- College of Agriculture, Liaocheng University, Liaocheng, China
Agrobacterium rhizogenes–mediated (ARM) transformation is an efficient and powerful tool to generate transgenic roots to study root-related biology. For loss-of-function studies, transgenic-root-induced indel mutations by CRISPR/Cas9 only with homozygous/biallelic mutagenesis can exhibit mutant phenotype(s) (excluding recessive traits). However, a low frequency of homozygous mutants was produced by a constitutive promoter to drive Cas9 expression. Here, we identified a highly efficient Arabidopsis thaliana gamma-glutamylcysteine synthetase promoter, termed AtGCSpro, with strong activity in the region where the root meristem will initiate and in the whole roots in broad eudicots species. AtGCSpro achieved higher homozygous/biallelic mutation efficiency than the most widely used CaMV 35S promoter in driving Cas9 expression in soybean, Lotus japonicus, and tomato roots. Using the pAtGCSpro-Cas9 system, the average homozygous/biallelic mutation frequency is 1.7-fold and 8.3-fold higher than the p2 × 35Spro-Cas9 system for single and two target site(s) in the genome, respectively. Our results demonstrate the advantage of the pAtGCSpro-Cas9 system used in ARM transformation, especially its great potential in diploids with multiple-copy genes targeted mutations and polyploid plants with multiplex genome editing. AtGCSpro is conservatively active in various eudicots species, suggesting that AtGCSpro might be applied in a wide range of dicots species.
Introduction
Agrobacterium rhizogenes–mediated (ARM) transformation has revolutionized biological research through its ability to rapidly, simply, and conveniently generate transgenic roots of plant species, including in species recalcitrant to genetic transformation mediated by A. tumefaciens (Fan et al., 2020a; Fan et al., 2020b). Transgenic hairy roots co-transformed with the T-DNA from both the Ri plasmid of A. rhizogenes (carrying root locus [rol] genes, inducing the production of hairy roots) and the binary vector (Chilton et al., 1982; Irigoyen et al., 2020) can be generated. ARM transformation has already been established in a wide variety of plant taxa of more than 100 species and has widely been used for the modification of root traits, either because no protocols for stable A. tumefaciens–mediated transformation to generate transgenic plant (whole plant is genetically modified) or because root-related biological traits were analyzed. The composite plant generated by ARM transformation is composed of transgenic roots and wild shoot, which has already broadly applied for interactions between roots and microbes (e.g., rhizobia, arbuscular mycorrhizal fungi, pathogens, and nematode), signal transduction between root and shoot, and interactions between plant roots and environment (biotic/abiotic stresses). In addition, transgenic hairy roots can be rapidly induced and produced higher biomass by ARM transformation for the fast production of secondary metabolites and phytoremediation (e.g., Plasencia et al., 2016; Fan et al., 2017; Wang et al., 2017; Yang et al., 2017; Jenei et al., 2020; Fan et al., 2020a; Zhang et al., 2021).
To knock out gene(s) for loss-of-function studies in roots by ARM transformation, clustered regularly interspaced short palindromic repeats (CRISPR)–associated Cas (CRISPR/Cas) systems provide a convenient and powerful tool. The CRISPR/Cas9 system is the most frequently and widely employed targeted genome editing tool due to its simplicity, high specificity, efficiency, and multiplexing capacity (Hua et al., 2019). The CRISPR/Cas9 system is composed of the single-guide RNA (sgRNA) for target DNA recognition and the Cas9 nuclease for DNA cleavage. Previous studies had shown that the editing efficiency and mutation types (homozygous, heterozygous, or bi-allelic) mediated by the CRISPR/Cas9 system varied considerably in different plant tissues and species when different promoters were used to drive the expression of Cas9 via A. tumefaciens–mediated stable genetic transformation (Wang et al., 2015; Yan et al., 2015; Eid et al., 2016; Gao et al., 2016; Mao et al., 2016; Tsutsui and Higashiyama, 2017; Feng et al., 2018). However, genome editing efficiencies mediated by CRISPR/Cas9 using different promoters to drive the expression of Cas9 by ARM transient transformation have not been evaluated. In most cases, cauliflower mosaic virus (CaMV) 35S is used to drive the expression of Cas9 (Yan et al., 2015; Tang et al., 2016; Ma et al., 2016; Fan et al., 2017; Feng et al., 2018; Zhang et al., 2020) in ARM transformation but with a low genome editing efficiency. In the transformed hairy roots mediated by ARM transformation, except for recessive traits, mutant phenotype(s) can be observed only when all alleles are edited and homozygous/biallelic mutations (H/BM) generated. This is a challenge and bottleneck to achieving multiple targeted loci simultaneous homozygous/biallelic mutagenesis in the transient expression of the CRISPR/Cas9 system in diploid and polyploid plants with multiple gene copies. Large numbers of transformants need to be selected and further identified whether homozygous/biallelic mutagenesis was induced at all the target sites, which is labor intensive, time consuming, tedious, and costly.
Here, we describe a highly efficient Arabidopsis thaliana gamma-glutamylcysteine synthetase promoter named AtGCSpro (7-GCS; EC 6.3.2.2; May and Leaver, 1994). pAtGCSpro::GUSPlus transformed whole roots showed a GUS signal and a high level of GUS activity in the initiation region of root meristem undergoing active cell division in broad eudicots diploid soybean (Glycine max), tomato (Solanum lycopersicum), cucumber (Cucumis sativus L.), Lotus japonicus, as well as in polyploid tobacco (Nicotiana tabacum L.), cotton (Gossypium spp), and sweet potato (Ipomoea batatas). Our results indicate the advantage of using AtGCSpro for CRISPR/Cas9 genome editing in inducing H/BM rate applied in ARM transformation of L. japonicus (a model leguminous plant species), soybean, and tomato. This approach has great potential in research addressing multiplex gene copies or gene families with functional redundancy. The conserved and high activity of AtGCSpro in roots covering a wide range of dicots species suggests that AtGCSpro might have great potential to be applied broadly to achieve high H/BM rates at target sites by CRISPR/Cas9 via ARM transformation.
Materials and methods
Plant materials and growth conditions
Soybean (Glycine max) Williams 82, L. japonicus (Gifu-129), cucumber (Cucumis sativus L.) “Chinese long” inbred line 9930, tomato (Solanum lycopersicum) local variety Maofeng802, sweet potato (Ipomoea batatas) local variety Jishu25, cotton (Gossypium spp.) local variety Lumianyan28, tobacco (Nicotiana benthamiana), and Arabidopsis thaliana Columbia (Col-0) were used in this study. The plants were grown in a greenhouse under a photoperiod of 16h light (80 µM photons m-2 s-1)/8h dark at 24 ± 2C.
Cloning of AtGCSpro, construction of various lengths AtGCSpro to drive the expression of GUSPlus plasmid vectors, histological GUS staining, and qRT-PCR analysis
To isolate AtGCSpro, a 2411-bp upstream promoter region of the translation start site of gamma-glutamylcysteine synthetase gene (GenBank accession no. AF068299.1) was amplified by PCR using a GaBa1 primer containing a BamHI site combined with a GaBNR primer containing a BsaI site (produced 5′-CATG sticky end) from A. thaliana Columbia (Col-0) genomic DNA as a template. All primers sequences used in this paper are listed in Supplementary Table S1.
To generate GUSPlus expressing vectors with various AtGCSpro promoter lengths, a recombinant binary vector pRed1305 (Fan et al., 2020b) harboring a GUS gene driven by CaMV35S with an intron from the catalase gene was used as the backbone. The CaMV35S promoter in pRed1305 was replaced by various lengths of AtGCSpro, respectively. Shortened lengths of AtGCSpro were PCR-amplified from the 2411-bp AtGCSpro with a reverse primer (GaBNR) and a forward primer (GaBa2, GaBa3, GaBa4, or GaBa5). The PCR amplification products, including a BamHI restriction site at the 5′ end and a BsaI restriction site at the 3′ end, were digested and directly ligated into pRed1305 previously digested with BamHI and NcoI, thus producing the pRedGa1 (AtGCSpro2411:: GUSPlus), pRedGa2 (AtGCSpro1977:: GUSPlus), pRedGa3 (AtGCSpro1629:: GUSPlus), pRedGa4 (AtGCSpro1178:: GUSPlus), and pRedGa5 (AtGCSpro833:: GUSPlus) vectors. Schematic diagrams of the constructs are shown in Figure 1A. All constructs mentioned in the paper were confirmed by Sanger sequencing. The DNA ladder DL2000 in this paper was bought from Sangon Biotech (China, Shanghai).
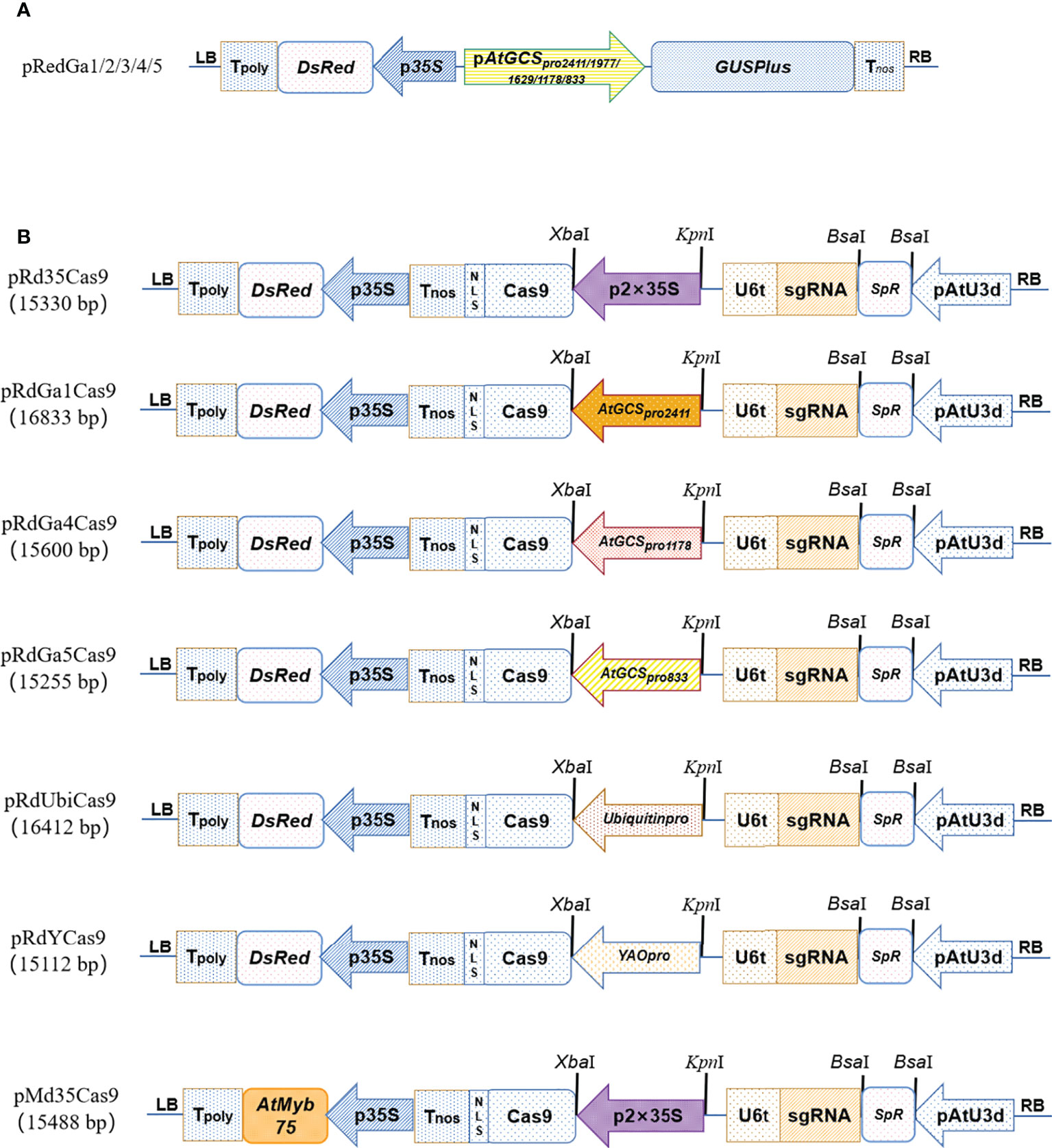
Figure 1 The schematic diagrams of vectors. The schematic diagrams of the pRedGa1 (AtGCSpro2411:: GUSPlus), pRedGa2 (AtGCSpro1977:: GUSPlus), pRedGa3 (AtGCSpro1629:: GUSPlus), pRedGa4 (AtGCSpro1178:: GUSPlus), and pRedGa5 (AtGCSpro833:: GUSPlus) (A). CRISPR/Cas9-mediated gene knockout vector backbones pRd35Cas9, pRdGa1Cas9, pRdGa4Cas9, pRdGa5Cas9, pRdUbiCas9, pRdYCas9, and pMd35Cas9 (B).
Histological GUS staining was performed as previously described (Fan et al., 2020b). Relative expression levels of GUS were performed by a quantitative Real-time PCR (qRT-PCR) assay according to Lü et al. (2010) with the following minor modifications. The amplification of the soybean GmActin gene was used for normalization, and the primer pairs GmActinF and GmActinR were used according to Fan et al. (2020a). The gene-specific primer pairs GUSPF and GUSPR for GUSPlus gene were used. qRT-PCR experiments were performed with three replicates. In a biological replicate, for each independent transformed event (transformed pRedGa1, pRedGa2, pRedGa3, pRedGa4, or pRedGa5 construct), total 10 independent transgenic positive roots (with ~4 cm root lengths) for each independent construct were individually sampled, ground in liquid nitrogen, and used for total RNA extraction, respectively.
Construction of CRISPR/Cas9-mediated gene knockout vector backbones
We first generated a series of CRISPR/Cas9-mediated gene knockout vector backbones: pRd35Cas9 (p2×35Spro-Cas9), pRdGa1Cas9 (pAtGCSpro2411-Cas9), pRdGa4Cas9 (pAtGCSpro1178-Cas9), pRdGa5Cas9 (pAtGCSpro833-Cas9), pRdUbiCas9 (pUbiquitinpro-Cas9), pRdYCas9 (pYAOpro-Cas9), and pMd35Cas9 (Figure 1B).
We first recombined an intermediate vector pRSE401 (Supplementary Figure S1) based on the backbone of pHSE401 (Xing et al., 2014), a DsRed reporter gene driven by CaMV35S promoter that replaced the Hpt II (Hygromycin Phosphotransferase II), which can be easily screened for transgenic positive hairy roots (Supplementary Figure S2). To introduce the DsRed driven by the CaMV35S promoter into pHSE401, the p35S-DsRed-CaMV poly(A)-LB cassettes (primary regions from 8,392 to 11,034 in pCAMBIA1305) were produced from the vector pRed1305 (Fan et al., 2020b) digested by EcoRI and SacII and then inserted into pHSE401.
In addition, to shorten the vector sizes, the AtU6-26 promoter (424 bp) was replaced by the AtU3d promoter (121 bp) to drive the gRNA expression (Ma et al., 2015). The complete gRNA expression cassettes of AtU3d-gRNA-Sc-U6-26t were generated by recombinant PCR. To substitute AtU6-26 for AtU3d in pRSE401, the AtU3d promoter (primary regions 144–264 bp in pYLsgRNA-AtU3d) was amplified by PCR with primers Sap401 and Rd4012 using pYLsgRNA-AtU3d plasmid (Ma et al., 2015) as the template. BsaI-gRNA-Sc-U6-26t cassettes (primary regions 1327–2190 bp in pHSE401, Addgene No. 62201) were amplified by PCR with primers Rd4013 and Sap402 using pHSE401 plasmid (Ma et al., 2015) as the template. The two PCR fragments were recombined to generate the sgRNA expression cassettes of AtU3d-BsaI-SpR-BsaI-gRNA-Sc-U6-26t by recombinant PCR using primers Sap401 and Sap402, followed by digestion using SapI, which was cloned into pRSE401 (Supplementary Figure S1) and pPG35Cas9 (Fan et al., 2020c), respectively, previously digested by HindIII followed by added with an “A” at the 3′ end of cohesive ends using KOD DNA polymerase with dATP. Therefore, the recombinant CRISPR-Cas9 vectors pRd35Cas9 and pMd35Cas9 were generated (Figure 1B). Based on the backbone of pRd35Cas9, an AtGCSpro2411 promoter replaced the 2 × 35S and produced the pRdGa1Cas9 (Figure 1B).
pRdGa1Cas9 generation was as follows. Full-length AtGCSpro2411 was amplified by PCR with primer GaK1 with a BsaI restriction enzyme digestion site (produce 5′-GTAC sticky end) at the 5′ end and primer GaX2 with an XbaI at the 3′ end and then digested using restriction enzymes for cloning into pRd35Cas9 previously digested by Acc651 and XbaI and, therefore, produced vector pRdGa1Cas9 (Figure 1B).
The Ubiquitin promoter, YAO promoter, AtGCSpro1178, and AtGCSpro833 were amplified by PCR with the primers Ubikp/Ubixp (for Ubiquitinpro), YAOF18/PYao2 (for YAOpro), GaBa4/GaX2 (for AtGCSpro1178), and GaK5/GaX2 (for AtGCSpro833) using pYLCRISPR/Cas9Pubi-B (Ma et al., 2015), pYGUS1305 (Fan et al., 2020b), AtGCSpro2411, and AtGCSpro2411 as the templates, respectively, and digested by KpnI/BsaI, KpnI/BsaI, KpnI/XbaI, and KpnI/XbaI, respectively, for cloning into the KpnI/XbaI restriction sites of the pRd35Cas9, and therefore generated pRdUbiCas9, pRdYCas9, pRdGa4Cas9, and pRdGa5Cas9 (Figure 1B).
Construction of CRISPR/Cas9 genome editing vectors using different promoters to drive Cas9 expression
To construct genome editing vectors to knockout soybean Rj7, pRdGa1Cas9, pRdUbiCas9, pRdYCas9, pRdGa4Cas9, pRdGa5Cas9, and pRd35Cas9 were used as a backbone, respectively. Oligos Ktrj71 and Ktrj72 specifically targeted the soybean Rj7 for construction of p2×35Spro-Cas9-Rj7, pUbiquitinpro-Cas9-Rj7, pYAOpro-Cas9-Rj7, pAtGCSpro2411-Cas9-Rj7, pAtGCSpro1178-Cas9-Rj7, and AtGCSpro833-Cas9-Rj7 vector, respectively.
To construct genome editing vectors to knockout L. japonicus LjNLP4, oligos KtLjNL1 and KtLjNL2 were designed and located in the exon of the open reading frame of LjNLP4 (position: 28146509-28146531). The CRISPR/Cas9 vectors pMd35Cas9, pRdGa1Cas9, pRdUbiCas9, pRdYCas9, and pRdGa4Cas9 and pPG35Cas9 (Figure 1B) were used and generated the p2×35Spro-Cas9-LjNLP4, pAtGCSpro2411-Cas9-LjNLP4, pUbiquitinpro-Cas9-LjNLP4, pYAOpro-Cas9-LjNLP4, and pAtGCSpro1178-Cas9-LjNLP4 vectors, respectively.
To construct simultaneously targeting two genome sites, oligos ktGmR11 and ktGmR12 were designed to specifically target soybean GmNNL1 (Zhang et al., 2021) and Rfg1 (Fan et al., 2017) using pRd35Cas9 and pRdGa4Cas9 (Figure 1B) as backbones for generating p2×35Spro-Cas9-GmNNL1Rfg1 and pAtGCSpro1178-Cas9-GmNNL1Rfg1 vectors, respectively. Oligos ktLjSNF and ktLjSNR were designed to specifically target L. japonicus LjNLP4 and LjSYMRK (Wang et al., 2016) using pMd35Cas9 and pRdGa4Cas9 (Figure 1B) as backbones for construction of the p2×35Spro-Cas9-LjNLP4LjSYMRK and AtGCSpro1178-Cas9-LjNLP4LjSYMRK, respectively. Oligos ktSlTRY1 and ktSlTRY2 were designed to specifically target two different sites within the first and second exon of tomato SlTRY (Tominaga-Wada et al., 2013), respectively, using pRd35Cas9 and pRdGa4Cas9 (Figure 1B) as backbones for generating p2×35Spro-Cas9-SlTRY and pAtGCSpro1178-Cas9-SlTRY, respectively. The Optimized CRISPR Plant Design Tool (http://cbi.hzau.edu.cn/cgi-bin/CRISPR) was used to design the oligos for constructing of CRISPR/Cas9 vector(s) (Lei et al., 2014). CRISPR/Cas9-mediated gene mutation vectors were constructed according to the procedure described previously by Xing et al. (2014). Specifically, for cloning a single gRNA into BsaI sites of Cas9 expression vector, oligo primers annealing was carried out; for construction of two-gRNA-expressing vectors for gene targeting, the fragments were amplified via over-lapping PCR with designed primers using the vector pCBC-DT1T2 (Xing et al., 2014) as a template, and then inserted into Cas9 expression vector that was linearized by BsaI through the Golden Gate cloning method.
ARM hairy root transformation
The constructs were transformed into the A. rhizogenesis strain K599 (for soybean, cucumber, tomato, cotton, sweet potato, and tobacco) and ARqual (for L. japonicus) by electroporation, respectively. Composite soybean, cucumber, tomato, cotton, sweet potato, and tobacco plants were generated by one-step ARM transformation (Fan et al., 2020a; Fan et al., 2020b). Composite L. japonicus was generated according to the protocol (Okamoto et al., 2013). L. japonicus nodulation assay was performed as described by Fan et al. (2020c). L. japonicus composite plants with ~5 cm root lengths were inoculated with Mesorhizobium loti MAFF303099. For the nitrate response assay, 10 mM KNO3 was used and watered the transformed roots.
Transgenic hairy roots screening, mutation type(s), and validation of genome editing
Previously, we successfully generated purple/red anthocyanin accumulation by overexpression of AtMyb75 in transgenic L. japonicus hairy roots, which can be tracked as a directly visual selection marker of transgenic roots with the naked eyes in the study of rhizobia-legume symbiosis (Fan et al., 2020c). The transgenic positive hairy roots were screened by the purple/red anthocyanin accumulation on roots depending on the expression of AtMyb75 or by visual DsRed fluorescence produced from the expression of DsRed reporter gene due to the different the CRISPR/Cas9 genome editing vector backbones used (Figure 1B and Supplementary Figure S2).
To analyze the mutations caused by CRISPR/Cas9, PCR/RE, (restriction enzyme) and Sanger sequencing assays were performed. Genomic DNA was extracted from independent transgenic positive hairy roots (co-transformed primary root) of 5–10 cm in length. The DNA sequences covering the CRISPR target sites of the transformed plants were amplified by PCR using gene-specific primers (Supplementary Table S1). Rj7, GmNNL1, Rfg1, LjNLP4, LjSYMRK, and SlTRY-specific fragments were amplified using pairs of primers Rj71/Rj72 (for Rj7), GmRHin1/GmRHin2 (for GmNNL1), GmRNco1/GmRNc4 (for Rfg1), LjNLP1F/LjNLP1R (for LjNLP4), LjSYF/LjSYR (for LjSYMRK), and SLTRY1/SLTRY2 (for SlTRY) and subsequently subjected to restriction enzyme digestion analyses and sequenced to identify the gene-edited type(s). About 10 clones for each amplicon were individually sequenced to further determine the mutation type. The experiments were replicated for three biological replicates for each transformed construct. The mean values were used for statistical analysis.
Results
AtGCSpro root expression
To assess the promoter activity of AtGCS at an earlier stage of the initiation of hairy roots and in developing root, a 2411-bp upstream promoter region of AtGCS was cloned and used to drive the expression of GUS (β-Glucuronidase). pAtGCSpro:: GUSPlus was transformed into soybean by ARM hairy roots transformation. A high level of GUS activity is found in the teratoma that is formed, from which hairy roots can emerge, and ubiquitously in the roots (Figures 2A, B).
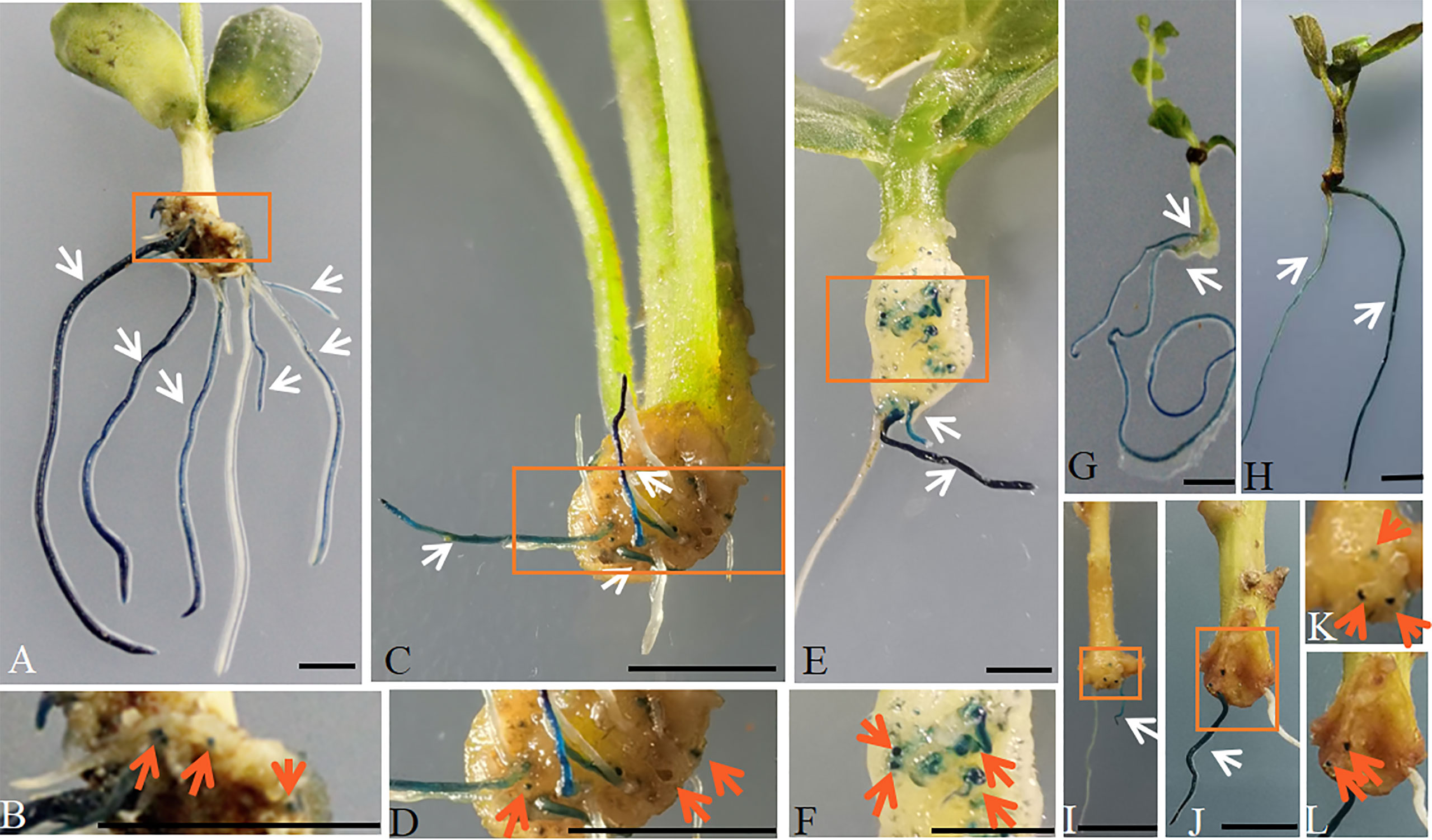
Figure 2 Histochemical localization of GUS activity in the pAtGCSpro2411:: GUSPlus hairy roots in broad dicots species by ARM transformation. High level of GUS activity was accumulated in the whole transformed pAtGCSpro2411:: GUSPlus roots in soybean (A, B), tomato (C, D), cucumber (E, F), L. japonicas (G), cotton (H), tobacco (I, K), sweet potato (J, L), respectively. White arrows indicate the transgenic roots. Pictures B, D, F, K, L are closed up of sections A, C, E, I, J marked in the orange boxes, respectively. The GUS signal was found in the teratoma where hairy roots can emerge (indicated by orange arrows). All composite plants were observed from 16 to 21 d post-infected seedlings with K599 carrying pRedGa1 construct. Bars = 5 mm.
In addition to experiments in soybean, we also tested the AtGCSpro activity in broad eudicot species, including diploid species tomato (Figures 2C, D), cucumber (Figures 2E, F), and L. japonicus (Figure 2G), as well as polyploid species cotton (Figure 2H), tobacco (Figures 2I, K), and sweet potato (Figures 2J, L). These results are in agreement with that of AtGCSpro in soybean (Figures 2A, B). Whole transformed pAtGCSpro::GUSPlus roots show a strong GUS signal in the initiation emergence regions of hairy roots and the whole roots (Figures 2C–L). These results indicate that AtGCSpro activity is broadly conserved in eudicots.
To further analyze the promoter activity with different shortened lengths, truncated lengths AtGCSpro with 5′ deletion fragments were produced and used to drive the expression of GUS in soybean hairy roots. Here, we designated the full length 2411-bp sequences as AtGCSpro2411 and truncated lengths 1977-bp, 1629-bp, 1178-bp, and 833-bp sequences as AtGCSpro1977, AtGCSpro1629, AtGCSpro1178, and AtGCSpro833, respectively. There were no distinct differences in the GUS signals when comparing the transformed pAtGCSpro1977::GUSPlus (Figures 3A, B), pAtGCSpro1629::GUSPlus (Figures 3C, D), and pAtGCSpro2411::GUSPlus roots (Figures 2A, B; Supplementary Figure S3). In contrast, a little bit low GUS activity was observed in the transformed AtGCSpro1178::GUSPlus roots by histological GUS staining (Figures 3E, F). This result was in accordance with the relative expression analysis of GUS by qRT-PCR in transgenic roots, showing a little bit low expression levels of GUS but with no significant difference with those of AtGCSpro2411::GUSPlus, AtGCSpro1977::GUSPlus, and AtGCSpro1629::GUSPlus roots (Supplementary Figure S3). The expression level of GUS was the lowest in the hairy roots transformed with pAtGCSpro833::GUSPlus (Figures 3G–I and Supplementary Figure S3). No GUS expression is in the root tips, indicating that AtGCSpro833 has no activity in the root tip tissues (Figures 3G, I). In addition, we also analyzed the activity of AtGCSpro1178 in cucumber and tomato by ARM transformation (Figures 4A–D). The AtGCSpro1178 activity in cucumber (Figures 4A–C) and tomato (Figures 4B, D) is in agreement with those in soybean (Figures 3E, F). The GUS signals are strong and can be detected in the region that will develop into root meristem and in the whole developing roots.
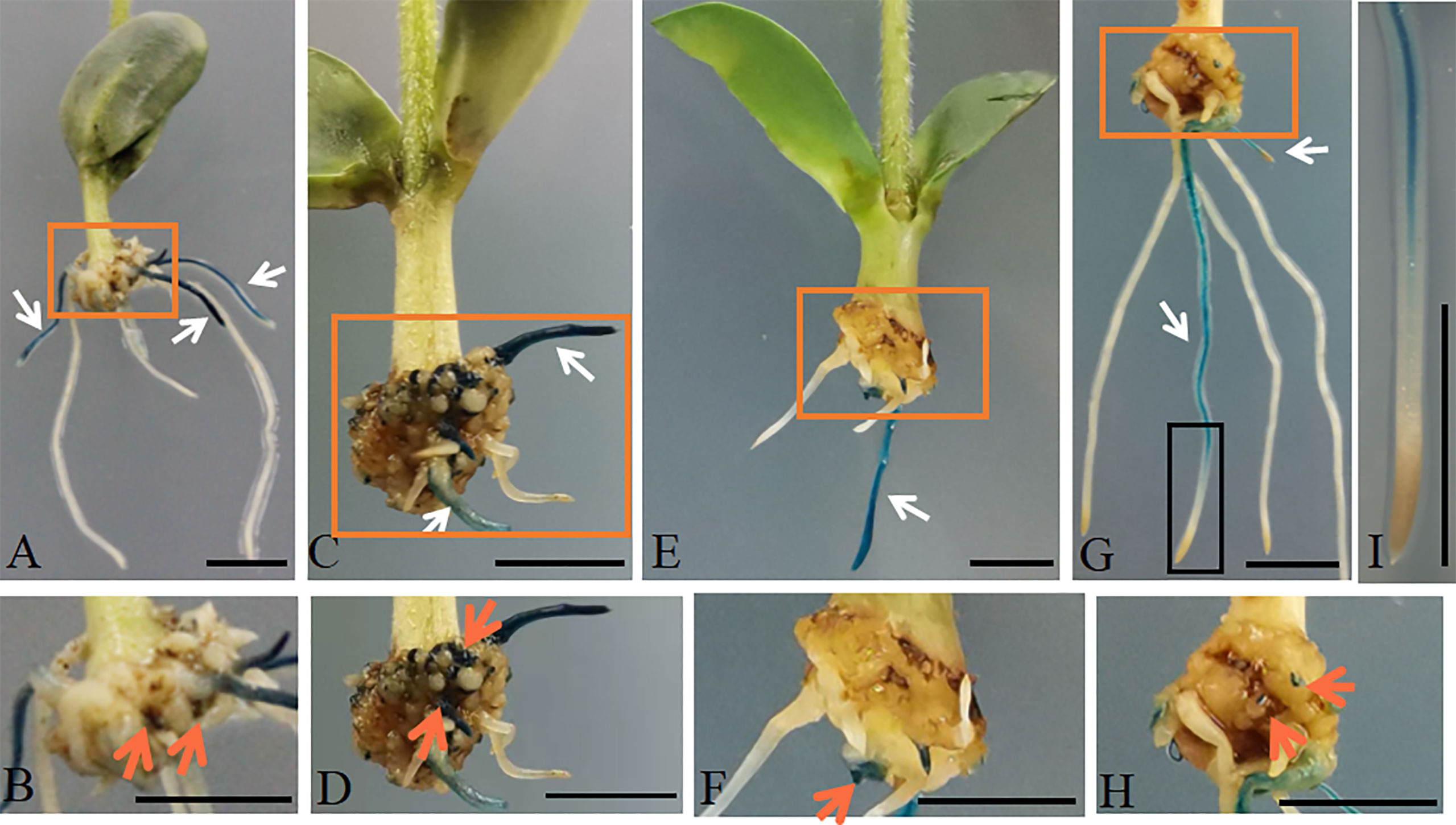
Figure 3 AtGCSpro activity assay with different shortened lengths in soybean hairy roots by ARM transformation. AtGCSpro1977:: GUSPlus (A, B). AtGCSpro1629:: GUSPlus (C, D). AtGCSpro1178:: GUSPlus (E, F). AtGCSpro833:: GUSPlus in the soybean hairy roots (G, H). The transgenic root marked in the black box in picture (G) is closed-up (I). White arrows indicate the transgenic roots. Pictures B, D, F, and H are closed-up of sections (A, C, E), and G marked in the orange boxes, respectively. The GUS signal was found in the teratoma where hairy roots can initiate (indicated by orange arrows). All composite plants were observed from 16-day-old post-infected seedlings. Bars = 1 cm.
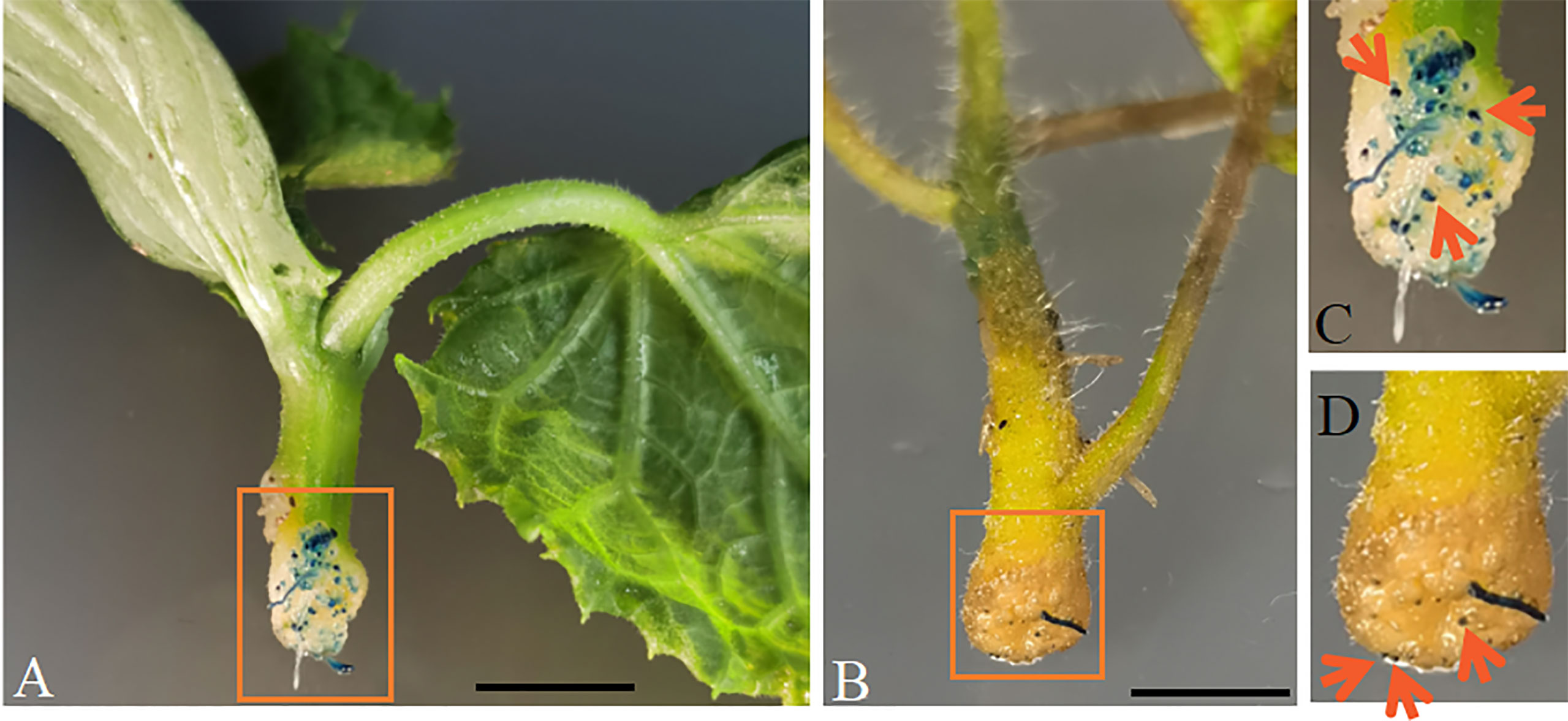
Figure 4 AtGCSpro1178 activity assay in other dicots hairy roots by ARM transformation. Cucumber (A) and tomato (B) transformed with AtGCSpro1178:: GUSPlus. GUS signal can be observed in the region where will produce transgenic roots (Shown by orange arrows in (C and D) (C, D). Pictures C and D are closed-up of sections (A, B) marked in the orange boxes, respectively. All composite plants were observed from 16-day-old post-infected seedlings with K599 carrying pAtGCSpro1178:: GUSPlus. Bars = 1 cm.
High H/BM induction rates in transgenic soybean and L. japonicus hairy roots using the pAtGCSpro-Cas9 system
Based on AtGCSpro activity analyses, we reasoned that Cas9 driven by the AtGCSpro could be specifically transcribed in the meristematic region where the root meristem will initiate and in the whole root. To test whether AtGCSpro might improve H/BM frequencies in ARM transformation, we first aimed to knockout a single target site in two leguminous plant species, the important crop soybean and the model plant L. japonicus. The dominant traits are the most prevalent in the genome, such as, in soybean, most of characterized genes are dominant genes (Zhang et al., 2022). Due to only the transgenic roots generated H/BM at the dominant target gene site can result in loss-of-function mutation phenotype(s) in the ARM transient transformation; here, we only analyzed the H/BM-induced frequency. The soybean GmNARK (Rj7) played a crucial role in the autoregulation of nodulation (Searle et al., 2003; Lin et al., 2010) and was selected as a target site. To knock out Rj7, one target site in the first exon of Rj7 containing an EcoRI restriction endonuclease digestion site next to the NGG region (Figure 5A) was selected to identify the mutation genotypes. To determine and quantify gene editing efficiency, genomic DNA was extracted from 30 independently transformed hairy roots. PCR amplicon spanning the target site was subjected to digestion by the EcoRI restriction enzyme, and sequenced to verify mutations-type (Figures 5B–F and Table 1). The hairy roots transformed with the p2×35Spro-Cas9-Rj7 vector, 17 lines (#1, #4–7, #9–12, #15–17, #21, #24–26, and #30) among 30 independent transgenic lines are homozygous or biallelic mutations. Compared with the transgenic hairy roots transformed with the p2×35Spro-Cas9-Rj7, in the pAtGCSpro2411-Cas9-Rj7 roots, 23 lines (#1–2, #4–14, #17–19, #21–22, #24–26, and #29–30) among 30 independent transgenic lines are homozygous or biallelic mutations (Figure 5D). The results indicate that the pAtGCSpro2411-Cas9 system yields 76.7% (23/30) homozygous/biallelic mutants compared with 56.7% (17/30) for the p2×35Spro-Cas9 system (Figures 5B–E; Table 1). AtGCSpro2411 exhibits higher efficiency than 2×35Spro in inducing the H/BM in soybean. Various types of insertions or deletions (indels) at the target site are shown. Most of the mutation events were small indels (± 1–8 bp). No large indels (> 50 bp) were observed in 30 randomly selected sequencing mutants. Noticeably, some H/BM mutants with 3n indels (Figures 5C, E and Supplementary Figure S4).
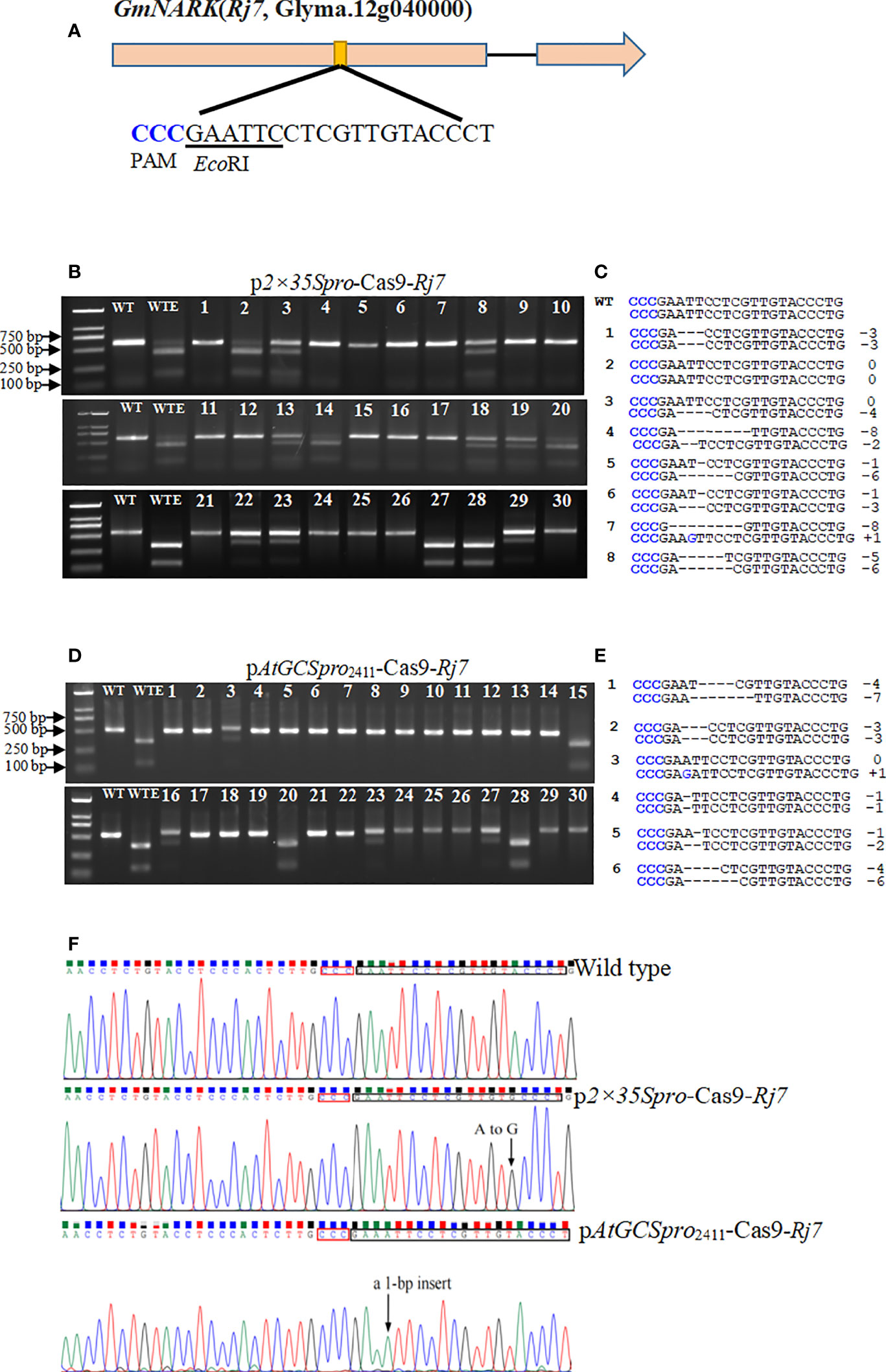
Figure 5 Identification of CRISPR/Cas9-induced mutation in the GmNARK (Rj7) target loci in soybean with AtGCSpro2411 and 2×35S to drive Cas9, respectively. The sequence of an sgRNA designed to target a site within the first exon region of Rj7. The protospacer-adjacent motif (PAM) sequence is highlighted in blue and the EcoRI restriction site is underlined (A). PCR-RE assays to detect CRISPR/Cas9-induced mutation in the Rj7 target loci from 30 different independent p2×35Spro-Cas9 hairy roots (B). Genotypes of eight representative mutants from transformed with p2×35Spro-Cas9 hairy roots identified by sequencing (C). PCR-RE assays to detect CRISPR/Cas9-induced mutation in the Rj7 target loci from 30 different independent pAtGCSpro2411-Cas9 hairy roots (D). Genotypes of six representative rj7 mutants from transformed with pAtGCSpro2411-Cas9 hairy roots identified by sequencing (E). In sections B and D, Lanes WT and WTE, undigested PCR amplification fragment and digested wild-type controls by EcoRI, respectively. Lanes 1–30, different independent transgenic hairy roots. In sections C and E, deletions and insertions are indicated as dashes and blue letter, respectively. The types and number(s) of indels are indicated in the right column. Examples given of mutation at target site in the p2×35Spro-Cas9 and pAtGCSpro2411-Cas9 hairy root, respectively (F). Black arrows indicate the site of indels mutation. The PAM regions and mutated target sites are shown in the black box.

Table 1 Comparison of the targeted mutation efficiency in transgenic soybean hairy roots by ARM transformation using different truncated promoters to drive the expression of Cas9.
To determine the shortest AtGCSpro length used to drive the expression of Cas9 without sacrificing H/BM efficiency, we also generated pAtGCSpro1178-Cas9 and pAtGCSpro833-Cas9 systems to knockout Rj7. There was a slightly decreased H/BMs efficiency by pAtGCSpro1178-Cas9 (21/30; 70%) compared with pAtGCSpro2411-Cas9 (76.7%) (Supplementary Figures S5A, Figure 5D, and Table 1). However, the pAtGCSpro833-Cas9 system substantially affects the genome editing efficiency of H/BM at a rate of 50% (10/20) compared with 76.7% (23/30) for the pAtGCSpro2411-Cas9 system, which is even less than the p2×35Spro-Cas9 system (Supplementary Figures S5A–C, Figure 5D, and Table 1). Therefore, to minimize the construct’s size, for genome editing, we recommend using pAtGCSpro1178-Cas9 system.
In L. japonicus, the NRSYM1/LjNLP4 (Lj5g3v1999250.1) functions as a master regulator for nitrate-dependent symbiotic gene expression and inhibits nodulation when surplus nitrate is in soil. LjNLP4 was selected as the targeted gene because mutations in LjNLP4 result in conveniently observable “nodule” phenotypes, such as defects in high nitrate concentrations. The ljnlp4 mutant can form mature nitrogen-fixing nodules in the presence of a high nitrate concentration (Suzuki et al., 2013; Nishida and Suzaki, 2018; Nishida et al., 2021). One sgRNA was designed to target the LjNLP4 in L. japonicus (Figure 6A). To estimate the H/BM rate for ljnlp4, as the criterion of success, we used whether leghemoglobin-rich pink mature nodules formed on transgenic hairy roots in the presence of a high nitrate concentration. Using this classification, 40 transgenic plants were analyzed, and 33 of them (82.5%) were independent transgenic hairy roots lines transformed with the pAtGCSpro1178-Cas9-LjNLP4 showing mature nodules in the presence of high nitrate concentrations, compared with 32.5% (13/40) for p2×35Spro1178-Cas9-LjNLP4 (Figure 6B). To further accurately determine the gene-editing efficiency, PCR amplicons amplified from each independent transgenic hairy root covering the target site were subjected to digestion by restriction enzyme BamHI and Sanger sequencing. Sixteen independent transgenic roots were tested. The LjNLP4 targeted site was successfully edited using the p2×35Spro-Cas9 and pAtGCSpro1178-Cas9 systems (Figures 6C–E). However, the editing efficiencies are distinct between the p2×35Spro-Cas9-LjNLP4 and pAtGCSpro1178-Cas9-LjNLP4 systems (Figures 6C–E and Table 2). The H/BM rate for ljnlp4 was 31.3% (5/16) for the p2×35Spro-Cas9 system; much lower than the 81.3% (13/16) achieved using the pAtGCSpro-Cas9 system (Figures 6B–D and Table 2). Based on the results and previous mutation frequency results in soybean, on average, pAtGCSpro1178-Cas9 system shows a 1.7 times higher H/BM frequency than the p2×35Spro-Cas9 system for a single target site in the genome (proportion of H/BM-induced frequency using pAtGCSpro1178-Cas9 system compared with that of the p2×35Spro-Cas9 system in soybean and L. japonicus).
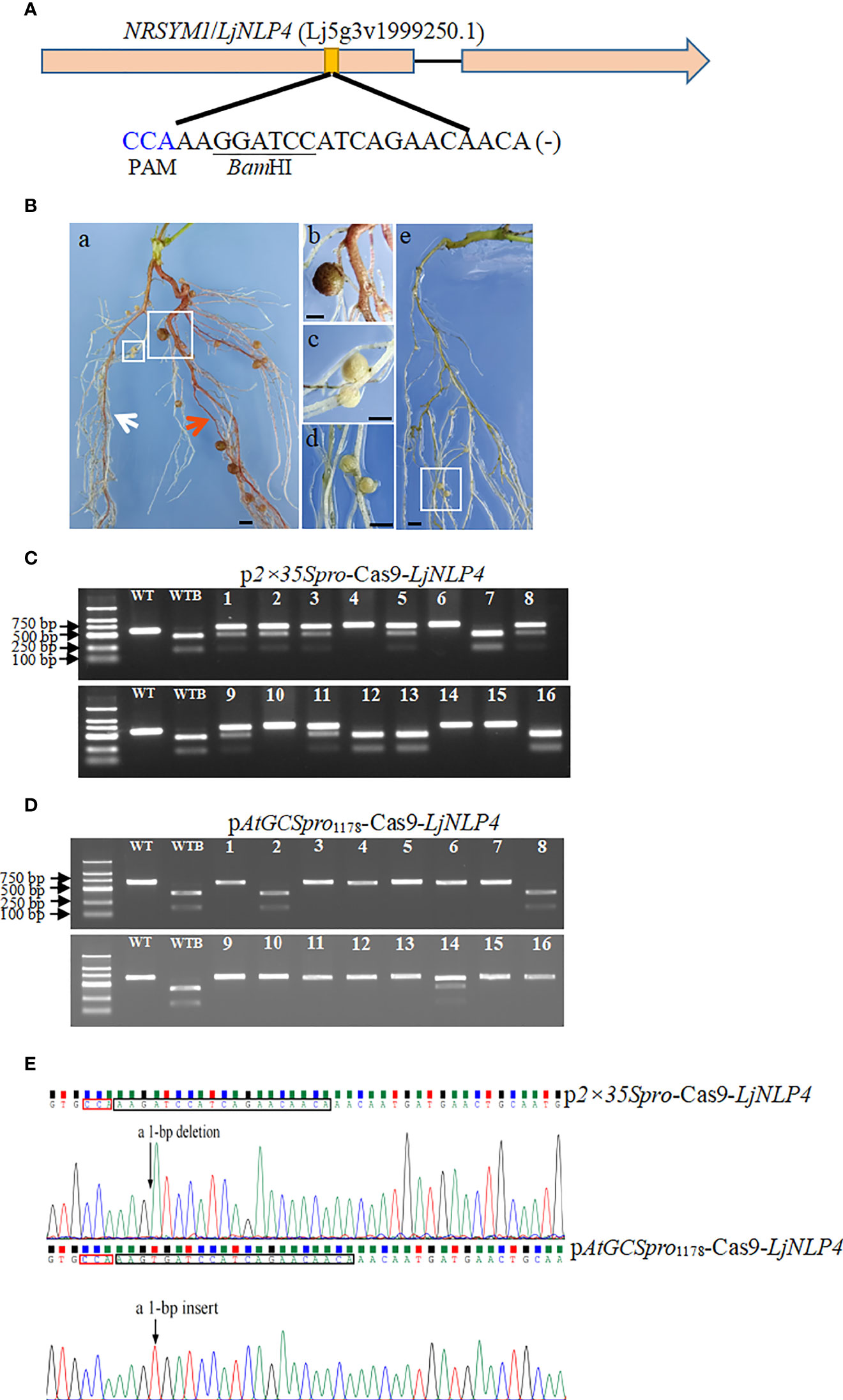
Figure 6 PCR-RE assay mutation efficiency in the NRSYM1/LjNLP4 target loci in L. japonicus. Sequence of an sgRNA designed to target a site within the first exon region of LjNLP4. The PAM sequence is highlighted in blue and the BamHI restriction site is underlined (A). L. japonicus hairy roots with edited NRSYM1/LjNLP4 allele. Transgenic hairy roots with edited LjNLP4Ljnlp4 (white arrow indicated), Ljnlp4Ljnlp4 allele (red arrow indicated) in picture a, and the wild type in picture e; pictures b, c, and d are closed-up of sections a (big white box), a (small white box) and e marked in the boxes, respectively. Bars = 1 mm (B). PCR-RE assays to detect mutation efficiency in the NRSYM1/LjNLP4 target loci. Lanes 1–16, different independent transgenic hairy roots. Lanes WT and WTB, undigested PCR amplification fragment and digested wild-type controls by BamHI, respectively (C, D). Five lines (#4, #6, #10, #14, and #15) were homozygous or biallelic mutations (C). 13 lines (#1, #3-7, #9-13, #15, and #16) were homozygous or biallelic mutations (D). An example shown of mutation in the target site in p2×35Spro-Cas9 and pAtGCSpro1178-Cas9 hairy root, respectively (E).

Table 2 Comparison of pAtGCSpro1178-Cas9-LjNLP4 and p2×35Spro-Cas9-LjNLP4 genome editing efficiency in L. japonicus hairy roots.
Besides AtGCSpro, we also evaluated the ubiquitin promoter (Ma et al., 2015) and YAO promoter (a high-efficiency germ cell-specific promoter in Agrobacterium-mediated genetic transformation and with high activity in roots) (Li et al., 2010; Yan et al., 2015; Feng et al., 2018; Fan et al., 2020a; Fan et al., 2020b). The results indicate that AtGCSpro is the most efficient promoter for inducing H/BM, outperforming the ubiquitin, YAO, and CaMV 35S promoters in both transgenic soybean and L. japonicus hairy roots (Tables S2 and S3).
Using the pAtGCSpro-Cas9 system for simultaneously targeting multiple genome loci(s) in ARM transformation
In plants, multiple genomic sites need to be edited simultaneously, resulting in the observable phenotype(s), such as studying multiple functionally related genes or the knockout of functionally redundant genes (Ma et al., 2016). Due to high-efficiency H/BM induction rates for a single target site using the AtGCSpro-Cas9 system relative to other systems, we next assessed the efficiency of pAtGCSpro1178-Cas9-induced H/BMs when simultaneously targeting two genome sites in soybean, L. japonicus, and tomato. In soybean, which is resistant to nodulation, GmNNL1 (Glyma.02g076900) (Zhang et al., 2021) and Rfg1 gene (Fan et al., 2017) were targeted simultaneously. p2×35Spro-Cas9-GmNNL1Rfg1 and pAtGCSpro1178-Cas9-GmNNL1Rfg1 achieved H/BM frequencies of 0% (0/30) and 6.7% (2/30) at the GmNNL1 site, 33.3% (10/30) and 83.3% (25/30) at the Rfg1 site, respectively (Supplementary Figures S6A–H and Table 3). The H/BM frequencies for gmnnl1gmnnl1rfg1rfg1 were 0% (0/30) (p2×35Spro-Cas9-GmNNL1Rfg1) and 6.7% (2/30) (pAtGCSpro1178-Cas9-GmNNL1Rfg1), respectively (Supplementary Figures S6A–H and Table 3).
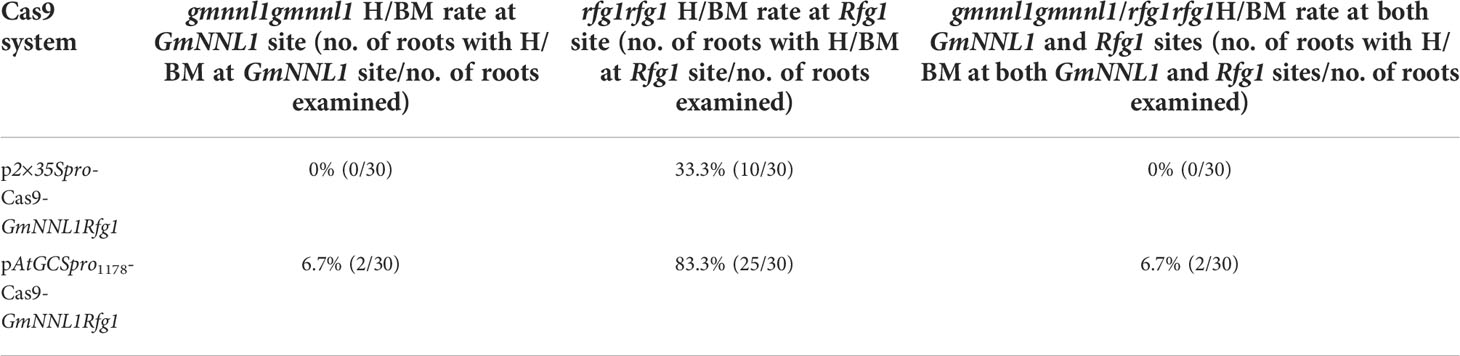
Table 3 Comparison of pAtGCSpro1178-Cas9-GmNNL1Rfg1 and p2×35Spro-Cas9-GmNNL1Rfg1 genome editing efficiency in soybean hairy roots.
In L. japonicus, two genomic target sites were analyzed, LjNLP4 (Suzuki et al., 2013; Nishida and Suzaki, 2018; Nishida et al., 2021) and LjSYMRK (Wang et al., 2016). Compared with p2×35Spro-Cas9-LjNLP4LjSYMRK, H/BM frequencies of the two LjNLP4 and LjSYMRK target sites were significantly increased from 30.0% (9/30) to 83.3% (25/30) at the LjNLP4 site, from 26.7% (8/30) to 83.3% (25/30) at the LjSYMRK site, when using pAtGCSpro1178-Cas9-LjNLP4LjSYMRK. The H/BM frequencies for ljnlp4ljnlp4ljsymrkljsymrk were 10.0% (3/30) (p2×35Spro-Cas9-LjNLP4LjSYMRK) and 66.7% (20/30) (pAtGCSpro1178-Cas9-LjNLP4LjSYMRK), respectively (Supplementary Figures S7A–G and Table 4).
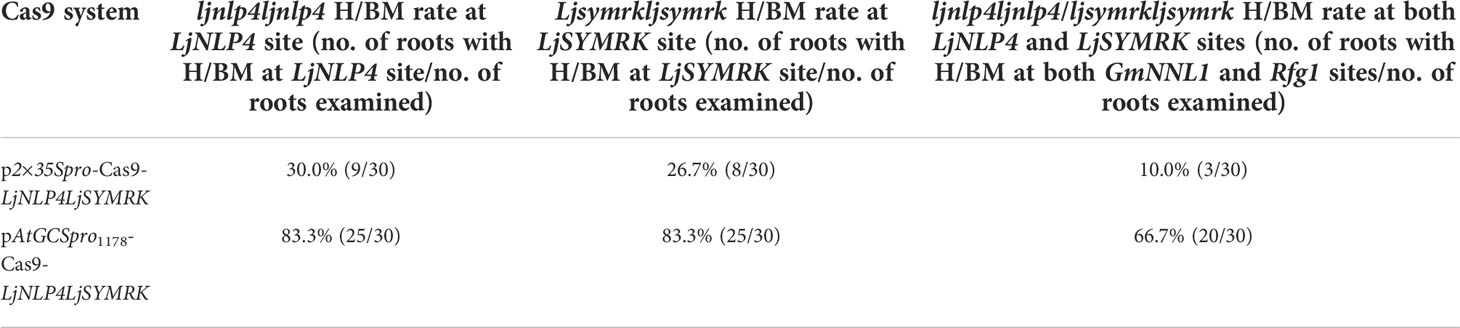
Table 4 Comparison of pAtGCSpro1178-Cas9-LjNLP4LjSYMRK and p2×35Spro-Cas9-LjNLP4LjSYMRK genome editing efficiency in L. japonicus hairy roots.
In tomato (Solanum lycopersicum), two gRNAs were designed to introduce mutations into the tomato endogenous gene TRYPTICHON (SlTRY, Solyc01g095640.1.1) (Tominaga-Wada et al., 2013). Consistent with these previous observations, using the AtGCSpro1178 promoter to direct Cas9 expression can lead to a higher H/BM induction rates. At the sgRNA1 target site, p2×35Spro-Cas9-SlTRY and pAtGCSpro1178-Cas9-SlTRY result in H/BM frequencies of 23.3% (7/30) and 91.3% (21/23), and at the sgRNA2 target site, 13.3% (4/30) and 78.3% (18/23), respectively. The H/BM induction rates for the two simultaneously targeted sites were 6.7% (2/30) (p2×35Spro-Cas9-SlTRY) and 65.2% (15/23) (pAtGCSpro1178-Cas9-SlTRY), respectively (Supplementary Figures S8A–D and Table 5).

Table 5 Comparison of pAtGCSpro1178-Cas9-SlTRY and p2×35Spro-Cas9-SlTRY genome editing efficiency in tomato hairy roots.
Based on the above results, the pAtGCSpro-Cas9 system always substantially enhances the H/BM-induced frequency over the p2×35Spro-Cas9 system in soybean, L. japonicus, and tomato. By using the pAtGCSpro-Cas9 system, we achieved an average H/BM frequency 8.3-fold (proportion of H/BM-induced frequency using pAtGCSpro1178-Cas9 system compared with that of the p2×35Spro-Cas9 system in soybean, L. japonicus and tomato) higher than the p2×35Spro-Cas9 system for two targeted site(s) in the genome.
Discussion
The pAtGCSpro-Cas9 system significantly improves H/BM efficiencies relative to the p2×35Spro-Cas9 system by ARM transformation
In Arabidopsis, AtGCS encodes the first enzyme of glutathione (GSH; 7-glutamylcys teinyl glycine) biosynthesis, γ-glutamylcysteine synthetase (7-GCS; EC 6.3.2.2; May and Leaver, 1994). AtGCSpro is involved in the control of mitosis cell cycle during the G1 to S phase and regulates the initiation and maintenance of cell division in the root apex (Vernoux et al., 2000). However, the promoter activity of AtGCS has not been reported. In this study, our results indicated that AtGCSpro had a high activity in the initiation emergence regions of hairy roots, later, in the root meristem, and in the developing roots. The pAtGCSpro-Cas9 system markedly improves H/BM efficiencies relative to the p2×35Spro-Cas9 system in soybean, L. japonicus, and tomato by ARM transformation. Combined the expression of AtGCSpro in this study with previous studies on the functions of that in specific cell cycle (Cheng et al., 1995; Vernoux et al., 2000), we reasoned that large numbers of Cas9-driven by AtGCSpro expressed in the roots during the G1-to-S phase and loosened chromatin DNA structure and single-strand DNA condition contribute Cas9’s cutting of the DNA strands to generate a DNA-strand break. In particular, single-strand chromatin DNA is subjected to cutting and is introduced to the mutations, which will result in homozygous mutants following cell mitosis cycles. Therefore, it is reasonable that the AtGCSpro-Cas9 system can produce higher H/BM rates than p2×35Spro-Cas9.
Previous studies have indicated that using the YAO promoter to drive Cas9 expression in CRISPR/Cas9 constructs leads to high-efficiency genome editing in Arabidopsis by A. tumefaciens–mediated genetic transformation (Yan et al., 2015; Feng et al., 2018). In contrast, in this study, the YAO promoter showed a much lower efficiency than the 2×35S promoter in driving Cas9 expression in soybean and L. japonicus roots mediated by ARM transformation (Supplementary Tables S2 and S3), despite high YAO promoter activity in roots (Fan et al., 2020a; Fan et al., 2020b). This might be because of the target of the T-DNA in ARM transformation but not the germline cells in A. tumefaciens–mediated stable genetic transformation in Arabidopsis. Additionally, AtGCSpro is higher efficient promoter in inducing H/BM, outperforming the constitutive expression promoter ubiquitin in both transgenic soybean and L. japonicus hairy roots (Supplementary Tables S2 and S3). Based on these results, we concluded that Cas9 expression timing and tissue specificity are crucial to the editing efficiency of the CRISPR/Cas9 system in ARM transformation.
The pAtGCSpro-Cas9 system indicates great potential in multiplex genome editing
Gene duplications are especially prevalent in plants, and the genomes of most extant angiosperm species result from a series of segmental or whole-genome duplication events. At least 70% of all angiosperms underwent at least one episode of polyploidization in their evolutionary history (Leitch and Bennett, 1997; Comai, 2000; Soltis and Soltis, 2000; Wendel, 2000; Qiao et al., 2019). Some species have undergone multiple occurrences of polyploidization in the coding portions of the genome, which are organized hierarchically into families or superfamilies. More than 50% of genes belong to gene family members in eukaryotes (Chervitz et al., 1998; Koonin et al., 1998; Semple and Wolfe, 1999; Thornton and DeSalle, 2000; Blanc and Wolfe, 2004; Xu et al., 2022). Many agriculturally important crops are polyploid plants, such as tetraploid potato (Solanum tuberosum), oilseed rape (Brassica napus), tobacco, cotton (Gossypium spp.), hexaploid bread wheat, sweet potato, and octoploid strawberry (Fragaria × ananassa) (Yang et al., 2017; Abe et al., 2019; Edger et al., 2019; Gao, 2021). To analyze the mutant phenotype(s), these duplicated genes with redundant functions must be simultaneously mutated to generate homozygous/multi-allelic changes for dominant target genes at alleles site. Using the pAtGCSpro-Cas9 system, the average H/BM frequency is 8.3-fold higher than the p2×35Spro-Cas9 system for two simultaneously targeted sites in the genome. Compared with targeting a single genomic site (1.7-fold increased), the efficiency of simultaneous homozygous/biallelic mutagenesis in a single event is significantly increased for targeting two genomic sites using the pAtGCSpro-Cas9 system. Therefore, with the increasing of genomic targeted sites, the H/BM frequency is more significantly increased using the pAtGCSpro-Cas9 system. The pAtGCSpro-Cas9 system provides a powerful tool for analyzing the loss-of-function phenotypes of duplicated genes in the diploid and polyploids plants for multiple genomic targeted editing.
In the traditional genetic transformation mediated by Agrobacterium, generating recessive change at multiple target sites is also very important. Although homozygous mutants can also be obtained from heterozygotes mutants at the sgRNA target site by plant self-crossing, a longer experiment was required, and it was laborious to screen and identify the homozygous mutants. Previous research has indicated that regeneration plants using the root or root tip as explants had been reported in some plants, such as L. japonicus (Lombari et al., 2003), tomato (Peres et al., 2001), Chicory (Matvieieva et al., 2011), and Medicago truncatula (Iantcheva et al., 2005). This suggests that the transgenic hairy roots with H/BM at multiple targeted sites could be used as explants to induce the regeneration plants in some plants. Bernard et al. (2019) reported that the edited hairy roots can be used for explants to generate the whole transgenic plant in chicory. The pAtGCSpro-Cas9 system is a greatly convenient for plant genetic engineering breeding involving the simultaneous alteration of multiple homoeologs with H/BM in the transformation of T0 generation. This is a promising technical breakthrough for accelerating plant breeding for simultaneous H/BM at multiple genome target sites to eliminate “deleterious” genes with establishing regeneration plants using the root or root tip as explants in some plant species. The pAtGCSpro-Cas9 system would propel plant breeding and accelerate the generation of homologous mutants with multiplexed genome modifications of homologous genes or gene families in a much shorter time than conventional breeding techniques. Additionally, the genotyping screening of H/BM will greatly reduce the working burden at multiple sites.
The conserved activity of AtGCSpro in eudicots species suggests that the AtGCSpro-Cas9 system might have much potential for generating high frequency H/BMs in a wide range of dicots plant species in ARM transformation
In this study, the AtGCSpro-Cas9 system always indicates notably increased homozygous/biallelic targeted mutation efficiency in selected species soybean, L. japonicus, and tomato tested than the p2×35Spro-Cas9 system, although the rates of H/BM-induced are different in different species at different target sites. Furthermore, AtGCSpro indicates a strong activity in broad eudicots species, such as soybean, tomato, cucumber, L. japonicus, tobacco, sweet potato, and cotton. The conserved activity of AtGCSpro in eudicots species suggests that the AtGCSpro-Cas9 system might induce higher H/BM in a wide range of dicots plant species in ARM transformation.
In this study, as expected, in the H/BM-induced mutants, we found that some homozygous/biallelic mutants with 3n indels at target site. As protein coding genes are read in units of three (codons), the 3n indels would result in only insert or delete 1 or several amino acids in the corresponding coding protein, and some homozygous/biallelic mutants with 3n indels (such as indels of 3bp, 6bp, 9bp, …) may not be loss-of-function mutants. The generated homozygous/biallelic mutants with non-3n indels at the dominant target gene site are required because they can produce complete loss-of function mutants. Therefore, it is crucial to establish a high-efficiency CRISPR/Cas9 system with higher H/BM mutation efficiency applied for ARM transformation because a certain ratios 3n indels mutants (randomly generated) are produced in the homozygous/biallelic mutants. Besides the AtGCS promoter-driven CRISPR/Cas9, in the future, H/BM efficiency may be improved by optimizing the AtGCSpro-Cas9 system, such as using plant endogenous GCS gene promoter to drive the Cas9 expression, endogenous U6 promoter-driven sgRNA, codon-optimized Cas9, tRNA for multiplexing, a modified sgRNA scaffold, and intronized Cas9 (Li et al., 2014; Dang et al., 2015; Xie et al., 2015; Grützner et al., 2020; Huang et al., 2022). For example, in soybean, the genome editing efficiency was increased by 1.8-fold to 6.3-fold when the GmU6-10 promoter drove the sgRNA expression by replacing the Arabidopsis AtU6-26 gene promoter with CRISPR/Cas9 (Sun et al., 2015). This study shows that the AtGCSpro-Cas9 system is a viable tool for use in inducing H/BMs in a wide scope of plant species in the ARM transformation.
Data availability statement
The original contributions presented in the study are included in the article/Supplementary Material. Further inquiries can be directed to the corresponding authors.
Author contributions
YF and SHL conceived and designed the experiments, and wrote the paper. SL, XW, QL, WP, ZZ, PC, and SG performed the work and analyzed data. All authors contributed to the article and approved the submitted version.
Funding
This work was supported by National Natural Science Foundation of China (no. 31271751), and partially funded by Natural Science Foundation of Shandong province (no. ZR2012CL16 and ZR2010CQ025) and Industrial Upgrading Project of Shandong Agricultural Science and Technology Park (2019YQ035).
Acknowledgments
We thank Prof. Zhe Yan (Northeast Institute of Geography and Agroecology, CAS, China) for kindly providing the L. japonicas seeds and strains Mesorhizobium loti MAFF303099, Prof. Yaoguang Liu and Letian Chen (South China Agricultural University) for pYLsgRNA-AtU3d and pYLCRISPR/Cas9Pubi-B, and Prof. Qijun Chen (China Agricultural University) for pHSE401.
Conflict of interest
The authors declare that the research was conducted in the absence of any commercial or financial relationships that could be construed as a potential conflict of interest.
Publisher’s note
All claims expressed in this article are solely those of the authors and do not necessarily represent those of their affiliated organizations, or those of the publisher, the editors and the reviewers. Any product that may be evaluated in this article, or claim that may be made by its manufacturer, is not guaranteed or endorsed by the publisher.
Supplementary material
The Supplementary Material for this article can be found online at: https://www.frontiersin.org/articles/10.3389/fpls.2022.952428/full#supplementary-material
Figure S1 | The schematic diagrams of the pRSE401.
Figure S2 | DsRed and AtMyb75 as visual reporter genes used for screening transgenic hairy roots in soybean, tomato, and L. japonicus, respectively. Hairy roots were induced from soybean (A-B), tomato (C-D), and L. japonicas (E-G), respectively. Sections A, C, E, and G were pictured under the bright light. DsRed fluorescence was observed in transgenic positive soybean (B), tomato (D), and L. japonicus (F) hairy roots, and purple/red anthocyannin in L. japonicus (G), respectively. Arrows indicated transgenic positive hairy roots. Bars=5 mm.
Figure S3 | Relative expression levels of GUS in the transformed with pRedGa1/2/3/4 soybean hairy roots by a qRT-PCR assay. Data were analyzed using Microsoft office Excel 2016 and Data Processing System (DPS) statistical software. The averages and standard deviations were calculated. ** represented very significantly different at the p=0.01 value given.
Figure S4 | Sanger sequencing chromatogram of analysis on the H/BM mutations at 15 Rj7 target site mediated by pAtGCSpro2411-Cas9-Rj7 system were given.
Figure S5 | Mutation at Rj7 target site mediated by pAtGCSpro1178-Cas9 and pAtGCSpro833-Cas9 system in soybean, respectively, by PCR-RE assays and Sanger sequencing analysis. PCR-RE assays mutation efficiency in the Rj7 target loci from different independent pAtGCSpro1178-Cas9 and pAtGCSpro833-Cas9 hairy roots, respectively (A, B). Lanes WT and WTE, undigested PCR amplification fragment and digested wild-type controls by EcoRI, respectively. Lanes 1-30, different independent transgenic hairy roots (A). Lane 1, 3-11, 13, 17-18, 20, 22-23, 25-28, and 30 were homozygous/ biallelic mutations (A). Lanes 1-20, different independent transgenic hairy roots (B). Lane 1, 3, 5-7, 12, 14, and 17-19 were homozygous/ biallelic mutations (B). An example of sequencing analysis on the mutation at Rj7 target site was given in pAtGCSpro1178-Cas9 and pAtGCSpro833-Cas9 system, respectively. Black arrows indicate the site of indels mutation. The PAM region and mutated target site are shown in the box (C).
Figure S6 | Mutation at GmNNL1Rfg1 target sites in soybean mediated by p2×35Spro-Cas9 and pAtGCSpro1178-Cas9 system, respectively, and PCR-RE assays and Sanger sequencing analysis. Sequence of an sgRNA designed to target a site within the fifth exon region of GmNNL1 (A) and Rfg1 (B), respectively. The HindIII (A) and NcoI (B) restriction site is underlined, respectively. The PAM sequence is highlighted in blue. PCR-RE analysis the targeted mutation at GmNNL1 site using HindIII restriction enzyme digestion. In the hairy roots transformed with the p2×35Spro-Cas9-GmNNL1Rfg1 vector, no homozygous or biallelic mutations lines were obtained among 30 independent transgenic lines (C). PCR-RE analysis the targeted mutation at Rfg1 site using NcoI restriction digestion. In the hairy roots transformed with the p2×35Spro-Cas9-GmNNL1Rfg1 vector, 41 10 lines (#5-7, #11, #14-17, #19, and #26) were homozygous or biallelic mutations among 30 independent transgenic lines (D). PCR-RE analysis the targeted mutation in GmNNL1 site in the hairy roots transformed with the pAtGCSpro1178-Cas9-GmNNL1Rfg1 vector using HindIII restriction enzyme digestion. 2 lines (#28, and #30) were homozygous or biallelic mutations among independent transgenic lines (E). PCR-RE analysis the targeted mutation at Rfg1 site in the hairy roots transformed with the pAtGCSpro1178-Cas9-GmNNL1Rfg1 vector using NcoI restriction enzyme digestion. 25 lines (#1-4, #7-11, #13-15, #17-28, and #30 ) were homozygous or biallelic mutations among 30 independent transgenic lines (F). An example of sequencing analysis on the mutation at GmNNL1 target site was given in pAtGCSpro1178-Cas9-GmNNL1Rfg1 and p2×35Spro-Cas9-GmNNL1Rfg1 system, respectively. WT represents wild type. Black arrows indicate the site of indels mutation (G). An example of sequencing analysis on the mutation at Rfg1 target site was given in pAtGCSpro1178-Cas9-GmNNL1Rfg1 and p2×35Spro-Cas9-GmNNL1Rfg1 system, respectively. Black arrows indicate the site of indels mutation (H).
Figure S7 | Mutation at LjNLP4LjSYMRK target sites in L. japonicus mediated by p2×35Spro-Cas9 and pAtGCSpro1178-Cas9 system, respectively, and PCR-RE assays and Sanger sequencing analysis. Sequence of an sgRNA designed to target a site within the first exon region of LjNLP4 (A) and within the thirteenth exon region of LjSYMRK (B), respectively. The BamHI (A) and PstI (B) restriction site is underlined, respectively. The protospaceradjacent motif (PAM) sequence is highlighted in blue. PCR-RE assays to detect CRISPR/Cas9-induced mutation in the LjNLP4 target loci using BamHI from 30 different independent p2×35Spro-Cas9 LjNLP4LjSYMRK (C) and pAtGCSpro1178-Cas9-LjNLP4LjSYMRK (D) hairy roots, respectively. 9 lines (#3, #4, #9, #10, #12, #17, #21, #24, and #29) were homozygous or biallelic mutations (C). 25 lines (#2-7, #9-15, #17-24, #26-28, and #30) were homozygous or biallelic mutations (D). PCR-RE assays to detect CRISPR/Cas9-induced mutation in the LjSYMRK target loci using PstI from 30 different independent p2×35Spro-Cas9-LjNLP4LjSYMRK (E) and pAtGCSpro1178-Cas9-LjNLP4LjSYMRK (F) hairy roots, respectively. 8 lines (#1, #4, #8, #12, #19, #22, #24, and #28) were homozygous or biallelic mutations (E). 25 lines (#1-6, #8-14, #16, #18-20, and #22-29) were homozygous or biallelic mutations (F). Several examples of sequencing analysis on the mutation at LjSYMRK target site was given in p2×35Spro-Cas9 and pAtGCSpro1178-Cas9 system, respectively (G).
Figure S8 | Mutation at SlTRY target sites in tomato mediated by p2×35Spro-Cas9 and pAtGCSpro1178-Cas9 system, respectively, and PCR-RE assays and Sanger sequencing analysis. Sequences of two sgRNA designed to target sites within the first and second exons region of SlTRY. The PAM sequence is highlighted in blue and the EcoRI restriction site is underlined (A). PCR-RE analysis the targeted mutation at SlTRY site using EcoRI restriction enzyme digestion. In the hairy roots transformed with the p2×35Spro-Cas9-SlTRY vector (B). PCR-RE analysis the targeted mutation at SlTRY site using EcoRI restriction digestion. In the hairy roots transformedwith the pAtGCSpro1178-Cas9-SlTRY vector (C). Several examples of Sanger sequencinganalysis on the mutation at SlTRY targeted sites were given in p2×35Spro-Cas9-SlTRY and pAtGCSpro1178-Cas9-SlTRY system, respectively (D).
Table S1 | All primer sequences used in this paper.
Table S2 | Comparison of pAtGCSpro1178-Cas9-Rj7, pUbiqutinpro-Cas9-Rj7, pYAOpro-Cas9-Rj7, and p2×35Spro-Cas9-Rj7 genome editing efficiency in soybean hairy roots.
Table S3 | Comparison of pAtGCSpro1178-Cas9-LjNLP4, pUbiqutinproCas9-LjNLP4, pYAOpro-Cas9-LjNLP4, and p2×35Spro-Cas9-LjNLP4 genome editing efficiency in L. japonicus hairy roots.
Abbreviations
ARM, Agrobacterium rhizogenes–mediated transformation; H/BM, Homozygous/Biallelic Mutations; AtGCSpro, gamma-glutamylcysteine synthetase gene promoter; AtGCSpro2411, AtGCSpro1977, AtGCSpro1629, AtGCSpro1178, AtGCSpro833, AtGCSpro with 2411 bp, 1977 bp, 1629 bp, 1178 bp, and 833 bp, respectively; 2×35Spro, double CaMV35S promoter; qRT-PCR, quantitative Real-time PCR; PCR/RE, Polymerase chain reaction/restriction enzyme.
References
Abe, F., Haque, E., Hisano, H., Tanaka, T., Kamiya, Y., Mikami, M., et al. (2019). Genome-edited triple recessive mutation alters seed dormancy in wheat. Cell Rep. 28, 1362–1369.e1364. doi: 10.1016/j.celrep.2019.06.090
Bernard, G., Gagneul, D., Santos, H. A. D., Etienne, A., Hilbert, J., Rambaud, C. (2019). Efficient genome editing using CRISPR/Cas9 technology in chicory. Int. J. Mol. Sci. 20, 1155. doi: 10.3390/ijms20051155
Blanc, G., Wolfe, K. H. (2004). Widespread paleopolyploidy in model plant species inferred from age distributions of duplicate genes. Plant Cell 16, 1667–1678. doi: 10.1105/tpc.021345
Cheng, J. C., Seeley, K., Sung, Z. R. (1995). RML1 and RML2, Arabidopsis genes required for cell proliferation at the root tip. Plant Physiol. 107, 365–376. doi: 10.2307/4276330
Chervitz, S. A., Aravind, L., Sherlock, G., Ball, C. A., Koonin, E. V., Dwight, S. S., et al. (1998). Comparison of the complete protein sets of worm and yeast: orthology and divergence. Science 282, 2022–2028. doi: 10.1126/science.282.5396.2022
Chilton, M. D., Tepfer, D. A., Petit, A., David, C., Casse-Delbart, F., Tempe, J. (1982). Agrobacterium rhizogenes inserts T-DNA into the genomes of the host plant root cells. Nature 295, 432–443. doi: 10.1038/295432a0
Comai, L. (2000). Genetic and epigenetic interactions in allopolyploid plants. Plant Mol. Biol. 43, 387–399. doi: 10.1007/978-94-011-4183-3_19
Dang, Y., Jia, G. X., Choi, J., Ma, H. M., Anaya, E., Ye, C. T., et al. (2015). Optimizing sgRNA structure to improve CRISPR-Cas9 knockout efficiency. Genome Biol. 16, 3. doi: 10.1186/s13059-015-0846-3
Edger, P. P., Poorten, T. J., VanBuren, R., Hardigan, M. A., Colle, M., McKain , M. R., et al. (2019). Origin and evolution of the octoploid strawberry genome. Nat. Genet. 51, 541–547. doi: 10.1038/s41588-019-0356-4
Eid, A., Ali, Z., Mahfouz, M. M. (2016). High efficiency of targeted mutagenesis in Arabidopsis via meiotic promoter-driven expression of Cas9 endonuclease. Plant Cell Rep. 35, 1555–1558. doi: 10.1007/s00299-016-2000-4
Fan, Y. L., Liu, J. G., Lyu, S. H., Wang, Q., Yang, S. M., Zhu, H. Y. (2017). The soybean Rfg1 gene restricts nodulation by Sinorhizobium fredii USDA193. Front. Plant Sci. 8, 1548. doi: 10.3389/fpls.2017.01548
Fan, Y. L., Wang, X. Y., Li, H. Y., Liu, S. R., Jin, L. S., Lyu, Y. Y., et al. (2020c). Anthocyanin, a novel and user-friendly reporter for convenient, non-destructive, low cost, directly visual selection of transgenic hairy roots in the study of rhizobia-legume symbiosis. Plant Methods 16, 94. doi: 10.1186/s13007-020-00638-w
Fan, Y. L., Xu, F. L., Zhou, H. Z., Liu, X. X., Yang, X. Y., Weng, K. X., et al. (2020b). A fast, simple, high efficient and one-step generation of composite cucumber plants with transgenic roots by Agrobacterium rhizogenes-mediated transformation. Plant Cell Tiss. Org. (PCTOC) 141, 207–216. doi: 10.1007/s11240-020-01781-x
Fan, Y. L., Zhang, X. H., Zhong, L. J., Wang, X. Y., Lyu, S. H. (2020a). One-step generation of composite soybean plants with transgenic roots by Agrobacterium rhizogenes-mediated transformation. BMC Plant Biol. 20 (1), 208. doi: 10.1186/s12870-020-02421-4
Feng, Z. Y., Zhang, Z. J., Hua, K., Gao, X. F., Mao, Y. F., Botella, J. R., et al. (2018). A highly efficient cell division-specific CRISPR/Cas9 system generates homozygous mutants for multiple genes in Arabidopsis. Int. J. Mol. Sci. 19 (12), 3925. doi: 10.3390/ijms19123925
Gao, C. X. (2021). Genome engineering for crop improvement and future agriculture. Cell 184 (6), 1621–1635. doi: 10.1016/j.cell.2021.01.005
Gao, X., Chen, J., Dai, X., Zhang, D., Zhao, Y. (2016). An effective strategy for reliably isolating heritable and Cas9-free Arabidopsis mutants generated by CRISPR/Cas9-mediated genome editing. Plant Physiol. 171 (3), 1794–1800. doi: 10.1104/pp.16.00663
Grützner, R., Martin, P., Horn, C., Mortensen, S., Cram, E. J., Lee-Parsons, C. W. T., et al. (2020). High-efficiency genome editing in plants mediated by a Cas9 gene containing multiple introns. Plant Commun. 2 (2), 100135. doi: 10.1016/j.xplc.2020.100135
Huang, X., Wang, Y., Wang, N. (2022). Highly efficient generation of canker-resistant sweet orange enabled by an improved CRISPR/Cas9 system. Front. Plant Sci. 12. doi: 10.3389/fpls.2021.769907
Hua, K., Zhang, J. S., Botella, R., Ma, C. L., Kong, F. J., Liu, B. H., et al. (2019). Perspectives on the application of genome-editing technologies in crop breeding. Mol. Plant 12 (8), 1047–1059. doi: 10.1016/j.molp.2019.06.009
Iantcheva, A., Slavov, S., Prinsen, E., Vlahova, M., van Onckelen, H., Atanassov, A. (2005). Embryo induction and regeneration from root explants of Medicago truncatula after osmotic pre-treatment. Plant Cell Tiss. Organ Cult. (PCTOC) 81, 37–43. doi: 10.1007/s11240-004-2774-x
Irigoyen, S., Ramasamy, M., Pant, S., Niraula, P., Bedre, R., Gurung, M., et al. (2020). Plant hairy roots enable high throughput identification of antimicrobials against Candidatus liberibacter spp. Nat. Commun. 11, 5802. doi: 10.1038/s41467-020-19631-x
Jenei, S., Tiricz, H., Szolomájer, J., Tímár, E., Klement, É., Bouni, M. A. A., et al. (2020). Potent chimeric antimicrobial derivatives of the Medicago truncatula NCR247 symbiotic peptide. Front. Microbiol. 11, 270. doi: 10.3389/fmicb.2020.00270
Koonin, E. V., Tatusov, R. L., Galperin, M. Y. (1998). Beyond complete genomes: from sequence to structure and function. Curr. Opin. Struct. Biol. 8, 355–363. doi: 10.1016/s0959-440x(98)80070-5
Lei, Y., Lu, L., Liu, H. Y., Li, S., Xing, F., Chen, L. L. (2014). CRISPR-p: a web tool for synthetic single-guide RNA design of CRISPR-system in plants. Mol. Plant 7, 1494–1496. doi: 10.1093/mp/ssu044
Leitch, L. J., Bennett, M. D. (1997). Polyploidy in angiosperms. Trends Plant Sci. 2, 470–476. doi: 10.1016/s1360-1385(97)01154-0
Li, H. J., Liu, N. Y., Shi, D. Q., Liu, J., Yang, W. C. (2010). YAO is a nucleolar WD40-repeat protein critical for embryogenesis and gametogenesis in Arabidopsis. BMC Plant Biol. 10, 169. doi: 10.1186/1471-2229-10-169
Lin, Y. H., Ferguson, B. J., Kereszt, A., Gresshoff, P. M. (2010). Suppression of hypernodulation in soybean by a leaf-extracted, NARK- and nod factor-dependent, low molecular mass fraction. New Phytol. 185, 1074–1086. doi: 10.1111/j.1469-8137.2009.03163.x
Li, J. F., Zhang, D., Sheen, J. (2014). Cas9-based genome editing in Arabidopsis and tobacco. Methods Enzymol. 546, 459–472. doi: 10.1016/B978-0-12-801185-0.00022-2
Lombari, P., Ercolano, E., Alaoui, H. E., Chiurazzi, M. (2003). A new transformation-regeneration procedure in the model legume Lotus japonicus: root explants as a source of large numbers of cells susceptible to Agrobacterium-mediated transformation. Plant Cell Rep. 21, 771–777. doi: 10.1007/s00299-003-0576-y
Lü, S. H., Fan, Y. L., Liu, L. K., Liu, S. J., Zhang, W. H., Meng, Z. (2010). Ectopic expression of TrPI, a Taihangia rupestris (Rosaceae) PI ortholog, causes modifications of vegetative architecture in Arabidopsis. J. Plant Physiol. 167 (18), 1613–1621. doi: 10.1016/j.jplph.2010.06.028
Mao, Y. F., Zhang, Z. J., Feng, Z. Y., Wei, P. L., Zhang, H. (2016). Development of germ-line-specific CRISPR-Cas9 systems to improve the production of heritable gene modifications in Arabidopsis. Plant Biotechnol. J. 14, 519–532. doi: 10.1111/pbi.12468
Matvieieva, N. A., Kishchenko, O. M., Potrochov, A. O., Shakhovsky, A. M., Kuchuk, M. V. (2011). Regeneration of transgenic plants from hairy roots of Cichorium intybus l. var. Foliosum hegi. Cytol. Genet. 45 (5), 11–16. doi: 10.3103/S0095452711050082
May, M. J., Leaver, C. J. (1994). Arabidopsis thaliana γ-glutamylcysteine synthetase is structurally unrelated to mammalian, yeast, and Escherichia coli homologs. Proc. Natl. Acad. Sci. U.S.A. 91, 10059–10063. doi: 10.1073/pnas.91.21.10059
Ma, X., Zhang, Q., Zhu, Q., Liu, W., Chen, Y., Qiu, R., et al. (2015). A robust CRISPR/Cas9 system for convenient high-efficiency multiplex genome editing in monocot and dicot plants. Mol. Plant 8 (8), 1274–1284. doi: 10.1016/j.molp.2015.04.007
Ma, X. L., Zhu, Q. L., Chen, Y. L., Liu, Y. G. (2016). CRISPR/Cas9 platforms for genome editing in plants: developments and applications. Mol. Plant 9, 961–974. doi: 10.1016/j.molp.2016.04.009
Nishida, H., Nosaki, S., Suzuki, T., Ito, M., Miyakawa, T., Nomoto, M., et al. (2021). Different DNA-binding specificities of NLP and NIN transcription factors underlie nitrate-induced control of root nodulation. Plant Cell 33 (7), 2340–2359. doi: 10.1093/plcell/koab103
Nishida, H., Suzaki, T. (2018). Two negative regulatory systems of root nodule symbiosis: How are symbiotic benefits and costs balanced? Plant Cell Physiol. 59, 1733–1738. doi: 10.1093/pcp/pcy102
Okamoto, S., Yoro, E., Suzaki, T., Kawaguchi, M. (2013). Hairy root transformation in Lotus japonicas. BioProtocol 3 (12), 10.21769/BioProtoc.795. doi: 10.21769/BioProtoc.795
Peres, L. E. P., Morgante, P. G., Vecchi, C., Kraus, J. E., van Sluys, M. A. (2001). Shoot regeneration capacity from roots and transgenic hairy roots of tomato cultivars and wild related species. Plant Cell Tiss. Organ Cult. (PCTOC) 65 (1), 37–44. doi: 10.1023/A:1010631731559
Plasencia, A., Soler, M., Dupas, A., Ladouce, N., Silva-Martins, G., Martinez, Y., et al. (2016). Eucalyptus hairy roots, a fast, efficient and versatile tool to explore function and expression of genes involved in wood formation. Plant Biotechnol. J. 14 (6), 1381–1393. doi: 10.1111/pbi.12502
Qiao, X., Li, Q., Yin, H., Qi, K., Li, L., Wang, R., et al. (2019). Gene duplication and evolution in recurring polyploidization-diploidization cycles in plants. Genome Biol. 20, 38. doi: 10.1186/s13059-019-1650-2
Searle, I. R., Men, A. E., Laniya, T. S., Buzas, D. M., Iturbe-Ormaetxe, I., Carroll, B. J., et al. (2003). Long-distance signaling in nodulation directed by a CLAVATA1-like receptor kinase. Science 299, 109–112. doi: 10.1126/science.1077937
Semple, C., Wolfe, K. H. (1999). Gene duplication and gene conversion in the Caenorhabditis elegans genome. J. Mol. Evol. 48, 555–564. doi: 10.1007/pl00006498
Soltis, P. S., Soltis, D. E. (2000). The role of genetic and genomic attributes in the success of polyploids. Proc. Natl. Acad. Sci. USA 97, 7051–7057. doi: 10.1073/pnas.97.13.7051
Sun, X. J., Hu, Z., Chen, R., Jiang, Q. Y., Song, G. H., Zhang, H., et al. (2015). Targeted mutagenesis in soybean using the CRISPR-Cas9 system. Sci. Rep. 5, 10342. doi: 10.1038/strep10342
Suzuki, W., Konishi, M., Yanagisawa, S. (2013). The evolutionary events necessary for the emergence of symbiotic nitrogen fixation in legumes may involve a loss of nitrate responsiveness of the NIN transcription factor. Plant Signal. Behav. 8, e25975. doi: 10.4161/psb.25975
Tang, F., Yang, S., Liu, J., Zhu, H. (2016). Rj4, a gene controlling nodulation specificity in soybeans, encodes a thaumatin-like protein but not the one previously reported. Plant Physiol. 170, 26–32. doi: 10.1104/pp.15.01661
Thornton, J. W., DeSalle, R. (2000). Gene family evolution and homology: genomics meets phylogenetics. Annu. Rev. Genom. Hum. G. 1, 41. doi: 10.1146/annurev.genom.1.1.41
Tominaga-Wada, R., Nukumizu, Y., Sato, S., Wada, T. (2013). Control of plant trichome and root-hair development by a tomato (Solanum lycopersicum) R3 MYB transcription factor. PloS One 8 (1), e54019. doi: 10.1371/journal.pone.0054019
Tsutsui, H., Higashiyama, T. (2017). pKAMA-ITACHI vectors for highly efficient CRISPR/Cas9-mediated gene knockout in Arabidopsis thaliana. Plant Cell Physiol. 58 (1), 46–56. doi: 10.1093/pcp/pcw191
Vernoux, T., Wilson, R. C., Seeley, K. A., Reichheld, J., Muroy, S., Brown, S., et al. (2000). The root ROOTMERISTEMLESS1/CADMIUM SENSITIVE2 gene defines a glutathione-dependent pathway involved in initiation and maintenance of cell division during postembryonic root development. Plant Cell 12, 97–109. doi: 10.1105/tpc.12.1.97
Wang, L. X., Wang, L. L., Tan, Q., Fan, Q. L., Zhu, H., Hong, Z. L., et al. (2016). Efficient inactivation of symbiotic nitrogen fixation related genes in L. japonicus using CRISPR-Cas9. Front. Plant Sci. 7, 1333. doi: 10.3389/fpls.2016.01333
Wang, Z. P., Xing, H. L., Dong, L., Zhang, H. Y., Han, C. Y., Wang, X. C., et al. (2015). Egg cell-specific promoter-controlled CRISPR/Cas9 efficiently generates homozygous mutants for multiple target genes in Arabidopsis in a single generation. Genome Biol. 16, 144. doi: 10.1186/s13059-015-0715-0
Wang, Q., Yang, S. M., Liu, J., Terecskei, K., Ábrahám, E., Gombár, A., et al. (2017). Host-secreted antimicrobial peptide enforces symbiotic selectivity in Medicago truncatula. Proc. Natl. Acad. Sci. U.S.A. 114, 6854–6859. doi: 10.1073/pnas.1700715114
Wendel, J. F. (2000). Genome evolution in polyploids. Plant Mol. Biol. 42, 225–249. doi: 10.1023/A:1006392424384
Xie, K., Minkenberg, B., Yang, Y. (2015). Boosting CRISPR/Cas9 multiplex editing capability with the endogenous tRNA-processing system. Proc. Natl. Acad. Sci. U.S.A. 2015, 3570–3575. doi: 10.1073/pnas.1420294112
Xing, H. L., Dong, L., Wang, Z. P., Zhang, H. Y., Han, C. Y., Liu, B., et al. (2014). A CRISPR/Cas9 toolkit for multiplex genome editing in plants. BMC Plant Biol. 14 (1), 327. doi: 10.1186/s12870-014-0327-y
Xu, Y., Zhang, H., Zhong, Y., Jiang, N., Zhong, X., Zhang, Q., et al. (2022). Comparative genomics analysis of bHLH genes in cucurbits identifies a novel gene regulating cucurbitacin biosynthesis. Hortic. Res. 9, uhac038. doi: 10.1093/hr/uhac038
Yang, J., Moeinzadeh, M. H., Kuhl, H., Helmuth, J., Xiao, P., Haas, S., et al. (2017). Haplotype-resolved sweet potato genome traces back its hexaploidization history. Nat. Plants 3, 696–703. doi: 10.1038/s41477-017-0002-z
Yang, S., Wang, Q., Fedorova, E., Liu, J. G., Qin, Q. L., Zheng, Q. L., et al. (2017). Microsymbiont discrimination mediated by a host-secreted peptide in Medicago truncatula. Proc. Natl. Acad. Sci. U.S.A. 114, 6848–6853. doi: 10.1073/pnas.1700460114
Yan, L. H., Wei, S. W., Wu, Y. R., Hu, R. L., Li, H. J., Yang, W. C., et al. (2015). High-efficiency genome editing in Arabidopsis using YAO promoter-driven CRISPR/Cas9 system. Mol. Plant 8, 1820–1823. doi: 10.1016/j.molp.2015.10.004
Zhang, H. L., Cao, Y. P., Zhang, H., Xu, Y., Zhou, C., Liu, W. W., et al. (2020). Efficient generation of CRISPR/Cas9-mediated homozygous/biallelic Medicago truncatula mutants using a hairy root system. Front. Plant Sci. 11, 294. doi: 10.3389/fpls.2020.00294
Zhang, M., Liu, S., Wang, Z., Yuan, Y., Zhang, Z., Liang, Q., et al. (2022). Progress in soybean functional genomics over the past decade. Plant Biotechnol. J. 20, 256–282. doi: 10.1111/pbi.13682
Keywords: CRISPR/Cas9, agrobacterium rhizogenes–mediated transformation (ARM), genome editing, homozygous/biallelic mutation, hairy root, gamma-glutamylcysteine synthetase gene
Citation: Liu S, Wang X, Li Q, Peng W, Zhang Z, Chu P, Guo S, Fan Y and Lyu S (2022) AtGCS promoter-driven clustered regularly interspaced short palindromic repeats/Cas9 highly efficiently generates homozygous/biallelic mutations in the transformed roots by Agrobacterium rhizogenes–mediated transformation. Front. Plant Sci. 13:952428. doi: 10.3389/fpls.2022.952428
Received: 25 May 2022; Accepted: 22 September 2022;
Published: 18 October 2022.
Edited by:
Goetz Hensel, Heinrich Heine University Düsseldorf, GermanyReviewed by:
Anshu Alok, University of Minnesota Twin Cities, United StatesXiaoen Huang, University of Florida, United States
Copyright © 2022 Liu, Wang, Li, Peng, Zhang, Chu, Guo, Fan and Lyu. This is an open-access article distributed under the terms of the Creative Commons Attribution License (CC BY). The use, distribution or reproduction in other forums is permitted, provided the original author(s) and the copyright owner(s) are credited and that the original publication in this journal is cited, in accordance with accepted academic practice. No use, distribution or reproduction is permitted which does not comply with these terms.
*Correspondence: Yinglun Fan, fanyinglun@lcu.edu.cn; Shanhua Lyu, lvshanhua@lcu.edu.cn; lvshanhua@foxmail.com
†These authors have contributed equally to this work and share senior authorship
‡ORCID: Shanhua Lyu, orcid.org/0000-0002-1895-3614