incorporating automated bioreactors (57, 140). These may assist production and provide complete automation and standardisation to improve product quality. The robotic systems are engineering advances that will move forward in parallel with medical advances. The 3D and 4D bioprinting fields coupled with the latest compatible bioinks are novel techniques that may rapidly advance the tissue engineering field (141–145).
In time, these technologies and advances in tissue engineering will at least reduce, and possibly replace, the need for skin autografts and enable easier clinical translation of an acceptable autologous engineered skin, suitable for patient use. The significance of this is that patients with life-threatening burns will no longer suffer the painful acute morbidity and later scarring that donor sites generate. Time in ICU and total hospitalisation will be reduced, the need for reconstructive surgery will decrease, with overall costs reduced. The success will also have implications for other dermatologic conditions, including but not limited to giant congenital naevi excision and engraftment, epidermolysis bullosa treatment, certain surgical reconstructions, and vitiligo. It can also contribute to the investigation and requirement for epidermal appendages, naturally matched skin pigmentation, vascular plexus, and sensory nerves (2, 139, 146, 147). As each of these advances is currently under investigation, there can be high degrees of confidence that many, if not most of these skin components (uniform skin colour, sweat glands, and hair follicles) will be incorporated into future models of skin substitutes and available clinically for the treatment of full-thickness skin wounds, including burns.
Author Contributions
BD developed the outline with contributions from both JG and SB. BD wrote the manuscript with editing by JG and SB.
Funding
The CCS studies were supported by a grant from Lifetime Support Authority (LSA, GA-00061) and the Health Services Charitable Gifts Board (HSGB, 05-88). The NovoSorb® biodegradable polyurethane platform is produced by PolyNovo Biomaterials Pty. Ltd. based in Port Melbourne, Victoria, Australia.
Conflict of Interest
At the time of the CCS studies JG was a major shareholder in PolyNovo Biomaterials Pty Ltd., the company that produced the NovoSorb® Polyurethane material. JG and BD were stakeholders in Skin Tissue Engineering Pty Ltd. a company that had no protectable IP and was used as a vehicle for attracting and dispersing funding from non-grant awarding bodies.
The remaining author declares that the research was conducted in the absence of any commercial or financial relationships that could be construed as a potential conflict of interest.
Publisher's Note
All claims expressed in this article are solely those of the authors and do not necessarily represent those of their affiliated organizations, or those of the publisher, the editors and the reviewers. Any product that may be evaluated in this article, or claim that may be made by its manufacturer, is not guaranteed or endorsed by the publisher.
References
1. Supp DM, Boyce ST. Engineered skin substitutes: practices and potentials. Clin Dermatol. (2005) 23:403–12. doi: 10.1016/j.clindermatol.2004.07.023
2. Boyce S, Supp D. Chapter 11 - biologic skin substitutes. in Skin Tissue Engineering and Regenerative Medicine, eds M. Z. Albanna and J. H. Holmes Iv (New York, NY: Academic Press/Elsevier), (2016). p. 211–38. doi: 10.1016/B978-0-12-801654-1.00011-5
3. MacNeil S. Progress and opportunities for tissue-engineered skin. Nature. (2007) 445:874–80. doi: 10.1038/nature05664
4. Chua AW, Khoo YC, Tan BK, Tan KC, Foo CL, Chong SJ. Skin tissue engineering advances in severe burns: review and therapeutic applications. Burns Trauma. (2016) 4:3. doi: 10.1186/s41038-016-0027-y
5. Nicholas MN, Yeung J. Current status and future of skin substitutes for chronic wound healing. J Cutan Med Surg. (2017) 21:23–30. doi: 10.1177/1203475416664037
6. Shevchenko RV, James SL, James SE. A review of tissue-engineered skin bioconstructs available for skin reconstruction. J R Soc Interface. (2010) 7:229–58. doi: 10.1098/rsif.2009.0403
7. Vig K, Chaudhari A, Tripathi S, Dixit S, Sahu R, Pillai S, et al. Advances in skin regeneration using tissue engineering. Int J Mol Sci. (2017) 18:789-807. doi: 10.3390/ijms18040789
8. Ong YS, Samuel M, Song C. Meta-analysis of early excision of burns. Burns. (2006) 32:145–50. doi: 10.1016/j.burns.2005.09.005
9. Ben-Bassat H, Chaouat M, Segal N, Zumai E, Wexler MR, Eldad A. How long can cryopreserved skin be stored to maintain adequate graft performance? Burns. (2001) 27:425–31. doi: 10.1016/S0305-4179(00)00162-5
10. Cortez Ghio S, Larouche D, Doucet EJ, Germain L. The role of cultured autologous bilayered skin substitutes as epithelial stem cell niches after grafting: a systematic review of clinical studies. Burns Open. (2021) 5:56–66. doi: 10.1016/j.burnso.2021.02.002
11. Greenwood JE, Damkat-Thomas L, Schmitt B, Dearman B. Successful proof of the ‘two-stage strategy' for major burn wound repair. Burns Open. (2020) 4:121–31. doi: 10.1016/j.burnso.2020.06.003
12. World Health Organization. A WHO Plan for Burn Prevention and Care. World Health Organization (2008). Available online at: https://apps.WHO.Int/iris/handle/10665/97852
13. Pointer S, Tovell A. Hospitalised Burn Injuries, Australia, 2013–14 Injury Research and Statistics Series no 102 Cat no INJCAT 178. Canberra, ACT: AIHW (2016).
14. Ahn CS, Maitz PK. The true cost of burn. Burns. (2012) 38:967–74. doi: 10.1016/j.burns.2012.05.016
15. Cleland H, Greenwood JE, Wood FM, Read DJ, Wong She R, Maitz P, et al. The burns registry of Australia and New Zealand: progressing the evidence base for burn care. Med J Austral. (2016) 204:1951e. doi: 10.5694/mja15.00989
16. Finkelstein EA, Corso PS, Miller TR. The Incidence and Economic Burden of Injuries in the United States. New York, NY: Oxford University Press (2006).
17. Hop MJ, Polinder S, van der Vlies CH, Middelkoop E, van Baar ME. Costs of burn care: a systematic review: costs of burn care. Wound Repair Regener. (2014) 22:436–50. doi: 10.1111/wrr.12189
18. Chua A, Song C, Chai A, Kong S, Tan KC. Use of skin allograft and its donation rate in Singapore: an 11-year retrospective review for burns treatment. Transplant Proc. (2007) 39:1314–6. doi: 10.1016/j.transproceed.2006.11.028
19. Herndon DN, Barrow RE, Rutan RL, Rutan TC, Desai MH, Abston S. A comparison of conservative versus early excision. Therapies in severely burned patients. Annal Surg. (1989) 209:547. doi: 10.1097/00000658-198905000-00006
20. Rose J, Herndon D. Advances in the treatment of burn patients. Burns. (1997) 23:S19–26. doi: 10.1016/S0305-4179(97)90096-6
21. Price LA, Milner SM. The totality of burn care. Trauma. (2012) 15:16–28. doi: 10.1177/1460408612462311
22. Fratianne RB, Brandt CP. Improved survival of adults with extensive burns. J Burn Care Rehab. (1997) 18:347. doi: 10.1097/00004630-199707000-00013
23. Wurzer P, Keil H, Branski LK, Parvizi D, Clayton RP, Finnerty CC, et al. The use of skin substitutes and burn care-a survey. J Surg Res. (2016) 201:293–8. doi: 10.1016/j.jss.2015.10.048
24. Meek CP. Successful microdermagrafting using the meek-wall microdermatome. Am J Surg. (1958) 96:557–8. doi: 10.1016/0002-9610(58)90975-9
25. Huang G, Ji S, Luo P, Zhang Y, Wang G, Zhu S, et al. Evaluation of dermal substitute in a novel co-transplantation model with autologous epidermal sheet. PLoS ONE. (2012) 7:e49448. doi: 10.1371/journal.pone.0049448
26. Ashcroft GS, Mills SJ, Ashworth JJ. Ageing and wound healing. Biogerontology. (2002) 3:337. doi: 10.1023/A:1021399228395
27. Gore DC. Utility of acellular allograft dermis in the care of elderly burn patients. J Surg Res. (2005) 125:37–41. doi: 10.1016/j.jss.2004.11.032
28. Shores JT, Gabriel A, Gupta S. Skin substitutes and alternatives: a review. Adv Skin Wound Care. (2007) 20:493. doi: 10.1097/01.ASW.0000288217.83128.f3
29. Davison-Kotler E, Sharma V, Kang NV, García-Gareta E. A new and universal classification system of skin substitutes inspired by factorial design. Tissue Eng Part B Rev. (2018) 24:279–88. doi: 10.1089/ten.teb.2017.0477
30. Boyce ST, Lalley AL. Tissue engineering of skin and regenerative medicine for wound care. Burns Trauma. (2018) 6:4. doi: 10.1186/s41038-017-0103-y
31. Boyce S, Cheng P, Warner P. Chapter 2.5.8 - burn dressings and skin substitutes. In: Wagner W, Sakiyama-Elbert SE, Zhang G, Yaszemski MJ, editors. Biomaterials Science, An Introduction to Materials in Medicine. 4th ed. (San Diego: CA, Academic Press/Elsevier) (2020). p. 1169–80.
32. Sun BK, Siprashvili Z, Khavari PA. Advances in skin grafting and treatment of cutaneous wounds. Science. (2014) 346:941–5. doi: 10.1126/science.1253836
33. Metcalfe AD, Ferguson MW. Tissue engineering of replacement skin: the crossroads of biomaterials, wound healing, embryonic development, stem cells and regeneration. J R Soc Interface. (2007) 4:413–37. doi: 10.1098/rsif.2006.0179
34. Chocarro-Wrona C, Lopez-Ruiz E, Peran M, Galvez-Martin P, Marchal JA. Therapeutic strategies for skin regeneration based on biomedical substitutes. J Eur Acad Dermatol Venereol. (2019) 33:484–96. doi: 10.1111/jdv.15391
35. Zeng Q, Macri LK, Prasad A, Clark RAF, Zeugolis DI, Hanley C, et al. 5.534 - Skin Tissue Engineering, (Amsterdam, NL: Elsevier Ltd) (2011). p. 467–99. doi: 10.1016/B978-0-08-055294-1.00186-0
36. Zeng R, Lin C, Lin Z, Chen H, Lu W, Li H. Approaches to cutaneous wound healing: basics and future directions. Cell Tissue Res. (2018) 374:217–32. doi: 10.1007/s00441-018-2830-1
37. Moiemen NS, Lee KC. The role of alternative wound substitutes in major burn wounds and burn scar resurfacing. In: Herndon D, editor. Total Burn Care, 5th edn. Edinburgh, UK: Elsevier Ltd (2018). p. 633–9.e1.
38. Stojic M, López V, Montero A, Quílez C, de Aranda Izuzquiza G, Vojtova L, et al. Skin tissue engineering. In: Biomaterials for Skin Repair and Regeneration, ed. E. García-Gareta (Duxford, UK: Woodhead Publishing) (2019). p. 59–99.
39. Price RD, Das-Gupta V, Leigh IM, Navsaria HA. A comparison of tissue-engineered hyaluronic acid dermal matrices in a human wound model. Tissue Eng. (2006) 12:2985. doi: 10.1089/ten.2006.12.2985
40. Chan ES, Lam PK, Liew CT, Lau HC, Yen RS, King WW. A new technique to resurface wounds with composite biocompatible epidermal graft and artificial skin. J Trauma. (2001) 50:358–62. doi: 10.1097/00005373-200102000-00028
41. Golinski P, Menke H, Hofmann M, Valesky E, Butting M, Kippenberger S, et al. Development and characterization of an engraftable tissue-cultured skin autograft: alternative treatment for severe electrical injuries? Cells Tissues Organs. (2015) 200:227–39. doi: 10.1159/000433519
42. Boyce ST, Christianson DJ, Hansbrough JF. Structure of a collagen-GAG dermal skin substitute optimized for cultured human epidermal keratinocytes. J Biomed Mater Res. (1988) 22:939–57. doi: 10.1002/jbm.820221008
43. Powell HM, Boyce ST. EDC cross-linking improves skin substitute strength and stability. Biomaterials. (2006) 27:5821–7. doi: 10.1016/j.biomaterials.2006.07.030
44. Smiley AK, Klingenberg JM, Boyce ST, Supp DM. Keratin expression in cultured skin substitutes suggests that the hyperproliferative phenotype observed in vitro is normalized after grafting. Burns. (2006) 32:135–8. doi: 10.1016/j.burns.2005.08.017
45. Barai ND, Boyce ST, Hoath SB, Visscher MO, Kasting GB. Improved barrier function observed in cultured skin substitutes developed under anchored conditions. Skin Res Technol. (2008) 14:418–24. doi: 10.1111/j.1600-0846.2008.00225.x
46. Kalyanaraman B, Supp DM, Boyce ST. Medium flow rate regulates viability and barrier function of engineered skin substitutes in perfusion culture. Tissue Eng Part A. (2008) 14:583–93. doi: 10.1089/tea.2007.0237
47. Kalyanaraman B, Boyce ST. Wound healing on athymic mice with engineered skin substitutes fabricated with keratinocytes harvested from an automated bioreactor. J Surg Res. (2009) 152:296–302. doi: 10.1016/j.jss.2008.04.001
48. Klingenberg JM, McFarland KL, Friedman AJ, Boyce ST, Aronow BJ, Supp DM. Engineered human skin substitutes undergo large-scale genomic reprogramming and normal skin-like maturation after transplantation to athymic mice. J Invest Dermatol. (2010) 130:587–601. doi: 10.1038/jid.2009.295
49. Lander JM, Supp DM, He H, Martin LJ, Chen X, Weirauch MT, et al. Analysis of chromatin accessibility in human epidermis identifies putative barrier dysfunction-sensing enhancers. PLoS ONE. (2017) 12:e0184500. doi: 10.1371/journal.pone.0184500
50. Lloyd C, Besse J, Boyce S. Controlled-rate freezing to regulate the structure of collagen-glycosaminoglycan scaffolds in engineered skin substitutes. J Biomed Mater Res B Appl Biomater. (2015) 103:832–40. doi: 10.1002/jbm.b.33253
51. Greenwood JE, Li A, Dearman BL, Moore TG. Evaluation of novosorb novel biodegradable polymer for the generation of a dermal matrix part 2: in-vivo studies. Wound Prac Res. (2010) 18:24–34.
52. Greenwood JE, Li A, Dearman BL, Moore TG. Evaluation of novosorb novel biodegradable polymer for the generation of a dermal matrix part 1: in-vitro studies. Wound Prac Res. (2010) 18:14–22.
53. Dearman BL, Stefani K, Li A, Greenwood JE. “Take” of a polymer-based autologous cultured composite “skin” on an integrated temporizing dermal matrix: proof of concept. J Burn Care Res. (2013) 34:151–60. doi: 10.1097/BCR.0b013e31828089f9
54. Dearman BL, Li A, Greenwood JE. Optimization of a polyurethane dermal matrix and experience with a polymer-based cultured composite skin. J Burn Care Res. (2014) 35:437–48. doi: 10.1097/BCR.0000000000000061
55. Dearman B, Greenwood J. Treatment of a 95% total body surface burns patient with a novel polyurethane-based composite cultured skin. eCM Periodical. (2020) Collection 1(TERMIS EU Abstracts):187.
56. Dearman B, Greenwood J. A novel polyurethane-based composite cultured skin and a bespoke bioreactor in a porcine wound. eCM Periodical. (2020) Collection 1(TERMIS EU Abstracts):315.
57. Dearman BL, Greenwood JE. Scale-up of a composite cultured skin using a novel bioreactor device in a porcine wound model. J Burn Care Res. (2021). doi: 10.1093/jbcr/irab034
58. Germain L, Larouche D, Nedelec B, Perreault I, Duranceau L, Bortoluzzi P, et al. Autologous bilayered self-assembled skin substitutes (SASSs) as permanent grafts: a case series of 14 severely burned patients indicating clinical effectiveness. Eur Cell Mater. (2018) 36:128–41. doi: 10.22203/eCM.v036a10
59. Isbester K, Wee C, Boas S, Sopko N, Kumar A. Regeneration of functional, full-thickness skin with minimal donor site contribution using autologous homologous skin construct. Plastic Surg Case Stud. (2020) 6:2513826X1989881. doi: 10.1177/2513826X19898810
60. Mundinger GS, Armstrong DG, Smith DJ, Sailon AM, Chatterjee A, Tamagnini G, et al. Autologous homologous skin constructs allow safe closure of wounds: a retrospective, noncontrolled, multicentered case series. Plast Reconstr Surg Glob Open. (2020) 8:e2840. doi: 10.1097/GOX.0000000000002840
61. Granick MS, Baetz NW, Labroo P, Milner S, Li WW, Sopko NA. In vivo expansion and regeneration of full-thickness functional skin with an autologous homologous skin construct: clinical proof of concept for chronic wound healing. Int Wound J. (2019) 16:841–46. doi: 10.1111/iwj.13109
62. Mazlyzam AL, Aminuddin BS, Fuzina NH, Norhayati MM, Fauziah O, Isa MR, et al. Reconstruction of living bilayer human skin equivalent utilizing human fibrin as a scaffold. Burns. (2007) 33:355–63. doi: 10.1016/j.burns.2006.08.022
63. Seet WT, Manira M, Khairul Anuar K, Chua KH, Ahmad Irfan AW, Ng MH, et al. Shelf-life evaluation of bilayered human skin equivalent, Myderm. PLoS ONE. (2012) 7:e40978. doi: 10.1371/journal.pone.0040978
64. Bt Hj Idrus R, Abas A, Ab Rahim F, Saim AB. Clinical translation of cell therapy, tissue engineering, and regenerative medicine product in Malaysia and its regulatory policy. Tissue Eng Part A. (2015) 21:2812–6. doi: 10.1089/ten.tea.2014.0521
65. Mohamed Haflah NH, Ng MH, Mohd Yunus MH, Naicker AS, Htwe O, Abdul Razak KA, et al. Massive traumatic skin defect successfully treated with autologous, bilayered, tissue-engineered myderm skin substitute: a case report. JBJS Case Connect. (2018) 8:e38. doi: 10.2106/JBJS.CC.17.00250
66. Centanni JM, Straseski JA, Wicks A, Hank JA, Rasmussen CA, Lokuta MA, et al. Stratagraft skin substitute is well-tolerated and is not acutely immunogenic in patients with traumatic wounds: results from a prospective, randomized, controlled dose escalation trial. Ann Surg. (2011) 253:672–83. doi: 10.1097/SLA.0b013e318210f3bd
67. Sheryl R. Stratatech Corporation Biologics License Application (Approval Letter). (2021). Available online at: https://www.FDA.Gov/media/150131/download
68. Schiestl C, Meuli M, Vojvodic M, Pontiggia L, Neuhaus D, Brotschi B, et al. Expanding into the future: combining a novel dermal template with distinct variants of autologous cultured skin substitutes in massive burns. Burns Open. (2021) 5:145–53. doi: 10.1016/j.burnso.2021.06.002
69. Greenwood JE. Chapter 10 - hybrid biomaterials for skin tissue engineering. in Skin Tissue Engineering and Regenerative Medicine, eds M. Z. Albanna and J. H. Holmes Iv (Amsterdam, NL: Academic Press) (2016). p. 185–210.
70. Yannas IV, Burke JF, Huang C, Gordon PL. Correlation of in vivo collagen degradation rate with in vitro measurements. J Biomed Mater Res. (1975) 9:623–28. doi: 10.1002/jbm.820090608
71. Yannas IV, Burke JF. Design of an artificial skin. I. Basic design principles. J Biomed Mater Res. (1980) 14:65–81. doi: 10.1002/jbm.820140108
72. MacNeil S. Biomaterials for tissue engineering of skin. Mater Today. (2008) 11:26–35. doi: 10.1016/S1369-7021(08)70087-7
73. Wagstaff MJD, Schmitt BJ, Coghlan P, Finkemeyer JP, Caplash Y, Greenwood JE. A biodegradable polyurethane dermal matrix in reconstruction of free flap donor sites: a pilot study. Eplasty. (Springfield, IL: Open Science Co.) (2015) 15:e13.
74. Cheshire PA, Herson MR, Cleland H, Akbarzadeh S. Artificial dermal templates: a comparative study of novosorb biodegradable temporising matrix (btm) and integra((r)) dermal regeneration template (drt). Burns. (2016) 42:1088–96. doi: 10.1016/j.burns.2016.01.028
75. Lodescar RJ, Gibson CJ, Gallagher J. 722 an experience with a biodegradable temporizing matrix in a metropolitan burn center. J Burn Care Res. (2020) 41:S192. doi: 10.1093/jbcr/iraa024.306
76. Wagstaff MJ, Caplash Y, Greenwood JE. Reconstruction of an anterior cervical necrotizing fasciitis defect using a biodegradable polyurethane dermal substitute. Eplasty. (2017) 17:e3.
77. Greenwood JE, Schmitt BJ, Wagstaff MJ. Experience with a synthetic bilayer biodegradable temporising matrix in significant burn injury. Burns Open. (2018) 2:17–34. doi: 10.1016/j.burnso.2017.08.001
78. Damkat-Thomas L, Greenwood JE, Wagstaff MJD. A synthetic biodegradable temporising matrix in degloving lower extremity trauma reconstruction: a case report. Plast Reconstr Surg Glob Open. (2019) 7:e2110. doi: 10.1097/GOX.0000000000002110
79. Sreedharan S, Morrison E, Cleland H, Ricketts S, Bruscino-Raiola F. Biodegradable temporising matrix for necrotising soft tissue infections: a case report. Austral J Plastic Surg. (2019) 2:106–9. doi: 10.34239/ajops.v2i1.72
80. Greenwood JE, Dearman BL. Comparison of a sealed, polymer foam biodegradable temporizing matrix against integra(r) dermal regeneration template in a porcine wound model. J Burn Care Res. (2012) 33:163–73. doi: 10.1097/BCR.0b013e318233fac1
81. Greenwood JE, Wagstaff MJD, Rooke M, Caplash Y. Reconstruction of extensive calvarial exposure after major burn injury in 2 stages using a biodegradable polyurethane matrix. Eplasty. (2016) 16:e17.
82. Solanki NS, York B, Gao Y, Baker P, Wong She RB. A consecutive case series of defects reconstructed using novosorb® biodegradable temporising matrix: initial experience and early results. J Plastic Reconstruc Aesthetic Surg. (2020) 73:1845. doi: 10.1016/j.bjps.2020.05.067
83. Cuono C, Langdon R, McGuire J. Use of cultured epidermal autografts and dermal allografts as skin replacement after burn injury. Lancet. (1986) 1:1123. doi: 10.1016/S0140-6736(86)91838-6
84. Compton CC, Hickerson W, Nadire K, Press W. Acceleration of skin regeneration from cultured epithelial autografts by transplantation to homograft dermis. J Burn Care Rehabil. (1993) 14:653. doi: 10.1097/00004630-199311000-00010
85. Carsin H, Ainaud P, Le Bever H, Rives J-M, Lakhel A, Stephanazzi J, et al. Cultured epithelial autografts in extensive burn coverage of severely traumatized patients: a five year single-center experience with 30 patients. Burns. (2000) 26:379–87. doi: 10.1016/S0305-4179(99)00143-6
86. Holmes JH, Molnar JA, Shupp JW, Hickerson WL, King BT, Foster KN, et al. Demonstration of the safety and effectiveness of the recell® system combined with split-thickness meshed autografts for the reduction of donor skin to treat mixed-depth burn injuries. Burns. (2019) 45:772–82. doi: 10.1016/j.burns.2018.11.002
87. Vanscheidt W, Ukat A, Horak V, Bruning H, Hunyadi J, Pavlicek R, et al. Treatment of recalcitrant venous leg ulcers with autologous keratinocytes in fibrin sealant: a multinational randomized controlled clinical trial. Wound Repair Regen. (2007) 15:308–15. doi: 10.1111/j.1524-475X.2007.00231.x
88. Ortega-Zilic N, Hunziker T, Lauchli S, Mayer DO, Huber C, Baumann Conzett K, et al. Epidex® Swiss field trial 2004-2008. Dermatology. (2010) 221:365–72. doi: 10.1159/000321333
89. Tausche AK, Skaria M, Böhlen L, Liebold K, Hafner J, Friedlein H, et al. An autologous epidermal equivalent tissue-engineered from follicular outer root sheath keratinocytes is as effective as split-thickness skin autograft in recalcitrant vascular leg ulcers. Wound Repair Regener. (2003) 11:248–52. doi: 10.1046/j.1524-475X.2003.11403.x
90. Limat A, Mauri D, Hunziker T. Successful treatment of chronic leg ulcers with epidermal equivalents generated from cultured autologous outer root sheath cells. J Investigative Dermatol. (1996) 107:128–35. doi: 10.1111/1523-1747.ep12298415
91. Moustafa M. Randomized, controlled, single-blind study on use of autologous keratinocytes on a transfer dressing to treat nonhealing diabetic ulcers. Regen Med. (2007) 2:887–902. doi: 10.2217/17460751.2.6.887
92. Moustafa M, Simpson C, Glover M, Dawson RA, Tesfaye S, Creagh FM, et al. A new autologous keratinocyte dressing treatment for non-healing diabetic neuropathic foot ulcers. Diabet Med. (2004) 21:786–9. doi: 10.1111/j.1464-5491.2004.01166.x
93. Zhu N, Warner RM, Simpson C, Glover M, Hernon CA, Kelly J, et al. Treatment of burns and chronic wounds using a new cell transfer dressing for delivery of autologous keratinocytes. Euro J Plastic Surg. (2005) 28:319–30. doi: 10.1007/s00238-005-0777-4
94. Middelkoop E, Sheridan RL. 15 - skin substitutes and the next level'. in: Total Burn Care, ed. D. Herndon, 5th edn. Edinburgh: Elsevier Ltd (2018). p. 167–73.e2.
95. Blight A, Mountford EM, Cheshire IM, Clancy JMP, Levick PL. Treatment of full skin thickness burn injury using cultured epithelial grafts. Burns. (1991) 17:495–98. doi: 10.1016/0305-4179(91)90079-V
96. Donati L, Magliacani G, Bormioli M, Signorini M, Baruffaldi Preis FW. Clinical experiences with keratinocyte grafts. Burns. (1992) 18:S19–26. doi: 10.1016/0305-4179(92)90106-5
97. Munster AM. Cultured skin for massive burns: a prospective, controlled trial. Ann Surg. (1996) 224:372–7. doi: 10.1097/00000658-199609000-00013
98. Raghunath M, Meuli M. Cultured epithelial autografts: diving from surgery into matrix biology. Pediatric Surg Int. (1997) 12:478–83. doi: 10.1007/s003830050188
99. Paddle-Ledinek JE, Cruickshank DG, Masterton JP. Skin replacement by cultured keratinocyte grafts: an Australian experience. Burns. (1997) 23:204–11. doi: 10.1016/S0305-4179(96)00123-4
100. Atiyeh BS, Costagliola M. Cultured epithelial autograft (CEA) in burn treatment: three decades later. Burns. (2007) 33:405–13. doi: 10.1016/j.burns.2006.11.002
101. Greaves NS, Iqbal SA, Baguneid M, Bayat A. The role of skin substitutes in the management of chronic cutaneous wounds. Wound Repair Regen. (2013) 21:194–210. doi: 10.1111/wrr.12029
102. Boyce ST, Supp AP, Swope VB, Warden GD. Vitamin C regulates keratinocyte viability, epidermal barrier, and basement membrane in vitro, and reduces wound contraction after grafting of cultured skin substitutes. J Invest Dermatol. (2002) 118:565–72. doi: 10.1046/j.1523-1747.2002.01717.x
103. Boyce ST, Warden GD. Principles and practices for treatment of cutaneous wounds with cultured skin substitutes. Am J Surg. (2002) 183:445–56. doi: 10.1016/S0002-9610(02)00813-9
104. Harriger MD, Warden GD, Greenhalgh DG, Kagan RJ, Boyce ST. Pigmentation and microanatomy of skin regenerated from composite grafts of cultured cells and biopolymers applied to full-thickness burn wounds. Transplantation. (1995) 59:702–7. doi: 10.1097/00007890-199503150-00011
105. Boyce ST, Kagan RJ, Yakuboff KP, Meyer NA, Rieman MT, Greenhalgh DG, et al. Cultured skin substitutes reduce donor skin harvesting for closure of excised, full-thickness burns. Ann Surg. (2002) 235:269. doi: 10.1097/00000658-200202000-00016
106. Boyce ST, Kagan RJ, Meyer NA, Yakuboff KP, Warden GD. The 1999 clinical research award. Cultured skin substitutes combined with Integra artificial skin to replace native skin autograft and allograft for the closure of excised full–thickness burns. J Burn Care Rehabil. (1999) 20:453–61. doi: 10.1097/00004630-199920060-00006
107. Boyce ST, Simpson PS, Rieman MT, Warner PM, Yakuboff KP, Bailey JK, et al. Randomized, paired-site comparison of autologous engineered skin substitutes and split-thickness skin graft for closure of extensive, full-thickness burns. J Burn Care Res. (2017) 38:61–70. doi: 10.1097/BCR.0000000000000401
108. Boyce ST, Zimmerman RL, Supp DM. Tumorigenicity testing in athymic mice of cultured human melanocytes for transplantation in engineered skin substitutes. Cell Transplant. (2015) 24:1423–9. doi: 10.3727/096368914X683052
109. Boyce ST, Lloyd CM, Kleiner MC, Swope VB, Abdel-Malek Z, Supp DM. Restoration of cutaneous pigmentation by transplantation to mice of isogeneic human melanocytes in dermal-epidermal engineered skin substitutes. Pigment Cell Melanoma Res. (2017) 30:531–40. doi: 10.1111/pcmr.12609
110. Supp DM, Wilson-Landy K, Boyce ST. Human dermal microvascular endothelial cells form vascular analogs in cultured skin substitutes after grafting to athymic mice. FASEB J. (2002) 16:797–804. doi: 10.1096/fj.01-0868com
111. Sriwiriyanont P, Lynch KA, McFarland KL, Supp DM, Boyce ST. Characterization of hair follicle development in engineered skin substitutes. PLoS ONE. (2013) 8:e65664. doi: 10.1371/journal.pone.0065664
112. Oostendorp C, Meyer S, Sobrio M, van Arendonk J, Reichmann E, Daamen WF, et al. Evaluation of cultured human dermal- and dermo-epidermal substitutes focusing on extracellular matrix components: comparison of protein and rna analysis. Burns. (2017) 43:520–30. doi: 10.1016/j.burns.2016.10.002
113. Pontiggia L, Klar A, Bottcher-Haberzeth S, Biedermann T, Meuli M, Reichmann E. Optimizing in vitro culture conditions leads to a significantly shorter production time of human dermo-epidermal skin substitutes. Pediatr Surg Int. (2013) 29:249–56. doi: 10.1007/s00383-013-3268-x
114. Grinnell F, Fukamizu H, Pawelek P, Nakagawa S. Collagen processing, crosslinking, and fibril bundle assembly in matrix produced by fibroblasts in long-term cultures supplemented with ascorbic acid. Exp Cell Res. (1989) 181:483–91. doi: 10.1016/0014-4827(89)90105-5
115. Ishikawa O, Kondo A, Okada K, Miyachi Y, Furumura M. Morphological and biochemical analyses on fibroblasts and self-produced collagens in a novel three-dimensional culture. Br J Dermatol. (1997) 136:6–11. doi: 10.1046/j.1365-2133.1997.d01-1134.x
116. El Ghalbzouri A, Lamme E, Ponec M. Crucial role of fibroblasts in regulating epidermal morphogenesis. Cell Tissue Res. (2002) 310:189–99. doi: 10.1007/s00441-002-0621-0
117. Ahlfors JE, Billiar KL. Biomechanical and biochemical characteristics of a human fibroblast-produced and remodeled matrix. Biomaterials. (2007) 28:2183–91. doi: 10.1016/j.biomaterials.2006.12.030
118. El Ghalbzouri A, Commandeur S, Rietveld MH, Mulder AA, Willemze R. Replacement of animal-derived collagen matrix by human fibroblast-derived dermal matrix for human skin equivalent products. Biomaterials. (2009) 30:71–8. doi: 10.1016/j.biomaterials.2008.09.002
119. Kailani MH, Jafar H, Awidi A. Chapter 9 - synthetic biomaterials for skin tissue engineering. in Skin Tissue Engineering and Regenerative Medicine, eds M. Z. Albanna and J. H. Holmes Iv (Amsterdam, NL: Academic Press/Elsevier Inc) (2016). p. 163–83.
120. Li A, Dearman BL, Crompton KE, Moore TG, Greenwood JE. Evaluation of a novel biodegradable polymer for the generation of a dermal matrix. J Burn Care Res. (2009) 30:717. doi: 10.1097/BCR.0b013e3181abffca
121. Uccioli L, TissueTech Autograph System Italian Study G. A clinical investigation on the characteristics and outcomes of treating chronic lower extremity wounds using the tissuetech autograft system. Int J Low Extrem Wounds. (2003) 2:140–51. doi: 10.1177/1534734603258480
122. Sheridan RL, Morgan JR, Cusick JL, Petras LM, Lydon MM, Tompkins RG. Initial experience with a composite autologous skin substitute. Burns. (2001) 27:421–24. doi: 10.1016/S0305-4179(00)00156-X
123. Takami Y, Yamaguchi R, Ono S, Hyakusoku H. Clinical application and histological properties of autologous tissue-engineered skin equivalents using an acellular dermal matrix. J Nippon Med School. (2014) 81:356–63. doi: 10.1272/jnms.81.356
124. Gomez C, Galan JM, Torrero V, Ferreiro I, Perez D, Palao R, et al. Use of an autologous bioengineered composite skin in extensive burns: clinical and functional outcomes. A multicentric study. Burns. (2011) 37:580–9. doi: 10.1016/j.burns.2010.10.005
125. Llames S, Garcia E, Garcia V, del Rio M, Larcher F, Jorcano JL, et al. Clinical results of an autologous engineered skin. Cell Tissue Bank. (2006) 7:47–53. doi: 10.1007/s10561-004-7253-4
126. Baltazar T, Merola J, Catarino C, Xie CB, Kirkiles-Smith NC, Lee V, et al. Three dimensional bioprinting of a vascularized and perfusable skin graft using human keratinocytes, fibroblasts, pericytes, and endothelial cells. Tissue Eng Part A. (2020) 26:227–38. doi: 10.1089/ten.tea.2019.0201
127. Dehghani F, Annabi N. Engineering porous scaffolds using gas-based techniques. Curr Opin Biotechnol. (2011) 22:661–6. doi: 10.1016/j.copbio.2011.04.005
128. Jones JE, Nelson EA, Al-Hity A, Jones JE. Skin grafting for venous leg ulcers. Cochrane Libr. (2013) 2013:CD001737-CD37. doi: 10.1002/14651858.CD001737.pub4
129. Sheikholeslam M, Wright MEE, Jeschke MG, Amini-Nik S. Biomaterials for skin substitutes. Adv Healthc Mater. (2018) 7:1700897. doi: 10.1002/adhm.201700897
130. Augustine R, Kalarikkal N, Thomas S. Advancement of wound care from grafts to bioengineered smart skin substitutes. Progress Biomater. (2014) 3:103–13. doi: 10.1007/s40204-014-0030-y
131. Zheng MH, Pembrey R, Niutta S, Stewart-Richardson P, Farrugia A. Challenges in the evaluation of safety and efficacy of human tissue and cell based products. ANZ J Surg. (2006) 76:843–9. doi: 10.1111/j.1445-2197.2006.03880.x
132. Rajab T, Rivard AL, Wasiluk KR, Gallegos RP, Bianco RW. Chapter III.2.7 - Ethical issues in biomaterials and medical devices. in Biomaterials Science, eds B. D. Ratner, A. S. Hoffman, F. J. Schoen and J. E. Lemons 3rd ed. (Amsterdam: NL, Academic Press/Elsevier) (2013). p. 1425–31.
133. Al-Himdani S, Jessop ZM, Al-Sabah A, Combellack E, Ibrahim A, Doak SH, et al. Tissue-engineered solutions in plastic and reconstructive surgery: principles and practice. Front Surg. (2017) 4:4. doi: 10.3389/fsurg.2017.00004
134. Tolkoff J, Anders R. Chapter III.2.2 - commercialization: what it takes to get a product to market. In: Biomaterials Science. eds B. D. Ratner, A. S. Hoffman, F. J. Schoen and J. E. Lemons. 3rd edn. (Amsterdam NL: Academic Press/Elsevier) (2013). p. 1389–99.
135. Dhasmana A SS, Kadian S, Singh L. Skin tissue engineering: principles and advances. J Dermatol Skin. (2018) 1:101.
136. Hendrickx B, Vranckx JJ, Luttun A. Cell-based vascularization strategies for skin tissue engineering. Tissue Eng Part B Rev. (2011) 17:13–24. doi: 10.1089/ten.teb.2010.0315
137. Huang S, Xu Y, Wu C, Sha D, Fu X. in vitro constitution and in vivo implantation of engineered skin constructs with sweat glands. Biomaterials. (2010) 31:5520–25. doi: 10.1016/j.biomaterials.2010.03.060
138. Brandenburger M, Kruse C. Fabrication of a co-culture system with human sweat gland-derived cells and peripheral nerve cells. Methods Mol Biol. (2019) 1993:139–48. doi: 10.1007/978-1-4939-9473-1_11
139. Lalley AL, Boyce ST. Fabrication of chimeric hair follicles for skin tissue engineering. Methods Mol Biol. (2019) 1993:159–79. doi: 10.1007/978-1-4939-9473-1_13
140. Kalyanaraman B, Boyce S. Assessment of an automated bioreactor to propagate and harvest keratinocytes for fabrication of engineered skin substitutes. Tissue Eng. (2007) 13:983–93. doi: 10.1089/ten.2006.0338
141. Cubo N, Garcia M, Del Canizo JF, Velasco D, Jorcano JL. 3D bioprinting of functional human skin: production and in vivo analysis. Biofabrication. (2016) 9:015006. doi: 10.1088/1758-5090/9/1/015006
142. Pourchet LJ, Thepot A, Albouy M, Courtial EJ, Boher A, Blum LJ, et al. Human skin 3D bioprinting using scaffold-free approach. Adv Healthc Mater. (2017) 6:1601101–8. doi: 10.1002/adhm.201601101
143. Koch L, Michael S, Reimers K, Vogt PM, Chichkov B. Chapter 13 - bioprinting for skin. In: Zhang LG, Fisher JP and Leong KW, editors. 3D Bioprinting and Nanotechnology in Tissue Engineering and Regenerative Medicine. London, UK: Elsevier Inc., Academic Press (2015). p. 281–306.
144. Albanna M, Binder KW, Murphy SV, Kim J, Qasem SA, Zhao W, et al. In situ bioprinting of autologous skin cells accelerates wound healing of extensive excisional full-thickness wounds. Sci Rep. (2019) 9:1856. doi: 10.1038/s41598-018-38366-w
145. Smandri A, Nordin A, Hwei NM, Chin KY, Abd Aziz I, Fauzi MB. Natural 3D-printed bioinks for skin regeneration and wound healing: a systematic review. Polymers. (2020) 12:1782–1800. doi: 10.3390/polym12081782
146. Supp DM, Hahn JM, McFarland KL, Combs KA, Lee KS, Inceoglu B, et al. Soluble epoxide hydrolase inhibition and epoxyeicosatrienoic acid treatment improve vascularization of engineered skin substitutes. Plast Reconstr Surg Glob Open. (2016) 4:e1151. doi: 10.1097/GOX.0000000000001151
Keywords: skin, bioengineering, burns, wound closure, skin substitutes, clinical translation, tissue engineering, biopolymers
Citation: Dearman BL, Boyce ST and Greenwood JE (2021) Advances in Skin Tissue Bioengineering and the Challenges of Clinical Translation. Front. Surg. 8:640879. doi: 10.3389/fsurg.2021.640879
Received: 12 December 2020; Accepted: 31 July 2021;
Published: 24 August 2021.
Edited by:
Claudia Di Bella, The University of Melbourne, AustraliaReviewed by:
Marc G. Jeschke, University of Toronto, CanadaLars-Peter Kamolz, Medical University of Graz, Austria
Copyright © 2021 Dearman, Boyce and Greenwood. This is an open-access article distributed under the terms of the Creative Commons Attribution License (CC BY). The use, distribution or reproduction in other forums is permitted, provided the original author(s) and the copyright owner(s) are credited and that the original publication in this journal is cited, in accordance with accepted academic practice. No use, distribution or reproduction is permitted which does not comply with these terms.
*Correspondence: Bronwyn L. Dearman, bronwyn.dearman@sa.gov.au