- 1Department of Physiology and Pharmacology, University of Georgia, Athens, GA, United States
- 2Regenerative Biosciences Center, University of Georgia, Athens, GA, United States
- 3School of Kinesiology, University of Minnesota, Minneapolis, MN, United States
Volumetric muscle loss (VML) injuries are characterized by non-recoverable loss of tissue resulting in contractile and metabolic dysfunction. The characterization of metabolic dysfunction in volumetric muscle loss-injured muscle has been interpreted from permeabilized myofiber respiration experiments involving saturating ADP levels and non-physiologic ATP:ADP concentration ratios. The extent to which this testing condition obscures the analysis of mitochondrial (dys) function after volumetric muscle loss injury is unclear. An alternative approach is described that leverages the enzymatic reaction of creatine kinase and phosphocreatine to assess mitochondrial respiration and membrane potential at clamped physiologic ATP:ADP ratios, “CK Clamp.” The objective of this study was to validate the CK Clamp in volumetric muscle loss-injured muscle and to detect differences that may exist between volumetric muscle loss-injured and uninjured muscles at 1, 3, 5, 7, 10, and 14 days post-injury. Volumetric muscle loss-injured muscle maintains bioenergetic features of the CK Clamp approach, i.e., mitochondrial respiration rate (JO2) titters down and mitochondrial membrane potential is more polarized with increasing ATP:ADP ratios. Pyruvate/malate/succinate-supported JO2 was significantly less in volumetric muscle loss-injured muscle at all timepoints compared to uninjured controls (−26% to −84%, p < 0.001) and electron conductance was less at day 1 (−60%), 5 (−52%), 7 (−35%), 10 (−59%), and 14 (−41%) (p < 0.001). Palmitoyl-carnitine/malate-supported JO2 and electron conductance were less affected following volumetric muscle loss injury. volumetric muscle loss-injury also corresponded with a more polarized mitochondrial membrane potential across the clamped ATP:ADP ratios at day 1 and 10 (pyruvate and palmitoyl-carnitine, respectively) (+5%, p < 0.001). This study supports previous characterizations of metabolic dysfunction and validates the CK Clamp as a tool to investigate bioenergetics in traumatically-injured muscle.
Highlights
• This study validates the CK Clamp technique, an approach to assess mitochondrial respiration and membrane potential at physiological ATP:ADP concentration ratios, as a tool to investigate mitochondrial bioenergetics in VML-injured muscle
• This study demonstrates a relatively greater deficit in carbohydrate-supported metabolism compared to fat-supported metabolism during early VML pathology
• The findings from this study utilizing the CK Clamp technique support previous reports of VML-injured muscle metabolic dysfunction that involved supra-physiological ATP:ADP ratio experimental conditions
Introduction
Volumetric muscle loss (VML) injuries encompass a group of pathologies characterized by the frank loss of muscle tissue due to trauma or surgical removal (Grogan et al., 2011). The result of these injuries is the marked, sustained decline of muscle strength (Grogan et al., 2011; Corona et al., 2015; Greising et al., 2017), mobility, and greater fibrotic tissue deposition (Corona et al., 2015; Garg et al., 2015; Chao et al., 2019; Southern et al., 2019; Dalske et al., 2021). VML pathology is also associated with changes in skeletal muscle and whole-body metabolism. For example, following VML injury, there is less mitochondrial respiration in permeabilized myofibers (Greising et al., 2018; Southern et al., 2019; McFaline-Figueroa et al., 2022), less mitochondrial enzyme activities (Aurora et al., 2014), less activation of PGC1α (genetic marker of mitochondrial biogenesis) (Aurora et al., 2014; Southern et al., 2019), and changes in whole-body diurnal metabolism, resulting in lower oxidation of carbohydrates (Dalske et al., 2021; Raymond-Pope et al., 2022).
A particular limitation of the existing mitochondrial respiration studies in the context of VML-injured myofibers is that the experiments were conducted under non-physiological testing conditions (i.e., supra-physiological levels of ADP) that may exaggerate the findings and interpretations. To directly address this limitation, the current study was designed to validate a physiological stress test (Glancy et al., 2008; Fisher-Wellman et al., 2018) that assesses mitochondrial oxygen consumption and mitochondria membrane potential at specific ATP re-synthesis demand states in the context of a VML injury. To determine if fuel metabolism at the tissue-level mirrored whole-body changes in carbohydrate and fat oxidations after VML injury (Dalske et al., 2021), VML-injured myofibers ability to utilize carbohydrates and fat substrates during the physiological stress test was assessed. This study focused on the first 2 weeks of pathology following VML injury when there are documented changes to the mitochondrial network (Southern et al., 2019).
Materials and methods
Animals
Male C57BL/6 mice (n = 42) were housed at 20°C–23°C on a 12:12-h light-dark cycle, with food and water provided ad libitum. At the time of randomization to experimental groups, all mice were between 11 and 12 weeks of age. All procedures were approved and performed in accordance with the guidelines and regulations of the Institutional Animal Care and Use Committee at the University of Georgia.
Experimental design
VML-injured mice underwent unilateral VML injury and were sacrificed at the following timepoints post-injury (n = 6–8/group): 1, 3, 5, 7, 10, and 14-days. At the study endpoint, muscle mass, carbohydrate and fat-supported oxygen consumption and mitochondrial membrane potential were assessed. Sample size was determined based on the coefficient of variance for permeabilized muscle fiber respiration (∼15%), a capacity to detect at minimum 15% changes across groups, and a Power of 0.8 (calculated a priori in JMP®, Version 16.0. SAS Institute Inc., Cary, NC).
Volumetric muscle loss injury
Unilateral VML injury to the left hindlimb plantar flexors (gastrocnemius, soleus, plantaris) provided volumetric removal of muscle tissue from anesthetized (isoflurane 1.5%–3.0%) mice, as previously described (Greising et al., 2018; Southern et al., 2019). Mice were administered buprenorphine (1.2 mg/kg; s. q.) prior to a single incision being made in the mid-gastrocnemius from distal to proximal exposing posterior compartment muscles. Using a 4 mm standardized biopsy punch of the posterior muscles resulted in a removal of ∼15% (27.2 ± 1.2 mg) of muscle tissue. Any bleeding was stopped with light pressure. Skin incisions were closed with 6.0 silk sutures and mice were monitored through recovery.
CK Clamp mitochondrial respiration
Permeabilized myofiber high-resolution respirometry was performed using an Oroboros Oxygraph-2K (Oroboros Instruments, Innsburck, Austria). Experiments were performed at 30°C in Buffer Z supplemented with ATP (5 mM), creatine (5 mM), phosphocreatine (PCr, 1 mM) and creatine kinase (CK, 20 U/mL). Higher experimental temperatures (e.g., 37°C) are associated with greater respiration rates than can deplete chamber oxygen levels. Re-oxygenation of chambers can be monitored within the Oxygraph-2K chambers, but not in the device used for parallel mitochondrial membrane potential detail below. Therefore, 30°C was selected to maintain an appropriate oxygen concentration for the entirety of the experiment and be consistent between testing platforms. For each experiment, permeabilized skeletal myofiber bundles (∼2 mg) from the gastrocnemius muscles were energized with carbohydrates fuels (4 mM Pyruvate, 1 mM Malate, 20 mM Succinate) or fat fuels (4 mM Palmitoyl-carnitine, 1 mM Malate) and 5 mM ATP to energize the mitochondria. Mitochondrial oxygen consumption was determined using a CK Clamp technique where steady-state oxygen consumption rates are measured while manipulating the free energy of ATP hydrolysis (∆GATP), calculated through an online resource (https://dmpio.github.io/bioenergetic-calculators/ck_clamp/), as previously described (Fisher-Wellman et al., 2018). Sequential PCr titrations were made to final concentrations of 1, 2, 4, 7, 16, 31 mM. All high-resolution respirometry steady-state oxygen consumption data (JO2) were corrected for basal oxygen consumption and then normalized by citrate synthase (CS) activity, an indirect measure/estimate of mitochondrial content (Larsen et al., 2012).
Mitochondria membrane potential
Fluorescence determination of mitochondrial membrane potential was performed using permeabilized myofibers (∼2 mg) in parallel with high-resolution respirometry using a Horiba Spectrofluorometer (FluoroMax Plus-C; Horiba Instruments Inc., Atlanta, GE, United States) as previously described (Scaduto and Grotyohann, 1999; Fisher-Wellman et al., 2018). The membrane potential was determined via tetramethylrhodamine methyl ester (TMRM) at 30°C, which parallelly matched the protocol with the CK clamp assay with constant stirring. TMRM excitation/emission [(572/590 nm)/(551/590 nM)] fluorescence is quenched (i.e., 572/551 ratio increases) with greater mitochondrial membrane polarization. The 572/551 ratio is reported herein because there is currently no report of the 572/551 ratio conversion to millivolts validated in permeabilized muscle fiber bundles to our knowledge.
Citrate synthase activity
Mitochondrial content was analyzed by CS activity as previously described (Southern et al., 2019) using remaining muscle fiber collections for oxygen consumption experiments.
Statistical analysis
All data is represented as mean ± standard deviation. A one-way ANOVA was used to determine differences between uninjured controls and VML-injured myofibers across timepoints. Uninjured, contralateral control limbs from animals at all timepoints were not statistically different (one-way ANOVA, p ≥ 0.1078) and were, therefore, pooled together for analysis. All statistical analyses were performed using JMP statistical software, all graphs were constructed using Prism (GraphPad Prism version 9.4.1 for Windows, GraphPad Software, San Diego, California United States).
Results and discussion
In agreement with previous reports (Greising et al., 2018; McFaline-Figueroa et al., 2022), the posterior VML injury model resulted in a non-recoverable loss of muscle mass, even when accounting for differences in body mass (Table 1). Although contractile function was not assessed in this study, this model is associated with a ∼80% strength deficit at 3- and 7-days post-injury (Southern et al., 2019) that is largely sustained at 2-month post-injury [−36%, (McFaline-Figueroa et al., 2022)] compared to uninjured, age-matched controls.
Rationale for CK Clamp respirometry approach
Standardized mitochondrial oxygen consumption tests at supra-physiological concentrations of ADP can lead to misinterpretations of mitochondrial respiration because mitochondria rarely function in vivo at these extremely low ATP/ADP ratios (Schmidt et al., 2021). Furthermore, mitochondrial energy transduction involves a network of dehydrogenases influencing cellular redox status [e.g., NAD(P)H/NAD(P)], electron transport chain proton pumping and establishment of the proton motive force, and the dissipation of this proton motive force during ATP synthesis based on energy demand (i.e., ATP/ADP ratio) (Fisher-Wellman et al., 2018; Schmidt et al., 2021). A multi-dimensional diagnostic assessment is therefore appropriate to better assess mitochondrial function under physiological ranges of ATP/ADP ratios. The concept described by Glancy et al. (Glancy et al., 2013), then later optimized by Fisher-Wellman et al. (Fisher-Wellman et al., 2018; McLaughlin et al., 2020) and employed here, uses the enzymatic reaction of CK and PCr to stepwise “clamp” ATP/ADP ratios a different ATP free energies such that a high demand for ATP re-synthesis is −12.17 ∆GATP and a low demand for ATP re-synthesis is −14.37 ∆GATP (Figures 1A, B). When ADP phosphorylation (i.e., ATP re-synthesis) is strongly coupled to respiration (i.e., oxygen consumption), then JO2 is expected to be titrated down proportionately to a rise in ∆GATP. Herein, we simultaneously assessed JO2 and the proton motive force, or mitochondrial membrane potential (Figures 1C, D).
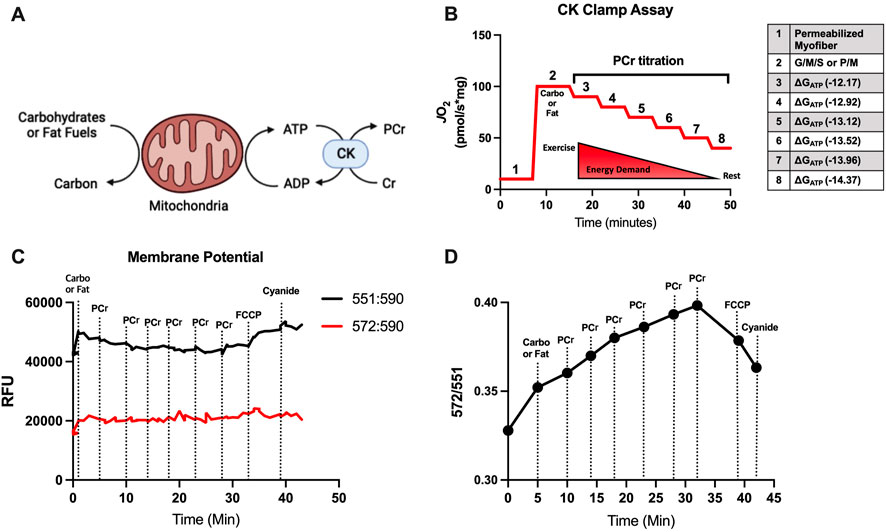
FIGURE 1. Conceptual framework for CK Clamp technique and mitochondrial membrane potential evaluation. (A) Creatine kinase (CK) is catalyzes the reversible reaction of creatine (Cr) and ATP to produce phosphocreatine (PCr) and ADP. (B) During the CK Clamp test, the ATP re-synthesis demand (∆GATP) can be manipulated by titrating PCr and has the effect of decreasing oxygen consumption (JO2). (C, D) A representative mitochondrial membrane test detecting the change in TMRM throughout the CK Clamp. The ratio of the 572-to-551 excitation/emission fluorescence is used to indirectly assess the polarization of the mitochondrial membrane. The reversing of mitochondrial membrane polarization was confirmed by sequential addition of an uncoupler (FCCP) and cyanide.
Validation of CK Clamp in VML-Injured myofibers
Independent of injury, JO2 diminished with more negative ∆GATP during the CK Clamp test (−35% with carbohydrate substrates, p < 0.001, Figure 2A; and −78% with fat substrates, p < 0.001; Figure 2E). This ∆GATP – JO2 relationship agrees with the theoretical and experimental relationship reported between ATP free energies and JO2 in other tissues (Curtis et al., 2005; U.S. Department of Health and Human Services and U.S. Department of Agriculture, 2015–2020; Southern et al., 2019). In general, a more negative ∆GATP also produced a greater polarized mitochondrial membrane independent of injury (+5% with carbohydrate substrates, p < 0.001, Figure 2B; and +7% with fat substrates, p < 0.001; Figure 2F). This JO2 – mitochondrial membrane potential relationship during the CK Clamp test agrees with similar reports in uninjured skeletal and cardiac muscle, brown adipose tissue, kidney, and liver using this technique (Ahima and Antwi, 2008; Iwasa et al., 2018; Southern et al., 2019), and further support the parallel measurement of these systems to comprehensively elucidate VML-injured myofiber mitochondria function.
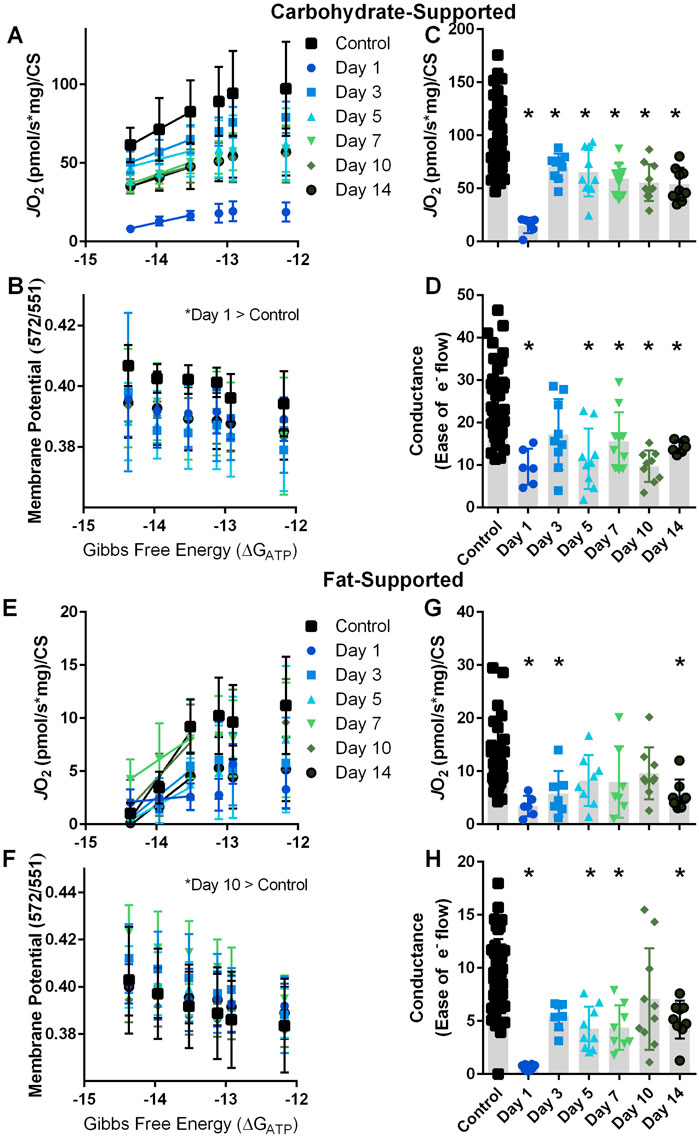
FIGURE 2. Carbohydrate- and fat-supported respiration and mitochondrial membrane potential at clamped ATP Gibbs free energy states. (A, B) Carbohydrate-supported JO2 and mitochondrial membrane potential were measured at decreasing ATP re-synthesis demand Gibbs free energy states in uninjured control myofibers and myofibers at 1, 3, 5, 7, 10, and 14 days post-VML injury. (C) JO2 at −12.17 ∆GATP. (D) Electron conductance calculated by the slope of the −14.37, −13.96, and −13.52 ∆GATP JO2 rates in panel (A). (E, F) Fat-supported JO2 and mitochondrial membrane potential were measured at decreasing ATP re-synthesis demand Gibbs free energy states in uninjured control myofibers and myofibers at 1, 3, 5, 7, 10, and 14 days post-VML injury. (G) JO2 at −12.17 ∆GATP. (H) Electron conductance calculated by the slope of the −14.37, −13.96, and −13.52 ∆GATP JO2 rates in panel (E). Data are shown as mean ± SD. * indicates statistically significant difference compared to uninjured control, p < 0.05 analyzed via one-way ANOVA.
General observation of Carbohydrate- and fat-supported JO2 and electron Conductance
In both uninjured and VML-injured myofibers, JO2 was greater for carbohydrate substrates than fat substrates (Figures 2A, C vs. 2E, G) implying that the oxidative capacity to metabolize pyruvate/malate/succinate is higher compared to palmitoyl-carnitine/malate. Additionally, the linear phase of the CK Clamp relationship between JO2 and ∆GATP has been used previously to define electron conductance (i.e., ease of flow) through the electron transport chain (Glancy et al., 2013; Fisher-Wellman et al., 2018), and reflects mitochondria sensitivity to changing energetic demands. Herein, electron conductance was calculated from the linear portion of the ∆GATP – JO2 curve and is represented as the slopes between data points at −14.37 and −13.52 ∆GATP (Figures 2A–E). In agreement with the literature (Fisher-Wellman et al., 2018), myofibers had a greater conductance with carbohydrate substrates compared to fat substrates (Figures 2D vs. 2H).
Recent reports have demonstrated that whole-body metabolism of carbohydrates and fats is influenced by VML injury. Specifically, when 24-h metabolic rate was tracked longitudinally, there was a 10% decrease at 2-week post-injury compared to pre-injury, and the metabolic rate remained attenuated out to 6-week post-injury (Dalske et al., 2021). This same report also assessed the respiratory exchange ratio (RER), a technique that analyzes O2 consumption to CO2 production to give an indication of carbohydrate and fat metabolism. VML-injured mice had a 4% decrease in RER at 6-week post-injury owing to a decline in carbohydrate metabolism. Whole-body RER estimates of substrate utilization and skeletal muscle-specific substrate-utilization are not the same. However, the latter can influence the former so we decided to interrogate the effect of VML injury on carbohydrate- and fat-supported JO2 and electron conductance.
Effect of VML injury on carbohydrate-supported JO2, electron conductance, and mitochondria membrane potential.
At the highest demand for ATP re-synthesis, JO2 was statistically different across the acute time course of VML pathology (Figure 2C). Electron conductance was significantly less at days 1 (−60%), 5 (−52%), 7 (−35%), 10 (−59%) and 14 (−41%) post-VML compared to uninjured control myofibers (Figure 2D). These data support that the VML injury sequela negatively influences the myofiber’s ability to metabolize the carbohydrate substrates at least in the initial 2 weeks following injury, a key time frame for the initiation of muscle regeneration (Quarta et al., 2017; Aguilar et al., 2018; Anderson et al., 2019).
JO2 and electron conductance are intertwined with mitochondrial membrane potential such that a higher energetic membrane (i.e., more polarized) can apply a greater backpressure on the proton pumping complexes of the electron transport chain and result in a slowing of electron conductance and decrease in JO2. There was a significant difference in mitochondrial membrane potential (TMRM 572/551 ratio) compared to uninjured controls at day 1 (+5.5%) post-VML injury (p < 0.001).
Effect of VML injury fat-supported JO2, electron Conductance, and mitochondrial membrane potential
In contrast to carbohydrate-supported metabolism, there were fewer acute time points with significant differences in JO2, i.e., days 1 (−70%), 3 (−50%), and 14 (−52%) were significantly different from uninjured control myofibers (p = 0.002; Figure 2G); while there were several differences in fat-supported electron conductance (p = 0.001) at day 1 (−93%), 5 (−54%), 7 (−53%), and 14 (−45%) compared to uninjured control myofibers (Figure 2H). It is possible fat-supported respiration is more resilient to changes during the acute VML pathology; but it is also possible that fat-respiration rates are too close to the lower detectable range of the device that it obscures the fidelity and interpretation of the results (aka “floor effect”). There was a significant difference in mitochondrial membrane potential (TMRM 572/551 ratio) compared to uninjured controls at day 10 (+8.8%) post-VML injury (p < 0.01).
All JO2 rates reported in Figure 2 are normalized to CS activity. It is notable that there wasn’t a statistical difference in CS activity across the acute time course of VML injury compared to uninjured controls (p = 0.257; CS activity avg. 334 ± 58 mmol/min/g) in agreement with a previous report on the early time course of VML injury (Corona et al., 2018).
The effect of VML injury on the JO2 and mitochondrial membrane potential relationship
We next plotted JO2 and mitochondrial membrane potential across the entire ∆GATP curve at two acute timepoints (1- and 10-day post-injury) for carbohydrate and fat substrates (Figure 3). This can be useful to observe the changing relationship between the proton motive force and oxygen consumption during the acute time course of VML injury, and 1- and 10-day post-injury were selected because these timepoints had significant difference in membrane potential, for carbohydrate- and fat-substrates, respectively (Figures 2B, F). For carbohydrate-supported respiration (Figure 3A), the effect of the VML injury is a downward-rightward shift such that for any ∆GATP tested, VML-injured myofibers had less JO2 and a greater polarized mitochondrial membrane at Day 1. The membrane potential component of this relationship was not present at Day 10.
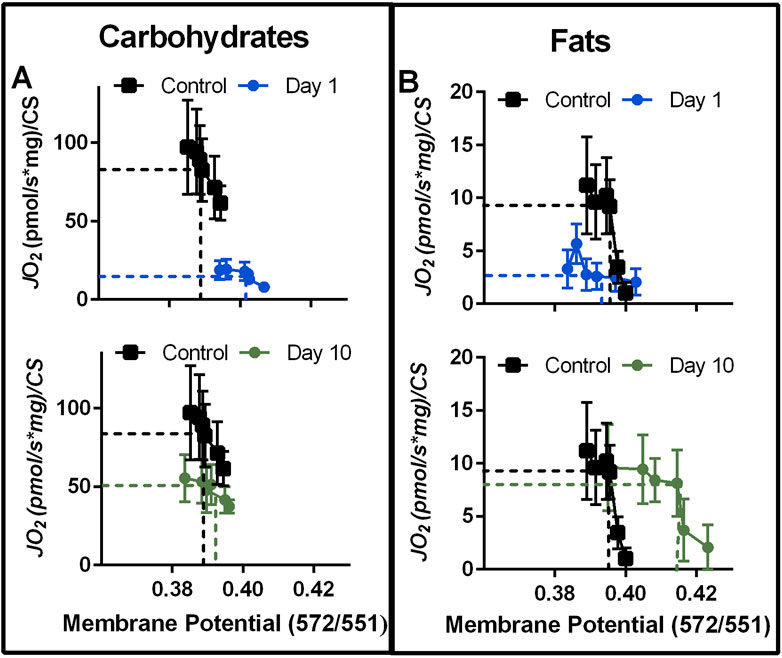
FIGURE 3. Representative time course of VML effect on the mitochondrial respiration—mitochondrial membrane potential relationship with carbohydrate or fat fuels. (A, B) The relationship between mitochondrial membrane potential and JO2 with carbohydrate fuels (A) and fat fuels (B) at 1 and 10 days post-VML injury relative to uninjured control. Data reflects JO2 and mitochondrial membrane potentials during the CK Clamp experiment. In both panels, dashed lines highlight JO2 and membrane potential relationships at −13.52 ∆GATP to help visualize shifting inter-relationships. Data are mean ± SD for JO2.
In contrast, fat-supported plots (Figure 3B) demonstrate a downward shift (driven by less JO2 for any given ∆GATP) at Day 1 and a rightward shift (driven by greater membrane polarization) at Day 10. A greater energetic potential at the mitochondrial membrane in concert with less JO2 could indicate a higher resistance to ATP synthesis. However, the mitochondrial network becomes highly fractured and disorganized during this time after VML injury (Southern et al., 2019; Forouhesh Tehrani et al., 2021) and one of the determinants of mitochondrial function is its location and structural organization (Ferreira et al., 2010; Glancy et al., 2015; Glancy et al., 2017). The mitochondrial network connectively has been shown to rapidly distribute changes in mitochondrial membrane potential (Glancy et al., 2015; Glancy et al., 2017), effectively distributing energy more rapidly in a cell than would otherwise be possible via substrate diffusion alone. Furthermore, the mitochondrial network can rapidly sequester damaged mitochondrial units to halt the spreading of damage to the network. Therefore, the relationship between JO2 and mitochondrial membrane potential is likely more complex than just a higher resistance to ATP synthesis.
Conclusion
The use of mitochondrial respiration techniques in general to evaluate the severity of muscle damage is a relatively unused approach in the field of VML. However, cataloguing the decline in mitochondrial metabolism after severe injury may prove important to describing whole-body metabolic changes after injury as well as identifying new therapeutic targets to improve the overall function of the remaining muscle after a VML injury.
Our results validate the CK Clamp approach as a useful tool in identifying a dynamic relationship in mitochondrial respiration and mitochondrial membrane potential for carbohydrate substrates during acute VML. Despite previous studies evaluating mitochondrial respiration using methods that don’t recapitulate in vivo physiological conditions, the findings reported in this brief report support their conclusion that VML injury causes damage to the metabolic function of the remaining myofibers. Several bioenergetic questions remain for the short- and long-term VML pathology; including, how the ATP/ADP and NAD(P)/NAD(P)H ratios are affected by the injury, specific electron transport chain complex sensitivity to the VML injury, reactive oxygen species production, and importantly, what is the initial mechanism of the metabolic pathology (e.g., loss of calcium homeostasis and/or mitochondria network disorganization). The CK Clamp technique is a valuable tool in pursuit of answering these questions following VML injury.
Data availability statement
The original contributions presented in the study are included in the article/supplementary material, further inquiries can be directed to the corresponding author.
Ethics statement
The animal study was reviewed and approved by University of Georgia IACUC.
Author contributions
JM-F, SG, and JC contributed to the conceptual design of the study. JM-F, EH, EW, and JH were responsible for data acquisition and analysis. JM-F, EH, EW, JH, SM, and JC contributed to the interpretation of data. JM-F drafted the manuscript. JH, SG, and JC critically evaluated the manuscript. JM-F, EH, EW, JH, SG, and JC provided approval for publication of the content. JC agrees to be accountable for all aspects of the work in ensuring that questions related to the accuracy or integrity of any part of the work are appropriately investigated and resolved.
Funding
This work was supported by the NIH (grant number R01 AR078903) to SG and JC.
Conflict of interest
The authors declare that the research was conducted in the absence of any commercial or financial relationships that could be construed as a potential conflict of interest.
Publisher’s note
All claims expressed in this article are solely those of the authors and do not necessarily represent those of their affiliated organizations, or those of the publisher, the editors and the reviewers. Any product that may be evaluated in this article, or claim that may be made by its manufacturer, is not guaranteed or endorsed by the publisher.
References
Aguilar, C. A., Greising, S. M., Watts, A., Goldman, S. M., Peragallo, C., Zook, C., et al. (2018). Multiscale analysis of a regenerative therapy for treatment of volumetric muscle loss injury. Cell. death Discov. 4, 33–11. doi:10.1038/s41420-018-0027-8
Ahima, R. S., and Antwi, D. A. (2008). Brain regulation of appetite and satiety. Endocrinol. Metab. Clin. North Am. 37, 811–823. doi:10.1016/j.ecl.2008.08.005
Anderson, S. E., Han, W. M., Srinivasa, V., Mohiuddin, M., Ruehle, M. A., Moon, J. Y., et al. (2019). Determination of a critical size threshold for volumetric muscle loss in the Mouse quadriceps. Tissue Eng. Part C. Methods 25, 59–70. doi:10.1089/ten.TEC.2018.0324
Aurora, A., Garg, K., Corona, B. T., and Walters, T. J. (2014). Physical rehabilitation improves muscle function following volumetric muscle loss injury. BMC Sports Sci. Med. Rehabilitation 6, 41. doi:10.1186/2052-1847-6-41
Chao, T., Burmeister, D. M., Corona, B. T., and Greising, S. M. (2019). Oxidative pathophysiology following volumetric muscle loss injury in a porcine model. J. Appl. Physiol. 126, 1541–1549. doi:10.1152/japplphysiol.00026.2019
Corona, B. T., Flanagan, K. E., Brininger, C. M., Goldman, S. M., Call, J. A., and Greising, S. M. (2018). Impact of volumetric muscle loss injury on persistent motoneuron axotomy. Muscle Nerve 57, 799–807. doi:10.1002/mus.26016
Corona, B. T., Rivera, J. C., Owens, J. G., Wenke, J. C., and Rathbone, C. R. (2015). Volumetric muscle loss leads to permanent disability following extremity trauma. J. Rehabilitation Res. Dev. 52, 785–792. doi:10.1682/JRRD.2014.07.0165
Curtis, K. S., Stratford, J. M., and Contreras, R. J. (2005). Estrogen increases the taste threshold for sucrose in rats. Physiol. Behav. 86, 281–286. doi:10.1016/j.physbeh.2005.08.002
Dalske, K. A., Raymond-Pope, C. J., McFaline-Figueroa, J., Basten, A. M., Call, J. A., and Greising, S. M. (2021). Independent of physical activity, volumetric muscle loss injury in a murine model impairs whole-body metabolism. PLoS One 16, e0253629. doi:10.1371/journal.pone.0253629
Ferreira, R., Vitorino, R., Alves, R. M., Appell, H. J., Powers, S. K., Duarte, J. A., et al. (2010). Subsarcolemmal and intermyofibrillar mitochondria proteome differences disclose functional specializations in skeletal muscle. Proteomics 10, 3142–3154. doi:10.1002/pmic.201000173
Fisher-Wellman, K. H., Davidson, M. T., Narowski, T. M., Lin, C-T., Koves, T. R., and Muoio, D. M. (2018). Mitochondrial diagnostics: A multiplexed assay platform for comprehensive assessment of mitochondrial energy fluxes. Cell. Rep. 24, 3593–3606. doi:10.1016/j.celrep.2018.08.091
Forouhesh Tehrani, K., Pendleton, E. G., Southern, W. M., Call, J. A., and Mortensen, L. J. (2021). Spatial frequency metrics for analysis of microscopic images of musculoskeletal tissues. Connect. Tissue Res. 62, 4–14. doi:10.1080/03008207.2020.1828381
Garg, K., Ward, C. L., Hurtgen, B. J., Wilken, J. M., Stinner, D. J., Wenke, J. C., et al. (2015). Volumetric muscle loss: Persistent functional deficits beyond frank loss of tissue. J. Orthop. Res. 33, 40–46. doi:10.1002/jor.22730
Glancy, B., Barstow, T., and Willis, W. T. (2008). Linear relation between time constant of oxygen uptake kinetics, total creatine, and mitochondrial content in vitro. Am. J. Physiol. Cell. Physiol. 294, C79–C87. doi:10.1152/ajpcell.00138.2007
Glancy, B., Hartnell, L. M., Combs, C. A., Femnou, A., Sun, J., Murphy, E., et al. (2017). Power grid protection of the muscle mitochondrial reticulum. Cell. Rep. 19, 487–496. doi:10.1016/j.celrep.2017.03.063
Glancy, B., Hartnell, L. M., Malide, D., Yu, Z. X., Combs, C. A., Connelly, P. S., et al. (2015). Mitochondrial reticulum for cellular energy distribution in muscle. Nature 523, 617–620. doi:10.1038/nature14614
Glancy, B., Willis, W. T., Chess, D. J., and Balaban, R. S. (2013). Effect of calcium on the oxidative phosphorylation cascade in skeletal muscle mitochondria. Biochemistry 52, 2793–2809. doi:10.1021/bi3015983
Greising, S. M., Rivera, J. C., Goldman, S. M., Watts, A., Aguilar, C. A., and Corona, B. T. (2017). Unwavering pathobiology of volumetric muscle loss injury. Sci. Rep. 7, 13179–13214. doi:10.1038/s41598-017-13306-2
Greising, S. M., Warren, G. L., Southern, W. M., Nichenko, A. S., Qualls, A. E., Corona, B. T., et al. (2018). Early rehabilitation for volumetric muscle loss injury augments endogenous regenerative aspects of muscle strength and oxidative capacity. BMC Musculoskelet. Disord. 19, 173. doi:10.1186/s12891-018-2095-6
Grogan, B. F., and Hsu, J. R., and Skeletal Trauma Research Consortium (2011). Volumetric muscle loss. J. Am. Acad. Orthop. Surg. 19 (1), S35–S37. doi:10.5435/00124635-201102001-00007
Iwasa, T., Matsuzaki, T., Yano, K., Yiliyasi, M., Kuwahara, A., Matsui, S., et al. (2018). Effects of chronic testosterone administration on the degree of preference for a high-fat diet and body weight in gonadal-intact and ovariectomized female rats. Behav. Brain Res. 349, 102–108. doi:10.1016/j.bbr.2018.02.021
Larsen, S., Nielsen, J., Hansen, C. N., Nielsen, L. B., Wibrand, F., Stride, N., et al. (2012). Biomarkers of mitochondrial content in skeletal muscle of healthy young human subjects. J. Physiol. 590, 3349–3360. doi:10.1113/jphysiol.2012.230185
McFaline-Figueroa, J., Schifino, A. G., Nichenko, A. S., Lord, M. N., Hunda, E. T., Winders, E. A., et al. (2022). Pharmaceutical agents for contractile-metabolic dysfunction after volumetric muscle loss. Tissue Eng. Part A 28, 795–806. doi:10.1089/ten.TEA.2022.0036
McLaughlin, K. L., Hagen, J. T., Coalson, H. S., Nelson, M. A. M., Kew, K. A., Wooten, A. R., et al. (2020). Novel approach to quantify mitochondrial content and intrinsic bioenergetic efficiency across organs. Sci. Rep. 10, 17599. doi:10.1038/s41598-020-74718-1
Quarta, M., Cromie, M., Chacon, R., Blonigan, J., Garcia, V., Akimenko, I., et al. (2017). Bioengineered constructs combined with exercise enhance stem cell-mediated treatment of volumetric muscle loss. Nat. Commun. 8, 15613. doi:10.1038/ncomms15613
Raymond-Pope, C. J., Basten, A. M., Bruzina, A. S., McFaline-Figueroa, J., Lillquist, T. J., Call, J. A., et al. (2022). Restricted physical activity after volumetric muscle loss alters whole-body and local muscle metabolism. J. Physiol 601 (4), 743–761.
Scaduto, R. C., and Grotyohann, L. W. (1999). Measurement of mitochondrial membrane potential using fluorescent rhodamine derivatives. Biophys. J. 76, 469–477. doi:10.1016/S0006-3495(99)77214-0
Schmidt, C. A., Fisher-Wellman, K. H., and Neufer, P. D. (2021). From OCR and ECAR to energy: Perspectives on the design and interpretation of bioenergetics studies. J. Biol. Chem. 297, 101140. doi:10.1016/j.jbc.2021.101140
U.S. Department of Health and Human Services and U.S. Department of Agriculture (2015–2020). Dietary Guidelines for Americans. 8th Edn. December 2015. Available at: https://health.gov/our-work/food-nutrition/previous-dietary-guidelines/2015.
Keywords: muscle metabolism, muscle bioenergetics, muscle injury, oroboros oxygraph 2K, HORIBA
Citation: McFaline-Figueroa J, Hunda ET, Heo J, Winders EA, Greising SM and Call JA (2023) The bioenergetic “CK Clamp” technique detects substrate-specific changes in mitochondrial respiration and membrane potential during early VML injury pathology. Front. Physiol. 14:1178213. doi: 10.3389/fphys.2023.1178213
Received: 02 March 2023; Accepted: 27 March 2023;
Published: 04 April 2023.
Edited by:
Norio Fukuda, Jikei University School of Medicine, JapanReviewed by:
Brian Glancy, National Institutes of Health (NIH), United StatesToshiko Yamazawa, Jikei University School of Medicine, Japan
Copyright © 2023 McFaline-Figueroa, Hunda, Heo, Winders, Greising and Call. This is an open-access article distributed under the terms of the Creative Commons Attribution License (CC BY). The use, distribution or reproduction in other forums is permitted, provided the original author(s) and the copyright owner(s) are credited and that the original publication in this journal is cited, in accordance with accepted academic practice. No use, distribution or reproduction is permitted which does not comply with these terms.
*Correspondence: Jarrod A. Call, call@uga.edu