- 1Department of Intensive Care Medicine, Inselspital, Bern University Hospital, University of Bern, Bern, Switzerland
- 2Department of Anaesthesia, SV Hospital Group, Institute of Clinical Sciences at the Sahlgrenska Academy, University of Gothenburg, Gothenburg, Sweden
- 3Department of Anaesthesiology and Intensive Care, University of Tartu, Tartu, Estonia
Heart-lung interactions occur due to the mechanical influence of intrathoracic pressure and lung volume changes on cardiac and circulatory function. These interactions manifest as respiratory fluctuations in venous, pulmonary, and arterial pressures, potentially affecting stroke volume. In the context of functional hemodynamic monitoring, pulse or stroke volume variation (pulse pressure variation or stroke volume variability) are commonly employed to assess volume or preload responsiveness. However, correct interpretation of these parameters requires a comprehensive understanding of the physiological factors that determine pulse pressure and stroke volume. These factors include pleural pressure, venous return, pulmonary vessel function, lung mechanics, gas exchange, and specific cardiac factors. A comprehensive knowledge of heart-lung physiology is vital to avoid clinical misjudgments, particularly in cases of right ventricular (RV) failure or diastolic dysfunction. Therefore, when selecting monitoring devices or technologies, these factors must be considered. Invasive arterial pressure measurements of variations in breath-to-breath pressure swings are commonly used to monitor heart-lung interactions. Echocardiography or pulmonary artery catheters are valuable tools for differentiating preload responsiveness from right ventricular failure, while changes in diastolic function should be assessed alongside alterations in airway or pleural pressure, which can be approximated by esophageal pressure. In complex clinical scenarios like ARDS, combined forms of shock or right heart failure, additional information on gas exchange and pulmonary mechanics aids in the interpretation of heart-lung interactions. This review aims to describe monitoring techniques that provide clinicians with an integrative understanding of a patient’s condition, enabling accurate assessment and patient care.
Introduction and clinical assessment
Heart-lung interactions describe cardio-circulatory phenomena that are caused by the breathing pattern. The source of these phenomena is the coupling of the respiratory rate and heartrate, and thereby arterial and venous pulse pressures (Fisher et al., 2022). In contrast to some monitoring techniques in the field of intensive care medicine, which may be highly elaborate and technical, clinical assessment of respiratory rate and pulse pressure is simple. Several forms of heart-lung interactions are easily recognized from observation and bedside examination. An excellent clinical example is pulsus paradoxus - a drop of arterial pressure upon inspiration of more than 10 mmHg - which may occur in cardiac tamponade, severe airflow obstruction in acute asthma, tension pneumothorax, or severe RV failure. Another phenomenon is respiratory sinus arrhythmia (Sarkar et al., 2018). Palpation of the arterial pulse pressure can be aided by observing the pulse oximeter, where PPV can be visualized directly on the monitor without the need for an invasive pressure monitoring (Hartert et al., 1999). Other examples of heart-lung interactions are the reaction of the jugular vein and the arterial pulse to the Müller or Valsalva maneuvers (forced inspiration or expiration against a closed airway, respectively). In patients with decompensated left heart failure, the jugular vein collapses and the arterial pressure drops during a Müller maneuver, while a Valsalva maneuver will distend the jugular veins and increase the arterial pulse pressure (Buda et al., 1979; Marantz et al., 1990). These phenomena result from changes in intrathoracic and pericardial pressures and are physiologically linked through complex interactions with pre- and afterload (Grubler et al., 2017).
The clinician should remember that heart-lung interactions are part of normal physiology, occurring also in health. However, these phenomena are exaggerated under pathological conditions and can be provoked by changes in intrathoracic pressures, which makes them potentially useful in a clinical scenario of impaired hemodynamics. In the intensive care patient, intrathoracic pressure changes are mainly caused by mechanical ventilation. Within the framework of functional hemodynamic monitoring (Pinsky, 2014a) and depending on the clinical context, heart-lung interactions may provide diagnostic clues on the patient’s cardio-circulatory status. Advanced monitoring techniques gather information on the underlying pathophysiology of the observed phenomenon and enable the clinician to diagnose and treat the patient correctly.
The explanatory model: optimal monitoring within the framework of venous return and cardiac function
To understand heart-lung interactions, one must briefly review Guyton’s framework of venous return (Guyton et al., 1957): the outflow from the heart is completely dependent on the inflow (Starling, 1918). The inflow—that is venous return—depends on the elastic recoil and the volume in the vascular system (Moller et al., 2019). The volume and elastic recoil create the mean systemic filling pressure (MSFP), which pushes the blood towards the right atrium (Magder, 2016). The right atrial pressure (RAP) acts as a back pressure to venous return (Moller et al., 2017). As with any system of related pressure and flow, the flow to the right atrium is opposed by the resistance to venous return (RVR) (Berger et al., 2016a; Bloch et al., 2016; Berger et al., 2019; Moller et al., 2019). The mean systemic filling pressure is the equilibrated vascular pressure of the systemic circulation at zero flow (Magder and De Varennes, 1998). A central function of the RV is to actively lower right atrial pressure to facilitate the return of blood, while the left ventricle pumps the blood back to the volume reservoir in the vascular system. This can be expressed in a simple term (VR: venous return, CO: cardiac output, MSFP: mean systemic filling pressure, RVR: resistance to venous return):
In a venous return curve, right atrial pressure is plotted against venous return. When venous return and cardiac function curves (Starling curve, Figure 1) are superimposed, it becomes apparent that the right atrial (or central venous pressure) serves as the equilibrium point for any given cardiovascular state. With this graphical framework, changes in inflow to the heart explain the heart-lung interactions. When right atrial pressure rises during positive pressure ventilation, venous return and thereby stroke volume must fall. The cardiac function curve shifts to the right (red arrow Figure 1A) resulting in higher RAP and lower cardiac output (and thus venous return). As the venous return curve intersects the cardiac function curve at its steep part, volume expansion (increase in MSFP, dotted blue line Figure 1A) will increase stroke volume (Moller et al., 2019). This situation would be considered as “volume responsive” or “limited by venous return”. If the venous return curve intersects the Starling curve at its flat part (higher venous return and lower RAP, green line Figure 1A), volume expansion will cause minimal increase in stroke volume. As most ICU patients are volume unresponsive (Cecconi et al., 2015), one must always consider the factors shown in panel B of Figure 1. Breath-to-breath increases in afterload (or decreases in inotropy, red dotted line Figure 1B) may flatten the cardiac response curve, particularly in scenarios of RV failure or pulmonary hypertension, and may mimic cyclic changes in stroke volume (Vieillard-Baron et al., 1999; Daudel et al., 2010; Valenti et al., 2021). Such patients would be considered “cardiac limited”, and their hemodynamic state would not benefit from volume expansion (no increase in venous return through rightward shift of venous return curve, Figure 1B). Administration of inotropic therapy to restore the cardiac function curve may allow the patient to benefit from increases in MSFP (green dotted line, Figure 1B). In conclusion, the extent of pulse pressure or stroke volume variation in itself is not sufficient to correctly diagnose the underlying condition and apply the correct treatment strategy (Sondergaard, 2013).
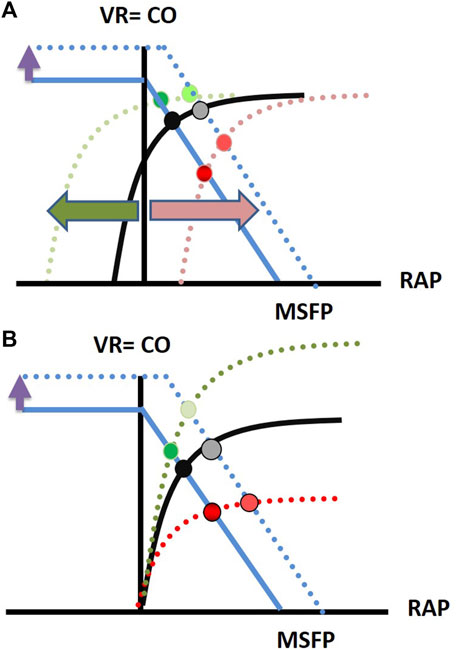
FIGURE 1. The superimposition of a Starling curve and a venous return curve provides a graphical solution to the question if a patient’s circulation is limited by venous return or cardiac function. This explains the key factors of heart-lung interactions: (A) Effect of volume expansion on normal Starling curve (volume responsive or limited by venous return situation) (B) Effect of volume expansion on flattened and steepened Starling curve (cardiac limited situation). Details are found in the text.
The optimal monitoring tool for the comprehensive assessment and interpretation of a patient showing heart-lung interactions would provide a prospective, combined image of venous return and cardiac function. This is not available. Of the variables necessary to plot a venous return function, only cardiac output (which equals venous return) and central venous pressure (e.g., right atrial pressure) are often assessed in shocked ICU patients. In order to fully assess the venous return function, resistance to venous return and mean systemic filling pressure should be available. Mean systemic filling pressure can be measured during circulatory standstill (Repesse et al., 2015; Repesse et al., 2017), but only surrogate measures can make their way to the bedside. Versprille and others developed a method of constructing venous return curves by progressively increasing airway pressures with consecutive inspiratory hold maneuvers (Versprille and Jansen, 1985; Hartog et al., 1996), which allows to extrapolate mean systemic filling pressure. The method was later refined by the research group of Maas and others (Maas et al., 2009; Maas et al., 2011; Maas et al., 2012; Persichini et al., 2012; Wijnberge et al., 2022). With this method, the expected hemodynamic responses according to the physiological framework of venous return, could be confirmed following volume expansion and vasoconstriction in patients after cardiac surgery, and during septic shock (Maas et al., 2009; Wijnberge et al., 2022; van den Berg et al., 2002; Aya et al., 2017; Cecconi et al., 2013), but the extrapolated values of MSFP may be inaccurate (Berger et al., 2016b). The underlying volume state (i.e., the state of interest) influences the accuracy of the measurements (Berger et al., 2016b; Werner-Moller et al., 2019), which may render this method invalid at the bedside (Moller and Berger, 2023). Parkin and Leaning developed a simple formula to calculate an analogue for mean systemic pressure (Pmsa) based on the readily available variables of right atrial pressure, mean arterial pressure and cardiac output (Parkin and Wright, 1991; Parkin et al., 1994; Parkin, 1999; Parkin and Leaning, 2008; Sondergaard et al., 2016). The method also allows for quantitative assessment of heart efficiency and the effect of volume expansion (Gupta et al., 2015; Sondergaard et al., 2016) and was validated with good accuracy and precision against a zero-flow reference method (Werner-Moller et al., 2022).
Monitoring of arterial and central venous pressure swings
One of the first descriptions of heart lung interactions goes back to the 18th century, when Sir Stephen Hales placed a glass tube into the jugular vein of a mare and observed a cyclic change of the blood column during respiration (Sette et al., 2012). Ever since, invasive pressure measurements have been a cornerstone for heart-lung interactions, where the pulse pressure variation serves as a surrogate for the stroke volume variation caused by heart-lung interactions. As invasive monitoring of central venous and arterial pressure still constitutes the best practice standard for shocked patients in the ICU (Cecconi et al., 2014), these swings will be readily observable, with most research interest and clinical attention given to the arterial pressure. However, interpretation is only possible in patients without spontaneous respiration (Jardin, 2004; Magder, 2004). Most commonly, the arterial pressure swings are taken as signs of overt or relative hypovolemia or volume responsiveness. At this point, we must remember a first caveat: a circulation which is not cardiac limited will always be fluid responsive (Magder, 2004). Fluid responsiveness is neither a pathological condition, nor does it indicate that fluid administration is necessary or beneficial. The question of fluid responsiveness must be preceded by a clinical assessment of whether fluid expansion and/or increased flow is at all warranted (Magder, 2004; Takala, 2016).
Based on experimental work by Morgan et al. (1966), Perel et al. (1987), Michard et al. (2000) showed in a landmark paper that arterial pulse pressure variability over the respiratory cycle predicted fluid responsiveness (defined as a pulse pressure variability of >13% for an increase in cardiac output of 15%) in septic patients with circulatory failure.
Pulse pressure variation (PPV) is a dynamic test: a reproducible change in the pleural pressure over the respiratory cycle elicits a response from the cardiovascular system. The introduction of dynamic testing was a major advance, since static one-point measurements like central venous or pulmonary artery occlusion pressure was proven unreliable for the prediction of volume responsiveness (Osman et al., 2007; Marik and Cavallazzi, 2013). Assessment of PPV inspired a high research interest and was developed further to include additional monitoring modalities like stroke volume variability (SVV) from pulse contour analysis or analysis of pulse-oximetry plethysmograms, and specific maneuvers to increase the diagnostic yield, discussed below.
The central physiological rationale behind these techniques is the cyclic increase in RAP caused by an increase in pleural pressure from mechanical inspiration. This immediately lowers venous return (Moller et al., 2017) and RV stroke volume. This smaller stroke volume is forwarded to the left ventricle, where the lower pulse pressure appears during expiration. This idea fits well with the isolated concept of venous return, but it neglects all factors from the right heart, cardiac valves and pulmonary factors (Sondergaard, 2013). The meta-analytic pooled sensitivity and specificity reached almost 90% for volume responsiveness (albeit with tidal volumes larger than 8 mL/kg, which is not currently standard of care) (Yang and Du, 2014) and may be increased with provocation maneuvers. In situations of lung protective ventilation, a stepwise increase of the tidal volume (tidal volume challenge) or the end-expiratory occlusion test may increase the diagnostic performance for pulse pressure or stroke volume variation (Monnet et al., 2009; Wang et al., 2023).
The critical care physician is well advised to interpret PPV and provocation tests, with a second caveat: as the entire pulmonary compartment including the RV lies before the measurement of systemic arterial PPV, it signals a preload dependency of the left ventricle (Magder, 2007) - but not of the entire circulation. Factors in the lung like atelectasis, changes in tidal volumes, hypoxic vasoconstriction, and pulmonary hypertension may blunt PPV while RV failure may cause PPV, but further volume administration in RV failure can be detrimental (Sondergaard, 2013; Slobod et al., 2022). Therefore, RV failure and pulmonary hypertension render PPV invalid for the prediction of volume responsiveness (Daudel et al., 2010; Wyler von Ballmoos et al., 2010). In such context, PPV and related techniques may rather reflect an afterload-dependency of the RV (Vieillard-Baron et al., 1999; Vieillard-Baron et al., 2015; Valenti et al., 2021). Several pulmonary factors like compliance (Robotham et al., 1983; de Waal et al., 2009; Mesquida et al., 2011), respiratory rate, tidal volume (De Backer et al., 2005; De Backer et al., 2009; Slobod et al., 2022), and airway driving pressure (Muller et al., 2010) influence PPV, as will circulatory factors like the need for a sinus rhythm and aortic elastance and thus ventricular-aortic coupling (Magder, 2010; Sondergaard, 2013). Since the introduction of PPV derived methods at the bedside, a complex series of clinical and physiological conditions were identified as relevant influences. The clinician should account for these factors before taking decisions based on PPV (Sondergaard, 2013; Vieillard-Baron et al., 2016). In order to assess PPV within an integrative picture, additional information (e.g., specific monitoring) is needed.
Besides invasive arterial pressure, invasive measurement of central venous or right atrial pressure are routinely available (Cecconi et al., 2014). A single static measurement of CVP neither predicts blood volume nor volume responsiveness (Marik and Cavallazzi, 2013), but the CVP is the equilibrium or interaction point of the cardiac and venous return function. When understood as such, changes in CVP over time or during specific maneuvers may provide valuable information: a healthy RV will keep central venous pressure low. An increasing CVP therefore reflects a declining cardiac function, and/or an increased venous return from increased blood volume or vasoconstriction (Magder, 2017). A rapid, sustained rise in CVP from a volume bolus, without increase in stroke volume, is a strong indicator of right heart dysfunction and an important safety limit against further volume expansion (Magder, 2017). The inspiratory drop of CVP is one of the few measurements that allow estimation of fluid responsiveness in the spontaneously breathing patient. The absence of an inspiratory negative swing makes fluid responsiveness unlikely (high negative predictive value), but the positive predictive value is poor (Magder et al., 1992; Magder, 2017).
We have now an integrated assessment of arterial and central venous pressure - one measured downstream and the other upstream of the heart. If these measurements cannot identify the relevant factors to assess the clinical situation, the use of more advanced monitoring techniques is necessary.
Advanced bedside techniques: pulmonary artery catheterization, transpulmonary thermodilution, and echocardiography
Even though use of the pulmonary artery catheter (PAC) has declined over the last decades after a series of negative clinical trials (Parker et al., 2023), it remains a cornerstone for complex hemodynamic monitoring and is still recommended for situations with impending right heart failure and for patients unresponsive to initial treatment (Vieillard-Baron et al., 2016). As increases in RV afterload are common in pulmonary diseases and may exacerbate heart-lung interactions (Vieillard-Baron et al., 1999; Schmitt et al., 2001; Vieillard-Baron and Jardin, 2003; Repessé et al., 2016; Valenti et al., 2021), the PAC’s ability to monitor RV dysfunction with an increased ratio of CVP to pulmonary artery occlusion pressure is helpful (Monchi et al., 1998; Osman et al., 2009). Additionally, since disturbed gas exchange may contribute to hypoxic pulmonary vasoconstriction - often presenting as an increased transpulmonary pressure gradient (Bull et al., 2010) and an increased isovolumetric contraction pressure in pulmonary artery pressure tracings (Jardin et al., 1987; Jardin et al., 1989; Berger et al., 2014; Slobod et al., 2022) - the continuous assessment of mixed venous oxygenation can help assess the extent of vasoconstriction and shunt, and the contribution of venous admixture to oxygenation disorders (Takala, 2007; Bootsma et al., 2022). The pulmonary artery pulsatility index has rarely been studied for heart-lung interactions but offers a theoretically promising tool (Lim and Gustafsson, 2020; Bootsma et al., 2022). A shortcoming of the PAC—stemming from the thermodilution technique - is its inability to report immediate changes in stroke volume. However, pulmonary pulse contour analysis with direct assessment of RV stroke volume is feasible (Berger et al., 2020) and this limitation may be overcome by advanced PAC models with shorter thermodilution response times (Bootsma et al., 2022).
Monitoring tools that enhance the accuracy of continuous pulse contour analysis by intermittent calibration using transpulmonary thermodilution offer the advantage of beat-to-beat stroke volume assessment. They also report volumetric preload parameters like global end-diastolic or intrathoracic blood volumes (Reuter et al., 2002; Reuter et al., 2010). Importantly, both derive from a (common) global measure of indicator distribution volume and as such are unable to distinguish between anatomical structures of the right and left heart. Therefore, the technique cannot in itself recognize right heart failure, which is the main imitator of fluid responsiveness.
Critical care echocardiography has become an indispensable bedside tool for hemodynamic assessment, both for diagnosis and monitoring (Vieillard-Baron et al., 2019). Doppler echocardiography with measurements of pulmonary venous flow velocity and left ventricular (LV) velocity time integrals contributed significantly to the elucidation of the pathophysiology of heart-lung interactions. The inspiratory increases in arterial pulse pressure are caused by increased LV filling (Vieillard-Baron et al., 2003). For the RV, an inspiratory drop of stroke volume is caused by increased afterload (Vieillard-Baron et al., 1999).
Various dynamic echocardiographic tests were developed to predict fluid responsiveness: change in the diameter of the superior vena cava over the respiratory cycle has been proposed as a volume gauge (Vieillard-Baron et al., 2004), but it is influenced by thoracic compliance and may become decoupled from stroke volume in cases of high RV afterload (Lansdorp et al., 2014; Valenti et al., 2021). Diameter changes in the inferior cava are not valid in situations of increased abdominal pressure (Vieillard-Baron et al., 2018a). The sensitivity to predict fluid responsiveness for both methods is moderate: Vignon et al. (2008) demonstrated—in a direct comparison of both caval vein methods—“classic” arterial pulse pressure variation and changes in the aortic flow velocity, that respiratory changes of the maximum aortic flow velocity had the highest diagnostic yield and outperformed invasive pulse pressure variation for the prediction of fluid responsiveness (Vignon et al., 2017). VEXUS (venous excess ultrasound score) is a relatively new approach, not based on dynamic indices, that may allow assessment of a patient’s volume state in relation to the right ventricular function. It is based on IVC diameter and the flow pattern of hepatic, portal, and renal veins as well as renal arterial resistive index and grades the level of venous congestion (Argaiz et al., 2022; Longino et al., 2023). This promising tool may add to the integral assessment of a patient’s hemodynamic condition but needs further validation in critically ill patients.
Echocardiography is also a key modality for the diagnosis of diastolic dysfunction. The role of diastolic dysfunction for heart-lung interactions is unclear. Since all echocardiographic parameters for diastolic dysfunction depend on cardiac loading conditions, this interdependency should be evaluated in future research projects (Vignon et al., 2007; Juhl-Olsen et al., 2012; Juhl-Olsen et al., 2013; Berger et al., 2022a; Berger et al., 2022b).
A particular strength of echocardiography is its ability not only to monitor hemodynamics, but to diagnose specific conditions like tamponade or acute core pulmonale, which may aggravate or mimic heart-lung interactions (Vieillard-Baron et al., 2018b; Vieillard-Baron et al., 2020). The major differential diagnosis for the occurrence of heart-lung interactions is volume responsiveness vs. right heart failure (Vieillard-Baron et al., 2016), or a preload-vs. an afterload problem (18). This includes the visualization of the diastolic interventricular dependency with leftward septal shift and increased right ventricular and decreased left ventricular end-diastolic volumes as key components for the understanding of why right ventricular failure may lead to PPV. Here, echocardiography plays a crucial role, but the clinician must remember several caveats: there is no common echocardiographic definition of RV failure (Vieillard-Baron et al., 2018c). Standard measures like TAPSE may not be applicable in critically ill patients (Vieillard-Baron et al., 2020). Since the RV works below its stressed volume (Pinsky et al., 1992; Pinsky, 2014b; Bachmann et al., 2020), RV dilation is a normal adaption to increased load, until the compliance reserve is exhausted and central venous pressure rises. Table 1 summarizes the diagnostic approaches.
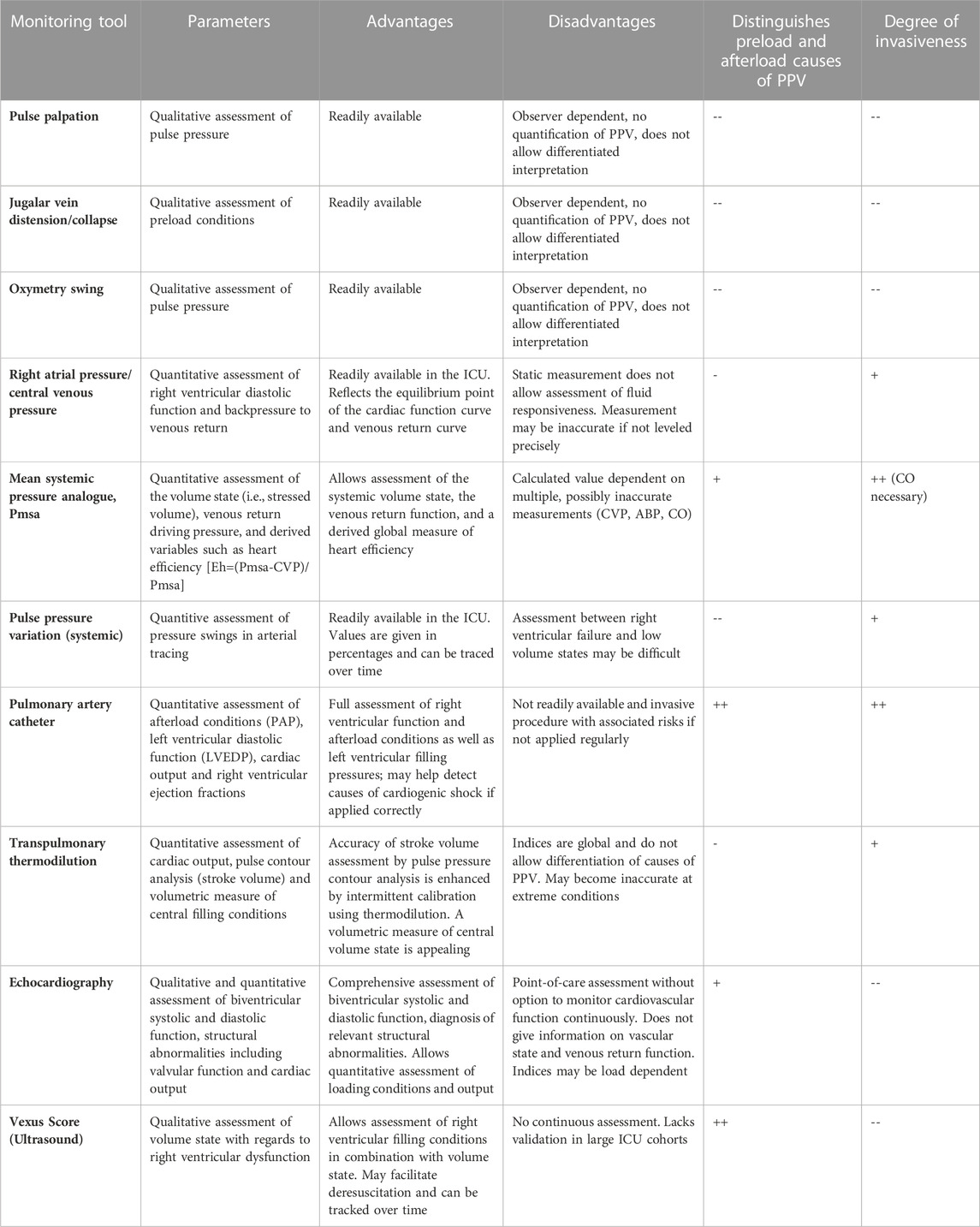
TABLE 1. Monitoring tools presented within their respective category and their advantages and disadvantages. Distinguishment between preload and afterload causes of PPV as well as degree of invasiveness were rated by the authors using the following scale (--) strongly disagree, (−) partially disagree, (0) no statement possible, (+) partially agree, (++) strongly agree.
Conclusion
Heart-lung interactions provide valuable insights into the pathophysiology of patients, with the potential to provide crucial information for personalizing treatment. The best choice of monitoring tool depends on several factors, including the clinical scenario, the local accessibility and proficiency of a specific technique, as well as the familiarity, training, and confidence of the clinician in utilizing a particular method. Optimal information can be obtained through the integration of multiple sources, employing dynamic testing and trend analysis, and gradually advancing the monitoring process if the initial treatment proves ineffective. When uncertainty arises, it is essential to actively consider the possibility of RV failure.
Author contributions
DB wrote the initial draft of the manuscript. KB and PW revised it for intellectual content. All authors contributed to the article and approved the submitted version.
Conflict of interest
The Department of Intensive Care Medicine at the Inselspital has, or has had in the past, research contracts with Abionic SA, AVA AG, CSEM SA, Cube Dx GmbH, Cyto Sorbents Europe GmbH, Edwards Lifesciences LLC, GE Healthcare, ImaCor Inc., MedImmune LLC, Orion Corporation, Phagenesis Ltd. and research and development/consulting contracts with Edwards Lifesciences LLC, Nestec SA, Wyss Zurich. The money was paid into a departmental fund; DB and the other authors received no personal financial gain. The Department of Intensive Care Medicine has received unrestricted educational grants from the following organizations for organizing a quarterly postgraduate educational symposium, the Berner Forum for Intensive Care (until 2015): Abbott AG, Anandic Medical Systems, Astellas, AstraZeneca, Bard Medica SA, Baxter, B. Braun, CSL Behring, Covidien, Fresenius Kabi, GSK, Lilly, Maquet, MSD, Novartis, Nycomed, Orion Pharma, Pfizer, Pierre Fabre Pharma AG (formerly known as RobaPharm). The Department of Intensive Care Medicine has received unrestricted educational grants from the following organizations for organizing bi-annual postgraduate courses in the fields of critical care ultrasound, management of ECMO and mechanical ventilation: Abbott AG, Anandic Medical Systems, Bard Medica SA., Bracco, Dräger Schweiz AG, Edwards Lifesciences AG, Fresenius Kabi (Schweiz) AG, Getinge Group Maquet AG, Hamilton Medical AG, Pierre Fabre Pharma AG (formerly known as RobaPharm), PanGas AG Healthcare, Pfizer AG, Orion Pharma, Teleflex Medical GmbH.
The remaining authors declare that the research was conducted in the absence of any commercial or financial relationships that could be construed as a potential conflict of interest.
Publisher’s note
All claims expressed in this article are solely those of the authors and do not necessarily represent those of their affiliated organizations, or those of the publisher, the editors and the reviewers. Any product that may be evaluated in this article, or claim that may be made by its manufacturer, is not guaranteed or endorsed by the publisher.
References
Argaiz, E. R., Rola, P., Haycock, K. H., and Verbrugge, F. H. (2022). Fluid management in acute kidney injury: From evaluating fluid responsiveness towards assessment of fluid tolerance. Eur. Heart J. Acute Cardiovasc Care 11 (10), 786–793. doi:10.1093/ehjacc/zuac104
Aya, H. D., Rhodes, A., Chis Ster, I., Fletcher, N., Grounds, R. M., and Cecconi, M. (2017). Hemodynamic effect of different doses of fluids for a fluid challenge: A quasi-randomized controlled study. Crit. Care Med. 45 (2), e161–e168. doi:10.1097/CCM.0000000000002067
Bachmann, K. F., Zwicker, L., Nettelbeck, K., Casoni, D., Heinisch, P. P., Jenni, H., et al. (2020). Assessment of right heart function during Extracorporeal therapy by Modified thermodilution in a porcine model. Anesthesiology 133 (4), 879–891. doi:10.1097/ALN.0000000000003443
Berger, D., Bloechlinger, S., Takala, J., Sinderby, C., and Brander, L. (2014). Heart-lung interactions during neurally adjusted ventilatory assist. Crit. Care 18 (5), 499. doi:10.1186/s13054-014-0499-8
Berger, D., Hobi, J., Moller, P. W., Haenggi, M., Takala, J., and Jakob, S. M. (2020). Right ventricular stroke volume assessed by pulmonary artery pulse contour analysis. Intensive Care Med. Exp. 8 (1), 58. doi:10.1186/s40635-020-00347-7
Berger, D., Moller, P. W., and Takala, J. (2019). Reply to "Is the Guytonian framework justified in explaining heart lung interactions?" and "Venous return, mean systemic pressure and getting the right answer for the wrong reason. Ann. Transl. Med. 7 (8), 186. doi:10.21037/atm.2019.04.50
Berger, D., Moller, P. W., and Takala, J. (2016). Reply to "Letter to the editor: Why persist in the fallacy that mean systemic pressure drives venous return? Am. J. Physiol. Heart Circ. Physiol. 311 (5), H1336-H1337. doi:10.1152/ajpheart.00622.2016
Berger, D., Moller, P. W., Weber, A., Bloch, A., Bloechlinger, S., Haenggi, M., et al. (2016). Effect of PEEP, blood volume, and inspiratory hold maneuvers on venous return. Am. J. Physiol. Heart Circ. Physiol. 311 (3), H794–H806. doi:10.1152/ajpheart.00931.2015
Berger, D., Wigger, O., Bachmann, K. F., and Bloechlinger, S. (2022). Reply to La via and colleagues. Clin. Res. Cardiol. 112, 702. doi:10.1007/s00392-022-02062-7
Berger, D., Wigger, O., de Marchi, S., Grubler, M. R., Bloch, A., Kurmann, R., et al. (2022). The effects of positive end-expiratory pressure on cardiac function: A comparative echocardiography-conductance catheter study. Clin. Res. Cardiol. 111 (6), 705–719. doi:10.1007/s00392-022-02014-1
Bloch, A., Berger, D., and Takala, J. (2016). Understanding circulatory failure in sepsis. Intensive Care Med. 42 (12), 2077–2079. doi:10.1007/s00134-016-4514-1
Bootsma, I. T., Boerma, E. C., Scheeren, T. W. L., and de Lange, F. (2022). The contemporary pulmonary artery catheter. Part 2: Measurements, limitations, and clinical applications. J. Clin. Monit. Comput. 36 (1), 17–31. doi:10.1007/s10877-021-00673-5
Buda, A. J., Pinsky, M. R., Ingels, N. B., Daughters, G. T., Stinson, E. B., and Alderman, E. L. (1979). Effect of intrathoracic pressure on left ventricular performance. N. Engl. J. Med. 301 (9), 453–459. doi:10.1056/NEJM197908303010901
Bull, T. M., Clark, B., McFann, K., and Moss, M. (2010). Pulmonary vascular dysfunction is associated with poor outcomes in patients with acute lung injury. Am. J. Respir. Crit. Care Med. 182 (9), 1123–1128. doi:10.1164/rccm.201002-0250OC
Cecconi, M., Aya, H. D., Geisen, M., Ebm, C., Fletcher, N., Grounds, R. M., et al. (2013). Changes in the mean systemic filling pressure during a fluid challenge in postsurgical intensive care patients. Intensive Care Med. 39 (7), 1299–1305. doi:10.1007/s00134-013-2928-6
Cecconi, M., De Backer, D., Antonelli, M., Beale, R., Bakker, J., Hofer, C., et al. (2014). Consensus on circulatory shock and hemodynamic monitoring. Task force of the European Society of Intensive Care Medicine. Intensive Care Med. 40 (12), 1795–1815. doi:10.1007/s00134-014-3525-z
Cecconi, M., Hofer, C., Teboul, J. L., Pettila, V., Wilkman, E., Molnar, Z., et al. (2015). Fluid challenges in intensive care: The FENICE study: A global inception cohort study. Intensive Care Med. 41 (9), 1529–1537. doi:10.1007/s00134-015-3850-x
Daudel, F., Tuller, D., Krahenbuhl, S., Jakob, S. M., and Takala, J. (2010). Pulse pressure variation and volume responsiveness during acutely increased pulmonary artery pressure: An experimental study. Crit. Care 14 (3), R122. doi:10.1186/cc9080
De Backer, D., Heenen, S., Piagnerelli, M., Koch, M., and Vincent, J. L. (2005). Pulse pressure variations to predict fluid responsiveness: Influence of tidal volume. Intensive Care Med. 31 (4), 517–523. doi:10.1007/s00134-005-2586-4
De Backer, D., Taccone, F. S., Holsten, R., Ibrahimi, F., and Vincent, J. L. (2009). Influence of respiratory rate on stroke volume variation in mechanically ventilated patients. Anesthesiology 110 (5), 1092–1097. doi:10.1097/ALN.0b013e31819db2a1
de Waal, E. E., Rex, S., Kruitwagen, C. L., Kalkman, C. J., and Buhre, W. F. (2009). Dynamic preload indicators fail to predict fluid responsiveness in open-chest conditions. Crit. Care Med. 37 (2), 510–515. doi:10.1097/CCM.0b013e3181958bf7
Fisher, J. P., Zera, T., and Paton, J. F. R. (2022). Respiratory-cardiovascular interactions. Handb. Clin. Neurol. 188, 279–308. doi:10.1016/B978-0-323-91534-2.00006-0
Grubler, M. R., Wigger, O., Berger, D., and Blochlinger, S. (2017). Basic concepts of heart-lung interactions during mechanical ventilation. Swiss Med. Wkly. 147, w14491. doi:10.4414/smw.2017.14491
Gupta, K., Sondergaard, S., Parkin, G., Leaning, M., and Aneman, A. (2015). Applying mean systemic filling pressure to assess the response to fluid boluses in cardiac post-surgical patients. Intensive Care Med. 41 (2), 265–272. doi:10.1007/s00134-014-3611-2
Guyton, A. C., Lindsey, A. W., Abernathy, B., and Richardson, T. (1957). Venous return at various right atrial pressures and the normal venous return curve. Am. J. Physiol. 189 (3), 609–615. doi:10.1152/ajplegacy.1957.189.3.609
Hartert, T. V., Wheeler, A. P., and Sheller, J. R. (1999). Use of pulse oximetry to recognize severity of airflow obstruction in obstructive airway disease: Correlation with pulsus paradoxus. Chest 115 (2), 475–481. doi:10.1378/chest.115.2.475
Hartog, E. A., Jansen, J. R., Moens, G. H., and Versprille, A. (1996). Systemic filling pressure in the intact circulation determined with a slow inflation procedure. Pflugers Arch. 431 (6), 863–867. doi:10.1007/s004240050078
Jardin, F., Brun-Ney, D., Cazaux, P., Dubourg, O., Hardy, A., and Bourdarias, J. P. (1989). Relation between transpulmonary pressure and right ventricular isovolumetric pressure change during respiratory support. Cathet Cardiovasc Diagn 16 (4), 215–220. doi:10.1002/ccd.1810160402
Jardin, F., Dubourg, O., Margairaz, A., and Bourdarias, J. P. (1987). Inspiratory impairment in right ventricular performance during acute asthma. Chest 92 (5), 789–795. doi:10.1378/chest.92.5.789
Jardin, F. (2004). Cyclic changes in arterial pressure during mechanical ventilation. Intensive Care Med. 30 (6), 1047–1050. doi:10.1007/s00134-004-2254-0
Juhl-Olsen, P., Frederiksen, C. A., Hermansen, J. F., Jakobsen, C. J., and Sloth, E. (2012). Echocardiographic measures of diastolic function are preload dependent during Triggered positive pressure ventilation: A controlled crossover study in healthy subjects. Crit. Care Res. Pract. 2012, 703196. doi:10.1155/2012/703196
Juhl-Olsen, P., Hermansen, J. F., Frederiksen, C. A., Rasmussen, L. A., Jakobsen, C. J., and Sloth, E. (2013). Positive end-expiratory pressure influences echocardiographic measures of diastolic function: A randomized, crossover study in cardiac surgery patients. Anesthesiology 119 (5), 1078–1086. doi:10.1097/ALN.0b013e3182a10b40
Lansdorp, B., Hofhuizen, C., van Lavieren, M., van Swieten, H., Lemson, J., van Putten, M. J., et al. (2014). Mechanical ventilation-induced intrathoracic pressure distribution and heart-lung interactions. Crit. Care Med. 42 (9), 1983–1990. doi:10.1097/CCM.0000000000000345
Lim, H. S., and Gustafsson, F. (2020). Pulmonary artery pulsatility index: Physiological basis and clinical application. Eur. J. Heart Fail. 22 (1), 32–38. doi:10.1002/ejhf.1679
Longino, A., Martin, K., Leyba, K., Siegel, G., Gill, E., Douglas, I. S., et al. (2023). Correlation between the VExUS score and right atrial pressure: A pilot prospective observational study. Crit. Care 27 (1), 205. doi:10.1186/s13054-023-04471-0
Maas, J. J., Geerts, B. F., and Jansen, J. R. (2011). Evaluation of mean systemic filling pressure from pulse contour cardiac output and central venous pressure. J. Clin. Monit. Comput. 25 (3), 193–201. doi:10.1007/s10877-011-9294-0
Maas, J. J., Geerts, B. F., van den Berg, P. C., Pinsky, M. R., and Jansen, J. R. (2009). Assessment of venous return curve and mean systemic filling pressure in postoperative cardiac surgery patients. Crit. Care Med. 37 (3), 912–918. doi:10.1097/CCM.0b013e3181961481
Maas, J. J., Pinsky, M. R., Aarts, L. P., and Jansen, J. R. (2012). Bedside assessment of total systemic vascular compliance, stressed volume, and cardiac function curves in intensive care unit patients. Anesth. Analg. 115 (4), 880–887. doi:10.1213/ANE.0b013e31825fb01d
Magder, S. (2004). Clinical usefulness of respiratory variations in arterial pressure. Am. J. Respir. Crit. Care Med. 169 (2), 151–155. doi:10.1164/rccm.200211-1360CC
Magder, S., and De Varennes, B. (1998). Clinical death and the measurement of stressed vascular volume. Crit. Care Med. 26 (6), 1061–1064. doi:10.1097/00003246-199806000-00028
Magder, S. (2010). Further cautions for the use of ventilatory-induced changes in arterial pressures to predict volume responsiveness. Crit. Care 14 (5), 197. doi:10.1186/cc9223
Magder, S., Georgiadis, G., and Cheong, T. (1992). Respiratory variations in right atrial pressure predict the response to fluid challenge. J. Crit. care 7 (2), 76–85. doi:10.1016/0883-9441(92)90032-3
Magder, S. (2017). Right atrial pressure in the critically ill: How to measure, what is the value, what are the limitations? Chest 151 (4), 908–916. doi:10.1016/j.chest.2016.10.026
Magder, S. (2007). The left heart can only be as good as the right heart: Determinants of function and dysfunction of the right ventricle. Crit. Care Resusc. 9 (4), 344–351. doi:10.3316/informit.515301487949676
Magder, S. (2016). Volume and its relationship to cardiac output and venous return. Crit. Care 20, 271. doi:10.1186/s13054-016-1438-7
Marantz, P. R., Kaplan, M. C., and Alderman, Ml (1990). Clinical diagnosis of congestive heart failure in patients with acute dyspnea. Chest 97 (4), 776–781. doi:10.1378/chest.97.4.776
Marik, P. E., and Cavallazzi, R. (2013). Does the central venous pressure predict fluid responsiveness? An updated meta-analysis and a plea for some common sense. Crit. Care Med. 41 (7), 1774–1781. doi:10.1097/CCM.0b013e31828a25fd
Mesquida, J., Kim, H. K., and Pinsky, M. R. (2011). Effect of tidal volume, intrathoracic pressure, and cardiac contractility on variations in pulse pressure, stroke volume, and intrathoracic blood volume. Intensive Care Med. 37 (10), 1672–1679. doi:10.1007/s00134-011-2304-3
Michard, F., Boussat, S., Chemla, D., Anguel, N., Mercat, A., Lecarpentier, Y., et al. (2000). Relation between respiratory changes in arterial pulse pressure and fluid responsiveness in septic patients with acute circulatory failure. Am. J. Respir. Crit. Care Med. 162 (1), 134–138. doi:10.1164/ajrccm.162.1.9903035
Moller, P. W., and Berger, D. C. (2023). Commentary: Feasibility to estimate mean systemic filling pressure with inspiratory holds at the bedside. Front. Physiol. 14, 1135769. doi:10.3389/fphys.2023.1135769
Moller, P. W., Hana, A., Heinisch, P. P., Liu, S., Djafarzadeh, S., Haenggi, M., et al. (2019). The effects of vasoconstriction and volume expansion on veno-arterial ECMO flow. Shock 51 (5), 650–658. doi:10.1097/SHK.0000000000001197
Moller, P. W., Winkler, B., Hurni, S., Heinisch, P. P., Bloch, A., Sondergaard, S., et al. (2017). Right atrial pressure and venous return during cardiopulmonary bypass. Am. J. Physiol. Heart Circ. Physiol. 313 (2), H408–H20. doi:10.1152/ajpheart.00081.2017
Monchi, M., Bellenfant, F., Cariou, A., Joly, L. M., Thebert, D., Laurent, I., et al. (1998). Early predictive factors of survival in the acute respiratory distress syndrome. Amultivariate analysis. Am. J. Respir. Crit. Care Med. 158, 1076–1081. doi:10.1164/ajrccm.158.4.9802009
Monnet, X., Osman, D., Ridel, C., Lamia, B., Richard, C., and Teboul, J. L. (2009). Predicting volume responsiveness by using the end-expiratory occlusion in mechanically ventilated intensive care unit patients. Crit. care Med. 37 (3), 951–956. doi:10.1097/CCM.0b013e3181968fe1
Morgan, B. C., Martin, W. E., Hornbein, T. F., Crawford, E. W., and Guntheroth, W. G. (1966). Hemodynamic effects of intermittent positive pressure respiration. Anesthesiology 27 (5), 584–590. doi:10.1097/00000542-196609000-00009
Muller, L., Louart, G., Bousquet, P. J., Candela, D., Zoric, L., de La Coussaye, J. E., et al. (2010). The influence of the airway driving pressure on pulsed pressure variation as a predictor of fluid responsiveness. Intensive Care Med. 36 (3), 496–503. doi:10.1007/s00134-009-1686-y
Osman, D., Monnet, X., Castelain, V., Anguel, N., Warszawski, J., Teboul, J. L., et al. (2009). Incidence and prognostic value of right ventricular failure in acute respiratory distress syndrome. Intensive Care Med. 35 (1), 69–76. doi:10.1007/s00134-008-1307-1
Osman, D., Ridel, C., Ray, P., Monnet, X., Anguel, N., Richard, C., et al. (2007). Cardiac filling pressures are not appropriate to predict hemodynamic response to volume challenge. Crit. Care Med. 35 (1), 64–68. doi:10.1097/01.CCM.0000249851.94101.4F
Parker, M. M., Pinsky, M. R., Takala, J., and Vincent, J-L. (2023). The story of the pulmonary artery catheter: Five decades in critical care medicine. Crit. Care Med. 51 (2), 159–163. doi:10.1097/CCM.0000000000005718
Parkin, G., Wright, C., Bellomo, R., and Boyce, N. (1994). Use of a mean systemic filling pressure analogue during the closed-loop control of fluid replacement in continuous hemodiafiltration. J. Crit. Care 9 (2), 124–133. doi:10.1016/0883-9441(94)90023-x
Parkin, W. G., and Leaning, M. S. (2008). Therapeutic control of the circulation. J. Clin. Monit. Comput. 22 (6), 391–400. doi:10.1007/s10877-008-9147-7
Parkin, W. G., and Wright, C. A. (1991). Three dimensional closed loop control of the human circulation. Int. J. Clin. Monit. Comput. 8 (1), 35–42. doi:10.1007/BF02916090
Perel, A., Pizov, R., and Cotev, S. (1987). Systolic blood pressure variation is a sensitive indicator of hypovolemia in ventilated dogs subjected to graded hemorrhage. Anesthesiology 67 (4), 498–502. doi:10.1097/00000542-198710000-00009
Persichini, R., Silva, S., Teboul, J. L., Jozwiak, M., Chemla, D., Richard, C., et al. (2012). Effects of norepinephrine on mean systemic pressure and venous return in human septic shock. Crit. Care Med. 40 (12), 3146–3153. doi:10.1097/CCM.0b013e318260c6c3
Pinsky, M. R., Desmet, J. M., and Vincent, J. L. (1992). Effect of positive end-expiratory pressure on right ventricular function in humans. Fam. Rev. Respir. Dis. 146 (3), 681–687. doi:10.1164/ajrccm/146.3.681
Pinsky, M. R. (2014). Functional haemodynamic monitoring. Curr. Opin. Crit. Care 20 (3), 288–293. doi:10.1097/MCC.0000000000000090
Pinsky, M. R. (2014). My paper 20 years later: Effect of positive end-expiratory pressure on right ventricular function in humans. Intensive Care Med. 40 (7), 935–941. doi:10.1007/s00134-014-3294-8
Repesse, X., Charron, C., Fink, J., Beauchet, A., Deleu, F., Slama, M., et al. (2015). Value and determinants of the mean systemic filling pressure in critically ill patients. Am. J. Physiol. Heart Circ. Physiol. 309 (5), H1003–H1007. doi:10.1152/ajpheart.00413.2015
Repesse, X., Charron, C., Geri, G., Aubry, A., Paternot, A., Maizel, J., et al. (2017). Impact of positive pressure ventilation on mean systemic filling pressure in critically ill patients after death. J. Appl. Physiol. 122 (6), 1373–1378. doi:10.1152/japplphysiol.00958.2016
Repessé, X., Charron, C., and Vieillard-Baron, A. (2016). Assessment of the effects of inspiratory load on right ventricular function. Curr. Opin. Crit. Care 22 (3), 254–259. doi:10.1097/MCC.0000000000000303
Reuter, D. A., Felbinger, T. W., Schmidt, C., Kilger, E., Goedje, O., Lamm, P., et al. (2002). Stroke volume variations for assessment of cardiac responsiveness to volume loading in mechanically ventilated patients after cardiac surgery. Intensive Care Med. 28 (4), 392–398. doi:10.1007/s00134-002-1211-z
Reuter, D. A., Huang, C., Edrich, T., Shernan, S. K., and Eltzschig, H. K. (2010). Cardiac output monitoring using indicator-dilution techniques: Basics, limits, and perspectives. Anesth. Analg. 110 (3), 799–811. doi:10.1213/ANE.0b013e3181cc885a
Robotham, J. L., Cherry, D., Mitzner, W., Rabson, J. L., Lixfeld, W., and Bromberger-Barnea, B. (1983). A re-evaluation of the hemodynamic consequences of intermittent positive pressure ventilation. Crit. Care Med. 11 (10), 783–793. doi:10.1097/00003246-198310000-00005
Sarkar, M., Bhardwaj, R., Madabhavi, I., Gowda, S., and Dogra, K. (2018). Pulsus paradoxus. Clin. Respir. J. 12 (8), 2321–2331. doi:10.1111/crj.12912
Schmitt, J. M., Vieillard-Baron, A., Augarde, R., Prin, S., Page, B., and Jardin, F. (2001). Positive end-expiratory pressure titration in acute respiratory distress syndrome patients: Impact on right ventricular outflow impedance evaluated by pulmonary artery Doppler flow velocity measurements. Crit. Care Med. 29 (6), 1154–1158. doi:10.1097/00003246-200106000-00012
Sette, P., Dorizzi, R. M., and Azzini, A. M. (2012). Vascular access: An historical perspective from Sir william Harvey to the 1956 Nobel prize to André F. Cournand, werner forssmann, and dickinson W. Richards. J. Vasc. Access 13 (2), 137–144. doi:10.5301/jva.5000018
Slobod, D., Assanangkornchai, N., Alhazza, M., Mettasittigorn, P., and Magder, S. (2022). Right ventricular loading by lung inflation during controlled mechanical ventilation. Am. J. Respir. Crit. Care Med. 205 (11), 1311–1319. doi:10.1164/rccm.202111-2483OC
Sondergaard, S., Parkin, G., and Aneman, A. (2016). Central venous pressure: Soon an outcome-associated matter. Curr. Opin. Anaesthesiol. 29 (2), 179–185. doi:10.1097/ACO.0000000000000305
Sondergaard, S. (2013). Pavane for a pulse pressure variation defunct. Crit. Care 17 (6), 327. doi:10.1186/cc13109
Starling, E. (1918). The linacre lecture on the law of the heart. London, UK: Longmans Green, and Company.
Takala, J. (2007). Hypoxemia due to increased venous admixture: Influence of cardiac output on oxygenation. Intensive Care Med. 33 (5), 908–911. doi:10.1007/s00134-007-0546-x
Takala, J. (2016). Volume responsive, but does the patient need volume? Intensive Care Med. 42 (9), 1461–1463. doi:10.1007/s00134-015-4172-8
Valenti, E., Moller, P. W., Takala, J., and Berger, D. (2021). Collapsibility of caval vessels and right ventricular afterload: Decoupling of stroke volume variation from preload during mechanical ventilation. J. Appl. Physiol. 130 (5), 1562–1572. doi:10.1152/japplphysiol.01039.2020
van den Berg, P. C., Jansen, J. R., and Pinsky, M. R. (2002). Effect of positive pressure on venous return in volume-loaded cardiac surgical patients. J. Appl. Physiol. 92 (3), 1223–1231. doi:10.1152/japplphysiol.00487.2001
Versprille, A., and Jansen, J. R. (1985). Mean systemic filling pressure as a characteristic pressure for venous return. Pflugers Arch. 405 (3), 226–233. doi:10.1007/BF00582565
Vieillard-Baron, A., Chergui, K., Augarde, R., Prin, S., Page, B., Beauchet, A., et al. (2003). Cyclic changes in arterial pulse during respiratory support revisited by Doppler echocardiography. Am. J. Respir. Crit. Care Med. 168 (6), 671–676. doi:10.1164/rccm.200301-135OC
Vieillard-Baron, A., Chergui, K., Rabiller, A., Peyrouset, O., Page, B., Beauchet, A., et al. (2004). Superior vena caval collapsibility as a gauge of volume status in ventilated septic patients. Intensive Care Med. 30 (9), 1734–1739. doi:10.1007/s00134-004-2361-y
Vieillard-Baron, A., Evrard, B., Repesse, X., Maizel, J., Jacob, C., Goudelin, M., et al. (2018). Limited value of end-expiratory inferior vena cava diameter to predict fluid responsiveness impact of intra-abdominal pressure. Intensive Care Med. 44 (2), 197–203. doi:10.1007/s00134-018-5067-2
Vieillard-Baron, A., and Jardin, F. (2003). Why protect the right ventricle in patients with acute respiratory distress syndrome? Curr. Opin. Crit. Care 9 (1), 15–21. doi:10.1097/00075198-200302000-00004
Vieillard-Baron, A., Loubieres, Y., Schmitt, J. M., Page, B., Dubourg, O., and Jardin, F. (1999). Cyclic changes in right ventricular output impedance during mechanical ventilation. J. Appl. Physiol. 87 (5), 1644–1650. doi:10.1152/jappl.1999.87.5.1644
Vieillard-Baron, A., Matthay, M., Teboul, J. L., Bein, T., Schultz, M., Magder, S., et al. (2016). Experts' opinion on management of hemodynamics in ARDS patients: Focus on the effects of mechanical ventilation. Intensive Care Med. 42 (5), 739–749. doi:10.1007/s00134-016-4326-3
Vieillard-Baron, A., Millington, S. J., Sanfilippo, F., Chew, M., Diaz-Gomez, J., McLean, A., et al. (2019). A decade of progress in critical care echocardiography: A narrative review. Intensive Care Med. 45 (6), 770–788. doi:10.1007/s00134-019-05604-2
Vieillard-Baron, A., Naeije, R., Haddad, F., Bogaard, H. J., Bull, T. M., Fletcher, N., et al. (2018). Diagnostic workup, etiologies and management of acute right ventricle failure: A state-of-the-art paper. Intensive Care Med. 44 (6), 774–790. doi:10.1007/s00134-018-5172-2
Vieillard-Baron, A., Naeije, R., Haddad, F., Bogaard, H. J., Bull, T. M., Fletcher, N., et al. (2018). Diagnostic workup, etiologies and management of acute right ventricle failure: A state-of-the-art paper. Intensive Care Med. 44 (6), 774–790. doi:10.1007/s00134-018-5172-2
Vieillard-Baron, A., Prigent, A., Repesse, X., Goudelin, M., Prat, G., Evrard, B., et al. (2020). Right ventricular failure in septic shock: Characterization, incidence and impact on fluid responsiveness. Crit. Care 24 (1), 630. doi:10.1186/s13054-020-03345-z
Vieillard-Baron, A., Repesse, X., and Charron, C. (2015). Heart-lung interactions: Have a look on the superior vena cava and on the changes in right ventricular afterload. Crit. Care Med. 43 (2), e52. doi:10.1097/CCM.0000000000000740
Vignon, P., AitHssain, A., Francois, B., Preux, P. M., Pichon, N., Clavel, M., et al. (2008). Echocardiographic assessment of pulmonary artery occlusion pressure in ventilated patients: A transoesophageal study. Crit. Care 12 (1), R18. doi:10.1186/cc6792
Vignon, P., Allot, V., Lesage, J., Martaille, J. F., Aldigier, J. C., Francois, B., et al. (2007). Diagnosis of left ventricular diastolic dysfunction in the setting of acute changes in loading conditions. Crit. care (London, Engl. 11 (2), R43. doi:10.1186/cc5736
Vignon, P., Repessé, X., Bégot, E., Léger, J., Jacob, C., Bouferrache, K., et al. (2017). Comparison of echocardiographic indices used to predict fluid responsiveness in ventilated patients. Am. J. Respir. Crit. Care Med. 195 (8), 1022–1032. doi:10.1164/rccm.201604-0844OC
Wang, X., Liu, S., Gao, J., Zhang, Y., and Huang, T. (2023). Does tidal volume challenge improve the feasibility of pulse pressure variation in patients mechanically ventilated at low tidal volumes? A systematic review and meta-analysis. Crit. Care 27 (1), 45. doi:10.1186/s13054-023-04336-6
Werner-Moller, P., Heinisch, P. P., Hana, A., Bachmann, K. F., Sondergaard, S., Jakob, S. M., et al. (2022). Experimental validation of a mean systemic pressure analog against zero-flow measurements in porcine VA-ECMO. J. Appl. Physiol. 132 (3), 726–736. doi:10.1152/japplphysiol.00804.2021
Werner-Moller, P., Sondergaard, S., Jakob, S. M., Takala, J., and Berger, D. (2019). Effect of volume status on the estimation of mean systemic filling pressure. J. Appl. Physiol. 126 (6), 1503–1513. doi:10.1152/japplphysiol.00897.2018
Wijnberge, M., Jansen, J. R. C., Pinsky, M. R., Klanderman, R. B., Terwindt, L. E., Bosboom, J. J., et al. (2022). Feasibility to estimate mean systemic filling pressure with inspiratory holds at the bedside. Front. Physiology 13, 1041730. doi:10.3389/fphys.2022.1041730
Wyler von Ballmoos, M., Takala, J., Roeck, M., Porta, F., Tueller, D., Ganter, C. C., et al. (2010). Pulse-pressure variation and hemodynamic response in patients with elevated pulmonary artery pressure: A clinical study. Crit. Care 14 (3), R111. doi:10.1186/cc9060
Keywords: heart-lung interactions, volume responsiveness, monitoring, right ventricular failure, ECMO - extracorporeal membrane oxygenation
Citation: Berger D, Werner Moller P and Bachmann KF (2023) Cardiopulmonary interactions—which monitoring tools to use?. Front. Physiol. 14:1234915. doi: 10.3389/fphys.2023.1234915
Received: 05 June 2023; Accepted: 18 July 2023;
Published: 09 August 2023.
Edited by:
Christina Maria Pabelick, Mayo Clinic, United StatesReviewed by:
Ivón Johanna Rodríguez, National University of Colombia, ColombiaAndrás Lovas, Kiskunhalas Semmelweis Hospital, Hungary
Copyright © 2023 Berger, Werner Moller and Bachmann. This is an open-access article distributed under the terms of the Creative Commons Attribution License (CC BY). The use, distribution or reproduction in other forums is permitted, provided the original author(s) and the copyright owner(s) are credited and that the original publication in this journal is cited, in accordance with accepted academic practice. No use, distribution or reproduction is permitted which does not comply with these terms.
*Correspondence: David Berger, David.Berger@insel.ch