- 1Institute of Cash Crops, Hebei Academy of Agriculture and Forestry Sciences, Shijiazhuang, China
- 2Ministry of Education Key Laboratory of Molecular and Cellular Biology, Hebei Collaboration Innovation Center for Cell Signaling and Environmental Adaptation, Hebei Key Laboratory of Molecular and Cellular Biology, College of Life Sciences, Hebei Normal University, Shijiazhuang, China
The disease Fusarium crown and root rot (FCRR), caused mainly by Fusarium oxysporum f. sp. radicis-lycopersici (FORL), seriously affects commercial tomato [Solanum lycopersicum (Sl)] yields. However, the genes that offer resistance to FORL are limited and the mechanism of resistance to FCRR is poorly understood. Lectin receptor-like kinases (LecRKs) play critical roles in defensive responses and immunity in many plant species; however, whether specific LecRKs are involved in the response of tomato plants to FORL is unclear. Here, we report that the expression of SlLecRK1/Solyc09g011070.1 was obviously induced by the infection of FORL. Biochemical and cell biological data revealed that SlLecRK1 is an active kinase that is located at the cell membrane, while real-time quantitative PCR data suggested that SlLecRK1 is mainly expressed in stems and roots. Genetic studies showed that overexpression of SlLecRK1 significantly improved the resistance of tomato plants to FORL but did not cause visible changes in plant growth and development compared with wild-type control plants. RNA-Seq data suggested that the positive effects of SlLecRK1 on the resistance of tomato plants to FORL occur mainly by triggering the expression of ethylene-responsive transcription factor (ERF) genes. Together, our findings not only identify a new target for the development of FCRR-resistant tomato varieties, they also demonstrate a molecular mechanism linking SlLecRK1 and ERFs in regulating the immune responses of tomato plants to FORL.
Introduction
Decades of research have revealed that crop succession can lead to soil-borne diseases such as Fusarium crown and root rot (FCRR), an emerging disease that seriously threatens tomato (Solanum lycopersicum L.) production in most countries (Polizzi et al., 2011; Cordero-Ramirez et al., 2013; Sepulveda-Chavera et al., 2014). FCRR can occur from the seedling stage to the fruiting stage in tomato plants. At the start of the disease, no phenotypic change is visible in the above-ground parts of the plant; however, as the disease progresses wilting and browning are observed leading to plant death. Thus, FCRR causes reduced numbers or a lack of tomatoes. FCRR is mainly caused by Fusarium oxysporum f. sp. radicis-lycopersici (FORL), a fungus that can survive in soil as spores for up to 6 years (Rekah et al., 2000; Gordon, 2017; Srinivas et al., 2019). The application of disinfectants and fungicides is a major approach to reduce FCRR; such chemical control is extensively used in the commercial production of tomatoes (Benhamou and Belanger, 1998; Myresiotis et al., 2012). Additionally, biological resources, including bacteria and fungi, have been used to control FCRR in tomato (Chin et al., 2000; Grauwet et al., 2005; Kavroulakis et al., 2007; Nefzi et al., 2019). However, these methods are of limited usefulness during a disease outbreak. Though the development of resistant tomato varieties would be an efficient and economical approach to the control of FCRR in tomato, our understanding of the genes conferring resistance to FORL is limited and the mechanisms of resistance are poorly understood (Mazzeo et al., 2014; Manzo et al., 2016).
Several studies have demonstrated that plants recognize pathogenic microorganisms via cell membrane proteins, termed pattern recognition receptors (PRRs; Nurnberger and Kemmerling, 2006; Monaghan and Zipfel, 2012; Park et al., 2012; Tang et al., 2017; Kong et al., 2021). Most PRRs are receptor-like kinases (RLKs) or receptor-like proteins (RLPs). Both RLKs and RLPs contain an extracellular domain that perceives extracellular signals; RLKs transduce these signals via their intracellular kinase domain to trigger defense-related gene expression and the synthesis of defensive chemicals, including plant hormones, reactive oxygen species, and callose to enhance plant disease resistance (Park et al., 2012; Tang et al., 2017). The roles of RLKs in response to pathogenic bacteria have been extensively studied in many plant species, and some RLKs are considered ideal candidates for molecular breeding programs (Robatzek et al., 2006; Schwessinger et al., 2015; Pizarro et al., 2018; Piazza et al., 2021). However, little is known about whether and which RLKs are involved in tomato resistance to FORL.
RLKs comprise a vast protein family encoded by more than 600 genes in Arabidopsis and 1,100 genes in rice. Based on their extracellular domains, RLKs are classified into 15 subfamilies (Vaid et al., 2013). Among these subfamilies, lectin RLKs (LecRKs) are different from RLKs in that their extracellular domain resembles that of carbohydrate-binding lectin proteins (Bouwmeester and Govers, 2009). LecRKs are thought to play vital roles in the defense of plants against various pathogens and pests (Singh and Zimmerli, 2013; Bellande et al., 2017; Wang and Bouwmeester, 2017; Sun et al., 2020), and accumulating evidence indicates that some LecRLKs act as PRRs or important regulators of plant immune responses. For instance, in Arabidopsis, LecRK-V.5, LecRK-IX.1, and LecRK-IX.2 contribute to resistance against Phytophthora spp. LecRK-VI.2 is also a positive regulator of stomatal innate immunity (Singh et al., 2012). Overexpression of LecRK-IX.2 or LecRK-IV.3 also results in enhanced resistance to Pseudomonas syringae pv. tomato DC3000 and Botrytis cinerea, respectively (Wang et al., 2015). LecRK-I.8 is required for the perception of insect egg-derived elicitors (Gouhier-Darimont et al., 2019). More importantly, recent studies have shown that LecRK-I.9/DORN1 is involved in plant immune responses by acting as a PRR for the damage-associated molecular pattern eATP; further, the extracellular domain of LecRK-I.9 binds ATP with high affinity. This binding is required for ATP-induced calcium responses, MAPK activation, and gene expression. Therefore, LecRK-I.9 probably plays a variety of roles in plant stress resistance (Choi et al., 2014; Chen et al., 2017; Wang et al., 2018).
Since LecRKs are involved in the response of plants to various pathogens, as well as in biological recognition processes involving cells and proteins (Vaid et al., 2013), the members of this subfamily also likely participate in the responses of tomato plants to FORL. In this study, we found that SlLecRK1, the homolog of LecRK-I.9/DORN1 (Choi et al., 2014; Chen et al., 2017; Wang et al., 2018) in tomato, was induced at the mRNA and protein levels by inoculation with FORL. Using genetic, molecular, and physiological strategies, we found that the overexpression of SlLecRK1 improved tomato resistance to FORL by triggering the expression of ERFs. Notably, SlLecRK1-overexpressing (OE) plants displayed no visible phenotypic change compared with wild-type (WT) control plants, demonstrating the potential of the gene in the development of FCRR-resistant tomato varieties. Thus, we conclude that SlLecRK1 may be used in molecular breeding programs to enhance the resistance of tomato plants to FORL.
Materials and Methods
Plant Materials and Growth Conditions
The FORL-susceptible tomato (S. lycopersicum) variety Moneymaker was used in this study. Tomato plants were grown in a greenhouse under a 16-h light/8-h dark photoperiod at 25°C/22°C, or in a greenhouse under natural conditions (Mar-Jul in Hebei, China) for seed production. Seeds were sown on half-strength Murashige and Skoog medium containing 1.5% (w/v) sucrose and grown in a light chamber under a 16-h light/8-h dark photoperiod at 25°C/22°C.
Fungal Isolation and Infection Assay
FORL was isolated from field grown, infected tomato plants, and the isolate was identified by PCR using specific primers as described previously (Validov et al., 2011). The isolate was kept in 50% glycerol at −80°C for use in our experiments. The isolate was grown in potato dextrose broth on a rotary shaker for 5 days at 23°C. The culture was then filtered through two layers of antiseptic gauze. The suspension was centrifuged at 3000 rpm for 10 min and conidia were resuspended in ddH2O at a spore concentration of 107 CFU/ml. Roots of 10-day-old seedlings were dipped in the suspension and then transplanted at normal photoperiods for 1–3 days. For 30-day-old tomatoes, the stem bases were punctured and the suspension was poured into the soil, after which the tomatoes were allowed to keep growing.
Plasmid Construction and the Generation of Transgenic Plants
To produce the expression constructs SlLecRK1Pro:SlLecRK1-GFP and SlLecRK1Pro:SlLecRK1-Myc, a 4,353-bp fragment of the SlLecRK1 promoter and coding sequence was amplified by PCR (FastPfu Fly, Cat. #AP231; TransGen Biotech) using primers flanked by a HindIII site (SlLecRK1Pro F) and BamHI site (cSlLecRK1 R); the primers are listed in Supplementary Table S1. The fragment was cloned into pCAMBIA1300-MH between the HindIII and BamHI sites by ligation (Cat. #M0202S; New England Biolabs) to replace the existing 35S promoter. The SlLecRK1Pro:SlLecRK1-Myc construct was sequenced before proceeding to tomato transformation with Agrobacterium tumefaciens strain EHA105. The SlLecRK1Pro:SlLecRK1-GFP construct was used for transient expression in N. benthamiana leaves.
Fungal DNA Concentration Analysis
Plants inoculated with FORL were pulverized in liquid nitrogen and then mixed with 1 ml of cetyl-trimethyl-ammonium bromide. Each mixture was incubated at 95°C for 1 h and then centrifuged at 12000 × g. The supernatant was extracted with one volume of chloroform. The upper phase was transferred to a new Eppendorf tube and the DNA was precipitated by adding one volume of isopropanol followed by centrifugation at 12000 × g. The DNA pellets were washed with 70% ethanol and dried. Total DNA was dissolved in ddH2O and then quantified using a NanoDrop 1,000 Spectrophotometer (Thermo Fisher). To determine the fungal DNA concentration, qPCR was performed as described previously (Validov et al., 2011), using the primers OMP1049 and OMP1050, by SYBR Premix Ex Taq (Cat. #RR420A; Takara Bio Inc.) and a C1000 Touch Thermal Cycler CFX96 (Bio-Rad). A standard curve for quantification was generated by plotting the log of the concentrations (from 50 fg to 2 ng) of total DNA isolated from FORL; the primers used are listed in Supplementary Table S1.
Real-Time Quantitative PCR
Total RNA was extracted from specific tissues treated (or not), as indicated, with TRIzol reagent (Cat. #15596024; Invitrogen) according to the manufacturer’s instructions. First-strand cDNA was synthesized using a PrimeScript RT Reagent Kit with Genomic DNA Eraser (Cat. #RR047A; Takara Bio Inc.) Gene expression was analyzed by qRT-PCR using SYBR Premix Ex Taq (Cat. #RR420A; Takara Bio Inc.) and a C1000 Touch Thermal Cycler CFX96 (Bio-Rad). All primers are listed in Supplementary Table S1. The relative abundance of the target transcripts was determined by the comparative threshold cycle method (Schmittgen and Livak, 2008) using Elongation Factor 1 alpha (eEF1α,Solyc06g069020) as an internal reference (Campos et al., 2019).
Immunoblotting
Tomato seedlings were ground in liquid nitrogen as indicated and then boiled in SDS sample buffer [50 mm Tris, pH 6.8, 2 mm EDTA, 10% (w/v) glycerol, 2% SDS, and 6% 2-mercaptoethanol; 2 μg/μl of tissue]. A 10 μl sample from each supernatant after centrifugation at 10000 × g for 10 min was subjected to 10% SDS-PAGE. Separated proteins were transferred to a nitrocellulose membrane and blocked with 5% skimmed milk in phosphate-buffered saline (PBS) for 1 h. Then, the membrane was probed with the indicated primary antibodies (1:3000 in 5% skimmed milk in PBS; Mouse monoclonal anti-Myc, Cat. #M4439, Sigma-Aldrich; Mouse monoclonal anti-GFP, Cat. #G1544, Sigma-Aldrich) for at least 3 h. After three washes with wash buffer (PBS, supplemented with 0.001% Tween) for 10 min each, the membrane was probed with horseradish peroxidase (HRP)-conjugated antibodies against mouse-IgG for 1 h (Cat. #BA1051; Boster). After three washes with wash buffer (PBS, supplemented with 0.001% Tween) for 10 min each, the membrane was incubated with SuperSignal West Femto Maximum Sensitivity Substrate (Cat. #34095; Thermo Scientific) and signals were detected by CCD imaging (Zhao et al., 2019).
Subcellular Localization
The constructs SlLecRK1Pro:SlLecRK1-GFP and 35SPro:GFP were transiently expressed in N. benthamiana leaves, incubated with FM4-64 (Cat. #T13320; Invitrogen) for 5 min for plasma membrane staining and then visualized under laser scanning confocal microscopy (FLUOVIEW FV3000, Olympus; GFP: excitation at 488 nm and emission at 510 nm; FM4-64: excitation at 587 and emission at 610 nm).
Preparation of Recombinant Proteins and Kinase Assay
To prepare GST-ICD and GST-mICD, the coding sequence of SlLecRK1-ICD (amino acids 310–681) was recombined into vector pGEX-6p-1. The resulting constructs, pGEX-6p-1-SlLecRK1 ICD and pGEX-6p-1-mSlLecRK1 ICD, were transformed into E. coli strain Rosetta (DE3) and the recombinant proteins were produced and purified using Glutathione Sepharose 4 Fast Flow (Cat. #17513202; Cytiva) following the manufacturer’s instructions. For GST-ICD and GST-mICD phosphorylation, 3 μg of recombinant purified proteins were incubated in kinase buffer (50 mm HEPES, pH 7.5, 10 mm MgCl2, 5 mm MnCl2, and 1 mm DTT) containing 0.2 mm ATP at 30°C for 3 h (Oh et al., 2012; Zhao et al., 2019) prior to SDS-PAGE followed by immunoblotting. Anti-phosphoserine/threonine (Cat. #PP2551; ECM) was used to detect kinase phosphorylation levels, and Anti-GST HRP (Cat. #1006–3; HuaAn Biotechnology) was used to detect GST-SlLecRK1 protein loading.
RNA Sequencing
Total RNA was isolated from tomato seedlings using a TRIzol Kit (Invitrogen). Paired-end sequencing libraries were prepared using a TruSeq RNA Sample Preparation Kit, version 2 (Illumina) and sequenced on a HiSeq Xten machine according to the manufacturer’s protocols. FastQC1 was initially run to assess the overall quality of the sequenced reads. Poor-quality reads were filtered out using Sickle with the parameters pe mode; −t Sanger -q 20 -l 50.2 The remaining high-quality reads were mapped to the S. lycopersicum reference genome (ITAG4.0) using TopHat2, version 2.09 (Kim et al., 2013), with the parameters “-N 3-read- edit-dist 3-segment-mismatches 1 -p 20 -r 0 -g 20—microexon-search -b2 -D 20 -b2-R3-no-coverage-search.” Only reads showing unique alignments were retained for the following analysis. HTseq software3 was used to determine the read counts mapped to each of the genes. Next, genes with more than 10 reads in at least one sample were kept for differential expression analysis. Batch effects were removed from the filtered read count table using RUVseq (normalization of RNA-Seq data using a factor analysis of control genes or samples) with the residual RUVr approach. The Bioconductor package “edgeR” (Robinson et al., 2010) was used for PCA and differential expression analysis. Only genes with an FDR < 0.05 and absolute value of log2 (fold change) ≥ 0.58 (OE-1 vs. wild type) or 1 (1 vs. 0 dpi) were considered DEGs. GO term enrichment was analyzed by singular enrichment analysis with AgriGO (Tian et al., 2017) using a hypergeometric test, with a FDR < 0.05 as the cutoff. Venn diagrams were drawn using Vennerable R.4
Quantification of Protein Abundance
Protein abundance in the immunoblots was quantified by measuring the band intensity with ImageJ 1.47v.5 The relative protein level was obtained by normalization to the loading control.
Results
Solyc09g011070.1 Transcript and Protein Levels Were Increased by FORL
In the tomato genome, there are more than 100 genes encoding LecRKs,6 and 10 of them show relatively higher identity (42–47%) to LecRK-I.9/DORN1. Phylogenetic analysis also showed that the most similar protein forms a separate cluster from Arabidopsis LecRK-I.9/DORN1, and Solyc09g011060.2 is the most closely related gene to LecRK-I.9/DORN1 in tomato (Figure 1A). To reveal their potential roles in the FORL defense response in tomato, we performed qRT-PCR to assess whether the transcript levels of these LecRKs were affected by FORL. Total RNA was extracted from 10-day-old WT tomato seedlings that had been treated with FORL 1 day earlier. The expression of Solyc05g053010.1 and Solyc09g011990.1 was unchanged, while six genes were downregulated, following FORL treatment compared with untreated control plants. However, the transcript levels of Solyc05g053010.1 and Solyc09g011070.1 were obviously upregulated, and the expression level of Solyc09g011070.1 was more than fivefold that in the control plants (Figure 1B).
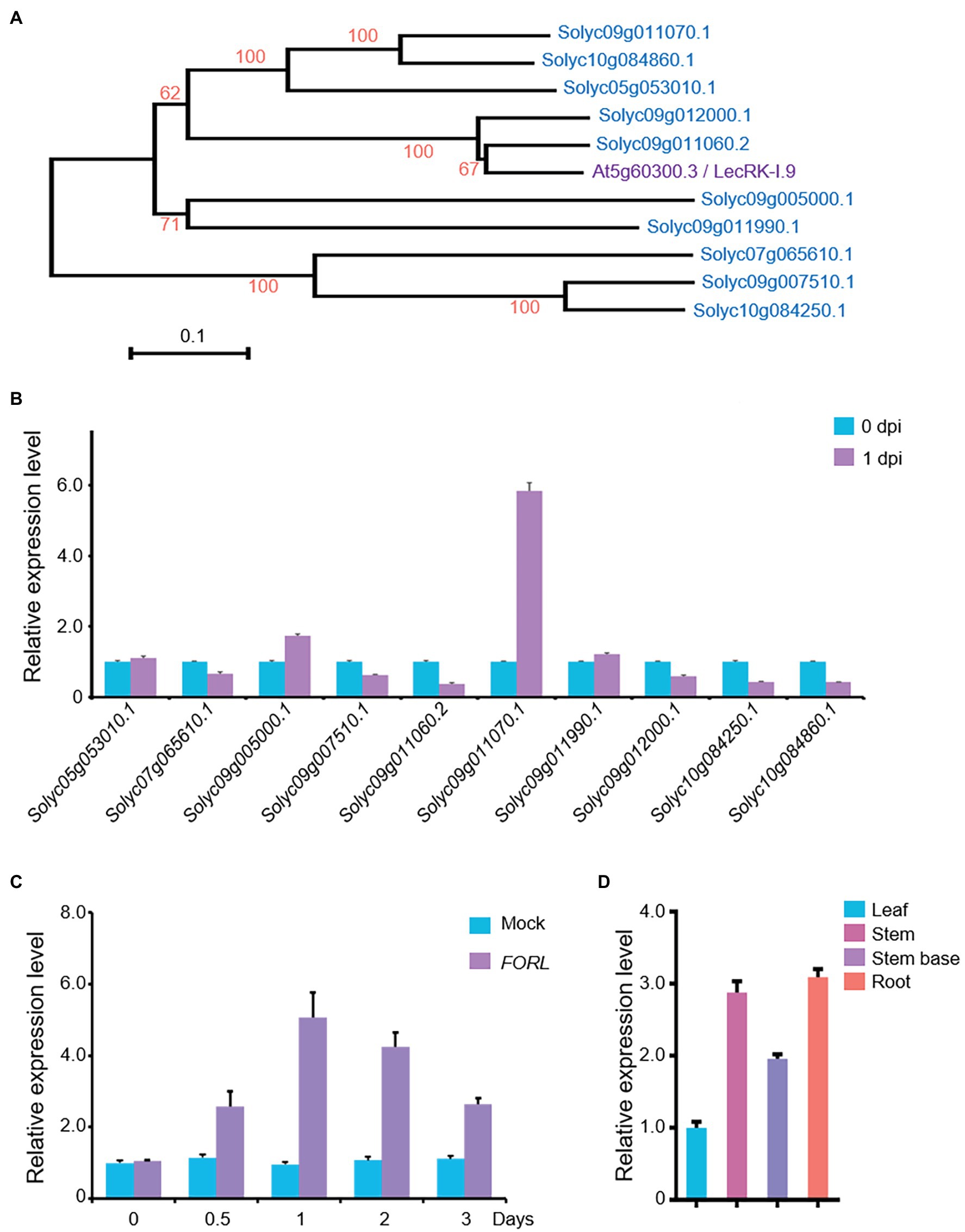
Figure 1. Expression of a LecRK-I.9 homolog in tomato in response to FORL. (A) A phylogenetic tree showing LecRK-I.9 and its homologs in tomato. Based on the predicted amino acid sequences using MEGA 6.0, the scale bar indicates the distance calculated from multiple alignments. (B) Relative expression levels of the LecRK-I.9 homolog following FORL treatment in 10-day-old WT tomato seedlings as measured by qRT-PCR. Total RNA was used for analysis. eEF1α served as a reference. The WT values at 0 dpi were set to 1. Data are shown as the mean ± standard deviation (SD) from three biological repeats. (C) Induced expression of Solyc09g011070.1 following FORL treatment at different time points. Total RNA was used for qRT-PCR analysis. eEF1α served as a reference. Ten-day-old WT tomato seedlings were treated with water (Mock) or FORL. Data are shown as the mean ± SD from three biological repeats. (D) Tissue-specific expression of SlLecRK1 in tomato seedlings. Total RNA from the indicated tissues was used for qRT-PCR analysis. eEF1α served as a reference. Leaf blade values were set to 1. Data are shown as the mean ± SD from three biological repeats.
Our qRT-PCR data further revealed that the mRNA expression level of Solyc09g011070.1 was induced by FORL treatment from 0.5 to 3 dpi and peaked at 1 dpi, compared with mock-treated control plants (Figure 1C). Interestingly, its mRNA expression levels in the roots, stems, and stem bases of the tomato seedlings were higher than that in the leaves (Figure 1D), consistent with our findings in the tomato plant tissues exposed to FORL through the soil. Ultimately, we assigned Solyc09g011070.1 the name SlLecRK1.
SlLecRK1 Encodes a LecRK
SlLecRK1 encodes a protein of 681 amino acids, which was predicted to be a LecRK by sequence alignment. The predicted extracellular N-terminal domain contains a predicted 21-residue cleavable secretory signal peptide and a typical legume lectin domain (between residues 27 and 266). The C-terminal intracellular region is predicted to contain a protein kinase domain (between residues 343 and 613; Figure 2A).
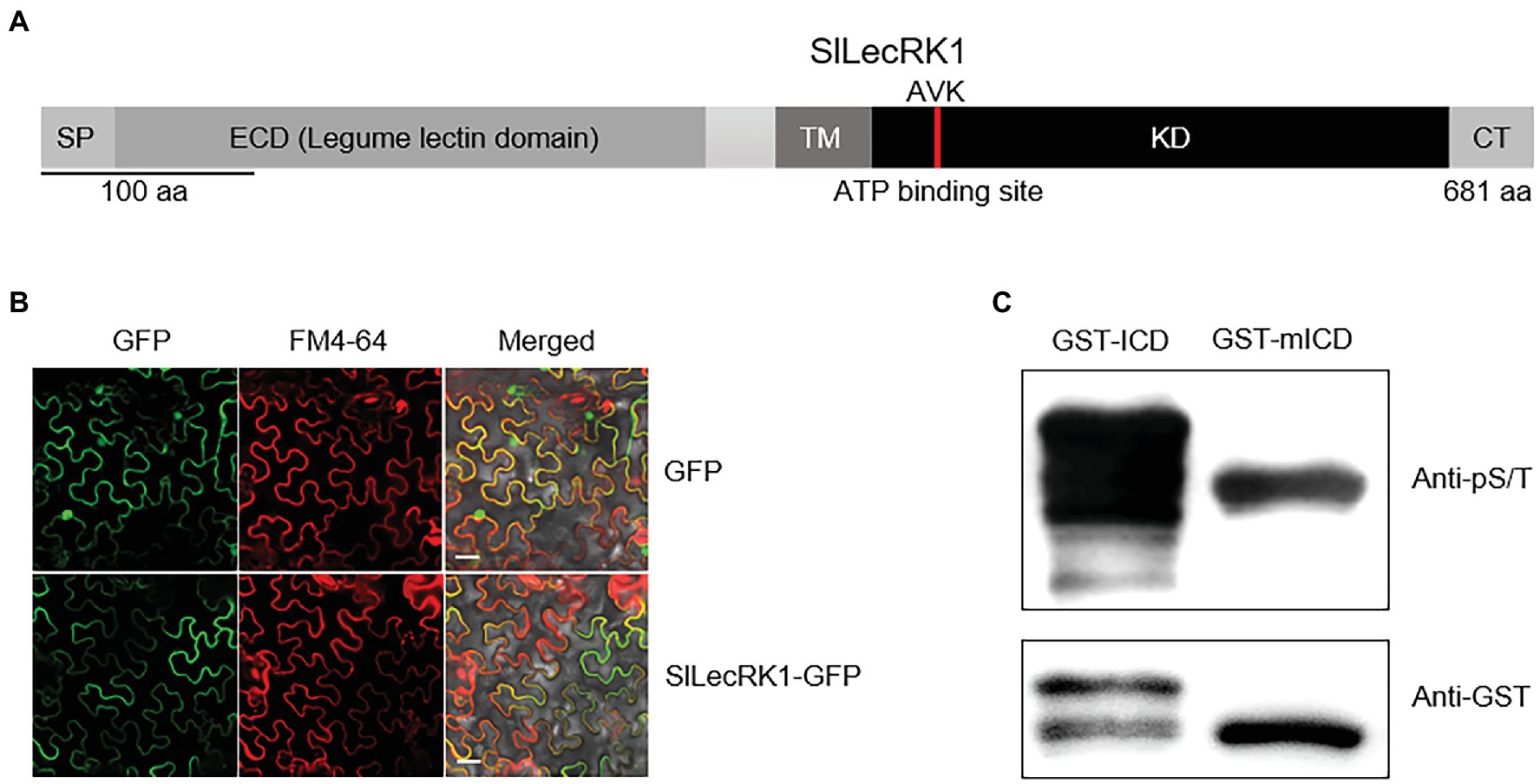
Figure 2. Characterization of SlLecRK1. (A) Diagram of the functional domains in SlLecRK1. SP, signal peptide; ECD, extracellular domain; TM, transmembrane domain; KD, kinase domain; CT, C-terminal region. The red bar in the KD marks the AVK motif (the ATP-binding site). (B) Confocal microscopic images of SlLecRK1Pro:SlLecRK1-GFP transiently expressed in N. benthamiana leaves. FM4-64 was used as a marker for the plasma membrane. Bars, 20 μm. (C) Immunoblot analysis of GST-ICD and GST-mICD recombinant proteins using antibodies specific for serine and threonine phosphorylation (pS/T). Anti-GST antibodies were used as a loading reference.
To validate the subcellular localization of SlLecRK1, SlLecRK1SPro:SlLecRK1-GFP and 35SPro: GFP (control) were transiently expressed in Nicotiana benthamiana leaves, respectively. Confocal observations revealed that the SlLecRK1-GFP fusion protein co-localized with the plasma membrane marker FM4-64, whereas the GFP control protein was located in the nucleus and at the cell surface (Figure 2B), indicating that SlLecRK1 localizes to the plasma membrane.
To determine whether SlLecRK1 possesses kinase activity, the conserved lysine (K372) in the ATP-binding region was mutated to generate SlLecRK1KDKA. The WT and mutated SlLecRK1 intracellular domains (ICDs) were expressed in Escherichia coli as glutathione S-transferase (GST) fusions, and the purified proteins were incubated with kinase buffer then detected with anti-phosphor-serine/−threonine antibodies (for details, see “Materials and Methods”). As shown in Figure 2C, the GST-ICD proteins were highly phosphorylated, whereas GST-mICD (mutated ICD) was barely phosphorylated, suggesting that SlLecRK1 has auto-phosphorylation activity and that K372 is required for this activity.
Together, these results suggested that SlLecRK1 is a typical LecRK in tomato.
Overexpression of SlLecRK1 Enhanced Tomato Resistance to FCRR
Given that SlLecRK1 was upregulated by inoculation with FORL, we predicted that enhancing the expression of SlLecRK1 might be required for tomato resistance to FCRR. We therefore generated transgenic tomato plants that overexpressed SlLecRK1. SlLecRK1Pro:SlLecRK1-Myc was transformed into WT calli, and then the transgenic plants were validated by hygromycin resistance screening and exogenous protein detection. We selected two independent lines, SlLecRK1-OE1 (OE-1) and -OE2 (OE-2), which expressed SlLecRK1-Myc, for further analysis (Figures 3A–D). Ten-day-old OE-1, OE-2, and WT seedlings were inoculated with FORL and then transferred to fresh medium. After 3 days of cultivation, the roots, stems, and leaves of the WT plants had rotted, whereas rotted tissues were found only at the stem bases of the two OE lines (Figure 3A). We also injected FORL into the stem bases of 30-day-old plants. Two weeks later, the WT roots had turned brown and rotted from the inside out, whereas in the OE plants only a few lateral roots showed browning and the main roots were not obviously affected (Figure 3B).
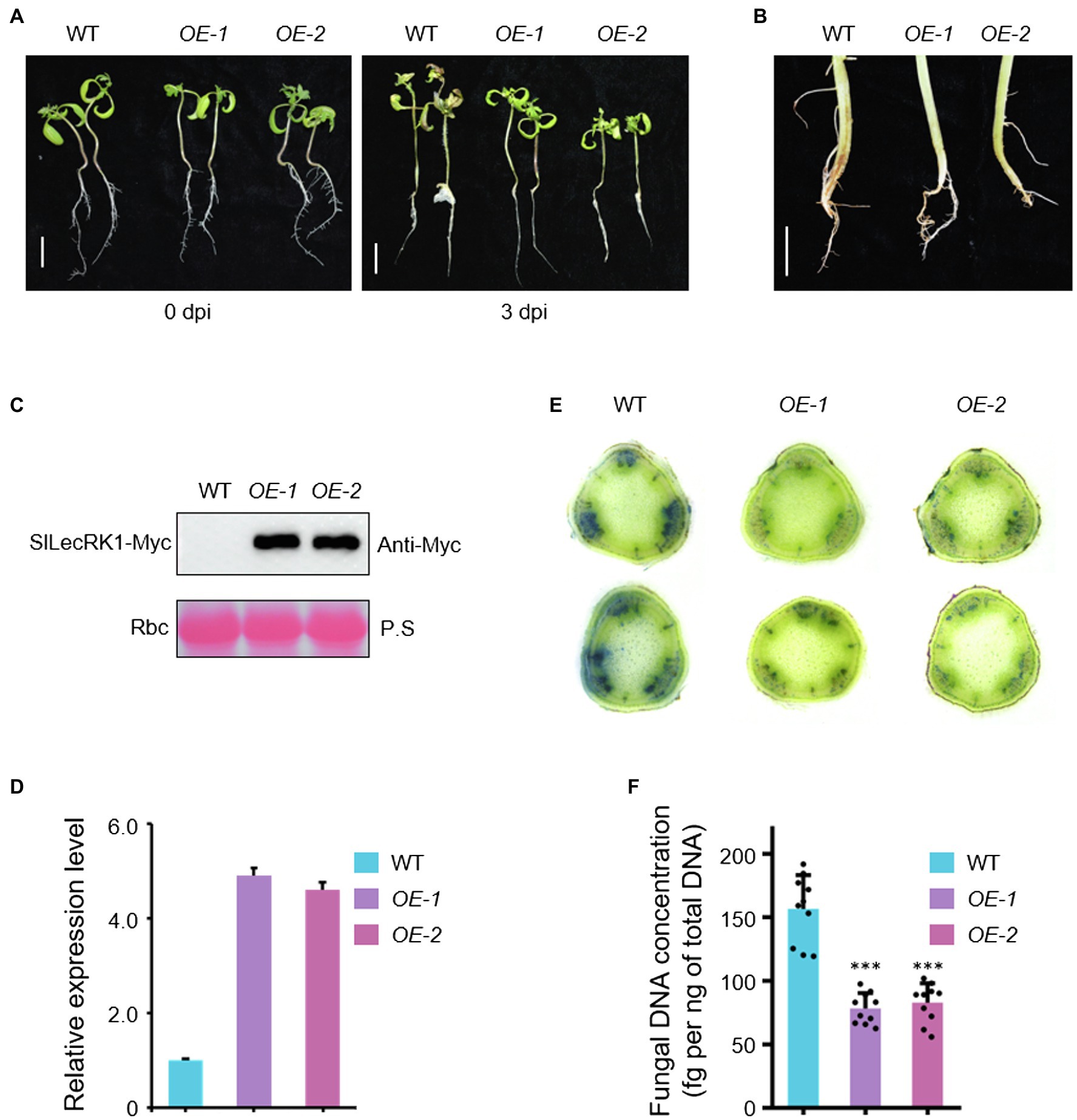
Figure 3. Phenotypes of SlLecRK1-OE plants in response to FCRR. (A) The post-FORL inoculation phenotypes of 10-day-old SlLecRK1-OE and WT tomato seedlings. Bar, 2 cm. (B) The post-FORL inoculation phenotypes of 30-day-old SlLecRK1-OE and WT tomato roots. Bar, 3 cm. (C) The SlLecRK1 protein levels in overexpression lines probed with anti-cMyc antibodies. Ponceau S (P.S.) staining of RubisCO (RBC) served as a loading reference. (D) The total SlLecRK1 mRNA levels in our overexpression lines. Total RNA was used for qRT-PCR analysis. eEF1α served as a reference. Data are shown as the mean ± SD from three biological repeats. (E) Cotton blue staining indicating FORL abundance. Stems from WT and OE plants after FORL inoculation for 2 weeks were stained with cotton blue. Representative images of WT and OE plants are shown. Bars, 1 mm. (F) Fungal DNA concentration in WT and OE plants after FORL inoculation for 2 weeks. Different letters denote significant differences by Student’s t-test (***p < 0.001), n = 10.
We next compared fungal accumulation at the stem in OE and WT plants at 2 weeks after FORL infestation. Cotton blue staining revealed fewer fungi in the stems of OE-1 and OE-2 plants compared to wild type (Figure 3E). Consistently, the concentrations of FORL DNA in OE-1 and OE-2 were about half of that in WT plants (Figure 3F). We also observed SlLecRK1 accumulation following inoculation with FORL (Figure 4), indicating the inhibitory effects of increased SlLecRK1 expression on fungal growth.
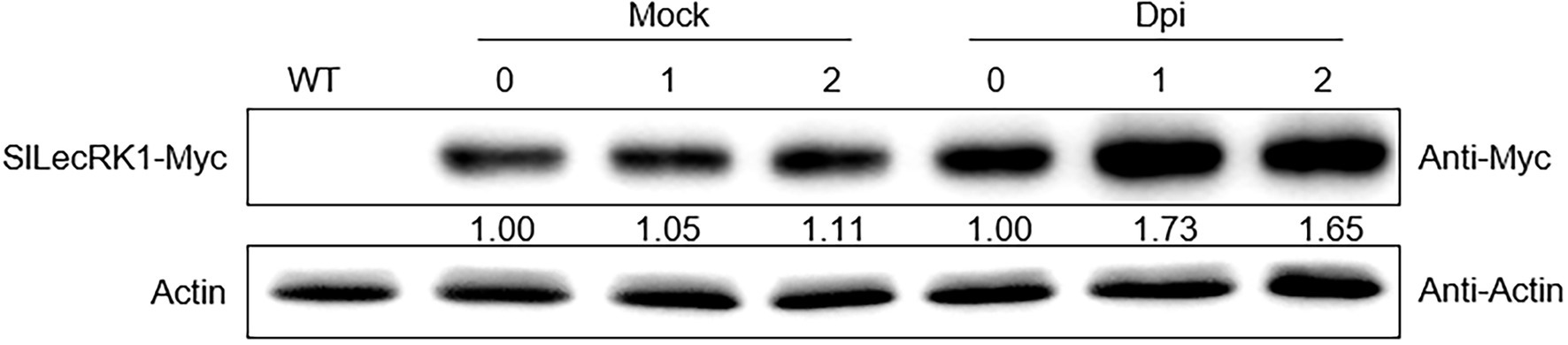
Figure 4. SlLecRK1 Accumulation Following Inoculation with FORL. Ten-day-old SlLecRK1-OE1 seedlings were inoculated (or not) with FORL for the indicated number of days, after which SlLecRK1 protein was detected using anti-cMyc antibodies. The signal intensity was quantified and normalized to that of actin. The values for the day 0 samples were set to 1.
Several agronomic traits were subsequently investigated in SlLecRK1 OE plants. We found no visible difference in the fruits, leaves, flowers, and yields between our OE and WT plants (Figure 5; Supplementary Figure S1). These observations suggested that the overexpression of SlLecRK1 does not alter plant growth and development but can improve the resistance of tomato plants to FORL; thus, SlLecRK1 is an ideal candidate for use in future molecular breeding programs.
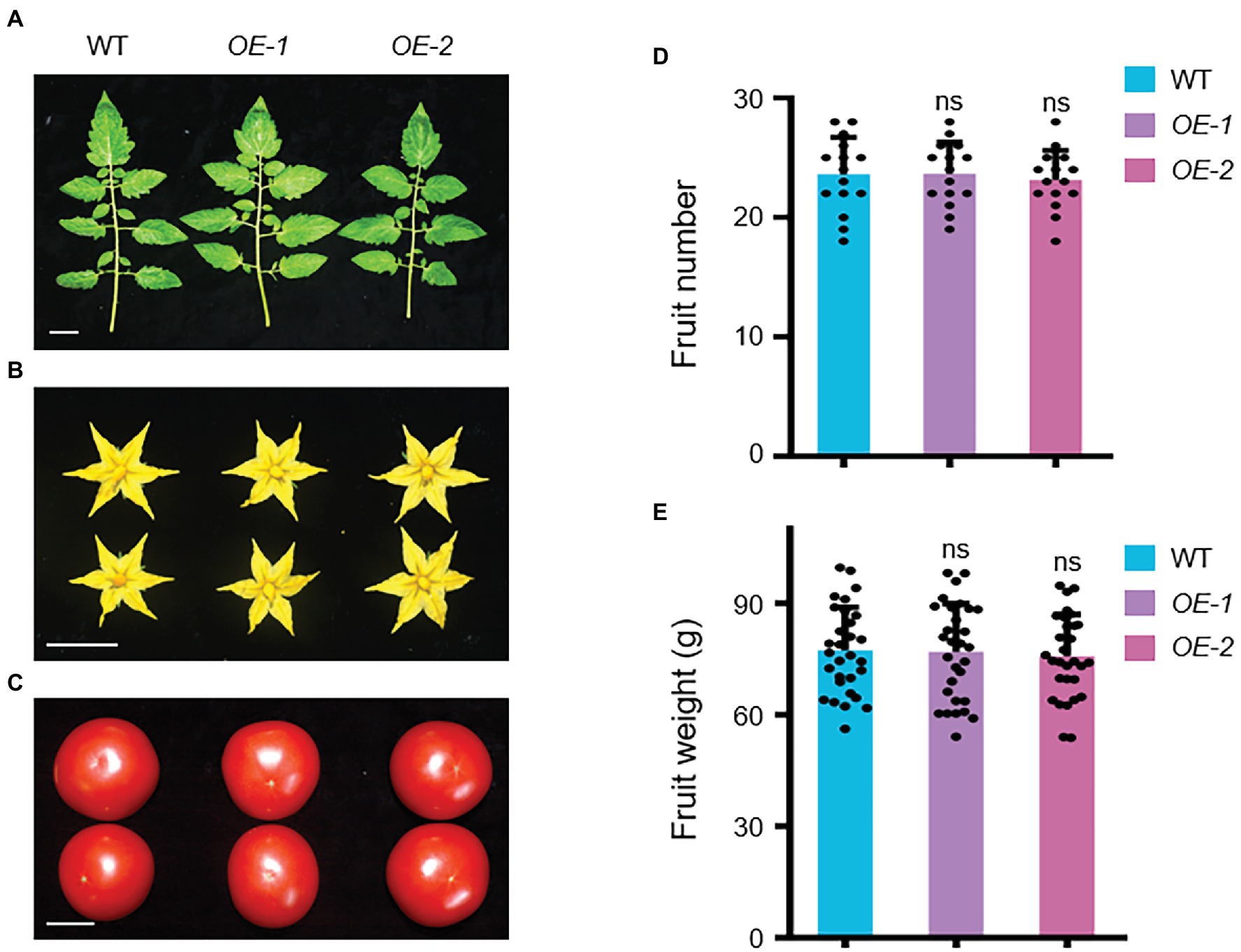
Figure 5. Agronomic Traits of SlLecRK1-OE Plants. The leaves (A), flowers (B), and fruits (C) were observed in two OE plants compared with the WT control at the harvest stage. Bars, 3 cm. (D) The statistical data for fruit number (n = 10 plants). (E) The statistical data for fruit weight (n = 30 from 10 plants). Different letters denote significant differences by Student’s t-test (ns, not significant).
Upregulation of ERF Genes in the SlLecRK1-OE1 Lines May Have Contributed to Improve FORL Resistance
To clarify the molecular basis for the enhanced resistance of the OE lines, we performed a transcriptome analysis using WT and OE-1 (OE) seedlings without inoculation and at 1 day post-inoculation (dpi) with FORL at the stem base; they were designated as WT-0dpi, WT-1dpi, OE-0dpi, and OE-1dpi, respectively. Three biological replicates were prepared for each sample, from which approximately 534.2 million 150-bp paired-end reads were generated (Supplementary Table S2). After discarding low-quality reads, 41.4 to 50.2 million reads were kept for subsequent analysis. More than 85% of reads containing no more than three mismatches were mapped to the reference genome, and more than 80% of the reads showed unique alignments with the samples without FORL inoculation, of which the percentage of corresponding reads was much higher than that for the samples after FORL inoculation (Supplementary Table S2). Principal component analysis (PCA) demonstrated high reproducibility among the biological replicates (Supplementary Figure S2). Furthermore, PCA revealed that the divergence in the expression profiles of the samples was primarily explained by FORL inoculation and that FORL inoculation amplified the difference in expression profiles between the OE and WT plants (Supplementary Figure S2).
To identify biological pathways that may be modulated by SlLecRK1 during FORL inoculation, we initially compared the transcriptomes of OE and WT plants under control conditions (0 dpi: OE/WT) and at 1 dpi (1 dpi: OE/WT) using a fold change >1.5 and false discovery rate (FDR) < 0.05 as our cutoff values. In total, we identified 186 and 861 upregulated genes and 144 and 569 downregulated genes in the OE compared to the WT plants at 0 dpi and 1 dpi, respectively (Figure 6A; Supplementary Dataset S1). Consistent with our PCA results, overexpression of SlLecRK1 resulted in a much greater number of differentially expressed genes (DEGs) at 1 dpi (1430) than under control conditions (330; Figure 6A; Supplementary Dataset S1). Moreover, the number of upregulated genes was higher than that of downregulated genes under both conditions (Figure 6A; Supplementary Dataset S1). Compared with 0 dpi: OE/WT comparison, 782 (90.82%) upregulated and 533 (93.67%) downregulated genes were found only in our 1 dpi: OE/WT comparison (Figure 6B); these were designated 1dpi-specific genes. Next, we examined whether the 1dpi-specific genes mediate tomato resistance to FORL inoculation. First, we evaluated transcriptional changes in response to FORL inoculation in WT and OE plants; these were designated WT:1dpi/0dpi and OE:1dpi/0dpi, respectively. Using a |log2-transformed fold change| ≥ 2 and FDR < 0.05 as cutoff values, we identified 4,131 DEGs in the WT:1dpi/0dpi comparison, of which 2,174 and 1957 genes were upregulated and downregulated, respectively (Figure 6C; Supplementary Dataset S1). The numbers of DEGs (4952), upregulated genes (2416), and downregulated genes (2536) in the OE plants were all higher than those in wild type (Figure 6C; Supplementary Dataset S1). Subsequent comparisons revealed that 87.07% of the activated (1893/2174) and 72.30% of the repressed (1,415/1957) genes in WT plants were also upregulated or downregulated in the OE plants (Figure 6D). Collectively, these results suggested that a comparable but more extensive and dynamic transcriptome reprofiling occurred in the OE plants following FORL inoculation.
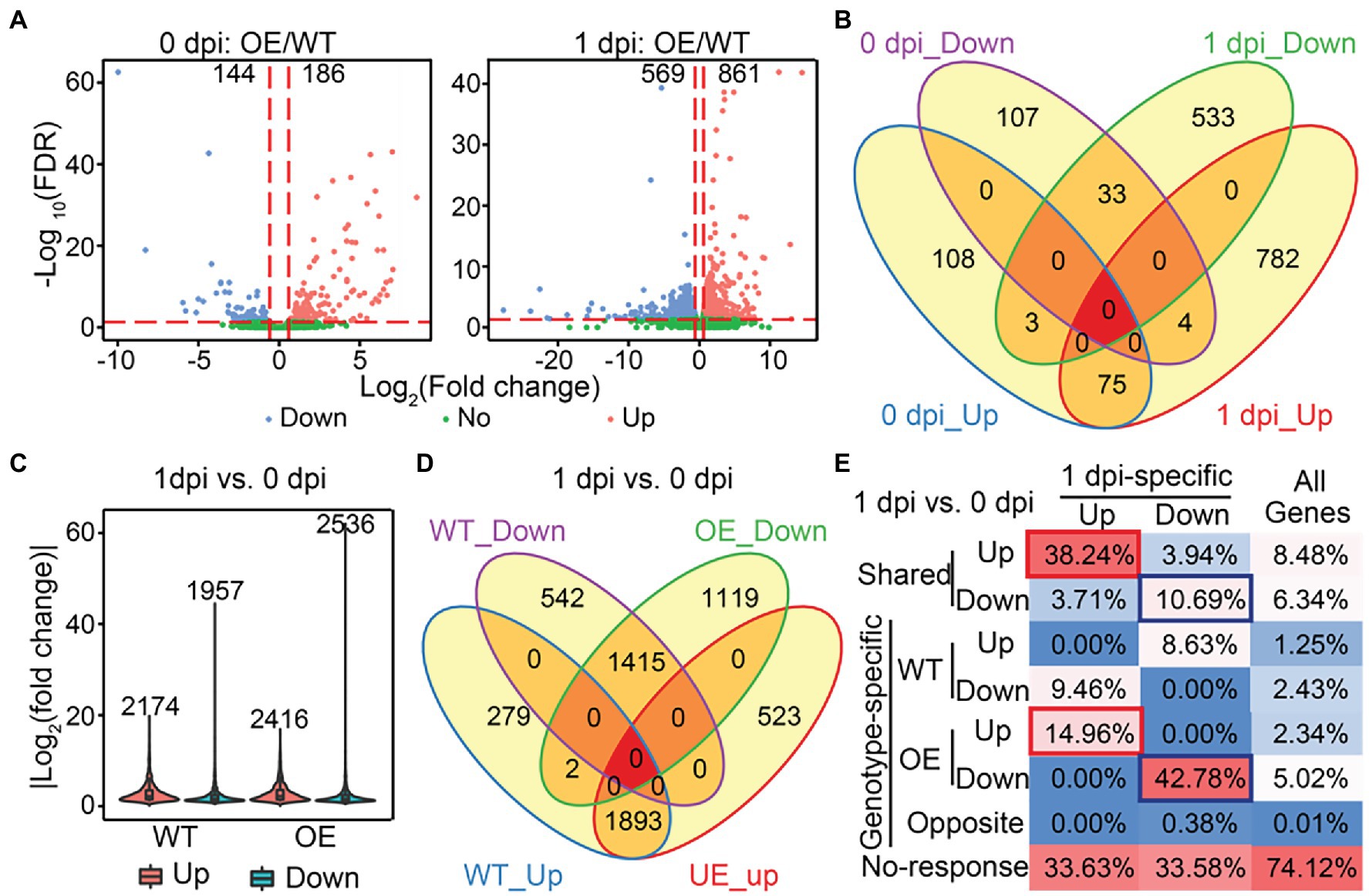
Figure 6. Identification of SlLecRK1-regulated genes during resistance to FORL inoculation. (A) Volcano plots of the transcriptional changes were generated using edgeR. The log2 of the fold change is shown on the horizontal axis, and the -log10 of the adjusted value of p (FDR) is shown on the vertical axis. Red and blue dots represent significantly upregulated and downregulated genes, respectively. Green dots are genes with a non-significant change. The vertical and horizontal red dashed lines indicate the cutoff of the log2-transformed fold change and -log10 of the FDR. Numbers indicate the counts for genes showing upregulation and downregulation. (B) A Venn diagram showing the numbers of upregulated and downregulated genes between OE-1 and WT plants at 0 and 1 dpi. (C) The numbers and fold change distributions of DEGs regulated by FORL inoculation in WT and OE-1 plants. (D) A Venn diagram showing the comparison of DEGs induced by FORL inoculation between WT and OE-1 plants. (E) The categories and percentages of 1dpi-specific upregulated and downregulated genes according to the DEGs caused by FORL inoculation in WT and OE-1 plants. Red and blue boxes indicate type I and type II target genes, respectively. “All genes” indicates genes that were subjected to differential expression analysis.
Subsequently, we examined the relationships between the SlLecRK1-mediated genes and FORL-triggered DEGs to determine the target genes whose transcription was affected by SlLecRK1 during FORL inoculation. Interestingly, FORL-responsive genes were highly enriched among the 1dpi-specific genes compared with the background (Figure 6E). Among 782 1dpi-specific upregulated genes, 299 (38.24%) and 117 (14.96%) genes, referred to as type I target genes, exhibited shared and OE-unique increased expression levels after FORL inoculation (Figure 6E; Supplementary Dataset S2), respectively. Meanwhile, 57 (10.69%) and 228 (42.78%) out of 533 1dpi-specific downregulated genes, referred to as type II target genes, exhibited both shared and OE-exclusive repression following FORL inoculation (Figure 6E; Supplementary Dataset S2), respectively. Considering that SlLecRK1 is a positive regulator of FORL resistance, the type I target genes were subjected to further analysis. Unexpectedly, Gene Ontology (GO) analysis revealed that no specific GO terms were significantly enriched among the type I target genes. Still, some transcription factors (TFs) or transcriptional regulators (TRs) might play important regulatory roles in plant resistance to FORL. Using the iTAK pipeline (Zheng et al., 2016), we identified and classified 38 putative TF (35) and TR (3) members from 20 families among the type I target genes, including nine genes encoding ethylene (ET)-responsive TFs (ERFs).
ERF gene family members have been identified as key mediators of pathogen responses and ET signaling plays important roles in defense responses to necrotrophic pathogens in plants (Spoel and Dong, 2008; Manzo-Valencia et al., 2016). We therefore used qRT-PCR to validate the expression levels of five selected ERFs. Consistent with our RNA-Seq data, our qRT-PCR results revealed that the expression levels of the five ERFs were substantially elevated in both OE-1 and OE-2 plants compared with wild type after FORL inoculation (Figure 7). Together, these findings suggest that the upregulation of ERF gene expression in response to increased SlLecRK1 expression contributed to the improved FORL resistance of tomato plants.
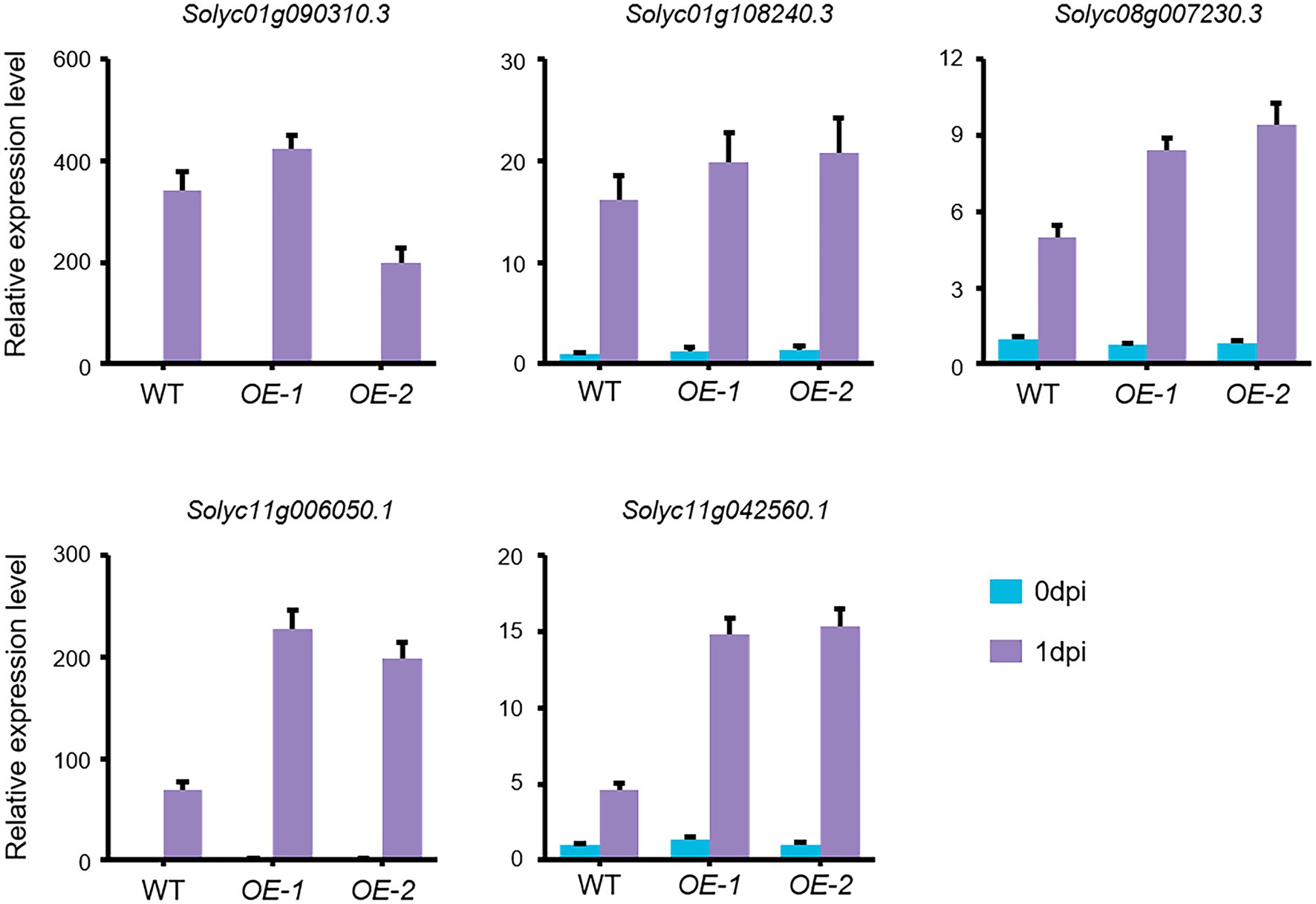
Figure 7. FORL-Induced ERF Expression in SlLecRK1-OE plants. The expression of five ERF genes in WT, OE-1, and OE-2 plants before (0 dpi) and after (1 dpi) FORL inoculation is shown. Total RNA was used for qRT-PCR analysis. eEF1α served as a reference. The WT values at 0 dpi were set to 1. Data are shown as the mean ± SD from three biological repeats.
Discussion
SlLecRK1, which is mainly expressed in the roots and stem bases of tomato plants, is a membrane-localized RLK. SlLecRK1 belongs to the LecRK subfamily in tomato, and it shows 44% identity to LecRK-I.9/DORN1 in Arabidopsis. The L-lectin domain in its extracellular region is predicted to bind monosaccharides and polypeptides, and it is regarded as a potential linker of the plasma membrane to the cell wall, which is the first barrier of plant cells against environmental stimuli (Vaid et al., 2013). Furthermore, SlLecRK1 mRNA and protein are mainly expressed in roots and stems, and their expression levels are increased by FORL inoculation. These features render SlLecRK1 able to directly resist FORL in soil. Importantly, overexpression of SlLecRK1 does not affect tomato plant growth and development. These data indicate that SlLecRK1 has great potential for use in molecular breeding programs aimed at developing FORL-resistant tomato varieties.
SlLecRK1 triggers ET signaling, which mediates defense responses in tomato. ET biosynthesis and signaling are not only modulated by multiple environmental factors such as light, temperature, mechanical pressure, and biotic stress, they also are involved in the regulation of plant growth, development, fruit ripening, and senescence (Cao et al., 2007; Bari and Jones, 2009; Merchante et al., 2013; Wang et al., 2013; Garcia et al., 2015; Larsen, 2015; Huang et al., 2016; Shi et al., 2016; Fei et al., 2019; Seo and Yoon, 2019; Binder, 2020; Husain et al., 2020; Li et al., 2020; Riyazuddin et al., 2020). ERF is a large TF family in plants. ERFs usually bind to the GCC-box cis-element GCCGCC, which is frequently present in the promoter region of pathogen-induced genes (Pre et al., 2008; Meng et al., 2013; Catinot et al., 2015). For instance, in Arabidopsis ERF6 activates two defense-related genes, PDF1.1 and PDF1.2, via an ET-independent pathway in response to infection by the necrotrophic pathogen B. cinerea (Meng et al., 2013). Meanwhile, AtERF72 is a positive regulator that mediates resistance to B. cinerea by activating the transcription of camalexin-biosynthesis enzyme genes (Li et al., 2021). There are 134 ERFs in tomato, and SlERF01 and SlERF2 positively regulate resistance to Stemphylium lycopersici (Yang et al., 2020, 2021). SlERF.A1, SlERF.B4, SlERF.C3, and SlERF.A3 are required for resistance to B. cinerea in tomato (Ouyang et al., 2016). Based on our RNA-Seq and qRT-PCR data, we found that the expression levels of at least five ERFs between WT and SlLecRK1-OE plants were slightly different before inoculation, whereas the expression levels of these ERFs in SlLecRK1-OE plants were significantly higher than those in WT plants after inoculation. These results suggest that SlLecRK1 improves FORL resistance in tomato by enhancing ERF expression. They also explain the phenotypic outcome observed in SlLecRK1-OE plants following FORL infestation. We also found that SlLecRK1 did not alter ERF expression in the absence of FORL, while expression was induced by FORL infestation. This indicates that the positive effects of SlLecRK1 on FORL infestation depend not just on its abundance; it is also likely achieved by another mechanism such as the activation of SlLecRK1 kinase activity by FORL. Given these results, we can easily understand why SlLecRK1 overexpression had a reduced impact on tomato plant growth and development. Additionally, SlLecRK1 triggers ET-related gene expression, probably via a conserved LecRK-regulated mechanism that has been reported in other plant species under different conditions (Li et al., 2014; Jewell and Tanaka, 2019).
Recently, the Fusarium crown and root rot resistance (Frl) locus, which confers resistance to FCRR, was mapped to a 900-kb region of chromosome 9 (Devran et al., 2018). Interestingly, SlLecRK1 is also located in this region. It will be interesting to test whether SlLecRK1 is Frl and to identify the association of SlLecRK1 with FCRR resistance using natural populations. In summary, we discovered that SlLecRK1 is a specific RLK that regulates the resistance of tomato plants to FORL. Additional studies aimed at uncovering the ligands and new component(s) of the SlLecRK1-regulated signaling pathway will aid in clarifying the regulatory mechanism underlying defensive responses to FORL and promote tomato improvement.
Data Availability Statement
The original contributions presented in the study are publicly available. This data can be found at: National Center for Biotechnology Information (NCBI) BioProject database under accession number PRJNA791339.
Author Contributions
Z-MW and Z-LY: conceptualization and funding acquisition. Z-LY: methodology, data curation, and writing—original draft preparation. Z-LY, Z-JT, J-WZ, and Y-DL: validation. Z-LY, S-WZ, and Z-MW: writing—review and editing. Z-MW: supervision and project administration. All authors contributed to the article and approved the submitted version.
Funding
This research was funded by Postdoctoral Research Foundation of Hebei Province (B2020003040). Talents Construction Project of Science and Technology Innovation, Hebei Academy of Agriculture and Forestry Sciences (2019-10-10). S&T Program of Hebei (19226342D).
Conflict of Interest
The authors declare that the research was conducted in the absence of any commercial or financial relationships that could be construed as a potential conflict of interest.
Publisher’s Note
All claims expressed in this article are solely those of the authors and do not necessarily represent those of their affiliated organizations, or those of the publisher, the editors and the reviewers. Any product that may be evaluated in this article, or claim that may be made by its manufacturer, is not guaranteed or endorsed by the publisher.
Acknowledgments
We thank Dr. Xin-Ye Liu (Hebei Normal University, China) for analyzing the RNA-Seq data.
Supplementary Material
The Supplementary Material for this article can be found online at: https://www.frontiersin.org/articless/10.3389/fpls.2022.836269/full#supplementary-material
Footnotes
1. ^http://www.bioinformatics.babraham.ac.uk/projects/fastqc/
2. ^https://github.com/najoshi/sickle
3. ^http://www-huber.embl.de/users/anders/HTSeq/doc/overview.html
4. ^https://github.com/js229/Vennerable
5. ^https://imagej.nih.gov/ij/
6. ^https://phytozome-next.jgi.doe.gov/info/Slycopersicum_ITAG4_0
References
Bari, R., and Jones, J. D. (2009). Role of plant hormones in plant defence responses. Plant Mol. Biol. 69, 473–488. doi: 10.1007/s11103-008-9435-0
Bellande, K., Bono, J. J., Savelli, B., Jamet, E., and Canut, H. (2017). Plant Lectins and Lectin receptor-Like kinases: how do they sense the outside? Int. J. Mol. Sci. 18:1164. doi: 10.3390/ijms18061164
Benhamou, N., and Belanger, R. R. (1998). Benzothiadiazole-mediated induced resistance to Fusarium oxysporum f. sp. radicis-lycopersici in tomato. Plant Physiol. 118, 1203–1212. doi: 10.1104/pp.118.4.1203
Binder, B. M. (2020). Ethylene signaling in plants. J. Biol. Chem. 295, 7710–7725. doi: 10.1074/jbc.REV120.010854
Bouwmeester, K., and Govers, F. (2009). Arabidopsis L-type lectin receptor kinases: phylogeny, classification, and expression profiles. J. Exp. Bot. 60, 4383–4396. doi: 10.1093/jxb/erp277
Campos, L., Lopez-Gresa, M. P., Fuertes, D., Belles, J. M., Rodrigo, I., and Lison, P. (2019). Tomato glycosyltransferase T wi1 plays a role in flavonoid glycosylation and defence against virus. BMC Plant Biol. 19:450. doi: 10.1186/s12870-019-2063-9
Cao, W. H., Liu, J., He, X. J., Mu, R. L., Zhou, H. L., Chen, S. Y., et al. (2007). Modulation of ethylene responses affects plant salt-stress responses. Plant Physiol. 143, 707–719. doi: 10.1104/pp.106.094292
Catinot, J., Huang, J. B., Huang, P. Y., Tseng, M. Y., Chen, Y. L., Gu, S. Y., et al. (2015). ETHYLENE RESPONSE FACTOR 96 positively regulates Arabidopsis resistance to necrotrophic pathogens by direct binding to GCC elements of jasmonate and ethylene-responsive defence genes. Plant Cell Environ. 38, 2721–2734. doi: 10.1111/pce.12583
Chen, D., Cao, Y., Li, H., Kim, D., Ahsan, N., Thelen, J., et al. (2017). Extracellular ATP elicits DORN1-mediated RBOHD phosphorylation to regulate stomatal aperture. Nat. Commun. 8:2265. doi: 10.1038/s41467-017-02340-3
Chin, AWTF Bloemberg, G. V., Mulders, I. H., Dekkers, L. C., and Lugtenberg, B. J. (2000). Root colonization by phenazine-1-carboxamide-producing bacterium pseudomonas chlororaphis PCL1391 is essential for biocontrol of tomato foot and root rot. Mol. Plant Microbe. Interact. 13, 1340–1345. doi: 10.1094/MPMI.2000.13.12.1340
Choi, J., Tanaka, K., Liang, Y., Cao, Y., Lee, S. Y., and Stacey, G. (2014). Extracellular ATP, a danger signal, is recognized by DORN1 in Arabidopsis. Biochem. J. 463, 429–437. doi: 10.1042/BJ20140666
Cordero-Ramirez, J. D., Lopez-Rivera, R., Figueroa-Lopez, A. M., Mancera-Lopez, M. E., Martinez-Alvarez, J. C., Apodaca-Sanchez, M. A., et al. (2013). Native soil bacteria isolates in Mexico exhibit a promising antagonistic effect against Fusarium oxysporum f. sp. radicis-lycopersici. J. Basic Microbiol. 53, 838–847. doi: 10.1002/jobm.201200128
Devran, Z., Kahveci, E., Hong, Y., Studholme, D. J., and Tor, M. (2018). Identifying molecular markers suitable for Frl selection in tomato breeding. Theor. Appl. Genet. 131, 2099–2105. doi: 10.1007/s00122-018-3136-0
Fei, Q., Zhang, J., Zhang, Z., Wang, Y., Liang, L., Wu, L., et al. (2019). Effects of auxin and ethylene on root growth adaptation to different ambient temperatures in Arabidopsis. Plant Sci. 281, 159–172. doi: 10.1016/j.plantsci.2019.01.018
Garcia, M. J., Romera, F. J., Lucena, C., Alcantara, E., and Perez-Vicente, R. (2015). Ethylene and the regulation of physiological and morphological responses to nutrient deficiencies. Plant Physiol. 169, 51–60. doi: 10.1104/pp.15.00708
Gordon, T. R. (2017). Fusarium oxysporum and the Fusarium wilt syndrome. Annu. Rev. Phytopathol. 55, 23–39. doi: 10.1146/annurev-phyto-080615-095919
Gouhier-Darimont, C., Stahl, E., Glauser, G., and Reymond, P. (2019). The Arabidopsis Lectin receptor kinase LecRK-I.8 is involved in insect egg perception. Front. Plant Sci. 10:623. doi: 10.3389/fpls.2019.00623
Grauwet, T. J., De Raedemaecker, J. H., Krause, M. S., Vanden, D. S., Vanachter, A. C., Aerts, R., et al. (2005). Optimization of biological control of fungal root diseases in tomato in stone wool culture. Commun. Agric. Appl. Biol. Sci. 70, 175–180.
Huang, P. Y., Catinot, J., and Zimmerli, L. (2016). Ethylene response factors in Arabidopsis immunity. J. Exp. Bot. 67, 1231–1241. doi: 10.1093/jxb/erv518
Husain, T., Fatima, A., Suhel, M., Singh, S., Sharma, A., Prasad, S. M., et al. (2020). A brief appraisal of ethylene signaling under abiotic stress in plants. Plant Signal. Behav. 15:1782051. doi: 10.1080/15592324.2020.1782051
Jewell, J. B., and Tanaka, K. (2019). Transcriptomic perspective on extracellular ATP signaling: a few curious trifles. Plant Signal. Behav. 14:1659079. doi: 10.1080/15592324.2019.1659079
Kavroulakis, N., Ntougias, S., Zervakis, G. I., Ehaliotis, C., Haralampidis, K., and Papadopoulou, K. K. (2007). Role of ethylene in the protection of tomato plants against soil-borne fungal pathogens conferred by an endophytic Fusarium solani strain. J. Exp. Bot. 58, 3853–3864. doi: 10.1093/jxb/erm230
Kim, D., Pertea, G., Trapnell, C., Pimentel, H., Kelley, R., and Salzberg, S. L. (2013). TopHat2: accurate alignment of transcriptomes in the presence of insertions, deletions and gene fusions. Genome Biol. 14:R36. doi: 10.1186/gb-2013-14-4-r36
Kong, L., Rodrigues, B., Kim, J. H., He, P., and Shan, L. (2021). More than an on-and-off switch: post-translational modifications of plant pattern recognition receptor complexes. Curr. Opin. Plant Biol. 63:102051. doi: 10.1016/j.pbi.2021.102051
Larsen, P. B. (2015). Mechanisms of ethylene biosynthesis and response in plants. Essays Biochem. 58, 61–70. doi: 10.1042/bse0580061
Li, Z., Liu, H., Ding, Z., Yan, J., Yu, H., Pan, R., et al. (2020). Low temperature enhances plant immunity via salicylic acid pathway genes That are repressed by ethylene. Plant Physiol. 182, 626–639. doi: 10.1104/pp.19.01130
Li, Y., Liu, K., Tong, G., Xi, C., Liu, J., Zhao, H., et al. (2021). MPK3/MPK6-mediated ERF72 phosphorylation positively regulates resistance to Botrytis cinerea Through directly and indirectly activating the transcription of Camalexin-biosynthesis enzymes. J. Exp. Bot. 73, 413–428. doi: 10.1093/jxb/erab415
Li, C. H., Wang, G., Zhao, J. L., Zhang, L. Q., Ai, L. F., Han, Y. F., et al. (2014). The receptor-Like kinase SIT1 mediates salt sensitivity by activating MAPK3/6 and regulating ethylene homeostasis in Rice. Plant Cell 26, 2538–2553. doi: 10.1105/tpc.114.125187
Manzo, D., Ferriello, F., Puopolo, G., Zoina, A., D’Esposito, D., Tardella, L., et al. (2016). Fusarium oxysporum f.sp. radicis-lycopersici induces distinct transcriptome reprogramming in resistant and susceptible isogenic tomato lines. BMC Plant Biol. 16:53. doi: 10.1186/s12870-016-0740-5
Manzo-Valencia, M. K., Valdes-Santiago, L., Sanchez-Segura, L., and Guzman-de-Pena, D. L. (2016). Naphthalene acetic acid potassium salt (NAA-K(+)) affects conidial germination, sporulation, Mycelial growth, cell surface morphology, and viability of Fusarium oxysporum f. sp. radici-lycopersici and F. oxysporum f. sp. cubense in vitro. J. Agric. Food Chem. 64, 8315–8323. doi: 10.1021/acs.jafc.6b03105
Mazzeo, M. F., Cacace, G., Ferriello, F., Puopolo, G., Zoina, A., Ercolano, M. R., et al. (2014). Proteomic investigation of response to FORL infection in tomato roots. Plant Physiol. Biochem. 74, 42–49. doi: 10.1016/j.plaphy.2013.10.031
Meng, X., Xu, J., He, Y., Yang, K. Y., Mordorski, B., Liu, Y., et al. (2013). Phosphorylation of an ERF transcription factor by Arabidopsis MPK3/MPK6 regulates plant defense gene induction and fungal resistance. Plant Cell 25, 1126–1142. doi: 10.1105/tpc.112.109074
Merchante, C., Alonso, J. M., and Stepanova, A. N. (2013). Ethylene signaling: simple ligand, complex regulation. Curr. Opin. Plant Biol. 16, 554–560. doi: 10.1016/j.pbi.2013.08.001
Monaghan, J., and Zipfel, C. (2012). Plant pattern recognition receptor complexes at the plasma membrane. Curr. Opin. Plant Biol. 15, 349–357. doi: 10.1016/j.pbi.2012.05.006
Myresiotis, C. K., Karaoglanidis, G. S., Vryzas, Z., and Papadopoulou-Mourkidou, E. (2012). Evaluation of plant-growth-promoting rhizobacteria, acibenzolar-S-methyl and hymexazol for integrated control of Fusarium crown and root rot on tomato. Pest Manag. Sci. 68, 404–411. doi: 10.1002/ps.2277
Nefzi, A., Abdallah, R. A. B., Jabnoun-Khiareddine, H., Ammar, N., and Daami-Remadi, M. (2019). Ability of endophytic fungi associated with Withania somnifera L. to control Fusarium crown and root rot and to promote growth in tomato. Braz. J. Microbiol. 50, 481–494. doi: 10.1007/s42770-019-00062-w
Nurnberger, T., and Kemmerling, B. (2006). Receptor protein kinases – pattern recognition receptors in plant immunity. Trends Plant Sci. 11, 519–522. doi: 10.1016/j.tplants.2006.09.005
Oh, M. H., Kim, H. S., Wu, X., Clouse, S. D., Zielinski, R. E., and Huber, S. C. (2012). Calcium/calmodulin inhibition of the Arabidopsis BRASSINOSTEROID-INSENSITIVE 1 receptor kinase provides a possible link between calcium and brassinosteroid signalling. Biochem. J. 443, 515–523. doi: 10.1042/BJ20111871
Ouyang, Z., Liu, S., Huang, L., Hong, Y., Li, X., Huang, L., et al. (2016). Tomato SlERF.A1, SlERF.B4, SlERF.C3 and SlERF.A3, members of B3 group of ERF family, are required for resistance to Botrytis cinerea. Front. Plant Sci. 7:1964. doi: 10.3389/fpls.2016.01964
Park, C. J., Caddell, D. F., and Ronald, P. C. (2012). Protein phosphorylation in plant immunity: insights into the regulation of pattern recognition receptor-mediated signaling. Front. Plant Sci. 3:177. doi: 10.3389/fpls.2012.00177
Piazza, S., Campa, M., Pompili, V., Costa, L. D., Salvagnin, U., Nekrasov, V., et al. (2021). The Arabidopsis pattern recognition receptor EFR enhances fire blight resistance in apple. Hortic. Res. 8:204. doi: 10.1038/s41438-021-00639-3
Pizarro, L., Leibman-Markus, M., Schuster, S., Bar, M., Meltz, T., and Avni, A. (2018). Tomato Prenylated RAB acceptor protein 1 modulates trafficking and degradation of the pattern recognition receptor LeEIX2, affecting the innate immune response. Front. Plant Sci. 9:257. doi: 10.3389/fpls.2018.00257
Polizzi, G., Aiello, D., Guarnaccia, V., Vitale, A., Perrone, G., and Stea, G. (2011). First report of Fusarium wilt on Philotheca myoporoides caused by Fusarium oxysporum in Italy. Plant Dis. 95:877. doi: 10.1094/PDIS-03-11-0243
Pre, M., Atallah, M., Champion, A., De Vos, M., Pieterse, C. M., and Memelink, J. (2008). The AP2/ERF domain transcription factor ORA59 integrates jasmonic acid and ethylene signals in plant defense. Plant Physiol. 147, 1347–1357. doi: 10.1104/pp.108.117523
Rekah, Y., Shtienberg, D., and Katan, J. (2000). Disease development following infection of tomato and basil foliage by airborne conidia of the Soilborne pathogens Fusarium oxysporum f. sp. radicis-lycopersici and F. oxysporum f. sp. basilici. Phytopathology 90, 1322–1329. doi: 10.1094/PHYTO.2000.90.12.1322
Riyazuddin, R., Verma, R., Singh, K., Nisha, N., Keisham, M., Bhati, K. K., et al. (2020). Ethylene: A master regulator of salinity stress tolerance in plants. Biomol. Ther. 10:959. doi: 10.3390/biom10060959
Robatzek, S., Chinchilla, D., and Boller, T. (2006). Ligand-induced endocytosis of the pattern recognition receptor FLS2 in Arabidopsis. Genes Dev. 20, 537–542. doi: 10.1101/gad.366506
Robinson, M. D., McCarthy, D. J., and Smyth, G. K. (2010). edgeR: a bioconductor package for differential expression analysis of digital gene expression data. Bioinformatics 26, 139–140. doi: 10.1093/bioinformatics/btp616
Schmittgen, T. D., and Livak, K. J. (2008). Analyzing real-time PCR data by the comparative C(T) method. Nat. Protoc. 3, 1101–1108. doi: 10.1038/nprot.2008.73
Schwessinger, B., Bahar, O., Thomas, N., Holton, N., Nekrasov, V., Ruan, D., et al. (2015). Transgenic expression of the dicotyledonous pattern recognition receptor EFR in rice leads to ligand-dependent activation of defense responses. PLoS Pathog. 11:e1004809. doi: 10.1371/journal.ppat.1004809
Seo, D. H., and Yoon, G. M. (2019). Light-induced stabilization of ACS contributes to hypocotyl elongation during the dark-to-light transition in Arabidopsis seedlings. Plant J. 98, 898–911. doi: 10.1111/tpj.14289
Sepulveda-Chavera, G., Huanca, W., Salvatierra-Martinez, R., and Latorre, B. A. (2014). First report of Fusarium oxysporum f. sp. lycopersici race 3 and F. oxysporum f. sp. radicis-lycopersici in tomatoes in the Azapa Valley of Chile. Plant Dis. 98:1432. doi: 10.1094/PDIS-03-14-0303-PDN
Shi, H., Liu, R., Xue, C., Shen, X., Wei, N., Deng, X. W., et al. (2016). Seedlings transduce the depth and mechanical pressure of covering soil using COP1 and ethylene to regulate EBF1/EBF2 for soil emergence. Curr. Biol. 26, 139–149. doi: 10.1016/j.cub.2015.11.053
Singh, P., Kuo, Y. C., Mishra, S., Tsai, C. H., Chien, C. C., Chen, C. W., et al. (2012). The lectin receptor kinase-VI.2 is required for priming and positively regulates Arabidopsis pattern-triggered immunity. Plant Cell 24, 1256–1270. doi: 10.1105/tpc.112.095778
Singh, P., and Zimmerli, L. (2013). Lectin receptor kinases in plant innate immunity. Front. Plant Sci. 4:124. doi: 10.3389/fpls.2013.00124
Spoel, S. H., and Dong, X. (2008). Making sense of hormone crosstalk during plant immune responses. Cell Host Microbe 3, 348–351. doi: 10.1016/j.chom.2008.05.009
Srinivas, C., Nirmala Devi, D., Narasimha Murthy, K., Mohan, C. D., Lakshmeesha, T. R., Singh, B., et al. (2019). Fusarium oxysporum f. sp. lycopersici causal agent of vascular wilt disease of tomato: biology to diversity- A review. Saudi J. Biol. Sci. 26, 1315–1324. doi: 10.1016/j.sjbs.2019.06.002
Sun, Y., Qiao, Z., Muchero, W., and Chen, J. G. (2020). Lectin receptor-Like kinases: The sensor and mediator at the plant cell surface. Front. Plant Sci. 11:596301. doi: 10.3389/fpls.2020.596301
Tang, D., Wang, G., and Zhou, J. M. (2017). Receptor kinases in plant-pathogen interactions: more Than pattern recognition. Plant Cell 29, 618–637. doi: 10.1105/tpc.16.00891
Tian, T., Liu, Y., Yan, H., You, Q., Yi, X., Du, Z., et al. (2017). agriGO v2.0: a GO analysis toolkit for the agricultural community, 2017 update. Nucleic Acids Res. 45, W122–W129. doi: 10.1093/nar/gkx382
Vaid, N., Macovei, A., and Tuteja, N. (2013). Knights in action: lectin receptor-like kinases in plant development and stress responses. Mol. Plant 6, 1405–1418. doi: 10.1093/mp/sst033
Validov, S. Z., Kamilova, F. D., and Lugtenberg, B. J. (2011). Monitoring of pathogenic and non-pathogenic Fusarium oxysporum strains during tomato plant infection. Microb. Biotechnol. 4, 82–88. doi: 10.1111/j.1751-7915.2010.00214.x
Wang, Y., and Bouwmeester, K. (2017). L-type lectin receptor kinases: new forces in plant immunity. PLoS Pathog. 13:e1006433. doi: 10.1371/journal.ppat.1006433
Wang, Y., Cordewener, J. H., America, A. H., Shan, W., Bouwmeester, K., and Govers, F. (2015). Arabidopsis Lectin receptor kinases LecRK-IX.1 and LecRK-IX.2 are functional analogs in regulating Phytophthora resistance and plant cell death. Mol. Plant Microbe Interact. 28, 1032–1048. doi: 10.1094/MPMI-02-15-0025-R
Wang, F., Cui, X., Sun, Y., and Dong, C. H. (2013). Ethylene signaling and regulation in plant growth and stress responses. Plant Cell Rep. 32, 1099–1109. doi: 10.1007/s00299-013-1421-6
Wang, L., Wilkins, K. A., and Davies, J. M. (2018). Arabidopsis DORN1 extracellular ATP receptor; activation of plasma membrane K(+) -and Ca(2+) -permeable conductances. New Phytol. 218, 1301–1304. doi: 10.1111/nph.15111
Yang, H., Shen, F., Wang, H., Zhao, T., Zhang, H., Jiang, J., et al. (2020). Functional analysis of the SlERF01 gene in disease resistance to S. lycopersici. BMC Plant Biol. 20:376. doi: 10.1186/s12870-020-02588-w
Yang, H., Sun, Y., Wang, H., Zhao, T., Xu, X., Jiang, J., et al. (2021). Genome-wide identification and functional analysis of the ERF2 gene family in response to disease resistance against Stemphylium lycopersici in tomato. BMC Plant Biol. 21:72. doi: 10.1186/s12870-021-02848-3
Zhao, J. L., Zhang, L. Q., Liu, N., Xu, S. L., Yue, Z. L., Zhang, L. L., et al. (2019). Mutual regulation of receptor-Like kinase SIT1 and B'kappa-PP2A shapes the early response of Rice to salt stress. Plant Cell 31, 2131–2151. doi: 10.1105/tpc.18.00706
Keywords: Fusarium crown and root rot, Fusarium oxysporum f. sp. radicis-lycopersici, tomato, lectin receptor-like kinase, disease resistance
Citation: Yue Z-L, Tian Z-J, Zhang J-W, Zhang S-W, Li Y-D and Wu Z-M (2022) Overexpression of Lectin Receptor-Like Kinase 1 in Tomato Confers Resistance to Fusarium oxysporum f. sp. Radicis-Lycopersici. Front. Plant Sci. 13:836269. doi: 10.3389/fpls.2022.836269
Edited by:
Huiquan Liu, Northwest A&F University, ChinaCopyright © 2022 Yue, Tian, Zhang, Zhang, Li and Wu. This is an open-access article distributed under the terms of the Creative Commons Attribution License (CC BY). The use, distribution or reproduction in other forums is permitted, provided the original author(s) and the copyright owner(s) are credited and that the original publication in this journal is cited, in accordance with accepted academic practice. No use, distribution or reproduction is permitted which does not comply with these terms.
*Correspondence: Zhi-Ming Wu, zhiming71@126.com