- 1Department of Neuroscience, Central Clinical School, Monash University, Prahran, VIC, Australia
- 2B’, Department of Neurology, Laboratory of Experimental Neurology and Neuroimmunology, AHEPA University Hospital, Thessaloniki, Greece
Multiple sclerosis (MS) is a heterogeneous autoimmune disease whereby the pathological sequelae evolve from oligodendrocytes (OLs) within the central nervous system and are targeted by the immune system, which causes widespread white matter pathology and results in neuronal dysfunction and neurological impairment. The progression of this disease is facilitated by a failure in remyelination following chronic demyelination. One mediator of remyelination is thyroid hormone (TH), whose reliance on monocarboxylate transporter 8 (MCT8) was recently defined. MCT8 facilitates the entry of THs into oligodendrocyte progenitor cell (OPC) and pre-myelinating oligodendrocytes (pre-OLs). Patients with MS may exhibit downregulated MCT8 near inflammatory lesions, which emphasizes an inhibition of TH signaling and subsequent downstream targeted pathways such as phosphoinositide 3-kinase (PI3K)-Akt. However, the role of the closely related mammalian target of rapamycin (mTOR) in pre-OLs during neuroinflammation may also be central to the remyelination process and is governed by various growth promoting signals. Recent research indicates that this may be reliant on TH-dependent signaling through β1-integrins. This review identifies genomic and non-genomic signaling that is regulated through mTOR in TH-responsive pre-OLs and mature OLs in mouse models of MS. This review critiques data that implicates non-genomic Akt and mTOR signaling in response to TH-dependent integrin receptor activation in pre-OLs. We have also examined whether this can drive remyelination in the context of neuroinflammation and associated sequelae. Importantly, we outline how novel therapeutic small molecules are being designed to target integrin receptors on oligodendroglial lineage cells and whether these are viable therapeutic options for future use in clinical trials for MS.
Introduction
Oligodendrocytes (OLs) are cells that developmentally myelinate axons within the mammalian central nervous system (CNS). The loss of these cells or specific developmental defects in oligodendrogenesis results in denudement of axons, potentiating the brain’s vulnerability to further neurodegeneration (Kotra et al., 2017). Specifically, the integrity of CNS neurons and their axons requires the structural and metabolic support of OLs (Robertson and Moreo, 2016). Support failure results in severe neurological disorders such as that manifested in multiple sclerosis (MS) or during inherited forms of leukoencephalopathy (Feinstein et al., 2015). Indeed, determining how to preserve OLs and their myelin sheaths would be a major contribution to neuroprotection and limit the permanent neurological deficit. To achieve CNS repair through remyelination, one viable option may be therapeutics that modify the diseased tissue environment and can be successfully administered through the tight blood-brain barrier (BBB) and directed to areas of lesions. However, a major limitation is the stalled maturation of endogenous oligodendrocyte progenitor cell (OPC) and the number of cells available to effectively perform repair within viable areas of lesions (Absinta et al., 2019). One main reason for remyelination failure in MS is stalled OL differentiation that is partially due to a lack of trophic support (Australia, 2022).
It is now evident that thyroid hormones (THs) are transported across the plasma membrane by specific TH transporters such as monocarboxylate transporter 8 (MCT8, encoded by the SLC16A2 gene) (Friesema et al., 2003). MCT8 was first identified in the brain and is highly expressed by neuronal populations of the cerebral and cerebellar cortex, hippocampus, striatum, and hypothalamus (Heuer et al., 2005); however, functional studies performed in Xenopus laevis oocytes elucidated its importance in TH transport (Friesema et al., 2003). In fact, MCT8 plays a critical role in brain development, particularly in the human brain, where it facilitates the uptake of T3 across the blood–brain barrier (BBB) (Ceballos et al., 2009). The monocarboxylate transporter 10 (MCT10), encoded by the SLC16A10 gene, has also been shown to transport TH in addition to performing its common T-type amino acid transporter function (Friesema et al., 2008). MCT10 shows overlapping expression with MCT8 and has recently been identified as an important transporter expressed within developed white matter tracts of the mouse brain (Friesema et al., 2008), which suggests a functional role in mature OLs. TH plays a central role in OL development and myelination in vivo by regulating the expression of specific maturation genes, such as myelin basic protein (MBP), and thereby regulates myelination (Younes-Rapozo et al., 2006). Furthermore, T3 affects the critical replication of OPCs and the myelin production and survival of OLs, which are developmentally-regulated events that lead to coordinated myelination (Fernandez et al., 2004).
Treatment options and therapeutic limitations
Within the past 29 years of disease-modifying therapies (DMTs) development and since the introduction of interferon-β (IFN-β) as a treatment for relapsing remitting MS (RRMS) (1993), there have been few therapeutic options for both primary progressive MS (PPMS) and secondary progressive MS (SPMS) (Feinstein et al., 2015; Robertson and Moreo, 2016; Kotra et al., 2017). Currently, 16 Therapeutic Goods Administration (TGA) and food and drug administration (FDA)-approved therapies exist with the intention of reducing clinical and sub-clinical disease activity, which otherwise contributes to long-term disability (Absinta et al., 2019; Australia, 2022). Additionally, existing therapies targeting inflammation in RRMS have proven effective in managing relapse rates. Generally, protection against relapse is 20–35%, 50–55%, and >60% in injectable, oral, and infusion therapies, respectively (Hauser et al., 2013). However, abrogating neurodegenerative processes in PPMS and SPMS, independent of inflammatory lesion activity (Ontaneda et al., 2017), remains ineffective. Examples are chronic active plaques seen in progressive disease, which persist unabated, and are associated with an aggressive disease-course and poor clinical outcomes (Absinta et al., 2019).
Despite the emergence of radiological indicators, the sole aim of DMTs is to manage symptomology and inflammation, but a lack of efficacy in treating progressive forms of MS indicates that progressive disease treatments remain an unmet medical need. Furthermore, none of the current therapies provide neuroprotection or neurorepair, which is required to prevent future neurological deficits (Robertson and Moreo, 2016). Consequently, recovery from progressive myelin degeneration remains unattainable (Sen et al., 2022).
However, one plausible avenue to attain neuroprotection and repair is to promote thyroid hormone (TH) signaling in OLs.
Thyroid hormone synthesis and regulation
TH are endocrine messengers that regulate several physiological processes, such as development, growth, and metabolic activities, in all mammalian cells (Brent, 2012). In vertebrates, particularly humans, TH is crucial for effective brain development and functioning by promoting the maturation of neuronal and glial cells while maintaining their cytoarchitecture (Salazar et al., 2019). Additionally, it stimulates the proliferation, differentiation, and migration of neural cells that target the formation of synaptogenesis, dendritic cell branching, and myelination (Bernal et al., 2000). Therefore, TH is a chief regulatory hormone in the homeostatic functioning of the developing and adult brain.
The physical structure of TH includes two main forms as follows: the pro-hormone as 3, 5, 3′, 5′-L-tetraiodothyronine or thyroxine (T4) and its active form with one less iodine atom as 3, 5, 3′-L-triiodothyronine (T3) (Bernal, 2005). The conversion of T4 to T3 can be achieved by selenocysteine-dependent deiodinase (DIO) enzymes that catalyze the removal of iodine (deiodination) and activate T4 to T3 via DIO2. Importantly, more than 80% of cellular T3 is derived from the deiodination from T4 by tissue-specific DIOs (Larsen et al., 1981; Pilo et al., 1990; Maia et al., 2005). The expression of DIO2 is restricted to the CNS (Visser et al., 1982), pituitary (Maeda and Ingbar, 1984), brown adipose tissue (Leonard et al., 1983), and placenta (Kaplan and Shaw, 1984). In the rat and human brain, there is differential expression of DIO2 in the hippocampus, cortex, and corpus callosum (Croteau et al., 1996), and at the cellular level, DIO2 is mostly expressed in tanycytes, astrocytes, OLs, and interneurons (Guadaño-Ferraz et al., 1997; Tu et al., 1997; Guadaño-Ferraz et al., 1999). This suggests that these supporting glia are the first port of call for converting thyroid hormone to the active form for intracellular metabolism that ensues within the axons and neurons they support. Alternatively, where DIO2 activates T4 to T3, the inactivation of T3 to rT3 can occur via specific DIO3 activity (Schweizer et al., 2014) that is also located within astrocytic and OL cells of the CNS (Bernal, 2007). This demonstrates the importance of these macroglia in governing the homeostatic regulation of TH within the brain.
The synthesis and secretion of TH are both regulated by the hypothalamic-pituitary-thyroid (HPT) axis that utilizes a negative feedback loop system (Medici et al., 2015) to maintain a homeostatic environment of T4 and T3 (Mendoza and Hollenberg, 2017). Environmental and physiological stimuli trigger the synthesis of TH, whereby thyrotropin-releasing hormone (TRH) neurons in the paraventricular nucleus (PVN) of the hypothalamus secrete TRH in the median eminence (Kopp, 2001; Medici et al., 2015). As a result, TRH binds to the TRH receptor located on the membrane of thyrotrophs to release thyroid-stimulating hormone (TSH) from the anterior-pituitary (Kopp, 2001; Medici et al., 2015; Mendoza and Hollenberg, 2017). TSH then acts through its specific receptor (the TSH receptor) to stimulate the growth and function of thyroid follicular cells, which regulate the synthesis and secretion of T4 and T3 from the thyroid gland and into the circulation (Kopp, 2001), with T4 constituting approximately 80% of that secreted into the circulation (Pirahanchi et al., 2021). To ensure efficient systemic TH delivery, the majority of TH are bound to specific plasma proteins (albumin, thyroxine-binding globulin, and transthyretin) of varying binding affinities and dissociation rates to create redundancies in the TH transport network (Schreiber and Richardson, 1997).
Thyroid hormone signaling drives oligodendrocyte differentiation and myelination
OLs are mature glial cells responsible for ensheathment of axons with myelin in the brain and permit rapid saltatory conduction between neurons (McTigue and Tripathi, 2008; Kim and Petratos, 2019). OPC are derived from neural precursor cells (NPCs), namely, glial fibrillary acidic protein (GFAP)-positive astrocytes (type-B cells) that originate in the subventricular zone (SVZ) adjacent to the lateral ventricle in the brain (Menn et al., 2006). These then migrate to the corpus callosum (cc), striatum, and fimbria fornix via platelet-derived growth factor (PDGF) signaling that promotes indiscrete motility of OPCs; furthermore, there is interaction with the basement membrane molecules, such as laminin (LN), in the extracellular matrix (ECM) (Tsai et al., 2016; Suzuki et al., 2019).
OPCs proliferate in response to both PDGF and fibroblast growth factor (FGF) with downregulation of the PDGF-α receptor that initiates differentiation to late progenitor cells (Baron et al., 2000). This eventually results in non-myelinating and myelinating OLs that are implicated in the repair of myelinated tracks (Petratos et al., 2004). These mature OLs extend and spirally wrap their lipid-rich membrane around neuronal axonal segments several times to form the myelin sheath and promote lower energy consumption and faster saltatory impulse conduction during neuronal communication (Pfeiffer et al., 1993; Zalc and Colman, 2000; Hartline and Colman, 2007; Nave, 2010; Lebel and Beaulieu, 2011; Hughes and Appel, 2016). As a result, the CNS quickly and efficiently acts to maintain the functional integrity, metabolism, and long-term survival of neurons and improves motor-sensory function, cognition, and neuroplasticity (Nave, 2010; Lebel and Beaulieu, 2011; Saab and Nave, 2017) (Figure 1).
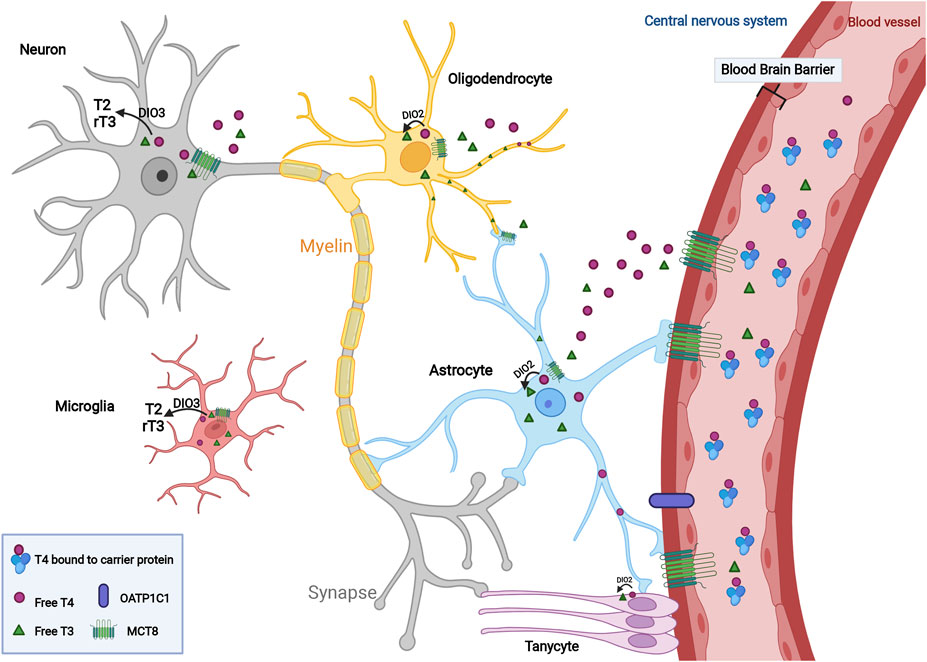
FIGURE 1. Transport and regulation of thyroid hormone within the central nervous system. In the circulation, a higher ratio of TH is in the form of free T4 compared to T3 level, which is sustained in an inactive status due to its binding with carrier protein such as albumin. T4 and T3 cross the blood-brain barrier (BBB) via the monocarboxylate transporter 8 (MCT8) and OATP1C1 and then transported into neurons and glial cells including astrocytes, oligodendrocytes, microglia and tanycytes within the CNS. To promote cellular proliferation, differentiation and myelination mediated by TH, T4 converts to its active form, T3, in the presence of deiodinase type 2 (DIO2). Active T3 mediates myelination by mature oligodendrocytes. Increased thyroid hormone concentrations contribute to the conversion of T3 and T4 to inactive metabolites, T2 and rT3, to maintain endogenous homeostasis under the regulation of DIO3.
Following the occurrence of a demyelinating lesion in the cc, SVZ-derived NPC populations increase in number, as reflected in cuprizone models of demyelination, with increased NPCs that contribute to neuroprotection (Fancy et al., 2004; Nait-Oumesmar et al., 2007; Nait-Oumesmar et al., 2008; Butti et al., 2019). This highlights OL maturation in progressive disease as an intuitive target with the capability of leveraging the already present population of OLs in the cc and inducing differentiation to mature myelinating OLs that permit remyelinating events to occur.
Remyelination can be induced through TH signaling, which acts as a regulatory trophic signal of OL maturation and subsequent myelination through terminal differentiation of OPCs into myelinating mature OLs; this further occurs through rapid cell-cycle arrest that is elicited through genomic and non-genomic signaling (Lee and Petratos, 2016; Kim and Petratos, 2019).
Thyroid hormone-dependent genomic signaling
Genomic TH signaling via TH receptors (TRs), TRα, and TRβ, which are expressed in the nucleus of OLs, are integral in the gene transcription involved in differentiation and following TH binding (Billon et al., 2001; Lee and Petratos, 2016). Furthermore, cytoplasmic T3 binds to TRα, TRβ1, and TRβ2. These TRs form heterodimers with other TRs such as the retinoid X receptor (RXR). Increased levels of TR-RXR heterodimerization located in oligodendroglia (Pombo et al., 1999) can actively increase binding to TH response elements (regulatory DNA sequences) (Cheng et al., 2010; Taylor and Heyland, 2017) and drive gene transcription, i.e., TH-dependent transcriptional activity (Hsu et al., 1995).
TRα is implicated in the initial cell-cycle exit and is predominantly expressed in OPCs, whereas TRβ1 occurs in later-developmental stages (pre-OLs) and is involved in terminal differentiation and myelination (Baas et al., 1998; Billon et al., 2002; Bury et al., 2002; Wallis et al., 2010; Lee and Petratos, 2016). Furthermore, the absence of TRα and TRβ results in persistent proliferation of OPCs and indicates their synergistic role in normal OL development (Baas et al., 2002). Despite the functional conclusions made through in vivo studies of TRβ, its role in vivo still remains largely unknown (Dong et al., 2009).
There is further confounding of genomic signaling where un-liganded TRs may repress T3 responsive genes. This is particularly important in the context of SVZ-derived OPCs where unbound TRs in hypothyroid conditions recruit the nuclear co-repressor responsible for repression of genes involved in OL differentiation from NSCs observed during development. Such repression can be associated with upregulation of histone deacetylases (HDACs), which repress myelin gene expression through increased inaccessibility of DNA to transcription factors via removal of acetyl groups from histones (Castelo-Branco et al., 2014a; Wheeler et al., 2015). However, the maintenance of neural cells involved in embryonic development are also regulated by T3 in adults. This was shown in adult hypothyroid rodents and increased proliferation of SVZ cells (Lemkine et al., 2005; Lopez-Juarez et al., 2012).
Thyroid hormone signaling in MCT8-deficient circumstances
Thyroid hormone transporters (THTs) that are localized to plasma membranes include MCT8 (SLC16A2), a high affinity TH-specific transporter of both T3 and its pro-hormone T4, that facilitates the passage of these insoluble hormones across the BBB with great efficacy into the CNS to exert neurobiological effects (Friesema et al., 2003; Ceballos et al., 2009; Kinne et al., 2010; Vatine et al., 2017). In addition, the homolog, MCT10 (49% amino acid overlap), is also capable of T3 uptake and efflux (Friesema et al., 2003; Friesema et al., 2008). These transporters exhibit the highest substrate specificity to TH (Bernal, 2006) relative to other known alternative THTs, such as L-type amino acid transporters (LATs) (Mayerl et al., 2014) and the organic anion transporter (OAT) family (Sun et al., 2014), present in other species.
Genomically, it has been identified that THs rely on THTs, such as MCT8, to elicit TH-dependent differentiation in OLs (Vatine et al., 2021). The MCT8s function is elucidated not only in T transport at the BBB, but locally at OL plasma membranes (Lee and Petratos, 2016; Lee et al., 2017).
In instances of SLC16A2 mutation, the dysfunction of MCT8 results in insufficient intracellular levels of T3 in the presence of increased serum free levels, which results in hypomyelination and the severe psychomotor retardation seen in Allan-Herndon-Dudley syndrome (AHDS) (Friesema et al., 2004; Armour et al., 2015; Valcárcel-Hernández et al., 2022). MCT8 dysfunction, as a prerequisite of AHDS, is supported by limited pre-clinical data from mouse models and clinical trials in AHDS patients and when treated with the TH analog 3,5-diiodothyropropionic acid (DITPA) resulted in amelioration of peripheral hyperthyroidism and overall hypermetabolism (Verge et al., 2012; Ferrara et al., 2015).
The TH treatment in animal models of MS yields similar beneficial effects and permits a reduction in demyelination and axonal injury through anti-inflammatory properties (Calza et al., 2002; Dell'Acqua et al., 2012). In addition to its ability to accelerate remyelination following acute cuprizone administration (Zendedel et al., 2016; Hartley et al., 2019), there is emerging evidence from our group suggesting that MCT8 is also downregulated in neuroinflammatory conditions seen in MS. This is similar to those observed in animal models of MS where the acute inflammatory-mediated downregulation of MCT8/10 is observed throughout the CNS of SPMS Chronic-active Lesion (preliminary data). Therefore, genomic signaling in a TH-dependent manner via MCT8 is not a viable treatment option.
However, the thyroid hormone analog, DITPA has been shown to function in an MCT8-independent manner as suggested earlier in treating patients clinically identified as having hyperthyroidism as a consequence of AHDS (Verge et al., 2012). Indeed, in the slc16a2−/− zebrafish model of AHDS, the administration of DITPA also led to the restoration of myelin and normalization of locomotor behavior (Zada et al., 2014). These paradigms are consistent with our own findings in a cell culture model that utilized human embryonic stem cell hESC-OPCs. In this case, the administration of the small molecule DITPA [AU2015372427B2, 2020-07-02 (Granted), EP3237605B1, 2021-02-24 (Granted), US10640748B2, 2020-05-05] (Granted) led to OPC differentiation, maturation, and subsequent myelination. In addition, salvaging TH-deficient OPCs permits their maturation to myelinating OLs (Lee et al., 2017).
Therefore, it is postulated that OL-specific MCT8 deficiency can uncouple TH-dependent genomic signaling and thus the lack of differentiation of myelin-producing OPCs that may ensue promote the development of neurological diseases such as MS (Kim and Petratos, 2019). This hypothesis is currently under investigation within our laboratory.
The role of integrins as alternate thyroid hormone-dependent receptors
The function of the ECM involves a complex interplay between several extracellular molecules that collectively govern the structural integrity of tissues and organs while providing a selective and protective barrier between the extracellular and intracellular environment (Pompili et al., 2021). Additionally, the ECM is essential for regulating cellular processes such as growth, migration, differentiation, and adhesion (Plow et al., 2000; Hynes, 2009; Barczyk et al., 2010; Wen et al., 2019; Pompili et al., 2021). The composition of the ECM encompasses glycosaminoglycans (GAGs), proteoglycans, growth factors, and other ECM proteins, such as collagen and adhesion proteins, where the latter includes Laminin LN that binds to receptors on the ECM that are known as integrins (Bonnans et al., 2014; Wen et al., 2019).
Integrins are transmembrane heterodimers consisting of α and β subunits linked by a non-covalent bond that modulates the interaction between the ECM and surrounding cells (Campbell and Humphries, 2011). In mammals, there are 24 different integrin receptors formed from a combination of 18α and 8β subunits (Hynes, 2002), which are displayed as two membrane-spanning helices (one for each subunit) and a small cytoplasmic tail located on the basement membrane of the ECM (Ruppert et al., 1995; Montgomery et al., 1996; Campbell and Humphries, 2011).
The biological function of integrin receptors acts as an adhesion molecule and contributes to the action of the ECM by providing cell-cell, cell-ECM, and ligand binding (Plow et al., 2000; Campbell and Humphries, 2011; Wen et al., 2019; Pompili et al., 2021). These structural and pleiotropic interactions include cell surface adhesion proteins, soluble vascular cell adhesion molecule , and ECM ligands such as vitronectin, fibronectin, vascular cell adhesion molecule VCAM-1, and mucosal addressin cell adhesion molecule MAdCAM-1 (Plow et al., 2000; Campbell and Humphries, 2011; Wen et al., 2019; Pompili et al., 2021). Thus, integrin receptors contribute to the structural maintenance and integrity of tissue, cellular trafficking, differentiation, migration, and adhesion (Plow et al., 2000; Barczyk et al., 2010). Furthermore, integrins play a critical role in the immune system by enabling leukocyte trafficking, with the migration and formation of the immunological synapse, and phagocytosis during adaptive and innate immune responses (Rocha-Perugini et al., 2016).
OLs display a high expression of several integrins with distinct functions. These include αvβ8 integrin expressed during oligodendroglial development, while αvβ1, α6β1, αvβ3, and αvβ5 integrins are sequentially expressed during OL differentiation in vitro (Milner and Ffrench-Constant, 1994). For example, during differentiation, the expression of αvβ3 integrin is strictly regulated, where at its highest peak, it can enable the transition from O2-A progenitors to post-mitotic OLs (Milner and Ffrench-Constant, 1994; Lee and Petratos, 2016). Comparatively, β1 integrin is also heavily involved during OL development. One study utilized a ligand-integrin modified alginate hydrogel in a three-dimensional culture to demonstrate the proliferation and differentiation of NPCs into OLs (Wen et al., 2019). In this study, it was determined that the interaction of ligand LXY30 with an α3β1 integrin receptor promoted human neural precursor cells hNPCs toward OL lineage cells, whereas the ligand LXW64 interaction with the αvβ3 integrin receptor shared a similar but less potent differentiation outcome (Wen et al., 2019). Furthermore, the conditional ablation of the β1 integrin gene in OPCs demonstrated OL survival but not axon ensheathment, myelination, or remyelination as seen in the lysolecithin model of demyelination (Benninger et al., 2006). Another study that utilized the dominant-negative inhibition of β1 integrin in OPCs identified α6β1 integrin as a driver for myelination (Relvas et al., 2001). Moreover, its interaction with LN promoted in vitro and in vivo OL survival (Frost et al., 1999; Colognato et al., 2002).
In the human genome, LN is composed of α, β, and γ subunits where the α subunit is encoded by 5 genes and β and γ subunits by 3 genes (Aumailley, 2013). A combination of these chains generated a total of 16 heterotrimeric shapes of LN (Aumailley, 2013).
Similar to the oligodendroglial lineage in the CNS, Schwann cell differentiation and myelination in the peripheral nervous system (PNS) is also mediated by interactions between LN and β1-integrin as supported by the conditional ablation of β1 integrin (Feltri et al., 2002). However, the major difference is the availability of LN. For example, in the PNS, it is readily sourced from the basal lamina for Schwann cells, while in the CNS, it only becomes available for α6β1 integrin just prior to myelination (Colognato et al., 2002). Whether this is universally observed for all other integrins in the CNS is yet to be determined.
Role of thyroid hormone on oligodendroglial development and myelination via integrin-mediated signaling
Given the importance of integrins in regulating oligodendroglial development, a major ligand that drives this action is TH (Baas et al., 1997). Therefore, the precise level and timing of TH stimulation can modulate cell-specific stages of development, proliferation, differentiation, myelin production, and survival of oligodendroglia (Jones et al., 2003; Younes-Rapozo et al., 2006; Williams, 2008). This premise is supported by a study confirming that the serum level of TH is at its peak during the active myelination phase of the fetal and postnatal stages demonstrating that TH controls the timing and development of OLs (Sarlieve et al., 2004).
Under hypoxic-ischemic (HI) injury conditions, treatment with T4 was shown to significantly alleviate OL death and hypomyelination (Hung et al., 2018). Moreover, its role in hypoxia-related white matter pathologies involved the stimulation of oligodendrogenesis and the regulation of OL metabolism by inducing angiogenesis, which indicates its importance in maintaining OL viability (Yuen et al., 2014). Data has also implicated T4 as a modulator of WM injury from the premature brain by promoting OPC proliferation during early developmental stages, followed by changes to the morphology of post-mitotic OLs, which is a maturation process that increases myelination (Hung et al., 2018).
Given the action of T4, the effect of T3 (the active form of TH) has also been observed in vivo and in vitro. A comprehensive study in rats demonstrated that OPCs, which were observed under conditions of acute and chronic T3 deficiencies, produced higher numbers of pre-OLs but lower numbers of mature OLs (Younes-Rapozo et al., 2006). Similarly, an in vitro study on the cerebral cortices of rat neonates with T3-deficiency revealed a deprivation of the cytoplasm-filled myelin membrane. This finding suggests the importance of T3 during the later stages of OL development in the maturation of myelination (Younes-Rapozo et al., 2006), where the latter is supported by the upregulation of myelin gene expression, including myelin basic protein (MBP) and proteolipid protein (PLP), during T3 administration (Younes-Rapozo et al., 2006).
Collectively, these data propose that T4 and T3 act as mitogens and can induce development, differentiation, and maturation while regulating the cell cycle of OLs that result in the formation of the complex myelin membrane around neuronal axons (Kim and Petratos, 2019). However, their bioavailability is controlled by TH transporters, DIOs, and TH receptors in PNS and CNS tissues (Mendoza and Hollenberg, 2017).
Potentiation of Akt/mTOR signaling by the synthetic thyroid hormone analog
Given that DITPA signals independently of MCT8, its mechanism of action remains largely unknown. However, it has been postulated that the effects of DITPA are due to activation of the non-genomic pathways, Akt and MAPK (mitogen-activated protein kinase), through integrin αvβ3, which is a known non-genomic receptor (Lee et al., 2017). TH can act via the αvβ3 integrin receptor and upregulate activity in the Akt/mTOR signaling pathway (Cao et al., 2005; Cheng et al., 2010). However, one major criticism of those studies positing the non-genomic actions of DITPA is the lack of consideration of this pathway. Moreover, despite MCT8 ablation, no consideration was made in confirming the ability of DITPA to bind to integrin receptors through use of the integrin-antagonist arginylglycylaspartic acid (RGD peptide) (Plow et al., 2000; Davis et al., 2021).
This is vital because two binding sites exist on the integrin αvβ3 for TH (Figure 2). The S1 site binds T3 resulting in PI3K activation; the S2 site predominantly binds T4 and T3 to a lesser extent and is involved in activation of MAPK (Davis et al., 2021). Hence, using the RGD peptide permits delineation between these non-genomic signaling pathways. However, an increase in Akt phosphorylation was seen following DITPA administration to hESC-derived OPCs and in the event integrin αvβ3 could be implicated to be a result of S1 binding (Lee et al., 2017).
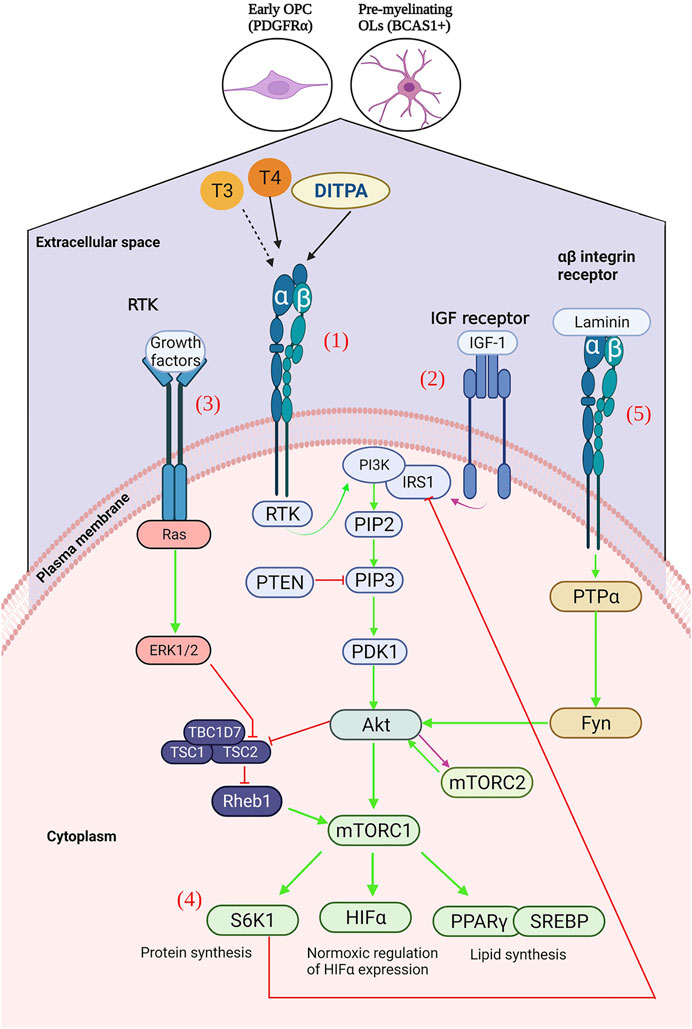
FIGURE 2. (1) Ligation of T3, T4 or DITPA to αβ integrins results in the activation of receptor tyrosine kinase (RTK), activating an intracellular signalling cascade – activating 3-phosphoinositide 3 kinase (PI3K), of which leads to the generation of Ptdlns(4,5)P3 (PIP3). PIP3 is then able to activate phosphoinositide-dependent kinase-1 (PDK1), which results in Akt activation, and subsequent mTOR upregulation128. Downstream activation of S6K1 (protein synthesis), HIFα (glycolytic metabolism), PPARγ and SREBP (lipid synthesis). (2) mTORC2 is mainly activated by growth factors, such as IGF-1, which can directly activate PI3K via the insulin-receptor substrate-1 (IRS-1) – leading to the downstream activation of mTORC2130. (3) Activation of the receptor tyrosine kinase by growth factors, leads to the activation of the Ras signalling cascade, and subsequent Erk1/2 activation – resulting in TSC2 inhibition and consequently mTORC1 upregulation. (4) S6K1 inhibits IRS-1, leading to subsequent ERK2 inhibition and consequent downregulation of S6K1. (5) Laminin binding to αβ integrins results in (Created with BioRender.com).
Non-genomic initiation of the Akt/mTOR signaling pathway
Non-genomic TH-dependent signaling, independent of TR-T3 complexes, involves binding of T4 to integrin αvβ3, which is a plasma membrane receptor present in most vertebrate cells (Taylor and Heyland, 2017). This results in translocation of the TRβ1 receptor from the cytoplasm to the nucleus and permits signal transduction through the tyrosine kinase/PI3K/Akt/mTOR pathway (Lin et al., 2005; Moeller et al., 2006; Moeller et al., 2011) (Figure 2). The mTOR protein is a serine/threonine kinase and a central coordinator of cell anabolism and catabolism. It is comprised of two distinct protein complexes as follows: mTOR complex 1 (mTORC1) and mTOR complex 2 (mTORC2) (Saxton and Sabatini, 2017). Complex mTORC1, which possesses a higher sensitivity to its antagonist rapamycin, contains adaptor protein raptor (regulatory associated protein of mTOR), and mTORC2 contains the adaptor protein Rictor (rapamycin-insensitive companion of mTOR), which showcases greater tolerance but similar inhibition at protracted high doses (Sarbassov et al., 2006; Lamming et al., 2012). mTORC1 predominates during cellular growth and is activated through phosphorylation of downstream effector molecules involved in mitochondrial, glycolytic, and lipid metabolism. In mTORC2, it is implicated in the cytoskeletal organization of cells and is a contributor to the complete activation of Akt (Sarbassov et al., 2005; Guertin et al., 2006).
Akt is a serine/threonine protein kinase responsible for the phosphorylation and regulation of substrates involved in principal cellular functions (Manning and Cantley, 2007). T4 signaling downstream of integrin αVβ3 binding results in activation of mTOR via the Akt/PI3K/mTOR pathway. Akt can activate mTORC1 directly or through inhibition of TSC2 (tuberous sclerosis complex 2), which is a guanosine triphosphate hydrolase (GTPase)-activating protein that is responsible for the inhibition of the Ras family GTPase (Rhes). Otherwise, it is responsible for the activation of mTORC1 following Akt signaling (Zou et al., 2011). The extracellular signal-regulated kinase 1 (ERK1)/MAPK pathway is another notable signal transduction mechanism shown to inhibit TSC2 and results in mTOR activation (Lin et al., 2009; Gaesser and Fyffe-Maricich, 2016).
Furthermore, LN acting via αβ integrins results in protein tyrosine phosphatase α (PTPα)-Fyn activation and subsequent Akt activation (Ye et al., 2008). In addition to insulin growth factor-1 (IGF-1) acting at the IGF-1 receptor (IGF-1R), it can also activate the Akt signaling pathway (Manning and Cantley, 2007). However, mTORC2 can be regulated through growth factor-dependent signaling that may include IGF-1 (Yuan et al., 2012) (Figure 2). It is postulated that the thyroid hormone analog DITPA can act in a TH-dependent manner to promulgate OL differentiation, proliferation, maturation, and remyelination via the Akt/mTOR signaling pathway (Gaesser and Fyffe-Maricich, 2016).
Akt in differentiation and myelination
Several studies have demonstrated the activation of Akt and its involvement in myelination. This was demonstrated in experiments by Goebbels et al. (2010) whereby the PI3K/Akt inhibitor, phosphatase, and tensin homolog (PTEN) were conditionally knocked out in transgenic mice, which resulted in hypermyelination of the CNS concordant with increased levels of Akt phosphorylation. However, no increase was observed in mature OLs (Goebbels et al., 2010). This suggests that Akt activation is associated with CNS myelination but not remyelination associated with the actions of mature myelinating OLs. However, given that the OPC population was not tested, the involvement of Akt in differentiation during early brain developmental stages cannot be ruled out. Furthermore, a study by Chan et al. (2014) established that increased Akt activity was associated with an increased population of OPCs, which could later serve as myelinating OLs in remyelination (Chan et al., 2014).
Affirmation of the effects of Akt signaling on myelination was procured through combinative administration of PTEN and growth factor administration, which is a known activator of Akt and resulted in MAPK activation, in addition to mTOR downstream, and confirmed the involvement of Akt and parallel activation to MAPK in myelination and mTOR as a downstream effector (De Paula et al., 2014). The proposed regulatory role that mTOR has on myelination was further supported following a reduction in hypermyelination after rapamycin treatment in constitutively active Akt mice (Narayanan et al., 2009).
mTOR in differentiation and myelination
mTOR is the primary effector mechanism in Akt-induced myelination (Narayanan et al., 2009) and permits enhanced myelination and myelin protein expression in accordance with upregulated mTORC1 activity (Flores et al., 2008). The inactivation of mTOR leads to decreased myelin RNA and protein levels (Bercury et al., 2014). Furthermore, mTORC1 may be implicated to a greater degree in MBP expression and the mTORC2 influence on myelin gene expression (Tyler et al., 2009). This suggests the function that mTOR has in transcription and translation during OL development.
Validation of the maturation role performed by mTOR was derived from three studies performing conditional ablation of mTORC1 in 2′, 3′-cyclic-nucleotide 3′-phosphodiesterase (CNP)-Cre; Raptor, Olig1-Cre; Rheb1, and CNP-Cre; Rheb1 mice that resulted in hypomyelination (Bercury et al., 2014; Lebrun-Julien et al., 2014; Zou et al., 2014). In addition, OPC differentiation defects occurred in two studies (Bercury et al., 2014; Zou et al., 2014) but not the third (Lebrun-Julien et al., 2014). The important role of mTORC1 in myelin maintenance with respect to the dynamic nature of myelin in adult CNS was shown by Lebrun-Julien et al. (2014), whereby mTORC1 ablation resulted in hypomyelination but mTORC2 did not (Gaesser and Fyffe-Maricich, 2016).
While mTORC1 may modulate myelination, there is disagreement concerning the role of mTORC2 in both myelination and OL differentiation. It was demonstrated by Bercury et al. (2014) that the absence of myelin delay at P14 (myelinating OLs) was insignificant compared to mTORC1 following its ablation; however, OL development was accelerated–with a decrease in OPCs and an increase in mature OLs (Gaesser and Fyffe-Maricich, 2016). Conversely, Lebrun-Julien et al. (2014) demonstrated hypomyelination at P14 but no alteration in OPC differentiation. This may be due to the role of mTORC2 in myelin gene expression opposed to MBP expression, or was perhaps due to a greater implication of mTORC2 in the regulation of OL process dynamics through cytoskeletal reorganization and MBP localization (Sarbassov et al., 2005; Guertin et al., 2006; Musah et al., 2020).
Interplay between Akt/mTOR and MAPK signaling
Despite the intricacies of each mTOR complex, it is posited that both mTORC1 and mTORC2 have distinct roles in OL differentiation, specifically the transition of late progenitor cells to immature OLs (Tyler et al., 2009). This follows the synergistic relationship between Akt/mTOR and ERK1/2 signaling, whereby two points of convergence exist between these pathways determining OL differentiation, namely, at the level of the insulin receptor substrate-1 (IRS-1) and in addition to S6K1 (p70S6K) (Gaesser and Fyffe-Maricich, 2016). Whereby S6K1 inhibits IRS-1 and prevents ERK1/EKR2 activation, ERK2 inhibition results in S6K1 downregulation (Gaesser and Fyffe-Maricich, 2016). Thus, for proper differentiation to occur from OPCs to pre-myelinating OLs (ERK1/2 driven) and pre-myelinating to mature myelinating OLs (Akt/mTOR driven), some homeostasis must exist between the two signaling pathways (Gaesser and Fyffe-Maricich, 2016).
Akt/mTOR signaling in remyelination
There are limited studies demonstrating the ability of Akt signaling in remyelinating events in the CNS or in OL cultures. However, recent significant evidence indicates that the Akt/mTOR pathway may regulate remyelination following spinal cord injury and convert astrocytes to OL lineage cells (Ding et al., 2021). However, the role of the Akt/mTOR pathway remains disputed by a few studies and suggests that its activation may confer a negative immunomodulatory outcome with no therapeutic potential in its ability to induce remyelination (Mammana et al., 2018).
In the circumstance that Akt/mTOR signaling does induce myelination and differentiation of OPCs, two principal limitations associated with the potentiality of remyelinating events in MS can be addressed. The first was the stalled maturation of endogenous OPCs; the second was an insufficient number of OPCs to successfully conduct neurorepair (Sher et al., 2008).
Possible pre-conditions of integrin signaling by mTOR
Integrin receptors are dormant up until their activation with the mechanism of TH-induced activation still widely unknown. One plausible pre-condition of integrin activation in the context of mTOR signaling is the hypoxic induction of integrin receptors (Davis et al., 2021). Hypoxia-inducible factor-1 alpha (HIF-1α) activates transcription of genes facilitating integrin avβ3 (Sesé et al., 2017). Furthermore, Akt/mTOR signaling acts as a condition of normoxia to stabilize hypoxic HIF-1α expression (Corcoran and O’Neill, 2016; Davis et al., 2021). A hypoxic microenvironment may be favorable for remyelination, as the chronic inflammatory milieu (seen in early stages of MS) has been shown to promote the removal of HIF-1α+ monocytes in the hypoxic microenvironment and thereby facilitate neurorepair (Kotter et al., 2001; Bieber et al., 2003). In a rodent model where suppression of acute inflammation via corticosteroid administration led to delayed remyelination, no effect on OPC migration toward lesions was observed and the inhibitory effects exerted upon oligodendroglial cell differentiation is argued to be the result of an abrogated inflammatory environment (Chari et al., 2006; Clarner et al., 2011). This may in part be due to increases in the pro-inflammatory cytokine and tumor necrosis factor-alpha (TNF-α) in toxin-mediated demyelination that is produced by M1 macrophages (Voss et al., 2012; Sen et al., 2022). Furthermore, TNF-α has been shown to activate the plasma membrane phagocytic-triggering receptors expressed in myeloid cells 2 (TREM2) and expressed on microglia and macrophages, which in turn upregulates phagocytosis and suppresses interleukin 6 (IL6)-activated macrophages–necessary for the transition from acute to chronic inflammation. In addition, TREM2 can be activated by lipid ligands derived from myelin debris (Ferrara et al., 2022). In this way, TREM2-activated microglia are converted from an inflammatory phenotype to an anti-inflammatory one that potentiates neurorepair (Xu et al., 2021; Ferrara et al., 2022). The function of TREM2 in myelin debris clearance has been clinically highlighted through gene mutation and resulted in Nasu-Hakola disease–premature dementia and myelin loss (Xing et al., 2015).
Until recently, TREM2 was deemed “undruggable.” However, recent evidence determined TREM2 to be a positive endocrinologically regulated TH gene (Ferrara et al., 2022). Furthermore, activation of mTOR has shown to increase the viability of TREM2+ cells and permit long-term cell survival and growth in a mouse model of Alzheimer’s disease (Ulland et al., 2017).
This may be of great therapeutic benefit as there is evidence suggesting that genomic TH signaling is dampened in an inflammatory environment as observed in EAE-induced hypoxia. This has also been demonstrated to be a result of dysregulated TH transport (Davies et al., 2013). In addition to the association of HIF1-α with DIO3 in the SVZ-derived NPCs, abrogated TH signaling during neuroinflammation can initiate oligodendrogliopathy and delay myelination (Lee and Petratos, 2016). Hence, the activation of TREM2+ microglia may be regulated by TH and thyromimetics to promote neurorepair (Ferrara et al., 2022), and mTOR-regulated autophagy has been shown to polarize microglia to an M2 phenotype in sepsis-induced neuroinflammation (Zhuang et al., 2020).
However, competing evidence exists suggesting that the PI3K/Akt/mTOR signaling pathway is implicated in the upregulation of inducible nitric oxide synthase (iNOS) production under hypoxic conditions, which is associated with microglial activity consisting of an inflammatory phenotype (Weinstein et al., 2000; Lu et al., 2006). Nitric oxide (NO) is necessary for the dissolution of neuroinflammation and responsible for the induction of T-cell apoptosis (Zettl et al., 1997). To support this, apoptosis of T cells via microglial phagocytosis results in the downregulation of the inflammatory phenotype in microglia (Chan et al., 2006). Furthermore, complete inhibition of NOS-2 is associated with worsening clinical scores in EAE and suggests a beneficial threshold of NO production (Dalton and Wittmer, 2005). Thus, some interplay between mTOR regulation in both M1 and M2 phenotypes may exist to achieve homeostasis during myelin clearance and remyelination, and further research is required to elucidate this mechanism.
Thyroid hormone-induced hypertrophy and mitochondrial metabolic regulation
The function of mTOR signaling in hypoxia is of continued significance because mTOR is implicated in cardiac hypertrophy in which hypoxia and oxidative stress predominate. TH can genomically signal via TH-sensitive promoter elements in nuclear-encoded mitochondrial genes during oxidative stress in myocardial cells. This can enhance mitochondrial biogenesis and alleviate the energetic demands of myocytes (Goldenthal et al., 2004; Marín-García, 2010). Iodothyronines, which are inclusive of TH analogs such as DITPA, induce similar pro-angiogenic and hypertrophic effects in infarcted heart tissue through non-genomic activation of cell surface receptor integrin αvβ3 that induces MAPK signaling (Bergh et al., 2005; Mousa et al., 2006) and it can be argued that this is a result of Akt/mTOR activation.
The effects seen in cardiac hypertrophy models showcase the ability of TH to genomically signal via TRα1 and TRβ1 and induce Akt/mTOR survival signaling, which confers cytoprotection and leads to increased synthesis of normal contractile proteins and hypertrophic gene upregulation (Ojamaa, 2010). A study conducted by Kuzman et al. (2007) supports the role of Akt/mTOR in the development of TH-induced cardiac hypertrophy as treatment with rapamycin in mice caused complete inhibition of hypertrophic development. Conversely, there is recent data suggesting that TH-deprivation imparts cardioprotective effects as TH addition led to maladaptive cardiac growth and mTOR upregulation in a mouse model (Kerp et al., 2021). However, this can be largely attributed to the altered adrenergic responsiveness of mouse hearts utilized in experiments that induced left-ventricular pressure overload, which is a pathological form of cardiac hypertrophy. It was determined that the hypertrophy was associated with G protein-coupled receptor (GPCR) activation as opposed to the physiological hypertrophy induced with TH treatment via Akt signaling that exhibits an increased myocardial mass in the absence of adverse effects (Dorn and Force, 2005; Ojamaa, 2010; Kerp et al., 2021) and thereby permits the cardioprotective nature of TH to remain largely unattested. Mitochondrial biogenesis pathways critical for axo-myelin integrity require effective Akt/mTOR signaling in OLs but specific pathway analysis dependent on TH and integrin activation remain unresolved (Fünfschilling et al., 2012).
However, the genomic signaling capacity of TH is not limited to the heart as a reduction in TRα-dependent transcription factors, such as peroxisome proliferator-activated receptor γ coactivator 1 alpha (PGC1α), mitochondrial transcription factor A (TFAM), and estrogen-related receptor α (ERRα), are all responsible for mitochondrial biogenesis as observed in the TRα mutation in skeletal muscle (Zhou et al., 2021). The upregulation of PGC-1α, which is the master transcriptional regulator of nuclear respiratory factors (NRFs), by TRα is also responsible for local DIO2 production in cells enhancing localized T3 production (Marín-García, 2010). Since the genomic activation of mTORC1 leads to an upregulation of PGC-1α associated with mitochondrial biogenesis, non-genomic activation of the Akt/mTOR pathway could provide beneficial genomic increases in local T3 production in OLs through an increase in mTORC1 signaling.
It should be noted that an increased rate of mitochondrial transcription and energy metabolism occurs in parallel with an increased production of reactive oxygen species (ROS), which are a by-product of the electron transport chain (ETC). Chronic exposure of mitochondrial DNA (mtDNA) to ROS, due to the proximity of mtDNA to oxidative machinery, can lead to the functional decline of mtDNA under conditions of oxidative stress (Ballinger et al., 2000; Tsutsui et al., 2009). This is particularly true in more severe cases of mitochondrial respiration, which involves the production of H2O2 (a major endogenous ROS) following electron leakage from the ETC (Chen et al., 2009). However, despite the potential of TH-induced mitochondrial biogenesis to induce such ROS-mediated damage, T3 protects from H2O2-induced oxidative stress via the PI3K-Akt signaling pathway through inhibition of mitochondrial ROS over-production both in vivo and in vitro (Zeng et al., 2019). Furthermore, T3-induced redox activation of c-Jun N-terminal kinase 2 alpha 2 (JNK2α2), which is a JNK member of the MAPK family, results in enhanced IGF-1 expression and activation of proliferative ERK1/2 signaling (Tan et al., 2019). Therefore, it is plausible that a non-canonical pathway activated by thyromimetic small molecules can induce OL proliferation. Actions of TH and thyromimetics, in the context of OL oxidative stress and mitochondrial biogenesis, may therefore drive remyelination by eliciting Akt/mTOR signaling and permit myelin biogenesis, neurorepair, and neuroprotection.
Treatment for myelin diseases of the brain using thyroid hormone and thyromimetic analogs
Prior to neurotherapeutic advancements with thyromimetic analogs that include but are not limited to rT3, Sobetirome, tetraiodothyroacteic acid (TETRAC), triiodothyroacetic acid (TRIAC), and DITPA (Senese et al., 2019), individuals that presented with clinical symptoms related to TH deficiency or thyrotoxicosis were treated with TH to re-establish homeostasis of the HPT axis to reinstate proper functioning of tissues in the body (Wooliscroft et al., 2020).
In the context of neurodegenerative diseases where demyelinating events are prominent, provision of T3 and T4 were considered potential therapeutic interventions given their role in promoting myelination (Bernal, 2005). In cases related to local hypothyroidism and DIO2 dysregulation, peripheral immune cells secrete pro-inflammatory cytokines, such as interleukin-1β (IL-1β) and interferon-γ (IFN-γ), and cause an overall decrease of TH in the CNS that inhibits the differentiation of OLs (Merrill, 1991; Andrews et al., 1998; Boelen et al., 2004). Additionally, local administration of nanoparticles coated with TH were shown to reduce side-effects in the peripheral immune system and promote remyelination in the CNS (Mdzinarishvili et al., 2013).
In the EAE model of MS, the subcutaneous administration of T4 or T3 was demonstrated to ameliorate neurological deficits associated with the disease after onset (Fernandez et al., 2004; Calza et al., 2002; D'Intino et al., 2011). Similarly, in an EAE model using dark agouti rats co-treated with valproic acid, HDAC inhibitor, and T4 provision, there was an elevation in the expression of several myelin genes in O4+ (a surface antigen present in OLs) pre-OLs of the brain during the remission stage of EAE (Castelo-Branco et al., 2014b). Furthermore, a T4 treatment in rabbits (Vose et al., 2013) and rats (Hung et al., 2013) models associated with white matter damage predisposed to intraventricular hemorrhage and hypoxic ischemia-induced demyelination and inflammation, revealed an increase in myelination during early postnatal stages. These data were also supported by immunohistochemical analysis showing an increase in MBP expression after T4 and T3 administration and the promotion of increased myelination, which is a neuroprotective mechanism under neuroinflammatory conditions (Fernandez et al., 2004; Calza et al., 2002; D'Intino et al., 2011). The number of O4+/O1+ mature OLs increased via T4 administration in pre-term infants with intraventricular hemorrhage (IVH) compared to controls (Vose et al., 2013). Moreover, T3 administration through intraperitoneal (Harsan et al., 2008), subcutaneous (Franco et al., 2008) or intranasal (Silvestroff et al., 2012) delivery promoted remyelination in an MS-like animal model, i.e., the cuprizone-induced neurotoxicant model of demyelination and remyelination. Therefore, the preservation of OLs and remyelination may be a therapeutic option for repair of the CNS through TH administration (Sher et al., 2008; Kotter et al., 2011).
The development of TH analogs with thyromimetic effects in the periphery and CNS without off-target adverse events are essential to address therapeutic gaps in neuroprotection and repair related to TH signaling in white matter diseases.
The TH analog TRIAC is a natural metabolite found in the human body in lower concentrations than T3 (Menegay et al., 1989). Originally tested in male MCT8−/− mice, TRIAC was administered to postnatal day 1–12 mice in doses between 50 and 400 ng/g/bodyweight. Results from this study demonstrated increased TH-dependent differentiation of neural precursors and cortical myelination (Horn et al., 2013a; Kersseboom et al., 2014). Studies assessing the safety of TRIAC administration were validated in TH-resistant patients by delivering 38.3 µg/kg/body weight to overcome thyrotoxicosis in male children with MCT8 deficiency (Groeneweg et al., 2017). However, studies by Groeneweg et al. (2017) and Anzai et al. (2012) also revealed a therapeutic caveat of TRIAC indicating that its half-life in humans was limited to just 6 h. As a corollary, TRIAC administration in mice deficient for MCT8 cannot upregulate thyroid hormone-responsive genes in the cerebral cortex and striatum, which suggests that despite the peripheral effects of this drug being clinically proven, it may be ineffective in promoting thyroid hormone-dependent signaling in the brain (Bárez-López et al., 2016).
Another TH analog, TETRAC, can also enter cells independent of MCT8. It was previously reported in studies of male MCT8−/− mice that a daily injectable dose of TETRAC (400 ng/kg/body weight) in the first week during neonatal development successfully promoted neuronal development in the cerebral cortex, cerebellum, and striatum (Horn et al., 2013b). Unfortunately, from a translational standpoint, there is still limited pre-clinical evidence related to the mechanism of action, suggesting that TETRAC can be considered a safe and efficacious treatment option.
The synthetic TH analog DITPA has also been shown to enter cells in the brain independent of MCT8 and there is promising data indicating that DITPA can overcome MCT8 deficiencies (Lee et al., 2017). Additionally, it has been demonstrated that DITPA can successfully cross restrictive barriers such as the BBB and placenta, which make it an ideal candidate for antenatal treatment (Di Cosmo et al., 2009; Ferrara et al., 2014). In a noteworthy clinical trial of 4 children aged 8–25 months with MCT8 mutations, DITPA was administered in a dosing regimen starting at 1.8 mg/day and increasing to 30 mg/day (during 26–40 months). Results from this trial indicated that DITPA dosed at 1.2 mg/kg/day restored myelin to normal levels, improved weight gain, and sustained normal thyroid function. Additionally, there were no reports of hypothyroidism, thyrotoxicity, or other adverse events (Verge et al., 2012). These findings from pre-clinical in vitro and in vivo studies (Lee et al., 2017) support DITPA as an ideal candidate in treating white matter disorders associated by OL loss and/or dysmyelination, which occurs in the evolution of MS lesions.
TH analogs that do not require MCT8 have been suggested as a potential therapy to treat the X-linked psychomotor disorder Allan-Herndon-Dudley syndrome (AHDS). For example, DITPA can normalize peripheral hyperthyroidism and reduce hypermetabolism in AHDS patients. However, the exact mechanism by which DITPA acts is largely unknown. In the last few years, the off-label clinical trial (on compassionate grounds) reported by Verge et al. (2012) administered DITPA with an increasing dosage to a final maximum dose of 2–2.4 mg/kg per day over 15–29 months of treatment to 4 boys with AHDS (2 of which were monozygotic twin boys) and that ranged from 8.5 to 25 months of age. The analysis of these four boys over the 29-month treatment duration exhibited late myelination in the twin boys defined by repeat MRI imaging at 47 months-of-age. Outside beneficial normalization of hyperthyroid levels in the periphery, significant physiological changes were observed that included no weight loss and reduced sex hormone-binding globulin (SHBG) and heart rate. None of the children developed seizures or required assisted feeding through a gastric tube. However, a phase II clinical trial initiated by the Veterans Affairs Office of Research and Development (NCT00032643) in a randomized double blind interventional study for post-ischemic heart failure did report non-compliance in the DITPA treatment group with only 21 patients completing the study. This study reported adverse effects in this cohort of patients due to the clinical trial design that incorporated an increasing dose over the 24 week treatment period. This clinical trial highlighted the need for lower doses to ensure patient compliance and safety, but the therapeutic dose required to demonstrate efficacy for CNS pathology requires further elucidation.
Conclusion
MS is a chronic, neurodegenerative disease that is characterized by autoimmune inflammatory lesions located within the CNS. However, the etiology of MS is not well defined, and current therapeutic approaches that are effective in MS treatment can only address the relapse rate in individuals living with MS. However, recent research has determined that drugs that target and elicit TH-dependent mechanisms within OLs may hold clinical promise during acute inflammatory demyelination by independently activating these mature cells along with their lineage precursor population. Due to their ability to bypass the monocarboxylate transporter 8, TH analogs such as TRIAC, TETRAC, and DITPA may be effective at promoting mature PLP+ mature oligodendrocyte neuroprotection and may potentiate the migration of OPCs that display acute downregulation of MCT8 as a consequence of neuroinflammatory disease. Moreover, oligodendroglial cells that are targeted through TH analogs may occur through the activation of the downstream P-Akt/P-mTOR pathway that promotes oligodendroglial cell viability and maturation. Neurological recovery following TH analog administration that is a direct result of the preservation of oligodendrocytes and/or remyelination needs to be clearly documented in the context of a neuroinflammatory challenge. Importantly, we describe an alternate TH-dependent mechanism of action in targeted oligodendroglial cells by outlining the binding and activation through integrin receptors to signal through the non-genomic PI3K/Akt/mTOR pathway facilitating cell viability and maturation. Further studies defining the function of MCT8 during the mobilization and differentiation of OPCs during neuroinflammation are required and may identify how OPCs can be metabolically salvaged and stimulate differentiation during MS and other diseases with profound myelin pathology. These approaches modify the TH-dependent non-genomic signaling pathways and may permit endogenous repair of demyelinated lesions, and ultimately, potentiate neurological recovery.
Author contributions
RE, MD, and MM wrote the manuscript and generated the figures. SP, PT, NG, MP, SB and SY edited the manuscript. SP generated the conceptual framework of the manuscript.
Conflict of interest
SP is the founder and Chief Scientific Officer of the start-up company NeuOrphan Pty Ltd. (currently 50% shareholder) that will be commercializing the development of DITPA-like molecules in collaboration with Monash University.
The remaining authors declare that the research was conducted in the absence of any commercial or financial relationships that could be construed as a potential conflict of interest.
Publisher’s note
All claims expressed in this article are solely those of the authors and do not necessarily represent those of their affiliated organizations, or those of the publisher, the editors, or reviewers. Any product that may be evaluated in this article or claim that may be made by its manufacturer is not guaranteed or endorsed by the publisher.
References
Absinta, M., Sati, P., Masuzzo, F., Nair, G., Sethi, V., Kolb, H., et al. (2019). Association of chronic active multiple sclerosis lesions with disability in vivo. JAMA Neurol. 76 (12), 1474–1483. doi:10.1001/jamaneurol.2019.2399
Andrews, T., Zhang, P., and Bhat, N. R. (1998). TNFalpha potentiates IFNgamma-induced cell death in oligodendrocyte progenitors. J. Neurosci. Res. 54 (5), 574–583. doi:10.1002/(SICI)1097-4547(19981201)54:5<574::AID-JNR2>3.0.CO;2-0
Anzai, R., Adachi, M., Sho, N., Muroya, K., Asakura, Y., and Onigata, K. (2012). Long-term 3, 5, 3′-triiodothyroacetic acid therapy in a child with hyperthyroidism caused by thyroid hormone resistance: Pharmacological study and therapeutic recommendations. Thyroid 22 (10), 1069–1075. doi:10.1089/thy.2011.0450
Armour, C. M., Kersseboom, S., Yoon, G., and Visser, T. J. (2015). Further insights into the allan-herndon-dudley syndrome: Clinical and functional characterization of a novel MCT8 mutation. PLOS ONE 10 (10), e0139343. doi:10.1371/journal.pone.0139343
Baas, D., Bourbeau, D., Sarlieve, L. L., Ittel, M. E., Dussault, J. H., and Puymirat, J. (1997). Oligodendrocyte maturation and progenitor cell proliferation are independently regulated by thyroid hormone. Glia 19 (4), 324–332. doi:10.1002/(sici)1098-1136(199704)19:4<324::aid-glia5>3.0.co;2-x
Baas, D., Legrand, C., Samarut, J., and Flamant, F. (2002). Persistence of oligodendrocyte precursor cells and altered myelination in optic nerve associated to retina degeneration in mice devoid of all thyroid hormone receptors. Proc. Natl. Acad. Sci. U. S. A. 99 (5), 2907–2911. doi:10.1073/pnas.052482299
Baas, D., Puymirat, J., and Sarliève, L. L. (1998). Posttranscriptional regulation of oligodendroglial thyroid hormone (T3) receptor beta 1 by T3. Int. J. Dev. Neurosci. 16 (6), 461–467. doi:10.1016/s0736-5748(98)00053-7
Ballinger, S. W., Patterson, C., Yan, C-N., Doan, R., Burow, D. L., Young, C. G., et al. (2000). Hydrogen peroxide– and peroxynitrite-induced mitochondrial DNA damage and dysfunction in vascular endothelial and smooth muscle cells. Circulation Res. 86 (9), 960–966. doi:10.1161/01.res.86.9.960
Barczyk, M., Carracedo, S., and Gullberg, D. (2010). Integrins.. Cell Tissue Res. 339 (1), 269–280. doi:10.1007/s00441-009-0834-6
Bárez-López, S., Obregon, M. J., Martínez-de-Mena, R., Bernal, J., Guadaño-Ferraz, A., and Morte, B. (2016). Effect of triiodothyroacetic acid treatment in Mct8 deficiency: A word of caution. Thyroid 26 (5), 618–626. doi:10.1089/thy.2015.0388
Baron, W., Metz, B., Bansal, R., Hoekstra, D., and de Vries, H. (2000). PDGF and FGF-2 signaling in oligodendrocyte progenitor cells: Regulation of proliferation and differentiation by multiple intracellular signaling pathways. Mol. Cell. Neurosci. 15 (3), 314–329. doi:10.1006/mcne.1999.0827
Benninger, Y., Colognato, H., Thurnherr, T., Franklin, R. J., Leone, D. P., Atanasoski, S., et al. (2006). Beta1-integrin signaling mediates premyelinating oligodendrocyte survival but is not required for CNS myelination and remyelination. J. Neurosci. 26 (29), 7665–7673. doi:10.1523/JNEUROSCI.0444-06.2006
Bercury, K. K., Dai, J., Sachs, H. H., Ahrendsen, J. T., Wood, T. L., and Macklin, W. B. (2014). Conditional ablation of raptor or rictor has differential impact on oligodendrocyte differentiation and CNS myelination. J. Neurosci. 34 (13), 4466–4480. doi:10.1523/JNEUROSCI.4314-13.2014
Bergh, J. J., Lin, H-Y., Lansing, L., Mohamed, S. N., Davis, F. B., Mousa, S., et al. (2005). Integrin alphaVbeta3 contains a cell surface receptor site for thyroid hormone that is linked to activation of mitogen-activated protein kinase and induction of angiogenesis.. Endocrinology 146 (7), 2864–2871. doi:10.1210/en.2005-0102
Bernal, J. (2000). “Thyroid hormones in brain development and function,” in Endotext. L. J. De Groot, G. Chrousos, K. Dungan, K. R. Feingold, A. Grossman, J. M. Hershmanet al. (South Dartmouth (MA.
Bernal, J. (2006). Role of monocarboxylate anion transporter 8 (MCT8) in thyroid hormone transport: Answers from mice. Endocrinology 147 (9), 4034–4035. doi:10.1210/en.2006-0695
Bernal, J. (2007). Thyroid hormone receptors in brain development and function. Nat. Clin. Pract. Endocrinol. Metab. 3 (3), 249–259. doi:10.1038/ncpendmet0424
Bernal, J. (2005)., 71. Academic Press, 95–122.Thyroid hormones and brain developmentVitamins Hormones.
Bieber, A. J., Kerr, S., and Rodriguez, M. (2003). Efficient central nervous system remyelination requires T cells. Ann. Neurol. 53 (5), 680–684. doi:10.1002/ana.10578
Billon, N., Jolicoeur, C., Tokumoto, Y., Vennström, B., and Raff, M. (2002). Normal timing of oligodendrocyte development depends on thyroid hormone receptor alpha 1 (TRalpha1). EMBO J. 21 (23), 6452–6460. doi:10.1093/emboj/cdf662
Billon, N., Tokumoto, Y., Forrest, D., and Raff, M. (2001). Role of thyroid hormone receptors in timing oligodendrocyte differentiation. Dev. Biol. 235 (1), 110–120. doi:10.1006/dbio.2001.0293
Boelen, A., Kwakkel, J., Platvoet-ter Schiphorst, M., Baur, A., Köhrle, J., and Wiersinga, W. M. (2004). Contribution of interleukin-12 to the pathogenesis of non-thyroidal illness. Horm. Metab. Res. 36 (2), 101–106. doi:10.1055/s-2004-814219
Bonnans, C., Chou, J., and Werb, Z. (2014). Remodelling the extracellular matrix in development and disease. Nat. Rev. Mol. Cell Biol. 15 (12), 786–801. doi:10.1038/nrm3904
Brent, G. A. (2012). Mechanisms of thyroid hormone action. J. Clin. Invest.. 122 (9), 3035–3043. doi:10.1172/JCI60047
Bury, F., Carré, J-L., Vega, S., Ghandour, M. S., Rodriguez-Peña, A., Langley, K., et al. (2002). Coexpression of thyroid hormone receptor isoforms in mouse oligodendrocytes. J. Neurosci. Res. 67 (1), 106–113. doi:10.1002/jnr.10111
Butti, E., Bacigaluppi, M., Chaabane, L., Ruffini, F., Brambilla, E., Berera, G., et al. (2019). Neural stem cells of the subventricular zone contribute to neuroprotection of the corpus callosum after cuprizone-induced demyelination. J. Neurosci. 39 (28), 5481–5492. doi:10.1523/JNEUROSCI.0227-18.2019
Calza, L., Fernandez, M., Giuliani, A., Aloe, L., and Giardino, L. (2002). Thyroid hormone activates oligodendrocyte precursors and increases a myelin-forming protein and NGF content in the spinal cord during experimental allergic encephalomyelitis. Proc. Natl. Acad. Sci. U. S. A. 99 (5), 3258–3263. doi:10.1073/pnas.052704499
Campbell, I. D., and Humphries, M. J. (2011). Integrin structure, activation, and interactions. Cold Spring Harb. Perspect. Biol. 3 (3), a004994. doi:10.1101/cshperspect.a004994
Cao, X., Kambe, F., Moeller, L. C., Refetoff, S., and Seo, H. (2005). Thyroid hormone induces rapid activation of akt/protein kinase B-mammalian target of rapamycin-p70S6K cascade through phosphatidylinositol 3-kinase in human fibroblasts. Mol. Endocrinol. 19 (1), 102–112. doi:10.1210/me.2004-0093
Castelo-Branco, G., Lilja, T., Wallenborg, K., Falcão, A. M., Marques, S. C., Gracias, A., et al. (2014). Neural stem cell differentiation is dictated by distinct actions of nuclear receptor corepressors and histone deacetylases. Stem Cell Rep. 3 (3), 502–515. doi:10.1016/j.stemcr.2014.07.008
Castelo-Branco, G., Stridh, P., Guerreiro-Cacais, A. O., Adzemovic, M. Z., Falcão, A. M., Marta, M., et al. (2014). Acute treatment with valproic acid and l-thyroxine ameliorates clinical signs of experimental autoimmune encephalomyelitis and prevents brain pathology in DA rats. Neurobiol. Dis. 71, 220–233. doi:10.1016/j.nbd.2014.08.019
Ceballos, A., Belinchon, M. M., Sanchez-Mendoza, E., Grijota-Martinez, C., Dumitrescu, A. M., Refetoff, S., et al. (2009). Importance of monocarboxylate transporter 8 for the blood-brain barrier-dependent availability of 3, 5, 3′-Triiodo-l-Thyronine. Endocrinology 150 (5), 2491–2496. doi:10.1210/en.2008-1616
Chan, A., Hummel, V., Weilbach, F. X., Kieseier, B. C., and Gold, R. (2006). Phagocytosis of apoptotic inflammatory cells downregulates microglial chemoattractive function and migration of encephalitogenic T cells. J. Neurosci. Res. 84 (6), 1217–1224. doi:10.1002/jnr.21029
Chan, C. B., Liu, X., Zhao, L., Liu, G., Lee, C. W., Feng, Y., et al. (2014). PIKE is essential for oligodendroglia development and CNS myelination. Proc. Natl. Acad. Sci. U. S. A. 111 (5), 1993–1998. doi:10.1073/pnas.1318185111
Chari, D. M., Zhao, C., Kotter, M. R., Blakemore, W. F., and Franklin, R. J. (2006). Corticosteroids delay remyelination of experimental demyelination in the rodent central nervous system. J. Neurosci. Res. 83 (4), 594–605. doi:10.1002/jnr.20763
Chen, Y., Azad, M. B., and Gibson, S. B. (2009). Superoxide is the major reactive oxygen species regulating autophagy. Cell Death Differ. 16 (7), 1040–1052. doi:10.1038/cdd.2009.49
Cheng, S-Y., Leonard, J. L., and Davis, P. J. (2010). Molecular aspects of thyroid hormone actions. Endocr. Rev. 31 (2), 139–170. doi:10.1210/er.2009-0007
Clarner, T., Parabucki, A., Beyer, C., and Kipp, M. (2011). Corticosteroids impair remyelination in the corpus callosum of cuprizone-treated mice. J. Neuroendocrinol. 23 (7), 601–611. doi:10.1111/j.1365-2826.2011.02140.x
Colognato, H., Baron, W., Avellana-Adalid, V., Relvas, J. B., Baron-Van Evercooren, A., Georges-Labouesse, E., et al. (2002). CNS integrins switch growth factor signalling to promote target-dependent survival. Nat. Cell Biol. 4 (11), 833–841. doi:10.1038/ncb865
Corcoran, S. E., and O’Neill, L. A. J. (2016). HIF1α and metabolic reprogramming in inflammation. J. Clin. Invest.. 126 (10), 3699–3707. doi:10.1172/JCI84431
Croteau, W., Davey, J. C., Galton, V. A., and St Germain, D. L. (1996). Cloning of the mammalian type II iodothyronine deiodinase. A selenoprotein differentially expressed and regulated in human and rat brain and other tissues. J. Clin. Invest.. 98 (2), 405–417. doi:10.1172/JCI118806
D'Intino, G., Lorenzini, L., Fernandez, M., Taglioni, A., Perretta, G., Del Vecchio, G., et al. (2011). Triiodothyronine administration ameliorates the demyelination/remyelination ratio in a non-human primate model of multiple sclerosis by correcting tissue hypothyroidism. J. Neuroendocrinol. 23 (9), 778–790. doi:10.1111/j.1365-2826.2011.02181.x
Dalton, D. K., and Wittmer, S. (2005). Nitric-oxide-dependent and independent mechanisms of protection from CNS inflammation during Th1-mediated autoimmunity: Evidence from EAE in iNOS KO mice. J. Neuroimmunol. 160 (1), 110–121. doi:10.1016/j.jneuroim.2004.11.004
Davies, A. L., Desai, R. A., Bloomfield, P. S., McIntosh, P. R., Chapple, K. J., Linington, C., et al. (2013). Neurological deficits caused by tissue hypoxia in neuroinflammatory disease. Ann. Neurol. 74 (6), 815–825. doi:10.1002/ana.24006
Davis, P. J., Mousa, S. A., and Lin, H. Y. (2021). Nongenomic actions of thyroid hormone: The integrin component. Physiol. Rev. 101 (1), 319–352. doi:10.1152/physrev.00038.2019
De Paula, M. L., Cui, Q-L., Hossain, S., Antel, J., and Almazan, G. (2014). The PTEN inhibitor bisperoxovanadium enhances myelination by amplifying IGF-1 signaling in rat and human oligodendrocyte progenitors. Glia 62 (1), 64–77. doi:10.1002/glia.22584
Dell'Acqua, M. L., Lorenzini, L., D'Intino, G., Sivilia, S., Pasqualetti, P., Panetta, V., et al. (2012). Functional and molecular evidence of myelin- and neuroprotection by thyroid hormone administration in experimental allergic encephalomyelitis. Neuropathol. Appl. Neurobiol. 38 (5), 454–470. doi:10.1111/j.1365-2990.2011.01228.x
Di Cosmo, C., Liao, X-H., Dumitrescu, A. M., Weiss, R. E., and Refetoff, S. (2009). A thyroid hormone analog with reduced dependence on the monocarboxylate transporter 8 for tissue transport. Endocrinology 150 (9), 4450–4458. doi:10.1210/en.2009-0209
Ding, Z., Dai, C., Zhong, L., Liu, R., Gao, W., Zhang, H., et al. (2021). Neuregulin-1 converts reactive astrocytes toward oligodendrocyte lineage cells via upregulating the PI3K-AKT-mTOR pathway to repair spinal cord injury. Biomed. Pharmacother. 134, 111168. doi:10.1016/j.biopha.2020.111168
Dong, H., Yauk, C. L., Rowan-Carroll, A., You, S-H., Zoeller, R. T., Lambert, I., et al. (2009). Identification of thyroid hormone receptor binding sites and target genes using ChIP-on-chip in developing mouse cerebellum. PloS one 4 (2), e4610. doi:10.1371/journal.pone.0004610
Dorn, G. W., and Force, T. (2005). Protein kinase cascades in the regulation of cardiac hypertrophy. J. Clin. Invest.. 115 (3), 527–537. doi:10.1172/JCI24178
Fancy, S. P., Zhao, C., and Franklin, R. J. (2004). Increased expression of Nkx2.2 and Olig2 identifies reactive oligodendrocyte progenitor cells responding to demyelination in the adult CNS. Mol. Cell. Neurosci. 27 (3), 247–254. doi:10.1016/j.mcn.2004.06.015
Feinstein, A., Freeman, J., and Lo, A. C. (2015). Treatment of progressive multiple sclerosis: What works, what does not, and what is needed. Lancet. Neurol. 14 (2), 194–207. doi:10.1016/S1474-4422(14)70231-5
Feltri, M. L., Porta, D. G., Previtali, S. C., Nodari, A., Migliavacca, B., Cassetti, A., et al. (2002). Conditional disruption of β1 integrin in Schwann cells impedes interactions with axons. J. Cell Biol. 156 (1), 199–209. doi:10.1083/jcb.200109021
Fernandez, M., Giuliani, A., Pirondi, S., D'Intino, G., Giardino, L., Aloe, L., et al. (2004). Thyroid hormone administration enhances remyelination in chronic demyelinating inflammatory disease. Proc. Natl. Acad. Sci. U. S. A. 101 (46), 16363–16368. doi:10.1073/pnas.0407262101
Ferrara, A. M., Liao, X-H., Gil-Ibáñez, P., Bernal, J., Weiss, R. E., Dumitrescu, A. M., et al. (2014). Placenta passage of the thyroid hormone analog DITPA to male wild-type and mct8-deficient mice. Endocrinology 155 (10), 4088–4093. doi:10.1210/en.2014-1085
Ferrara, A. M., Liao, X-H., Ye, H., Weiss, R. E., Dumitrescu, A. M., and Refetoff, S. (2015). The thyroid hormone analog DITPA ameliorates metabolic parameters of male mice with Mct8 deficiency. Endocrinology 156 (11), 3889–3894. doi:10.1210/en.2015-1234
Ferrara, S. J., Chaudhary, P., DeBell, M. J., Marracci, G., Miller, H., Calkins, E., et al. (2022). TREM2 is thyroid hormone regulated making the TREM2 pathway druggable with ligands for thyroid hormone receptor. Cell Chem. Biol. 29 (2), 239–248.e4. e4. doi:10.1016/j.chembiol.2021.07.014
Flores, A. I., Narayanan, S. P., Morse, E. N., Shick, H. E., Yin, X., Kidd, G., et al. (2008). Constitutively active Akt induces enhanced myelination in the CNS. J. Neurosci. 28 (28), 7174–7183. doi:10.1523/JNEUROSCI.0150-08.2008
Franco, P. G., Silvestroff, L., Soto, E. F., and Pasquini, J. M. (2008). Thyroid hormones promote differentiation of oligodendrocyte progenitor cells and improve remyelination after cuprizone-induced demyelination. Exp. Neurol. 212 (2), 458–467. doi:10.1016/j.expneurol.2008.04.039
Friesema, E. C., Jansen, J., Jachtenberg, J. W., Visser, W. E., Kester, M. H., and Visser, T. J. (2008). Effective cellular uptake and efflux of thyroid hormone by human monocarboxylate transporter 10. Mol. Endocrinol. 22 (6), 1357–1369. doi:10.1210/me.2007-0112
Friesema, E. C. H., Ganguly, S., Abdalla, A., Fox, J. E. M., Halestrap, A. P., and Visser, T. J. (2003). Identification of monocarboxylate transporter 8 as a specific thyroid hormone transporter. J. Biol. Chem. 278 (41), 40128–40135. doi:10.1074/jbc.M300909200
Friesema, E. C. H., Grueters, A., Biebermann, H., Krude, H., von Moers, A., Reeser, M., et al. (2004). Association between mutations in a thyroid hormone transporter and severe X-linked psychomotor retardation. Lancet 364 (9443), 1435–1437. doi:10.1016/S0140-6736(04)17226-7
Frost, E. E., Buttery, P. C., Milner, R., and ffrench-Constant, C. (1999). Integrins mediate a neuronal survival signal for oligodendrocytes. Curr. Biol. 9 (21), 1251–1254. doi:10.1016/s0960-9822(99)80506-5
Fünfschilling, U., Supplie, L. M., Mahad, D., Boretius, S., Saab, A. S., Edgar, J., et al. (2012). Glycolytic oligodendrocytes maintain myelin and long-term axonal integrity. Nature 485 (7399), 517–521. doi:10.1038/nature11007
Gaesser, J. M., and Fyffe-Maricich, S. L. (2016). Intracellular signaling pathway regulation of myelination and remyelination in the CNS. Exp. Neurol. 283, 501–511. doi:10.1016/j.expneurol.2016.03.008
Goebbels, S., Oltrogge, J. H., Kemper, R., Heilmann, I., Bormuth, I., Wolfer, S., et al. (2010). Elevated phosphatidylinositol 3, 4, 5-trisphosphate in glia triggers cell-autonomous membrane wrapping and myelination. J. Neurosci. 30 (26), 8953–8964. doi:10.1523/JNEUROSCI.0219-10.2010
Goldenthal, M. J., Weiss, H. R., and Marín-García, J. (2004). Bioenergetic remodeling of heart mitochondria by thyroid hormone. Mol. Cell. Biochem. 265 (1-2), 97–106. doi:10.1023/b:mcbi.0000044321.17680.a2
Groeneweg, S., Peeters, R. P., Visser, T. J., and Visser, W. E. (2017). Triiodothyroacetic acid in health and disease. J. Endocrinol. 234 (2), R99–R121. doi:10.1530/JOE-17-0113
Guadaño-Ferraz, A., Escámez, M. J., Rausell, E., Bernal, J., and GuAdAno-FerrAz, A. (1999). Expression of type 2 iodothyronine deiodinase in hypothyroid rat brain indicates an important role of thyroid hormone in the development of specific primary sensory systems. J. Neurosci. 19 (9), 3430–3439. doi:10.1523/jneurosci.19-09-03430.1999
Guadaño-Ferraz, A., Obregón, M. J., St Germain, D. L., Bernal, J., and GuAdAno-FerrAz, A. (1997). The type 2 iodothyronine deiodinase is expressed primarily in glial cells in the neonatal rat brain. Proc. Natl. Acad. Sci. U. S. A. 94 (19), 10391–10396. doi:10.1073/pnas.94.19.10391
Guertin, D. A., Stevens, D. M., Thoreen, C. C., Burds, A. A., Kalaany, N. Y., Moffat, J., et al. (2006). Ablation in mice of the mTORC components raptor, rictor, or mLST8 reveals that mTORC2 is required for signaling to Akt-FOXO and PKCalpha, but not S6K1. Dev. Cell 11 (6), 859–871. doi:10.1016/j.devcel.2006.10.007
Harsan, L. A., Steibel, J., Zaremba, A., Agin, A., Sapin, R., Poulet, P., et al. (2008). Recovery from chronic demyelination by thyroid hormone therapy: Myelinogenesis induction and assessment by diffusion tensor magnetic resonance imaging. J. Neurosci. 28 (52), 14189–14201. doi:10.1523/JNEUROSCI.4453-08.2008
Hartley, M. D., Banerji, T., Tagge, I. J., Kirkemo, L. L., Chaudhary, P., Calkins, E., et al. (2019). Myelin repair stimulated by CNS-selective thyroid hormone action. JCI Insight 4 (8), 126329. doi:10.1172/jci.insight.126329
Hartline, D. K., and Colman, D. R. (2007). Rapid conduction and the evolution of giant axons and myelinated fibers. Curr. Biol. 17 (1), R29–R35. doi:10.1016/j.cub.2006.11.042
Hauser, S. L., Chan, J. R., and Oksenberg, J. R. (2013). Multiple sclerosis: Prospects and promise. Ann. Neurol. 74 (3), 317–327. doi:10.1002/ana.24009
Heuer, H., Maier, M. K., Iden, S., Mittag, J., Friesema, E. C., Visser, T. J., et al. (2005). The monocarboxylate transporter 8 linked to human psychomotor retardation is highly expressed in thyroid hormone-sensitive neuron populations. Endocrinology 146 (4), 1701–1706. doi:10.1210/en.2004-1179
Horn, S., Kersseboom, S., Mayerl, S., Muller, J., Groba, C., Trajkovic-Arsic, M., et al. (2013). Tetrac can replace thyroid hormone during brain development in mouse mutants deficient in the thyroid hormone transporter mct8. Endocrinology 154 (2), 968–979. doi:10.1210/en.2012-1628
Horn, S., Kersseboom, S., Mayerl, S., Müller, J., Groba, C., Trajkovic-Arsic, M., et al. (2013). Tetrac can replace thyroid hormone during brain development in mouse mutants deficient in the thyroid hormone transporter Mct8. Endocrinology 154 (2), 968–979. doi:10.1210/en.2012-1628
Hsu, J. H., Zavacki, A. M., Harney, J. W., and Brent, G. A. (1995). Retinoid-X receptor (RXR) differentially augments thyroid hormone response in cell lines as a function of the response element and endogenous RXR content. Endocrinology 136 (2), 421–430. doi:10.1210/endo.136.2.7835272
Hughes, E. G., and Appel, B. (2016). The cell biology of CNS myelination. Curr. Opin. Neurobiol. 39, 93–100. doi:10.1016/j.conb.2016.04.013
Hung, P-L., Huang, C-C., Huang, H-M., Tu, D-G., and Chang, Y-C. (2013). Thyroxin treatment protects against white matter injury in the immature brain via brain-derived neurotrophic factor. Stroke 44 (8), 2275–2283. doi:10.1161/STROKEAHA.113.001552
Hung, P. L., Hsu, M. H., Yu, H. R., Wu, K. L. H., and Wang, F. S. (2018). Thyroxin protects white matter from hypoxic-ischemic insult in the immature Sprague⁻Dawley rat brain by regulating periventricular white matter and cortex BDNF and CREB pathways. Int. J. Mol. Sci. 19 (9), E2573. doi:10.3390/ijms19092573
Hynes, R. O. (2002). Integrins: Bidirectional, allosteric signaling machines. Cell 110 (6), 673–687. doi:10.1016/s0092-8674(02)00971-6
Hynes, R. O. (2009). The extracellular matrix: Not just pretty fibrils. Sci. (New York, NY) 326 (5957), 1216–1219. doi:10.1126/science.1176009
Jones, I., Srinivas, M., Ng, L., and Forrest, D. (2003). The thyroid hormone receptor beta gene: Structure and functions in the brain and sensory systems. Thyroid 13 (11), 1057–1068. doi:10.1089/105072503770867228
Kaplan, M. M., and Shaw, E. A. (1984). Type II iodothyronine 5'-deiodination by human and rat placenta in vitro. J. Clin. Endocrinol. Metab. 59 (2), 253–257. doi:10.1210/jcem-59-2-253
Kerp, H., Hönes, G. S., Tolstik, E., Hönes-Wendland, J., Gassen, J., Moeller, L. C., et al. (2021). Protective effects of thyroid hormone deprivation on progression of maladaptive cardiac hypertrophy and heart failure. Front. Cardiovasc. Med. 8, 683522. doi:10.3389/fcvm.2021.683522
Kersseboom, S., Horn, S., Visser, W. E., Chen, J., Friesema, E. C. H., Vaurs-Barrière, C., et al. (2014). In vitro and mouse studies supporting therapeutic utility of triiodothyroacetic acid in MCT8 deficiency.. Mol. Endocrinol. 28 (12), 1961–1970. doi:10.1210/me.2014-1135
Kim, M. J., and Petratos, S. (2019). Oligodendroglial lineage cells in thyroid hormone-deprived conditions. Stem Cells Int. 2019, 5496891. doi:10.1155/2019/5496891
Kinne, A., Kleinau, G., Hoefig, C. S., Grüters, A., Köhrle, J., Krause, G., et al. (2010). Essential molecular determinants for thyroid hormone transport and first structural implications for monocarboxylate transporter 8. J. Biol. Chem. 285 (36), 28054–28063. doi:10.1074/jbc.M110.129577
Kopp, P. (2001). The TSH receptor and its role in thyroid disease. Cell. Mol. Life Sci. 58 (9), 1301–1322. doi:10.1007/pl00000941
Kotra, L. P., and Park, J. (2017). “5.14 - therapeutic approaches to MS and other neurodegenerative diseases,” in Comprehensive medicinal chemistry III. Editors S. Chackalamannil, D. Rotella, and S. E. Ward (Oxford: Elsevier), 439–473.
Kotter, M. R., Setzu, A., Sim, F. J., Van Rooijen, N., and Franklin, R. J. (2001). Macrophage depletion impairs oligodendrocyte remyelination following lysolecithin-induced demyelination. Glia 35 (3), 204–212. doi:10.1002/glia.1085
Kotter, M. R., Stadelmann, C., and Hartung, H. P. (2011). Enhancing remyelination in disease--can we wrap it up? Brain 134 (7), 1882–1900. doi:10.1093/brain/awr014
Kuzman, J. A., O'Connell, T. D., and Gerdes, A. M. (2007). Rapamycin prevents thyroid hormone-induced cardiac hypertrophy. Endocrinology 148 (7), 3477–3484. doi:10.1210/en.2007-0099
Lamming, D. W., Ye, L., Katajisto, P., Goncalves, M. D., Saitoh, M., Stevens, D. M., et al. (2012). Rapamycin-induced insulin resistance is mediated by mTORC2 loss and uncoupled from longevity. Science 335 (6076), 1638–1643. doi:10.1126/science.1215135
Larsen, P. R., Silva, J. E., and Kaplan, M. M. (1981). Relationships between circulating and intracellular thyroid hormones: Physiological and clinical implications. Endocr. Rev. 2 (1), 87–102. doi:10.1210/edrv-2-1-87
Lebel, C., and Beaulieu, C. (2011). Longitudinal development of human brain wiring continues from childhood into adulthood. J. Neurosci. 31 (30), 10937–10947. doi:10.1523/JNEUROSCI.5302-10.2011
Lebrun-Julien, F., Bachmann, L., Norrmén, C., Trötzmüller, M., Köfeler, H., Rüegg, M. A., et al. (2014). Balanced mTORC1 activity in oligodendrocytes is required for accurate CNS myelination. J. Neurosci. 34 (25), 8432–8448. doi:10.1523/JNEUROSCI.1105-14.2014
Lee, J. Y., Kim, M. J., Deliyanti, D., Azari, M. F., Rossello, F., Costin, A., et al. (2017). Overcoming monocarboxylate transporter 8 (MCT8)-Deficiency to promote human oligodendrocyte differentiation and myelination. EBioMedicine 25, 122–135. doi:10.1016/j.ebiom.2017.10.016
Lee, J. Y., and Petratos, S. (2016). Thyroid hormone signaling in oligodendrocytes: From extracellular transport to intracellular signal. Mol. Neurobiol. 53 (9), 6568–6583. doi:10.1007/s12035-016-0013-1
Lemkine, G. F., Raj, A., Alfama, G., Turque, N., Hassani, Z., Alegria-Prevot, O., et al. (2005). Adult neural stem cell cycling in vivo requires thyroid hormone and its alpha receptor. FASEB J. official Publ. Fed. Am. Soc. Exp. Biol. 19 (7), 863–865. doi:10.1096/fj.04-2916fje
Leonard, J. L., Mellen, S. A., and Larsen, P. R. (1983). Thyroxine 5'-deiodinase activity in Brown adipose tissue. Endocrinology 112 (3), 1153–1155. doi:10.1210/endo-112-3-1153
Lin, H-Y., Hopkins, R., Cao, H. J., Tang, H-Y., Alexander, C., Davis, F. B., et al. (2005). Acetylation of nuclear hormone receptor superfamily members: Thyroid hormone causes acetylation of its own receptor by a mitogen-activated protein kinase-dependent mechanism. Steroids 70 (5), 444–449. doi:10.1016/j.steroids.2005.03.001
Lin, H-Y., Sun, M., Tang, H-Y., Lin, C., Luidens, M. K., Mousa, S. A., et al. (2009). l-Thyroxine vs. 3, 5, 3′-triiodo-l-thyronine and cell proliferation: activation of mitogen-activated protein kinase and phosphatidylinositol 3-kinase. Am. J. Physiol. Cell Physiol. 296 (5), C980–C991. doi:10.1152/ajpcell.00305.2008
Lopez-Juarez, A., Remaud, S., Hassani, Z., Jolivet, P., Pierre Simons, J., Sontag, T., et al. (2012). Thyroid hormone signaling acts as a neurogenic switch by repressing Sox2 in the adult neural stem cell niche. Cell stem Cell 10 (5), 531–543. doi:10.1016/j.stem.2012.04.008
Lu, D-Y., Liou, H-C., Tang, C-H., and Fu, W-M. (2006). Hypoxia-induced iNOS expression in microglia is regulated by the PI3-kinase/Akt/mTOR signaling pathway and activation of hypoxia inducible factor-1alpha.. Biochem. Pharmacol. 72 (8), 992–1000. doi:10.1016/j.bcp.2006.06.038
Maeda, M., and Ingbar, S. H. (1984). Evidence that the 5'-monodeiodinases for thyroxine and 3, 3', 5'-triiodothyronine in the rat pituitary are separate enzymes. Endocrinology 114 (3), 747–752. doi:10.1210/endo-114-3-747
Maia, A. L., Kim, B. W., Huang, S. A., Harney, J. W., and Larsen, P. R. (2005). Type 2 iodothyronine deiodinase is the major source of plasma T3 in euthyroid humans. J. Clin. Invest.. 115 (9), 2524–2533. doi:10.1172/JCI25083
Mammana, S., Bramanti, P., Mazzon, E., Cavalli, E., Basile, M. S., Fagone, P., et al. (2018). Preclinical evaluation of the PI3K/Akt/mTOR pathway in animal models of multiple sclerosis. Oncotarget 9 (9), 8263–8277. doi:10.18632/oncotarget.23862
Manning, B. D., and Cantley, L. C. (2007). AKT/PKB signaling: Navigating downstream. Cell 129 (7), 1261–1274. doi:10.1016/j.cell.2007.06.009
Marín-García, J. (2010). Thyroid hormone and myocardial mitochondrial biogenesis. Vasc. Pharmacol. 52 (3), 120–130. doi:10.1016/j.vph.2009.10.008
Mayerl, S., Müller, J., Bauer, R., Richert, S., Kassmann, C. M., Darras, V. M., et al. (2014). Transporters MCT8 and OATP1C1 maintain murine brain thyroid hormone homeostasis. J. Clin. Invest.. 124 (5), 1987–1999. doi:10.1172/JCI70324
McTigue, D. M., and Tripathi, R. B. (2008). The life, death, and replacement of oligodendrocytes in the adult CNS. J. Neurochem. 107 (1), 1–19. doi:10.1111/j.1471-4159.2008.05570.x
Mdzinarishvili, A., Sutariya, V., Talasila, P. K., Geldenhuys, W. J., and Sadana, P. (2013). Engineering triiodothyronine (T3) nanoparticle for use in ischemic brain stroke. Drug Deliv. Transl. Res. 3 (4), 309–317. doi:10.1007/s13346-012-0117-8
Medici, M., Visser, W. E., Visser, T. J., and Peeters, R. P. (2015). Genetic determination of the hypothalamic-pituitary-thyroid Axis: Where do we stand? Endocr. Rev. 36 (2), 214–244. doi:10.1210/er.2014-1081
Mendoza, A., and Hollenberg, A. N. (2017). New insights into thyroid hormone action. Pharmacol. Ther. 173, 135–145. doi:10.1016/j.pharmthera.2017.02.012
Menegay, C., Juge, C., and Burger, A. G. (1989). Pharmacokinetics of 3, 5, 3'-triiodothyroacetic acid and its effects on serum TSH levels. Acta Endocrinol. 121 (5), 651–658. doi:10.1530/acta.0.1210651
Menn, B., Garcia-Verdugo, J. M., Yaschine, C., Gonzalez-Perez, O., Rowitch, D., and Alvarez-Buylla, A. (2006). Origin of oligodendrocytes in the subventricular zone of the adult brain. J. Neurosci. 26 (30), 7907–7918. doi:10.1523/JNEUROSCI.1299-06.2006
Merrill, J. E. (1991). Effects of interleukin-1 and tumor necrosis factor-alpha on astrocytes, microglia, oligodendrocytes, and glial precursors in vitro. Dev. Neurosci. 13 (3), 130–137. doi:10.1159/000112150
Milner, R., and Ffrench-Constant, C. (1994). A developmental analysis of oligodendroglial integrins in primary cells: Changes in alpha v-associated beta subunits during differentiation. Development 120 (12), 3497–3506. doi:10.1242/dev.120.12.3497
Moeller, L. C., Cao, X., Dumitrescu, A. M., Seo, H., and Refetoff, S. (2006). Thyroid hormone mediated changes in gene expression can be initiated by cytosolic action of the thyroid hormone receptor beta through the phosphatidylinositol 3-kinase pathway. Nucl. Recept. Signal. 4, e020. doi:10.1621/nrs.04020
Moeller, L. C., Haselhorst, N. E., Dumitrescu, A. M., Cao, X., Seo, H., Refetoff, S., et al. (2011). Stanniocalcin 1 induction by thyroid hormone depends on thyroid hormone receptor β and phosphatidylinositol 3-kinase activation. Exp. Clin. Endocrinol. Diabetes 119 (2), 81–85. doi:10.1055/s-0030-1262860
Montgomery, A. M., Becker, J. C., Siu, C. H., Lemmon, V. P., Cheresh, D. A., Pancook, J. D., et al. (1996). Human neural cell adhesion molecule L1 and rat homologue NILE are ligands for integrin alpha v beta 3. J. Cell Biol. 132 (3), 475–485. doi:10.1083/jcb.132.3.475
Mousa, S. A., O'Connor, L., Davis, F. B., and Davis, P. J. (2006). Proangiogenesis action of the thyroid hormone analog 3, 5-diiodothyropropionic acid (DITPA) is initiated at the cell surface and is integrin mediated. Endocrinology 147 (4), 1602–1607. doi:10.1210/en.2005-1390
Musah, A. S., Brown, T. L., Jeffries, M. A., Shang, Q., Hashimoto, H., Evangelou, A. V., et al. (2020). Mechanistic target of rapamycin regulates the oligodendrocyte cytoskeleton during myelination. J. Neurosci. 40 (15), 2993–3007. doi:10.1523/JNEUROSCI.1434-18.2020
Nait-Oumesmar, B., Picard-Riéra, N., Kerninon, C., Baron-Van Evercooren, A., and BAron-VAn Evercooren, A. (2008). The role of SVZ-derived neural precursors in demyelinating diseases: From animal models to multiple sclerosis. J. Neurol. Sci. 265 (1-2), 26–31. doi:10.1016/j.jns.2007.09.032
Nait-Oumesmar, B., Picard-Riera, N., Kerninon, C., Decker, L., Seilhean, D., Höglinger, G. U., et al. (2007). Activation of the subventricular zone in multiple sclerosis: Evidence for early glial progenitors. Proc. Natl. Acad. Sci. U. S. A. 104 (11), 4694–4699. doi:10.1073/pnas.0606835104
Narayanan, S. P., Flores, A. I., Wang, F., and Macklin, W. B. (2009). Akt signals through the mammalian target of rapamycin pathway to regulate CNS myelination. J. Neurosci. 29 (21), 6860–6870. doi:10.1523/JNEUROSCI.0232-09.2009
Nave, K. A. (2010). Myelination and the trophic support of long axons. Nat. Rev. Neurosci. 11 (4), 275–283. doi:10.1038/nrn2797
Ojamaa, K. (2010). Signaling mechanisms in thyroid hormone-induced cardiac hypertrophy. Vasc. Pharmacol. 52 (3-4), 113–119. doi:10.1016/j.vph.2009.11.008
Ontaneda, D., Thompson, A. J., Fox, R. J., and Cohen, J. A. (2017). Progressive multiple sclerosis: Prospects for disease therapy, repair, and restoration of function. Lancet 389 (10076), 1357–1366. doi:10.1016/S0140-6736(16)31320-4
Petratos, S., Gonzales, M. F., Azari, M. F., Marriott, M., Minichiello, R. A., Shipham, K. A., et al. (2004). Expression of the low-affinity neurotrophin receptor, p75NTR, is upregulated by oligodendroglial progenitors adjacent to the subventricular zone in response to demyelination. Glia 48 (1), 64–75. doi:10.1002/glia.20056
Pfeiffer, S. E., Warrington, A. E., and Bansal, R. (1993). The oligodendrocyte and its many cellular processes. Trends Cell Biol. 3 (6), 191–197. doi:10.1016/0962-8924(93)90213-k
Pilo, A., Iervasi, G., Vitek, F., Ferdeghini, M., Cazzuola, F., and Bianchi, R. (1990). Thyroidal and peripheral production of 3, 5, 3'-triiodothyronine in humans by multicompartmental analysis. Am. J. Physiol. 258, E715–E726. doi:10.1152/ajpendo.1990.258.4.E715
Pirahanchi, Y., Toro, F., and Jialal, I. (2021). “Physiology, Thyroid Stimulating Hormone,” in StatPearls [Internet]. (Treasure Island, FL: StatPearls Publishing).
Plow, E. F., Haas, T. A., Zhang, L., Loftus, J., and Smith, J. W. (2000). Ligand binding to integrins. J. Biol. Chem. 275 (29), 21785–21788. doi:10.1074/jbc.R000003200
Pombo, P. M., Barettino, D., Ibarrola, N., Vega, S., Rodríguez-Peña, A., and Rodriguez-PenA, A. (1999). Stimulation of the myelin basic protein gene expression by 9-cis-retinoic acid and thyroid hormone: Activation in the context of its native promoter. Brain Res. Mol. Brain Res. 64 (1), 92–100. doi:10.1016/s0169-328x(98)00311-8
Pompili, S., Latella, G., Gaudio, E., Sferra, R., and Vetuschi, A. (2021). The charming world of the extracellular matrix: A dynamic and protective network of the intestinal wall. Front. Med. 8, 610189. doi:10.3389/fmed.2021.610189
Relvas, J. B., Setzu, A., Baron, W., Buttery, P. C., LaFlamme, S. E., Franklin, R. J., et al. (2001). Expression of dominant-negative and chimeric subunits reveals an essential role for beta1 integrin during myelination. Curr. Biol. 11 (13), 1039–1043. doi:10.1016/s0960-9822(01)00292-5
Robertson, D., and Moreo, N. (2016). Disease-modifying therapies in multiple sclerosis: Overview and treatment considerations. Fed. Pract. 33 (6), 28–34.
Rocha-Perugini, V., Sánchez-Madrid, F., and Martínez del Hoyo, G. (2016). Function and dynamics of tetraspanins during antigen recognition and immunological synapse formation. Front. Immunol. 6. doi:10.3389/fimmu.2015.00653
Ruppert, M., Aigner, S., Hubbe, M., Yagita, H., and Altevogt, P. (1995). The L1 adhesion molecule is a cellular ligand for VLA-5. J. Cell Biol. 131 (6), 1881–1891. doi:10.1083/jcb.131.6.1881
Saab, A. S., and Nave, K. A. (2017). Myelin dynamics: Protecting and shaping neuronal functions. Curr. Opin. Neurobiol. 47, 104–112. doi:10.1016/j.conb.2017.09.013
Salazar, P., Cisternas, P., Martinez, M., and Inestrosa, N. C. (2019). Hypothyroidism and cognitive disorders during development and adulthood: Implications in the central nervous system. Mol. Neurobiol. 56 (4), 2952–2963. doi:10.1007/s12035-018-1270-y
Sarbassov, D. D., Ali, S. M., Sengupta, S., Sheen, J. H., Hsu, P. P., Bagley, A. F., et al. (2006). Prolonged rapamycin treatment inhibits mTORC2 assembly and Akt/PKB. Mol. Cell 22 (2), 159–168. doi:10.1016/j.molcel.2006.03.029
Sarbassov, D. D., Guertin, D. A., Ali, S. M., and Sabatini, D. M. (2005). Phosphorylation and regulation of Akt/PKB by the rictor-mTOR complex. Science 307 (5712), 1098–1101. doi:10.1126/science.1106148
Sarlieve, L. L., Rodriguez-Pena, A., and Langley, K. (2004). Expression of thyroid hormone receptor isoforms in the oligodendrocyte lineage. Neurochem. Res. 29 (5), 903–922. doi:10.1023/b:nere.0000021235.83952.9a
Saxton, R. A., and Sabatini, D. M. (2017). mTOR signaling in growth, metabolism, and disease. Cell 168 (6), 960–976. doi:10.1016/j.cell.2017.02.004
Schreiber, G., and Richardson, S. J. (1997). The evolution of gene expression, structure and function of transthyretin. Comp. Biochem. Physiol. B Biochem. Mol. Biol. 116 (2), 137–160. doi:10.1016/s0305-0491(96)00212-x
Schweizer, U., Schlicker, C., Braun, D., Köhrle, J., and Steegborn, C. (2014). Crystal structure of mammalian selenocysteine-dependent iodothyronine deiodinase suggests a peroxiredoxin-like catalytic mechanism. Proc. Natl. Acad. Sci. U. S. A. 111 (29), 10526–10531. doi:10.1073/pnas.1323873111
Sen, M. K., Mahns, D. A., Coorssen, J. R., and Shortland, P. J. (2022). The roles of microglia and astrocytes in phagocytosis and myelination: Insights from the cuprizone model of multiple sclerosis. Glia 70, 1215–1250. doi:10.1002/glia.24148
Senese, R., Cioffi, F., Petito, G., Goglia, F., and Lanni, A. (2019). Thyroid hormone metabolites and analogues. Endocrine 66 (1), 105–114. doi:10.1007/s12020-019-02025-5
Sesé, M., Fuentes, P., Esteve-Codina, A., Béjar, E., McGrail, K., Thomas, G., et al. (2017). Hypoxia-mediated translational activation of ITGB3 in breast cancer cells enhances TGF-β signaling and malignant features in vitro and in vivo. Oncotarget. 8 (70). doi:10.18632/oncotarget.23145
Sher, F., Balasubramaniyan, V., Boddeke, E., and Copray, S. (2008). Oligodendrocyte differentiation and implantation: New insights for remyelinating cell therapy. Curr. Opin. Neurol. 21 (5), 607–614. doi:10.1097/WCO.0b013e32830f1e50
Silvestroff, L., Bartucci, S., Pasquini, J., and Franco, P. (2012). Cuprizone-induced demyelination in the rat cerebral cortex and thyroid hormone effects on cortical remyelination. Exp. Neurol. 235 (1), 357–367. doi:10.1016/j.expneurol.2012.02.018
Sun, Y. N., Liu, Y. J., Zhang, L., Ye, Y., Lin, L. X., Li, Y. M., et al. (2014). Expression of organic anion transporting polypeptide 1c1 and monocarboxylate transporter 8 in the rat placental barrier and the compensatory response to thyroid dysfunction. PloS one 9 (4), e96047. doi:10.1371/journal.pone.0096047
Suzuki, N., Hyodo, M., Hayashi, C., Mabuchi, Y., Sekimoto, K., Onchi, C., et al. (2019). Laminin α2, α4, and α5 chains positively regulate migration and survival of oligodendrocyte precursor cells. Sci. Rep. 9 (1), 19882. doi:10.1038/s41598-019-56488-7
Tan, L., Bogush, N., Naib, H., Perry, J., Calvert, J. W., Martin, D. I. K., et al. (2019). Redox activation of JNK2α2 mediates thyroid hormone-stimulated proliferation of neonatal murine cardiomyocytes. Sci. Rep. 9 (1), 17731. doi:10.1038/s41598-019-53705-1
Taylor, E., and Heyland, A. (2017). Evolution of thyroid hormone signaling in animals: Non-genomic and genomic modes of action. Mol. Cell. Endocrinol. 459, 14–20. doi:10.1016/j.mce.2017.05.019
Tsai, H. H., Niu, J., Munji, R., Davalos, D., Chang, J., Zhang, H., et al. (2016). Oligodendrocyte precursors migrate along vasculature in the developing nervous system. Science 351 (6271), 379–384. doi:10.1126/science.aad3839
Tsutsui, H., Kinugawa, S., and Matsushima, S. (2009). Mitochondrial oxidative stress and dysfunction in myocardial remodelling. Cardiovasc. Res. 81 (3), 449–456. doi:10.1093/cvr/cvn280
Tu, H. M., Kim, S. W., Salvatore, D., Bartha, T., Legradi, G., Larsen, P. R., et al. (1997). Regional distribution of type 2 thyroxine deiodinase messenger ribonucleic acid in rat hypothalamus and pituitary and its regulation by thyroid hormone. Endocrinology 138 (8), 3359–3368. doi:10.1210/endo.138.8.5318
Tyler, W. A., Gangoli, N., Gokina, P., Kim, H. A., Covey, M., Levison, S. W., et al. (2009). Activation of the mammalian target of rapamycin (mTOR) is essential for oligodendrocyte differentiation. J. Neurosci. 29 (19), 6367–6378. doi:10.1523/JNEUROSCI.0234-09.2009
Ulland, T. K., Song, W. M., Huang, S. C-C., Ulrich, J. D., Sergushichev, A., Beatty, W. L., et al. (2017). TREM2 maintains microglial metabolic fitness in Alzheimer’s disease. Cell 170 (4), 649–663. e13. doi:10.1016/j.cell.2017.07.023
Valcárcel-Hernández, V., López-Espíndola, D., Guillén-Yunta, M., García-Aldea, Á., López de Toledo Soler, I., Bárez-López, S., et al. (2022). Deficient thyroid hormone transport to the brain leads to impairments in axonal caliber and oligodendroglial development. Neurobiol. Dis. 162, 105567. doi:10.1016/j.nbd.2021.105567
Vatine, G. D., Al-Ahmad, A., Barriga, B. K., Svendsen, S., Salim, A., Garcia, L., et al. (2017). Modeling psychomotor retardation using iPSCs from MCT8-deficient patients indicates a prominent role for the blood-brain barrier. Cell stem Cell 20 (6), 831–843. e5. doi:10.1016/j.stem.2017.04.002
Vatine, G. D., Shelest, O., Barriga, B. K., Ofan, R., Rabinski, T., Mattis, V. B., et al. (2021). Oligodendrocyte progenitor cell maturation is dependent on dual function of MCT8 in the transport of thyroid hormone across brain barriers and the plasma membrane. Glia 69 (9), 2146–2159. doi:10.1002/glia.24014
Verge, C. F., Konrad, D., Cohen, M., Di Cosmo, C., Dumitrescu, A. M., Marcinkowski, T., et al. (2012). Diiodothyropropionic acid (DITPA) in the treatment of MCT8 deficiency. J. Clin. Endocrinol. Metab. 97 (12), 4515–4523. doi:10.1210/jc.2012-2556
Visser, T. J., Leonard, J. L., Kaplan, M. M., and Larsen, P. R. (1982). Kinetic evidence suggesting two mechanisms for iodothyronine 5'-deiodination in rat cerebral cortex. Proc. Natl. Acad. Sci. U. S. A. 79 (16), 5080–5084. doi:10.1073/pnas.79.16.5080
Vose, L. R., Vinukonda, G., Jo, S., Miry, O., Diamond, D., Korumilli, R., et al. (2013). Treatment with thyroxine restores myelination and clinical recovery after intraventricular hemorrhage. J. Neurosci. 33 (44), 17232–17246. doi:10.1523/JNEUROSCI.2713-13.2013
Voss, E. V., Škuljec, J., Gudi, V., Skripuletz, T., Pul, R., Trebst, C., et al. (2012). Characterisation of microglia during de- and remyelination: Can they create a repair promoting environment? Neurobiol. Dis. 45 (1), 519–528. doi:10.1016/j.nbd.2011.09.008
Wallis, K., Dudazy, S., Hogerlinden, M. V., Nordström, K., Mittag, J., and Vennstrom, B. (2010). The thyroid hormone receptor alpha1 protein is expressed in embryonic postmitotic neurons and persists in most adult neurons.. Mol. Endocrinol. 24 (10), 1904–1916. doi:10.1210/me.2010-0175
Weinstein, S. L., Finn, A. J., Davé, S. H., Meng, F., Lowell, C. A., Sanghera, J. S., et al. (2000). Phosphatidylinositol 3-kinase and mTOR mediate lipopolysaccharide-stimulated nitric oxide production in macrophages via interferon-beta.. J. Leukoc. Biol. 67 (3), 405–414. doi:10.1002/jlb.67.3.405
Wen, H., Xiao, W., Biswas, S., Cong, Z. Q., Liu, X. M., Lam, K. S., et al. (2019). Alginate hydrogel modified with a ligand interacting with α3β1 integrin receptor promotes the differentiation of 3D neural spheroids toward oligodendrocytes in vitro. ACS Appl. Mat. Interfaces 11 (6), 5821–5833. doi:10.1021/acsami.8b19438
Wheeler, N. A., Lister, J. A., and Fuss, B. (2015). The autotaxin-lysophosphatidic acid Axis modulates histone acetylation and gene expression during oligodendrocyte differentiation. J. Neurosci. 35 (32), 11399–11414. doi:10.1523/JNEUROSCI.0345-15.2015
Williams, G. R. (2008). Neurodevelopmental and neurophysiological actions of thyroid hormone. J. Neuroendocrinol. 20 (6), 784–794. doi:10.1111/j.1365-2826.2008.01733.x
Wooliscroft, L., Altowaijri, G., Hildebrand, A., Samuels, M., Oken, B., Bourdette, D., et al. (2020). Phase I randomized trial of liothyronine for remyelination in multiple sclerosis: A dose-ranging study with assessment of reliability of visual outcomes. Mult. Scler. Relat. Disord. 41, 102015. doi:10.1016/j.msard.2020.102015
Xing, J., Titus, A. R., and Humphrey, M. B. (2015). The TREM2-DAP12 signaling pathway in nasu-hakola disease: A molecular genetics perspective. Res. Rep. Biochem. 5, 89–100. doi:10.2147/RRBC.S58057
Xu, X., Du, L., Jiang, J., Yang, M., Wang, Z., Wang, Y., et al. (2021). Microglial TREM2 mitigates inflammatory responses and neuronal apoptosis in angiotensin II-induced hypertension in middle-aged mice. Front. Aging Neurosci. 13, 716917. doi:10.3389/fnagi.2021.716917
Ye, H., Tan, Y. L., Ponniah, S., Takeda, Y., Wang, S. Q., Schachner, M., et al. (2008). Neural recognition molecules CHL1 and NB-3 regulate apical dendrite orientation in the neocortex via PTP alpha. EMBO J. 27 (1), 188–200. doi:10.1038/sj.emboj.7601939
Younes-Rapozo, V., Berendonk, J., Savignon, T., Manhaes, A. C., and Barradas, P. C. (2006). Thyroid hormone deficiency changes the distribution of oligodendrocyte/myelin markers during oligodendroglial differentiation in vitro. Int. J. Dev. Neurosci. 24 (7), 445–453. doi:10.1016/j.ijdevneu.2006.08.004
Yuan, M., Pino, E., Wu, L., Kacergis, M., and Soukas, A. A. (2012). Identification of Akt-independent regulation of hepatic lipogenesis by mammalian target of rapamycin (mTOR) complex 2. J. Biol. Chem. 287 (35), 29579–29588. doi:10.1074/jbc.M112.386854
Yuen, T. J., Silbereis, J. C., Griveau, A., Chang, S. M., Daneman, R., Fancy, S. P. J., et al. (2014). Oligodendrocyte-encoded HIF function couples postnatal myelination and white matter angiogenesis. Cell 158 (2), 383–396. doi:10.1016/j.cell.2014.04.052
Zada, D., Tovin, A., Lerer-Goldshtein, T., Vatine, G. D., and Appelbaum, L. (2014). Altered behavioral performance and live imaging of circuit-specific neural deficiencies in a zebrafish model for psychomotor retardation. PLoS Genet. 10 (9), e1004615. doi:10.1371/journal.pgen.1004615
Zalc, B., and Colman, D. R. (2000). Origins of vertebrate success. Science 288 (5464), 271–272. doi:10.1126/science.288.5464.271c
Zendedel, A., Kashani, I. R., Azimzadeh, M., Pasbakhsh, P., Omidi, N., Golestani, A., et al. (2016). Regulatory effect of triiodothyronine on brain myelination and astrogliosis after cuprizone-induced demyelination in mice. Metab. Brain Dis. 31 (2), 425–433. doi:10.1007/s11011-015-9781-y
Zeng, B., Liu, L., Liao, X., Zhang, C., and Ruan, H. (2019). Thyroid hormone protects cardiomyocytes from H(2)O(2)-induced oxidative stress via the PI3K-AKT signaling pathway. Exp. Cell Res. 380 (2), 205–215. doi:10.1016/j.yexcr.2019.05.003
Zettl, U. K., Mix, E., Zielasek, J., Stangel, M., Hartung, H-P., and Gold, R. (1997). Apoptosis of myelin-reactive T cells induced by reactive oxygen and nitrogen intermediatesin vitro. Cell. Immunol. 178 (1), 1–8. doi:10.1006/cimm.1997.1113
Zhou, J., Gauthier, K., Ho, J. P., Lim, A., Zhu, X-G., Han, C. R., et al. (2021). Thyroid hormone receptor α regulates autophagy, mitochondrial biogenesis, and fatty acid use in skeletal muscle. Endocrinology 162 (9), bqab112. doi:10.1210/endocr/bqab112
Zhuang, X., Yu, Y., Jiang, Y., Zhao, S., Wang, Y., Su, L., et al. (2020). Molecular hydrogen attenuates sepsis-induced neuroinflammation through regulation of microglia polarization through an mTOR-autophagy-dependent pathway. Int. Immunopharmacol. 81, 106287. doi:10.1016/j.intimp.2020.106287
Zou, J., Zhou, L., Du, X. X., Ji, Y., Xu, J., Tian, J., et al. (2011). Rheb1 is required for mTORC1 and myelination in postnatal brain development. Dev. Cell 20 (1), 97–108. doi:10.1016/j.devcel.2010.11.020
Zou, Y., Jiang, W., Wang, J., Li, Z., Zhang, J., Bu, J., et al. (2014). Oligodendrocyte precursor cell-intrinsic effect of Rheb1 controls differentiation and mediates mTORC1-dependent myelination in brain. J. Neurosci. 34 (47), 15764–15778. doi:10.1523/JNEUROSCI.2267-14.2014
Glossary
AHDS Allan-Herndon-Dudley syndrome
APC Antigen-presenting cell
Akt Ak strain transforming
BBB Blood-brain barrier
CNS Central nervous system
CNP 2′, 3′-Cyclic-nucleotide-3′-phosphodiesterase
CoA Co-activator protein
CoR Co-repressor protein
cc Corpus callosum
DMTs Disease-modifying therapies
DITPA 3, 5-diiodothyropropionic acid
DIO Deiodinase
EAE Experimental autoimmune encephalitis
EDSS Expanded disability status scale
ECM Extracellular matrix
ERK Extracellular signal-regulated kinase
ETC Electron transport chain
ERR Estrogen-related receptor
FGF Fibroblast growth factor
GAG Glycosaminoglycans
GFAP Glial fibrillary acidic protein
GPCR G protein-coupled receptor
GTPase Guanosine triphosphate hydrolase
HDAC Histone deacetylase
HI Hypoxic-ischemic
HIF-1α hypoxia-inducible factor-1 alpha
HLA-DR15 HLA-DRB1*15:01-DQB1*602
IGF Insulin growth factor
IFN-β Interferon-β
iNOS Inducible nitric oxide synthase
IL-1β Interleukin-1β
IRS Insulin receptor substrate
JNK2α2 c-Jun N-terminal kinase 2 alpha 2
LAT L-type amino acid transporter
LN Laminin
MAPK Mitogen-activated protein kinase
MBP Myelin basic proteins
MCT8 Monocarboxylate transporter 8
MCT10 Monocarboxylate transporter 10
MOG Myelin oligodendrocyte protein
MRI Magnetic resonance imaging
MS Multiple sclerosis
mtDNA Mitochondrial DNA
mTOR Mammalian target of rapamycin
mTORC1 Mammalian target of rapamycin complex 1
mTORC2 Mammalian target of rapamycin complex 2
NPCs Neural precursor cells
NRF Nuclear respiratory factor
OAT Organic anion transporter
OL Oligodendrocyte
OPC Oligodendrocyte progenitor cell
PDGF Platelet-derived growth factor
PDK1 Phosphoinositide-dependent kinase-1
PGC1 Proliferator-activated γ receptor coactivator 1
PI3K Phosphoinositide 3-kinase
PLP Proteolipid protein
PPMS Primary progressive multiple sclerosis
PRMS Progressive-relapsing multiple sclerosis
pt Pertussin toxin
PTEN Phosphatase and tensin homolog
PTPα Protein tyrosine phosphatase alpha
RGD Arginylglycylaspartic acid
Rhes Ras family GTPase
ROS Reactive oxygen species
RRMS Relapsing-remitting multiple sclerosis
RTK Receptor tyrosine kinase
RXR Retinoid-X-receptors
SHBG Sex hormone-binding globulin
SPMS Secondary progressive multiple sclerosis
SVZ Sub-ventricular zone
T3 Triiodothyronine
T4 Thyroxine
TETRAC Tetraiodothyroacteic acid
TFAM Mitochondrial transcription factor
TREM2 Triggering receptors expressed in myeloid cells 2
TRIAC Triiodothyroacetic acid
TSC Tuberous sclerosis complex
TGA Therapeutic Goods Administration
THT Thyroid hormone transporter
TH Thyroid hormone
TNF Tumor necrosis factor
TR Thyroid hormone receptor
TRE Thyroid hormone response elements
TRH Thyrotropin-releasing hormone
Keywords: integrin, thyroid hormone, oligodendrocyte (OL), Akt, mTOR-mammalian target of rapamycin, monocarboxylate transporter 8 (MCT8)
Citation: Emamnejad R, Dass M, Mahlis M, Bozkurt S, Ye S, Pagnin M, Theotokis P, Grigoriadis N and Petratos S (2022) Thyroid hormone-dependent oligodendroglial cell lineage genomic and non-genomic signaling through integrin receptors. Front. Pharmacol. 13:934971. doi: 10.3389/fphar.2022.934971
Received: 05 May 2022; Accepted: 18 July 2022;
Published: 05 September 2022.
Edited by:
Sandra Incerpi, Roma Tre University, ItalyReviewed by:
Laura Calza, University of Bologna, ItalyStella E. Tsirka, Stony Brook University, United States
Copyright © 2022 Emamnejad, Dass, Mahlis, Bozkurt, Ye, Pagnin, Theotokis, Grigoriadis and Petratos. This is an open-access article distributed under the terms of the Creative Commons Attribution License (CC BY). The use, distribution or reproduction in other forums is permitted, provided the original author(s) and the copyright owner(s) are credited and that the original publication in this journal is cited, in accordance with accepted academic practice. No use, distribution or reproduction is permitted which does not comply with these terms.
*Correspondence: Steven Petratos, steven.petratos@monash.edu
†These authors contributed equally to this manuscript