- 1Center for Underground Physics, Institute for Basic Science (IBS), Daejeon, Republic of Korea
- 2Center for Exotic Nuclear Studies, Institute for Basic Science (IBS), Daejeon, Republic of Korea
- 3IBS School, University of Science and Technology (UST), Daejeon, Republic of Korea
- 4Department of Physics, Kyungpook National University, Daegu, Republic of Korea
- 5Froumkin’s Institute of Physical Chemistry and Electrochemistry of the Russian Academy of Sciences (IPCE RAS), Moscow, Russia
- 6Department of Accelerator Science, Korea University, Sejong, Republic of Korea
This paper describes preparing radiopure molybdenum trioxide powder enriched with Mo-100 isotope for the AMoRE-II experiment. AMoRE-II, the second phase of the AMoRE experiments, will search for the neutrinoless double-beta decay (0νDBD) of the 100Mo isotope using over 100 kg of 100Mo embedded in 200 kg of ultra-pure Li2100MoO4 bolometric crystals. Efficient purification technology was developed and adapted to purify 100MoO3 powder with a 5 kg per month production capacity. Based on the ICP-MS analysis of purified powder, the 232Th and 238U were reduced to <9.4 μBq/kg and <50 μBq/kg, respectively. The concentrations of potassium, transition metals, and heavy metals were lower than 1 ppm. HPGe counting confirmed the reduction of progenies from the 232Th and 238U decay chains, reporting upper limits of <27 μBq/kg for 228Ac and <16 μBq/kg for 228Th. The 226Ra activity was acceptable at 110 ± 30 μBq/kg. In the last 3 years, 100 kg of pure 100MoO3 powder was produced. The production yield for the final purified product was above 90%, while irrecoverable losses were under 1.5%, and all by-products could be recycled further.
1 Introduction
Searches for neutrinoless double beta decay (0νDBD) are pivotal experiments that can reveal the unanswered nature of the neutrinos, whether the neutrinos are their own antiparticles (Majorana-type) or not (Dirac-type) and may provide information about neutrino mass and mass hierarchy [1, 2]. There are dozens of naturally occurring double beta decay candidates, and 100Mo is notable. Relative to most candidates, the Mo-100 isotope has a comparatively high natural abundance (9.74%) [3] and a high Qββ-value of 3034.40 (17) keV [4], while most natural gamma radiation has energies below 2615 keV. However, searching for the 0νDBD is challenging because of the long half-lives, greater than 1.1 × 1024 yr for 100Mo [5, 6]. This limit corresponds to ∼5.5 count/kg·year, while the next generation of the 0νDBD experiments aims to probe the 1026–1027 years half-live range [7]. The exceptional rarity of these events necessitates a significant source containing tens or hundreds of kilograms of the isotope. To increase the experiment’s sensitivity and make discovery more likely, we need a detector with a high concentration of the isotope. Fortunately, it is possible to get hundreds of kilograms of Mo-100 with an isotopic enrichment level of over 95% using gaseous centrifugal technology. Another essential condition in rare process search experiments is to minimize the background rate. The half-live sensitivity level of zero-background rare process experiments is proportional to the exposure. Still, for sizable backgrounds, it becomes proportional to the square root of the exposure and inversely proportional to the square root of the mass-normalized background rate [2]. Thus, many efforts must be made to reduce the background level in the region of interest (ROI). Every detector material and component must be thoroughly selected to minimize its contribution to the projected background. Since molybdenum is not suited for fabrication in a semiconductor and there is neither a commercially used gaseous nor liquid scintillator, there are two techniques to study the 0νDBD of 100Mo. One way is to use pure metallic molybdenum foil as source material, and the other is to synthesize molybdate-based crystals and use the calorimetric particle detection method.
Several experimental research groups have chosen 100Mo as an isotope of interest to search for 0νDBD, and the AMoRE collaboration is one of them. The AMoRE project is a series of experiments to search for the neutrinoless double beta decay of 100Mo embedded in molybdate-based bolometric crystals using low-temperature calorimeters [8]. AMoRE-Pilot, the initial phase, was carried out in 2015—2018 at the Yangyang Underground Laboratory (Y2L) utilizing 0.9 kg of 100Mo embedded in six calcium molybdate crystals (Ca100MoO4, CMO). These crystals were produced at JSC “Fomos Materials” [9] by double crystallization with a normal gradient Czochralski method. As the first results of the AMoRE-Pilot, a background rate of ∼0.5 count/keV·kg·year (ckky) in the energy range of 2850–3150 keV, and the corresponding decay half-life limit of
The project’s next phase, AMoRE-II, is being prepared and will operate at the 1,100 m underground Yemilab of the Center for Underground Physics (CUP) Institute for Basic Science (IBS) in Korea. The AMoRE-II detector is an array of about 400 molybdate crystals (∼100 kg of 100Mo), such as CMO and LMO. The projected sensitivity goal for the corresponding decay half-life limit is
The development of reproducible crystallization and detector technologies is vital to make it applicable to a large-scale 0νDBD experiment, like AMoRE-II. The following requirements must be met for the bolometers to be compatible: large enough crystal boule size, high light yield and excellent optical properties, ultra-low radioactive contamination, and reasonably low irrecoverable losses of the high-cost enriched isotope [8, 21, 22]. Considering that the price of 100Mo isotope with enrichment over 95% is about 100 $/g, a loss of a few % is acceptable in the whole bolometer preparation chain. To meet the stringent radioactivity constraints of the AMoRE-II at the level of 10−4 ckky, a crystal bulk contamination on the level of 10 μBq/kg of 228Th and even 100 μBq/kg of 226Ra would provide a minor contribution to ROI [8, 23, 24, 34]. The radioactivity originating from other nuclides from Th/U chains may not exceed a few mBq/kg levels to avoid pile-up effects.
The conventional Czochralski [25] and low-temperature gradient Czochralski (LTG-Cz) [26] methods are used in growing the single bulk crystals for the AMoRE. Molybdenum trioxide 100MoO3 enriched with 100Mo isotope and metals’ (Ca, Li, etc.) carbonates or oxides are used as precursors for the synthesis. The segregation effect could differ depending on the purity of initial materials, the method of crystallization and its yield efficiency, and the nature of grown crystals and impurities. To ensure the reduction of contaminants, sequential double crystallization and preliminary purification of the precursors must be implemented. The presented paper focuses on developing the purification method for enriched 100MoO3 powder for the AMoRE-II and its adaptation for preparing about 150 kg of pure product at CUP.
Recently, a certain number of research articles discussing methods of the radio-purification of molybdenum were published by other research groups. The NEMO-3 experiment [5] used about 7 kg of 100Mo metallic foil as a source material to study the 0νDBD. To reach the projected background, metallic molybdenum (ITEP, Moscow) was purified by chemical and physical methods [27]. The metal was dissolved in nitric acid to remove the contamination of thorium, uranium, and radium. The wet-chemistry method with the following steps was implemented: co-precipitation with Ba-carrier (80 mBq of 226Ra per 1 kg of Ba(NO3)2), synthesis of 100MoO3, and calcination in an H2 environment to reduce to metal.
Additionally, the produced metal was purified by zone refining to remove transition and alkali-earth elements giving an overall procedural reduction factor of over two orders of magnitude for all measured radionuclides. Activities at the <3 mBq/kg level for 40K, <10 mBq/kg for products of 238U and 235U decay chains, and <1 mBq/kg for 232Th decay chains were achieved. However, the authors express concerns about the production rates and cost. It was also suggested to modify the co-precipitation step, which is also a problem due to the unavailability of the carrier.
The LUMINEU project, also searching for 0νDBD, used zinc molybdate (Zn100MoO4) crystal scintillators enriched with Mo-100 isotope [28]. Enriched molybdenum trioxide powder used to produce the crystals was purified by successive double sublimation of the powder under vacuum. The sublimed powder was dissolved in ammonia to make an ammonium molybdate solution, and high-purity zinc oxide (by UMICORE) was introduced to initiate the co-precipitation of impurities with the zinc molybdate sediment. The purified solution was evaporated to 70% in a volume, and double recrystallization of ammonium polymolybdates from aqueous ammonium solutions was implemented. The experiment confirmed the reduction of alkali and transition elements with the sublimation technique, while recrystallization from an aqueous solution was inefficient for Ca and Fe removal. The paper did not show the reduction of the most dangerous radioactive impurities like thorium, uranium, and radium.
Further, to demonstrate the applicability of the LUMINEU technology, the CUPID-0/Mo experiment with ∼5 kg of 100Mo embedded into Li2100MoO4 crystals was running at the Modane underground laboratory (France) [29]. The LUMINEU purification technique was employed for the preparation of the molybdenum powder. The limits on the radiopurity, ≤3 μBq/kg of 226Ra and ≤2 μBq/kg of 228Th, were reported for the crystals grown from the purified 100MoO3 powder.
At this moment, purification of the raw enriched molybdenum material is a crucial step to achieve the sources’ radiopurity levels required for 0νDBD searches. However, the development of the purification method is complicated by the difficulties associated with confirming its effectiveness. Even though it is efficiently used for material examination at the ppt level, gamma spectrometry with high purity germanium (HPGe) needs a long counting time of up to a few months. It also requires quite bulky samples of a few-kilogram scale [30]. However, the sensitivity of standard ICP-MS techniques is limited at the ppb level due to matrix and polyatomic interference effects [31–33].
The AMoRE-II collaboration searches for the 0νDBD using 120 kg of Mo-100 isotope embedded in 200 kilograms of molybdate crystals. 180 kg of enriched molybdenum trioxide powder 100MoO3, equivalent to 120 kg of 100Mo, is used to grow ultra-pure Li2100MoO4 bolometric crystals. The 100MoO3 powder produced by gaseous centrifugal technique with enrichment to about 96% shows high initial purity of 99.997% [34]. According to the producer’s certificate of analysis (COA), the limits of <0.2 ppb for 238U and <1 ppb for 232Th were reported. Our earlier research investigated a method to purify molybdenum trioxide powder, practicing with the commercially available natural (unenriched) powder [35]. The method includes a process similar to the LUMINEU sublimation technique [36] but differs in the “wet-chemistry” approach. A Ca-containing carrier purified at CUP (having 4 mBq of 226Ra per 1 kg of CaCO3) is used for co-precipitation, followed by membrane filtration. The final ammonium polymolybdate powder is synthesized from the highly acidic media (unlike the LUMINEU fractional recrystallization from an aqueous solution). Further, with the help of the ICP-MS facility at CUP, efficient thorium and uranium removal from enriched 100MoO3 powder to the ppt level was confirmed, reaching the ppt level for the first kilogram of produced powder using the developed purification method [37].
The current study summarises our experience of purifying over 100 kg of raw 100MoO3 powder and describes purification efficiency and capacity. We will report the results of an extensive HPGe and ICP-MS examination of powders before and after purification. We will derive the decontamination factors for thorium, uranium, radium, and other impurities. The paper is structured as follows: the purification method and materials used are described in Section 2. Section 3 describes ICP-MS and HPGe array measurements of the powders. Finally, Section 4 provides the conclusion and discussion of the results.
2 Materials and methods
Powder purification and ICP-MS analysis were performed in class 1,000 clean rooms (ISO6) at CUP. The purification procedure was conducted in successive steps [1]: sublimation under low vacuum [2], separation of impurities with co-precipitation using a Ca-containing carrier [3], synthesis of ammonium polymolybdate (APM) from acidic media by interaction with HCl [4], thermal decomposition of the APM in a pure air environment. All labware used in the purification procedure was made of high-purity quartz, PTFE, PFA, or HDPE. Before use, every apparatus was cleaned with 1% HNO3 with sonication at 60°C. Thermal decomposition of the APM powder was performed using an in-house designed machine where the powder was heated inside a quartz chamber. The chamber was equipped with outlets for vacuum or pure air environment maintenance.
Molybdenum trioxide powder (JCS ECP, Russia) enriched with Mo-100 isotope (>96% enrichment) was used as the initial material. After the sublimation, 100MoO3 was dissolved in an aqueous ammonium hydroxide solution supplied by Sigma Aldrich (∼25% Puriss). A calcium-based carrier for co-precipitation was produced from commercially available calcium carbonate and purified at CUP. For APM synthesis, hydrochloric acid (Acros Organics, ACS reagent, ca., 37% solution in water) was used after sub-boiling purification using a Savillex® DST acid purification system. All water was Millipore® 18.2 MΩ cm−1 deionized water. Each batch of powder produced was measured with ICP-MS to confirm purity. The powders approved with ICP-MS were stored at the Yangyang Underground Laboratory (Y2L) in a desiccator filled with N2 gas.
Quantitative chemical analyses of molybdenum trioxide powders were performed using an Agilent 7900 ICP-MS. The column chromatography method was used to measure thorium and uranium concentrations after their extraction. Al, K, Fe, Ni, Cu, Ti, Cr, W, Sr, Ba, and Pb concentrations were obtained by directly analyzing reconstituted solutions after microwave digestion [38]. Radioactivity levels for 226Ra, 228Ac, 228Th, and 40K were measured with the CAGe (CUP Array of Germanium) detector system that consists of fourteen high-purity germanium detectors installed at Y2L [39].
3 Results
The AMoRE-II purification process has been ongoing at CUP since the beginning of 2019. Of 180 kg of the original 100MoO3 powder, 113 kg was treated by the end of 2022. Usually, about 1.4 kg of powder was purified in one cycle. The cycle began with the raw powder’s sublimation and ended by collecting about 1.3 kg of the final annealed product. The process continued for 2 weeks; 1 week was for the sublimation, and the other was for “wet chemistry” and the APM thermal decomposition procedures. These two processes could be done in parallel for two successive cycles so that 1.3 kg of the product could be collected weekly. Each produced batch of the powder was sampled for the ICP-MS at CUP. With the help of ICP-MS analysis, we could confirm the powder’s radiopurity in a comparatively short time. The powder with confirmed purity was vacuum-sealed with Al/Mylar® bag and stored at Y2L in a desiccator filled with N2 gas (5N purity).
3.1 ICP-MS
Trace ICP-MS analysis is a powerful tool for low-background experiments. The ICP-MS screening helps to estimate the K, Th, and U contamination and select material with the required purity in a relatively short time. When developing the purification method, it is vital to determine the reduction factors for contaminants and understand the possibility of achieving the required radiopurities. In the case of routine purification and production, the ICP-MS help is necessary for incoming materials screening and outgoing product control.
The ICP-MS team at CUP has extensive experience analyzing high-purity molybdenum trioxide and other Mo compounds. The Th and U sensitivities of ICP-MS at CUP are comparatively high, having detection limits at the ppt level. When delivered from the producer, each lot of raw 100MoO3 powder was tested at the CUP (13 lots) to determine the real thorium and uranium concentrations and understand how they differ from lot to lot (Table 1).
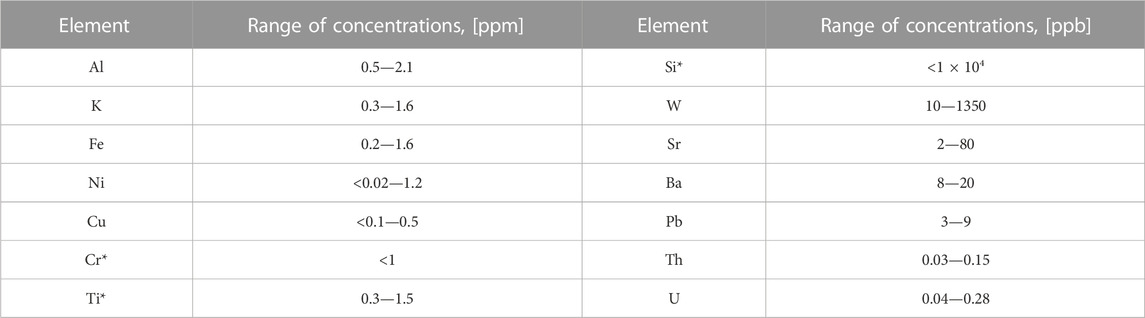
TABLE 1. Range of concentrations for measured contaminants in different lots of raw 100MoO3 powder. * Data of the producer.
As mentioned above, Mo enrichment was performed through the centrifugation of MoF6 gas. After multiple centrifugation cycles, the exceptionally pure 100MoF6 gas was converted into 100MoO3 product by interaction with HNO3, which could be secondary cross-contaminated from labware and chemicals. Common impurities in quartz or glass (K, Ba, Si) and in the composition of stainless steel (Fe, Cr, Ni, Ti) were found to be at a few ppm. The concentration of isovalent with Mo tungsten did not exceed 1.5 ppm, which indicates efficient centrifugal separation. Tungsten mimics the chemical behavior of molybdenum and may not be separated using the standard chemical methods of separation and purification. Concentrations of other metallic contaminants (Na, Mg, Zn, Ca, etc.) were below ppm level. In agreement with the producer’s COA, >99.997% purity grade of the thirteen lots of received powder was confirmed by analysis at CUP. The radiopurity of the raw powders was significantly higher than shown in [14, 27] for all listed contaminants. Nevertheless, the concentrations of the measured impurities lead us to conclude that the received 100MoO3 powder must be purified below ppm for potassium to reduce the background rate in ROI caused by random coincidences of 40K [40] and transition (Al, Fe) metals to avoid staining the grown crystal [14]. The initial W concentration of about 1 ppm level was acceptable for the AMoRE project. Th and U must be reduced by one order of magnitude to grow the crystals compatible with the required radiopurity.
The 100MoO3 powder must be uniform in purity and grain size to ensure successive production of low-background crystals. After the sublimation procedure, the powder was chunky and non-stoichiometric. Followed “wet chemistry” techniques were implemented to provide adequate density, uniformity, and consistent purity of produced powders regardless of the purity of starting materials. Using ICP-MS, we tested every produced batch of the purified CUP powder. Analysis of representative samples of the powders produced at different times (from different initial lots of raw powder) is shown in Table 2.
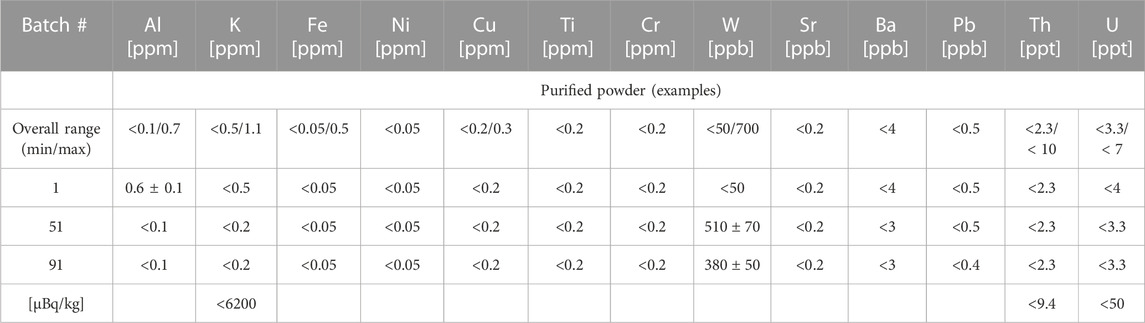
TABLE 2. Purity of 100MoO3 powder treated at CUP. In the last row, the values are expressed in μBq/kg (1 ppm = 31 mBq/kg for 40K, 1 ppt = 4.1 μBq/kg for 232Th, and 12.4 μBq/kg for 238U). Values and upper limits are given at 95% C.L.
In general, the ICP-MS screening showed a reduction of all measured elements by one order of magnitude. The concentrations of transition and heavy metals for all tested powders were reduced to the required level below about 1 ppm. These low concentrations would not affect the transparency of the grown crystal. Al, Ni, Fe, Cu, Ti, and Cr concentrations were tracked to ensure that no contamination happened during the thermal decomposition of ammonium polymolybdate, which is the last step of powder purification. The concentrations of Sr and Ba, which are isovalent with radium, were reduced by one order of magnitude. The reduction of Si with the developed method was supposed to be efficient, considering the insolubility and non-volatility of SiO2 under conditions suggested by the method. Still, due to the incompatibility of the CUP ICP-MS system, the Si concentration was not measured in the purified 100MoO3. The concentration of tungsten in the purified powders was slightly reduced from 1.3 ppm to 0.7 ppm indicating weak separation efficiency. As for the radiopurity, the radioactive contaminants, 232Th and 238U, were below the method detection limits of about 3 ppt. The concentration of K was always controlled to be below 1 ppm. The reproducibility of the CUP purification method for 100MoO3 powder was confirmed in 3 years of continuous routine work. One hundred purification cycles were recently performed successfully, and each batch of produced powder was tested with the ICP-MS. The range of impurities levels in the powders approved for the crystals’ production (100 batches) is summarized in Table 2. The concentrations of Th and U for all approved powder batches were below 10 and 7 ppt, respectively. Potassium levels varied in the range from a minimum of <0.5 ppm to a maximum of 1.1 ppm. The powders that did not meet the purity requirements were not used for crystal synthesis, but were re-purified and tested. The possibility of quickly confirming the purity of the powder and tracing the contamination was a helpful approach to avoid cross-contamination.
3.2 HPGe array
With the help of ICP-MS at CUP, we could trace the reduction of K and radioactive isotopes of the Th and U decay chains in pure 100MoO3 powder. Considering significant Sr and Ba reduction, we may expect the removal of Ra, which belongs to the same family group of the periodic table. Using gamma-ray spectrometry with the CAGe operating 700 m deep underground at Y2L, we could measure progenies’ activities in the uranium and thorium chains. From the 232Th decay chain, the activity of 228Ac was acquired in equilibrium with 228Ra, and 212Pb and 208Tl were assumed to be in equilibrium and representative of (with branching-ratio correction) 228Th. From the 238U decay chain, the 214Pb and 214Bi were measured as representative of the 226Ra activity.
About 12.7 kg of raw 100MoO3 powder from one representative lot was installed into the CAGe and counted for 146 days. After the measurement, the powder was purified at CUP according to the usual routine, and 12 kg of the purified powder was measured with the CAGe for 170 days (Table 3). The activities of 228Ac and 228Th from the thorium chain were below upper limits, <27 μBq/kg and <16 μBq/kg, respectively. The activity of 226Ra was reduced twice in the purified powder resulting in an acceptable level of about one hundred μBq/kg.
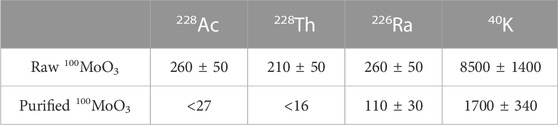
TABLE 3. Values and 90% C.L. upper limits on 228Ac, 228Th, 226Ra, and 40K in the raw and purified 100MoO3 powders in units of μBq/kg. The decision to report values or limits is determined using statistical errors only, while the reported results include systematic errors.
4 Discussion
The 100MoO3 purification method was developed from the lab-scale experiment to purify 180 kg of powder. The yield efficiency for the final pure product was above 90%, and 5 kg per month was the current production capacity at CUP. The irrecoverable losses were optimized to be below 1.5% through careful collection and recycling of all by-products: washing waters, calcium molybdate co-precipitate, wiping tissues, etc.
Over 100 kg of enriched 100MoO3 powder was purified at CUP for the last 3 years with the developed purification method. The ICP-MS and HPGe analysis of produced powders confirm the high reproducibility of the developed purification technique. Regardless of the purity of initial materials, concentrations of transition and heavy metals in the produced powders were reduced to below 1 ppm. The segregation effect of crystal growth will ensure high transparency and optical properties of the crystals grown with this product.
Radioactive thorium, uranium, radium, and potassium contamination was reduced to the required level and tested using ICP-MS and HPGe methods. The ICP-MS upper limits of <9.4 μBq/kg and <50 μBq/kg were for 232Th and 238U, respectively. Cross-measuring the activities of progenies in the thorium and uranium chains with CAGe in 12.7 kg of raw and 12 kg of purified powders confirms the radiopurity reporting <27 μBq/kg of 228Ac and <16 μBq/kg of 228Th. The 226Ra from the 238U chain was reduced by a factor of two, resulting in an acceptable level of 110 ± 30 μBq/kg. The reduction factor for barium was similar to those of radium and varied from 2 to 6, based on the ICP-MS screening. HPGe and ICP-MS analysis of potassium were in good agreement reporting a reduction by one order of magnitude.
The purity of 100MoO3 powder produced with the above-described technology satisfies the stringent AMoRE-II requirements for radiopurity and production efficiency. However, there is still room for improvement. Varying the sublimation rate and applying a few successive sublimation cycles, it is possible to reduce non-volatile contaminants like Th, U, Ba, Ra, K. Elimination of potential contamination from stainless steel parts of the annealing machine at the final step of the process may help to reduce K, Fe, Al, Cr, etc. The equipment capacity could be scaled up comparatively easily to increase the production rate.
Data availability statement
The raw data supporting the conclusions of this article will be made available by the authors, without undue reservation.
Author contributions
OG, VM, KS, HP, HK, YK, and ML contributed to the conception, original design and technical performance of the study. HY and YK performed routine purification. JC performed the ICP-MS analysis. KH, WK, GK, EL, DL, and S-YP contributed to the HPGe array measurement performance. OG wrote the first draft of the manuscript. All authors contributed to the manuscript revision, read, and approved the submitted version.
Funding
This work was supported by the Institute for Basic Science (IBS), funded by the Ministry of Science and ICT, Korea (Grant id: IBS-R016-D1).
Conflict of interest
The authors declare that the research was conducted in the absence of any commercial or financial relationships that could be construed as a potential conflict of interest.
Publisher’s note
All claims expressed in this article are solely those of the authors and do not necessarily represent those of their affiliated organizations, or those of the publisher, the editors and the reviewers. Any product that may be evaluated in this article, or claim that may be made by its manufacturer, is not guaranteed or endorsed by the publisher.
References
1. Giuliani A, Poves A. Neutrinoless double-beta decay. Adv High Energ Phys (2012) 2012:1–38. doi:10.1155/2012/857016
2. Dell'Oro S. Neutrinoless double beta decay: Expectations and uncertainties. Nucl Part Phys Proc (2015) 265-266:31–3. doi:10.1016/j.nuclphysbps.2015.06.008
3. Haynes WM, Lide DR, Bruno TJ. CRC handbook of chemistry and Physics: A ready-reference book of chemical and physical data. Boca Raton, Florida: CRC Press (2016)
4. Rahaman S, Elomaa VV, Eronen T, Hakala J, Jokinen A, Julin J, et al. Q values of the 76Ge and 100Mo double-beta decays. Phys Lett B (2008) 662(2):111–6. doi:10.1016/j.physletb.2008.02.047
5. Arnold R, Augier C, Baker JD, Barabash AS, Basharina-Freshville A, Blondel S, et al. Results of the search for neutrinoless double-β decay in 100Mo with the NEMO-3 experiment. Phys Rev D (2015) 92:072011. (NEMO-3 collaboration). doi:10.1103/PhysRevD.92.072011
6. Augier C, Barabash AS, Bellini F, Benato G, Beretta M, Berge L, et al. Final results on the 0νββ decay half-life limit of 100Mo from the CUPID-Mo experiment. The Eur Phys J C (2022) 82:1033. doi:10.1140/epjc/s10052-022-10942-5
7. Vergados JD, Ejiri H, Simkovic F. Theory of neutrinoless double-beta decay. Rep Prog Phys (2012) 75:106301. doi:10.1088/0034-4885/75/10/106301
8. Alenkov V, Aryal P, Beyer J, Boiko RS, Boonin K, Buzanov O, et al. Technical design report for the AMoRE 0νββ decay search experiment (2015). arXiv:1512.05957
9.JSC. Fomos materials. Available at: https://en.newpiezo.com (Accessed March 1, 2023).
10. Alenkov V, Bae HW, Boiko RS, Boonin K, Buzanov O, Beyer J, et al. First results from the AMoRE-Pilot neutrinoless double beta decay experiment. Eur Phys J C (2019) 79:791. doi:10.1140/epjc/s10052-019-7279-1
11. Alenkov V, Bae HW, Boiko RS, Boonin K, Buzanov O, Chanthima N, et al. Alpha backgrounds in the AMoRE-Pilot experiment. Eur Phys J C (2022) 82:1140. doi:10.1140/epjc/s10052-022-11104-3
12. Lee MH. AMoRE: A search for neutrinoless double-beta decay of 100Mo using low-temperature molybdenum-containing crystal detectors. J Instrumentation (2020) 15:C08010. doi:10.1088/1748-0221/15/08/c08010
13. Alenkov V, Buzanov OA, Dosovitskii AE, Kornoukhov VN, Mikhlin AL, Moseev PS, et al. Ultrapurification of isotopically enriched materials for 40Ca100MoO4 crystal growth. Inorg Mater (2013) 49:1220–3. doi:10.1134/s0020168513120029
14. Shlegel VN, Berge L, Boiko R, Chapellier M, Chernyak D, Coron N, et al. Purification of molybdenum oxide, growth and characterization of medium size zinc molybdate crystals for the LUMINEU program. EPJ Web of Conferences (2014) 65:03001. doi:10.1051/epjconf/20136503001
15.NIIC SB RAS. Nikolaev Institute of Inorganic Chemistry, Siberian Branch of Russian Academy of Sciences (NIIC SB RAS). Available at: http://niic.nsc.ru/institute/881-niic (Accessed March 1, 2023).
16. Kim HB, Ha DH, Jeon EJ, Jeon JA, Jo HS, Hang CS, et al. Status and performance of the AMoRE-I experiment on neutrinoless double beta decay. J Low Temperature Phys (2022) 209:962–70. doi:10.1007/s10909-022-02880-z
17. Oh Y. AMoRE [Conference presentation]. Virtual Seoul, Korea: NEUTRINO (2022). May 30 – June 4. Available at: https://zenodo.org/record/6683386#.Y_wLRS8RpMQ (Accessed March 1, 2023).
18. Kim WT, Kim SC, Sharma B, Jeon JA, Kim HL, Kim SG, et al. Test measurements of an MMC-based 516-g lithium molybdate crystal detector for the AMoRE-II experiment. J Low Temperature Phys (2022) 209:299–307. doi:10.1007/s10909-022-02832-7
19. Seo J, Lee MH, Leon EJ. Estimation of muon induced background event rate of AMoRE-II. IL NUOVO CIMENTO (2022) 45:23. doi:10.1393/ncc/i2022-22023-x
20. Bae HW, Kim H, Kim Y, Jeon E, Lee M. Simulation studies for neutron and muon-induced backgrounds in AMoRE-II. J Phys Conf Ser (2020) 1468:012245. doi:10.1088/1742-6596/1468/1/012245
21. Chen MC. Double beta decay: Scintillators. J Phys Conf Ser (2008) 136:022035. doi:10.1088/1742-6596/136/2/022035
22. Dell'Oro S, Marcocci S, Viel M, Vissani F. Neutrinoless double beta decay: 2015 review. Adv High Energ Phys (2016) 2016:1–37. doi:10.1155/2016/2162659
23. Kim YD. AMoRE [Conference presentation]. Seoul, Korea: NEPLES-2019, KIAS (2019). September 23-27. Available at: https://indico.cern.ch/event/803690/contributions/3572814/attachments/1917092/3169927/neples-ydkim.pdf (Accessed March 1, 2023).
24. Kim G. Low background study for AMoRE through material screening facility at Y2L and Yemilab [Conference presentation]. South Dakota Mines, USA: LRT 2022 (2022). June 14 - 17. Available at: https://indico.sanfordlab.org/event/29/contributions/397/attachments/359/880/LRT2022_GWKim.pdf (Accessed March 1, 2023).
25. Son JK, Choe J, Gileva O, Hahn I, Kang W, Kim D, et al. Growth and development of pure Li2MoO4 crystals for rare event experiment at CUP. J Instrumentation (2020) 15:C07035. doi:10.1088/1748-0221/15/07/c07035
26. Grigorieva V, Shlegel V, Bekker T, Ivannikova N, Giuliani A, de Marcillac P, et al. Li2MoO4 crystals by low-thermal-gradient Czochralski technique. J Mater Sci Eng B (2017) 7(3-4):63–70. doi:10.17265/2161-6221/2017.3-4.002
27. Arnold R, Augier C, Baker J, Barabash A, Bing O, Blum D, et al. Chemical purification of molybdenum samples for the NEMO 3 experiment. Nucl Instr Methods Phys Res A (2001) 474:93–100. doi:10.1016/s0168-9002(01)00869-5
28. Danevich FA, Berge L, Boiko RS, Chapellier M, Chernyak DM, Coron N, et al. Status of LUMINEU program to search for neutrinoless double beta decay of 100Mo with cryogenic ZnMoO4 scintillating bolometers. AIP Conf Proc (2015) 1686:020007. doi:10.1063/1.4934896
29. Armengaud E, Augier C, Barabash AS, Bellini F, Benato G, Benoit A, et al. The CUPID-Mo experiment for neutrinoless double-beta decay: Performance and prospects. Eur Phys J C (2020) 80:44. doi:10.1140/epjc/s10052-019-7578-6
30. Laubenstein M, Lawson I. Low background radiation detection techniques and mitigation of radioactive backgrounds. Front Phys (2020) 8:577734. doi:10.3389/fphy.2020.577734
31. Karandashev VK, Turanov AN, Nosenko SV. Analysis of molybdenum oxide by inductively coupled plasma atomic emission and mass spectrometry. J Anal Chem (2011) 66(1):37–43. doi:10.1134/s1061934811010059
32. Khomyakov AV, Mozhevitina EN, Sadovskii AP, Sukharev VA, Avetissov IC. Purity of MoO3 from different manufacturers. Inorg Mater (2016) 52(3):285–93. doi:10.1134/s0020168516030055
33. Kipphardt H, Czerwensky M, Matschat R. ICP-MS analysis of high purity molybdenum used as SI-traceable standard of high metrological quality. J Anal At Spectrom (2005) 20:28–34. doi:10.1039/b409973g
34.ECP. Electrochemical plant, joint-stock company (2022). [Online]. cited 2022 December 12. Available from: https://www.ecp.ru/eng/ (Accessed March 1, 2023).
35. Gileva O, Aryal P, Karki S, Kim H, Kim Y, Milyutin V, et al. Investigation of the molybdenum oxide purification for the AMoRE experiment. J Radioanal Nucl Chem (2017) 314:1695–700. doi:10.1007/s10967-017-5568-4
36. Karki S, Aryal P, Gileva O, Kim H, Kim Y, Lee DY, et al. Reduction of radioactive elements in molybdenum trioxide powder by sublimation method and its technical performance. J Instrumentation (2019) 14:T11002. doi:10.1088/1748-0221/14/11/t11002
37. Gileva O, Choe J, Kim H, Kim Y, Lee M, Park H, et al. Purification and recovery of 100MoO3 in crystal production for AMoRE experiment. J Instrumentation (2020) 15:C07032. doi:10.1088/1748-0221/15/07/c07032
38. Gileva O, Choe J, Kim Y, Lee MH, Leonard DS, Shin K, et al. Thorium and uranium trace ICP-MS analysis for AMoRE project. Appl Radiat Isot (2023) 194:110673. doi:10.1016/j.apradiso.2023.110673
39. Park SY, Hahn K, Kang W, Kazalov V, Kim G, Kim Y, et al. Measurement of the background activities of a 100Mo-enriched powder sample for an AMoRE crystal material by using fourteen high-purity germanium detectors. Nucl Instr Methods Phys Res A (2021) 992:165020. doi:10.1016/j.nima.2021.165020
Keywords: 100MoO3, ultra-low radioactivity, purification, AMoRE-II, ICP-MS, HPGe array
Citation: Yeon H, Choe J, Gileva O, Hahn KI, Kang WG, Kim GW, Kim HJ, Kim Y, Kim Y, Lee EK, Lee MH, Leonard DS, Milyutin V, Park H, Park S-Y and Shin K (2023) Preparation of low-radioactive high-purity enriched 100MoO3 powder for AMoRE-II experiment. Front. Phys. 11:1142136. doi: 10.3389/fphy.2023.1142136
Received: 11 January 2023; Accepted: 06 March 2023;
Published: 30 March 2023.
Edited by:
Frank Franz Deppisch, University College London, United KingdomReviewed by:
Jose Marques, Instituto Superior Tecnico, PortugalLuciano Pandola, Universities and Research, Italy
Copyright © 2023 Yeon, Choe, Gileva, Hahn, Kang, Kim, Kim, Kim, Kim, Lee, Lee, Leonard, Milyutin, Park, Park and Shin. This is an open-access article distributed under the terms of the Creative Commons Attribution License (CC BY). The use, distribution or reproduction in other forums is permitted, provided the original author(s) and the copyright owner(s) are credited and that the original publication in this journal is cited, in accordance with accepted academic practice. No use, distribution or reproduction is permitted which does not comply with these terms.
*Correspondence: Olga Gileva, gilevaolga@ibs.re.kr