- 1State Key Laboratory for Rice Biology, China National Rice Research Institute, Chinese Academy of Agricultural Sciences, Hangzhou, China
- 2Agricultural Genomics Institute at Shenzhen, Chinese Academy of Agricultural Sciences, Shenzhen, China
- 3Department of Agronomy, Khulna Agricultural University, Khulna, Bangladesh
- 4Agricultural Genetic Engineering Research Institute, Giza, Egypt
Soil salinity poses a serious threat to the sustainable production of rice (Oryza sativa L.) throughout the world. Thus, the detection of loci and alleles responsible for salt tolerance is fundamental to accelerating the improvement of rice and producing the resilient varieties that will ensure future harvests. In this study, we collected a set of 191 mini-core rice populations from around the world, evaluated their salt tolerance based on plant growth and development phenotypes at the seedling stage, and divided a standard evaluation score (SES) of visual salt injury into five different grades. We used ∼3.82 million single nucleotide polymorphisms (SNPs) to identify 155 significant SNPs and 275 genes associated with salt sensitivity based on a genome-wide association study (GWAS) of SES. In particular, two candidate genes, ZFP179 and OsDSR2, were associated with salt tolerance, and OsHKT1;1 was co-detected in the entire GWAS of all the panels and indica. Additionally, we investigated the transcriptional changes in cultivars 93-11 and PA64s under normal and salinity stress conditions and found 517 co-upregulated and 223 co-downregulated genes. These differentially expressed genes (DEGs) were highly enriched in “response to chemical” and “stress” based on the gene ontology enrichment analysis. Notably, 30 candidate genes that were associated with the salt tolerance analysis were obtained by integrating GWAS and transcriptomic DEG analyses, including 13 cloned genes that had no reports of tolerance to salt and 17 candidate genes whose functions were unknown. To further explore these genes and their alleles, we performed haplotype analysis, genome-wide domestication detection, and transcriptome analysis to breed improved varieties. This data and the genetic resources provided will be valuable for the development of salt tolerant rice varieties.
Introduction
The levels of salinization in the soil are increasing significantly owing to improper irrigation and limited freshwater supplies. Soil salinity inhibits plant growth and development, metabolic changes, and ion sequestration and exclusion (Chen et al., 2018; Van Zelm et al., 2020). Consequently, salinization of the soil represents a considerable abiotic stress for agricultural production and the ecological environment, limits the utilization of arable land, and thus constitutes a global problem that represents the primary source of agricultural crisis in the world (Bailey-Serres et al., 2019; Hassani et al., 2020). In light of this, the utilization of naturally evolved traits and population genetics to accelerate crop improvement and develop resilient production systems is necessary to ensure the viability of future harvests.
Rice (Oryza sativa L.) is one of three major food crops and the main source of nutrients for the global human population; it plays a significant role in facilitating economic development and maintaining national security (Chen et al., 2022). In addition, rice is the first choice to improve and utilize coastal areas and saline-alkali land (Yeo and Flowers, 1986). Despite the prominent increase in the production of rice, we currently face an enormous challenge to enhance the salt tolerance of rice and increase the yield of this crop on salinized agricultural land (Bhatt et al., 2020). Thus, the study of the molecular mechanisms behind salinity resistance is crucial for cultivating new varieties of rice.
Plants have evolved complex and interconnected regulatory networks that enable them to respond and adapt to different environments to withstand salinity stress. For example, sodium (Na+) transport and accumulation in plant cells are important for enhancing salt tolerance. This is accomplished by transporters that are responsible for Na+ uptake, export and compartmentation. Previous studies demonstrated that the Salt Overly Sensitive (SOS) signaling pathway mediates Na+ exclusion (Zhu, 2001). The High affinity K+ transporter (HKT)-type transporters are involved in Na+ transport (Berthomieu et al., 2003; Hamamoto et al., 2015), and the NHX (Na+/H+ antiporter)-type transporters facilitate the compartmentation of Na+ (Blumwald and Poole, 1985). Studies in Arabidopsis have resulted in significant progress toward understanding the function of SOSs (Quintero et al., 2011; Gong et al., 2020). The SOS functional module is conserved and comprises the SOS1, SOS2, and SOS3 proteins that operate in cereals and possess a high degree of structural conservation between dicots and monocots (Quintero et al., 2002; Martínez-Atienza et al., 2007). In a similar fashion to AtSOS1, the OsSOS1 plasma membrane Na+/H+ exchanger also plays a substantial role in controlling Na+ uptake and root-shoot partitioning to confer higher salt tolerance to rice plants (El Mahi et al., 2019). Members of the NHX family are equally conserved and important for transporting intercellular potassium (K+) and maintaining the pH of endomembrane during salt stress (Van Zelm et al., 2020). In Arabidopsis, plants that overexpress NHX1 display increased tolerance to salt and accumulate Na+ in their shoots under conditions of high salinity (Apse et al., 1999). NHXs also transport K+ and Na+ in crop plants, which has demonstrated relevance for salt tolerance (Almeida et al., 2017). However, the associated haplotypes within these genes remain unknown.
Unlike SOSs and NHXs, Arabidopsis encodes only one HKT gene (AtHKT1;1). This contrasts with the multiple copies of HKT-type transporters found in monocot species, such as rice, wheat (Triticum aestivum L.) and barley (Hordeum vulgare L.) (Hamamoto et al., 2015). In particular, seven functional HKT-type transporters are present in rice, and they encompass two classes (I and II) based on their respective transport activity (Platten et al., 2006). Previous studies reported that rice plants require OsHKT1;1, a member of class I, to adapt to salinity stress by reducing the accumulation of Na+ in the shoots (Wang et al., 2015). Additionally, a cross between Nona Bokra, a salt tolerant indica variety, and Koshihikari, a susceptible japonica variety, made it possible to map an essential QTL designated SKC1 (Shoot K+ Content) that encodes another member of the HKT family and showed that OsHKT1;5 mediates the accumulation of K+ and Na+ in the shoots and xylem sap of rice plants (Ren et al., 2005). Among the HKT gene family, OsHKT1;5 is an ortholog of AtHKT1;1, which primarily detoxifies elevated Na+ levels to confer salt tolerance to both Arabidopsis and rice (Garciadeblás et al., 2003). Unlike class I HKT transporters, such as OsHKT1;1 and OsHKT1;5, there is little evidence on the physiological functions of class II genes in plants except for the OsHKT2;1-mediated influx of Na+ (Horie et al., 2007; Park et al., 2019; Wei et al., 2021a). Additionally, Takagi et al. (2015) used the MutMap method to identify OsRR22, a gene responsible for salt tolerance in rice, which allowed the researchers to develop a salt tolerant variety. These data showed that OsRR22 regulates the transcription of many salt response genes, such as OsHKT1;1 (Takagi et al., 2015). Although it is well established that HKT proteins play a major role in the salt tolerance of cereal crops, the distinct evolutionary pathways between varieties and the optimal haplotypes remain unknown.
Various transcription factor families, such as MYB, WRKY, and NAC, are also involved in responses to salt stress. In particular, NAC transcription factors are among the largest transcription regulatory gene families in plants, and their members play a vital role in response to abiotic stress (Jeong et al., 2013). Previous studies (Wang et al., 2012b; Yuan et al., 2020) showed that OMTN proteins have characteristics that are typical of NAC transcriptional factors, including the overexpression of OMTN2, OMTN3, OMTN4, and OMTN6, which leads to drought sensitivity at the reproductive stage, and changes in the expression of several genes related to stress responses (Fang et al., 2014).
The advent of genome-wide association studies (GWAS) emerged as a powerful strategy to uncover the molecular basis of salt tolerance in rice. GWAS studies can identify more historical recombination, alleles, and wider genetic variation than traditional linkage analysis (Min et al., 2021). With respect to the analysis of genetic variation in different rice accessions, previous studies suggested that wild rice represents an important model for studying salinity resistance, which was preserved by natural selection, and has the potential to improve cultivated rice (Yuan et al., 2020). Using high density SNPs of the rice population to perform GWAS is an available way to identify salt-tolerance genes. Seven genes were identified under salinity stress conditions through GWAS analysis (Liu et al., 2019). Similarly, 295 rice accessions were used to perform GWAS of salt tolerance analysis during the germination stage, and 17 salt-tolerance-related genes were characterized (Yu et al., 2018). Therefore, it is critical to study the extensive genetic diversity of wild rice to identify valuable genes that are involved in salt stress tolerance. Additionally, rice domestication could provide a reliable basis to identify favorable variants for modern breeding (Meyer et al., 2016). In our previous studies, we analyzed the phenotype of 225 rice variety population under normal and low nitrogen conditions and conducted GWAS analysis to obtain four candidate genes (Lv et al., 2021). In this study, we analyzed the salt resistance of 191 rice varieties, performed a GWAS analysis, and combined this information with evaluation scoring results for salt tolerance, genetic differentiation and transcriptomic analysis to identify the genetic underlying basis for salt resistance in rice. Notably, we isolated 275 candidate genes associated with salt tolerance, including the known salt genes OsHKT1;1 and SLR1, which highlighted the reliability and accuracy of our methods. Simultaneously, we conducted haplotype and genetic diversity analyses to explore the predicted salt tolerant genes and the dominant haplotypes to improve rice varieties. The data and genetic resources of this study are highly valuable for correctly characterizing the molecular basis of salt tolerance in rice.
Materials and Methods
Plant Materials
The mini-core population used in this study consisted of 184 Oryza sativa accessions obtained from the 3,010 Rice Genomes Project (Wang et al., 2018). Seven Oryza sativa accessions were provided by Wang et al. (2020). These germplasms originated from 27 different countries (Supplementary Table 1).
NaCl Treatment and Phenotypic Analysis
Hydroponic experiments were performed at the greenhouse of the China National Rice Research Institute in Hangzhou, China, during the summer of 2021. The grains were germinated in water at 37°C in the dark for 3 days. We examined the effects of salt stress in rice at the seedling stage and set different levels of NaCl concentration (50 mM NaCl, 100 mM NaCl, 200 mM NaCl) for pre-experiments. Descriptive statistics of the phenotypes related to salt tolerance at the seedling stage of 191 rice accessions are presented in Supplementary Table 2. A wide range of phenotype values were observed under 100 mM NaCl solution in the salt traits evaluation, which resulted in the most diverse phenotypic distribution and facilitated discrimination of accessions with different salt tolerance levels. The seedlings were cultured in a nutrient solution (pH 5.5–5.8) with the following composition (Peethambaran et al., 2018): 1.425 NH4NO3, 0.42 NaH2PO4, 0.510 K2SO4, 0.998 CaCl2, 1.643 MgSO4, 0.168 Na2SiO3, 0.125 Fe-EDTA, 0.019 H3BO3, 0.009 MnCl2, 0.155 CuSO4, 0.152 ZnSO4, and 0.075 Na2MoO4. The solutions were changed every 2 days. After 2 weeks of hydroponic culture, 48 plants per line of the 14-day-old seedlings with uniform growth were exposed to a 100 mM solution of NaCl as the salt stress treatment, while the remaining 48 plants per line with uniform growth were cultured in a normal solution for an additional 14 days as the control (Jahan et al., 2020, 2021). The whole experiment was conducted in a randomized complete block design with two biological replications, and all of the tested samples were efficient.
Ten seedlings of each accession were evaluated for salt tolerance grades, and five salt tolerance indices (1, 3, 5, 7, and 9) were divided based on plant growth and development phenotypes after salt treatment (Gregorio et al., 1997). This scoring discriminated the susceptible from the tolerant and moderately tolerant genotypes. Plants with lower salt tolerance scores displayed visual symptoms that manifested as brown leaf tips, yellowing leaves, dry leaves, reduced shoot growth, and stunted height. These phenotypes increased with the time of stress.
Genome-Wide Association Study
The sequence data rice accessions used for GWAS were obtained from the 3,000 Rice Genomes Project (Wang et al., 2018). The SNP data were filtered with the following criteria: minor allele frequency (MAF) >0.05 (Visscher et al., 2012) and missing rate ≤30% (Yu et al., 2021). The linkage disequilibrium (LD) parameter r2 between pairwise SNPs was calculated using PopLDdecay. The physical LD decay distance was estimated as the position where r2 dropped to half of its maximum value. We estimated an LD decay distance of 86.8 kb. The efficient mixed model analysis feature of the EMMA eXpedited (EMMAX) software was utilized for GWAS analysis. The significance threshold was calculated using the formula “−log10 (1/the effective number of independent SNPs).” The threshold was set at −log P = 5 to identify significant association signals (Gao et al., 2020; Lv et al., 2021), and the candidate genes were detected as those within 200 kb of the significant association signals (Yu et al., 2017) using a mixed linear model (MLM) model (Kang et al., 2010). Plots that represented the GWAS results (Manhattan and Quantile-Quantile plots) were generated using the package qqman in R 3.4.2 (Turner, 2014). Annotations were added to the filtered VCF files using SnpEff software, and missense variant sites were selected for haplotype analysis of the target gene as the result.
Data Samples and Single Nucleotide Polymorphism Calling for Genetic Differentiation
A total of 159 indica, 146 temperate japonica, and 41 tropical japonica varieties, as well as 53 Oryza rufipogon, 163 Oryza glaberrima, and 83 Oryza barthii samples, were obtained from previous studies (Huang et al., 2012b; Cubry et al., 2018; Wang et al., 2018). The raw Illumina short reads were filtered using Trimmomatic 0.36 (Bolger et al., 2014). The clean reads were mapped to the Nipponbare reference genome (MSUv7) (Kawahara et al., 2013) using BOWTIE2 v2.2.1 (Langmead and Salzberg, 2012) with default parameters. SAMtools v1.8 was used for SNP calling, and the SNPs were filtered using VCFtools (Danecek et al., 2021) with the following parameters: “–maf 0.001 –max-missing 0.9 –remove-indels –min-alleles 2 –max-alleles 2.”
Population Differentiation Statistics
The population differentiation statistics (FST) between wild and indica, wild and japonica, and O. glaberrima and O. barthii were estimated separately for each window using VCFtools (Danecek et al., 2011). FST was calculated with the following parameters: “–fst-window-size 100,000 –fst-window-step 10,000.” Sliding windows with the top 5% of FST values were identified as divergent windows. Genes that overlapped the FST divergent windows were annotated based on the MSUv7 annotation (Kawahara et al., 2013).
RNA-Seq
The cultivars 93-11 and PA64s, as well as their salt tolerant plants (based on phenotyping results), were selected for RNA-Seq analysis (Accession: PRJNA831421)1. Total RNA was extracted with TRIzol, and the RNA-Seq libraries were prepared using two biological replicates for each species. After filtering out non-conforming sequences with Trimmomatic (0.36) (Bolger et al., 2014), TopHat2 (v2.0.12) (Trapnell et al., 2012) was used to align the cleaned data to the reference genome (Kawahara et al., 2013). The default parameters in the Cufflinks (v2.2.1) were used to obtain the gene expression level (Fragments per kilobase per million, FPKM) of each gene (Trapnell et al., 2012). Meanwhile, genes with P-value < 0.05, Q-value < 0.05 and FPKM difference greater than 1.5 times between the control group and the treatment group obtained by Cuffdiff (v2.2.1) were regarded as differentially expressed genes (DEGs). TBtools (v1.098723) (Chen et al., 2020) was applied to map the DEGs of the GO database2, and it was also be used to calculate the number of genes in each term to obtain a list of genes with a certain GO function and the statistics of the number of genes. The differential genes of GO item species with significantly enriched differential genes and P-value < 0.05 will be retained for analysis.
Results
Geographic Distribution and Population Structure
The different accessions used in this study are shown in Figure 1. A total of 191 O. sativa accessions were collected from 27 different regions of the world (Figure 1A), which represents a large variation of geographical origins and genetic diversity for the accessions of cultivated rice. A principal component analysis (PCA) was performed with the high-quality SNPs/indels to mine the population structure in the rice accessions. Clear subpopulation structures were observed, which resulted in three subpopulations designated indica and japonica with the admixture accessions located between the two groups (Figure 1B). The resulting neighbor-joining tree showed consistency with the PCA analysis and identified 129 indica, 57 japonica, and five intermediate type accessions, which largely supported the classification of the 191 accessions (Figure 1C). There were five intermediate accessions, which could have resulted from occasional historical hybrids between indica and japonica that experienced partial reproductive isolation.
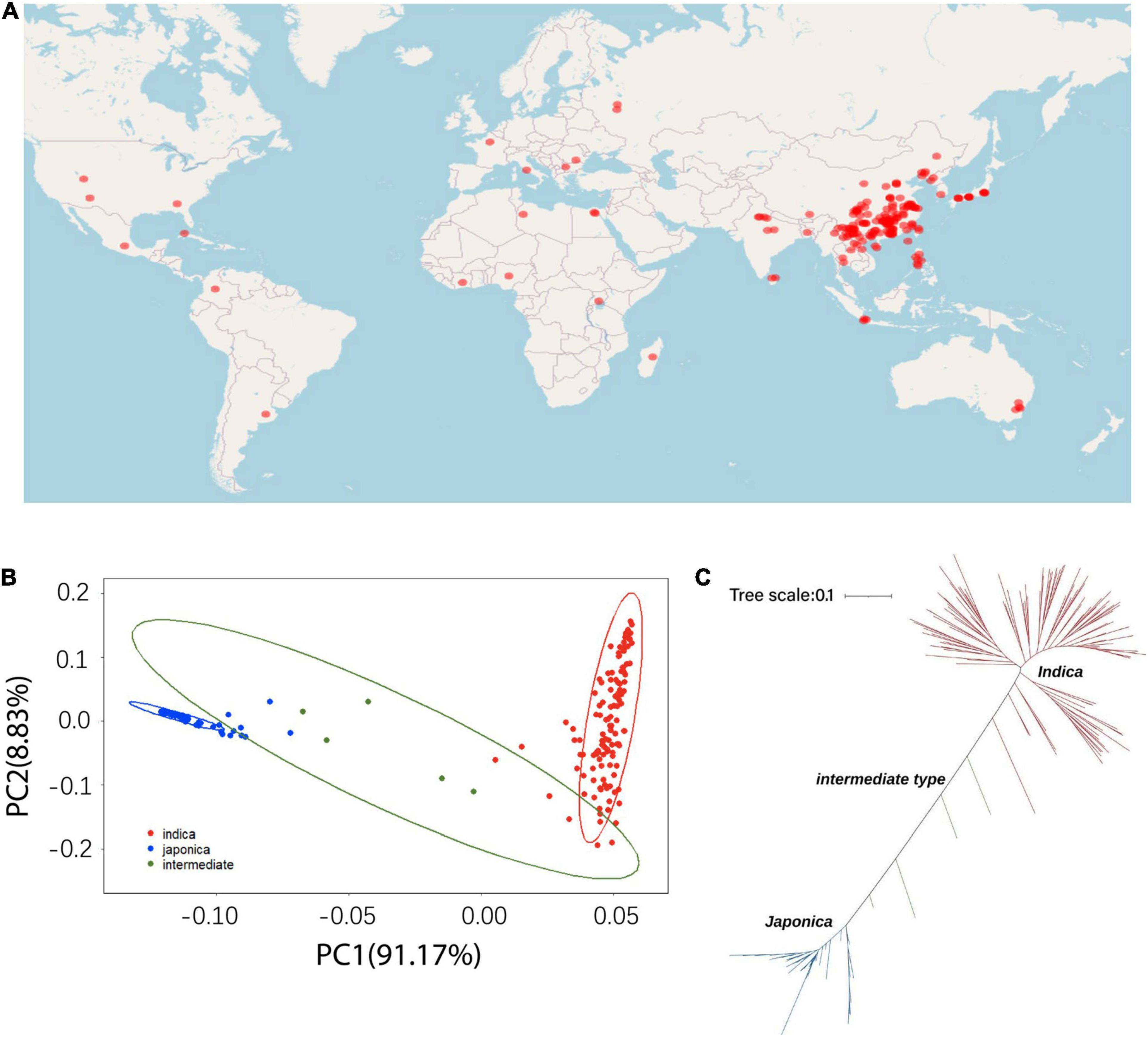
Figure 1. The geographic distribution and population genetic structure across the 191 rice cultivated accessions. (A) Geographic distribution. (B) Principal component analysis. (C) Neighbor-joining tree of the 191 cultivated rice accessions.
Evaluation of Salt Tolerance Among the Accessions
We examined the effects of salt stress in rice at the seedling stage and established different levels of NaCl concentrations for pre-experiments. A wide range of phenotypic values was observed when the accessions were treated with a 100 mM solution of NaCl to evaluate the salt traits. This resulted in the most diverse phenotypic distribution and facilitated the discrimination of accessions with different levels of salt tolerance. We treated 14-day-old seedlings from the 191 rice cultivated accessions with control (0 mM–1) and high NaCl/stress (100 mM–1) solutions. The plants displayed distinct salt tolerance variation across these populations that were analyzed. We then evaluated salt tolerance based on plant growth and development phenotypes. The evaluation of salt tolerance scores was divided into five grades (1, 3, 5, 7, and 9) using a modified standard evaluating score (Table 1) based on the visual symptoms of salt toxicity. This evaluation score distinguishes susceptible from tolerant and moderately tolerant phenotypes (Figure 2). We found no significant differences in plant growth and development between these accessions, and all the plants, such as Qiuqianbai (NS47) and Hongainuo (NS112), displayed a high SES under both normal and high salt stress conditions. We classified the accessions with a score of being highly tolerant to salt. Compared with normal conditions, the low SES accessions, particularly Heibiao (NS1) and Vietnam Zaodao (NS6), grew and developed poorly. In fact, almost all the plants died or were severely damaged under high salt stress conditions, and these accessions appear to be highly susceptible to salt stress. There was also a clear distinction between visual symptoms among the tolerant, moderate, and susceptible plants. The mean, standard deviation (SD), and coefficient of variation values for the SES are shown in Supplementary Table 2. This indicates the 191 cultivated rice accessions in the GWAS panel exhibited considerable natural variation in their degree of salt tolerance and had a very high level of genetic diversity.
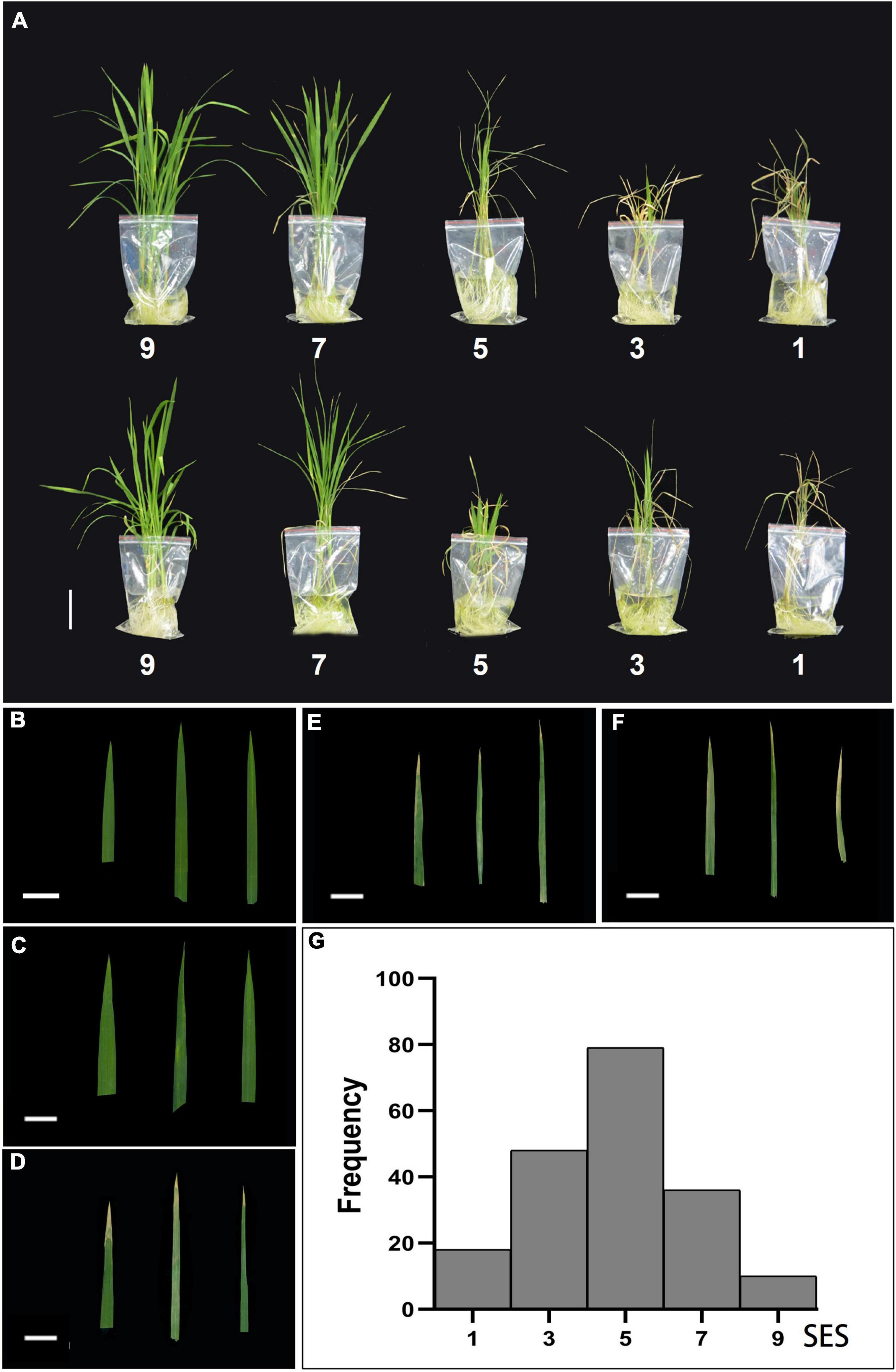
Figure 2. Classification and frequency histogram of the salt tolerance evolution score (SES) in the entire population. (A) Morphological responses of ten different rice varieties under 100 mM NaCl stress based on the salt tolerance evolution score (SES). Scale bar: 5 cm. Phenotypic characterization of five SES [9 (B), 7 (C), 5 (D), 3 (E), 1 (F)] of the visual symptoms in leaves. Scale bar: 2 cm. (G) Frequency histogram of the SES in the entire population.
Genome-Wide Association Study and Candidate Genes for Salt Tolerance
A GWAS was performed to identify candidate genes based on ∼3.82 million SNPs, with missing rates ≤30% and MAF >0.05. These polymorphisms covered the whole rice genome, and we used MLM to calculate associations. In particular, we established a threshold of −log P = 5 as a significant association standard. Overall, we identified 155 SNPs associated with salt tolerance, including 24 that were found across the entire panel, 37 in the indica panel and 94 in the japonica panel (Figure 3).
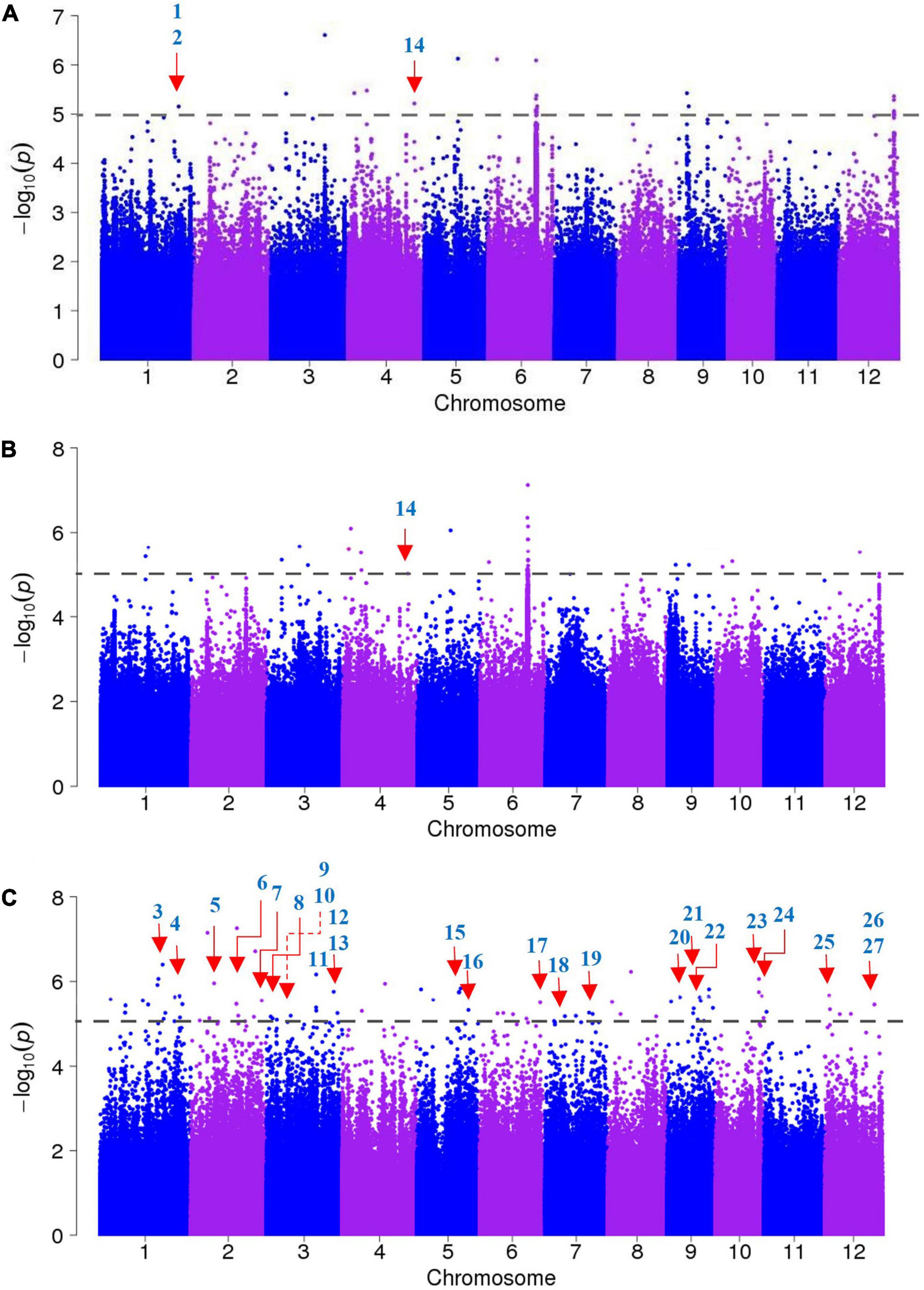
Figure 3. Genome-wide association studies of salt tolerance evolution scores (SES). (A) Manhattan plots of the entire accessions panel, (B) the indica accessions panel, (C) the japonica accessions panel. The known candidate genes responsible for salt sensitivity are indicated with red arrows, the numbers correspond to the genes presented in Table 2.
We searched for candidate genes within the genomic intervals of significant SNPs using the Rice Genome Annotation Project3 and published research on salt tolerance as references. We found 275 candidate genes in total (Supplementary Tables 3–5), including 27 genes that had been previously associated with salt sensitivity (Table 2). In the entire GWAS panel, we found 74 candidate genes in the intervals that corresponded to the 24 significant SNPs. We identified two candidate genes, ZFP179 and OsDSR2, in the SNP peak Chr1_36063662 that were associated with salt tolerance and located at 61.6 kb and 58.6 kb, respectively. Additionally, OsHKT1;1 (LOC_Os04g51820) was co-detected in the entire GWAS and indica panels. This gene is a member of the HKT family and plays a significant role in preventing sodium toxicity in leaf blades by reducing the accumulation of Na+ in shoots under conditions of high salinity. At the transcription level, OsHKT1;1 is activated by the OsMYBc transcription factor. We further studied the role of OsHKT1;1 by conducting haplotype analyses on the 191 rice varieties to identify elite haplotypes using all non-synonymous SNPs within their open reading frames (ORFs; Figure 4). We identified four distinct haplotypes based on four SNPs, which were responsible for the genetic differences observed between indica and japonica. Hap.1 was solely present in indica; Hap.2 was present in both indica and japonica, and Hap.3 and Hap.4 were predominantly present in japonica. Significant differences in salt tolerance indices were detected between Hap.2 and Hap.3. Moreover, the accessions that harbored the Hap.2 genotype displayed a higher SES than the other accessions, particularly Haobayong1 (NS153) and Menjiading (NS154). This indicates that Hap.2 confers salt tolerance to seedlings. These results further validate the role played by OsHKT1;1 in the regulation of variation in salt tolerance at the seedling stage.
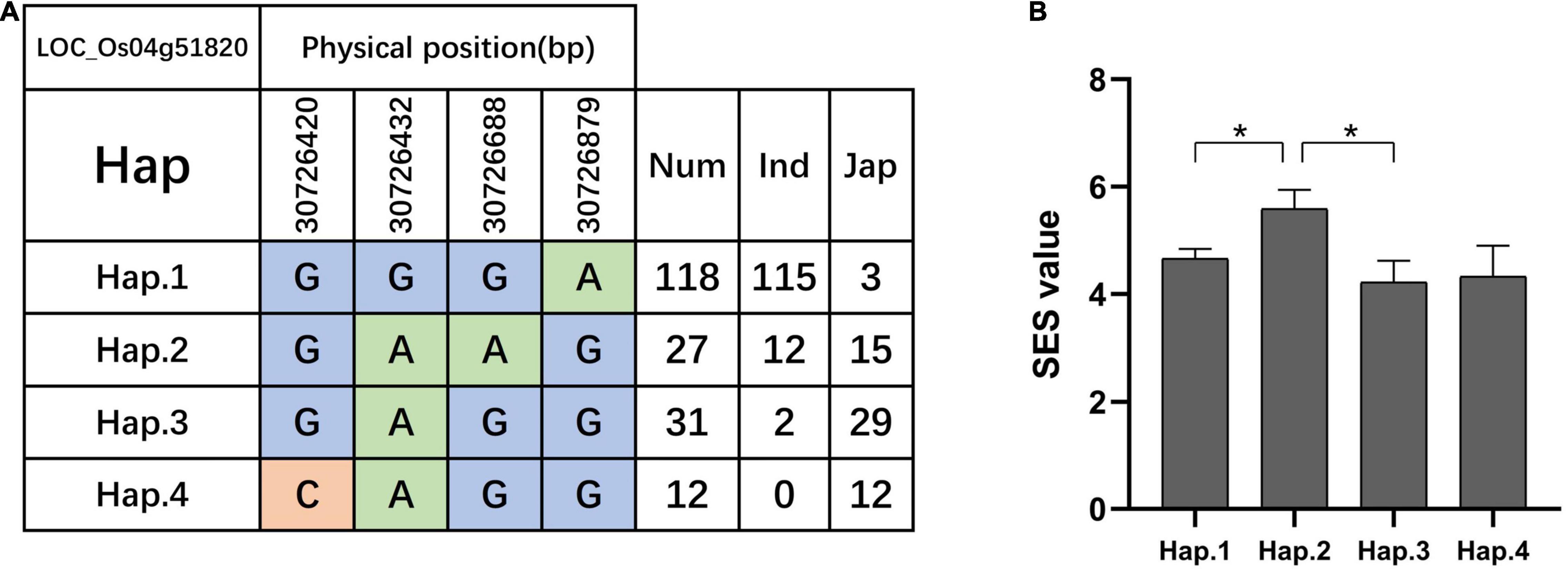
Figure 4. Haplotype analyses of OsHKT1;1 (LOC_Os04g51820). Gene structure (A) and SES (B) of different haplotypes of OsHKT1;1. T-test, *P < 0.05.
Among the remaining 24 candidate genes, OsPUP4, OsNBL3, MHZ6, OsIDS1, and OsJAZ8 were associated with salt tolerance. Additionally, 19 genes, including OsCLC1, OsCBL8, OsVPE3, OsDHODH1, OsLEA3-2, SLR1, ZFP182, OsAKT2, OsLEA5, OsSIDP366, OsSIK2, OsMsr9, OsDSG1, OsRPK1, OsMPK6, OsMSRA4.1, CYP94C2b, OsHSP23.7, and OsMYB91, were identified as salt sensitivity genes.
Genetic Differentiation of Candidate Genes Between Different Subpopulations
To examine the genetic basis behind the differences in salt tolerance observed among subpopulations at the seedling stage, we assessed 159 indica (ind), 146 temperate japonica and 41 tropical japonica (jap), as well as 53 wild O. rufipogon (Or), 163 O. glaberrima (Og) and 83 O. barthii (Ob) samples. We performed an FST analysis (Figure 5) and haplotype analysis (Supplementary Figure 1) separately, while the combined analysis was performed using the significant differentiated regions and the candidate genes as obtained by GWAS. We observed seven candidate genes in the domestication region of Asian and African rice, namely LOC_Os02g21810, LOC_Os05g35170 (IDEF2), LOC_Os10g41130, LOC_Os10g41200 (MYBS3), LOC_Os10g41260, LOC_Os10g41330, and LOC_Os12g41680 (OMTN3). These genes could have been selected simultaneously during the domestication of Asian and African rice varieties (Supplementary Table 6). Additionally, among the 27 genes previously associated with salt sensitivity, OsVPE3 (LOC_Os02g43010) was observed in the domestication region between the Ob and Og subpopulations; MHZ6 (LOC_Os03g20790) was found in the domestication region between the Or and indica subpopulations, and OsCBL8 (LOC_Os02g18930), OsDHODH1 (LOC_Os02g50350), SLR1 (LOC_Os03g49990), OsRPK1 (LOC_Os09g37949) were identified in the domestication region between the Or and japonica subpopulations. These results suggest that these candidate genes reside in selective sweep regions and could act as targets for the molecular improvement of rice salt tolerance.
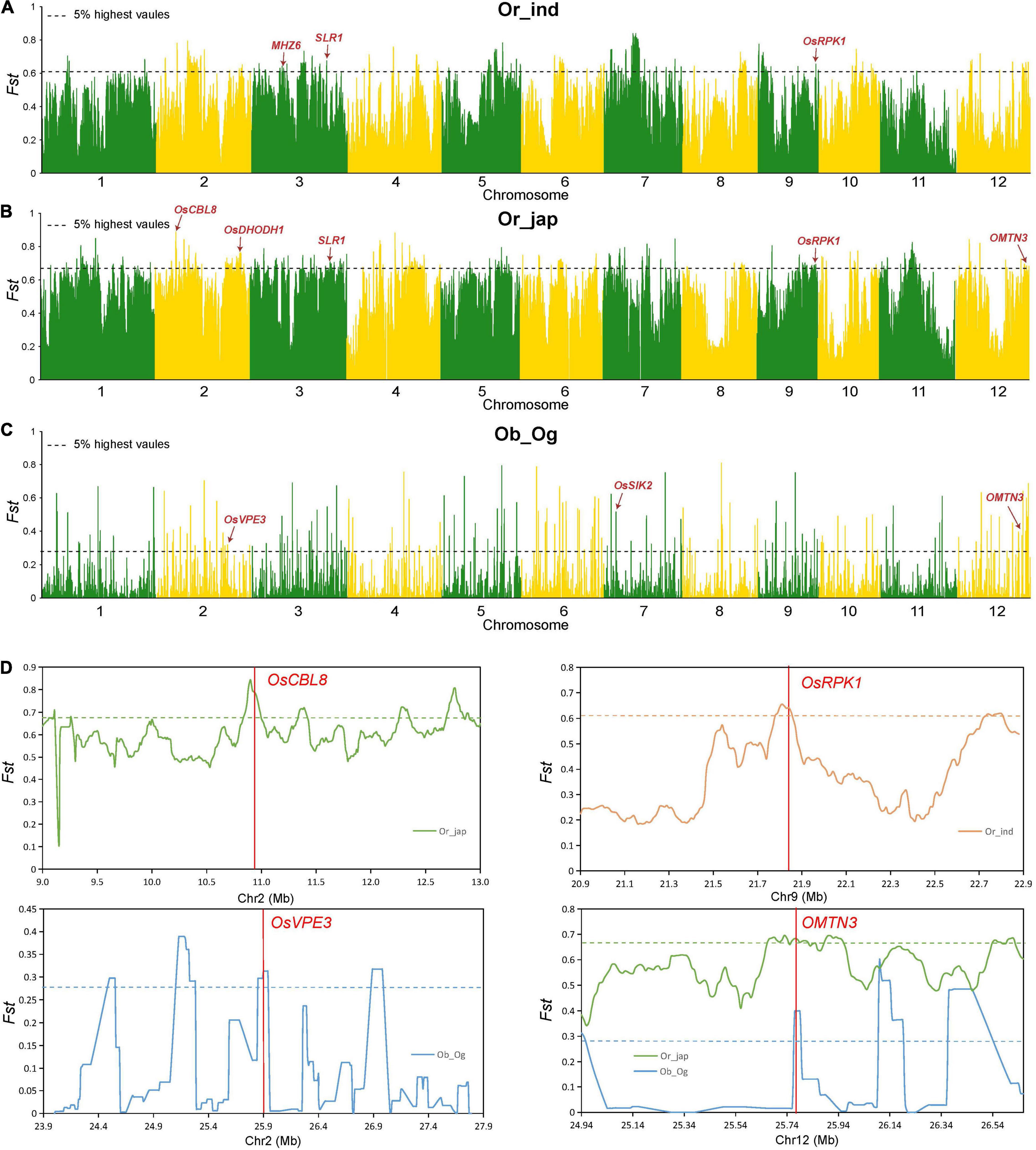
Figure 5. Genomic differentiation statistics. (A–C) Genomic differentiation between O. rufipogon and indica (ind) (A); O. rufipogon (Or) and japonica (jap) (B); and O. barthii (Ob) and O. glaberrima (Og) (C). Red arrows indicate the divergent regions overlapping with reported salt tolerance genes. (D) Genetic differentiation of several important genes. Green, orange, and blue lines represent the FST between O. rufipogon and indica, O. rufipogon and japonica, and O. barthii and O. glaberrima, respectively. Horizontal dashed lines correspond to the top 5% threshold. Red vertical lines represent the location of the reported genes.
Differentially Expressed Rice Genes From RNA-Seq Data in Response to Salt Stress
A transcriptome analysis is an effective strategy to identify the genes associated with a particular trait. To investigate transcriptional changes in rice under salinity stress, we selected two varieties of plants from the 191 accessions panel, specifically 93-11 and PA64s. These plants exhibited significant phenotypic differences under 0 mM (93-11ST0; PA64sST0) and 100 mM (93-11ST100; PA64sST100) NaCl conditions and were used for further RNA-Seq analysis. Clearly observable symptoms of salt injury were apparent in the 93-11ST100 seedlings after treatment with NaCl. The visual symptoms consisted of leaves that were mostly dried, the complete cessation of plant growth, and death of the plants. These effects were exacerbated by the amount of time under stress. We found similar but milder symptoms in PA64sST100.
To evaluate the potential molecular mechanisms that underlie salt tolerance, we combined the 93-11 and PA64s cultivars with their corresponding salt tolerant treatment plants and screened the DEGs of the two salt-tolerant control groups. A total of 2,821 and 1,249 DEGs that corresponded to two salt-stress pairwise comparisons were identified in ST1 (93-11ST0 vs. 93-11ST100) and ST2 (PA64sST0 vs. PA64sST100), respectively. We also noted that a higher number of DEGs were identified in ST1 (1,452 upregulated and 1,368 downregulated) than ST2 (843 upregulated and 406 downregulated).
The GO enrichment analysis indicated that the upregulated DEGs in ST1 and ST2 were highly enriched in the GO terms “response to chemical” and “stress.” The DEGs in ST1 that were enriched in the GO terms related to “membrane process” and “plasma membrane” were downregulated, while the genes enriched in “response to stress” and “membrane” were downregulated in ST2 (Supplementary Tables 7, 8). There was a total of 517 co-upregulated and 223 co-downregulated genes in ST1 and ST2 (Figure 6).
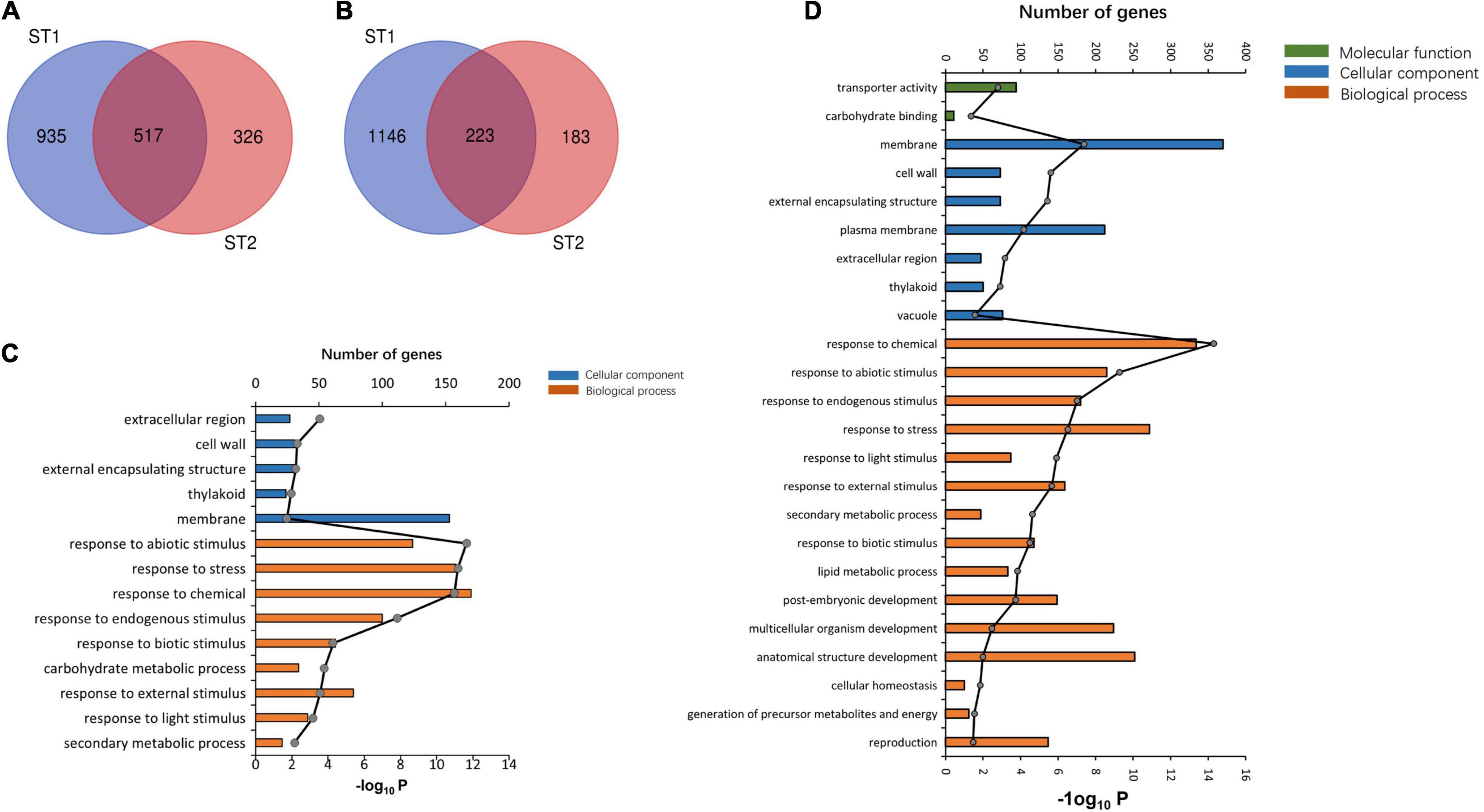
Figure 6. The transcriptome analysis of salt stress between 93 and 11 and PA64s. Venn diagrams showing the common (A) upregulated and (B) downregulated DEGs among ST1 (93-11ST0 vs. 93-11ST100) and ST2 (PA64sST0 vs. PA64sST100). GO classification at (C) ST1 and (D) ST2.
The DEGs investigated by RNA-Seq provide important clues to identify potential candidate genes responsible for rice growth and development under salt stress. A total of 30 candidate genes associated with salt tolerance were obtained by integrating GWAS and transcriptomic DEGs analyses. These cloned genes included 13 with no known association with salt tolerance and 17 candidate genes whose functions were unknown. Using the previously reported genes and published research on salt sensitivity as references, we found LOC_Os12g41680 (OMTN3) to be associated with the response to endogenous stimulus process on the candidate interval Chr12_25666325. This gene encodes the no apical meristem protein. Interestingly, OMTN3 was a DEG in ST2 and upregulated in PA64s under salt stress. Simultaneously, previous studies indicated that the OMTN genes were responsive to abiotic stresses, showed diverse spatiotemporal patterns of expression in rice and regulated numerous stress response genes; in particular, development and metabolism were altered in plants that overexpressed OMTN3. The candidate gene LOC_Os10g41260 encodes a MYB family transcription factor and was detected in the candidate interval Chr10_22142925. The gene was significantly enriched for GO biological processes related to chemical and endogenous stimulus response. In addition, LOC_Os12g41680 and LOC_Os10g41260 were selected during the diversification of the Or and japonica subspecies and the Ob and Og subspecies. We further performed haplotype analysis on the candidate gene LOC_Os12g41680 and LOC_Os10g41260 on 159 indica (ind), 146 temperate japonica (Tej), 41 tropical japonica (Trj), and 53 O. rufipogon (Or) to investigated different haplotypes under selection during domestication. LOC_Os10g41260 was classified into two haplotypes, which Hap.1 only distributed in cultivated rice (mainly in temperate japonica) and Hap.2 mainly distributed in indica varieties (Supplementary Figure 1A). For the candidate gene LOC_Os12g41680 haplotype analysis, we identified three types that Hap.1 mainly distributed in japonica subspecies, Hap.2 mainly distributed in indica and Hap.3 mainly distributed in wild rice (Or) (Supplementary Figure 1B). The haplotype analysis showed consistency with the Fst analysis, suggested that there are different haplotypes emerged during the domestication between the different subpopulations. Based on the evaluation scores for salt tolerance, GWAS, genetic differentiation and transcriptome analysis, LOC_Os12g41680 and LOC_Os10g41260 can be used as candidate genes for salt tolerance in rice.
Discussion
The growth and development of rice are inhibited under salt stress, and rice varieties were required to rapidly adapt to high salinity environments and grow well under salt stress over the course of evolution (Yamaguchi and Blumwald, 2005). To date, despite previous research that focused on the molecular mechanisms behind salt tolerance in rice, study of the adaptive mechanisms that integrate various pathways and molecular components remains challenging (Flowers and Flowers, 2005). Over the past few decades, several genes and QTLs associated with different traits for salt tolerance have been identified. However, only SalTol/SKC1 has been utilized in rice breeding (Thomson et al., 2010; Wang et al., 2015). There is a critical current challenge to enhance the tolerance to salt in rice to increase yields on salinized agricultural land (Ponce et al., 2021). Accordingly, the detection and mapping of genes and QTLs is crucial to identify new salt tolerance genes in the rice seedling stages.
Unraveling the genetic factors for salt tolerance in plants is a challenging endeavor. Association mapping emerged as a useful tool to identify alleles and QTLs associated with agronomically important traits (Thomson et al., 2010; Lv et al., 2021; Wei et al., 2021b). The collection of a wide range of germplasm resources with different genetic backgrounds is an essential step in association analysis. In this study, we collected a set of mini-core populations from different regions around the world, primarily from the 3,000 Rice Genomes Project (Fang et al., 2014). However, Wang et al. (2018) also provided seven accessions. Controlling for population structure has considerable implications for GWAS analysis, and population stratification can either introduce or remove spurious associations between genotypes and phenotypes (Stich et al., 2006). The population analyzed consisted of 191 rice genotypes that originated from 27 countries, corresponding to a sample with sufficient genetic variation for feasible association analysis to be conducted with the ultimate goal of discovering beneficial candidate genes that improve salt tolerance in rice. An ideal germplasm resource population should contain rich genotypic and phenotypic data (Pace et al., 2015; Valdisser et al., 2020). The data shown here consist of genotypic data for ∼3.82 million SNP markers from 191 germplasms, with the phenotypic variation used to conduct a GWAS analysis for salt tolerance. We considered the salt tolerance evaluation score to be a reliable measure to evaluate salt tolerance with phenotypic variation.
We identified 275 candidate genes within the candidate intervals. These genes were detected using the Rice Genome Annotation Project and published research on salt tolerance as references. Among these genes, there were 27 that had been previously identified as related to salt sensitivity, including five salt tolerant and 22 salt sensitivity genes. Surprisingly, OsHKT1;1 was identified as a candidate gene in our association analysis. This gene is located in the candidate interval chr4_30893016, and previous studies reported that this member of the HKT family plays an important role in reducing the accumulation of Na+ in shoots to circumvent salt stress (Platten et al., 2006). Sodium transporters mediate Na+-specific transport or Na+-K+ co-transport and are known to play key roles in plant tolerance to salt stress, particularly in HKT transporter-dependent fashion (Ren et al., 2005; Wang et al., 2012a). Association analysis enables the evaluation of a large number of alleles in different populations (Krill et al., 2010). To further explore allelic differences, we performed haplotype analysis on the candidate gene OsHKT1;1 and identified four distinct haplotypes based on four SNPs that differentiated the indica and japonica varieties. Accessions that harbored the Hap.2 genotype displayed a higher SES than those that harbored other haplotypes, particularly Haobayong1 and Menjiading. This indicates that salt tolerance is present in seedlings. Elite Hap.2 alleles of OsHKT1;1 was studied and will serve as potential candidates to genetically improve salt tolerant rice.
Salt tolerance diverged during the domestication of cultivated rice and enabled the plants to adapt to changing ecological habitats. To clarify the genetic basis of differences in salt tolerance among subgroups, we assessed African and Asian wild and cultivated rice by separately performing an FST analysis. The combined analysis was conducted using the significantly differentiated sites, and the candidate genes were obtained using a GWAS analysis. We found that seven genes were simultaneously selected during the domestication of Asian and African rice, namely OsVPE3 (LOC_Os02g43010), MHZ6 (LOC_Os03g20790), OsCBL8 (LOC_Os02g18930), OsDHODH1 (LOC_Os02g50350), OsSIK2 (LOC_Os07g08860), SLR1 (LOC_Os03g49990), and OsRPK1 (LOC_Os09g37949). The genes for salt tolerance that were identified can be differentiated and applied at the seedling stage to provide important information for the identification and pyramid breeding of salt tolerance genes in rice plants.
In addition to performing a GWAS analysis on salt tolerance across 191 rice cultivars, we combined RNA-Seq data of a susceptible indica cultivar (93-11) and a salt tolerant japonica cultivar (PA64s) to identify the genetic loci that confers salt resistance in rice. By integrating GWAS and transcriptomic analyses, 30 genes from both DEGs and GWAS candidate genes were identified. Among these genes, OMTN3 (LOC_Os12g41680) is a NAC transcription factor (Jeong et al., 2013), a family of genes that are widely distributed in plant species. For example, the OsNAC6 gene is one of the many NAC genes in rice that are associated with cold, salt, drought and abscisic acid (ABA) responses (Nakashima et al., 2007). In addition, the overexpression of another gene NAC transcription factor, SNAC1, significantly improves drought and salt tolerance in rice and regulates the expression of many stress-related genes (Redillas et al., 2012). OMTN3 is a DEG that was identified in the PA64s test group and is upregulated under salt stress in these plants. Moreover, it has been previously reported that OMTN3 negatively regulates drought tolerance in rice. Combining annotation and metabolic function information enabled the initial prediction that OMTN3 was a candidate gene associated with salt tolerance. Furthermore, we also focused on another candidate gene, LOC_Os10g41260, which encodes a MYB family transcription factor. LOC_Os10g41260 was significantly enriched for GO biological processes related to the chemical and endogenous stimulus responses. Interestingly, LOC_Os12g41680 and LOC_Os10g41260 were selected simultaneously during the domestication of Asian and African rice, that the results of haplotype analysis showed consistency with the Fst analysis. The salt tolerance evaluation scores, GWAS, genetic differentiation and transcriptome analysis led us to propose that the genes LOC_Os12g41680 and LOC_Os10g41260 can be used as candidate genes to affect the regulation of rice salt tolerance, even though further molecular functional verification needs to be conducted.
Overall, our study provides a theoretical basis to select and breed salt tolerant rice varieties. In particular, enhanced knowledge on the genetic information behind the complex mechanisms associated with this trait in rice will help to facilitate this endeavor.
Data Availability Statement
The datasets presented in this study can be found in online repositories. The names of the repository/repositories and accession number(s) can be found below: https://www.ncbi.nlm.nih.gov/, PRJNA831421.
Author Contributions
LG, LS, and QQ designed the experiment. JM, YW, and NJ performed all the phenotype evaluations. YL, FX, and MH performed analysis and interpretation of the data. YL and HW drafted the manuscript. All authors have read and agreed to the published version of the manuscript.
Funding
This work was supported by grants from the Shandong Agricultural Elite Variety Project (2019LZGC003), Agricultural Science and Technology Innovation Program, Shenzhen Science and Technology Program (Grant No. KQTD2016113010482651), Guangdong Basic and Applied Basic Research Foundation (Grant No. 2019A1515110557), and Hainan Yazhou Bay Seed Laboratory Project (B21HJ0223).
Conflict of Interest
The authors declare that the research was conducted in the absence of any commercial or financial relationships that could be construed as a potential conflict of interest.
Publisher’s Note
All claims expressed in this article are solely those of the authors and do not necessarily represent those of their affiliated organizations, or those of the publisher, the editors and the reviewers. Any product that may be evaluated in this article, or claim that may be made by its manufacturer, is not guaranteed or endorsed by the publisher.
Supplementary Material
The Supplementary Material for this article can be found online at: https://www.frontiersin.org/articles/10.3389/fpls.2022.912637/full#supplementary-material
Footnotes
References
Almeida, D. M., Oliveira, M. M., and Saibo, N. J. M. (2017). Regulation of Na+ and K+ homeostasis in plants: towards improved salt stress tolerance in crop plants. Genet Mol. Biol. 40, (Suppl. 1), 326–345. doi: 10.1590/1678-4685-gmb-2016-2106
Apse, M. P., Aharon, G. S., Snedden, W. A., and Blumwald, E. (1999). Salt tolerance conferred by overexpression of a vacuolar Na+/H+ antiport in Arabidopsis. Science 285, 1256–1258. doi: 10.1126/science.285.5431.1256
Bailey-Serres, J., Parker, J. E., Ainsworth, E. A., Oldroyd, G. E. D., and Schroeder, J. I. (2019). Genetic strategies for improving crop yields. Nature 575, 109–118. doi: 10.1038/s41586-019-1679-1670
Berthomieu, P., Conéjéro, G., Nublat, A., Brackenbury, W. J., Lambert, C., Savio, C., et al. (2003). Functional analysis of AtHKT1 in Arabidopsis shows that Na(+) recirculation by the phloem is crucial for salt tolerance. EMBO J. 22, 2004–2014. doi: 10.1093/emboj/cdg207
Bhatt, T., Sharma, A., Puri, S., and Minhas, A. P. (2020). Salt tolerance mechanisms and approaches: future scope of halotolerant genes and rice landraces. Rice Sci. 27, 368–383.
Blumwald, E., and Poole, R. J. (1985). Na+/H+ antiport in isolated tonoplast vesicles from storage tissue of beta vulgaris. Plant Physiol. 78, 163–167. doi: 10.1104/pp.78.1.163
Bolger, A. M., Lohse, M., and Usadel, B. (2014). Trimmomatic: a flexible trimmer for Illumina sequence data. Bioinformatics 30, 2114–2120. doi: 10.1093/bioinformatics/btu170
Chen, C., Chen, H., Zhang, Y., Thomas, H. R., Frank, M. H., He, Y., et al. (2020). TBtools: an integrative Toolkit developed for interactive analyses of big biological data. Mol. Plant 13, 1194–1202. doi: 10.1016/j.molp.2020.06.009
Chen, C., Rui, X., Hao, C., and He, Y. (2018). TBtools, a Toolkit for Biologists integrating various HTS-data handling tools with a user-friendly interface. bioRxiv [preprint] doi: 10.1101/289660
Chen, L. J., Wuriyanghan, H., Zhang, Y. Q., Duan, K. X., Chen, H. W., Li, Q. T., et al. (2013). An S-Domain receptor-like kinase, OsSIK2, confers abiotic stress tolerance and delays dark-induced leaf senescence in rice. Plant Physiol. 163, 1752–1765. doi: 10.1104/pp.113.224881
Chen, R., Deng, Y., Ding, Y., Guo, J., Qiu, J., Wang, B., et al. (2022). Rice functional genomics: decades’ efforts and roads ahead. Sci. China Life Sci. 65, 33–92. doi: 10.1007/s11427-021-2024-2020
Cheng, Y., Qi, Y., Zhu, Q., Chen, X., Wang, N., Zhao, X., et al. (2009). New changes in the plasma-membrane-associated proteome of rice roots under salt stress. Proteomics 9, 3100–3114. doi: 10.1002/pmic.200800340
Cubry, P., Tranchant-Dubreuil, C., Thuillet, A. C., Monat, C., Ndjiondjop, M. N., Labadie, K., et al. (2018). The rise and fall of african rice cultivation revealed by analysis of 246 new genomes. Curr. Biol. 28, 2274–2282.e6. doi: 10.1016/j.cub.2018.05.066.
Danecek, P., Auton, A., Abecasis, G., Albers, C. A., Banks, E., DePristo, M. A., et al. (2011). The variant call format and VCFtools. Bioinformatics 27, 2156–2158. doi: 10.1093/bioinformatics/btr330
Danecek, P., Bonfield, J. K., Liddle, J., Marshall, J., Ohan, V., Pollard, M. O., et al. (2021). Twelve years of SAMtools and BCFtools. Gigascience 10:giab008. doi: 10.1093/gigascience/giab008
Diedhiou, C., and Golldack, D. (2006). Salt-dependent regulation of chloride channel transcripts in rice. Plant Sci. 170, 793–800. doi: 10.1016/j.plantsci.2005.11.014
Duan, J., and Cai, W. (2012). OsLEA3-2, an abiotic stress induced gene of rice plays a key role in salt and drought tolerance. PLoS One 7:e45117. doi: 10.1371/journal.pone.0045117
El Mahi, H., Pérez-Hormaeche, J., De Luca, A., Villalta, I., Espartero, J., Gámez-Arjona, F., et al. (2019). A critical role of sodium flux via the plasma membrane Na+/H+ exchanger SOS1 in the salt tolerance of rice. Plant Physiol. 180, 1046–1065. doi: 10.1104/pp.19.00324
Fang, Y., Xie, K., and Xiong, L. (2014). Conserved miR164-targeted NAC genes negatively regulate drought resistance in rice. J. Exp. Bot. 65, 2119–2135. doi: 10.1093/jxb/eru072
Flowers, T. J., and Flowers, S. A. (2005). Why does salinity pose such a difficult problem for plant breeders? Agricultural Water Manag. 78, 15–24. doi: 10.1016/j.agwat.2005.04.015
Gao, H. S., Zhang, C., He, H. Y., Yu, C. Y., and Shang, L. G. (2020). Loci and alleles for submergence responses revealed by GWAS and transcriptional analysis in rice. Mol. Breeding 40, 1–16. doi: 10.1007/s11032-020-01160-6
Garciadeblás, B., Senn, M. E., Bañuelos, M. A., and Rodríguez-Navarro, A. (2003). Sodium transport and HKT transporters: the rice model. Plant J. 34, 788–801. doi: 10.1046/j.1365-313x.2003.01764.x
Gong, Z., Xiong, L., Shi, H., Yang, S., Herrera-Estrella, L. R., Xu, G., et al. (2020). Plant abiotic stress response and nutrient use efficiency. Sci. China Life Sci. 63, 635–674. doi: 10.1007/s11427-020-1683-x
Gregorio, G. B., Senadhira, D., and Mendoza, R. D. (1997). Screening Rice for Salinity Tolerance. Laguna: International Rice Research Institute. IRRI Discussion Papers.
Guo, C., Luo, C., Guo, L., Li, M., Guo, X., Zhang, Y., et al. (2016). OsSIDP366, a DUF1644 gene, positively regulates responses to drought and salt stresses in rice. J. Int. Plant Biol. 58, 492–502. doi: 10.1111/jipb.12376
Guo, X., Wu, Y., Wang, Y., Chen, Y., and Chu, C. (2009). OsMSRA4.1 and OsMSRB1.1, two rice plastidial methionine sulfoxide reductases, are involved in abiotic stress responses. Planta 230, 227–238. doi: 10.1007/s00425-009-0934-932
Hamamoto, S., Horie, T., Hauser, F., Deinlein, U., Schroeder, J. I., and Uozumi, N. (2015). HKT transporters mediate salt stress resistance in plants: from structure and function to the field. Curr. Opin. Biotechnol. 32, 113–120. doi: 10.1016/j.copbio.2014.11.025
Hassani, A., Azapagic, A., and Shokri, N. (2020). Predicting long-term dynamics of soil salinity and sodicity on a global scale. Proc. Natl. Acad. Sci. U S A. 117, 33017–33027. doi: 10.1073/pnas.2013771117
He, S., Tan, L., Hu, Z., Chen, G., Wang, G., and Hu, T. (2012). Molecular characterization and functional analysis by heterologous expression in E. coli under diverse abiotic stresses for OsLEA5, the atypical hydrophobic LEA protein from Oryza sativa L. Mol. Genet. Genom. 287, 39–54. doi: 10.1007/s00438-011-0660-x
Horie, T., Costa, A., Kim, T. H., Han, M. J., Horie, R., Leung, H. Y., et al. (2007). Rice OsHKT2;1 transporter mediates large Na+ influx component into K+-starved roots for growth. EMBO J. 26, 3003–3014. doi: 10.1038/sj.emboj.7601732
Huang, J., Sun, S., Xu, D., Lan, H., Sun, H., Wang, Z., et al. (2012a). A TFIIIA-type zinc finger protein confers multiple abiotic stress tolerances in transgenic rice (Oryza sativa L.). Plant Mol. Biol. 80, 337–350. doi: 10.1007/s11103-012-9955-9955
Huang, X., Kurata, N., Wei, X., Wang, Z. X., Wang, A., Zhao, Q., et al. (2012b). A map of rice genome variation reveals the origin of cultivated rice. Nature 490, 497–501. doi: 10.1038/nature11532
Jahan, N., Lv, Y., Song, M., Zhang, Y., and Guo, L. B. (2021). Transcriptomic analysis of short-term salt-stress response in mega hybrid rice seedlings. Agronomy 11:1328. doi: 10.3390/agronomy11071328
Jahan, N., Zhang, Y., Lv, Y., Song, M., and Guo, L. J. P. G. R. (2020). QTL analysis for rice salinity tolerance and fine mapping of a candidate locus qSL7 for shoot length under salt stress. Plant Growth Regulation 90, 307–319. doi: 10.1007/s10725-019-00566-3
Jeong, J. S., Kim, Y. S., Redillas, M. C., Jang, G., Jung, H., Bang, S. W., et al. (2013). OsNAC5 overexpression enlarges root diameter in rice plants leading to enhanced drought tolerance and increased grain yield in the field. Plant Biotechnol. J. 11, 101–114. doi: 10.1111/pbi.12011
Julkowska, M. M. (2018). Stress Management: OsIDS1 modulates histone deacetylation to repress salt tolerance genes. Plant Physiol. 178, 505–506. doi: 10.1104/pp.18.01029
Kang, H. M., Sul, J. H., Service, S. K., Zaitlen, N. A., Kong, S. Y., Freimer, N. B., et al. (2010). Variance component model to account for sample structure in genome-wide association studies. Nat. Genet. 42, 348–354. doi: 10.1038/ng.548
Kawahara, Y., de la Bastide, M., Hamilton, J. P., Kanamori, H., McCombie, W. R., Ouyang, S., et al. (2013). Improvement of the Oryza sativa Nipponbare reference genome using next generation sequence and optical map data. Rice 6:4. doi: 10.1186/1939-8433-6-4
Krill, A. M., Kirst, M., Kochian, L. V., Buckler, E. S., and Hoekenga, O. A. (2010). Association and linkage analysis of aluminum tolerance genes in Maize. PLoS One 5:e9958. doi: 10.1371/journal.pone.0009958
Kurotani, K.-I., Hayashi, K., Hatanaka, S., Toda, Y., Ogawa, D., Ichikawa, H., et al. (2015). Elevated levels of CYP94 family gene expression alleviate the jasmonate response and enhance salt tolerance in rice. Plant Cell Physiol. 56, 779–789. doi: 10.1093/pcp/pcv006
Langmead, B., and Salzberg, S. L. (2012). Fast gapped-read alignment with Bowtie 2. Nat. Methods 9, 357–359. doi: 10.1038/nmeth.1923
Liu, C., Chen, K., Zhao, X., Wang, X., Shen, C., Zhu, Y., et al. (2019). Identification of genes for salt tolerance and yield-related traits in rice plants grown hydroponically and under saline field conditions by genome-wide association study. Rice 12, 1–13. doi: 10.1186/s12284-019-0349-z
Liu, W. Y., Wang, M. M., Huang, J., Tang, H. J., Lan, H. X., and Zhang, H. S. (2009). The OsDHODH1 gene is involved in salt and drought tolerance in rice. J. Int. Plant Biol. 51, 825–833. doi: 10.1111/j.1744-7909.2009.00853.x
Lu, W., Deng, M., Guo, F., Wang, M., Zeng, Z., Han, N., et al. (2016). Suppression of OsVPE3 enhances salt tolerance by attenuating vacuole rupture during programmed cell death and affects stomata development in rice. Rice 9:65. doi: 10.1186/s12284-016-0138-x
Luo, C., Guo, C., Wang, W., Wang, L., and Chen, L. (2014). Overexpression of a new stress-repressive gene OsDSR2 encoding a protein with a DUF966 domain increases salt and simulated drought stress sensitivities and reduces ABA sensitivity in rice. Plant Cell Rep. 33, 323–336. doi: 10.1007/s00299-013-1532-1530
Lv, Y., Ma, J., Wang, Y. Y., Wang, Q., Lu, X. L., Hu, H. T., et al. (2021). Loci and natural alleles for low-Nitrogen-Induced growth response revealed by the genome-wide association study analysis in rice (Oryza sativa L.). Front. Plant Sci. 12:770736. doi: 10.3389/fpls.2021.770736
Ma, B. J., Gu, Z. M., Tang, H. J., Chen, X. F., Liu, F., and Zhang, H. S. (2010). Preliminary study on function of calcineurin B-like protein gene OsCBL8 in rice. Rice Sci. 17, 10–18. doi: 10.1016/s1672-6308(08)60099-60092
Martínez-Atienza, J., Jiang, X., Garciadeblas, B., Mendoza, I., Zhu, J. K., Pardo, J. M., et al. (2007). Conservation of the salt overly sensitive pathway in rice. Plant Physiol. 143, 1001–1012. doi: 10.1104/pp.106.092635
Meyer, R. S., Choi, J. Y., Sanches, M., Plessis, A., Flowers, J. M., Amas, J., et al. (2016). Domestication history and geographical adaptation inferred from a SNP map of African rice. Nat. Genet. 48:1083. doi: 10.1038/ng.3633
Min, M. H., Maung, T. Z., Cao, Y., Phitaktansakul, R., Lee, G. S., Chu, S. H., et al. (2021). Haplotype analysis of BADH1 by next-generation sequencing reveals association with salt tolerance in rice during domestication. Int. J. Mol. Sci. 22:7578. doi: 10.3390/ijms22147578
Mo, W., Tang, W., Du, Y., Jing, Y., Bu, Q., and Lin, R. (2020). PHYTOCHROME-INTERACTING FACTOR-LIKE14 and SLENDER RICE1 interaction controls seedling growth under salt stress. Plant Physiol. 184, 506–517. doi: 10.1104/pp.20.00024
Nakashima, K., Tran, L. S. P., Van Nguyen, D., Fujita, M., Maruyama, K., Todaka, D., et al. (2007). Functional analysis of a NAC-type transcription factor OsNAC6 involved in abiotic and biotic stress-responsive gene expression in rice. Plant J. 51, 617–630. doi: 10.1111/j.1365-313X.2007.03168.x
Pace, J., Gardner, C., Romay, C., Ganapathysubramanian, B., and Lubberstedt, T. (2015). Genome-wide association analysis of seedling root development in maize (Zea mays L.). BMC Genom. 16:47. doi: 10.1186/s12864-015-1226-1229
Park, G. G., Park, J. J., Yoon, J., Yu, S. N., and An, G. (2010). A RING finger E3 ligase gene, Oryza sativa delayed seed germination 1 (OsDSG1), controls seed germination and stress responses in rice. Plant Mol. Biol. 74, 467–478. doi: 10.1007/s11103-010-9687-9683
Park, Y. C., Lim, S. D., Moon, J. C., and Jang, C. S. (2019). A rice really interesting new gene H2-type E3 ligase, OsSIRH2-14, enhances salinity tolerance via ubiquitin/26S proteasome-mediated degradation of salt-related proteins. Plant Cell Environ. 42, 3061–3076. doi: 10.1111/pce.13619
Peethambaran, P. K., Glenz, R., Hoeninger, S., Islam, S. M. S., Hummel, S., Harter, K., et al. (2018). Salt-inducible expression of OsJAZ8 improves resilience against salt-stress. BMC Plant Biol. 18:331. doi: 10.1186/s12870-018-1521-1520
Platten, J. D., Cotsaftis, O., Berthomieu, P., Bohnert, H., Davenport, R. J., Fairbairn, D. J., et al. (2006). Nomenclature for HKT transporters, key determinants of plant salinity tolerance. Trends Plant Sci. 11, 372–374. doi: 10.1016/j.tplants.2006.06.001
Ponce, K. S., Guo, L. B., Leng, Y. J., Meng, L. J., and Ye, G. Y. (2021). Advances in sensing, response and regulation mechanism of salt tolerance in rice. Int. J. Mol. Sci. 22:2254. doi: 10.3390/ijms22052254
Qiu, T., Zhao, X., Feng, H., Qi, L., Yang, J., Peng, Y.-L., et al. (2021). OsNBL3, a mitochondrion-localized pentatricopeptide repeat protein, is involved in splicing nad5 intron 4 and its disruption causes lesion mimic phenotype with enhanced resistance to biotic and abiotic stresses. Plant Biotechnol. J. 19, 2277–2290. doi: 10.1111/pbi.13659
Quintero, F. J., Martinez-Atienza, J., Villalta, I., Jiang, X., Kim, W. Y., Ali, Z., et al. (2011). Activation of the plasma membrane Na/H antiporter Salt-Overly-Sensitive 1 (SOS1) by phosphorylation of an auto-inhibitory C-terminal domain. Proc. Natl. Acad. Sci. U S A. 108, 2611–2616. doi: 10.1073/pnas.1018921108
Quintero, F. J., Ohta, M., Shi, H., Zhu, J. K., and Pardo, J. M. (2002). Reconstitution in yeast of the Arabidopsis SOS signaling pathway for Na+ homeostasis. Proc. Natl. Acad. Sci. U S A. 99, 9061–9066. doi: 10.1073/pnas.132092099
Redillas, M., Jeong, J. S., Kim, Y. S., Jung, H., Bang, S. W., Choi, Y. D., et al. (2012). The overexpression of OsNAC9 alters the root architecture of rice plants enhancing drought resistance and grain yield under field conditions. Plant Biotechnol. J. 10, 792–805. doi: 10.1111/j.1467-7652.2012.00697.x
Ren, Z. H., Gao, J. P., Li, L. G., Cai, X. L., Huang, W., Chao, D. Y., et al. (2005). A rice quantitative trait locus for salt tolerance encodes a sodium transporter. Nat. Genet. 37, 1141–1146. doi: 10.1038/ng1643
Stich, B., Maurer, H. P., Melchinger, A. E., Frisch, M., Heckenberger, M., van der Voort, J. R., et al. (2006). Comparison of linkage disequilibrium in elite European maize inbred lines using AFLP and SSR markers. Mol. Breed. 17, 217–226. doi: 10.1007/s11032-005-5296-5292
Sun, S. J., Guo, S.-Q., Yang, X., Bao, Y.-M., Tang, H.-J., Sun, H., et al. (2010). Functional analysis of a novel Cys2/His2-type zinc finger protein involved in salt tolerance in rice. J. Exp. Bot. 61, 2807–2818. doi: 10.1093/jxb/erq120
Takagi, H., Tamiru, M., Abe, A., Yoshida, K., Uemura, A., Yaegashi, H., et al. (2015). MutMap accelerates breeding of a salt-tolerant rice cultivar. Nat. Biotechnol. 33, 445–449. doi: 10.1038/nbt.3188
Thomson, M. J., de Ocampo, M., Egdane, J., Rahman, M. A., Sajise, A. G., Adorada, D. L., et al. (2010). Characterizing the saltol quantitative trait locus for salinity tolerance in rice. Rice 3, 148–160. doi: 10.1007/s12284-010-9053-9058
Tian, Q., Shen, L., Luan, J., Zhou, Z., Guo, D., Shen, Y., et al. (2021). Rice shaker potassium channel OsAKT2 positively regulates salt tolerance and grain yield by mediating K+ redistribution. Plant Cell Environ. 44, 2951–2965. doi: 10.1111/pce.14101
Trapnell, C., Roberts, A., Goff, L., Pertea, G., Kim, D., Kelley, D. R., et al. (2012). Differential gene and transcript expression analysis of RNA-seq experiments with TopHat and Cufflinks. Nat. Protoc. 7, 562–578. doi: 10.1038/nprot.2012.016
Turner, S. D. J. B. (2014). qqman: an R package for visualizing GWAS results using Q-Q and manhattan plots. BioRxiv [preprint] doi: 10.1101/005165
Valdisser, P., Müller, B., Filho, J., Junior, O., and Vianello, R. P. (2020). Genome-wide association studies detect multiple QTLs for productivity in mesoamerican diversity panel of common bean under drought stress. Front. Plant Sci. 11:574674. doi: 10.3389/fpls.2020.574674
Van Zelm, E., Zhang, Y., and Testerink, C. (2020). Salt tolerance mechanisms of plants. Annu. Rev. Plant Biol. 71, 403–433. doi: 10.1146/annurev-arplant-050718-100005
Visscher, P. M., Brown, M. A., McCarthy, M. I., and Yang, J. (2012). Five years of GWAS discovery. Am. J. Hum. Genet. 90, 7–24. doi: 10.1016/j.ajhg.2011.11.029
Wang, F., Jing, W., and Zhang, W. (2014). The mitogen-activated protein kinase cascade MKK1-MPK4 mediates salt signaling in rice. Plant Sci. 227, 181–189. doi: 10.1016/j.plantsci.2014.08.007
Wang, H., Zhang, M., Guo, R., Shi, D., Liu, B., Lin, X., et al. (2012a). Effects of salt stress on ion balance and nitrogen metabolism of old and young leaves in rice (Oryza sativa L.). BMC Plant Biol. 12:194. doi: 10.1186/1471-2229-12-194
Wang, Z. F., Chen, Z. W., Cheng, J. P., Lai, Y. Y., Wang, J. F., Bao, Y. M., et al. (2012b). QTL Analysis of Na+ and K+ concentrations in roots and shoots under different levels of NaCl stress in rice (Oryza sativa L.). PLoS One 7:e51202. doi: 10.1371/journal.pone.0051202
Wang, R., Jing, W., Xiao, L., Jin, Y., Shen, L., and Zhang, W. (2015). The rice high-affinity potassium transporter1;1 is involved in salt tolerance and regulated by an MYB-Type transcription factor. Plant Physiol. 168, 1076–1090. doi: 10.1104/pp.15.00298
Wang, W. S., Mauleon, R., Hu, Z. Q., Chebotarov, D., Tai, S. S., Wu, Z. C., et al. (2018). Genomic variation in 3,010 diverse accessions of Asian cultivated rice. Nature 557, 43–49. doi: 10.1038/s41586-018-0063-69
Wang, Y. X., Shang, L. G., Yu, H., Zeng, L. J., Hu, J., Ni, S., et al. (2020). A strigolactone biosynthesis gene contributed to the green revolution in rice. Mol. Plant 13, 923–932. doi: 10.1016/j.molp.2020.03.009
Wei, H., Wang, X., He, Y., Xu, H., and Wang, L. (2021a). Clock component OsPRR73 positively regulates rice salt tolerance by modulating OsHKT2;1-mediated sodium homeostasis. EMBO J. 40:e105086. doi: 10.15252/embj.2020105086
Wei, Z. R., Yuan, Q. L., Lin, H., Li, X. X., Zhang, C., Gao, H. S., et al. (2021b). Linkage analysis, GWAS, transcriptome analysis to identify candidate genes for rice seedlings in response to high temperature stress. BMC Plant Biol. 21:85. doi: 10.1186/s12870-021-02857-2852
Xu, G., Cui, Y., Wang, M., Li, M., Yin, X., and Xia, X. (2014). OsMsr9, a novel putative rice F-box containing protein, confers enhanced salt tolerance in transgenic rice and Arabidopsis. Mol. Breed. 34, 1055–1064. doi: 10.1007/s11032-014-0096-91
Yamaguchi, T., and Blumwald, E. (2005). Developing salt-tolerant crop plants: challenges and opportunities. Trends Plant Sci. 10, 615–620. doi: 10.1016/j.tplants.2005.10.002
Yang, C., Ma, B., He, S. J., Xiong, Q., Duan, K. X., Yin, C. C., et al. (2015). MAOHUZI6/ETHYLENE INSENSITIVE3-LIKE1 and ETHYLENE INSENSITIVE3-LIKE2 regulate ethylene response of roots and coleoptiles and negatively affect salt tolerance in rice. Plant Physiol. 169, 148–165. doi: 10.1104/pp.15.00353
Yeo, A. R., and Flowers, T. J. (1986). Salinity resistance in rice (Oryza sativa L.) and a pyramiding approach to breeding varieties for saline soils. Aust. J. Plant Physiol. 13, 161–173.
Yin, W., Xiao, Y., Niu, M., Meng, W., Li, L., Zhang, X., et al. (2020). ARGONAUTE2 enhances grain length and salt tolerance by activating BIG GRAIN3 to modulate cytokinin distribution in rice. Plant Cell 32, 2292–2306. doi: 10.1105/tpc.19.00542
Yu, J., Liu, C., Lin, H., Zhang, B., Li, X., Yuan, Q., et al. (2021). Loci and natural alleles for cadmium-mediated growth responses revealed by a genome wide association study and transcriptome analysis in rice. BMC Plant Biol. 21:374. doi: 10.1186/s12870-021-03145-3149
Yu, J., Zao, W. G., He, Q., Kim, T. S., and Park, Y. J. (2017). Genome-wide association study and gene set analysis for understanding candidate genes involved in salt tolerance at the rice seedling stage. Mol. Genet. Genomics 292, 1391–1403. doi: 10.1007/s00438-017-1354-1359
Yu, J., Zhao, W., Tong, W., He, Q., Yoon, M. Y., Li, F. P., et al. (2018). A genome-wide association study reveals candidate genes related to salt tolerance in rice (Oryza sativa) at the germination stage. Int. J. Mol. Sci. 19: 3145.
Yuan, J., Wang, X. Q., Zhao, Y., Khan, N. U., Zhao, Z. Q., Zhang, Y. H., et al. (2020). Genetic basis and identification of candidate genes for salt tolerance in rice by GWAS. Sci. Rep. 10:9958. doi: 10.1038/s41598-020-66604-66607
Zhu, J. K. (2001). Cell signaling under salt, water and cold stresses. Curr. Opin. Plant Biol. 4, 401–406. doi: 10.1016/s1369-5266(00)00192-198
Zhu, N., Cheng, S., Liu, X., Du, H., Dai, M., Zhou, D.-X., et al. (2015). The R2R3-type MYB gene OsMYB91 has a function in coordinating plant growth and salt stress tolerance in rice. Plant Sci. 236, 146–156. doi: 10.1016/j.plantsci.2015.03.023
Keywords: rice, genome-wide association study (GWAS), salt tolerance, transcriptome analysis, domestication detection
Citation: Lv Y, Ma J, Wei H, Xiao F, Wang Y, Jahan N, Hazman M, Qian Q, Shang L and Guo L (2022) Combining GWAS, Genome-Wide Domestication and a Transcriptomic Analysis Reveals the Loci and Natural Alleles of Salt Tolerance in Rice (Oryza sativa L.). Front. Plant Sci. 13:912637. doi: 10.3389/fpls.2022.912637
Received: 04 April 2022; Accepted: 23 May 2022;
Published: 16 June 2022.
Edited by:
Loredana F. Ciarmiello, University of Campania Luigi Vanvitelli, ItalyReviewed by:
Mueen Alam Khan, Islamia University of Bahawalpur, PakistanJindong Liu, Institute of Crop Sciences (CAAS), China
Copyright © 2022 Lv, Ma, Wei, Xiao, Wang, Jahan, Hazman, Qian, Shang and Guo. This is an open-access article distributed under the terms of the Creative Commons Attribution License (CC BY). The use, distribution or reproduction in other forums is permitted, provided the original author(s) and the copyright owner(s) are credited and that the original publication in this journal is cited, in accordance with accepted academic practice. No use, distribution or reproduction is permitted which does not comply with these terms.
*Correspondence: Longbiao Guo, guolongbiao@caas.cn; Lianguang Shang, shanglianguang@163.com
†These authors have contributed equally to this work