- 1Fondazione Policlinico Universitario “Agostino Gemelli” Istituto di ricovero e cura a carattere scientifico (IRCCS), Rome, Italy
- 2Section of Psychiatry, Department of Neuroscience, Università Cattolica del Sacro Cuore, Rome, Italy
- 3Section of Psychiatry, Department of Medical Sciences and Public Health, University of Cagliari, Cagliari, Italy
- 4Unit of Clinical Psychiatry, University Hospital Agency of Cagliari, Cagliari, Italy
- 5Department of Pharmacology, Dalhousie University, Halifax, NS, Canada
- 6Menninger Department of Psychiatry and Behavioral Sciences, Baylor College of Medicine, Houston, TX, United States
- 7Department of Neurology and Psychiatry, Sapienza University of Rome, Rome, Italy
- 8Department of Psychiatry, Icahn School of Medicine at Mount Sinai, New York, NY, United States
Severe mental disorders (SMD) are highly prevalent psychiatric conditions exerting an enormous toll on society. Therefore, prevention of SMD has received enormous attention in the last two decades. Preventative approaches are based on the knowledge and detailed characterization of the developmental stages of SMD and on risk prediction. One relevant biological component, so far neglected in high risk research, is microbiota. The human microbiota consists in the ensemble of microbes, including viruses, bacteria, and eukaryotes, that inhabit several ecological niches of the organism. Due to its demonstrated role in modulating illness and health, as well in influencing behavior, much interest has focused on the characterization of the microbiota inhabiting the gut. Several studies in animal models have shown the early modifications in the gut microbiota might impact on neurodevelopment and the onset of deficits in social behavior corresponding to distinct neurosignaling alterations. However, despite this evidence, only one study investigated the effect of altered microbiome and risk of developing mental disorders in humans, showing that individuals at risk for SMD had significantly different global microbiome composition than healthy controls. We then offer a developmental perspective and provided mechanistic insights on how changes in the microbiota could influence the risk of SMD. We suggest that the analysis of microbiota should be included in the comprehensive assessment generally performed in populations at high risk for SMD as it can inform predictive models and ultimately preventative strategies.
Introduction
Severe mental disorders (SMD), including schizophrenia, bipolar disorder and major depressive disorder, are commonly occurring psychiatric conditions exerting an enormous toll on society (1). The 2010 estimate of Gustavsson and co-authors showed that cumulatively direct and indirect costs associated to SMD amount at ~€140 billion per year in Europe (2). Several factors, other than the elevated prevalence in the general population, determine the substantial burden of SMD. First, their longitudinal trajectory start during late adolescence-young adulthood with a life-long duration in the vast majority of cases (3, 4). Second, the clinical course of SMD is often chronic with recurrent episodes of psychopathological disturbances and presence of persistent residual symptoms that significantly affect functioning and quality of life. Indeed, SMD represent a major contributor to the total amount of disability-adjusted life-years attributed to communicable and non-communicable diseases at a global level (5). This appears to be mainly determined by the third determinant of burden, i.e., the presence of suboptimal patterns of response to treatments, either pharmacological or non-pharmacological, leading to only a minority of patients achieving psychopathological and functional remission. Finally, SMD are associated with a considerable excess morbidity and mortality (6–8), which cause a significant reduction in life expectancy (on average 10–20 years) compared to the general population (9, 10). In this context, there has been a constant attempt to improve outcomes of SMD. This strategy has mainly focused on prevention, with the most validated paradigm focusing on primary prevention in individuals presenting subtle symptoms and at clinical high risk for SMD (11). Although the early phases of SMD appear to have distinct developmental trajectories for major affective disorders (4) and schizophrenia (3), particularly in the prodromal phases, there is a general consensus that individuals at risk for SMD are those having a genetic liability due to a high familial loading and/or the presence of antecedents such as basic symptoms, cognitive development, affective lability, anxiety, sleep problems, and psychotic-like experiences (11–13).
In this context, risk prediction of SMD is of paramount importance. Several modeling approaches have been developed using clinical (phenotypic) (14), genomic (15, 16), epigenomic (17), or integrated phenotypic-omics datasets (18). However, although the accuracy of prediction in the proposed models appears adequate for clinical purposes (18), and/or feasible in their implementations (14), there is still need of replication and validation of their predictive power in real life clinical settings. One biological component, partly inherited (19), that has been so far neglected in risk prediction of SMD, is the microbiota. The human microbiota consists in the ensemble of microbes, including viruses, bacteria, and eukaryotes, that inhabit several ecological niches of the organism (20, 21). Due to its demonstrated role in modulating illness and health, much interest has focused on the characterization of the microbiota inhabiting the gut (20). In fact, alterations of the gut microbiota have been linked, among the others, to obesity (22), maturation of the immune system (23), and response to drugs (24). Of particular interest is the modulating role that the microbiota acquires in human behavior (25), raising the interest for the investigation of its modifications in SMD. Indeed, several studies have shown substantial alterations, mainly decreased diversity in species within the microbiota, in schizophrenia (26, 27), in bipolar disorder (28), and major depressive disorder (29, 30). For instance, Zhu and coauthors found that, compared to 81 healthy controls, the gut microbiota of 90 medication-free patients with schizophrenia harbored many facultative anaerobes such as Lactobacillus fermentum, Enterococcus faecium, Alkaliphilus oremlandii, and Cronobacter sakazakii/turicensis, typically rare in a healthy gut (31). Of note the schizophrenia-associated bacterium Streptococcus vestibularis, which contributed to the microbiota metagenomic-based discrimination of patients with schizophrenia from healthy controls, when transplanted to mice gut induced deficits in social behaviors, altering neurotransmitter levels in peripheral tissues of recipient animals (31). In bipolar disorder, Painold and co-authors found that gut microbiota alpha-diversity decreased with increasing illness duration and that Actinobacteria and Coriobacteria were overrepresented in patients compared to healthy controls (HC) (28). Finally, patients with major depressive disorder showed a statistically significant overrepresentation of Bacteroides enterotype 2 compared to controls (32). In addition, a recent systematic review showed that gut dysbiosis and the leaky gut may affect pathways implicated in the neurobiology of major depressive disorder, such immune regulation, oxidative and nitrosative stress, and neuroplasticity (29). However, there is still limited evidence on how microbiota might vary in individuals at risk for SMD compared to healthy controls, as well as to individuals in later stages of SMD. However, there is extensive evidence that the microbiota has a key role in neurodevelopment and can be a modulating factor of the maturity of the central nervous system (CNS) in early developmental stages (33). In this scenario, the aim of this mini review is to present the current evidence on microbiota changes in individuals at high risk for SMD, offering a developmental perspective and providing mechanistic insights on how changes in the gut microbiota make-up could influence the risk of SMD.
Gut Microbiota in at Risk Mental States: A Developmental Perspective
Recent evidence suggests that the shaping of the microbiome occurs in parallel with the growth of CNS and that they have similar critical developmental windows (34). Consequently, the influence of alterations of gut microbiota on brain maturation trajectories, as well as their relationship with an increased risk for mental disorders later in life have been extensively investigated by preclinical studies (35, 36). In fact, alterations in maternal microbiome have been shown to impact offspring's brain maturation and post-natal development of psychopathology. Buffington et al. (37), observed that the offspring of high-fat diet exposed mice showed autism spectrum disorders/schizophrenia-like symptoms, such as reduced social interactions, poor interest in social novelty, and altered sociability compared to the offspring of normal fed mice (37). These behavioral alterations were coupled with a 9-fold reduction of Lactobacillus reuteri and a reduced number of cells producing oxytocin in the paraventricular nuclei of the hypothalamus (37). Other studies investigated the effect of altered maternal gut microbiome on the offspring's behavior through the administration of antibiotics during or immediately before mice pregnancy. A plethora of postnatal aberrant behavior, such as decreased locomotor and explorative activity, low prepulse inhibition, poor social interactions, and anxiety emerged (38, 39). Interestingly, aberrant behavior was completely reversed after fostering the pups by control dams (39). Other factors, such as maternal exposure to stress, can alter the offspring's gut microbiome and affect behavior. Several studies showed that the offspring exposed to perinatal maternal stress showed decreased levels of Lactobacillus and Bifidobacterium (40–42). These alterations were associated to increased anxiety and impaired cognitive functions, which started early during development and lasted until adulthood (40–42). Furthermore, gut microbiome composition and behavioral alterations were paired with increased levels of interleukin-1β and decreased brain-derived neurotropic factor (BDNF) in the amygdala (41).
Together with the intrauterine stage, the postnatal period represents a critical moment for both gut microbiota and brain development (34). This developmental stage represents the time when the most dramatic changes in the composition of the intestinal microbiota take place. These are mainly driven by a series of factors, spanning from maternal delivery modalities to genetic diathesis (43–45). Therefore, the interactions between the developing gut microbiota and brain structure and function in this specific developmental phase have undergone extensive investigations. Sudo et al. reported that germ-free (GF) mice, i.e., animals that have never had contact with any microorganism, showed heightened hypothalamic-pituitary-adrenal (HPA) system response to acute restraint stress as compared to mice with a normal gut flora (46). Such phenotype was accompanied by reduced expression of hippocampal and cortical brain-derived neurotrophic factor (BDNF). When GF were administered with a single strain of bacterium, Bifidobacterium infantis, stress response normalized (46). However, normalization processes were only possible in GF at early developmental stage, whereas the same procedure in later stages had no effects (46). Another study (47) demonstrated that GF mice showed anxious behavior and increased levels of serotonin in the hippocampus. Even in this case, gut colonization after weaning, which is comparable to adolescence in humans, was uncapable of restoring normal serotonin levels, even though anxiety normalized. Accordingly, in another study (48), post-weaning bacteria colonization was not able to normalize myelin oligodendrocyte glycoprotein levels in GF mice. Cumulatively, these data point toward the existence of specific, and limited, critical periods for the gut microbiota to act on neuronal circuits function and plasticity. The work of Desbonnet et al. (49) further expanded such concept. In their work, post-weaning colonization only partially corrected autism-spectrum-disorder-like behavior in GF mice: self-grooming and social avoidance improved, whereas social cognition did not (49). The authors suggested that the window of opportunity for the microbiota to impact brain circuits might be different for distinct emotional/social behaviors and, eventually, sensory modalities (49). These findings are summarized in Table 1.
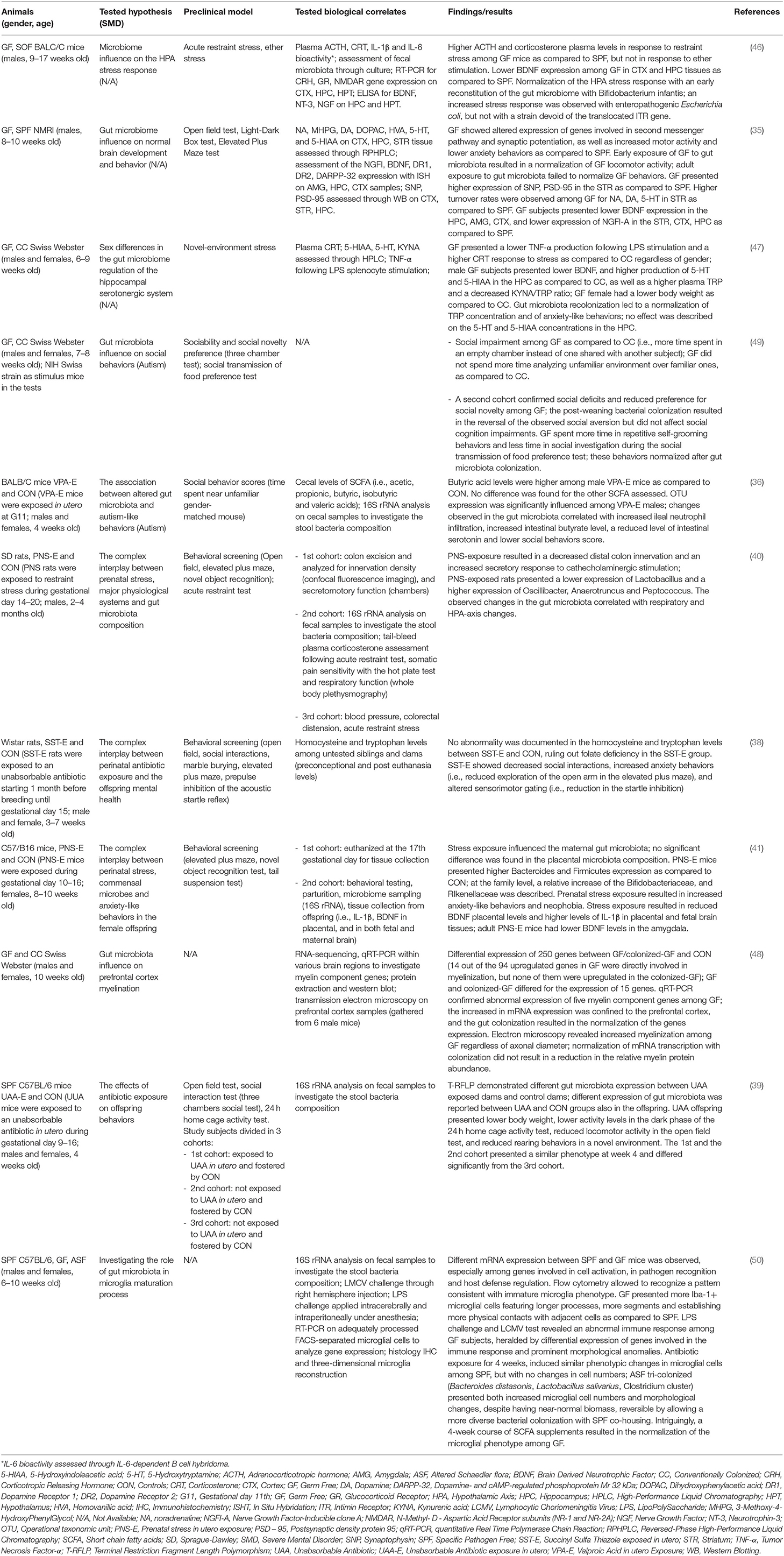
Table 1. Summary of findings of the pre-clinical studies and/or postulated biological underpinnings of SMD on gut microbiota.
Gut Microbiota in at Risk Mental States: Clinical Data
Despite the relatively large amount of studies investigating the relationship between gut microbiota composition and neurodevelopmental alterations in mice, only one study investigated the effect of altered microbiome and risk of developing mental disorders in humans (51). Specifically, He et al. (51) investigated alpha-diversity (i.e., the bacterial diversity within a single sample) and beta-diversity (differences in species composition among samples) metrics of gut microbiome in high-risk (HR), ultra-high-risk (UHR) subjects for developing schizophrenia and HC (51). Beta-diversity analysis revealed that UHR and HR had significantly different global microbiome composition than HC. Furthermore, UHR showed greater levels of Clostridiales, Lactobacillales, Bacteroidales, higher levels of Acetyl coenzyme A synthesis and greater anterior cingulate choline levels than the both HR and HC. The authors pointed out that the alterations in microbiome overlapped with those identified in schizophrenia and autism-spectrum disorder (52, 53). Additionally, higher levels of choline were interpreted as resultant of altered membrane metabolism due to microglial activation, which is one of the possible mechanisms mediating the effects of an altered gut microbiome on neural development (51). Putative mechanisms of the interplay between microbiota and genetic predisposition in modulating the liability toward the development of a SMD is discussed below. We have summarized clinical evidence in Table 2.
Mechanistic Hypotheses on the Influence of Gut Microbiota on at Risk Status for Severe Mental Disorders
There is compelling evidence that the products of gut microbiota might influence behavior in mammals through the action of their byproducts on the CNS (25). For instance, metabolic waste products of the gut microbiota such as the short-chain fatty acids (SCFAs) can influence neuromodulation via inhibition of the histone deacetylases (25, 54). In addition, another byproduct such as butyrate helps maintaining the integrity of the blood-brain barrier (25, 55), while acetate appears to exert anorectic effects via preferential accumulation in the hypothalamus (56). Other sets of findings have pointed to the link between gut dysbiosis and increased gut permeability and alterations of mitochondrial function, with significant repercussions at the CNS level (57). This amount of evidence, supported by the clinical and preclinical findings on the impact of gut microbiota on neurodevelopment, has fostered several mechanistic hypotheses (58, 59). While an extensive discussion of these mechanisms is out of the scope of the present mini review, we present a synthesis that we reckon as relevant for the high-risk construct of SMD. An altered neurodevelopment due to maternal gut flora modifications might be the resultant of poor regulation of maternal/fetus inflammatory state mediated by the maternal gut microbiome (58). Adequate gut microbial colonization in pregnant mice was associated to expression of regulatory T-cells (Tregs). Tregs normalize systemic levels of proinflammatory cytokines, such as IL-17 and interferon-γ (60), thus maintaining correct inflammatory/non-inflammatory balance. The lack of gut microbiota in GF pregnant mice resulted in a decrease of Tregs, with a general imbalance toward maternal and fetal inflammatory state (60). High levels of proinflammatory cytokines have been shown to induce fetal abnormal cortical development and surge of post-natal autism-like behavior (61). Alteration of maternal gut microbiome might also increase levels of fermentation products (CFAs), namely acetate, propionate and butyrate (62). Indeed, CFAs are capable to massively activate microglia, the immune cells of the CNS playing an important role in CNS homeostasis (50). Microglia activity might initiate/exacerbate the inflammatory cascade leading to the massive release of cytokines as well as to associated alterations in the endothelial permeability, including the blood-brain barrier. Such cascade has been shown to predispose to the development of neurodegenerative disorders, including schizophrenia and Parkinson's disease (59, 63).
Another putative mechanism might involve alterations in neurogenesis and specifically the BDNF which is involved in neural growth and cell survival. As previously shown, the gut microbiota is involved in the expression of BDNF (64). Prenatal/postnatal alterations of the gut microbiota can alter BDNF expression, and these changes could alter maturation trajectories of neural circuitry, leading to the development of SMD (65–68). Furthermore, gut microbiota can modify oligodendrocyte products and affect myelination, particularly in the prefrontal cortex, a brain region involved in attention, memory, emotional learning and critically connected to SMD such as ASD (69), schizophrenia (70), major depressive disorder (71), bipolar disorder (72), and substance abuse (73). Specifically, altered myelination has been related to changes in synaptic formation and function, which could lead to the surge of specific cognitive deficits typically seen in schizophrenia, namely deficits in attention, working memory, and executive function (74).
Another interesting, but still under-investigated, mechanism is represented by the effect of the gut microbiome on the Wnt pathways. These are signal transduction pathways mainly involved in human development, cell migration and proliferation and tissue regeneration (75). Wnt pathways are also involved in neural morphogenesis, axon guidance, neurite outgrowth, and synaptic plasticity (76, 77). Alterations in Wnt pathways have been recently related to higher risk to develop SMD, such as schizophrenia or bipolar disorder (78, 79). Of note, GF mice showed poor Wnt pathway activity in intestinal stem cells (80), supporting the speculation of a possible link between alteration of gut microglia, altered neurodevelopment and consequent increased risk for SMD. However, proper investigation of the relationship between gut microbiome alterations and altered Wnt pathways is still underdeveloped and needs further research. All these mechanisms are illustrated in Figure 1.
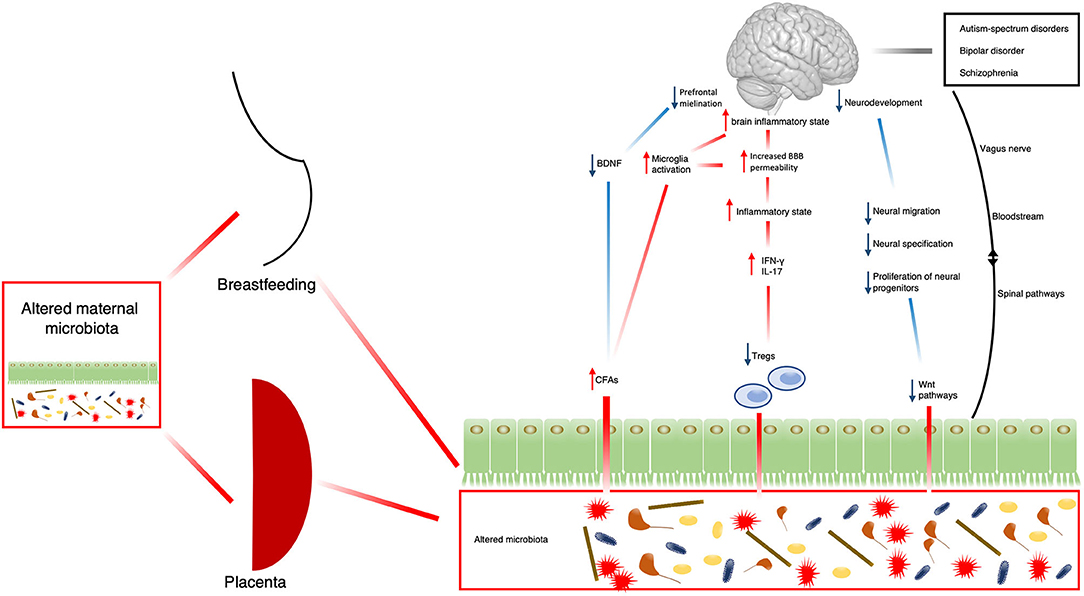
Figure 1. Schematic representation of possible mechanisms by which the microbiota might contribute to the development of SMD. BDNF, brain-derived neurotrophic factor; CFAs, fermentation products; IFN-γ, interferon-γ; IL-17, Interleukin-17; Tregs, regulatory T-cells; Wnt, homologous wingless and Int-1.
Conclusions
The findings of our review prompt a series of considerations. First, despite the consensus that microbiota plays a fundamental role in neurodevelopment and substantial changes are detectable in individuals affected by SMD, there is a dearth of studies investigating its modifications during the developmental trajectories of these disorders, particularly in high-risk populations. This could be feasible particularly in consideration that appropriate clinical chemistry and molecular immunology assays to assess for the presence of biological markers of “leaky gut” might be easily implementable in clinical settings (81). Second, only a longitudinal perspective could shed light on the direction of these changes, i.e., whether microbiota modifications precede the onset of psychopathology (of whatever severity) or vice versa. This perspective could be applied, but should not be limited, to the early stages of SMD. Indeed, prospective analysis of microbiota changes are starting to shed light on the longitudinal variation of mood in the course of bipolar disorder (82). Third, this approach can help decrease the confounding associated with the use of drug treatments (if the analyses are performed in pre-diagnostic stages), and at the same time inform on changes that might favor, or be predictive of, response to treatment. In conclusion, we suggest that the analysis of microbiota should be included in the comprehensive assessment generally performed in populations at high risk for SMD as it can inform predictive models and ultimately preventative strategies.
Author Contributions
MM and GS drafted the first version of the article. AS, DJ, and PP helped with the search of the literature and contributed to the draft of the manuscript. FP and BC oversaw the project and revised the text critically for important intellectual content. All authors gave final approval of the version to be published and agree to be accountable for all aspects of the work.
Funding
This paper was partly funded by Fondo Integrativo per la Ricerca (FIR)-2019 granted to MM, FP, and BC.
Conflict of Interest
The authors declare that the research was conducted in the absence of any commercial or financial relationships that could be construed as a potential conflict of interest.
Acknowledgments
The authors wish to thank all patients affected by severe mental illness who make our research possible, and most importantly meaningful.
References
1. Rehm J, Shield KD. Global burden of disease and the impact of mental and addictive disorders. Curr Psychiatr Rep. (2019) 21:10. doi: 10.1007/s11920-019-0997-0
2. Gustavsson A, Svensson M, Jacobi F, Allgulander C, Alonso J, Beghi E, et al. Cost of disorders of the brain in Europe 2010. Eur Neuropsychopharmacol. (2011) 21:718–79. doi: 10.1016/j.euroneuro.2011.08.008
3. Millan MJ, Andrieux A, Bartzokis G, Cadenhead K, Dazzan P, Fusar-Poli P, et al. Altering the course of schizophrenia: progress and perspectives. Nat Rev Drug Discov. (2016) 15:485–515. doi: 10.1038/nrd.2016.28
4. Duffy A, Goodday S, Keown-Stoneman C, Grof P. The emergent course of bipolar disorder: observations over two decades from the Canadian high-risk offspring cohort. Am J Psychiatr. (2019) 176:720–9. doi: 10.1176/appi.ajp.2018.18040461
5. Hmwe Kyu H, Abate D, Hassen Abate K, Abay SM, Abbafati C, Abbasi N, et al. 2018 Global, regional, and national disability-adjusted life-years (DALYs) for 359 diseases and injuries and healthy life expectancy (HALE) for 195 countries and territories, 1990–2017: a systematic analysis for the global burden of disease study 2017. Lancet. 392:1859–922. doi: 10.1016/S0140-6736(18)32335-3
6. Harris C, Barraclough B. Excess mortality of mental disorder. Br J Psychiatr. (1998) 173:11–53. doi: 10.1192/bjp.173.1.11
7. De Hert M, Correll CU, Bobes J, Cetkovich-Bakmas M, Cohen D, Asai I, et al. Physical illness in patients with severe mental disorders. I Prevalence, impact of medications and disparities in health care. World Psychiatr. (2011) 10:52–77. doi: 10.1002/j.2051-5545.2011.tb00014.x
8. Chesney E, Goodwin GM, Fazel S. Risks of all-cause and suicide mortality in mental disorders: a meta-review. World Psychiatr. (2014) 13:153–60. doi: 10.1002/wps.20128
9. Druss BG, Zhao L, Von Esenwein S, Morrato EH, Marcus SC. Understanding excess mortality in persons with mental illness: 17-year follow up of a nationally representative US survey. Med Care. (2011) 49:599–604. doi: 10.1097/MLR.0b013e31820bf86e
10. Nordentoft M, Wahlbeck K, Hällgren J, Westman J, Ösby U, Alinaghizadeh H, et al. Excess mortality, causes of death and life expectancy in 270,770 patients with recent onset of mental disorders in Denmark, Finland and Sweden. PLoS ONE. (2013) 8:e0055176. doi: 10.1371/journal.pone.0055176
11. Fusar-Poli P, Borgwardt S, Bechdolf A, Addington J, Riecher-Rössler A, Schultze-Lutter F, et al. The psychosis high-risk state: A comprehensive state-of-the-art review. Arch Gen Psychiatr. (2013) 70:107–20. doi: 10.1001/jamapsychiatry.2013.269
12. Zwicker A, MacKenzie LE, Drobinin V, Howes Vallis E, Patterson VC, Stephens M, et al. Basic symptoms in offspring of parents with mood and psychotic disorders. BJPsych Open. (2019) 5:e54. doi: 10.1192/bjo.2019.40
13. Zwicker A, Drobinin V, MacKenzie LE, Howes Vallis E, Patterson VC, Cumby J, et al. Affective lability in offspring of parents with major depressive disorder, bipolar disorder and schizophrenia. Eur Child Adolesc Psychiatr. (2020) 29:445–51. doi: 10.1007/s00787-019-01355-z
14. Oliver D, Spada G, Englund A, Chesney E, Radua J, Reichenberg A, et al. Real-world digital implementation of the psychosis polyrisk score (PPS): a pilot feasibility study. Schizophr Res. (2020) 226:176–83. doi: 10.1016/j.schres.2020.04.015
15. Docherty AR, Shabalin AA, Adkins DE, Mann F, Krueger RF, Bacanu A, et al. Molecular genetic risk for psychosis is associated with psychosis risk symptoms in a population-based UK cohort: findings from generation scotland. Schizophr Bull. (2020) 46:1045–52. doi: 10.1093/schbul/sbaa042
16. Musliner KL, Krebs MD, Albiñana C, Vilhjalmsson B, Agerbo E, Zandi PP, et al. Polygenic risk progression to bipolar or psychotic disorders among individuals diagnosed with unipolar depression in early life. Am J Psychiatr. (2020) 177:936–43. doi: 10.1176/appi.ajp.2020.19111195
17. Watkeys OJ, Cohen-Woods S, Quidé Y, Cairns MJ, Overs B, Fullerton JM, et al. Derivation of poly-methylomic profile scores for schizophrenia. Prog Neuro Psychopharmacol Biol Psychiatr. (2020) 101:109925. doi: 10.1016/j.pnpbp.2020.109925
18. Bhak Y, Jeong H-O, Cho YS, Jeon S, Cho J, Gim JA, et al. Depression and suicide risk prediction models using blood-derived multi-omics data. Transl Psychiatr. (2019) 9:262. doi: 10.1038/s41398-019-0595-2
19. Bevins CL, Salzman NH. The potter's wheel: the host's role in sculpting its microbiota. Cell Mol Life Sci. (2011) 68:3675. doi: 10.1007/s00018-011-0830-3
20. Almeida A, Mitchell AL, Boland M, Forster SC, Gloor GB, Tarkowska A, et al. A new genomic blueprint of the human gut microbiota. Nature. (2019) 568:499–504. doi: 10.1038/s41586-019-0965-1
21. Proctor LM, Creasy HH, Fettweis JM, Lloyd-Price J, Mahurkar A, Zhou W, et al. The integrative human microbiome project. Nature. (2019) 569:641–8. doi: 10.1038/s41586-019-1238-8
22. Scarpellini E, Campanale M, Leone D, Purchiaroni F, Vitale G, Lauritano EC, et al. Gut microbiota and obesity. Intern Emerg Med. (2010) 5:53–6. doi: 10.1007/s11739-010-0450-1
23. Mazmanian SK, Liu CH, Tzianabos AO, Kasper DL. An immunomodulatory molecule of symbiotic bacteria directs maturation of the host immune system. Cell. (2005) 122:107–18. doi: 10.1016/j.cell.2005.05.007
24. Spanogiannopoulos P, Bess EN, Carmody RN, Turnbaugh PJ. The microbial pharmacists within us: a metagenomic view of xenobiotic metabolism. Nat Rev Microbiol. (2016) 14:273. doi: 10.1038/nrmicro.2016.17
25. Johnson KV-A, Foster KR. Why does the microbiome affect behaviour? Nat Rev Microbiol. (2018) 16:647–55. doi: 10.1038/s41579-018-0014-3
26. Cuomo A, Maina G, Rosso G, Crescenzi BB, Bolognesi S, Muro A, et al. The microbiome: A new target for research and treatment of schizophrenia and its resistant presentations? A systematic literature search and review. Front Pharmacol. (2018) 9:1040. doi: 10.3389/fphar.2018.01040
27. Rodrigues-Amorim D, Rivera-Baltanás T, Regueiro B, Spuch C, de las Heras ME, Vázquez-Noguerol Méndez R, et al. The role of the gut microbiota in schizophrenia: current and future perspectives. World J Biol Psychiatr. (2018) 19:571–85. doi: 10.1080/15622975.2018.1433878
28. Painold A, Mörkl S, Kashofer K, Halwachs B, Dalkner N, Bengesser S, et al. A step ahead: exploring the gut microbiota in inpatients with bipolar disorder during a depressive episode. Bipolar Disord. (2019) 21:40–9. doi: 10.1111/bdi.12682
29. Slyepchenko A, Maes M, Jacka FN, Köhler CA, Barichello T, McIntyre RS, et al. Gut microbiota, bacterial translocation, and interactions with diet: pathophysiological links between major depressive disorder and non-communicable medical comorbidities. Psychother Psychosom. (2017) 86:31–46. doi: 10.1159/000448957
30. Cheung SG, Goldenthal AR, Uhlemann AC, Mann JJ, Miller JM, Sublette ME. Systematic review of gut microbiota and major depression. Front Psychiatr. (2019) 10:34. doi: 10.3389/fpsyt.2019.00034
31. Zhu F, Ju Y, Wang W, Wang Q, Guo R, Ma Q, et al. Metagenome-wide association of gut microbiome features for schizophrenia. Nat Commun. (2020) 11:1–10. doi: 10.1038/s41467-020-15457-9
32. Valles-Colomer M, Falony G, Darzi Y, Tigchelaar EF, Wang J, Tito RY, et al. The neuroactive potential of the human gut microbiota in quality of life and depression. Nat Microbiol. (2019) 4:623–32. doi: 10.1038/s41564-018-0337-x
33. Dinan TG, Borre YE, Cryan JF. Genomics of schizophrenia: time to consider the gut microbiome? Mol Psychiatr. (2014) 19:1252–7. doi: 10.1038/mp.2014.93
34. Borre YE, O'Keeffe GW, Clarke G, Stanton C, Dinan TG, Cryan JF. Microbiota and neurodevelopmental windows: implications for brain disorders. Trends Mol Med. (2014) 20:509–18. doi: 10.1016/j.molmed.2014.05.002
35. Heijtz RD, Wang S, Anuar F, Qian Y, Björkholm B, Samuelsson A, et al. Normal gut microbiota modulates brain development and behavior. Proc Natl Acad Sci USA. (2011) 108:3047–52. doi: 10.1073/pnas.1010529108
36. De Theije CGM, Wopereis H, Ramadan M, van Eijndthoven T, Lambert J, Knol J, et al. Altered gut microbiota and activity in a murine model of autism spectrum disorders. Brain Behav Immun. (2014) 37:197–206. doi: 10.1016/j.bbi.2013.12.005
37. Buffington SA, Di Prisco GV, Auchtung TA, Ajami NJ, Petrosino JF, Costa-Mattioli M. Microbial reconstitution reverses maternal diet-induced social and synaptic deficits in offspring. Cell. (2016) 165:1762–75. doi: 10.1016/j.cell.2016.06.001
38. Degroote S, Hunting DJ, Baccarelli AA, Takser L. Maternal gut and fetal brain connection: increased anxiety and reduced social interactions in Wistar rat offspring following peri-conceptional antibiotic exposure. Prog Neuro Psychopharmacol Biol Psychiatr. (2016) 71:76–82. doi: 10.1016/j.pnpbp.2016.06.010
39. Tochitani S, Ikeno T, Ito T, Sakurai A, Yamauchi T, Matsuzaki H. Administration of non-absorbable antibiotics to pregnant mice to perturb the maternal gut microbiota is associated with alterations in offspring behavior. PLoS ONE. (2016) 11:e0138293. doi: 10.1371/journal.pone.0138293
40. Golubeva AV, Crampton S, Desbonnet L, Edge D, O'Sullivan O, Lomasney KW, et al. Prenatal stress-induced alterations in major physiological systems correlate with gut microbiota composition in adulthood. Psychoneuroendocrinology. (2015) 60:58–74. doi: 10.1016/j.psyneuen.2015.06.002
41. Gur TL, Shay L, Palkar AV, Fisher S, Varaljay VA, Dowd S, et al. Prenatal stress affects placental cytokines and neurotrophins, commensal microbes, and anxiety-like behavior in adult female offspring. Brain Behav Immun. (2017) 64:50–8. doi: 10.1016/j.bbi.2016.12.021
42. Jašarević E, Howard CD, Misic AM, Beiting DP, Bale TL. Stress during pregnancy alters temporal and spatial dynamics of the maternal and offspring microbiome in a sex-specific manner. Sci Rep. (2017) 7:44182. doi: 10.1038/srep44182
43. Lozupone CA, Stombaugh JI, Gordon JI, Jansson JK, Knight R. Diversity, stability and resilience of the human gut microbiota. Nature. (2012) 489:220–30. doi: 10.1038/nature11550
44. Maynard CL, Elson CO, Hatton RD, Weaver CT. Reciprocal interactions of the intestinal microbiota and immune system. Nature. (2012) 489:231–41. doi: 10.1038/nature11551
45. Parfrey LW, Knight R. Spatial and temporal variability of the human microbiota. Clin Microbiol Infect. (2012) 18:5–7. doi: 10.1111/j.1469-0691.2012.03861.x
46. Sudo N, Chida Y, Aiba Y, Sonoda J, Oyama N, Yu X, et al. Postnatal microbial colonization programs the hypothalamic–pituitary–adrenal system for stress response in mice. J Physiol. (2004) 558:263–75. doi: 10.1113/jphysiol.2004.063388
47. Clarke G, Grenham S, Scully P, Fitzgerald P, Moloney RD, Shanahan F, et al. The microbiome-gut-brain axis during early life regulates the hippocampal serotonergic system in a sex-dependent manner. Mol Psychiatr. (2013) 18:666–73. doi: 10.1038/mp.2012.77
48. Hoban AE, Stilling RM, Ryan FJ, Shanahan F, Dinan TG, Claesson MJ, et al. Regulation of prefrontal cortex myelination by the microbiota. Transl Psychiatr. (2016) 6:e774. doi: 10.1038/tp.2016.42
49. Desbonnet L, Clarke G, Shanahan F, Dinan TG, Cryan JF. Microbiota is essential for social development in the mouse. Mol Psychiatr. (2014) 19:146–8. doi: 10.1038/mp.2013.65
50. Erny D, de Angelis ALH, Jaitin D, Wieghofer P, Staszewski O, David E, et al. Host microbiota constantly control maturation and function of microglia in the CNS. Nat Neurosci. (2015) 18:965–77. doi: 10.1038/nn.4030
51. He Y, Kosciolek T, Tang J, Zhou Y, Li Z, Ma X, et al. Gut microbiome and magnetic resonance spectroscopy study of subjects at ultra-high risk for psychosis may support the membrane hypothesis. Eur Psychiatr. (2018) 53:37–45. doi: 10.1016/j.eurpsy.2018.05.011
52. de Angelis M, Piccolo M, Vannini L, Siragusa S, De Giacomo A, Serrazzanetti DI, et al. Fecal microbiota and metabolome of children with autism and pervasive developmental disorder not otherwise specified. PLoS ONE. (2013) 8:e76993. doi: 10.1371/journal.pone.0076993
53. Shen Y, Xu J, Li Z, Huang Y, Yuan Y, Wang J, et al. Analysis of gut microbiota diversity and auxiliary diagnosis as a biomarker in patients with schizophrenia: a cross-sectional study. Schizophr Res. (2018) 197:470–7. doi: 10.1016/j.schres.2018.01.002
54. Koh A, De Vadder F, Kovatcheva-Datchary P, Bäckhed F. From dietary fiber to host physiology: short-chain fatty acids as key bacterial metabolites. Cell. (2016) 165:1332–45. doi: 10.1016/j.cell.2016.05.041
55. Braniste V, Al-Asmakh M, Kowal C, Anuar F, Abbaspour A, Tóth M, et al. The gut microbiota influences blood-brain barrier permeability in mice. Sci Transl Med. (2014) 6:263ra158. doi: 10.1126/scitranslmed.3009759
56. Frost G, Sleeth ML, Sahuri-Arisoylu M, Lizarbe B, Cerdan S, Brody L, et al. The short-chain fatty acid acetate reduces appetite via a central homeostatic mechanism. Nat Commun. (2014) 5:4611. doi: 10.1038/ncomms4611
57. Anderson G, Maes M. Gut dysbiosis dysregulates central and systemic homeostasis via suboptimal mitochondrial function: assessment, treatment and classification implications. Curr Top Med Chem. (2020) 20:524–39. doi: 10.2174/1568026620666200131094445
58. Lowry CA, Smith DG, Siebler PH, Schmidt D, Stamper CE, Hassell JE, et al. The microbiota, immunoregulation, and mental health: implications for public health. Curr Environ Heal Rep. (2016) 3:270–86. doi: 10.1007/s40572-016-0100-5
59. Warner BB. The contribution of the gut microbiome to neurodevelopment and neuropsychiatric disorders. Pediatr Res. (2019) 85:216–24. doi: 10.1038/s41390-018-0191-9
60. Rook GAW, Raison CL, Lowry CA. Microbial ‘old friends’, immunoregulation and socioeconomic status. Clin Exp Immunol. (2014) 177:1–12. doi: 10.1111/cei.12269
61. Choi GB, Yim YS, Wong H, Kim S, Kim H, Kim SV, et al. The maternal interleukin-17a pathway in mice promotes autism-like phenotypes in offspring. Science. (2016) 351:933–9. doi: 10.1126/science.aad0314
62. Houser MC, Tansey MG. The gut-brain axis: Is intestinal inflammation a silent driver of Parkinson's disease pathogenesis? NPJ Park Dis. (2017) 3:3. doi: 10.1038/s41531-016-0002-0
63. Sampson TR, Debelius JW, Thron T, Janssen S, Shastri GG, Ilhan ZE, et al. Gut microbiota regulate motor deficits and neuroinflammation in a model of Parkinson's disease. Cell. (2016) 167:1469–80. doi: 10.1016/j.cell.2016.11.018
64. Lu B, Nagappan G, Lu Y. BDNF synaptic plasticity. cognitive function, and dysfunction. Handb Exp Pharmacol. (2015) 220:223–50. doi: 10.1007/978-3-642-45106-5_9
65. Atladóttir HÓ, Thorsen P, Østergaard L, Schendel DE, Lemcke S, Abdallah M, et al. Maternal infection requiring hospitalization during pregnancy autism spectrum disorders. J Autism Dev Disord. (2010) 40:1423–30. doi: 10.1007/s10803-010-1006-y
66. Atladóttir HÓ, Henriksen TB, Schendel DE, Parner ET. Autism after infection, febrile episodes, and antibiotic use during pregnancy: an exploratory study. Pediatrics. (2012) 130:e1447–54. doi: 10.1542/peds.2012-1107
67. Li M, Chang H, Xiao X. BDNF Val66Met polymorphism and bipolar disorder in European populations: a risk association in case-control, family-based and GWAS studies. Neurosci Biobehav Rev. (2016) 68:218–33. doi: 10.1016/j.neubiorev.2016.05.031
68. Di Carlo P, Punzi G, Ursini G. Brain-derived neurotrophic factor and schizophrenia. Psychiatr Genet. (2020) 29:200–10. doi: 10.1097/YPG.0000000000000237
69. Karim HT, Wang M, Andreescu C, Tudorascu D, Butters MA, Karp JF, et al. Acute trajectories of neural activation predict remission to pharmacotherapy in late-life depression. NeuroImage Clin. (2018) 19:831–9. doi: 10.1016/j.nicl.2018.06.006
70. Zhou Y, Fan L, Qiu C, Jiang T. Prefrontal cortex and the dysconnectivity hypothesis of schizophrenia. Neurosci Bull. (2015) 31:207–19. doi: 10.1007/s12264-014-1502-8
71. Janiri D, Moser DA, Doucet GE, Luber MJ, Rasgon A, Lee WH, et al. Shared neural phenotypes for mood and anxiety disorders: a meta-analysis of 226 task-related functional imaging studies. JAMA Psychiatr. (2020) 77:172–9. doi: 10.1001/jamapsychiatry.2019.3351
72. Phillips ML, Swartz HA. A critical appraisal of neuroimaging studies of bipolar disorder: toward a new conceptualization of underlying neural circuitry and a road map for future research. Am J Psychiatr. (2014) 171:829–43. doi: 10.1176/appi.ajp.2014.13081008
73. Rapinesi C, Del Casale A, Di Pietro S, Ferri VR, Piacentino D, Sani G, et al. Add-on high frequency deep transcranial magnetic stimulation (dTMS) to bilateral prefrontal cortex reduces cocaine craving in patients with cocaine use disorder. Neurosci Lett. (2016) 629:43–7. doi: 10.1016/j.neulet.2016.06.049
74. Takahashi N, Sakurai T, Davis KL, Buxbaum JD. Linking oligodendrocyte and myelin dysfunction to neurocircuitry abnormalities in schizophrenia. Prog Neurobiol. (2011) 93:13–24. doi: 10.1016/j.pneurobio.2010.09.004
75. Wodarz A, Nusse R. Mechanisms of Wnt signaling in development. Annu Rev Cell Dev Biol. (1998) 14:59–88. doi: 10.1146/annurev.cellbio.14.1.59
76. Cotter D, Kerwin R, Al-Sarraji S, Brion JP, Chadwich A, Lovestone S, et al. Abnormalities of Wnt signalling in schizophrenia–evidence for neurodevelopmental abnormality. Neuroreport. (1998) 9:1379–83. doi: 10.1097/00001756-199805110-00024
77. Hur EM, Zhou FQ. GSK3 signalling in neural development. Nat Rev Neurosci. (2010) 11:539–51. doi: 10.1038/nrn2870
78. Sani G, Napoletano F, Maria Forte A, Kotzalidis G, Panaccione I, Maria Porfiri G, et al. The wnt pathway in mood disorders. Curr Neuropharmacol. (2012) 10:239–53. doi: 10.2174/157015912803217279
79. Panaccione I, Napoletano F, Maria Forte A, Kotzalidis G, Del Casale A, Rapinesi C, et al. Neurodevelopment in schizophrenia: the role of the wnt pathways. Curr Neuropharmacol. (2013) 11:535–58. doi: 10.2174/1570159X113119990037
80. Moossavi S. Location-specific effect of microbiota and MyD88-dependent signaling on Wnt/β-catenin pathway and intestinal stem cells. Gut Microbes. (2014) 5:11–4. doi: 10.4161/gmic.27291
81. Simeonova D, Ivanovska M, Murdjeva M, Carvalho AF, Maes M. Recognizing the leaky gut as a trans-diagnostic target for neuro-immune disorders using clinical chemistry and molecular immunology assays. Curr Top Med Chem. (2018) 18:1641–55. doi: 10.2174/1568026618666181115100610
82. Manchia M, Squassina A, Pisanu C, Congiu D, Garzilli M, Guiso B, et al. Investigating the relationship between melatonin levels, melatonin system, microbiota composition and bipolar disorder psychopathology across the different phases of the disease. Int J Bipolar Disord. (2019) 7:27. doi: 10.1186/s40345-019-0163-y
Keywords: microbiome, schizophrenia, depression, genomics, animal models, autism spectrum disorder, Shannon index, alpha diversity
Citation: Sani G, Manchia M, Simonetti A, Janiri D, Paribello P, Pinna F and Carpiniello B (2021) The Role of Gut Microbiota in the High-Risk Construct of Severe Mental Disorders: A Mini Review. Front. Psychiatry 11:585769. doi: 10.3389/fpsyt.2020.585769
Received: 21 July 2020; Accepted: 15 December 2020;
Published: 12 January 2021.
Edited by:
Sven Haller, Rive Droite SA, SwitzerlandReviewed by:
Ravinder Nagpal, Wake Forest School of Medicine, United StatesDrozdstoy Stoyanov Stoyanov, Plovdiv Medical University, Bulgaria
Copyright © 2021 Sani, Manchia, Simonetti, Janiri, Paribello, Pinna and Carpiniello. This is an open-access article distributed under the terms of the Creative Commons Attribution License (CC BY). The use, distribution or reproduction in other forums is permitted, provided the original author(s) and the copyright owner(s) are credited and that the original publication in this journal is cited, in accordance with accepted academic practice. No use, distribution or reproduction is permitted which does not comply with these terms.
*Correspondence: Federica Pinna, fedepinna@inwind.it
†These authors have contributed equally to this work