- 1German Aerospace Center (DLR), Institute of Aerospace Medicine, Cologne, Germany
- 2Centre for Human Drug Research (CHDR), Leiden, Netherlands
- 3Leiden Academic Centre for Drug Research, Leiden, Netherlands
- 4Medical Faculty, University of Cologne, Cologne, Germany
- 5Leiden University Medical Center, Leiden, Netherlands
- 6Central Committee on Research Involving Human Subjects (CCMO), The Hague, Netherlands
Acute exposure to hypoxia can lead to cognitive impairment. Therefore, hypoxia may become a safety concern for occupational or recreational settings at altitude. Cognitive tests are used as a tool to assess the degree to which hypoxia affects cognitive performance. However, so many different cognitive tests are used that comparing studies is challenging. This structured literature evaluation provides an overview of the different cognitive tests used to assess the effects of acute hypoxia on cognitive performance in healthy volunteers. Less frequently used similar cognitive tests were clustered and classified into domains. Subsequently, the different cognitive test clusters were compared for sensitivity to different levels of oxygen saturation. A total of 38 articles complied with the selection criteria, covering 86 different cognitive tests. The tests and clusters showed that the most consistent effects of acute hypoxia were found with the Stroop test (where 42% of studies demonstrated significant abnormalities). The most sensitive clusters were auditory/verbal memory: delayed recognition (83%); evoked potentials (60%); visual/spatial delayed recognition (50%); and sustained attention (47%). Attention tasks were not particularly sensitive to acute hypoxia (impairments in 0%–47% of studies). A significant hypoxia level-response relationship was found for the Stroop test (p = 0.001), as well as three clusters in the executive domain: inhibition (p = 0.034), reasoning/association (p = 0.019), and working memory (p = 0.024). This relationship shows a higher test sensitivity at more severe levels of hypoxia, predominantly below 80% saturation. No significant influence of barometric pressure could be identified in the limited number of studies where this was varied. This review suggests that complex and executive functions are particularly sensitive to hypoxia. Moreover, this literature evaluation provides the first step towards standardization of cognitive testing, which is crucial for a better understanding of the effects of acute hypoxia on cognition.
Introduction
Hypoxemia is defined as low oxygen levels in the blood (Bhutta et al., 2022). It can occur in obstructive sleep apnea (OSA), in various pulmonary diseases such as COPD and COVID-19, in neuromuscular disorders like Guillain-Barré syndrome, Pompe’s disease or myasthenia gravis, and in central nervous system conditions like Alzheimer’s (Sivak et al., 1999; Orešič et al., 2011; Branson and Faarc, 2018; Chokesuwattanaskul et al., 2021; Rahman et al., 2021). Hypoxemia may also develop at high altitudes, where less oxygen is available due to the low atmospheric pressure (Mulroney et al., 2015).
Hypoxia research is divided into three arbitrary exposure designs: chronic, intermittent and acute hypoxia. Research on chronic hypoxia has shown the ability of the human body to adapt to prolonged exposure to low oxygen levels or pressures (Gilbert-Kawai et al., 2014). Hence, individuals residing at high altitudes or those who have had recent altitude exposure are frequently ineligible to participate in clinical trials investigating the impacts of hypoxia. In recent years, intermittent hypoxia (IH) has been studied, attempting to use the human body’s adaptability to IH as a therapeutic effect for various diseases (Navarrete-Opazo and Mitchell, 2014). This review will focus only on acute non-intermittent hypoxia.
Studying acute hypoxia is of particular importance in assessing the safety of occupational or recreational settings that involve acute exposure to high altitude, e.g., pilots, military personnel, mountaineers, etc. Hypoxia facilities can induce either hypobaric hypoxia by reducing barometric pressure or normobaric hypoxia by diluting oxygen fraction with nitrogen (DeHart and Davis, 2002). There has long been debate as to whether there is a difference in physiological response to isolated changes in barometric pressure, with normal levels of oxygen and carbon dioxide (Naughton et al., 2012). More recent studies suggest that there is indeed a significant impact of pressure alone (Savourey et al., 2003; Savourey et al., 2007; Millet et al., 2012; Coppel et al., 2015; McMorris et al., 2017).
One of the first symptoms of hypoxia exposure is impaired cognitive functioning. The level of oxygen saturation is considered to be the key predictor in determining the extend of cognitive impairment caused by hypoxia (McMorris et al., 2017). During high-altitude activities, cognitive performance plays a critical role in tasks that require attention, decision-making and memorization of protocols, as it can mitigate the risk of potential disasters. Over the years, numerous cognitive tests have been developed to evaluate cognitive performance. However, due to the wide range of cognitive tests used in studies examining cognitive performance during acute hypoxia exposure, it is challenging to compare results across different studies.
A useful test for the cognitive effects of acute hypoxia can be characterized as any response measure that shows a clear, consistent response to meaningful hypoxia levels, across studies from a sufficient number of different research groups. A hypoxia level-response relationship and a plausible relationship between the function test and the physiological response to hypoxia are indications that the test reflects physiological activity.
Previously, similar criteria were used to evaluate the usefulness of central nervous system (CNS)-tests for the effects of antipsychotic drugs (De Visser et al., 2001), benzodiazepines (De Visser et al., 2003), selective serotonin re-uptake inhibitors (SSRIs) (Dumont et al., 2005), 3,4-methyleendioxymethamfetamine (MDMA) (Dumont and Verkes, 2006), D9-tetrahydrocannabinol (THC) (Zuurman et al., 2009) and alcohol (Zoethout et al., 2011) in healthy subjects. In general, these systematic reviews showed that only a small number of tests actually display proper characteristics for a meaningful effect biomarker, and that these tests differ between drug classes (Van Gerven et al., 2019).
This review aims to provide an overview of the wide range of cognitive tests used to assess cognitive performance during acute hypoxia in healthy volunteers, to evaluate the differences in test sensitivity to different levels of hypoxia, to explore the role of barometric pressure, and to ultimately identify which tests best meets the criteria to serve as a meaningful test of the functional effects of hypoxia.
Methods
Structured literature evaluation
A literature search was performed up to 9 of November 2022 using Pubmed, Embase, Web of Science, Cochrane Library, Emcare, PsycINFO and Academic Search Premier. Key words used in the searches were combinations of “hypoxia”, “cognitive function”, “cognition”, “neuropsychological tests”, “biomarkers”, “cognitive dysfunction” and “mental deterioration”. The searches were limited to healthy adults and articles in English. The resulting studies were subject to several selection criteria. Reviews, studies in experimental animals or patients, ≤5 healthy subjects and confounding factors like exercise, acclimatization, sleep, brain stimulation and breathing exercise were excluded. The review was restricted to the effects of hypoxia exposure of <8 h and the presense of a normoxia condition, served as baseline or control group. Studies that contained a confounding factor but included both a normoxia and hypoxia group in the study design were eligible for analysis. Controlling the end-tidal CO2 can impact oxygen saturation, making isocapnia a potential confounding factor. Nevertheless, studies with isocapnic hypoxia were included when the oxygen saturation was documented.
Mapping of results
Most studies compared hypoxia and normoxia groups, using one or more cognitive tests. In addition, some studies examined different exposure durations and/or severities. The normoxia condition represented either a placebo-controlled group or served as a baseline measurement of the group that would later be exposed to the hypoxia condition. Each primary test parameter for each cognitive test used in a study was considered a unique data point. Thus, a single study usually accounted for multiple data points. In some cases, there was no significant difference between groups for the primary effect parameter, while there was a significant difference for a secondary effect parameter. However, including these secondary effects would result in an overestimation of the cluster sensitivity. Hence, only primary effect parameters were considered.
All data points were collected in a Microsoft Excel® database and recorded as a significant impairment or decrease (−), as no significant effect (=) or as a significant improvement or increase (+) of the test parameter during the hypoxia condition compared to the normoxia condition. The absolute values of the test effects or the levels of significant impairment were not included in the analysis. Consequently, this approach addresses the likelihood that a study with a hypoxia condition produces a statistically significant effect on a given cognitive test.
Study characteristics that were recorded in the database were: effect [+(improvement/increase)/= (no change)/- (deterioration/reduction)], test domain, test cluster, barometric pressure (hypobaric or normobaric), hypoxia intensity (“dose”, displayed as FiO2/altitude), dose normalization (four levels), oxygen saturation, exposure duration (including test duration), test duration, oxygen administration (mask/chamber/altitude), blinding (open/single-blind/double blind), randomization (randomized/non-randomized), control (baseline/control group), design (parallel/crossover), number of subjects, sex and age. Although blood CO2 levels are relevant for hypoxia research, they were not included in the database, as most studies did not document this information.
Analysis of individual tests and test clusters
Cognitive tests with at least five data points (i.e., five different results from different experiments) were included in the analysis of the individual test results. Cognitive tests that were alternate versions of the original tests, or which tested the same cognitive functionality, were clustered to increase the number of evaluable data points. The clusters were divided into six domains for a better overview. The allocation of domains and clustering of cognitive tests was based on “A Compendium of Neurophysiological tests: Administration, Norms, and Commentary” (Strauss et al., 2006), adapted according to comparable systemic biomarker reviews that we performed previously for drug classes (De Visser et al., 2001; De Visser et al., 2003; Dumont et al., 2005; Dumont and Verkes, 2006; Zuurman et al., 2009; Zoethout et al., 2011). The sensitivity of a test was expressed as the percentage of statistically significant outcomes, relative to the total number of times that the test was used in the literature. The percentages of tests that showed cognitive impairment were calculated for each cluster.
Level-response relationships
While analysis of individual tests and test clusters provides an overview of the sensitivity of different neuropsychological functions to hypoxia, this does not include information on the relationship between the level of hypoxia and the effect on cognitive test performance. This relationship was studied by first applying a four-level dose normalization to oxygen saturation. These four levels represent the severity of hypoxia as described by Castor and Borgvall (Castor and Borgvall, 2015) and based on Woodrow and Webb (Woodrow and Webb, 2011). The oxygen saturation levels are ≥90%, 89%–80%, 79%–70% and ≤69%. For studies that did not provide oxygen saturation, the fraction of inspired oxygen or altitude was used to estimate oxygen saturation (Woodrow and Webb, 2011; Castor and Borgvall, 2015). Only tests or clusters containing at least 10 data points were included in this analysis. Relationships were tested with simple linear regression analysis. Finally, a comparison was made between all similar tests/clusters that were measured both in normobaric hypoxia and hypobaric hypoxia. Similarly, these results were divided over the four levels of dose normalization.
Results
Study design
The literature search yielded 38 different studies on acute hypoxia that met all criteria, published between 1993 and 31 August 2022. The number of participants ranged from 6 to 50 and ages from 22 to 41 years (range of mean ages between different studies). In 40% of studies only healthy men were included, and 5% of studies included only women. Fifty-five percent of studies included men and women. A summary of the study characteristics is reported in Table 1.
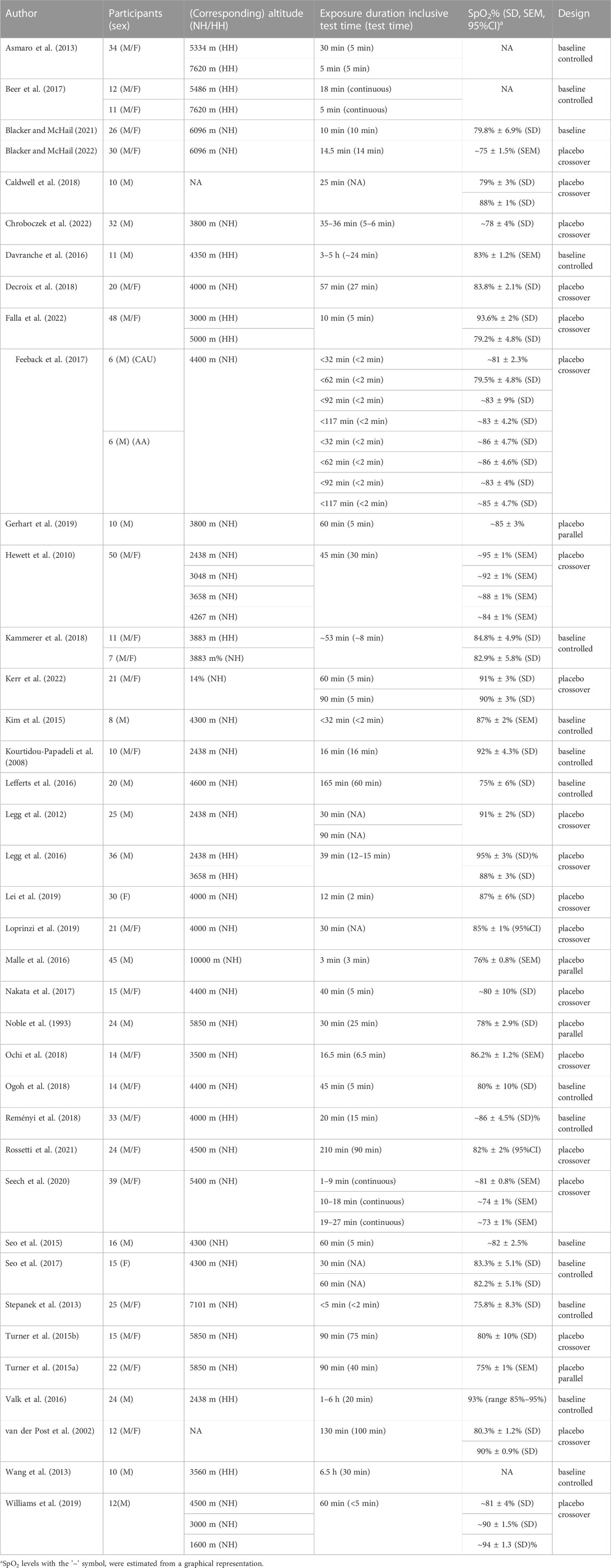
TABLE 1. Summary of general study characteristics. M = male, F = female, M/F = male and female, NH = normobaric hypoxia, HH = hypobaric hypoxia, SpO2 = oxygen saturation, CAU = Caucasian individuals, AA = African-American.
Forty-seven percent of the reviewed studies had an open design; 37% were single-blinded; 5% were double blinded and for 11% the blinding was unknown. In addition, a majority of the studies had a crossover design (89%) and 11% had a parallel design. Normoxia served in 61% of studies as a control group and in 39% as a baseline measurement.
Atmospheric conditioning of hypoxia
Eighteen studies were performed in a hypoxic chamber (Asmaro et al., 2013; Kim et al., 2015; Seo et al., 2015; Lefferts et al., 2016; Legg et al., 2016; Valk et al., 2016; Beer et al., 2017; Feeback et al., 2017; Seo et al., 2017; Decroix et al., 2018; Reményi et al., 2018; Gerhart et al., 2019; Williams et al., 2019; Seech et al., 2020; Blacker and McHail, 2021; Blacker and McHail, 2022; Chroboczek et al., 2022; Falla et al., 2022; Kerr et al., 2022), 16 with a breathing mask that induced hypoxia (Turner et al., 2015a; Noble et al., 1993; Ochi et al., 2018; Malle et al., 2016; Lei et al., 2019; Caldwell et al., 2018; Hewett et al., 2010; Legg et al., 2012; Ogoh et al., 2018; Stepanek et al., 2013; Nakata et al., 2017; Loprinzi et al., 2019; Kourtidou-Papadeli et al., 2008; Turner et al., 2015b), two studies were performed at altitude (Wang et al., 2013; Davranche et al., 2016), one both at altitude and in a chamber (Kammerer et al., 2018), and one both in a chamber and with a breathing mask (Rossetti et al., 2021). The exposure duration ranged from 10 min to 6.5 h with 92% of studies using durations of <3 h. The data points obtained were divided into exposure to normobaric hypoxia (133; 67%) and hypobaric hypoxia (66; 33%). The mean altitude and mean oxygen saturation for normobaric and hypobaric hypoxia were 4500 m and 84.4%, and 3300 m and 86.9%, respectively.Only two s tudies were performed with isocapnic hypoxia, so this condition was not analysed separately (Caldwell et al., 2018).
Tests, clusters and domains
A total of 86 different tests were used. Only six tests (including comparable variants) (7%) were used five times or more and consequently produced five or more data points for the analysis of hypoxia on individual tests. These most frequently used tests were the Trail making test A (14), Trail making test B (14), Stroop test (12), Finger tapping (5), Go/no Go (5) and Digit symbol substitution test (DSST) (5), shown in Table 2. Overall, the Stroop test and Finger tapping test showed the highest sensitivity to acute hypoxia (which produced significant effects in 42% and 40% of experiments, resp.), followed by Trail making B (29%), Trail making A (21%) and the Go/no Go task (20%). The DSST did not show any significant cognitive impairment.
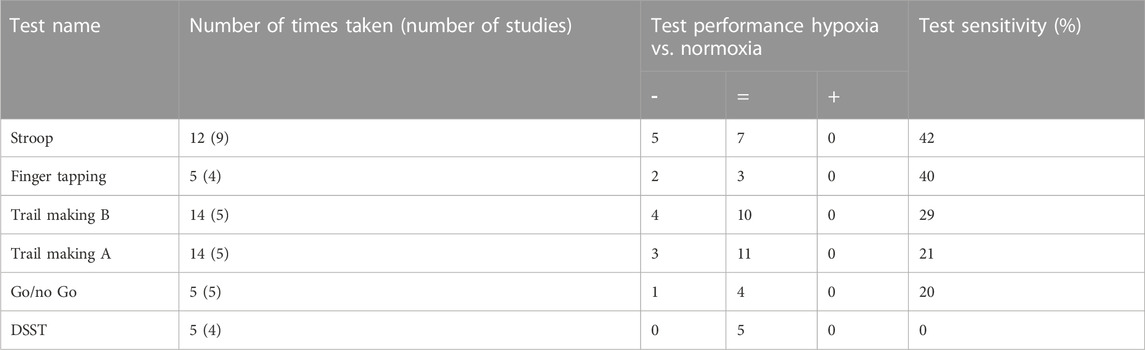
TABLE 2. A summary of the most frequently used tests (≥5 times) for measuring the effects of acute hypoxia on cognitive performance. The table shows the number of times tests were, and in brackets, the number of studies from which these data points were collected. The test performance is indicated by significant impairment or decrease (−), no significant effect (=) or significant improvement or increase (+). Test sensitivity was calculated as the number of times a test showed a significant impairment out of the total number of times the test was taken.
A cluster analysis was performed because of the small number of tests that were performed frequently enough to allow an individual test analysis. Tests used only incidentally were clustered together with other tests that require the same cognitive functionality. This allowed us to increase the sample size and thus perform a more reliable analysis on how hypoxia affects different cognitive functions. The tests were grouped into 32 functional clusters, covering six main neurocognitive domains (Table 3). Fourteen of the 32 clusters contained at least five data points and were used for further analysis of overall hypoxia effects (Table 4). Both delayed recognition clusters auditory/verbal memory (83%) and visual/spatial memory (50%), and the evoked potential cluster (60%) had the highest sensitivity to acute hypoxia (irrespective of level). In contrast, immediate recognition tests showed virtually no significant effects (auditory/verbal memory tests (20%); visual/spatial memory tests (0%)). In addition to delayed recognition and evoked potential, the clusters of sustained attention (47%), motor control (40%) and divided attention (36%) showed a higher sensitivity for hypoxia. The clusters inhibition (26%), shifting (23%) and reaction time (19%) rarely yielded significant results, whereas in the literature, tests from these clusters were used most frequently (inhibition 23/182, shifting 31/182, and reaction time 21/182 tests).
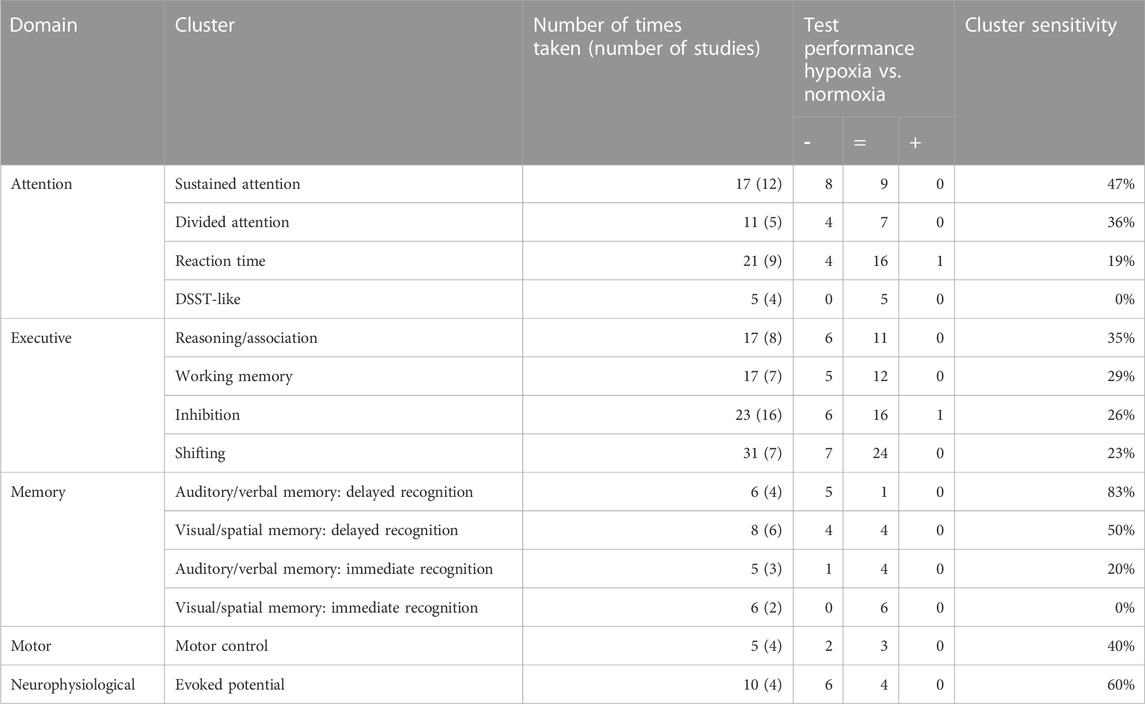
TABLE 4. An overview of clusters in which at least five times a test was taken. The table shows the number of times tests were taken in each cluster and, in brackets, the number of studies from which these tests were collected. The test performance is indicated by significant impairment or decrease (−), no significant effect (=) or significant improvement or increase (+). Cluster sensitivity was calculated as the number of times a test showed a significant impairment out of the total number of times the test was taken.
Level-response relationship
Individual tests and clusters with ≥10 datapoints were inspected for potential level-response relationships (Table 5; Table 6). (Near) significant associations were found for the Stroop test, Trail making A, Trail making B and 25% of clusters. The Stroop test (p = 0.001, R2 = 1.00) and clusters within the executive domain showed a significant relationship (reasoning/association p = 0.019, R2 = 0.96; working memory p = 0.024, R2 = 0.95; and inhibition p = 0.035, R2 = 0.93) or trend (shifting p = 0.057, R2 = 0.89) between the four defined levels of oxygen saturation and test sensitivity, with lower saturations more often leading to a higher test sensitivity (Figure 1). The Trail making A, Trail making B and clusters in the attention and neurophysiological domain did not show a level-response relationship between oxygen saturation and test sensitivity.
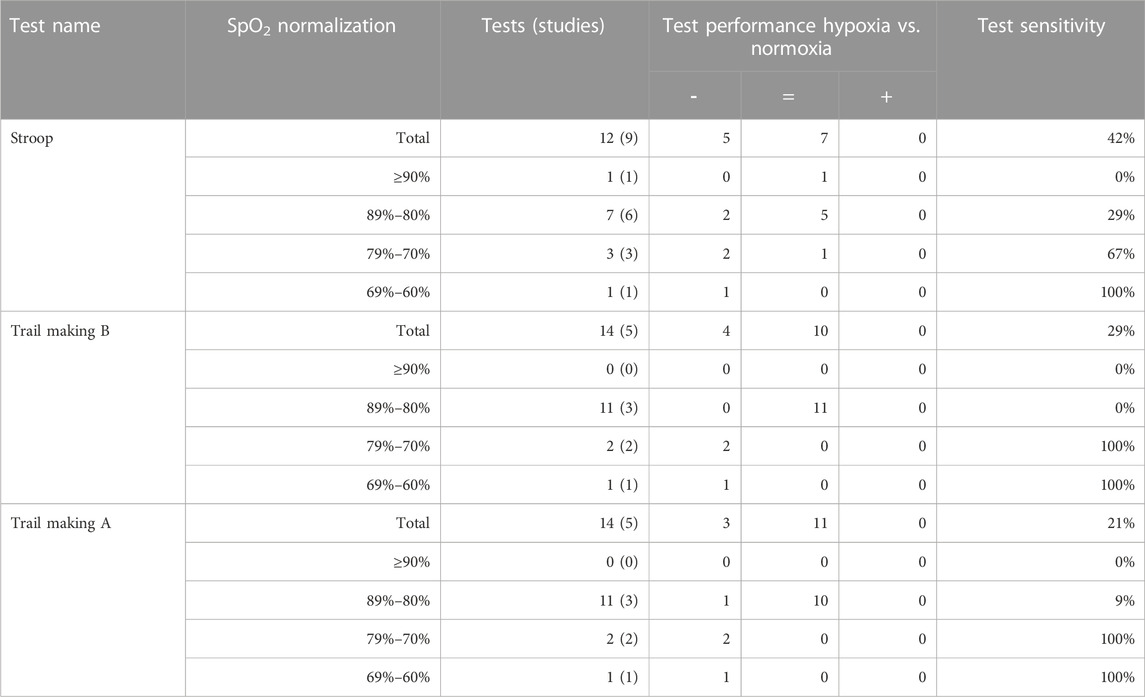
TABLE 5. The level-response relationship between oxygen saturation and sensitivity for tests used ≥10 times. Oxygen saturation is normalized into four groups: ≥90%, 89%–80%, 79%–70% and 69%–60%. The table shows the number of times tests were taken and, in brackets, the number of studies from which these tests were collected. The test performance is indicated by significant impairment or decrease (−), no significant effect (=) or significant improvement or increase (+). Test sensitivity was calculated as the number of times a test showed a significant impairment out of the total number of times the test was taken.
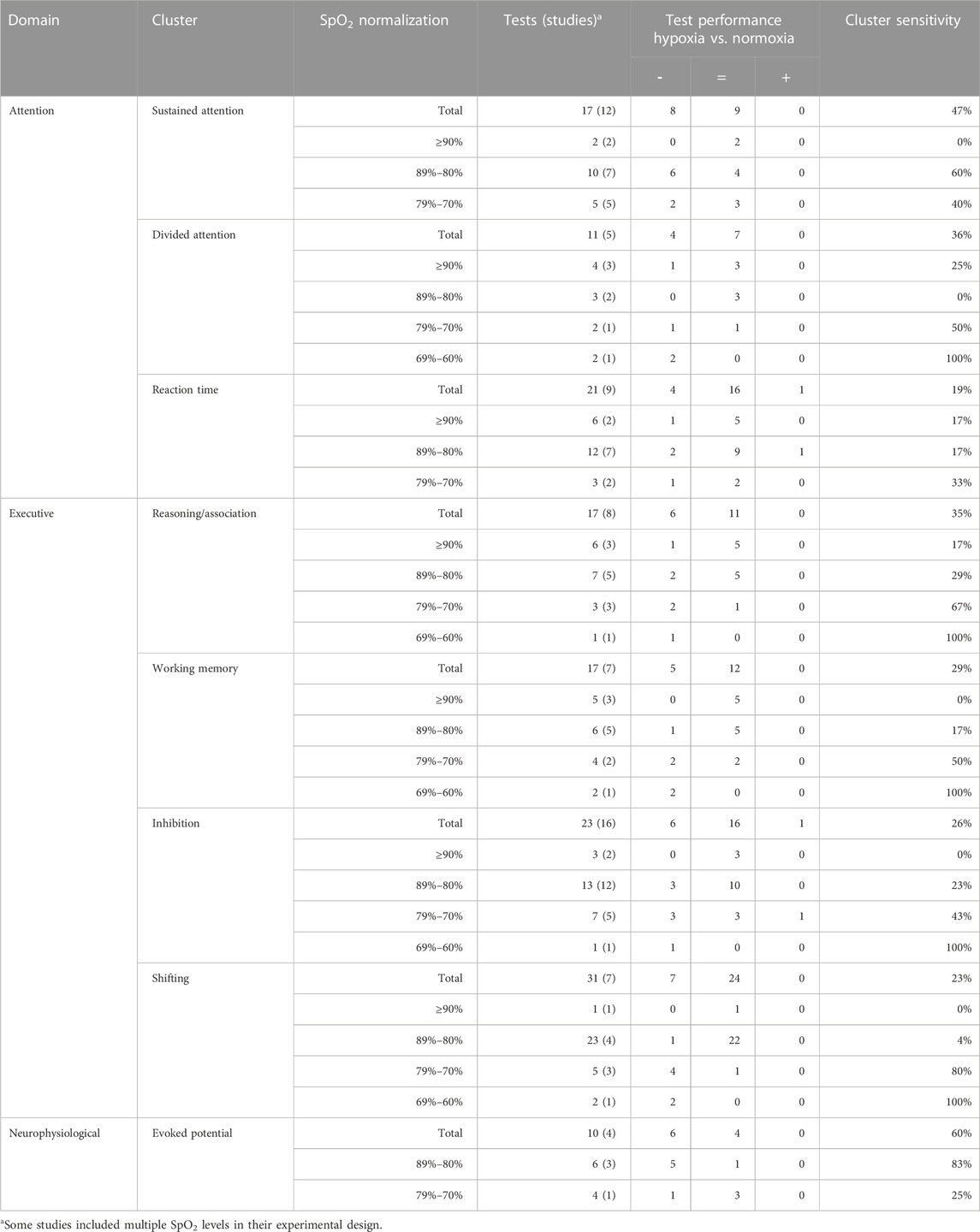
TABLE 6. The level-response relationship between oxygen saturation and sensitivity of clusters tested ≥10 times. Oxygen saturation is normalized into four groups: ≥90%, 89%–80%, 79%–70% and 69%–60%. The table shows the number of times tests were taken in each cluster and, in brackets, the number of studies from which these tests were collected. The test performance is indicated by significant impairment or decrease (−), no significant effect (=) or significant improvement or increase (+). Cluster sensitivity was calculated as the number of times a test showed a significant impairment out of the total number of times the test was taken.
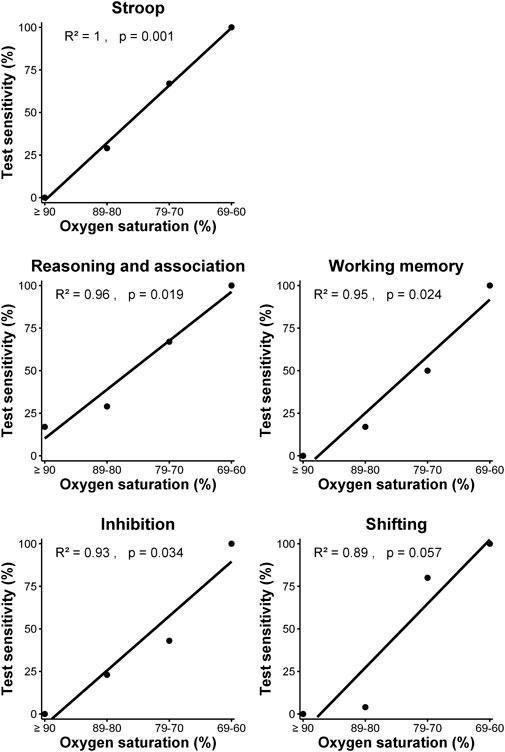
FIGURE 1. Relationship between test sensitivity and oxygen saturation of Stroop test and clusters within the executive domain using simple linear regression analysis.
Since sustained- and divided attention were sensitive to hypoxia, while also playing a role in executive functioning and memory, the contribution of attention deficits to reduced cognitive functioning was explored in more detail. At an oxygen saturation level of ≥90%, tests measuring sustained- and divided attention demonstrated sensitivity ranging from 0% to 25%, respectively, while the executive domain showed sensitivity between 0% and 17%. At an oxygen saturation level of 80%–90%, sustained attention showed a test sensitivity of 60%, whereas divided attention did not show any sensitivity, in contrast to the executive domain where sensitivity ranged from 4% to 29%. At an oxygen saturation level of 70%–80%, the sensitivity of sustained attention was 40%, while divided attention showed a 50% sensitivity, compared to the executive domain which ranged from 43% to 80%. Although sustained attention was not measured between an oxygen saturation level of 69%–60%, divided attention and all clusters within the executive domain demonstrated 100% sensitivity. There appears to be a cautious relationship between hypoxia-related declines in divided attention and executive performance (p = 0.059).
The effect of barometric pressure
Table 7 presents the effects of normobaric and hypobaric hypoxia on overall cognitive performance for the four levels of oxygen saturation. At oxygen saturation above 80%, almost no differences in sensitivity were observed between normobaric and hypobaric hypoxia (4% and 5% at SpO2 ≥ 90% and 27% and 27% at SpO2 89%–80%, respectively). At an oxygen saturation between 79% and 70%, hypobaric hypoxia affected cognitive performance more often (67%) than normobaric hypoxia (55%), although not significantly. At an oxygen saturation between 69% and 60%, no data were reported for normobaric hypoxia.
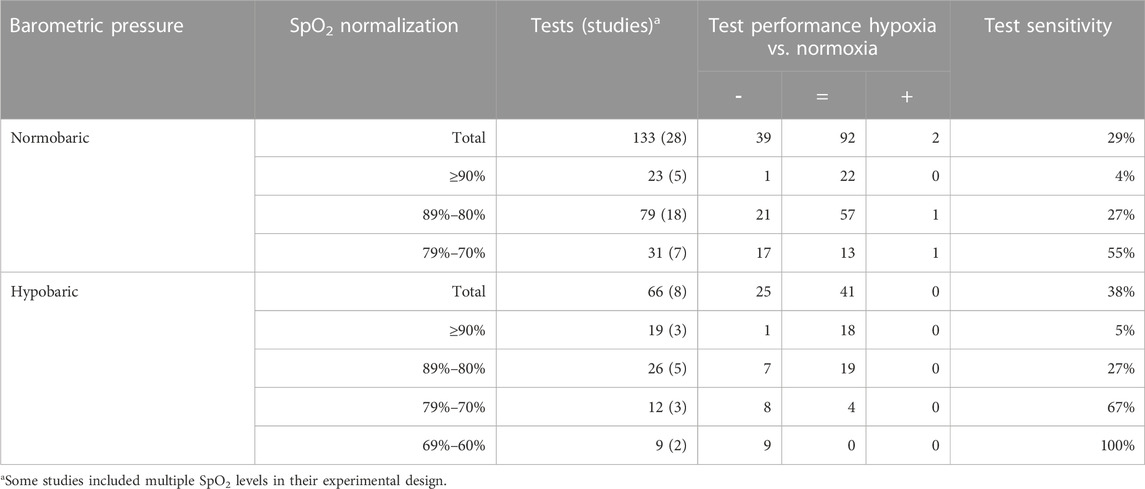
TABLE 7. Effects of barometric pressure on test sensitivity in all clusters. The table shows the number of times tests were taken and, in brackets, the number of studies from whish these tests were collected. Oxygen saturation is normalized into four groups: ≥90%, 89%–80%, 79%–70%, and 69%–60%. The number of times a significant effect was found is indicated by impairment or decrease (−), no significant effect (=) or improvement or increase (+).
Given that a level-response relationship between oxygen saturation and test sensitivity occurred solely in clusters belonging to the executive domain, the comparison between normobaric hypoxia and hypobaric hypoxia was narrowed to this particular domain (Table 8). Oxygen saturation levels of ≥90% and between 89% and 80% did not yield significantly different effects on cognitive performance between normobaric hypoxia and hypobaric hypoxia. The largest difference was found at an oxygen saturation between 79% and 70% (50% and 86%, respectively), but this difference was not statistically significant.
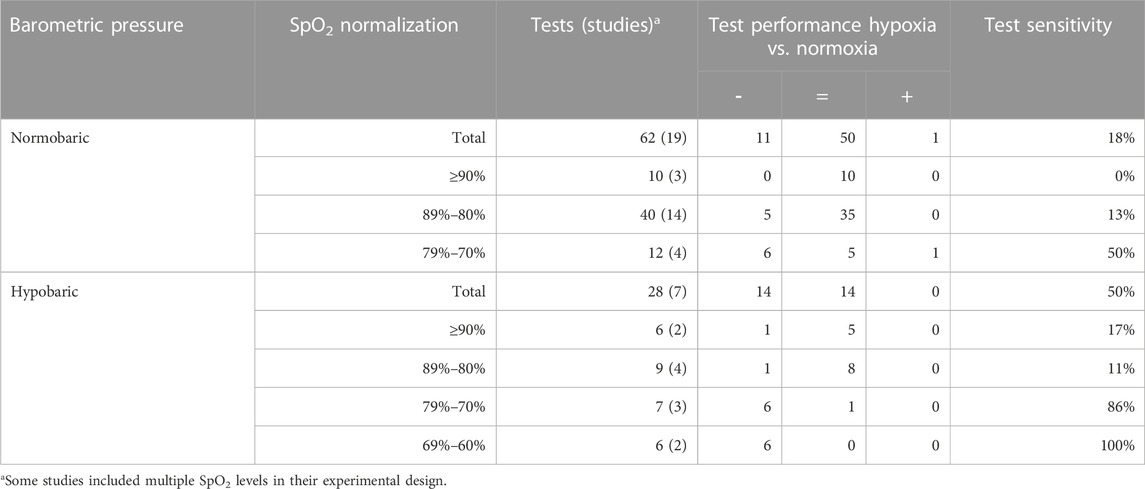
TABLE 8. Effects of normobaric and hypobaric hypoxia on test sensitivity in the executive domain. The table shows the number of times tests were taken and, in brackets, the number of studies from whish these tests were collected. Oxygen saturation is normalized into four groups: ≥90%, 89%–80%, 79%–70% and 69%–60%. The number of times a significant effect was found is indicated by impairment or decrease (−), no significant effect (=) or improvement or increase (+).
Discussion
A large number of tests were used in the literature to measure the acute CNS effects of hypoxia in healthy adults. As with similar reviews for drug classes (De Visser et al., 2001; De Visser et al., 2003; Dumont et al., 2005; Dumont and Verkes, 2006; Zuurman et al., 2009; Zoethout et al., 2011), there were more tests than studies: 86 in 38 studies. Only six individual tests, including their variants, (7%) were used five times or more and consequently provided sufficient data points for our individual test analysis. This wide variety of tests limits cross-study comparison. This limitation not only hampers the current review, but a virtually unrestricted use of a large number of distinct neurocognitive tests also thwarts the field of hypoxia research as a whole. Although it may be useful to study different distinct areas of cognitive performance, interpretations and insights would strongly benefit from standardization within cognitive domains and test clusters. Moreover, standardization of exposure protocols would also improve comparisons between studies. Given this suboptimal context, we grouped tests into test clusters and functional domains, to obtain more insights into potentially meaningful hypoxia CNS-effects and protocols. Prior reviews demonstrated that this approach is useful for assessing function tests or biomarkers for drug effects (De Visser et al., 2001; De Visser et al., 2003; Dumont et al., 2005; Dumont and Verkes, 2006; Zuurman et al., 2009; Zoethout et al., 2011). While this methodology inevitably results in the loss of some information, it ultimately yields a structured and comprehensive overview of the chance of measuring significant CNS effects of acute hypoxia in studies with healthy adults.
Attention is an important functional domain that underlies most of the other CNS-performance tasks. Hypoxia has an effect on attention, particularly, at levels below 80%, which caused significant abnormalities of divided or sustained attention in 36% and 47% of studies, respectively. This effect of hypoxia on more demanding (particularly, divided) attention tests may also have influenced other complex or multifunctional tasks, particularly those in the executive domain. These clusters represent aspects of cognition with a direct effect on safety for military personnel and pilots. Especially, impairments in sustained attention, which were already frequently observed at an oxygen saturation between 89% and 80% (60%), could lead to serious safety risks when military personnel have to stand guard at altitude (2438 m–4572 m) on hostile territory. Therefore, this aspect of cognition should be carefully considered during training and preparation for a mission or flight. The effect of hypoxia on reaction time seems limited, even under more severe hypoxic conditions (SpO2 < 80%) a low-test sensitivity is observed (33%). However, it should be noted that in some cognitive tests, secondary effects on reaction time were observed, which were not included in the analysis to prevent bias.
In the executive domain, the different test clusters rarely showed an effect of mild hypoxia (SpO2 ≥ 80%). However, when oxygen saturation fell below 80%, the test sensitivity increased. The level-response relationship between oxygen saturation and test sensitivity could indicate that cognitive testing of the executive domain gives the most accurate representation of the physiological response of hypoxia. Although the mechanism causing the impairment of executive function by hypoxia is not clearly understood, this could be related to impaired concentration or (divided) attention, which are required during performance of any executive task. It is also possible that hypoxia-induced executive impairment is related more directly to decreased neural activity of the prefrontal cortex (Bishop et al., 2010; Shaked et al., 2018; Rosen et al., 2019). A review by Beebe and Gozal linked executive impairment of OSA patients to prefrontal cortex dysfunction (Beebe and Gozal, 2002). To our knowledge, however, only one study has examined the role of the prefrontal cortex in reducing executive function after hypoxia exposure in healthy individuals (Ochi et al., 2018). Although this study by Ochi et al. found a statistically significant relationship between impaired Stroop test performance and lower dorsolateral prefrontal cortex activation after a combined intervention of exercise and hypoxia, this relationship was not significant in hypoxia at rest. However, the oxygen saturation was also lower in the combined exercise and hypoxia intervention than in the hypoxia at rest intervention, which is expected as, exercise acutely decreases the oxygen saturation both combined with and independent of hypoxia (Woorons and Richalet, 2021). Therefore, a similar effect on the lower dorsolateral prefrontal cortex might be found for more severe hypoxia. Further research into the interaction between hypoxia, prefrontal cortex activation and executive function could help to get a better understanding of the physiological response to hypoxia.
Within the inhibition cluster, the Stroop test more often showed impairments (42%) than when other inhibition tests were used (mean of 26%). This may reflect the dependence of the performance of the Stroop test on accurate colour discrimination. Although this systematic review did not specifically search for visual function studies, the retina has been reported to be highly sensitive to hypoxia (Selvam et al., 2018). Based on this observation we recommend choosing the Stroop test over other inhibition tasks.
In the memory domain, delayed recognition was the most sensitive to hypoxia. Delayed auditory/verbal recognition tests showed the highest sensitivity among all clusters (83%). Delayed recognition is an aspect that can be highly relevant while navigating through new terrain. Therefore, this should be considered especially for exploratory expeditions at altitude.
Although the number of studies using neurophysiological tests during acute hypoxia was limited, our data suggest that tests within the neurophysiological domain could be a particularly sensitive tool to assess CNS functions during hypoxia. At an oxygen saturation between 89% and 80%, the evoked potential cluster showed higher sensitivity than any other cluster. This is in line with previous research by Tsarouchas et al. which concluded that evoked brain responses can be used for early detection of cognitive alterations during exposure to moderate hypobaric hypoxia (Tsarouchas et al., 2008).
The impact of barometric pressure on the severity of hypoxia is a subject of debate (Savourey et al., 2003; Savourey et al., 2007; Millet et al., 2012; Naughton et al., 2012; Coppel et al., 2015; McMorris et al., 2017). In light of this, a secondary objective of this review was to explore the effects of barometric pressure on cognitive function. No significant difference was found in test sensitivity due to barometric pressure in the overall analysis at all oxygen saturation levels. Similarly, when only the (complex) attention or executive function domains were considered, no significant difference was found. Although the number of studies was too small to be conclusive, this review does not provide indications that barometric pressure has a large impact on hypoxia sensitivity. This supports the traditional consensus that normobaric and hypobaric hypoxia can be used interchangeably (Naughton et al., 2012). However, more recent findings of several studies indicate that normobaric and hypobaric hypoxia have different physiological effects (Savourey et al., 2003; Savourey et al., 2007; Millet et al., 2012; Coppel et al., 2015; McMorris et al., 2017). Coppel et al. suggested that the traditional view might be based on the barometric pressure only showing effects for longer exposure times (>3 h) (Coppel et al., 2015). This could explain why this review of acute hypoxia showed no effect of barometric pressure, as only 8% of our studies used exposure times of more than 3 h, and only one of these tested the executive domain.
One limitation of this study was its reliance on characterizing the literature based on inspired oxygen fraction or altitude levels. This approach is probably hiding relatively large interindividual SpO2 variability and therefore mixing within single studies participants with very different hypoxemic levels. This limitation is inherent to experiments where oxygen saturations are not individually controlled, which constituted the vast majority of the studies in this review. To address this limitation and provide a clearer understanding of the results, we expressed the variability in Table 1 within each study by providing measures such as standard deviation (SD), standard error of the mean (SEM), or 95% confidence interval (95% CI).
An associated limitation of this review may be the variation in the distribution and heterogeneity of SpO2 levels between different tests or clusters, which were derived from a heterogeneity of different studies. This disparity in SpO2 levels may account for the observed differences in sensitivity to hypoxia among these clusters. For instance, clusters with studies encompassing a wide range of hypoxic levels may have an increased likelihood of demonstrating higher sensitivity to hypoxia severity, whereas clusters with studies investigating a narrow range of hypoxic levels may have reduced chances of showing hypoxia sensitivity. Consequently, this effect could introduce bias in the analysis of the hypoxic-dose response.
A third limitation of this study is that the majority of included studies were not blinded. This lack of blinding may introduce bias, as participants and researchers being aware of the hypoxic or normoxic exposure could inadvertently influence cognitive performance assessments.
All these factors reflect variabilities and disparities between hypoxia studies, which limits the conclusions that can be reached from the literature, and the selection of the most sensitive and reliable tests of cognitive effects of acute hypoxia. This emphasizes the need for more standardisation of methodologies, to improve the comparability and generalizability of hypoxia experiments.
Conclusion
A large variety of cognitive tests were used in the literature to assess the effects of acute hypoxia on cognition in healthy adults. This huge methodological diversity is a major detriment to the investigation of the CNS effects of hypoxia. With these limitations, some suggestions can be made. The Stroop test as well as the clusters of sustained attention, auditory/verbal: delayed recognition, visual/spatial: delayed recognition, and evoked potential showed higher sensitivity than other tests and clusters. All clusters within the executive domain with more than 10 data points showed a clear level-response relationship or trend, with more frequent impairments at more severe levels of hypoxia. The data in our review showed no different physiological effect between hypobaric hypoxia and normobaric hypoxia. To further improve our understanding of the effects of acute hypoxia on cognition, standardization of exposure protocols and cognitive testing is crucial.
Data availability statement
The original contributions presented in the study are included in the article, further inquiries can be directed to the corresponding author.
Author contributions
TP and JvG designed the study. LH collected the data. TP and LH analyzed the data; JvG provided critical insights into the data analyses and interpretation; TP and LH wrote the original draft. All authors contributed to the article and approved the submitted version.
Conflict of interest
The authors declare that the research was conducted in the absence of any commercial or financial relationships that could be construed as a potential conflict of interest.
Publisher’s note
All claims expressed in this article are solely those of the authors and do not necessarily represent those of their affiliated organizations, or those of the publisher, the editors and the reviewers. Any product that may be evaluated in this article, or claim that may be made by its manufacturer, is not guaranteed or endorsed by the publisher.
References
Asmaro D., Mayall J., Ferguson S. (2013). Cognition at altitude: impairment in executive and memory processes under hypoxic conditions. Aviat. space, Environ. Med. 84 (11), 1159–1165. doi:10.3357/asem.3661.2013
Beebe D. W., Gozal D. (2002). Obstructive sleep apnea and the prefrontal cortex: towards a comprehensive model linking nocturnal upper airway obstruction to daytime cognitive and behavioral deficits. J. Sleep Res. 11 (1), 1–16. doi:10.1046/j.1365-2869.2002.00289.x
Beer J. M. A., Shender B. S., Chauvin D., Dart T. S., Fischer J. (2017). Cognitive deterioration in moderate and severe hypobaric hypoxia conditions. Aerosp. Med. Hum. Perform. 88 (7), 617–626. doi:10.3357/AMHP.4709.2017
Bishop N. A., Lu T., Yankner B. A. (2010). Neural mechanisms of ageing and cognitive decline. Nature 464 (7288), 529–535. doi:10.1038/nature08983
Blacker K. J., McHail D. G. (2022). Effects of acute hypoxia on early visual and auditory evoked potentials. Front. Neurosci. 16, 846001. doi:10.3389/fnins.2022.846001
Blacker K. J., McHail D. G. (2021). Time course of recovery from acute hypoxia exposure as measured by vigilance and event-related potentials. Physiology Behav. 239, 113508. doi:10.1016/j.physbeh.2021.113508
Branson R. D., Faarc R. (2018). Oxygen therapy in COPD. Respir. Care 63 (6), 734–748. doi:10.4187/respcare.06312
Caldwell H. G., Coombs G. B., Tymko M. M., Nowak-Flück D., Ainslie P. N. (2018). Severity-dependent influence of isocapnic hypoxia on reaction time is independent of neurovascular coupling. Physiology Behav. 188, 262–269. doi:10.1016/j.physbeh.2018.02.035
Castor M., Borgvall J. (2015). The effects of mild, acute hypoixa on cognitive performance. Report number: 2015:20. SSM Available at: https://www.researchgate.net/publication/280942574_The_effects_of_mild_acute_hypoxia_on_cognitive_performance (Accessed September 04, 2015).
Chokesuwattanaskul A., Chirakalwasan N., Jaimchariyatam N., Pitakvej N., Sarutikriangkri Y., Chunharas C., et al. (2021). Associations between hypoxia parameters in obstructive sleep apnea and cognition, cortical thickness, and white matter integrity in middle-aged and older adults. Sleep Breath. 25 (3), 1559–1570. doi:10.1007/s11325-020-02215-w
Chroboczek M., Kujach S., Łuszczyk M., Grzywacz T., Soya H., Laskowski R. (2022). Acute normobaric hypoxia lowers executive functions among young men despite increase of BDNF concentration. Int. J. Environ. Res. public health 19 (17), 10802. doi:10.3390/ijerph191710802
Coppel J., Hennis P., Gilbert-Kawai E., Grocott M. P. W. (2015). The physiological effects of hypobaric hypoxia versus normobaric hypoxia: A systematic review of crossover trials. Extreme Physiology Med. 4 (1), 2–20. doi:10.1186/s13728-014-0021-6
Davranche K., Casini L., Arnal P. J., Rupp T., Perrey S., Verges S. (2016). Cognitive functions and cerebral oxygenation changes during acute and prolonged hypoxic exposure. Physiology Behav. 164, 189–197. doi:10.1016/j.physbeh.2016.06.001
De Visser S. J., Van Der Post J., Pieters M. S. M., Cohen A. F., Van Gerven J. M. A. (2001). Biomarkers for the effects of antipsychotic drugs in healthy volunteers. Br. J. Clin. Pharmacol. 51 (2), 119–132. doi:10.1111/j.1365-2125.2001.01308.x
De Visser S. J., Van Der Post J. P., De Waal P. P., Cornet F., Cohen A. F., Van Gerven J. M. A. (2003). Biomarkers for the effects of benzodiazepines in healthy volunteers. Br. J. Clin. Pharmacol. 55 (1), 39–50. doi:10.1046/j.1365-2125.2002.t01-10-01714.x
Decroix L., De Pauw K., Van Cutsem J., Pattyn N., Heyman E., Meeusen R. (2018). Acute cocoa flavanols intake improves cerebral hemodynamics while maintaining brain activity and cognitive performance in moderate hypoxia. Psychopharmacology 235 (9), 2597–2608. doi:10.1007/s00213-018-4952-2
DeHart R. L., Davis J. R. (2002). Fundamentals of aerospace medicine. United States: Lippincott Williams & Wilkins.
Dumont G. J. H., De Visser S. J., Cohen A. F., Van Gerven J. M. A. (2005). Biomarkers for the effects of selective serotonin reuptake inhibitors (SSRIs) in healthy subjects. Br. J. Clin. Pharmacol. 59 (5), 495–510. doi:10.1111/j.1365-2125.2005.02342.x
Dumont G. J. H., Verkes R. J. (2006). A review of acute effects of 3,4-methylenedioxymethamphetamine in healthy volunteers. J. Psychopharmacol. Oxf. Engl. 20 (2), 176–187. doi:10.1177/0269881106063271
Falla M., Hüfner K., Falk M., Weiss E. M., Vögele A., Jan van Veelen M., et al. (2022). Simulated acute hypobaric hypoxia effects on cognition in helicopter emergency medical service personnel - a randomized, controlled, single-blind, crossover trial. Hum. factors 2022, 001872082210864. doi:10.1177/00187208221086407
Feeback M. R., Seo Y., Dancy M., Glickman E. L. (2017). The effect of psychomotor performance, cerebral and arterial blood saturation between african-American and caucasian males before, during and after normobaric hypoxic exercise. Int. J. Exerc. Sci. 10 (5), 655–665.
Gerhart H. D., Seo Y., Kim J. H., Followay B., Vaughan J., Quinn T., et al. (2019). Investigating effects of cold water hand immersion on selective attention in normobaric hypoxia. Int. J. Environ. Res. public health 16 (16), 2859. doi:10.3390/ijerph16162859
Gilbert-Kawai E. T., Milledge J. S., Grocott M. P. W., Martin D. S. (2014). King of the mountains: tibetan and sherpa physiological adaptations for life at high altitude. Physiology 29 (6), 388–402. doi:10.1152/physiol.00018.2014
Hewett K. J., Curry I. P., Gaydos S. J. (2010). Subtle cognitive effects of moderate hypoxia. Int. J. Appl. Aviat. Stud. 10 (1), 249–260.
Kammerer T., Faihs V., Hulde N., Bayer A., Hübner M., Brettner F., et al. (2018). Changes of hemodynamic and cerebral oxygenation after exercise in normobaric and hypobaric hypoxia: associations with acute mountain sickness. Ann. Occup. Environ. Med. 30 (1), 66. doi:10.1186/s40557-018-0276-2
Kerr P. M., Keeler J. M., Tourula E., Hite J., Baker T. B., Port N., et al. (2022). A gamified assessment does not identify alterations in cross-domain cognitive performance with acute moderate hypoxia. FASEB J. 36 (1), 792. doi:10.1096/fasebj.2022.36.s1.l7792
Kim C. H., Ryan E. J., Seo Y., Peacock C., Gunstad J., Muller M. D., et al. (2015). Low intensity exercise does not impact cognitive function during exposure to normobaric hypoxia. Physiology Behav. 151, 24–28. doi:10.1016/j.physbeh.2015.07.003
Kourtidou-Papadeli C., Papadelis C., Koutsonikolas D., Boutzioukas S., Styliadis C., Guiba-Tziampiri O. (2008). High altitude cognitive performance and COPD interaction. Hippokratia 12, 84–90.
Lefferts W. K., Babcock M. C., Tiss M. J., Ives S. J., White C. N., Brutsaert T. D., et al. (2016). Effect of hypoxia on cerebrovascular and cognitive function during moderate intensity exercise. Physiology Behav. 165, 108–118. doi:10.1016/j.physbeh.2016.07.003
Legg S., Hill S., Mundel T., Gilbey A., Schlader Z., Raman A. (2012). Could mild hypoxia impair pilot decision making in emergencies? Work (Reading, Mass) 41 (1), 198–203. doi:10.3233/WOR-2012-0156-198
Legg S. J., Gilbey A., Hill S., Raman A., Dubray A., Iremonger G., et al. (2016). Effects of mild hypoxia in aviation on mood and complex cognition. Appl. Ergon. 53, 357–363. doi:10.1016/j.apergo.2015.10.002
Lei O. K., Kong Z., Loprinzi P. D., Shi Q., Sun S., Zou L., et al. (2019). Severe hypoxia does not offset the benefits of exercise on cognitive function in sedentary young women. Int. J. Environ. Res. public health 16 (6), 1003. doi:10.3390/ijerph16061003
Loprinzi P. D., Matalgah A., Crawford L., Yu J. J., Kong Z., Wang B., et al. (2019). Effects of acute normobaric hypoxia on memory interference. Brain Sci. 9 (11), 323. doi:10.3390/brainsci9110323
Malle C., Bourrilhon C., Pierard C., Quinette P., Laisney M., Eustache F. (2016). Physiological and cognitive effects of acute normobaric hypoxia and modulations from oxygen breathing. Aerosp. Med. Hum. Perform. 87 (1), 3–12. doi:10.3357/AMHP.4335.2016
McMorris T., Hale B. J., Barwood M., Costello J., Corbett J. (2017). Effect of acute hypoxia on cognition: A systematic review and meta-regression analysis. Neurosci. Biobehav. Rev. 74, 225–232. doi:10.1016/j.neubiorev.2017.01.019
Millet G. P., Faiss R., Pialoux V. (2012). Point: hypobaric hypoxia induces different physiological responses from normobaric hypoxia. J. Appl. Physiol. 112 (10), 1783–1784. doi:10.1152/japplphysiol.00067.2012
Mulroney S. E., Myers A. K., Netter F. H. (2015). Netter's essential physiology. Netherlands: Elsevier, 168.
Nakata H., Miyamoto T., Ogoh S., Kakigi R., Shibasaki M. (2017). Effects of acute hypoxia on human cognitive processing: a study using ERPs and SEPs. J. Appl. physiology 123 (5), 1246–1255. doi:10.1152/japplphysiol.00348.2017
Naughton M. T., Rochford P. D., Pretto J. J., Pierce R. J., Cain N. F., Irving L. B. (2012). Is normobaric simulation of hypobaric hypoxia accurate in chronic airflow limitation? Am. J. Respir. Crit. Care Med. 152 (6), 1956–1960. doi:10.1164/ajrccm.152.6.8520762
Navarrete-Opazo A., Mitchell G. S. (2014). Therapeutic potential of intermittent hypoxia: a matter of dose. Am. J. Physiology 307 (10), R1181–R1197. doi:10.1152/ajpregu.00208.2014
Noble J., Jones J. G., Davis E. J. (1993). Cognitive function during moderate hypoxaemia. Anaesth. intensive care 21 (2), 180–184. doi:10.1177/0310057X9302100208
Ochi G., Yamada Y., Hyodo K., Suwabe K., Fukuie T., Byun K., et al. (2018). Neural basis for reduced executive performance with hypoxic exercise. NeuroImage 171, 75–83. doi:10.1016/j.neuroimage.2017.12.091
Ogoh S., Nakata H., Miyamoto T., Bailey D. M., Shibasaki M. (2018). Dynamic cerebral autoregulation during cognitive task: effect of hypoxia. J. Appl. physiology 124 (6), 1413–1419. doi:10.1152/japplphysiol.00909.2017
Orešič M., Hyötyläinen T., Herukka S. K., Sysi-Aho M., Mattila I., Seppänan-Laakso T., et al. (2011). Metabolome in progression to Alzheimer's disease. Transl. Psychiatry 1 (12), e57. doi:10.1038/tp.2011.55
Rahman A., Tabassum T., Araf Y., Al Nahid A., Ullah M. A., Hosen M. J. (2021). Silent hypoxia in COVID-19: pathomechanism and possible management strategy. Mol. Biol. Rep. 48 (4), 3863–3869. doi:10.1007/s11033-021-06358-1
Reményi Á., Grósz A., Szabó S. A., Tótka Z., Molnár D., Helfferich F. (2018). Comparative study of the effect of bilastine and cetirizine on cognitive functions at ground level and at an altitude of 4,000 m simulated in hypobaric chamber: a randomized, double-blind, placebo-controlled, cross-over study. Expert Opin. Drug Saf. 17 (9), 859–868. doi:10.1080/14740338.2018.1502268
Rosen M. L., Amso D., McLaughlin K. A. (2019). The role of the visual association cortex in scaffolding prefrontal cortex development: A novel mechanism linking socioeconomic status and executive function. Dev. Cogn. Neurosci. 39, 100699. doi:10.1016/j.dcn.2019.100699
Rossetti G. M. K., D'Avossa G., Rogan M., Macdonald J. H., Oliver S. J., Mullins P. G. (2021). Reversal of neurovascular coupling in the default mode network: evidence from hypoxia. J. Cereb. Blood Flow Metabolism 41 (4), 805–818. doi:10.1177/0271678X20930827
Savourey G., Launay J. C., Besnard Y., Guinet A., Travers S. (2003). Normo- and hypobaric hypoxia: are there any physiological differences? Eur. J. Appl. Physiology 89 (2), 122–126. doi:10.1007/s00421-002-0789-8
Savourey G., Launay J. C., Besnard Y., Guinet-Lebreton A., Alonso A., Sauvet F., et al. (2007). Normo or hypobaric hypoxic tests: propositions for the determination of the individual susceptibility to altitude illnesses. Eur. J. Appl. Physiology 100 (2), 193–205. doi:10.1007/s00421-007-0417-8
Seech T. R., Funke M. E., Sharp R. F., Light G. A., Blacker K. J. (2020). Impaired sensory processing during low-oxygen exposure: A noninvasive approach to detecting changes in cognitive states. Front. Psychiatry 11, 11. doi:10.3389/fpsyt.2020.00012
Selvam S., Kumar T., Fruttiger M. (2018). Retinal vasculature development in health and disease. Prog. Retin. Eye Res. 63, 1–19. doi:10.1016/j.preteyeres.2017.11.001
Seo Y., Burns K., Fennell C., Kim J. H., Gunstad J., Glickman E., et al. (2015). The influence of exercise on cognitive performance in normobaric hypoxia. High Alt. Med. Biol. 16 (4), 298–305. doi:10.1089/ham.2015.0027
Seo Y., Gerhart H. D., Stavres J., Fennell C., Draper S., Glickman E. L. (2017). Normobaric hypoxia and submaximal exercise effects on running memory and mood state in women. Aerosp. Med. Hum. Perform. 88 (7), 627–632. doi:10.3357/AMHP.4798.2017
Shaked D., Katzel L. I., Seliger S. L., Gullapalli R. P., Davatzikos C., Erus G., et al. (2018). Dorsolateral prefrontal cortex volume as a mediator between socioeconomic status and executive function. Neuropsychology 32 (8), 985–995. doi:10.1037/neu0000484
Sivak E. D., Shefner J. M., Sexton J. (1999). Neuromuscular disease and hypoventilation. Curr. Opin. Pulm. Med. 5 (6), 355–362. doi:10.1097/00063198-199911000-00006
Stepanek J., Cocco D., Pradhan G. N., Smith B. E., Bartlett J., Studer M., et al. (2013). Early detection of hypoxia-lnduced cognitive impairment using the King-Devick test. Aviat. Space, Environ. Med. 84 (10), 1017–1022. doi:10.3357/asem.3616.2013
Strauss E., Sherman E. M. S., Spreen O. (2006). A Compendium of neuropsychological tests: administration, Norms and commentary. New York: NY: Oxford University Press.
Tsarouchas N., Benedek K., Bezerianos A., Benedek G., Keri S. (2008). Effects of moderate hypobaric hypoxia on evoked categorical visuocognitive responses. Clin. Neurophysiol. 119 (7), 1475–1485. doi:10.1016/j.clinph.2008.02.021
Turner C. E., Barker-Collo S. L., Connell C. J. W., Gant N. (2015a). Acute hypoxic gas breathing severely impairs cognition and task learning in humans. Physiology Behav. 142, 104–110. doi:10.1016/j.physbeh.2015.02.006
Turner C. E., Byblow W. D., Gant N. N. (2015b). Creatine supplementation enhances corticomotor excitability and cognitive performance during oxygen deprivation. J. Neurosci. 35 (4), 1773–1780. doi:10.1523/JNEUROSCI.3113-14.2015
Valk P. J. L., Simons R., Jetten A. M., Valiente R., Labeaga L. (2016). Cognitive performance effects of bilastine 20 mg during 6 hours at 8000 ft cabin altitude. Aerosp. Med. Hum. Perform. 87 (7), 622–627. doi:10.3357/AMHP.4522.2016
van der Post J., Noordzij L. A., de Kam M. L., Blauw G. J., Cohen A. F., van Gerven J. M. (2002). Evaluation of tests of central nervous system performance after hypoxemia for a model for cognitive impairment. J. Psychopharmacol. 16 (4), 337–343. doi:10.1177/026988110201600408
Van Gerven J. M. A. (2019). “Functional measurements of central nervous system drug effects in early human drug development,” in Translational medicine in CNS drug development. Editors G. Nomikos, and D. Douglas Feltner (Netherlands: Elsevier Academic Press), 39–61.
Wang J., Ke T., Zhang X., Chen Y., Liu M., Chen J., et al. (2013). Effects of acetazolamide on cognitive performance during high-altitude exposure. Neurotoxicology Teratol. 35 (1), 28–33. doi:10.1016/j.ntt.2012.12.003
Williams T. B., Corbett J., McMorris T., Young J. S., Dicks M., Ando S., et al. (2019). Cognitive performance is associated with cerebral oxygenation and peripheral oxygen saturation, but not plasma catecholamines, during graded normobaric hypoxia. Exp. Physiol. 104 (9), 1384–1397. doi:10.1113/EP087647
Woodrow A. D., Webb J. T. (2011). Handbook of aerospace and operational physiology. Berlin, Germany: Springer.
Woorons X., Richalet J. P. (2021). Modelling the relationships between arterial oxygen saturation, exercise intensity and the level of aerobic performance in acute hypoxia. Eur. J. Appl. Physiology 121 (7), 1993–2003. doi:10.1007/s00421-021-04667-8
Zoethout R. W. M., Delgado W. L., Ippel A. E., Dahan A., Van Gerven J. M. A. (2011). Functional biomarkers for the acute effects of alcohol on the central nervous system in healthy volunteers. Br. J. Clin. Pharmacol. 71 (3), 331–350. doi:10.1111/j.1365-2125.2010.03846.x
Keywords: acute normobaric hypoxia, acute hypobaric hypoxia, cognitive function, healthy volunteers, sensitivity
Citation: Post TE, Heijn LG, Jordan J and van Gerven JMA (2023) Sensitivity of cognitive function tests to acute hypoxia in healthy subjects: a systematic literature review. Front. Physiol. 14:1244279. doi: 10.3389/fphys.2023.1244279
Received: 22 June 2023; Accepted: 25 September 2023;
Published: 11 October 2023.
Edited by:
Dieter Blottner, Charité University Medicine Berlin, GermanyReviewed by:
Samuel Verges, Université Grenoble Alpes, FranceMichele Salanova, Charité University Medicine Berlin, Germany
Copyright © 2023 Post, Heijn, Jordan and van Gerven. This is an open-access article distributed under the terms of the Creative Commons Attribution License (CC BY). The use, distribution or reproduction in other forums is permitted, provided the original author(s) and the copyright owner(s) are credited and that the original publication in this journal is cited, in accordance with accepted academic practice. No use, distribution or reproduction is permitted which does not comply with these terms.
*Correspondence: Titiaan E. Post, tpost@chdr.nl