- 1Qiqihar Branch of Heilongjiang Academy of Agricultural Sciences, Qiqihar, China
- 2Heilongjiang Provincial Key Laboratory of Crop Physiology and Ecology in Cold Region, Heilongjiang Provincial Engineering Technology Research Center of Crop Cold Damage, Harbin, China
- 3Northeast Branch of National Salt-Alkali Tolerant Rice Technology Innovation Center, Harbin, China
- 4Crop Cultivation and Tillage Institute of Heilongjiang Academy of Agricultural Sciences, Harbin, China
- 5Keshan Branch of Heilongjiang Academy of Agricultural Sciences, Qiqihar, China
Early-matured aromatic japonica rice from the Northeast is the most popular rice commodity in the Chinese market. The Qigeng10 (QG10) was one of the varieties with the largest planting area in this region in recent years. It was an early-matured japonica rice variety with a lot of superior traits such as semi-dwarf, lodging resistance, long grain, aromatic and good quality. Therefore, a high-quality assembly of Qigeng10 genome is critical and useful for japonica research and breeding. In this study, we produced a high-precision QG10 chromosome-level genome by using a combination of Nanopore and Hi-C platforms. Finally, we assembled the QG10 genome into 77 contigs with an N50 length of 11.80 Mb in 27 scaffolds with an N50 length of 30.55 Mb. The assembled genome size was 378.31Mb with 65 contigs and constituted approximately 99.59% of the 12 chromosomes. We identified a total of 1,080,819 SNPs and 682,392 InDels between QG10 and Nipponbare. We also annotated 57,599 genes by the Ab initio method, homology-based technique, and RNA-seq. Based on the assembled genome sequence, we detected the sequence variation in a total of 63 cloned genes involved in grain yield, grain size, disease tolerance, lodging resistance, fragrance, and many other important traits. Finally, we identified five elite alleles (qTGW2Nipponbare, qTGW3Nanyangzhan, GW5IR24, GW6Suyunuo, and qGW8Basmati385) controlling long grain size, four elite alleles (COLD1Nipponbare, bZIP73Nipponbare, CTB4aKunmingxiaobaigu, and CTB2Kunmingxiaobaigu) controlling cold tolerance, three non-functional alleles (DTH7Kitaake, Ghd7Hejiang19, and Hd1Longgeng31) for early heading, two resistant alleles (PiaAkihikari and Pid4Digu) for rice blast, a resistant allele STV11Kasalath for rice stripe virus, an NRT1.1BIR24 allele for higher nitrate absorption activity, an elite allele SCM3Chugoku117 for stronger culms, and the typical aromatic gene badh2-E2 for fragrance in QG10. These results not only help us to better elucidate the genetic mechanisms underlying excellent agronomic traits in QG10 but also have wide-ranging implications for genomics-assisted breeding in early-matured fragrant japonica rice.
Introduction
Rice (Oryza sativa L.) is a safe and staple food source for more than half of the world’s population and serves as a model plant for cereal genetic studies (Gross and Zhao, 2014). Novo sequencing and genomic technologies have been widely applied in rice to promote the shift of breeding schemes from conventional field selection to genomic-assisted breeding (Gu et al., 2022). O. sativa subsp. japonica/Geng and subsp. Indica/Xian are the two major subspecies of cultivated rice (Zhang et al., 2016a; Nie et al., 2017). The japonica/Geng rice planting area is 9.87 million ha, accounting for approximately 32.9 percent of the total rice planting area in China (Tang and Chen, 2021). Recently, the early-matured japonica/Geng rice is becoming more and more important, and its growing area was more than 4 million ha in Northeast China (Cui et al., 2022). Two genome draft sequences of the cultivated rice subspecies japonica/Geng Nipponbare and Indica/Xian 93-11 were released in 2002 (Goff et al., 2002; Yu et al., 2002). In 2005, the International Rice Genome Sequencing Project (IRGSP) published the first completed version of the Nipponbare sequence (International-Rice-Genome-Sequencing-Project, 2005). Over the last two decades, several pan-genomes including 66 rice genomes (Zhao et al., 2018b), 33 rice genomes (Qin et al., 2021), 111 rice genomes (Zhang et al., 2022a),251 rice genomes (Shang et al., 2022), and 12 japonica rice genome (Wang et al., 2023) were built including IR64, R498, Zhenshan 97, Minghui 63, Taichung Native 1, LTH, Kitaake, IR8, N22, Huajingxian74, HR12, Basmati 334, Dom Sufid, Huazhan and Tianfeng at the chromosome level, and Shennong265, DJ123, WR04-6, Suijing18, Koshihikari, Basmati, Kongyu-131, and Guangluai-4 at scaffold level have been assembled and released with unprecedented speed (Mahesh et al., 2016; Zhang et al., 2016b; Du et al., 2017; Li et al., 2017; Nie et al., 2017; Stein et al., 2018; Zhao et al., 2018b; Jain et al., 2019; Choi et al., 2020; Tanaka et al., 2020; Panibe et al., 2021; Li et al., 2021a; Yang et al., 2022; Zhang et al., 2022b). These assembled genome sequences will be helpful in pinpointing new causal variants that underlie complex agronomic traits and identifying many of the genome-specific loci that were absent from the Nipponbare reference genome. However, most of these varieties are Indica/Xian rice or landrace. Nevertheless, the genome of japonica/Geng differs significantly from that of indica/Xian (Nie et al., 2017). Since the release of the finished version genome of Nipponbare, only seven genomes at the scaffold level of early-matured japonica/Geng varieties in northern region of China including Shennong265, Liaogeng5, Yanfeng47, Suijing18, Longgeng31, Daohuaxiang2(Wuyoudao4), and Kongyu-131 were released (Nie et al., 2017; Li et al., 2018; Zhao et al., 2018b; Wang et al., 2023). Only Daohuaxiang2 and Suijing18 were belong to early-mature aromatic type. The public availability of japonica/Geng genomes at the chromosome level, especially for the early-mature aromatic type, remains largely blank (Nie et al., 2017). Moreover, a few genomes are not enough to represent the whole genomic content of the japonica rice. The novo assembled genomes of an early-mature aromatic variety would be advantageous for functional genomics and genome research. For example, if there is structural variation in the particular variety and the reference genome in the candidate region, the guiding role of the reference genome would be limited. So, there is still a need for de novo genome assembly for various purposes especially in early-mature aromatic rice breeding research.
Fragrant and long grain are key grain quality traits that directly influence the global market price of rice (Hui et al., 2022). The basmati rice and jasmine rice are the two most popular fragrant indica rice in the world. However, consumers from East Asia, including North China, Japan, and Korea tend to prefer japonica rice (Lu et al., 2022). So, the aromatic long grain rice from Northeast China, represented by Wuyoudao4 (WYD4), is the most famous rice in the Chinese market. WYD4 had a superior quality, but also had a number of defects, most importantly, poor lodging resistance, lack of cold tolerance and blast resistance, and late maturity (Gao et al., 2012). In 2019, Qiqihar Branch of Heilongjiang Academy of Agricultural Sciences developed Qigeng10 (QG10) to solved these defects of WYD4. The plant area of QG10 was 0.4 million ha in the recent three years. QG10 has been a major variety of early matured aromatic long grain rice in Northeast China. The construction of a high-quality chromosome-level genome of QG10 is very important for improving the efficiency of rice genetic mechanism studies for desirable agronomic traits, such as eating quality, cold tolerance, lodging tolerance and early maturity, as well as accelerating the process of high-quality rice breeding in cold region of northeast China by design (Li et al., 2021a). Here, we produced a high-precision QG10 chromosomal genome by performing whole-genome sequencing in the Nanopore platform (Lin et al., 2021), followed by the Hi-C-assisted assembly mount technology (Van Berkum et al., 2010). Our results provided several functionally important candidate alleles for the grain length, cold tolerance, early heading, disease resistance, lodging resistance, and nitrate-use of rice breeding in cold region of northeast China.
Materials and methods
Materials
The early-matured aromatic long-grain japonica rice variety QG10, which was developed by our own group, was licensed for release in 2019 and is now widely planted in Heilongjiang province in Northeast China. It was a semi-dwarf rice variety with a lot of superior traits such as long panicle, long grain, aromatic, and good quality (Figures 1A-D). It was selected from the cross between two aromatic japonica/Geng rice Wuyoudao4 (WYD4) and Suigeng4 (SG4) (Figure 1E). The seedlings of QG10 were grown on the agricultural farm of the Qiqihar Branch of Heilongjiang Academy of Agricultural Sciences. Field management practices were performed according to the most commonly followed agricultural practices of local farmers. The leaves, stems, roots, and panicles at heading stages from plants grown in the experimental station were collected in liquid nitrogen for isolating RNA. The young leaves of a single young plant were used to isolate genomic DNA.
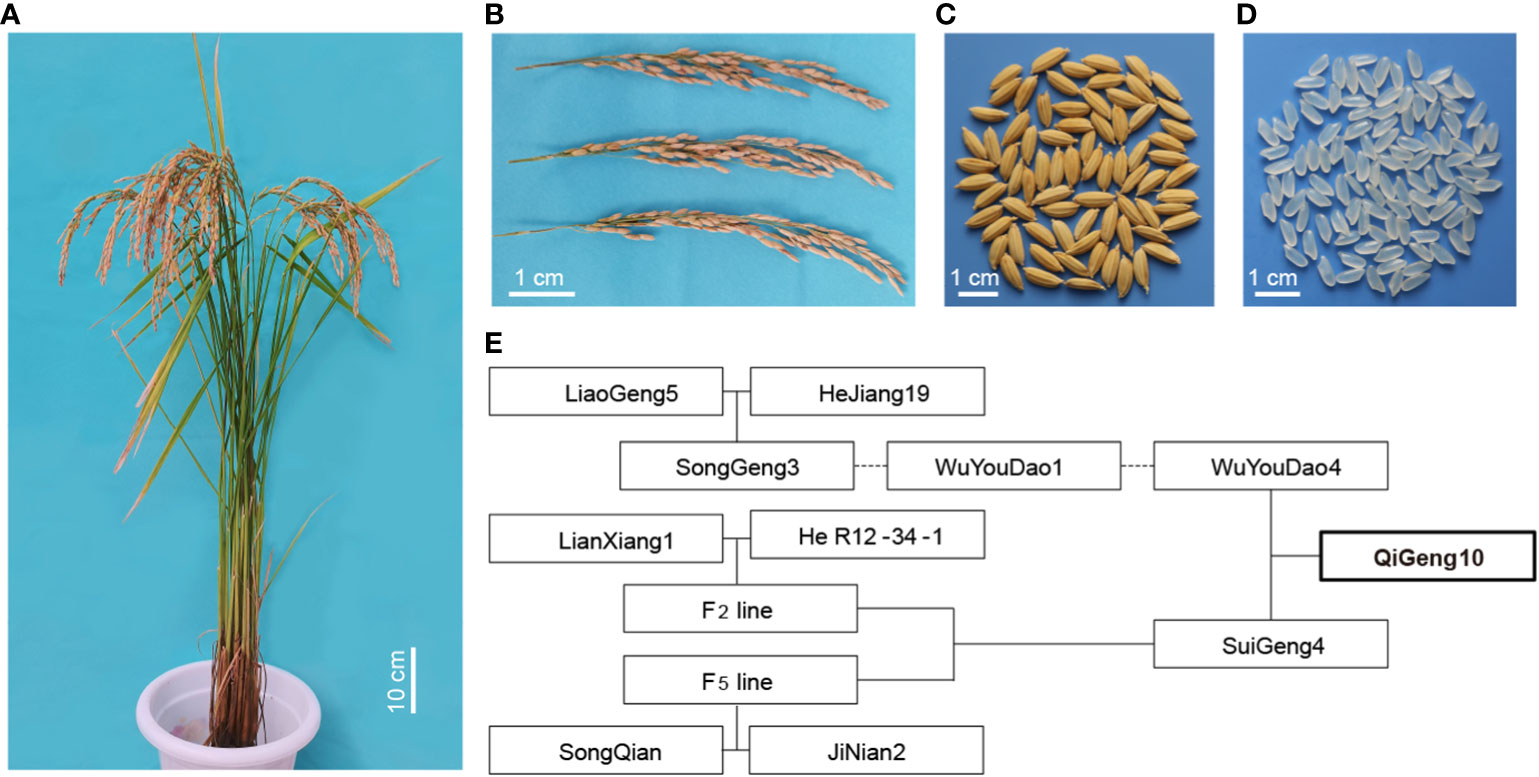
Figure 1 The of plant type, panicle phenotype, seeds phenotype, grains phenotype and pedigree of QG10. (A) The plant type of QG10; (B) The panicle morphology of QG 10; (C) The seed morphology of QG10; (D) The grain morphology of QG10; (E) The pedigree of QG10.
Oxford Nanopare sequencing and genome assembly
The high molecular weight genomic DNA of QG10 was extracted from the 15-day-old leaf tissues following a modified CTAB method. Whole genome sequencing was done following the standard instructions of the Ligation Sequencing Kit (Nanopore, Oxford shire, UK). The quantified DNA was randomly sheared, and fragments of ∼20 kb were enriched and purified. Then, a 20-kb library was constructed and sequenced on the Nanopore PromethION platform according to the manufacturer’s protocols (Jiang et al., 2020).
De novo genome assembly of Nanopore sequence was performed as follow: The raw Nanopore reads were error-corrected and assembled using CANU (v1.7.1) (Koren et al., 2017), followed by Smartdenovo (https://github.com/ruanjue/smartdenovo) assembly, followed by three rounds of polishing with Racon (Vaser et al., 2017), followed by three rounds with Pilon v0.3.0 using the Illumina PCR-free paired-end reads (Walker et al., 2014). Genome completeness was also assessed using the algae dataset of BUSCO v2.0 (Simão et al., 2015).
Hi-C library construction and sequencing
A Hi-C fragment library with a 300-700 bp insert size was constructed from the genomic DNA of the QG10. Briefly, adapter sequences of raw reads were trimmed and low-quality PE reads were removed for clean data. The library was sequenced on the Illumina HiSeq4000™ platform by Biomarker Technologies (Beijing, China). The clean Hi-C reads were first truncated at the putative Hi-C junctions and then the resulting trimmed reads were aligned to the assembly results with the software package bwa aligner (Li and Durbin, 2009). Only uniquely alignable pair reads whose mapping quality of more than 20 retained for further analysis. Invalid read pairs, including dangling-end and self-cycle, re-ligation, and dumped products, were filtered by the software package HiC-Pro v2.8.1 (Servant et al., 2015). The 96.25% of unique mapped read pairs were valid interaction pairs and were used for the correction of scaffolds and clustered, ordered, and orientated scaffolds onto chromosomes by the software package LACHESIS (Burton et al., 2013).
Genome assembly and Hi-C scaffolding
Before chromosome assembly, we first performed a preassembly for error correction of scaffolds which required the splitting of scaffolds into segments of 50 kb on average. The Hi-C data were mapped to these segments using the software package BWA (version 0.7.10-r789) (Li and Durbin, 2009). The uniquely mapped data were retained to perform assembly by using the software package LACHESIS (Burton et al., 2013). Any two segments which showed an inconsistent connection with information from the raw scaffold were checked manually. These corrected scaffolds were then assembled with the software package LACHESIS. After this step, placement and orientation errors exhibiting obvious discrete chromatin interaction patterns were manually adjusted.
Gene prediction and genome annotation
The RNA of QG10 were isolated from the mixed tissues (leaves, culms, roots, and panicles) following the manufacturer’s protocol (Wang et al., 2023). We then performed the sequencing on the Illumina HiSeq 2500 platform according to the manufacturer’s instructions. The repetitive sequence of the genome based on the principle of structure prediction and de-novo prediction was constructed with the software package LTR_FINDER v1.05 (Xu and Wang, 2007) and the software package RepeatScout v1.0.5 (Price et al., 2005). The PASTE Classifier was used to classify the database (Hoede et al., 2014). Then it was merged with the database of Repbase as the final repeat sequence database (Jurka et al., 2005). And then the software package RepeatMasker v4.0.6 was used to predict the repeat sequence of the QG10 genome based on the constructed repetitive sequence database (Tarailo‐Graovac and Chen, 2009). the software packages Genscan (Burge and Karlin, 1997), Augustus v2.4 (Stanke and Waack, 2003), GlimmerHMM v3.0.4 (Majoros et al., 2004), GeneID v1.4 (Alioto et al., 2018), and SNAP (Korf, 2004) were used for de-novo prediction. The software package GeMoMa v1.3.1 was used for prediction based on homologous species (Keilwagen et al., 2016; Keilwagen et al., 2018). The software packages Hisat v2.0.4 (Kim et al., 2015) and Stringtie v1.2.3 (Pertea et al., 2015) were used for assembly based on reference transcripts, and the software packages TransDecoder v2.0 and GeneMarkS-T v5.1 (Tang et al., 2015) were used for gene prediction. The software package PASA v2.0.2 was used to predict Unigene sequences without reference based on transcriptome data (Campbell et al., 2006). Finally, the software package EVM v1.1.1 (Haas et al., 2008) was used to integrate the prediction results obtained by the above three methods. The predicted gene sequences were compared with NR, KOG, GO, KEGG, TrEMBL, and other functional databases by the software package BLAST v2.2.31 (-evalue 1e-5) (Altschul et al., 1990) to perform KEGG pathway, KOG function, GO function and other genes functional annotation analysis.
Identification of genomic sequence variation in important genes
The whole-genome assemblies sequences of QG10 were compared with the rice reference genome sequence (Oryza_sativa_MSU7 version) using the software package MUMmer v3 (Kurtz et al., 2004). According to the results from the software package MUMmer, the sequence variations and SVs were further re-called using the software package BLAST. The synteny/inversion comparison were analysis by using GenomeSyn_Win.v1 (Zhou et al., 2022). At the site of each sequence variant, the genotypic information for QG10, Nipponbare, and the elite variety having important genes was called according to the results of the one-to-one alignments. The allelic information of sequence variants was detected based on gff files from the Oryza_sativa_MSU7 version. The software packages ClustalW v1.8.3(Thompson et al., 1994) and BLAST v2.2.31 were used for re-detected the sequence variations and detailed haplotype analyses for the well-characterized genes in rice (Zhao et al., 2018b).
Results
Nanopore sequencing and genome assembly
We sequenced QG10 genomic DNA to generate about 73.52Gb of Nanopore sequencing raw data. After data quality control, the clean data volume was 63.23Gb containing 3,279,893 reads with a total of 166.34-fold sequencing depth. The reads with 10 -20 kb and 20 - 30 kb sequencing length were account for 51.56% (Table 1). The mean reads length of clean sequencing data was 19.28 kb with an N50 length of 25.64 kb. The clean Nanopore sequencing data was re-corrected with the software package Canu (Koren et al., 2017). Then the third-generation sequencing data was re-corrected with the software package Racon (Vaser et al., 2017) for three rounds. Then, the second-generation data were used for three rounds of correction by Pilon (Walker et al., 2014) software, and the stain was removed according to NT alignment. Finally, we obtained a 380.15 Mb genome sequence with the contig N50 was 12.24 Mb. The completeness estimated by Benchmarking Universal Single-Copy Orthologs (BUSCO) was 98.12%.
Genome assembly and Hi-C scaffolding
We conducted the assembly in a stepwise fashion following a previously reported approach (Jiang et al., 2020). The Hi-C sequencing raw data was filtered, and the splice sequences and low-quality reads were removed to obtain high-quality clean data. The mapped data was obtained by sequence alignment of clean data with the preliminarily assembled genome. Finally, effective Hi-C data were used for further assembly of the draft genome sequence. LACHESIS software was used for clustering, sorting, and orientating the preliminary assembled genome sequence, and finally, the genomic sequence at the chromosome level was obtained. Finally, we assembled the genome (QG10) into 77 contigs with an N50 length of 11.80 Mb in 27 scaffolds with an N50 length of 30.55 Mb. The assembled genome size was 378.31Mb and the 65 contigs constituted approximately 99.59% of the whole genome. Visualization of the Hi-C signals indicated that 12 square matrix areas in the Hi-C heat map displayed significant differences from the background. These scaffolds were anchored into chromosomes 1–12, respectively (Figure 2A; Table 2).
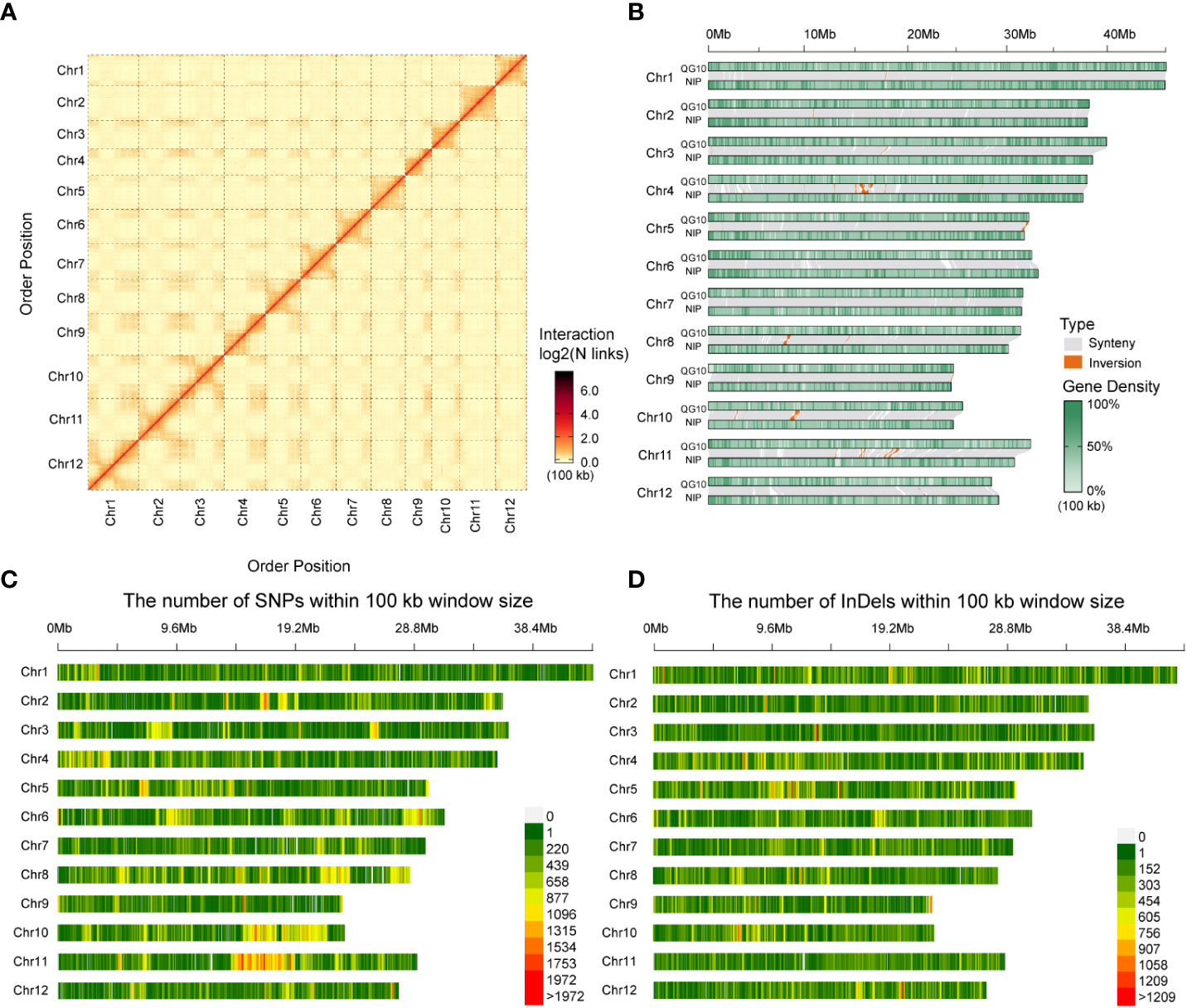
Figure 2 Characteristics of Qigeng10 (QG10) genome and synteny examining, SNPs and InDels mining, Synteny/Inversion comparison with Nipponbare. (A), Visualization of the Hi-C signals in the whole genome of QG10. (B), Chromosomal synteny among QG10 and Nipponbare reference genomes of rice. (C), Distribution of SNPs between QG10 and Nipponbare. (D), Distribution of InDels between QG10 and Nipponbare.
According to the whole genome comparison, the genome of QG10 showed four sequence inversions with a length of about 0.5-2 Mb compared with the Nipponbare genome at the position of about 14-16 Mb on chromosome 4, 30-31 Mb on chromosome 5, 5.5-6 Mb on chromosome 8, and 5.5-6 Mb on chromosome 10 (Figure 2B). We identified 1,080,819 SNPs and 682,392 InDels between QG10 and Nipponbare on 12 chromosomes (Figures 2C, D).
Genome annotation and repeat analysis
We annotated the repeat regions in our QG10 assembly by Repeat Masker and detected 492,503 repetitive regions with 177.52 Mb repeat length that contained 242,211 Class I retrotransposons, 223,439 Class II DNA transposons, 833 Potential Host Gene, and 2,222 simple sequence repeats (Table 3). The repeat regions make up 46.7% of the QG10 assembly genome.
We predicted 39,465 genes by the Ab initio method, 56,999 genes by the Homology-based method, and 24,998 by RNA-seq. Finally, a total of 57,599 genes were integrated with the prediction results obtained by the above three methods by using the software package EVM v1.1.1 (Table 4). A total of 723 tRNA, 306 rRNA, 194 miRNA, and 5,392 pseudogenes were also predicted. 94.11% of the genes could be annotated into NR, GO, KOG, KEGG, and other databases (Table 5).
Sequence variants of the genes controlling grain length
We investigated the sequence variations in 19 cloned genes controlling grain size, including GW2 (Song et al., 2007), GS2 (Hu et al., 2015), qTGW2 (Ruan et al., 2020), BG1 (Liu et al., 2015), OsLG3 (Yu et al., 2017), OsLG3b (Yu et al., 2018), GS3 (Fan et al., 2006), qTGW3 (Hu et al., 2018), GS5 (Li et al., 2011), GW5 (Weng et al., 2008), GS6 (Sun et al., 2013), GW6 (Shi et al., 2020), TGW6 (Ishimaru et al., 2013), GW6a (Song et al., 2015), GL6 (Wang et al., 2019), GLW7 (Si et al., 2016), GW7 (Wang et al., 2015), qGW8 (Wang et al., 2012), and GS9 (Zhao et al., 2018a), to explain the long grain of QG10. A total of five grain size elite alleles (qTGW2Nipponbare, qTGW3Nanyangzhan, GW5IR24, GW6Suyunuo, and qGW8Basmati385) were identified controlling grain size in QG10 (Figure 3). The qTGW2 allele in QG10 was identical to Nipponbare having the key variants (G/A) at -1818 bp in the promoter region (Figure 3A). The qTGW3 allele in QG10 had the key splicing-site mutation as Nanyangzhan, which was a long-grain indica rice (Figure 3B). The GW5 allele in QG10 was found without the critical loss of the 1,212 bp deletion mutation as IR24 a long narrow grain indica rice (Figure 3C). The GW6 allele in QG10 had the key mutation (6 bp) as Suyunuo, which was a wider grain indica rice (Figure 3D). The qGW8 allele in QG10 had the five variants as Basmati385, a long narrow grain indica rice (Figure 3E). Tracing the origin of these genes, it was found that qTGW3 (Nanyangzhan type), GW5 (IR24 type), GW6 (Suyunuo type), and qGW8 (Basmati385 type) belonged to indica subspecies, while qTGW2 (Nipponbare type) were mainly derived from japonica subspecies. In addition, these genes controlling grain type are all rare genotypes in japonica rice and have important application value in long grain type japonica rice breeding.
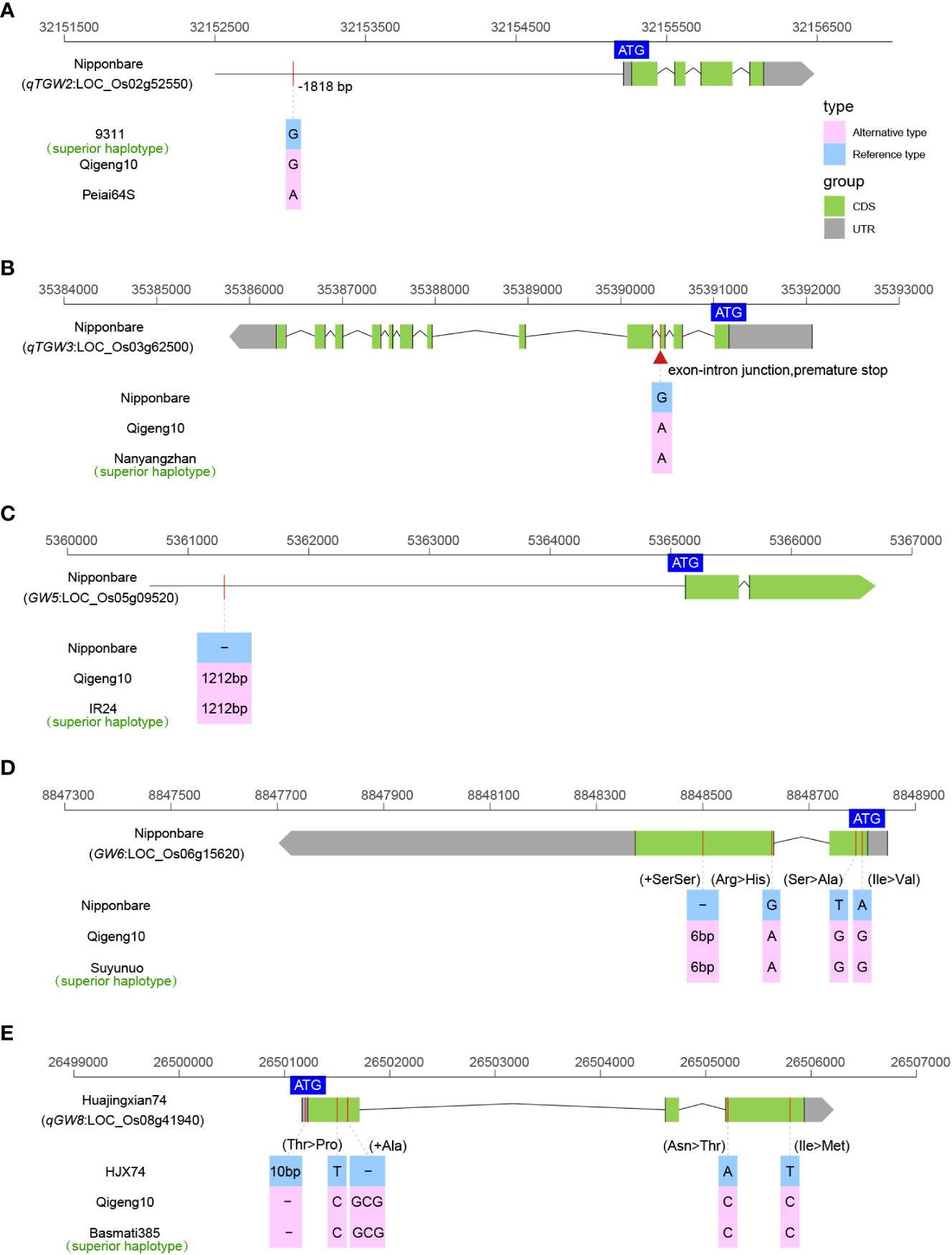
Figure 3 The allelic information of sequence variants in qTGW2 (A), qTGW3 (B), GW5 (C), GW6 (D), and qGW8 (E) controlling grain size.
Sequence variants of the genes controlling cold tolerance
Cold tolerance is the key agricultural trait controlling rice production and geographic distribution. We investigated the sequence variations in 10 cloned genes controlling cold tolerance, including bZIP73 (Liu et al., 2018), COLD1 (Ma et al., 2015), Ctb1 (Saito et al., 2010), CTB2 (Li et al., 2021b), CTB4a (Zhang et al., 2017), HAN1 (Mao et al., 2019), ltt1 (Xu et al., 2020), OsLTPL159 (Zhao et al., 2020), qLTG3-1 (Fujino et al., 2008), and qPSR10 (Xiao et al., 2018), to explain the high cold tolerance of QG10. A total of four elite alleles (COLD1Nipponbare, bZIP73Nipponbare, CTB4aKunmingxiaobaigu, and CTB2Kunmingxiaobaigu) were identified as controlling cold tolerance in QG10 (Figure 4). The COLD1 allele in QG10 was identical to Nipponbare to have the key SNP in the fourth exon region (Figure 4A). The bZIP73 allele in QG10 was found having the key SNP mutation (G/A) as Nipponbare (Figure 4B). The CTB4a allele in QG10 was found to have the ten mutations as Kunmingxiaobaigu, which was a cold tolerance variety from Yunnan Province (Figure 4C). The CTB2 allele in QG10 was found also have the ten key SNPs as Kunmingxiaobaigu (Figure 4D). The COLD1 (Nipponbare type) and bZIP73 (Nipponbare type) all belong to japonica subspecies. The CTB4a (Kunmingxiaobaigu type) and CTB2 (Kunmingxiaobaigu type) were all rare alleles in Northeast japonica rice and have important application value in Northeast japonica rice breeding.
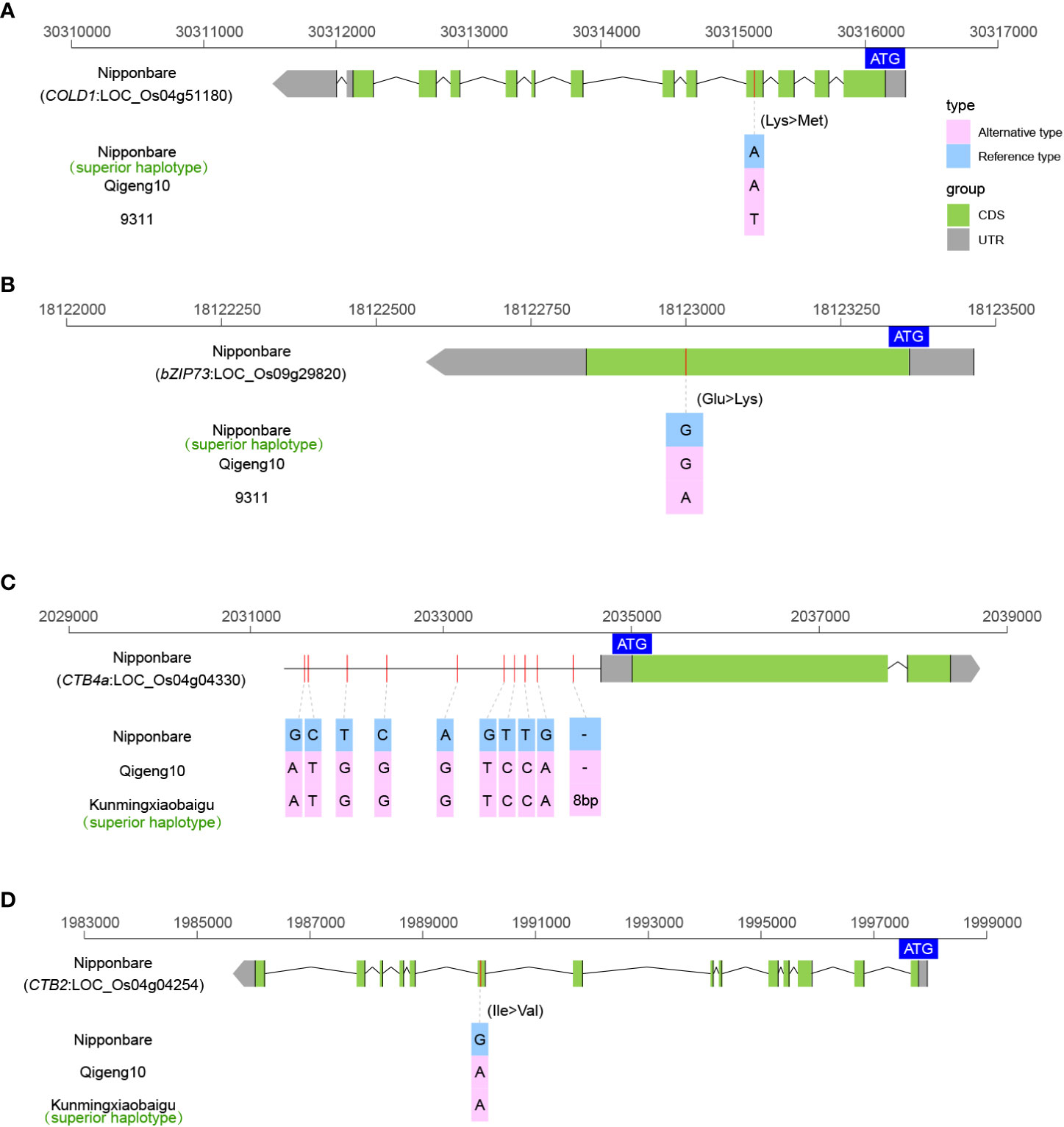
Figure 4 The allelic information of sequence variants in COLD1 (A), bZIP73 (B), CTB4a (C), and CTB2 (D) controlling cold tolerance in rice.
Sequence variants of the genes controlling early heading
The heading date is one of the most important factors determining rice distribution and the final yield. We investigated the sequence variations in 11 cloned genes related to early heading under long-day conditions, including DTH7 (Gao et al., 2014), Ghd7 (Xue et al., 2008), Ghd8 (Yan et al., 2011), Ehd1 (Doi et al., 2004), Ehd3 (Matsubara et al., 2011), Ehd4 (Gao et al., 2013), Hd1 (Yano et al., 2000), Hd3a (Kojima et al., 2002), Hd6 (Takahashi et al., 2001), Hd16 (Hori et al., 2013), and Hd17 (Matsubara et al., 2012). Among these heading date genes, only the DTH7, Ghd7, and Hd1 haplotypes were found to have non-functional alleles. The DTH7 allele in QG10 was found to have the three mutations as Kitaake, which originated at the northern limit of rice cultivation in Hokkaido, Japan (Figure 5A). Kitaake is reported insensitive to day length, short in stature, and completes its life cycle in about 9 weeks (Jain et al., 2019). The Ghd7 allele in QG10 was found having the critical mutations as Hejiang19, which is an early-maturity rice variety in Heilongjiang Province (Figure 5B). The Hd1 allele in QG10 was found to have the non-functional allele as Longgeng31, which is the major plant rice variety in Heilongjiang Province (Figure 5C). These results indicated that the heading gene combinations of Hd1, DTH7, and Ghd7 determined the early heading in QG10 in the northernmost province of China.
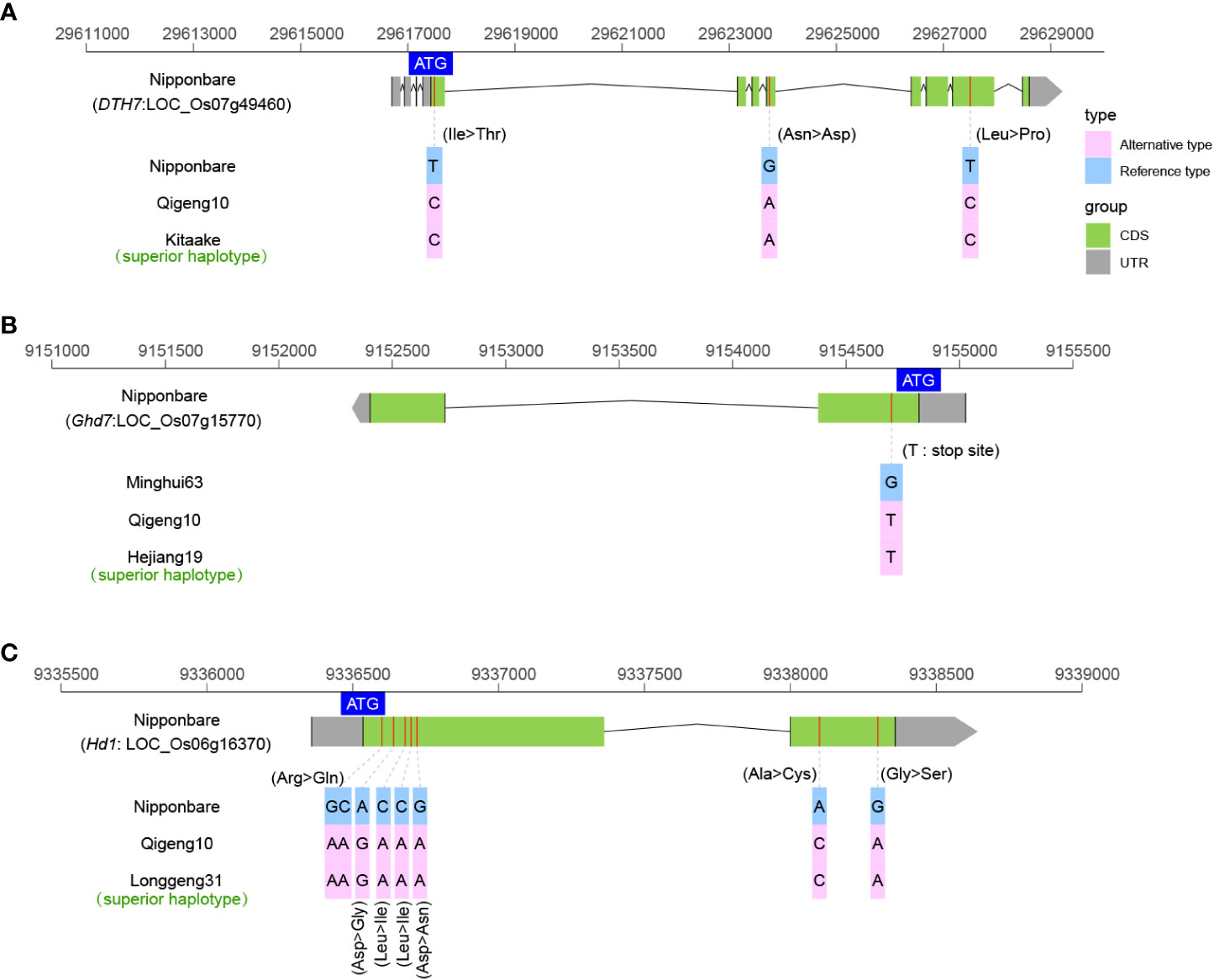
Figure 5 The allelic information of sequence variants in DTH7 (A), Ghd7 (B), and Hd1 (C) controlling heading date in rice.
Sequence variants of the genes controlling disease resistance
Blast is one of the most devastating rice diseases in Heilongjiang Province. We investigated the sequence variations in 14 cloned rice blast-resistant genes, including Pi5 (Lee et al., 2009), Pi21 (Fukuoka et al., 2009), Pi36 (Liu et al., 2007), Pi37 (Lin et al., 2007), Pi54 (Sharma et al., 2010), Pi56 (Liu et al., 2013), Pia (Okuyama et al., 2011), Pish (Takahashi et al., 2010), Pit (Hayashi and Yoshida, 2009), Pita (Bryan et al., 2000), Pigm (Deng et al., 2017), Pid2 (Chen et al., 2006), Pid3 (Shang et al., 2009), and Pid4 (Chen et al., 2018), to explain the blast resistance. Finally, only two blast resistance genes, Pia and Pid4, were found in QG10. The Pia allele in QG10 was found to have the resistant genotype as Akihikari, which encodes a nucleotide-binding site (NBS) and a C-terminal leucine-rich repeat (LRR) domain protein (Figure 6A). The Pid4 allele in QG10 was found to have the resistant genotype as Digu, which encodes a coiled-coil nucleotide-binding site leucine-rich repeat (CC-NBS-LRR) protein (Figure 6B). The Pia (Akihikari type) was the major blast-resistant gene in Northeast China. The Pid4 (Digu type) was a rare allele in japonica rice and has important application value in Northeast japonica rice breeding.
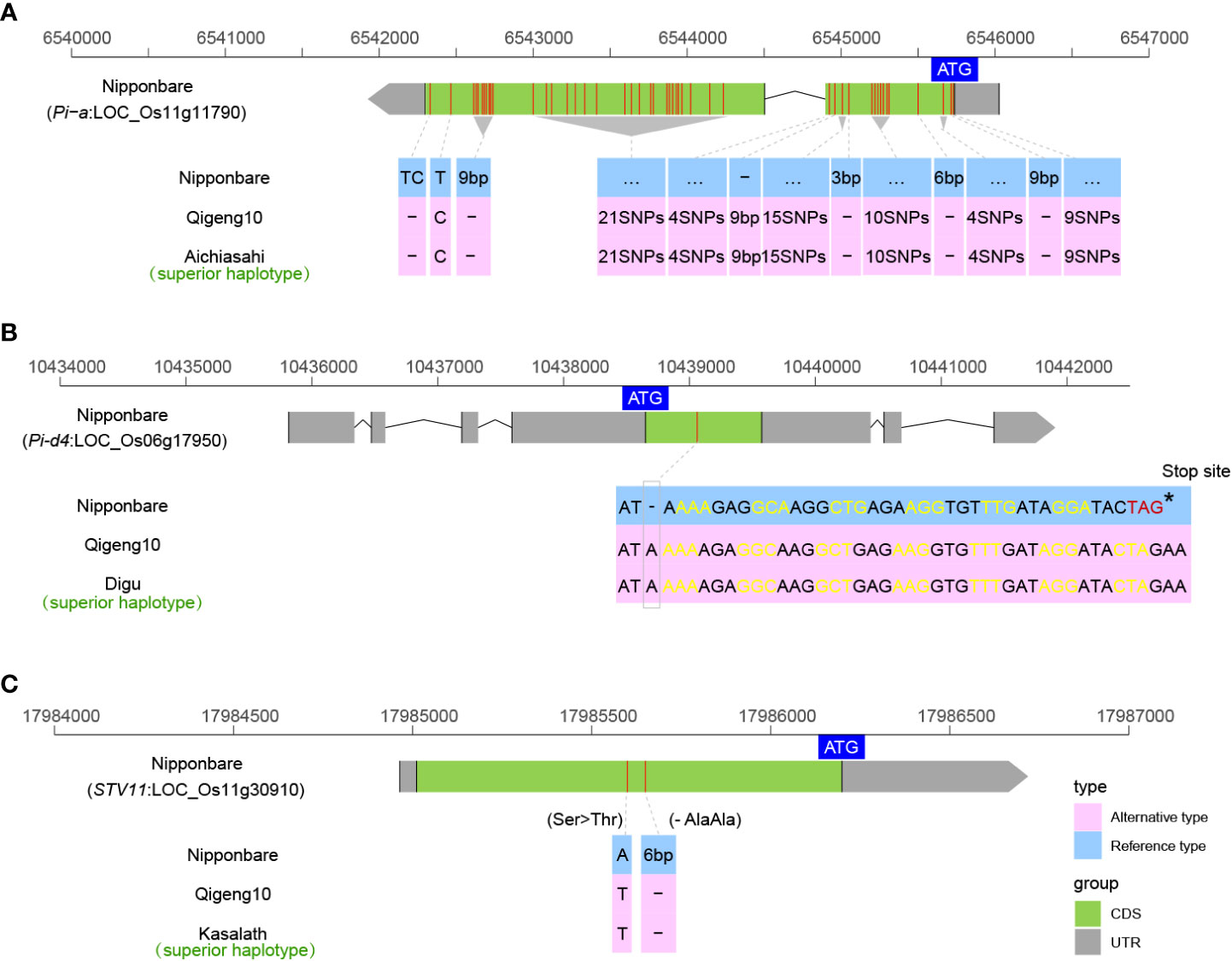
Figure 6 The allelic information of sequence variants in Pia (A), Pid4 (B), and STV11 (C) controlling disease resistance in rice.
Rice stripe virus (RSV), an RNA virus belonging to the genus Tenuivirus and transmitted by small brown planthoppers, causes one of the most destructive rice diseases (Wang et al., 2014). RSV has become more and more serious in Heilongjiang province in recent years. But, almost the majority of japonica varieties cultivated in Heilongjiang are highly susceptible to RSV (Wang et al., 2014). The STV11 was the first cloned resistant gene of RSV, which encodes a sulfotransferase (OsSOT1) catalyzing the conversion of salicylic acid (SA) into sulphonated SA (SSA) (Wang et al., 2014). The STV11 allele in QG10 was found to have the resistant genotype as Kasalath, which is a high-resistance indica landrace (Figure 6C). The STV11QG10 was a useful resistant gene in japonica rice breeding.
Sequence variants of fragrance, fertilizer use efficiency and other yield related genes
Fragrant rice is popular among consumers worldwide because its market price is much higher than that of nonfragrant rice. Fragrant was found to be controlled by BADH2 in rice (Chen et al., 2008). The BADH2 in QG10 was the typical badh2-E2 type of a 7-bp deletion as DHX2 that caused fragrance (Figure 7A). We also investigated the sequence variations in three cloned grain number genes (Gn1a (Ashikari et al., 2005), GNP1 (Wu et al., 2016), and SPIKE (Fujita et al., 2013)), and three lodging-resistance genes (Sd1 (Spielmeyer et al., 2002), SCM2 (Ookawa et al., 2010), and SCM3 (Yano et al., 2014)). Only the SCM3 allele in QG10 was found to have the key allele as Chugoku117, which is a stronger culms rice variety in Japan (Figure 7B). There was no elite grain number gene found in QG10. NRT1.1B is a key gene controlling the nitrogen-use efficiency (NUE) in rice (Hu et al., 2015). The NRT1.1B allele in QG10 was found to have the indica variation, which has higher nitrate absorption activity (Figure 7C).
Discussion
Indica genome introgression and large SVs were found in Qigeng10
Japonica/Geng and Indica/Xian are the two major subspecies of Asian cultivated rice (Zhang et al., 2016a). Owing to long-term differentiation and adaptation, both Indica and Japonica rice contain many favorable genes. Therefore, combining the favorable genes of the two subspecies has great value for creating genotypes with greater yield potential, stronger stress resistance, and better quality (Gu, 2010). Over the past 50 years, the combination of plant ideotypes and favorable vigor through hybridization between indica and japonica rice has greatly contributed to yield improvements in modern japonica rice in Northeast China (Tang and Chen, 2021). In recent years, a series of high-yielding and good-quality japonica cultivars have been obtained from hybridization of Indica/Japonica and the cultivation area of them was more than 4 million ha in Northeast China (Cui et al., 2022). The new fragrant early japonica rice cultivar QG10 was derived from a cross between ‘Wuyoudao4 and Suigeng4’, which were all derived from the hybridization of Indica/Japonica. In recently, Wang et al. (2023) chose six interrelated modern Chinese temperate japonica varieties and six related Japanese japonica varieties to investigate genome enhancement in temperate japonica varieties during modern breeding. They found many large SVs in Zhonghua11 (ZH11), Liaogeng5 (LG5), and Daohuaxiang2 (DHX2/WYD4). These large-fragment in the same location introgression from indica were also found in QG10 on chromosomes 4, 8, and 10 (Figure 2B). Several indica superior alleles including qTGW3Nanyangzhan, GW5IR24, GW6Suyunuo, qGW8Basmati385, Pid4Digu, STV11Kasalath, NRT1.1BIR24, and badh2-E2 were also be found in QG10. This information indicated that the indica genome introgression was common in the modern temperate japonica rice breeding in Northeast China. Superior alleles were found in Qigeng10 and had important value for breeding in Northeast China
Superior alleles were found in Qigeng10 and had important value for breeding in Northeast China
Greater yield potential, stronger stress resistance, and better quality (longer grain and fragrant) are key agronomy traits that directly influence the market price of rice. Consumers in East Asia, including North China, Japan, and Korea tend to prefer longer fragrant japonica rice (Lu et al., 2022). So, the longer fragrant japonica rice from Northeast China, represented by Daohuaxiang2 (DHX2/WYD4), is the most famous rice in the Chinese market. QG10 was derived from DHX2 and solved some defects of DHX2 including poor lodging resistance, lack of cold tolerance, weak blast resistance, and late maturity. Therefore, the construction of a high-quality genome of ‘QG10’ is essential for further improvement of this cultivar or its progenies, as well as accelerating the process of fragrant japonica rice breeding, by providing genomic resources that could be directly applied to fragrant japonica rice cultivars. In this study, we found five superior alleles (qTGW2Nipponbare, qTGW3Nanyangzhan, GW5IR24, GW6Suyunuo, and qGW8Basmati385) controlling long grain size in QG10. To compare the phenotype of different gene haplotypes in rice germplasm, we investigated the grain shape traits and days to heading of 3k cultivars in the website (https://www.rmbreeding.cn/)(Wang et al., 2020). The results showed that the functional haplotype of QG10 controlling longer and slider grain (Figures 8A-E) and early heading (Figure 8f-h). Most of them was belong to the rare alleles for controlling longer grain and were less application in rice breeding in Northeast China. The blast resistant alleles (Pid4Digu), RSV allele (STV11Kasalath), and NUE allele (NRT1.1BIR24) were also belong to the rare alleles for rice breeding in Northeast China. In the future, we will develop molecular assisted markers for the improvement of japonica rice varieties in Northeast China, and expanded the gene pool of japonica rice in Northeast China.
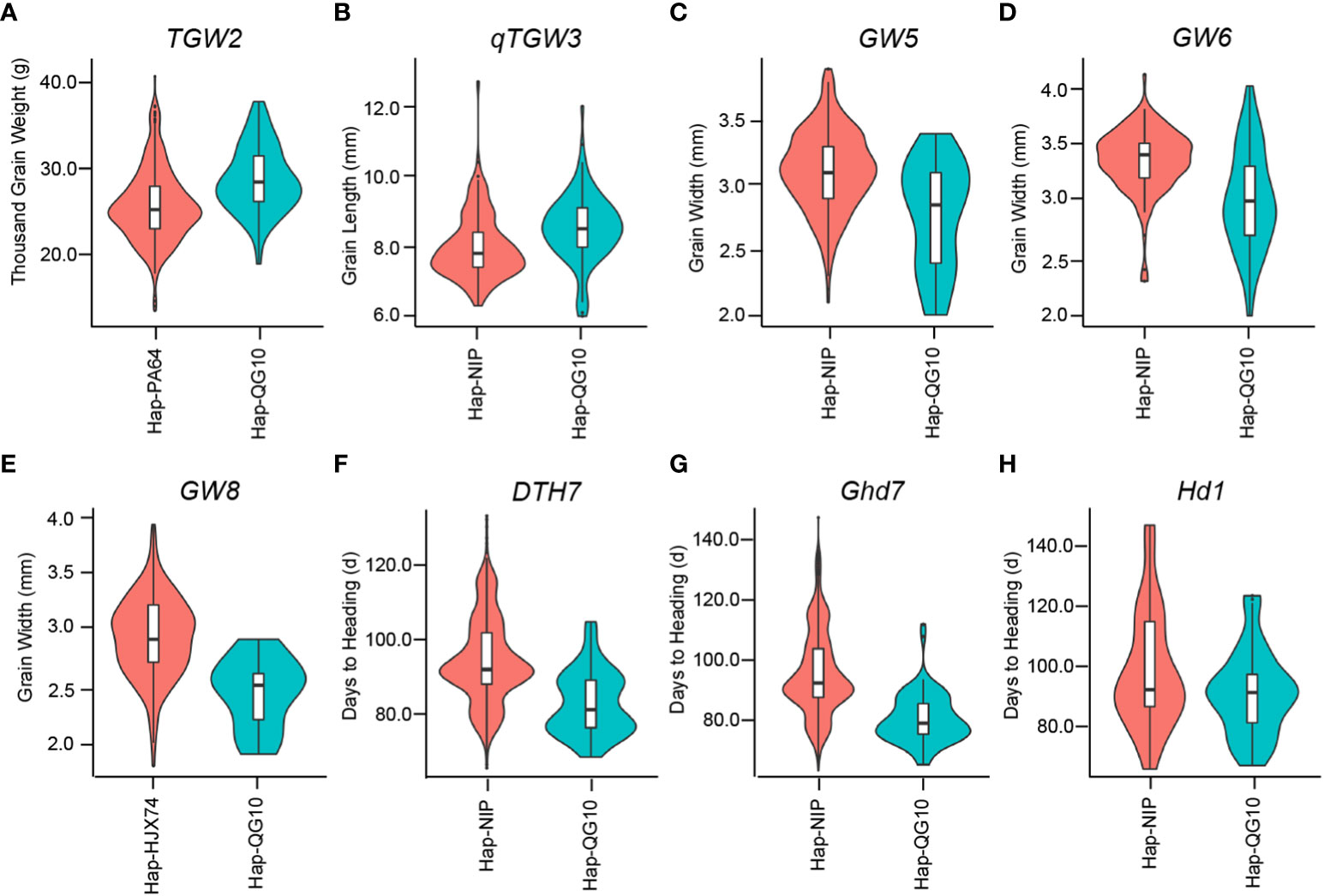
Figure 8 Comparison of grain shape traits and days to heading between different gene haplotypes in 3k panel.
Conclusions
In this study, we present chromosome-level genome assembly of an early-matured aromatic long-grain japonica rice variety Qigeng10 by using a combination of Nanopore and Hi-C platforms. The total assembly size is 378.31Mb with an N50 length of 30.55 Mb. A total of 18 superior haplotypes including five long-grain alleles (qTGW2Nipponbare, qTGW3Nanyangzhan, GW5IR24, GW6Suyunuo, and qGW8Basmati385), four cold tolerant alleles (COLD1Nipponbare, bZIP73Nipponbare, CTB4aKunmingxiaobaigu, and CTB2Kunmingxiaobaigu), three non-functional heading date alleles (DTH7Kitaake, Ghd7Hejiang19, and Hd1Longgeng31), two blast resistant alleles (Pia Akihikari and Pid4Digu), a rice stripe virus resistant allele STV11Kasalath, a higher nitrate absorption allele NRT1.1BIR24, a lodging resistant allele SCM3Chugoku117, and the typical aromatic allele badh2-E2, were identified in QG10. This information will accelerate the process of fragrant japonica rice breeding in Northeast China, by providing genomic resources that could be directly applied to fragrant japonica rice cultivars or development of molecular assisted markers for the improvement of japonica rice varieties.
Data availability statement
The data presented in the study are deposited in the Data Center of Beijing Institute of Genomics(Big) repository, accession number WGS029943 (PRJCA013131; SAMC988458).
Author contributions
Conceptualization: SJ and JW. Methodology: SJ. Software: XZ, XY, BM. Validation: SJ, HJ and YW. Formal analysis: JH and KT. Investigation: CL and LW. Resources: JW. Data curation: SJ. Writing—original draft preparation: SJ. Writing—review and editing: SJ. Visualization: SJ. Project administration: SJ. Funding acquisition: SJ and XZ. All authors contributed to the article and approved the submitted version.
Funding
This research was funded by Fundamental Research Funds for the Research Institutes of Heilongjiang Province, Grant/Award Number: CZKYF2021B009, CZKYF2022-1-B004, National Natural Science Foundation of China, Grant/Award Number: 32071889, Key Research and Development Program of Heilongjiang Province, Grant/Award Number: CZ20210090, and Heilongjiang Province Agricultural Science and Technology Innovation Project, Grant/Award Number: 2021QKPY009, 2021CQJC003.
Conflict of interest
The authors declare that the research was conducted in the absence of any commercial or financial relationships that could be construed as a potential conflict of interest.
Publisher’s note
All claims expressed in this article are solely those of the authors and do not necessarily represent those of their affiliated organizations, or those of the publisher, the editors and the reviewers. Any product that may be evaluated in this article, or claim that may be made by its manufacturer, is not guaranteed or endorsed by the publisher.
References
Alioto, T., Blanco, E., Parra, G., Guigó, R. (2018). Using geneid to identify genes. Curr. Protoc. Bioinf. 64, e56. doi: 10.1002/cpbi.56
Altschul, S., Gish, W., Miller, W., Myers, E., Lipman, D. (1990). Basic local alignment search tool. J. Mol. Biol. 215, 403–410. doi: 10.1016/S0022-2836(05)80360-2
Ashikari, M., Sakakibara, H., Lin, S., Yamamoto, T., Takashi, T., Nishimura, A., et al. (2005). Cytokinin oxidase regulates rice grain production. Science 309, 741–745. doi: 10.1126/science.1113373
Bryan, G., Wu, K., Farrall, L., Jia, Y., Hershey, H., Mcadams, S., et al. (2000). A single amino acid difference distinguishes resistant and susceptible alleles of the rice blast resistance gene pi-ta. Plant Cell 12, 2033–2046. doi: 10.2307/3871103
Burge, C., Karlin, S. (1997). Prediction of complete gene structures in human genomic DNA. J. Mol. Biol. 268, 78–94. doi: 10.1006/jmbi.1997.0951
Burton, J., Adey, A., Patwardhan, R., Qiu, R., Kitzman, J., Shendure, J. (2013). Chromosome-scale scaffolding of de novo genome assemblies based on chromatin interactions. Nat. Biotechnol. 31, 1119–1125. doi: 10.1038/nbt.2727
Campbell, M., Haas, B., Hamilton, J., Mount, S., Buell, C. (2006). Comprehensive analysis of alternative splicing in rice and comparative analyses with arabidopsis. BMC Genomics 7, 327. doi: 10.1186/1471-2164-7-327
Chen, X., Shang, J., Chen, D., Lei, C., Zou, Y., Zhai, W., et al. (2006). A b-lectin receptor kinase gene conferring rice blast resistance. Plant J. 46, 794–804. doi: 10.1111/j.1365-313X.2006.02739.x
Chen, S., Yang, Y., Shi, W., Ji, Q., He, F., Zhang, Z., et al. (2008). Badh2, encoding betaine aldehyde dehydrogenase, inhibits the biosynthesis of 2-acetyl-1-pyrroline, a major component in rice fragrance. Plant Cell 20, 1850–1861. doi: 10.1105/tpc.108.058917
Chen, Z., Zhao, W., Zhu, X., Zou, C., Yin, J., Chern, M., et al. (2018). Identification and characterization of rice blast resistance gene Pid4 by a combination of transcriptomic profiling and genome analysis. J. Genet. Genomics 45, 663–672. doi: 10.1016/j.jgg.2018.10.007
Choi, J., Lye, Z., Groen, S., Dai, X., Rughani, P., Zaaijer, S., et al. (2020). Nanopore sequencing-based genome assembly and evolutionary genomics of circum-basmati rice. Genome Biol. 21, 21. doi: 10.1186/s13059-020-1938-2
Cui, D., Zhou, H., Ma, X., Lin, Z., Sun, L., Han, B., et al. (2022). Genomic insights on the contribution of introgressions from Xian/Indica to the genetic improvement of Geng/Japonica rice cultivars. Plant Commun. 3, 100325. doi: 10.1016/j.xplc.2022.100325
Deng, Y., Zhai, K., Xie, Z., Yang, D., Zhu, X., Liu, J., et al. (2017). Epigenetic regulation of antagonistic receptors confers rice blast resistance with yield balance. Science 355, 962–965. doi: 10.1126/science.aai8898
Doi, K., Izawa, T., Fuse, T., Yamanouchi, U., Kubo, T., Shimatani, Z., et al. (2004). Ehd1, a b-type response regulator in rice, confers short-day promotion of flowering and controls FT-like gene expression independently of Hd1. Genes Dev. 18, 926–936. doi: 10.1101/gad.1189604
Du, H., Yu, Y., Ma, Y., Gao, Q., Cao, Y., Chen, Z., et al. (2017). Sequencing and de novo assembly of a near complete indica rice genome. Nat. Commun. 8, 15324. doi: 10.1038/ncomms15324
Fan, C., Xing, Y., Mao, H., Lu, T., Han, B., Xu, C., et al. (2006). GS3, a major QTL for grain length and weight and minor QTL for grain width and thickness in rice, encodes a putative transmembrane protein. Theor. Appl. Genet. 112, 1164–1171. doi: 10.1007/s00122-006-0218-1
Fujino, K., Sekiguchi, H., Matsuda, Y., Sugimoto, K., Ono, K., Yano, M. (2008). Molecular identification of a major quantitative trait locus, qLTG3-1, controlling low-temperature germinability in rice. Proc. Natl. Acad. Sci. U.S.A. 105, 12623–12628. doi: 10.1073/pnas.0805303105
Fujita, D., Trijatmiko, K., Tagle, A., Sapasap, M., Koide, Y., Sasaki, K., et al. (2013). NAL1 allele from a rice landrace greatly increases yield in modern indica cultivars. Proc. Natl. Acad. Sci. U.S.A. 110, 20431–20436. doi: 10.1073/pnas.1310790110
Fukuoka, S., Saka, N., Koga, H., Ono, K., Shimizu, T., Ebana, K., et al. (2009). Loss of function of a proline-containing protein confers durable disease resistance in rice. Aug 21. Science 325, 998–1001. doi: 10.1126/science.1175550
Gao, H., Jin, M., Zheng, X., Chen, J., Yuan, D., Xin, Y., et al. (2014). Days to heading 7, a major quantitative locus determining photoperiod sensitivity and regional adaptation in rice. Proc. Natl. Acad. Sci. U.S.A. 111, 16337–16342. doi: 10.1073/pnas.1418204111
Gao, H., Zhao, B., Zhang, J., Song, L., Wu, H., Yu, Y., et al. (2012). High-quality and high-yield cultivation techniques of a new high-quality rice variety wuyoudao no. 4. Heilongjiang Agric. Sci. 154-155.
Gao, H., Zheng, X., Fei, G., Chen, J., Jin, M., Ren, Y., et al. (2013). Ehd4 encodes a novel and oryza-genus-specific regulator of photoperiodic flowering in rice. PloS Genet. 9, e1003281. doi: 10.1371/journal.pgen.1003281
Goff, S., Ricke, D., Lan, T., Presting, G., Wang, R., Dunn, M., et al. (2002). A draft sequence of the rice genome (Oryza sativa l. ssp. japonica). Science 296, 92–100. doi: 10.1126/science.1068275
Gross, B., Zhao, Z. (2014). Archaeological and genetic insights into the origins of domesticated rice. Proc. Natl. Acad. Sci. U.S.A. 111, 6190–6197. doi: 10.1073/pnas.1308942110
Gu, H. (2010). Discussion on the aspects of high-yielding breeding in rice. Acta Agronomica Sin. 36, 1431–1439.
Gu, H., Liang, S., Zhao, J. (2022). Novel sequencing and genomic technologies revolutionized rice genomic study and breeding. Agronomy 12, 218. doi: 10.3390/agronomy12010218
Haas, B., Salzberg, S., Zhu, W., Pertea, M., Allen, J., Orvis, J., et al. (2008). Automated eukaryotic gene structure annotation using EVidenceModeler and the program to assemble spliced alignments. Genome Biol. 9, R7. doi: 10.1186/gb-2008-9-1-r7
Hayashi, K., Yoshida, H. (2009). Refunctionalization of the ancient rice blast disease resistance gene pit by the recruitment of a retrotransposon as a promoter. Plant J. 57, 413–425. doi: 10.1111/j.1365-313X.2008.03694.x
Hoede, C., Arnoux, S., Moisset, M., Chaumier, T., Inizan, O., Jamilloux, V., et al. (2014). PASTEC: an automatic transposable element classification tool. PloS One 9, e91929. doi: 10.1371/journal.pone.0091929
Hori, K., Ogiso-Tanaka, E., Matsubara, K., Yamanouchi, U., Ebana, K., Yano, M. (2013). Hd16, a gene for casein kinase I, is involved in the control of rice flowering time by modulating the day-length response. Plant J. 76, 36–46. doi: 10.1111/tpj.12268
Hu, Z., Lu, S., Wang, M., He, H., Sun, L., Wang, H., et al. (2018). A novel QTL qTGW3 encodes the GSK3/SHAGGY-like kinase OsGSK5/OsSK41 that interacts with OsARF4 to negatively regulate grain size and weight in rice. Mol. Plant 11, 736–749. doi: 10.1016/j.molp.2018.03.005
Hu, J., Wang, Y., Fang, Y., Zeng, L., Xu, J., Yu, H., et al. (2015). A rare allele of GS2 enhances grain size and grain yield in rice. Mol. Plant 8, 1455–1465. doi: 10.1016/j.molp.2015.07.002
Hu, B., Wang, W., Ou, S., Tang, J., Li, H., Che, R., et al. (2015). Variation in NRT1.1B contributes to nitrate-use divergence between rice subspecies. Nat. Genet. 47, 834–838. doi: 10.1038/ng.3337
Hui, S., Li, H., Mawia, A., Zhou, L., Cai, J., Ahmad, S., et al. (2022). Production of aromatic three-line hybrid rice using novel alleles of BADH2. Plant Biotechnol. J. 20, 59–74. doi: 10.1111/pbi.13695
International-Rice-Genome-Sequencing-Project (2005). The map-based sequence of the rice genome. Nature 436, 793–800. doi: 10.1038/nature03895
Ishimaru, K., Hirotsu, N., Madoka, Y., Murakami, N., Hara, N., Onodera, H., et al. (2013). Loss of function of the IAA-glucose hydrolase gene TGW6 enhances rice grain weight and increases yield. Nat. Genet. 45, 707–711. doi: 10.1038/ng.2612
Jain, R., Jenkins, J., Shu, S., Chern, M., Martin, J., Copetti, D., et al. (2019). Genome sequence of the model rice variety KitaakeX. BMC Genomics 20, 905. doi: 10.1186/s12864-019-6262-4
Jiang, S., An, H., Xu, F., Zhang, X. (2020). Chromosome-level genome assembly and annotation of the loquat (Eriobotrya japonica) genome. Gigascience 9, giaa015. doi: 10.1093/gigascience/giaa015
Jurka, J., Kapitonov, V., Pavlicek, A., Klonowski, P., Kohany, O., Walichiewicz, J. (2005). Repbase update, a database of eukaryotic repetitive elements. Cytogenetic Genome Res. 110, 462–467. doi: 10.1159/000084979
Keilwagen, J., Hartung, F., Paulini, M., Twardziok, S., Grau, J. (2018). Combining RNA-seq data and homology-based gene prediction for plants, animals and fungi. BMC Bioinf. 19, 189. doi: 10.1186/s12859-018-2203-5
Keilwagen, J., Wenk, M., Erickson, J., Schattat, M., Jan, G., Frank, H. (2016). Using intron position Conserv. homology-based Gene prediction. Nucleic Acids Res. 44, e89. doi: 10.1093/nar/gkw092
Kim, D., Langmead, B., Salzberg, S. (2015). HISAT: a fast spliced aligner with low memory requirements. Nat. Methods 12, 357–360. doi: 10.1038/nmeth.3317
Kojima, S., Takahashi, Y., Kobayashi, Y., Monna, L., Sasaki, T., Araki, T., et al. (2002). Hd3a, a rice ortholog of the arabidopsis FT gene, promotes transition to flowering downstream of Hd1 under short-day conditions. Plant Cell Physiol. 43, 1096–1105. doi: 10.1093/pcp/pcf156
Koren, S., Walenz, B., Berlin, K., Miller, J., Bergman, N., Phillippy, A. (2017). Canu: scalable and accurate long-read assembly via adaptive k-mer weighting and repeat separation. Genome Res. 27, 722–736. doi: 10.1101/gr.215087.116
Kurtz, S., Phillippy, A., Delcher, A., Smoot, M., Shumway, M., Antonescu, C., et al. (2004). Versatile and open software for comparing large genomes. Genome Biol. 5, R12. doi: 10.1186/gb-2004-5-2-r12
Lee, S. K., Song, M. Y., Seo, Y. S., Kim, H. K., KO., S., Cao, P. J., et al. (2009). Rice Pi5-mediated resistance to magnaporthe oryzae requires the presence of two coiled-coil-nucleotide-binding-leucine-rich repeat genes. Genetics 181, 1627–1638. doi: 10.1534/genetics.108.099226
Li, H., Durbin, R. (2009). Fast and accurate short read alignment with burrows-wheeler transform. Bioinformatics 25, 1754–1760. doi: 10.1093/bioinformatics/btp324
Li, Y., Fan, C., Xing, Y., Jiang, Y., Luo, L., Sun, L., et al. (2011). Natural variation in GS5 plays an important role in regulating grain size and yield in rice. Nat. Genet. 43, 1266–1269. doi: 10.1038/ng.977
Li, F., Gao, Y., Wu, B., Cai, Q., Zhan, P., Yang, W., et al. (2021a). High-quality de novo genome assembly of huajingxian 74, a receptor parent of single segment substitution lines. Rice Sci. 28, 109–113. doi: 10.1016/j.rsci.2020.09.010
Li, G., Jain, R., Chern, M., Pham, N., Martin, J., Wei, T., et al. (2017). The sequences of 1504 mutants in the model rice variety kitaake facilitate rapid functional genomic studies. Plant Cell Physiol. 29, 1218–1231. doi: 10.1105/tpc.17.00154
Li, X., Wu, L., Wang, J., Sun, J., Xia, X., Geng, X., et al. (2018). Genome sequencing of rice subspecies and genetic analysis of recombinant lines reveals regional yield- and quality-associated loci. BMC Biol. 16, 102. doi: 10.1186/s12915-018-0572-x
Li, J., Zeng, Y., Pan, Y., Zhou, L., Zhang, Z., Guo, H., et al. (2021b). Stepwise selection of natural variations at CTB2 and CTB4a improves cold adaptation during domestication of japonica rice. New Phytol. 231, 1056–1072. doi: 10.1111/nph.17407
Lin, F., Chen, S., Que, Z., Wang, L., Liu, X., Pan, Q. (2007). The blast resistance gene Pi37 encodes a nucleotide binding site leucine-rich repeat protein and is a member of a resistance gene cluster on rice chromosome 1. Genetics 177, 1871–1880. doi: 10.1534/genetics.107.080648
Lin, B., Hui, J., Mao, H. (2021). Nanopore technology and its applications in gene sequencing. Biosensors 11, 214. doi: 10.3390/bios11070214
Liu, X., Lin, F., Wang, L., Pan, Q. (2007). The in silico map-based cloning of Pi36, a rice coiled-coil nucleotide-binding site leucine-rich repeat gene that confers race-specific resistance to the blast fungus. Genetics 176, 2541–2549. doi: 10.1534/genetics.107.075465
Liu, Y., Liu, B., Zhu, X., Yang, J., Bordeos, A., Wang, G., et al. (2013). Fine-mapping and molecular marker development for Pi56(t), a NBS-LRR gene conferring broad-spectrum resistance to magnaporthe oryzae in rice. Theor. Appl. Genet. 126, 985–998. doi: 10.1007/s00122-012-2031-3
Liu, C., Ou, S., Mao, B., Tang, J., Wang, W., Wang, H., et al. (2018). Early selection of bZIP73 facilitated adaptation of japonica rice to cold climates. Nat. Commun. 9, 3302. doi: 10.1038/s41467-018-05753-w
Liu, L., Tong, H., Xiao, Y., Che, R., Xu, F., Hu, B., et al. (2015). Activation of big Grain1 significantly improves grain size by regulating auxin transport in rice. Proc. Natl. Acad. Sci. U.S.A. 112, 11102–11107. doi: 10.1073/pnas.1512748112
Lu R, L. J., Wang, X., Song, Z., Ji, X., Li, N., Ma, G., et al. (2022). Chromosome-level genome assembly of a fragrant japonica rice cultivar ‘Changxianggeng 1813’ provides insights into genomic variations between fragrant and non-fragrant japonica rice. Int. J. Mol. Sci. 23, 9705. doi: 10.3390/ijms23179705
Ma, Y., Dai, X., Xu, Y., Luo, W., Zheng, X., Zeng, D., et al. (2015). COLD1 confers chilling tolerance in rice. Cell 160, 1209–1221. doi: 10.1016/j.cell.2015.01.046
Mahesh, H., Shirke, M., Singh, S., Rajamani, A., Hittalmani, S., Wang, G., et al. (2016). Indica rice genome assembly, annotation and mining of blast disease resistance genes. BMC Genomics 242. doi: 10.1186/s12864-016-2523-7
Majoros, W., Pertea, M., Salzberg, S. (2004). TigrScan and GlimmerHMM: Two open source ab initio eukaryotic gene-finders. Bioinformatics 20, 2878–2879. doi: 10.1093/bioinformatics/bth315
Mao, D., Xin, Y., Tan, Y., Hu, X., Bai, J., Liu, Z., et al. (2019). Natural variation in the HAN1 gene confers chilling tolerance in rice and allowed adaptation to a temperate climate. Proc. Natl. Acad. Sci. U.S.A. 116, 3494–3501. doi: 10.1073/pnas.1819769116
Matsubara, K., Ogiso-Tanaka, E., Hori, K., Ebana, K., Ando, T., Yano, M. (2012). Natural variation in Hd17, a homolog of arabidopsis ELF3 that is involved in rice photoperiodic flowering. Plant Cell Physiol. 53, 709–716. doi: 10.1093/pcp/pcs028
Matsubara, K., Yamanouchi, U., Nonoue, Y., Sugimoto, K., Wang, Z., Minobe, Y., et al. (2011). Ehd3, encoding a plant homeodomain finger-containing protein, is a critical promoter of rice flowering. Plant J. 66, 603–612. doi: 10.1111/j.1365-313X.2011.04517.x
Nie, S., Liu, Y., Wang, C., Gao, S., Xu, T., Liu, Q., et al. (2017). Assembly of an early-matured japonica (Geng) rice genome, Suijing18, ased on PacBio and illumina sequencing. Sci. Data 170195. doi: 10.1038/sdata.2017.195
Okuyama, Y., Kanzaki, H., Abe, A., Yoshida, K., Tamiru, M., Saitoh, H., et al. (2011). A multifaceted genomics approach allows the isolation of the rice pia-blast resistance gene consisting of two adjacent NBS-LRR protein genes. Plant J. 66, 467–479. doi: 10.1111/j.1365-313X.2011.04502.x
Ookawa, T., Hobo, T., Yano, M., Murata, K., Ando, T., Miura, H., et al. (2010). New approach for rice improvement using a pleiotropic QTL gene for lodging resistance and yield. Nat. Commun. 1, 132. doi: 10.1038/ncomms1132
Panibe, J., Wang, L., Li, J., Li, M., Lee, Y., Wang, C., et al. (2021). Chromosomal-level genome assembly of the semi-dwarf rice taichung native 1, an initiator of green revolution. Genomics 113, 2656–2674. doi: 10.1016/j.ygeno.2021.06.006
Pertea, M., Pertea, G. M., Antonescu, C. M., Chang, T. C., Mendell, J. T., Salzberg, Sl (2015). StringTie enables improved reconstruction of a transcriptome from RNA-seq reads. Nat. Biotechnol. 33, 290–295. doi: 10.1038/nbt.3122
Price, A., Jones, N., Pa., P. (2005). De novo identification of repeat families in large genomes. Bioinformatics 21, i351–i358. doi: 10.1093/bioinformatics/bti1018
Qin, P., Lu, H., Du, H., Wang, H., Chen, W., Chen, Z., et al. (2021). Pan-genome analysis of 33 genetically diverse rice accessions reveals hidden genomic variations. Cell 184, 3542–3558. doi: 10.1016/j.cell.2021.04.046
Ruan, B., Shang, L., Zhang, B., Hu, J., Wang, Y., Lin, H., et al. (2020). Natural variation in the promoter of TGW2 determines grain width and weight in rice. New Phytol. 227, 629–640. doi: 10.1111/nph.16540
Saito, K., Hayano-Saito, Y., Kuroki, M., Sato, Y. (2010). Map-based cloning of the rice cold tolerance gene Ctb1. Plant Sci. 179, 97–102. doi: 10.1016/j.plantsci.2010.04.004
Servant, N., Varoquaux, N., Lajoie, B., Viara, E., Chen, C., Vert, J., et al. (2015). HiC-pro: An optimized and flexible pipeline for Hi-c data processing. Genome Biol. 16, 259. doi: 10.1186/s13059-015-0831-x
Shang, L., Li, X., He, H., Yuan, Q., Song, Y., Wei, Z., et al. (2022). A super pan-genomic landscape of rice. Cell Res. 32, 878–896. doi: 10.1038/s41422-022-00685-z
Shang, J., Tao, Y., Chen, X., Zou, Y., Lei, C., Wang, J., et al. (2009). Identification of a new rice blast resistance gene, Pid3, by genomewide comparison of paired nucleotide-binding site–leucine-rich repeat genes and their pseudogene alleles between the two sequenced rice genomes. Genetics 182, 1303–1311. doi: 10.1534/genetics.109.102871
Sharma, T., Rai, A., Gupta, S., Singh, N. (2010). Broad-spectrum blast resistance gene pi-kh cloned from rice line tetep designated as Pi54. J. Plant Biochem. Biotechnol. 19, 87–89. doi: 10.1007/BF03323441
Shi, C., Dong, N., Guo, T., Ye, W., Shan, J., Lin, H. (2020). A quantitative trait locus GW6 controls rice grain size and yield through the gibberellin pathway. Plant J. 103, 1174–1188. doi: 10.1111/tpj.14793
Si, L., Chen, J., Huang, X., Gong, H., Luo, J., Hou, Q., et al. (2016). OsSPL13 controls grain size in cultivated rice. Nat. Genet. 48, 447–456. doi: 10.1038/ng.3518
Simão, F., Waterhouse, R., Ioannidis, P., Kriventseva, E., Zdobnov, E. (2015). BUSCO: assessing genome assembly and annotation completeness with single-copy orthologs. Bioinformatics 31, 3210–3212. doi: 10.1093/bioinformatics/btv351
Song, X., Huang, W., Shi, M., Zhu, M., Lin, H. (2007). A QTL for rice grain width and weight encodes a previously unknown RING-type E3 ubiquitin ligase. Nat. Genet. 39, 623–630. doi: 10.1038/ng2014
Song, X., Kuroha, T., Ayano, M., Furuta, T., Nagai, K., Komeda, N., et al. (2015). Rare allele of a previously unidentified histone H4 acetyltransferase enhances grain weight, yield, and plant biomass in rice. Proc. Natl. Acad. Sci. U.S.A. 112, 76–81. doi: 10.1073/pnas.1421127112
Spielmeyer, W., Ellis, M., Chandler, P. (2002). Semidwarf (sd-1), “green revolution” rice, contains a defective gibberellin 20-oxidase gene. Proc. Natl. Acad. Sci. U.S.A. 99, 9043–9048. doi: 10.1073/pnas.132266399
Stanke, M., Waack, S. (2003). Gene prediction with a hidden Markov model and a new intron submodel. Bioinformatics 19, ii215–ii225. doi: 10.1093/bioinformatics/btg1080
Stein, J., Yu, Y., Copetti, D., Zwickl, D., Zhang, L., Zhang, C., et al. (2018). Genomes of 13 domesticated and wild rice relatives highlight genetic conservation, turnover and innovation across the genus oryza. Nat. Genet. 50, 285–296. doi: 10.1038/s41588-018-0040-0
Sun, L., Li, X., Fu, Y., Zhu, Z., Tan, L., Liu, F., et al. (2013). GS6, a member of the GRAS gene family, negatively regulates grain size in rice. J. Integr. Plant Biol. 55, 938–949. doi: 10.1111/jipb.12062
Takahashi, A., Hayashi, N., Miyao, A., Hirochika, H. (2010). Unique features of the rice blast resistance pish locus revealed by large scale retrotransposon-tagging. BMC Plant Biol. 10, 175. doi: 10.1186/1471-2229-10-175
Takahashi, Y., Shomura, A., Sasaki, T., Yano, M. (2001). Hd6, a rice quantitative trait locus involved in photoperiod sensitivity, encodes the alpha subunit of protein kinase CK2. Proc. Natl. Acad. Sci. U.S.A. 98, 7922–7927. doi: 10.1073/pnas.111136798
Tanaka, T., Nishijima, R., Teramoto, S., Kitomi, Y., Hayashi, T., Uga, Y., et al. (2020). De novo genome assembly of the indica rice variety IR64 using linked-read sequencing and nanopore sequencing. G3 (Bethesda) 10, 1495–1501. doi: 10.1534/g3.119.400871
Tang, L., Chen, W. (2021). Development trend and prospect of geng rice in northeast China. China Rice, 28(5), 1–4.
Tang, S., Lomsadze, A., Borodovsky, M. (2015). Identification of protein coding regions in RNA transcripts. Nucleic Acids Res. 43, e78. doi: 10.1093/nar/gkv227
Tarailo-Graovac, M., Chen, N. (2009). Using RepeatMasker to identify repetitive elements in genomic sequences. Curr. Protoc. Bioinf. 4 (10), 11–14. doi: 10.1002/0471250953.bi0410s25
Thompson, J., Higgins, D., Gibson, T. (1994). CLUSTAL W: improving the sensitivity of progressive multiple sequence alignment through sequence weighting, position-specific gap penalties and weight matrix choice. Nucleic Acids Res. 22, 4673–4680. doi: 10.1093/nar/22.22.4673
Van Berkum, N., Lieberman-Aiden, E., Williams, L., Imakaev, M., Gnirke, A., Mirny, L. A., et al. (2010). Hi-C: A method to study the three-dimensional architecture of genomes. J. Vis. Exp. 39, 1869. doi: 10.3791/1869
Vaser, R., Sović, I., Nagarajan, N., Šikić, M. (2017). Fast and accurate de novo genome assembly from long uncorrected reads. Genome Res. 27, 737–746. doi: 10.1101/gr.214270.116
Walker, B., Abeel, T., Shea, T., Priest, M., Abouelliel, A., Sakthikumar, S., et al. (2014). Pilon: An integrated tool for comprehensive microbial variant detection and genome assembly improvement. PloS One 9, e112963. doi: 10.1371/journal.pone.0112963
Wang, A., Hou, Q., Si, L., Huang, X., Luo, J., Lu, D., et al. (2019). The PLATZ transcription factor GL6 affects grain length and number in rice. Plant Physiol. 180, 2077–2090. doi: 10.1104/pp.18.01574
Wang, S., Li, S., Liu, Q., Wu, K., Zhang, J., Wang, S., et al. (2015). The OsSPL16-GW7 regulatory module determines grain shape and simultaneously improves rice yield and grain quality. Nat. Genet. 47, 949–954. doi: 10.1038/ng.3352
Wang, Y., Li, F., Zhang, F., Wu, L., Xu, N., Sun, Q., et al. (2023). Time-ordering japonica/geng genomes analysis indicates the importance of large structural variants in rice breeding. Plant Biotechnol. J. 21, 202–218. doi: 10.1111/pbi.13938
Wang, Q., Liu, Y., He, J., Zheng, X., Hu, J., Liu, Y., et al. (2014). STV11 encodes a sulphotransferase and confers durable resistance to rice stripe virus. Nat. Commun. 5. doi: 10.1038/ncomms5768
Wang, S., Wu, K., Yuan, Q., Liu, X., Liu, Z., Lin, X., et al. (2012). Control of grain size, shape and quality by OsSPL16 in rice. Nat. Genet. 44, 950–954. doi: 10.1038/ng.2327
Wang, C., Yu, H., Huang, J., Wang, W., Faruquee, M., Zhang, F., et al. (2020). Towards a deeper haplotype mining of complex traits in rice with RFGB v2.0. Plant Biotechnol. J. 18, 14–16. doi: 10.1111/pbi.13215
Weng, J., Gu, S., Wan, X., Gao, H., Guo, T., Su, N., et al. (2008). Isolation and initial characterization of GW5, a major QTL associated with rice grain width and weight. Cell Res. 18, 1199–1209. doi: 10.1038/cr.2008.307
Wu, Y., Wang, Y., Mi, X., Shan, J., Li, X., Xu, J., et al. (2016). The QTL GNP1 encodes GA20ox1, which increases grain number and yield by increasing cytokinin activity in rice panicle meristems. PloS Genet. 12, e1006386. doi: 10.1371/journal.pgen.1006386
Xiao, N., Gao, Y., Qian, H., Gao, Q., Wu, Y., Zhang, D., et al. (2018). Identification of genes related to cold tolerance and a functional allele that confers cold tolerance. Plant Physiol. 177, 1108–1123. doi: 10.1104/pp.18.00209
Xu, Z., Wang, H. (2007). LTR_FINDER: an efficient tool for the prediction of full-length LTR retrotransposons. Nucleic Acids Res. 35, W265–W268. doi: 10.1093/nar/gkm286
Xu, Y., Wang, R., Wang, Y., Zhang, L., Yao, S. (2020). A point mutation in LTT1 enhances cold tolerance at the booting stage in rice. Plant Cell Environ. 43, 992–1007. doi: 10.1111/pce.13717
Xue, W., Xing, Y., Weng, X., Zhao, Y., Tang, W., Wang, L., et al. (2008). Natural variation in Ghd7 is an important regulator of heading date and yield potential in rice. Nat. Genet. 40, 761–767. doi: 10.1038/ng.143
Yan, W., Wang, P., Chen, H., Zhou, H., Li, Q., Wang, C., et al. (2011). A major QTL, Ghd8, plays pleiotropic roles in regulating grain productivity, plant height, and heading date in rice. Mol. Plant 4, 319–330. doi: 10.1093/mp/ssq070
Yang, L., Zhao, M., Sha, G., Sun, Q., Gong, Q., Yang, Q., et al. (2022). The genome of the rice variety LTH provides insight into its universal susceptibility mechanism to worldwide rice blast fungal strains. Comput. Struct. Biotechnol. J. 20, 1012–1026. doi: 10.1016/j.csbj.2022.01.030
Yano, M., Katayose, Y., Ashikari, M., Yamanouchi, U., Monna, L., Fuse, T., et al. (2000). Hd1, a major photoperiod sensitivity quantitative trait locus in rice, is closely related to the arabidopsis flowering time gene CONSTANS. Plant Cell 12, 2473–2484. doi: 10.1105/tpc.12.12.2473
Yano, K., Ookawa, T., Aya, K., Ochiai, Y., Hirasawa, T., Ebitani, T., et al. (2014). Isolation of a novel lodging resistance QTL gene involved in strigolactone signaling and its pyramiding with a QTL gene involved in another mechanism. Mol. Plant 6, ssu131. doi: 10.1093/mp/ssu131
Yu, J., Hu, S., Wang, J., Wong, G., Li, S., Liu, B., et al. (2002). A draft sequence of the rice genome (Oryza sativa l. ssp. indica). Science 296, 79–92.
Yu, J., Miao, J., Zhang, Z., Xiong, H., Zhu, X., Sun, X., et al. (2018). Alternative splicing of OsLG3b controls grain length and yield in japonica rice. Plant Biotechnol. J. 16, 1667–1678.
Yu, J., Xiong, H., Zhu, X., Zhang, H., Li, H., Miao, J., et al. (2017). OsLG3 contributing to rice grain length and yield was mined by ho-LAMap. BMC Biol. 15, 28. doi: 10.1186/s12915-017-0365-7
Zhang, J., Chen, L., Sun, S., Kudrna, D., Copetti, D., Li, W., et al. (2016a). Building two indica rice reference genomes with PacBio long-read and illumina paired-end sequencing data. Sci. Data, 3, 160076.
Zhang, J., Chen, L., Xing, F., Kudrna, D., Yao, W., Copetti, D., et al. (2016b). Extensive sequence divergence between the reference genomes of two elite indica rice varieties zhenshan 97 and minghui 63. Proc. Natl. Acad. Sci. U.S.A. 113, E5163–E5171.
Zhang, Z., Li, J., Pan, Y., Li, J., Zhou, L., Shi, H., et al. (2017). Natural variation in CTB4a enhances rice adaptation to cold habitats. Nat. Commun. 8, 14788.
Zhang, H., Wang, Y., Deng, C., Zhao, S., Zhang, P., Feng, J., et al. (2022b). High-quality genome assembly of huazhan and tianfeng, the parents of an elite rice hybrid tian-you-hua-zhan. Sci. China Life Sci. 65, 398–411.
Zhang, F., Xue, H., Dong, X., Li, M., Zheng, X., Li, Z., et al. (2022a). Long-read sequencing of 111 rice genomes reveals significantly larger pan-genomes. Genome Res. 32, 853–863.
Zhao, Q., Feng, Q., Lu, H., Li, Y., Wang, A., Tian, Q., et al. (2018b). Pan-genome analysis highlights the extent of genomic variation in cultivated and wild rice. Nat. Genet. 50, 278–284.
Zhao, D., Li, Q., Zhang, C., Zhang, C., Yang, Q., Pan, L., et al. (2018a). GS9 acts as a transcriptional activator to regulate rice grain shape and appearance quality. Nat. Commun. 9, 1240.
Zhao, J., Wang, S., Qin, J., Sun, C., Liu, F. (2020). The lipid transfer protein OsLTPL159 is involved in cold tolerance at the early seedling stage in rice. Plant Biotechnol. J. 18, 756–769.
Keywords: aromatic japonica rice, genome assembly, early-matured, northern limit region, functional genes
Citation: Jiang S, Zhang X, Yang X, Liu C, Wang L, Ma B, Miao Y, Hu J, Tan K, Wang Y, Jiang H and Wang J (2023) A chromosome-level genome assembly of an early matured aromatic Japonica rice variety Qigeng10 to accelerate rice breeding for high grain quality in Northeast China. Front. Plant Sci. 14:1134308. doi: 10.3389/fpls.2023.1134308
Received: 30 December 2022; Accepted: 10 February 2023;
Published: 23 February 2023.
Edited by:
Yue Feng, China National Rice Research Institute (CAAS), ChinaReviewed by:
Kuldeep Kumar, Indian Institute of Pulses Research (ICAR), IndiaFan Zhang, Institute of Crop Sciences (CAAS), China
Qingyun Bu, CAS, China
Jinsong Bao, Zhejiang University, China
Copyright © 2023 Jiang, Zhang, Yang, Liu, Wang, Ma, Miao, Hu, Tan, Wang, Jiang and Wang. This is an open-access article distributed under the terms of the Creative Commons Attribution License (CC BY). The use, distribution or reproduction in other forums is permitted, provided the original author(s) and the copyright owner(s) are credited and that the original publication in this journal is cited, in accordance with accepted academic practice. No use, distribution or reproduction is permitted which does not comply with these terms.
*Correspondence: Junhe Wang, wangjunhe@haas.cn; Hui Jiang, hui@haas.cn
†These authors have contributed equally to this work