- 1Key Laboratory of Liver and Kidney Diseases (Ministry of Education), Institute of Liver Diseases, Shanghai Key Laboratory of Traditional Chinese Clinical Medicine, Shuguang Hospital Affiliated to Shanghai University of Traditional Chinese Medicine, Shanghai, China
- 2Institute of Clinical Pharmacology, Shuguang Hospital Affiliated to Shanghai University of Traditional Chinese Medicine, Shanghai, China
The interaction between host and microorganism widely affects the immune and metabolic status. Indole and its derivatives are metabolites produced by the metabolism of tryptophan catalyzed by intestinal microorganisms. By activating nuclear receptors, regulating intestinal hormones, and affecting the biological effects of bacteria as signaling molecules, indole and its derivatives maintain intestinal homeostasis and impact liver metabolism and the immune response, which shows good therapeutic prospects. We reviewed recent studies on indole and its derivatives, including related metabolism, the influence of diets and intestinal commensal bacteria, and the targets and mechanisms in pathological conditions, especially progress in therapeutic strategies. New research insights into indoles will facilitate a better understanding of their druggability and application in intestinal and liver diseases.
Introduction
The crosstalk between the host and the gut microbiota has been widely studied in recent decades. Individuals carry more than 1,000 distinct bacterial species; each bacteria has significant genomic differences, which leads to variable protein expression and metabolite formation (Belizario and Faintuch, 2018). The gut microbiota and microbiota-derived small molecules, such as indole and its derivatives, interact with the host and exert a variety of local and heterotopic biological effects by circulating in the plasma (Schroeder and Backhed, 2016).
The liver and intestine are closely related through the liver–gut axis, and the disorder of intestinal–liver interaction plays an important role in the pathogenesis of gastrointestinal and liver diseases (Tripathi et al., 2018). Intestinal microbiota dysbiosis and impaired intestinal barrier function lead to the translocation of microbiota and its products, which triggers the innate and adaptive immune system, induces chronic inflammation, and leads to liver damage and systemic metabolic disorders (McLaughlin et al., 2017; Chen and Tian, 2020). Gut microbiota is involved in the bioconversion of indoles from tryptophan (Trp), which is an essential amino acid derived entirely from the diet. In the gastrointestinal tract, indole compounds can accumulate to millimolar concentrations (Liu et al., 2020); changes in food intake and gut microbiota also have a great impact on the metabolic concentration of indole in the intestine.
Recently, as a part of microbiotherapy, indole and its derivates were extensively explored in terms of antifungal, anti-platelet, and antioxidant effects in various diseases (Mucha et al., 2021). Accumulating shreds of evidence have demonstrated that indole and its derivatives derived from the intestine exert a profound effect on the intestinal barrier function and intestinal immunity (Alexeev et al., 2018; Scott et al., 2020). Indole and its derivatives can enter the liver through the circulation for further catalysis and affect liver metabolism and immune response as important intercellular signal molecules (Banoglu et al., 2001; Hwang et al., 2013; Brunius et al., 2016). In this review, we gathered the current knowledge of the gut microbiota–derived indoles, with a focus on related gut metabolic pathways and enzymes, the influence of dietary intake, the pathophysiological changes, and the prospect of potential biotechnological applications in intestinal and liver diseases (Table 1).
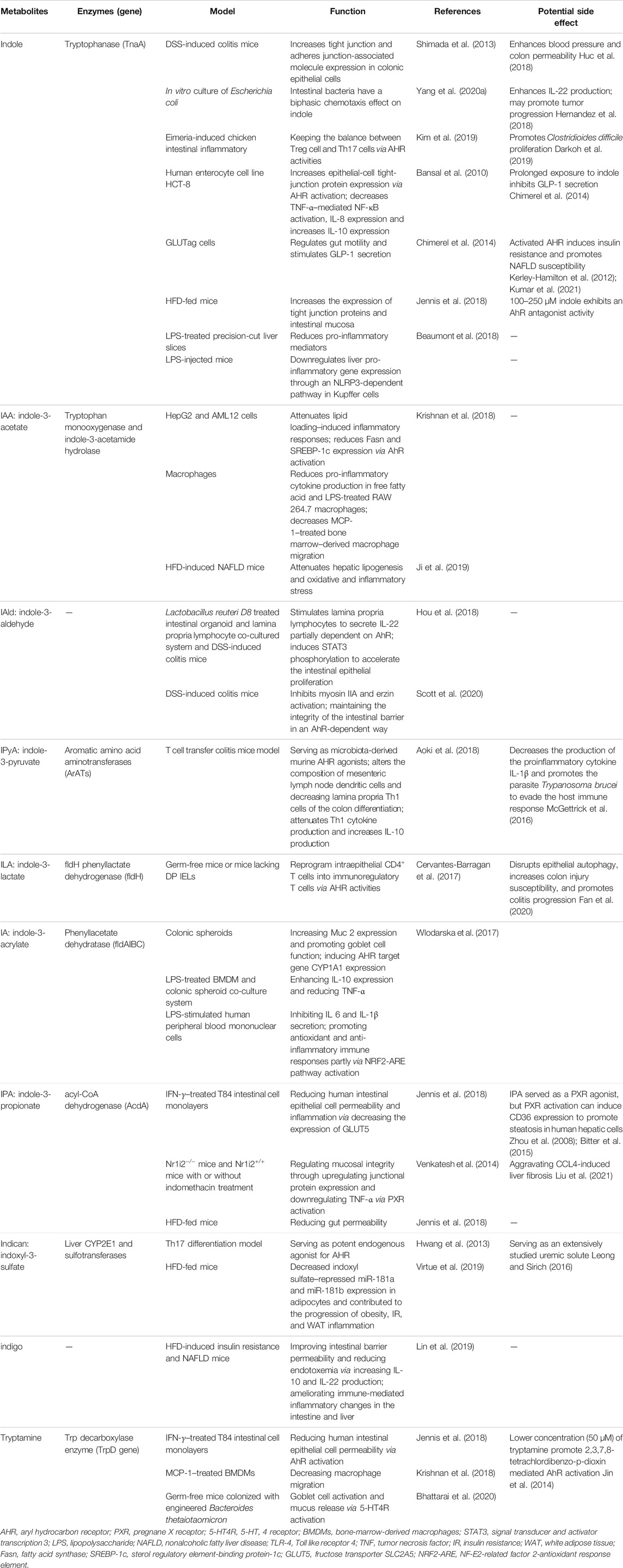
TABLE 1. Catalyze enzymes, functions, and potential side effects of microbial-origin tryptophan metabolites.
Metabolism of Indole and its Derivates: Pathways and Enzymes
Intestinal Trp Metabolism
Indole and its derivatives are derived from the metabolism of Trp by gut microorganisms. Trp is an essential aromatic amino acid that cannot be synthesized endogenously; therefore, the exogenous dietary source of Trp intake is decisive. A small portion of the ingested Trp serves as a substrate to synthesize proteins, and the remainder is metabolized by endogenous host cells (the kynurenine pathway and serotonin pathway) (Gostner et al., 2020) or intestinal microorganisms (indole and its derivatives pathway) (Figure 1).
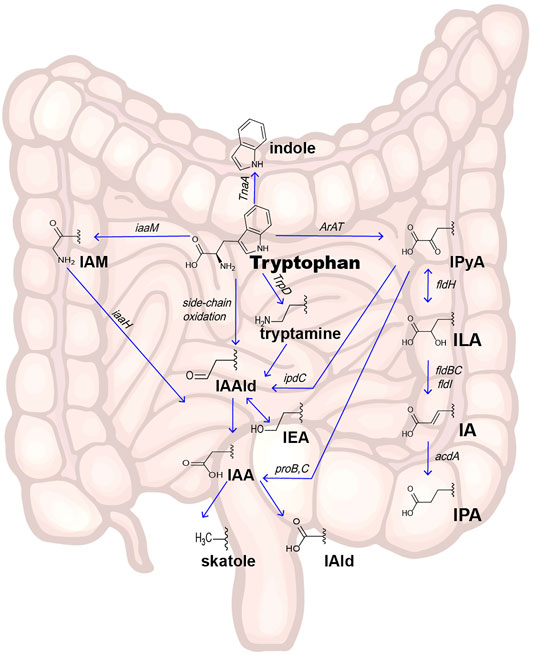
FIGURE 1. Pathways of intestinal Trp metabolism. Indole and its derivatives are derived from the metabolism of Trp by gut microorganisms. There are three main pathways in intestinal microorganism–derivated Trp metabolism: the Trp-Indole pathway, Trp-IPyA-ILA-IA-IPA pathway, and Trp-IAA-Skatole or IAld pathway. Abbreviations: IPyA, indole-3-pyruvate; ILA, indole-3-lactate; IA, indole-3-acrylate; IPA, indole-3-propionate; IAAld, indole-3-acetaldehyde; IAA, indole-3-acetate; IAld, indole-3-aldehyde; IAM, indole-3-acetamine; IEA, indole-3-ethanol; TnaA gene, encode Tryptophanase; iaaM gene, encode Tryptophan 2-monooxygenase; iaaH gene, encode indole-3-acetamide hydrolase; TrpD gene: encode Tryptophan decarboxylase enzyme; ArAT gene, encode aromatic amino acid aminotransferase; fldH gene, encode phenyllactate dehydrogenase; fldBC gene, encode (R)-phenyllactyl-CoA dehydratase alfa and beta subunits; acdA gene, encode acyl-CoA dehydrogenase; ipdC gene, encode Indole-3-pyruvate decarboxylase.
Trp→Indole Pathway
As the most abundant microbial Trp catabolites, more than 85 gram-positive and gram-negative species are known to hydrolyze Trp to indole by tryptophanase (Lee and Lee, 2010). Escherichia coli is one of the most widely studied intestinal indole-producing bacteria. The expression of tryptophanase in E. coli is controlled by the Trp operon, which consists of a promoter, a short peptide regulatory gene, and two structural genes, TnaA and TnaB (Yanofsky et al., 1991). Trp is imported into cells by TnaB and then reversibly catalyzed by TnaA to indole, pyruvate, and ammonia (Watanabe and Snell, 1972; Ma et al., 2018; Yang W. et al., 2020). Because eukaryotes cannot encode TnaA, this reaction occurs only in bacteria (Lee and Lee, 2010). The fecal concentration of indole was reported to be in a wide range (from 0.30 to 6.64 millimole in healthy adults), which indicates that there are very large individual differences in indole metabolism (Darkoh et al., 2015). The concentration of indole depends on the amount of exogenous Trp; under Trp-rich conditions, TnaA can be synthesized to convert more Trp into indole by E. coli (Li and Young, 2013). Intriguingly, though some bacteria, such as Aeromonas salmonicida, Pseudovibrio sp., etc., have a TnaA gene homolog, they cannot synthesize indole (Lee and Lee, 2010).
Trp→Tryptamine Pathway
Tryptamine is a tryptophan-derived monoamine, and two known commensal microorganisms, Ruminococcus gnavus and Clostridium sporogenes, convert Trp into tryptamine by the action of Trp decarboxylase enzyme (TrpD). In healthy individuals, the presence of TrpD homologs is at least 10% according to the analysis of the samples from the NIH Human Microbiome Project (HMP), which indicates that the production of tryptamine by gut microbiota could be prevalent in humans (Williams et al., 2014). Transplanting human gut microbiota to germ-free mice increases tryptamine concentrations by nearly 200-fold (Marcobal et al., 2013).
Trp→Indole-3-Pyruvate→Indole-3-Lactate→Indole-3-Acrylate→Indole-3-Propionate Pathway
IPyA is converted from Trp by the presence of the aromatic amino acid aminotransferase (ArAT). IPyA is a precursor of ILA, and phenyllactate dehydrogenase (fldH) is involved in this reduction reaction. Through dehydrating, bacterial species containing phenyllactate dehydratase (fldBC) along with its activator fldI convert ILA to IA. IA can be further converted into IPA by acyl-CoA dehydrogenase (AcdA), which is the final product of reductive Trp metabolism (Dodd et al., 2017).
A series of studies have reported that the intestinal microbiota plays a key role in this process. ArAT is an enzyme that is phylogenetically conserved in many bacterial species, including Lactobacilli and Clostridium sporogenes (Zelante et al., 2013; Aoki et al., 2018). Fifty-one species of Bifidobacterium are reported to convert Trp into ILA (Van Benschoten, 1979). Another group of probiotic Lactobacillus spp. convert Trp to ILA by an indolelactic acid dehydrogenase (ILDH) (Wilck et al., 2017; Lee et al., 2018). Several Peptostreptococcus spp. and Clostridium spp. promote the synthesis of IA and IPA due to the homologous gene cluster of phenyllactate dehydratase fldAIBC (Dodd et al., 2017; Wlodarska et al., 2017); fldC, especially, is essential for the reductive metabolism of Trp by gut bacteria, and its mutant can block the dehydration of ILA and increase the level of the other upstream metabolites. Bacteria such as Peptostreptococcus stomatis, which lacks the initiator fldI but encodes the phenyllactate dehydratase fldABC gene cluster, produce a lower level of IA (Wlodarska et al., 2017). Other bacteria, such as Lechevalieria aerocolonigenes, can synthesize IPA through Trp deamination, which is catalyzed by amino acid oxidase (Nishizawa et al., 2005).
Trp→Indole-3-Acetate →Methylindole (Skatole) or Indole-3-Aldehyde Pathway
IAA is one of the Trp degraded molecules by fungi and bacteria. In human fecal samples, the mean concentration of IAA is 5 nM/g (Lamas et al., 2016). IAA biosynthetic includes multiple coexisting pathways; at least five major Trp-dependent metabolic precursors to IAA have been postulated and identified (Zelante et al., 2021), namely IPyA, indole-3-acetamine (IAM), indole-3-acetonitrile (IAN), tryptamine, and the tryptophan side-chain oxidation pathway (only demonstrated in Pseudomonas fluorescens). Tryptamine and IPyA are the most prevalent synthetic precursors in bacteria (Zhang et al., 2019; Miyagi et al., 2020). Tryptophan monooxygenase (TMO, encoded by IaaM gene) catalyzes Trp to IAM, and then the indole-3-acetamide hydrolase (encoded by IaaH gene) covers IAM to IAA (Tsavkelova et al., 2012). In the IPyA-IAA pathway, IPyA decarboxylase is the key enzyme (encoded by ipdC gene) (Malhotra and Srivastava, 2008). IPyA is catalyzed by this enzyme to IAAld, and then IAAld can be converted into IAA via decarboxylation. In addition, candidate genes in the genome include pyruvate: ferredoxin oxidoreductases B and C (ProB and ProC) and putative flavin-containing monooxygenases (FMOs) are also involved in converting IPyA to IAA (Dai et al., 2013; Dodd et al., 2017). In addition to bacteria-derived IAA synthetic pathways, it was recently reported that interleukin-4-induced gene 1, which encodes a protein with l-amino acid activity that has a bias for catalyzing L-aromatic amino acids, could catalyze Trp to IAA in host cells (Zhang et al., 2020).
Skatole and IAld are the terminal products of Trp-IAA degradation. The concentration of precursor IAA could be the rate-limiting factor of skatole synthesis (Yokoyama and Carlson, 1979). Less than 0.01% of the total intestinal microbiota, mainly the Clostridium and Bacteroides genera, catalyzes the steps from IAA to skatole (Wesoly and Weiler, 2012). The fecal skatole concentration in healthy humans is 5 μg/g feces, whereas its level can increase to 80–100 μg/g feces in digestive disorder patients (Yokoyama and Carlson, 1979). IAA can also be oxidized to IAld through peroxidase-catalyzed aerobic oxidations, even under strong oxidation conditions; this step occurs spontaneously in vitro (Zhang et al., 2020). IAld is produced in a reduced number of species that belong to the Firmicutes phyla, such as Lactobacillus acidophilus, Lactobacillus murinus, and Lactobacillus reuteri. IAld is produced via several pathways, of which the IPyA route is the main pathway for IAld synthesis from Trp (Trp→IPyA→IAld) (Zelante et al., 2013; Zelante et al., 2021).
Metabolism of Indoles in Host
The absorption of indole and its derivatives through the intestinal epithelium is due to the ability to freely diffuse through lipid membranes (Pinero-Fernandez et al., 2011). A variety of metabolic enzymes, such as cytochrome P450 (CYP450), exist in human intestinal epithelial cells to facilitate the absorption of indoles (Gillam et al., 2000). Then, the indole compounds afflux into the liver through the portal vein and undergo further metabolism.
Indole metabolism in the liver has been widely studied. Initially, indole is absorbed and oxidized by microsomal CYP450 isozymes (especially the CYP2E1 isoform) to indoxyl and indican (Banoglu et al., 2001). CYP2A6 was also reported to oxidize indole at the C-2, C-3, and C-6 positions to synthesize oxindole, indoxyl (3-hydroxyindole), and 6-hydroxyindole, respectively (Gillam et al., 2000). Hepatic phase II drug metabolism of indoxyl is conjugated by sulfotransferases to generate indoxyl-3-sulfate (I3S), which is finally excreted by the kidney (Banoglu et al., 2001; Banoglu and King, 2002). As a uremic toxin, indoxyl sulfate is extensively studied in uremia (Lano et al., 2020; Wang et al., 2020) (Figure 2A).
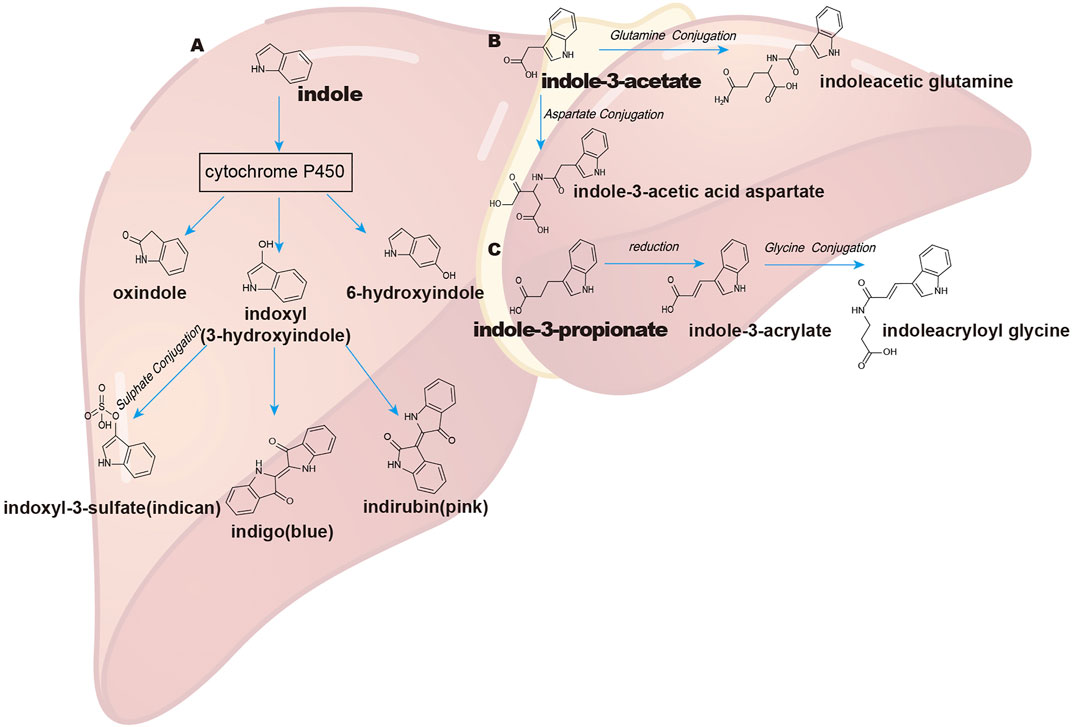
FIGURE 2. Pathways of indole and indole derivate metabolism in the liver. (A) indole; (B) indole-3-acetate (IAA); (C) indole-3-propionate (IPA). The absorption of indole and its derivatives through the intestinal epithelium and their further metabolism by liver CYP450 and sulfotransferase enzymes and conjugation with some other amino acids like glutamine and glycine and, finally, excretion by the kidney.
Apart from indole, liver CYP450, especially CYP1A, CYP2A, and CYP2E1, plays a key role in skatole metabolism and produces seven known skatole metabolites (indole-3-carbinol, 3-hydroxy-3-methyloxindole, 3-methyloxyindole, 3-hydroxy-3-methylindolenine, 5-hydroxy-3-methylindole, 6-hydroxy-3-methylindole, and 2-aminoacetophenone) (Brunius et al., 2016). In the liver, IAA can be oxidized by peroxidase to cytotoxic species (Folkes et al., 1999) or be further combined with glutamine to synthesize indoleacetic glutamine (Keszthelyi et al., 2009; Gao et al., 2018) (Figure 2B). IPA can be converted into IA and further conjugated with glycine to produce indoleacryloyl glycine in the liver (Smith et al., 1968; Gao et al., 2018; Ulaszewska et al., 2020) (Figure 2C). Tryptamine can be deaminated by monoamine oxidases A and B, which are highly expressed in the colonic epithelium and liver (Jones, 1982; Bhattarai et al., 2018).
Influence of Dietary Intake and Ingredients
Dietary intake has a wide influence on gut microbiota and the related indole metabolism. Microbial Trp metabolism activity can be reduced when alternative energy substrates are available. Indole is much lower in animals fed a high-nonstarch polysaccharide diet (Knarreborg et al., 2016). This finding is consistent with the report in 1919 that glucose repressed indole biosynthesis (Wyeth, 1919). Recently, some researchers have found that IPA was the metabolite most significantly and consistently related to the intake of fiber and inversely associated with the risk of type 2 diabetes (de Mello et al., 2017). The associated mechanism can be partially explained by the host functional LCT variant and fiber intake interaction on the gut bacteria shifting tryptophan metabolism (de Mello et al., 2017; Qi et al., 2021). In addition to carbohydrates, dietary cholesterol was reported to drive the formation of nonalcoholic fatty liver disease–associated hepatocellular carcinoma by inducing changes in mouse gut microbiota and metabolites. Dietary intake of high-cholesterol formula increases serum taurocholic acid but reduces IPA concentration in mice and can be prevented by cholesterol suppression therapy (Zhang et al., 2021). Compared with a low-fat diet, the high-fat diet–fed mice exhibited a significant depletion of IA and tryptamine in the liver and cecum (Krishnan et al., 2018). High salt intake also has an effect on consuming Lactobacillus murine and reducing fecal levels of ILA and IAA (Wilck et al., 2017). Excess alcoholic consumption exhibits an increasing level of IAM in the ceca of mice (Liu et al., 2019). It is worth noting that some dietary intervention studies did not specify the ratio of indole content in dietary formulas. However, indole, as an interspecies and interkingdom signaling molecule, is also widely present in plants. Therefore, some dietary intervention studies cannot exclude the effect of dietary intake factors on the benefit of increasing the concentration of indole and its derivatives.
The Effects of Indole and Its Derivatives
Activation of AhR
The aromatic hydrocarbon receptor (AhR) is distributed in almost all tissues in various mammals and is expressed abundantly in the placenta, liver, and lungs, where it plays multiple roles in regulating the immune response, carcinogenesis, metabolic diseases, and neurophysiology (Xue et al., 2018; Girer et al., 2020; Vogel et al., 2020). In the gut, AhR is expressed in epithelial cells and immune cells (Nguyen et al., 2013; Ikuta et al., 2016). AhR is a ligand-inducible transcription factor that exists in the cytoplasm when it is at rest. After binding to the ligand, AhR translocates into the nucleus, where it heterodimerizes with the AhR nuclear transport protein (ARNT) and affects the expression of target genes, such as Cyp1a1, Cyp1b1, AhRR, and IL-10 (Lamas et al., 2018) (Figure 3)
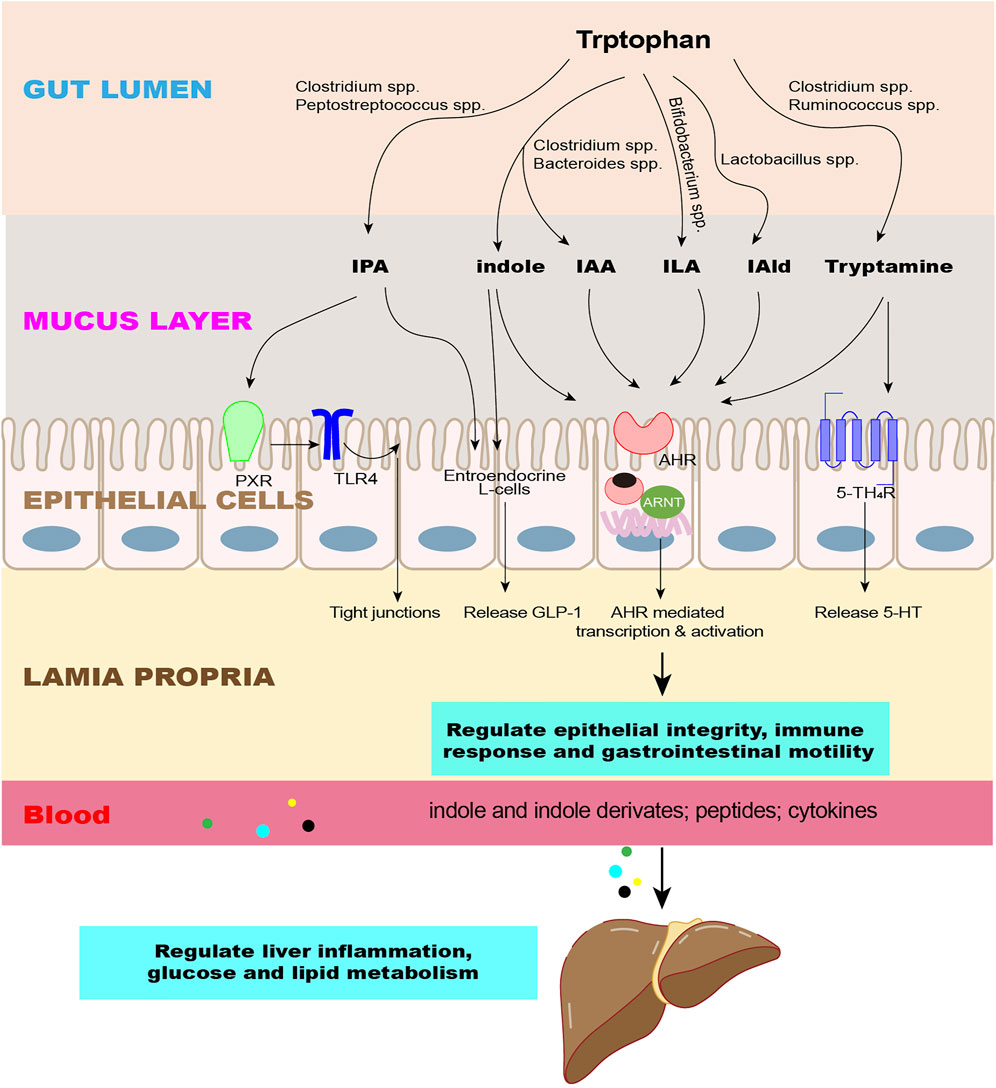
FIGURE 3. Effects of indole and its derivatives on the intestine and liver. The microbiota converts Trp into indole and its derivatives as signaling molecules to regulate epithelial integrity, immune response, and gastrointestinal motility through intestinal receptors and enter the liver through the circulation to regulate liver inflammation and glucose and lipid metabolism. Abbreviations: IPA, indole-3-propionate; IAA, indole-3-acetate; ILA, indole-3-lactate; IAld, indole-3-aldehyde; AhR, aromatic hydrocarbon receptor; PXR, pregnane X receptor; 5-HT4R, 5-HT4 receptors; GLP-1, glucagon-like peptide 1; TLR4, toll-like receptor 4.
A variety of indole-based compounds are ligands of AhR, differing in their pharmacological parameters. Using a reporter gene assay, a recent study found that microbial intestinal-catabolized indoles are full or partial agonists of human AhR. Indole, IAM, and IPyA are ligands with high efficacies of AhR; indole-3-ethanol (IEA), IA, skatole, and tryptamine have medium efficacy, but IPA, IAA, ILA, and IAld have no efficacy or low efficacy (Vyhlidalova et al., 2020b). Another study identified four new tryptophan metabolites that can activate AHR, which are 3-methyl-2-hydroxyindole, 5-hydroxyindole-3-acetic acid, 3-indole acrylic acid, and indole-3-carboxylic acid. The authors also found that human AHR is more effective at physiologically relevant concentrations of tryptophan metabolites than mice (Dong et al., 2020).
The immunomodulatory benefits of indole and its derivatives are partly based on the AhR-driven mechanisms in intestinal DCs, intraepithelial lymphocytes (IELs), and innate lymphoid cells (ILCs). As AhR ligands, indole and some derivatives act through the AhR/IL-22 axis (Zelante et al., 2013; Cervantes-Barragan et al., 2017). IL-22 plays a critical role in regulating epithelial integrity and the immune response, including intestinal stem cells (ISCs) and epithelial regeneration, intestinal barrier protection, antimicrobial defense, intestinal homeostasis, and shaping metabolism (Keir et al., 2020). By activating AHR in CD4+ T cells, ILA shapes CD4+CD8αα+ double-positive intraepithelial lymphocytes to have regulatory functions; other indole derivates have a similar effect (Cervantes-Barragan et al., 2017). The ILC3-derived IL-22 production was found to be reduced in an alcoholic liver disease mouse model; IAA supplementation restored the IL-22 level and protected the mice from ethanol-induced steatohepatitis (Hendrikx et al., 2019). IAld stimulates lamina propria lymphocytes (LPLs) to secrete IL-22 partially dependent on AhR and induces phosphorylation of STAT3 to accelerate intestinal epithelial proliferation, thereby restoring damaged intestinal mucosa (Hou et al., 2018). Indole-3-carbinol (I3C) is a natural plant product and a known ligand for AhR in many cruciferous vegetables. A recent study found that I3C reduces colitis by preventing microbial dysbiosis and increasing the abundance of butyrate-producing gram-positive bacteria in mice in an IL-22–dependent manner. Neutralization of IL-22 blocked the protective effect of I3C on colitis and prevents I3C from dysbiosis and butyrate-induced remission (Busbee et al., 2020).
Indole and its derivatives also promote intestinal immune homeostasis by activating AhR to protect the intestinal barrier. The activation of the AhR pathway in intestinal epithelial cells (IECs) is vital for protecting the stem cell niched and maintaining intestinal barrier integrity (Metidji et al., 2018). Recently, Scott et al. identified three bacterial metabolites of tryptophan, IEA, IPA, and IAAld, which protect against increased gut permeability and alleviated dextran sodium sulfate (DSS)-induced colitis in mice by maintaining the integrity of the intestinal barrier in an AhR-dependent manner (Scott et al., 2020). IA was reported to promote barrier function and immune tolerance in DSS-induced colitis mice, which is related to inducing the mRNA expression of the AhR target gene Cyp1a1 in the intestinal epithelium and immune cells by IA (Wlodarska et al., 2017). Indole and its derivatives also promote the expression of IL-10 by activating AhR, and functional IL-10 signaling is associated with barrier function. IAld increases the proliferation of epithelial cells and promotes the differentiation of goblet cells, reversing the decline of intestinal barrier integrity and systemic inflammation caused by aging in geriatric mice. This effect increases the expression of the cytokine IL-10 via AhR but does not depend on the type I interferon or IL-22 signaling (Powell et al., 2020). In a T cell–mediated colitis mice model, oral administration of IPyA, a microbiota-derived AHR agonist, decreases the frequency of IFN-γ+ IL-10- CD4+ T cells and increases that of IFN-γ- IL-10+ CD4+ T cells in the colon lamina propria. IPyA attenuates the severity of colon inflammation in mice, but treatment with an AHR antagonist inhibited the anti-inflammatory effect of IPyA (Aoki et al., 2018).
Activation of PXR
The pregnane X receptor (PXR) participates in drug, glucose, bile acid, and cholesterol metabolism, and is also essential in maintaining intestinal homeostasis and abrogating inflammation (Daujat-Chavanieu and Gerbal-Chaloin, 2020). There has been accumulating evidence of the beneficial effects of indole and its derivatives on intestinal barrier function based on the activation of PXR. Indole methylated at positions 1 and 2, which were reported ligands and partial agonists of human PXR, induced the expression of PXR-target genes, including CYP3A4 and MDR1, in vitro (Vyhlidalova et al., 2020a). IPA is the most reported PXR receptor and is enteric microbiome-derived (Alexeev et al., 2018). As a ligand of PXR, IPA downregulates enterocyte inflammation cytokines and upregulates mRNA expression of junctional protein-coding genes via PXR and Toll-like Receptor 4 (Venkatesh et al., 2014). IPA protects the hematopoietic system and gastrointestinal tract injuries against acute radiation exposure via PXR/acyl-CoA–binding protein signaling (Xiao et al., 2020). There is an 88% overlap of the AhR and PXR activators; therefore, crosstalk exists between AhR and PXR signaling (Rasmussen et al., 2017). IPA was reported to exert its effects by activating AHR and PXR, and higher expression of AHR and PXR was inversely related to cancer cell proliferation and the stage and grade of the tumor (Sari et al., 2020). However, a recent report pointed out that IPA is not a direct ligand of PXR. Peter and his colleagues examined the effects of 10 known intestinal microbial metabolites and identified indole and IAM as PXR ligands and agonists. Indole and IAM induced the PXR-regulated genes CYP3A4 and MDR1 in human intestinal cancer cells and enhanced the binding of PXR to the MDR1 promoter, but IPA alone did not have the above biological effects. The authors analyzed the controversial results of IPA in vivo studies and found that IPA can greatly increase the intestinal anti-inflammatory effect via PXR in the presence of indole (Illes et al., 2020). This explanation is in line with the report that IPA in combination with indole significantly activated human PXR to regulate intestinal permeability and inflammation, but IPA alone had a weak agonistic effect (Venkatesh et al., 2014).
Influence on Intestinal Hormone Release and Motility
Indole has a dual regulatory effect on the secretion of glucagon-like peptide 1 (GLP-1). Indole inhibits voltage-gated K+ channels and enhances Ca2+ entry to stimulate GLP-1 secretion acutely (6 min). However, over a longer period (240 min), indole slows ATP production, thus leading to a prolonged reduction in GLP-1 secretion (Chimerel et al., 2014). Tryptamine has been widely found to promote gastrointestinal motility. Tryptamine induces the release of 5-hydroxytryptamine (5-HT, serotonin) to modulate gastrointestinal motility as a neurotransmitter (Takaki et al., 1985; Mawe and Hoffman, 2013). Exogenous tryptamine can also act on 5-HT4R to increase anion and fluid secretion in the proximal colon and accelerate gastrointestinal transit (Bhattarai et al., 2018). Nevertheless, opposite effects reported that tryptamine significantly inhibits gastric emptying and acid secretion and reduces pepsin secretion (Bell and Webber, 1979).
Influence on Bacterial Physiology
As a signaling molecule, indole influences diverse aspects of bacterial physiology, such as biofilm formation, spore formation, plasmid stability, bacterial motility, antibiotic resistance, and host cell invasion (Lee and Lee, 2010; Li and Young, 2013; Kim and Park, 2015). Intestinal bacteria have a dynamic and biphasic chemotaxis effect on indoles. It was reported that when the indole concentration is below 1 mM, E. coli is only observed to have a repellent-only response. When the indole concentration exceeds 1 mM, E. coli changes to an attraction response in a time-dependent manner. Therefore, indoles might be able to repel foreign invasion through differences in the adaptation status of bacteria and, at the same time, attract the growth of symbiotic bacteria that have adapted to indoles (Yang J. et al., 2020). The gut microbiota coordinates its behavior by sensing signals derived from the host or microbiota. Indole is sensed by the bacterial membrane-bound histidine sensor kinase (HK) CpxA. The enteric pathogens E. coli and Citrobacter rodentium in the lumen decrease their virulence and downregulate gene expression at the locus of the enterocyte effacement (LEE) pathogenicity island to adjust to a high concentration of indole, which in turn affects their attachment (Kumar and Sperandio, 2019). Although microbiota metabolites affect the composition and function of the intestinal microbiota and regulate host immunity, most of them still lack identification and have unknown functions. Another recent study focused on a family of indole-functionalized bacterial metabolites termed indolokines, and the authors found that such substances are widely present in different bacteria and mouse feces and elicit immune responses in both plants and humans, which indicates that relatively conservative defense strategies exist in the biology (Kim et al., 2020).
Indole and Its Derivatives in Gastrointestinal and Liver Diseases
Intestinal Inflammation
Indole and its derivatives are first produced in the gastrointestinal tract, and their perturbation has a great influence on gastrointestinal disorders. An analysis of serum samples from more than 500 IBD patients observed a negative correlation between Trp levels and disease activity (Nikolaus et al., 2017). Indole metabolism also alters in IBD patients. The fecal levels of Trp and IAA in patients with IBD are decreased, but the Kyn content is increased, which indicates that IBD patients have an obvious Trp–Kyn conversion but less intestinal Trp metabolism, which corresponds to healthy subjects inducing greater AHR activation than IBD patients in fecal samples (Lamas et al., 2016). Serum IPA was reported to decrease by nearly 60% in subjects with active UC compared with healthy controls, which can also serve as a biomarker of remission (Wlodarska et al., 2017; Alexeev et al., 2018). Necrotizing enterocolitis (NEC) is an intestinal necrotizing inflammatory disease that occurs in premature infants. An in vitro study found that ILA, secreted by Bifidobacterium longum subsp infantis, is anti-inflammatory by interacting with Toll-like receptor 4 (TLR4) and AHR to prevent the transcription of inflammatory cytokines (Meng et al., 2020).
Alcoholic and Nonalcoholic Fatty Liver Diseases
Indole and several indole catabolic metabolite disorders have been confirmed by a large number of reports in metabolic liver diseases (Hendrikx and Schnabl, 2019). In a cohort of 137 NAFLD subjects, the circulating levels of indole were significantly lower than those of lean people and were negatively correlated with BMI (Ma et al., 2020). Compared with nonobese individuals, Trp metabolism translates more to Kyn but less to IAA in the feces of obese or diabetic patients (Laurans et al., 2018). In an HFD-induced obesity-associated NAFLD mouse model, the levels of IAA and tryptamine, two metabolites that have powerful anti-inflammatory responses, were decreased in both the liver and cecum (Krishnan et al., 2018). Reduced IPA levels in obese subjects have also been reported, which can be normalized by gastric bypass surgery (Jennis et al., 2018). Caseinolytic peptidase B protein homolog (ClpB) is a bacterial protein that is positively associated with IPA in human plasma and negatively associated with BMI, waist circumference, and total fat mass (Arnoriaga-Rodriguez et al., 2020). The expression of the TnaA gene in the gut microbiome was reduced in HFD-fed mice, which is accompanied by a reduction in plasma levels of indole and indoxyl sulfate. The reduction of indole and indoxyl sulfate repressed the expression of miR-181a and miR-181b in adipocytes of mice, contributing to the progression of obesity, IR, and WAT inflammation (Virtue et al., 2019).
Ongoing Research of Indole and Its Derivatives as an Intervention
Supplement With Related Strains
Indole and its derivatives may have therapeutic effects on gastrointestinal and liver disorders, and regulating dysbiosis to promote intestinal Trp metabolism homeostasis is both a goal and a means. Streptococcus rosenbergii has the fldAIBC gene cluster that produces IA. IA produced by commensal Peptostreptococcus species restores intestinal barrier function and suppresses inflammatory responses in DSS-induced colitis mice (Wlodarska et al., 2017). Inoculating Clostridium sporogenes into germ-free mice accompanied by L-Trp-supplement diets promoted the production of IPA to protect mice from DSS-induced colitis through PXR (Venkatesh et al., 2014). The role of Lactobacillus reuteri D8 in protecting the intestinal mucosal barrier and activating intestinal epithelium proliferation depends on the production of IAld. Mice colonized with live Lactobacillus reuteri D8 can ameliorate intestinal mucosa damage caused by DSS (Hou et al., 2018).
Supplement With Indole and Its Derivates
Direct addition of indole and its derivatives is another common intervention. Mucin is an energy source for certain symbionts to promote their colonization and benefit the epithelial barrier. A recent study indicated that supplementation with IPA strengthens the mucus barrier against LPS-induced inflammation by increasing mucins in vitro (Beaumont et al., 2018; Li et al., 2021). In comparison with AhR ligand-deprived diets, dietary I3C supplementation drives the expression of the AhR repressor (AhRR) in intestinal immune cells of AhRR-reporter mice, strengthens intestinal barrier integrity, and lowers susceptibility to colitis (Schanz et al., 2020).
Indole and its derivatives are also involved in liver disease therapy. Oral administration of indole can suppress the NF-
Microbial Metabolite Mimicry and Synthetic Indole
Natural metabolites are potent and well-tolerated drugs; however, the short half-life, poor oral bioavailability, and ubiquitous action of indole derivates are the limitations for their suitability as drugs (Kumar et al., 2020). The microbial metabolic mimicry can expand the potential drug repertoire and overcome some of the defects of natural compounds (Xiao et al., 2020). Dvorak Z et al. synthesized the FKK series that mimics the docking of the natural indole and IPA with PXR. These newly designed compounds exhibited significant intestinal anti-inflammatory effects and were nontoxic compared with other known PXR xenobiotics (Dvorak et al., 2020). Screening indole and indazole compounds with diverse structures and combined computational and experimental studies, Chen et al. generated multiheterocyclic AHR agonists, PY109 and PY108, which displayed biostability and achieved a therapeutic effect at a very low dose (Chen et al., 2020). 6-Phosphofructo-2-kinase/fructose-2,6-biphosphatase 3 (PFKFB3) is a master regulatory gene of glycolysis. Indole supplementation reduces hepatic steatosis and inflammation in HFD-fed mice in a myeloid cell PFKFB3–dependent manner. Therefore, indole mimics or specific activation of PFKFB3 expression in macrophages could be feasible methods to prevent and treat inflammation-related diseases, such as NAFLD (Ma et al., 2020).
Recently, medicinal chemists have designed various active pharmacophores of indole analogs with antidiabetic and antidyslipidemic activities (Kumari and Singh, 2019). For example, a series of enantiomerically pure indole derivatives, 3a-rvia Friedel-Crafts alkylation of indole 1 with enones 2a-r, were designed, some of which were identified as potent inhibitors of α-glucosidase (IC50 = 4.3 ± 0.13–43.9 ± 0.51 μM); the activity is several folds higher than that of acarbose (IC50 = 840 ± 1.73 μM) (Islam et al., 2018). Compound 13 m is a hybrid derivative of indole and triazole, showing effective anti-lipogenesis activity with an IC-50 value of 1.67 μM. Compound 13 m improves dyslipidemia in HFD-fed hamsters by activating reverse cholesterol transport, which has a therapeutic tendency for the intervention of obesity and metabolic syndrome (Rajan et al., 2018). However, compared with the progress of the new synthetic indole molecules in the field of anticancer, antibacterial, or anti–Alzheimer’s disease, no antidiabetic and antihyperlipidemic active molecules of indole are currently under clinical trials.
Genetic Manipulating
Apart from drug compounds, bacterial species engineering can be another effective avenue for modulating Trp metabolism. Genetic manipulation can transfer the gene of enzymes encoding Trp metabolism to some common and genetically tractable commensal. Codon optimizing and using a constitutively active phage-derived promoter to control the codon may be a good choice for facilitating robust expression in an exogenous host. IAA supplementation can restore the expression of IL-22 and have a protective effect on alcohol-induced steatohepatitis mice. Hendrikx et al. engineered Lactobacillus reuteri and found that supplementation of engineered probiotics can produce IL-22, reduce liver disease caused by chronic alcohol intake, and have clinical application prospects (Hendrikx et al., 2019). Compared with Bacteroides thetaiotaomicron Trp D-colonized mice, germ-free mice colonized with engineered Bacteroides thetaiotaomicron Trp D+ had significantly higher tryptamine levels to reduce DSS-induced epithelial barrier disruption and accelerate whole gut transit in mice (Bhattarai et al., 2018; Bhattarai et al., 2020). Lemon exosome-like nanoparticles (LELNs)-manipulated Streptococcus thermophilus ST-21 and Lactobacillus rhamnosus GG probiotic mixture can decrease the mortality of Clostridioides difficile infection in mice partly by increasing the levels of ILA and indole-3-carboxaldehyde (I3Ald) to activate the AhR/IL-22 signaling. Meanwhile, the increased lactic acid inhibited the expression of indole biosynthesis gene TnaA to decrease the production of indole in an AhR-independent manner, both of which led to a decrease in Clostridioides difficile fecal shedding (Lei et al., 2020).
Potential Side Effects of Indole and Its Derivates
Intestinal Side Effects
According to reports on the potential side effects of indole and some derivatives under pathological conditions, some cautions have been raised. The pathogenic nature of bacteria may lead to infections, cancer, or even death. In weaned pigs, Trp supplementation negatively affects the small intestinal structure. Compared with Trp-free and low Trp diets, the piglets fed a high Trp diet significantly increased crypt depth and significantly decreased villus height to the crypt depth ratio (VH/CD) in the jejunum, and the mRNA expressions of the tight junction proteins, occludin and ZO-1, were also decreased. (Tossou et al., 2016). It has been reported that ILA supplementation impairs the effect of total paeoniflorin in the treatment of colitis in mice. Under the background of intestinal inflammation, the local accumulation of ILA could lead to the disruption of epithelial autophagy, increase the susceptibility of colon injury, and promote the progression of colitis in mice (Fan et al., 2020). Indole and its derivates can promote the expression of anti-inflammatory factor IL-22 to regulate intestinal homeostasis, but in the later stage of cancer, IL-22 may promote tumor progression (Hernandez et al., 2018). Ruminococcus gnavus, the tryptamine producer, is also an avid mucin degrader and only transplantation R. gnavus may lead to the progression of IBD (Henke et al., 2019). Several Peptostreptococcus species contain a gene cluster enabling the production of IA, but patients with bacteremia from Peptostreptococcus species have an increased risk of colorectal cancer (Kwong et al., 2018). In addition, indole levels are increased in patients with Clostridioides difficile infection, and high indole levels might play a role in Clostridioides difficile proliferation by limiting the growth of beneficial indole-sensitive bacteria and altering the colonization resistance (Darkoh et al., 2019).
Liver Side Effects
A Trp-enriched diet and indole intake could raise the risk of portal hypertension in patients with liver cirrhosis. As a common complication of liver cirrhosis, portal hypertension is associated with a poor prognosis. However, a lower dose (<10 mg/kg) of indole can influence portal blood pressure by increasing the portal blood flow. Both healthy rats and rats with portal hypertension induced by liver cirrhosis show an increased portal blood level after intracolonic administration of indole (Huc et al., 2018). A recent study found that in CCL4-induced liver fibrosis mice, oral IPA intervention (20 mg/kg/d) aggravated liver damage and fibrosis by activating HSCs via the TGF-β1/Smad signaling pathway (Liu et al., 2021). Indole and its derivates can activate PXR and/or AhR; however, there are reports that both activation and knockout of PXR exhibit an increase in lipid accumulation in hepatoma cell lines (Bitter et al., 2015). Similarly, overexpression of AhR in the liver leads to insulin resistance (Remillard and Bunce, 2002; Kumar et al., 2021). Low-affinity AhR allele-expressing mice are less sensitive to HFD-induced obesity and liver steatosis (Kerley-Hamilton et al., 2012). However, these reports did not directly use indole and its derivatives as interventions. Therefore, further research is needed to clarify the relationship between Trp metabolic derivatives and the function of liver AhR/PXR signaling under pathological conditions.
Limitations and Perspectives
Increasing evidence has clarified the benefits of indole and its derivatives on immune homeostasis and metabolism. However, at present, the main reported bacterial genera are focused on Clostridium, Lactobacillus, Bacteroides, Peptostreptococcus, Parabacteroides, Bifidobacterium, Escherichia, and Ruminococcus (Gasaly et al., 2021), but bacterial metabolism is a much more complex and diverse process than we summarized above. Intestinal symbiotic bacteria express a variety of catalytic enzymes, and strains of the same bacterial species can also have different responses to environmental stressors (Zhao et al., 2018). The whole scenario of the related metabolism and the molecular mechanisms of physiology and pathology has not been fully revealed.
Currently, the emerging intervention tools offer a promising approach to therapeutic strategies, but the limitation of the technology is another obstacle. Take genetic engineering methods as an example. Enzymes derived from other species are heterologous and therefore may limit the high production of indole and its derivatives in human symbiotic bacteria. Compared to more common laboratory strains such as E. coli, there are relatively few genetic tools available for manipulating human symbiotic gut bacteria. In addition, in the context of the complex and dynamic gut ecosystem, heterologous enzymes may impair the adaptability of engineered bacteria. For example, the ratio of Bacteroides/Firmicutes in the intestines of NASH patients is significantly reduced (Sobhonslidsuk et al., 2018), so it may be difficult to ensure the therapeutic effect of engineered Bacteroides thetaiotaomicron transplanted to NAFLD patients. Therefore, it is necessary to promote the metabolism and stress tolerance of engineered bacteria.
The instability among existing research results also hinders further progress in drug development. The different doses also lead to different effects. In vitro, indole inhibits the release of GLP-1 at 0.3 mM, while enhancing GLP-1 release at 1 nM. It is not yet clear which concentration of indole effects is dominant in vivo (Chimerel et al., 2014). In addition, indole can activate AHR in most reports, but indole at 100–250 μM exhibits AHR antagonist activity, which inhibits TCDD-induced AhR activation and Cyp1a1 and Cyp1b1 expression in colonic crypts of mice (Jin et al., 2014). Therefore, a comprehensive understanding of the effects and causality of indole and its derivatives under pathological conditions is necessary to assist the development of individualized bacteriotherapy.
Author Contributions
XL and BZ: writing—original draft. YZ: writing—review and editing. YH and YZ: funding acquisition.
Funding
This work was supported by the National Natural Science Foundation of China (No. 81830119 and 82174249); the Clinical Research Plan of SHCD (No. SHD2020CR2049B); and the Shanghai Science and Technology Commission (No. 19401970300 and 20Y21901700).
Conflict of Interest
The authors declare that the research was conducted in the absence of any commercial or financial relationships that could be construed as a potential conflict of interest.
Publisher’s Note
All claims expressed in this article are solely those of the authors and do not necessarily represent those of their affiliated organizations, or those of the publisher, the editors, and the reviewers. Any product that may be evaluated in this article, or claim that may be made by its manufacturer, is not guaranteed or endorsed by the publisher.
References
Abildgaard, A., Elfving, B., Hokland, M., Wegener, G., and Lund, S. (2018). The Microbial Metabolite Indole-3-Propionic Acid Improves Glucose Metabolism in Rats, but Does Not Affect Behaviour. Arch. Physiol. Biochem. 124 (4), 306–312. doi:10.1080/13813455.2017.1398262
Alexeev, E. E., Lanis, J. M., Kao, D. J., Campbell, E. L., Kelly, C. J., Battista, K. D., et al. (2018). Microbiota-Derived Indole Metabolites Promote Human and Murine Intestinal Homeostasis through Regulation of Interleukin-10 Receptor. Am. J. Pathol. 188 (5), 1183–1194. doi:10.1016/j.ajpath.2018.01.011
Aoki, R., Aoki-Yoshida, A., Suzuki, C., and Takayama, Y. (2018). Indole-3-Pyruvic Acid, an Aryl Hydrocarbon Receptor Activator, Suppresses Experimental Colitis in Mice. J. Immunol. 201 (12), 3683–3693. doi:10.4049/jimmunol.1701734
Arnoriaga-Rodriguez, M., Mayneris-Perxachs, J., Burokas, A., Perez-Brocal, V., Moya, A., Portero-Otin, M., et al. (2020). Gut Bacterial ClpB-like Gene Function Is Associated with Decreased Body Weight and a Characteristic Microbiota Profile. Microbiome 8 (1), 59. doi:10.1186/s40168-020-00837-6
Banoglu, E., and King, R. S. (2002). Sulfation of Indoxyl by Human and Rat Aryl (Phenol) Sulfotransferases to Form Indoxyl Sulfate. Eur. J. Drug Metab. Pharmacokinet. 27 (2), 135–140. doi:10.1007/BF03190428
Banoglu, E., Jha, G. G., and King, R. S. (2001). Hepatic Microsomal Metabolism of Indole to Indoxyl, a Precursor of Indoxyl Sulfate. Eur. J. Drug Metab. Pharmacokinet. 26 (4), 235–240. doi:10.1007/BF03226377
Bansal, T., Alaniz, R. C., Wood, T. K., and Jayaraman, A. (2010). The Bacterial Signal Indole Increases Epithelial-Cell Tight-junction Resistance and Attenuates Indicators of Inflammation. Proc. Natl. Acad. Sci. U S A. 107 (1), 228–233. doi:10.1073/pnas.0906112107
Beaumont, M., Neyrinck, A. M., Olivares, M., Rodriguez, J., de Rocca Serra, A., Roumain, M., et al. (2018). The Gut Microbiota Metabolite Indole Alleviates Liver Inflammation in Mice. FASEB J. 32, fj201800544. doi:10.1096/fj.201800544
Belizario, J. E., and Faintuch, J. (2018). Microbiome and Gut Dysbiosis. Exp. Suppl. 109, 459–476. doi:10.1007/978-3-319-74932-7_13
Bell, F. R., and Webber, D. E. (1979). Gastric Emptying and Secretion in the Calf on Duodenal Infusion of Tryptophan, Tryptamine and 5-hydroxytryptamine. J. Physiol. 291, 413–423. doi:10.1113/jphysiol.1979.sp012822
Bhattarai, Y., Williams, B. B., Battaglioli, E. J., Whitaker, W. R., Till, L., Grover, M., et al. (2018). Gut Microbiota-Produced Tryptamine Activates an Epithelial G-Protein-Coupled Receptor to Increase Colonic Secretion. Cell Host Microbe 23 (6), 775–785 e775. doi:10.1016/j.chom.2018.05.004
Bhattarai, Y., Jie, S., Linden, D. R., Ghatak, S., Mars, R. A. T., Williams, B. B., et al. (2020). Bacterially Derived Tryptamine Increases Mucus Release by Activating a Host Receptor in a Mouse Model of Inflammatory Bowel Disease. iScience 23 (12), 101798. doi:10.1016/j.isci.2020.101798
Bitter, A., Rummele, P., Klein, K., Kandel, B. A., Rieger, J. K., Nussler, A. K., et al. (2015). Pregnane X Receptor Activation and Silencing Promote Steatosis of Human Hepatic Cells by Distinct Lipogenic Mechanisms. Arch. Toxicol. 89 (11), 2089–2103. doi:10.1007/s00204-014-1348-x
Brunius, C., Vidanarachchi, J. K., Tomankova, J., Lundstrom, K., Andersson, K., and Zamaratskaia, G. (2016). Skatole Metabolites in Urine as a Biological Marker of Pigs with Enhanced Hepatic Metabolism. Animal 10 (10), 1734–1740. doi:10.1017/S1751731116000574
Busbee, P. B., Menzel, L., Alrafas, H. R., Dopkins, N., Becker, W., Miranda, K., et al. (2020). Indole-3-carbinol Prevents Colitis and Associated Microbial Dysbiosis in an IL-22-Dependent Manner. JCI Insight 5 (1), e127551. doi:10.1172/jci.insight.127551
Cervantes-Barragan, L., Chai, J. N., Tianero, M. D., Luccia, B. D., Ahern, P. P., Merriman, J., et al. (2017). Lactobacillus Reuteri Induces Gut Intraepithelial CD4+CD8αα+ T Cells. Science 357 (6353), 806–810. doi:10.1126/science.aah5825
Chen, Y., and Tian, Z. (2020). Roles of Hepatic Innate and Innate-like Lymphocytes in Nonalcoholic Steatohepatitis. Front. Immunol. 11, 1500. doi:10.3389/fimmu.2020.01500
Chen, J., Haller, C. A., Jernigan, F. E., Koerner, S. K., Wong, D. J., Wang, Y., et al. (2020). Modulation of Lymphocyte-Mediated Tissue Repair by Rational Design of Heterocyclic Aryl Hydrocarbon Receptor Agonists. Sci. Adv. 6 (3), eaay8230. doi:10.1126/sciadv.aay8230
Chimerel, C., Emery, E., Summers, D. K., Keyser, U., Gribble, F. M., and Reimann, F. (2014). Bacterial Metabolite Indole Modulates Incretin Secretion from Intestinal Enteroendocrine L Cells. Cell Rep 9 (4), 1202–1208. doi:10.1016/j.celrep.2014.10.032
Dai, X., Mashiguchi, K., Chen, Q., Kasahara, H., Kamiya, Y., Ojha, S., et al. (2013). The Biochemical Mechanism of Auxin Biosynthesis by an Arabidopsis YUCCA Flavin-Containing Monooxygenase. J. Biol. Chem. 288 (3), 1448–1457. doi:10.1074/jbc.M112.424077
Darkoh, C., Chappell, C., Gonzales, C., and Okhuysen, P. (2015). A Rapid and Specific Method for the Detection of Indole in Complex Biological Samples. Appl. Environ. Microbiol. 81 (23), 8093–8097. doi:10.1128/AEM.02787-15
Darkoh, C., Plants-Paris, K., Bishoff, D., DuPont, H. L., and Turnbaugh, P. J. (2019). Clostridium difficile Modulates the Gut Microbiota by Inducing the Production of Indole, an Interkingdom Signaling and Antimicrobial Molecule. mSystems 4 (2), e00346–18. doi:10.1128/mSystems.00346-18
Daujat-Chavanieu, M., and Gerbal-Chaloin, S. (2020). Regulation of CAR and PXR Expression in Health and Disease. Cells 9 (11), 2395. doi:10.3390/cells9112395
de Mello, V. D., Paananen, J., Lindstrom, J., Lankinen, M. A., Shi, L., Kuusisto, J., et al. (2017). Indolepropionic Acid and Novel Lipid Metabolites Are Associated with a Lower Risk of Type 2 Diabetes in the Finnish Diabetes Prevention Study. Sci. Rep. 7, 46337. doi:10.1038/srep46337
Dodd, D., Spitzer, M. H., Van Treuren, W., Merrill, B. D., Hryckowian, A. J., Higginbottom, S. K., et al. (2017). A Gut Bacterial Pathway Metabolizes Aromatic Amino Acids into Nine Circulating Metabolites. Nature 551 (7682), 648–652. doi:10.1038/nature24661
Dong, F., Hao, F., Murray, I. A., Smith, P. B., Koo, I., Tindall, A. M., et al. (2020). Intestinal Microbiota-Derived Tryptophan Metabolites Are Predictive of Ah Receptor Activity. Gut Microbes 12 (1), 1–24. doi:10.1080/19490976.2020.1788899
Dvorak, Z., Kopp, F., Costello, C. M., Kemp, J. S., Li, H., Vrzalova, A., et al. (2020). Targeting the Pregnane X Receptor Using Microbial Metabolite Mimicry. EMBO Mol. Med. 12 (4), e11621. doi:10.15252/emmm.201911621
Fan, Q., Guan, X., Hou, Y., Liu, Y., Wei, W., Cai, X., et al. (2020). Paeoniflorin Modulates Gut Microbial Production of Indole-3-Lactate and Epithelial Autophagy to Alleviate Colitis in Mice. Phytomedicine 79, 153345. doi:10.1016/j.phymed.2020.153345
Folkes, L. K., Dennis, M. F., Stratford, M. R., Candeias, L. P., and Wardman, P. (1999). Peroxidase-catalyzed Effects of Indole-3-Acetic Acid and Analogues on Lipid Membranes, DNA, and Mammalian Cells In Vitro. Biochem. Pharmacol. 57 (4), 375–382. doi:10.1016/s0006-2952(98)00323-2
Gao, J., Xu, K., Liu, H., Liu, G., Bai, M., Peng, C., et al. (2018). Impact of the Gut Microbiota on Intestinal Immunity Mediated by Tryptophan Metabolism. Front Cel Infect Microbiol 8, 13. doi:10.3389/fcimb.2018.00013
Gasaly, N., de Vos, P., and Hermoso, M. A. (2021). Impact of Bacterial Metabolites on Gut Barrier Function and Host Immunity: A Focus on Bacterial Metabolism and its Relevance for Intestinal Inflammation. Front. Immunol. 12, 658354. doi:10.3389/fimmu.2021.658354
Gillam, E. M., Notley, L. M., Cai, H., De Voss, J. J., and Guengerich, F. P. (2000). Oxidation of Indole by Cytochrome P450 Enzymes. Biochemistry 39 (45), 13817–13824. doi:10.1021/bi001229u
Girer, N. G., Tomlinson, C. R., and Elferink, C. J. (2020). The Aryl Hydrocarbon Receptor in Energy Balance: The Road from Dioxin-Induced Wasting Syndrome to Combating Obesity with Ahr Ligands. Int. J. Mol. Sci. 22 (1), 49. doi:10.3390/ijms22010049
Gostner, J. M., Geisler, S., Stonig, M., Mair, L., Sperner-Unterweger, B., and Fuchs, D. (2020). Tryptophan Metabolism and Related Pathways in Psychoneuroimmunology: The Impact of Nutrition and Lifestyle. Neuropsychobiology 79 (1), 89–99. doi:10.1159/000496293
Hendrikx, T., and Schnabl, B. (2019). Indoles: Metabolites Produced by Intestinal Bacteria Capable of Controlling Liver Disease Manifestation. J. Intern. Med. 286 (1), 32–40. doi:10.1111/joim.12892
Hendrikx, T., Duan, Y., Wang, Y., Oh, J. H., Alexander, L. M., Huang, W., et al. (2019). Bacteria Engineered to Produce IL-22 in Intestine Induce Expression of REG3G to Reduce Ethanol-Induced Liver Disease in Mice. Gut 68 (8), 1504–1515. doi:10.1136/gutjnl-2018-317232
Henke, M. T., Kenny, D. J., Cassilly, C. D., Vlamakis, H., Xavier, R. J., and Clardy, J. (2019). Ruminococcus Gnavus, a Member of the Human Gut Microbiome Associated with Crohn's Disease, Produces an Inflammatory Polysaccharide. Proc. Natl. Acad. Sci. U S A. 116 (26), 12672–12677. doi:10.1073/pnas.1904099116
Hernandez, P., Gronke, K., and Diefenbach, A. (2018). A Catch-22: Interleukin-22 and Cancer. Eur. J. Immunol. 48 (1), 15–31. doi:10.1002/eji.201747183
Hou, Q., Ye, L., Liu, H., Huang, L., Yang, Q., Turner, J. R., et al. (2018). Lactobacillus Accelerates ISCs Regeneration to Protect the Integrity of Intestinal Mucosa through Activation of STAT3 Signaling Pathway Induced by LPLs Secretion of IL-22. Cell Death Differ 25 (9), 1657–1670. doi:10.1038/s41418-018-0070-2
Huc, T., Konop, M., Onyszkiewicz, M., Podsadni, P., Szczepanska, A., Turlo, J., et al. (2018). Colonic Indole, Gut Bacteria Metabolite of Tryptophan, Increases portal Blood Pressure in Rats. Am. J. Physiol. Regul. Integr. Comp. Physiol. 315 (4), R646–R655. doi:10.1152/ajpregu.00111.2018
Hwang, S. J., Hwang, Y. J., Yun, M. O., Kim, J. H., Oh, G. S., and Park, J. H. (2013). Indoxyl 3-sulfate Stimulates Th17 Differentiation Enhancing Phosphorylation of C-Src and STAT3 to Worsen Experimental Autoimmune Encephalomyelitis. Toxicol. Lett. 220 (2), 109–117. doi:10.1016/j.toxlet.2013.04.016
Ikuta, T., Kurosumi, M., Yatsuoka, T., and Nishimura, Y. (2016). Tissue Distribution of Aryl Hydrocarbon Receptor in the Intestine: Implication of Putative Roles in Tumor Suppression. Exp. Cel Res. 343 (2), 126–134. doi:10.1016/j.yexcr.2016.03.012
Illes, P., Krasulova, K., Vyhlidalova, B., Poulikova, K., Marcalikova, A., Pecinkova, P., et al. (2020). Indole Microbial Intestinal Metabolites Expand the Repertoire of Ligands and Agonists of the Human Pregnane X Receptor. Toxicol. Lett. 334, 87–93. doi:10.1016/j.toxlet.2020.09.015
Islam, M. S., Barakat, A., Al-Majid, A. M., Ali, M., Yousuf, S., Iqbal Choudhary, M., et al. (2018). Catalytic Asymmetric Synthesis of Indole Derivatives as Novel α-glucosidase Inhibitors In Vitro. Bioorg. Chem. 79, 350–354. doi:10.1016/j.bioorg.2018.05.004
Jennis, M., Cavanaugh, C. R., Leo, G. C., Mabus, J. R., Lenhard, J., and Hornby, P. J. (2018). Microbiota-derived Tryptophan Indoles Increase after Gastric Bypass Surgery and Reduce Intestinal Permeability In Vitro and In Vivo. Neurogastroenterol. Motil. 30 (2), e13178. doi:10.1111/nmo.13178
Ji, Y., Gao, Y., Chen, H., Yin, Y., and Zhang, W. (2019). Indole-3-Acetic Acid Alleviates Nonalcoholic Fatty Liver Disease in Mice via Attenuation of Hepatic Lipogenesis, and Oxidative and Inflammatory Stress. Nutrients 11 (9), 2062. doi:10.3390/nu11092062
Jin, U. H., Lee, S. O., Sridharan, G., Lee, K., Davidson, L. A., Jayaraman, A., et al. (2014). Microbiome-derived Tryptophan Metabolites and Their Aryl Hydrocarbon Receptor-dependent Agonist and Antagonist Activities. Mol. Pharmacol. 85 (5), 777–788. doi:10.1124/mol.113.091165
Jones, R. S. (1982). Tryptamine: a Neuromodulator or Neurotransmitter in Mammalian Brain? Prog. Neurobiol. 19 (1-2), 117–139. doi:10.1016/0301-0082(82)90023-5
Keir, M., Yi, Y., Lu, T., and Ghilardi, N. (2020). The Role of IL-22 in Intestinal Health and Disease. J. Exp. Med. 217 (3), e20192195. doi:10.1084/jem.20192195
Kerley-Hamilton, J. S., Trask, H. W., Ridley, C. J., Dufour, E., Ringelberg, C. S., Nurinova, N., et al. (2012). Obesity Is Mediated by Differential Aryl Hydrocarbon Receptor Signaling in Mice Fed a Western Diet. Environ. Health Perspect. 120 (9), 1252–1259. doi:10.1289/ehp.1205003
Keszthelyi, D., Troost, F. J., and Masclee, A. A. (2009). Understanding the Role of Tryptophan and Serotonin Metabolism in Gastrointestinal Function. Neurogastroenterol. Motil. 21 (12), 1239–1249. doi:10.1111/j.1365-2982.2009.01370.x
Kim, J., and Park, W. (2015). Indole: a Signaling Molecule or a Mere Metabolic Byproduct that Alters Bacterial Physiology at a High Concentration? J. Microbiol. 53 (7), 421–428. doi:10.1007/s12275-015-5273-3
Kim, W. H., Lillehoj, H. S., and Min, W. (2019). Indole Treatment Alleviates Intestinal Tissue Damage Induced by Chicken Coccidiosis through Activation of the Aryl Hydrocarbon Receptor. Front. Immunol. 10, 560. doi:10.3389/fimmu.2019.00560
Kim, C. S., Li, J. H., Barco, B., Park, H. B., Gatsios, A., Damania, A., et al. (2020). Cellular Stress Upregulates Indole Signaling Metabolites in Escherichia coli. Cell Chem Biol 27 (6), 698–707 e697. doi:10.1016/j.chembiol.2020.03.003
Knarreborg, A., Beck, J., Jensen, M. T., Laue, A., Agergaard, N., and Jensen, B. B. (2016). Effect of Non-Starch Polysaccharides on Production and Absorption of Indolic Compounds in Entire Male Pigs. Anim. Sci. 74 (3), 445–453. doi:10.1017/s1357729800052590
Krishnan, S., Ding, Y., Saedi, N., Choi, M., Sridharan, G. V., Sherr, D. H., et al. (2018). Gut Microbiota-Derived Tryptophan Metabolites Modulate Inflammatory Response in Hepatocytes and Macrophages. Cel Rep 23 (4), 1099–1111. doi:10.1016/j.celrep.2018.03.109
Kumar, A., and Sperandio, V. (2019). Indole Signaling at the Host-Microbiota-Pathogen Interface. mBio 10 (3), e01031–19. doi:10.1128/mBio.01031-19
Kumar, D., Sharma, S., Kalra, S., Singh, G., Monga, V., and Kumar, B. (2020). Medicinal Perspective of Indole Derivatives: Recent Developments and Structure-Activity Relationship Studies. Curr. Drug Targets 21 (9), 864–891. doi:10.2174/1389450121666200310115327
Kumar, A., Sundaram, K., Mu, J., Dryden, G. W., Sriwastva, M. K., Lei, C., et al. (2021). High-fat Diet-Induced Upregulation of Exosomal Phosphatidylcholine Contributes to Insulin Resistance. Nat. Commun. 12 (1), 213. doi:10.1038/s41467-020-20500-w
Kumari, A., and Singh, R. K. (2019). Medicinal Chemistry of Indole Derivatives: Current to Future Therapeutic Prospectives. Bioorg. Chem. 89, 103021. doi:10.1016/j.bioorg.2019.103021
Kwong, T. N. Y., Wang, X., Nakatsu, G., Chow, T. C., Tipoe, T., Dai, R. Z. W., et al. (2018). Association Between Bacteremia From Specific Microbes and Subsequent Diagnosis of Colorectal Cancer. Gastroenterology 155 (2), 383–390 e388. doi:10.1053/j.gastro.2018.04.028
Lamas, B., Richard, M. L., Leducq, V., Pham, H. P., Michel, M. L., Da Costa, G., et al. (2016). CARD9 Impacts Colitis by Altering Gut Microbiota Metabolism of Tryptophan into Aryl Hydrocarbon Receptor Ligands. Nat. Med. 22 (6), 598–605. doi:10.1038/nm.4102
Lamas, B., Natividad, J. M., and Sokol, H. (2018). Aryl Hydrocarbon Receptor and Intestinal Immunity. Mucosal Immunol. 11 (4), 1024–1038. doi:10.1038/s41385-018-0019-2
Lano, G., Burtey, S., and Sallee, M. (2020). Indoxyl Sulfate, a Uremic Endotheliotoxin. Toxins (Basel) 12 (4), 229. doi:10.3390/toxins12040229
Laurans, L., Venteclef, N., Haddad, Y., Chajadine, M., Alzaid, F., Metghalchi, S., et al. (2018). Genetic Deficiency of Indoleamine 2,3-dioxygenase Promotes Gut Microbiota-Mediated Metabolic Health. Nat. Med. 24 (8), 1113–1120. doi:10.1038/s41591-018-0060-4
Lee, J. H., and Lee, J. (2010). Indole as an Intercellular Signal in Microbial Communities. FEMS Microbiol. Rev. 34 (4), 426–444. doi:10.1111/j.1574-6976.2009.00204.x
Lee, Y., Kim, T., Kim, Y., Lee, S., Kim, S., Kang, S., et al. (2018). Microbiota-Derived Lactate Accelerates Intestinal Stem-Cell-Mediated Epithelial Development. Cell Host Microbe 24 (6), 833–846.e6. doi:10.1016/j.chom.2018.11.002
Lei, C., Mu, J., Teng, Y., He, L., Xu, F., Zhang, X., et al. (2020). Lemon Exosome-like Nanoparticles-Manipulated Probiotics Protect Mice from C. D Iff Infection. iScience 23 (10), 101571. doi:10.1016/j.isci.2020.101571
Leong, S. C., and Sirich, T. L. (2016). Indoxyl Sulfate-Review of Toxicity and Therapeutic Strategies. Toxins (Basel) 8 (12), 358. doi:10.3390/toxins8120358
Li, G., and Young, K. D. (2013). Indole Production by the Tryptophanase TnaA in Escherichia coli Is Determined by the Amount of Exogenous Tryptophan. Microbiology (Reading) 159 (Pt 2), 402–410. doi:10.1099/mic.0.064139-0
Li, J., Zhang, L., Wu, T., Li, Y., Zhou, X., and Ruan, Z. (2021). Indole-3-Propionic Acid Improved the Intestinal Barrier by Enhancing Epithelial Barrier and Mucus Barrier. J. Agric. Food Chem. 69 (5), 1487–1495. doi:10.1021/acs.jafc.0c05205
Lin, Y. H., Luck, H., Khan, S., Schneeberger, P. H. H., Tsai, S., Clemente-Casares, X., et al. (2019). Aryl Hydrocarbon Receptor Agonist Indigo Protects against Obesity-Related Insulin Resistance through Modulation of Intestinal and Metabolic Tissue Immunity. Int. J. Obes. (Lond) 43 (12), 2407–2421. doi:10.1038/s41366-019-0340-1
Liu, X., Zhao, K., Yang, X., and Zhao, Y. (2019). Gut Microbiota and Metabolome Response of Decaisnea Insignis Seed Oil on Metabolism Disorder Induced by Excess Alcohol Consumption. J. Agric. Food Chem. 67 (38), 10667–10677. doi:10.1021/acs.jafc.9b04792
Liu, Y., Hou, Y., Wang, G., Zheng, X., and Hao, H. (2020). Gut Microbial Metabolites of Aromatic Amino Acids as Signals in Host-Microbe Interplay. Trends Endocrinol. Metab. 31 (11), 818–834. doi:10.1016/j.tem.2020.02.012
Liu, F., Sun, C., Chen, Y., Du, F., Yang, Y., and Wu, G. (2021). Indole-3-Propionic Acid-Aggravated CCl4-Induced Liver Fibrosis via the TGF-β1/Smads Signaling Pathway. J. Clin. Transl. Hepatol. doi:10.14218/jcth.2021.00032
Ma, Q., Zhang, X., and Qu, Y. (2018). Biodegradation and Biotransformation of Indole: Advances and Perspectives. Front. Microbiol. 9, 2625. doi:10.3389/fmicb.2018.02625
Ma, L., Li, H., Hu, J., Zheng, J., Zhou, J., Botchlett, R., et al. (2020). Indole Alleviates Diet-Induced Hepatic Steatosis and Inflammation in a Manner Involving Myeloid Cell 6-Phosphofructo-2-Kinase/Fructose-2,6-Biphosphatase 3. Hepatology 72 (4), 1191–1203. doi:10.1002/hep.31115
Malhotra, M., and Srivastava, S. (2008). Organization of the ipdC Region Regulates IAA Levels in Different Azospirillum Brasilense Strains: Molecular and Functional Analysis of ipdC in Strain SM. Environ. Microbiol. 10 (5), 1365–1373. doi:10.1111/j.1462-2920.2007.01529.x
Marcobal, A., Kashyap, P. C., Nelson, T. A., Aronov, P. A., Donia, M. S., Spormann, A., et al. (2013). A Metabolomic View of How the Human Gut Microbiota Impacts the Host Metabolome Using Humanized and Gnotobiotic Mice. ISME J. 7 (10), 1933–1943. doi:10.1038/ismej.2013.89
Mawe, G. M., and Hoffman, J. M. (2013). Serotonin Signalling in the Gut-Ffunctions, Dysfunctions and Therapeutic Targets. Nat. Rev. Gastroenterol. Hepatol. 10 (8), 473–486. doi:10.1038/nrgastro.2013.105
McGettrick, A. F., Corcoran, S. E., Barry, P. J., McFarland, J., Cres, C., Curtis, A. M., et al. (2016). Trypanosoma Brucei Metabolite Indolepyruvate Decreases HIF-1alpha and Glycolysis in Macrophages as a Mechanism of Innate Immune Evasion. Proc. Natl. Acad. Sci. U S A. 113 (48), E7778–E7787. doi:10.1073/pnas.1608221113
McLaughlin, T., Ackerman, S. E., Shen, L., and Engleman, E. (2017). Role of Innate and Adaptive Immunity in Obesity-Associated Metabolic Disease. J. Clin. Invest. 127 (1), 5–13. doi:10.1172/JCI88876
Meng, D., Sommella, E., Salviati, E., Campiglia, P., Ganguli, K., Djebali, K., et al. (2020). Indole-3-lactic Acid, a Metabolite of Tryptophan, Secreted by Bifidobacterium Longum Subspecies Infantis Is Anti-inflammatory in the Immature Intestine. Pediatr. Res. 88 (2), 209–217. doi:10.1038/s41390-019-0740-x
Metidji, A., Omenetti, S., Crotta, S., Li, Y., Nye, E., Ross, E., et al. (2018). The Environmental Sensor AHR Protects from Inflammatory Damage by Maintaining Intestinal Stem Cell Homeostasis and Barrier Integrity. Immunity 49 (2), 353–362.e5. doi:10.1016/j.immuni.2018.07.010
Miyagi, M., Wilson, R., Saigusa, D., Umeda, K., Saijo, R., Hager, C. L., et al. (2020). Indole-3-acetic Acid Synthesized through the Indole-3-Pyruvate Pathway Promotes Candida tropicalis Biofilm Formation. PLoS One 15 (12), e0244246. doi:10.1371/journal.pone.0244246
Mucha, P., Skoczynska, A., Malecka, M., Hikisz, P., and Budzisz, E. (2021). Overview of the Antioxidant and Anti-inflammatory Activities of Selected Plant Compounds and Their Metal Ions Complexes. Molecules 26 (16), 4886. doi:10.3390/molecules26164886
Nguyen, N. T., Hanieh, H., Nakahama, T., and Kishimoto, T. (2013). The Roles of Aryl Hydrocarbon Receptor in Immune Responses. Int. Immunol. 25 (6), 335–343. doi:10.1093/intimm/dxt011
Nikolaus, S., Schulte, B., Al-Massad, N., Thieme, F., Schulte, D. M., Bethge, J., et al. (2017). Increased Tryptophan Metabolism Is Associated with Activity of Inflammatory Bowel Diseases. Gastroenterology 153 (6), 1504–1516.e2. doi:10.1053/j.gastro.2017.08.028
Nishizawa, T., Aldrich, C. C., and Sherman, D. H. (2005). Molecular Analysis of the Rebeccamycin L-Amino Acid Oxidase from Lechevalieria Aerocolonigenes ATCC 39243. J. Bacteriol. 187 (6), 2084–2092. doi:10.1128/JB.187.6.2084-2092.2005
Pinero-Fernandez, S., Chimerel, C., Keyser, U. F., and Summers, D. K. (2011). Indole Transport across Escherichia coli Membranes. J. Bacteriol. 193 (8), 1793–1798. doi:10.1128/JB.01477-10
Powell, D. N., Swimm, A., Sonowal, R., Bretin, A., Gewirtz, A. T., Jones, R. M., et al. (2020). Indoles from the Commensal Microbiota Act via the AHR and IL-10 to Tune the Cellular Composition of the Colonic Epithelium during Aging. Proc. Natl. Acad. Sci. U S A. 117 (35), 21519–21526. doi:10.1073/pnas.2003004117
Qi, Q., Li, J., Yu, B., Moon, J. Y., Chai, J. C., Merino, J., et al. (2021). Host and Gut Microbial Tryptophan Metabolism and Type 2 Diabetes: an Integrative Analysis of Host Genetics, Diet, Gut Microbiome and Circulating Metabolites in Cohort Studies. Gut, gutjnl-2021-324053. doi:10.1136/gutjnl-2021-324053
Rajan, S., Puri, S., Kumar, D., Babu, M. H., Shankar, K., Varshney, S., et al. (2018). Novel Indole and Triazole Based Hybrid Molecules Exhibit Potent Anti-adipogenic and Antidyslipidemic Activity by Activating Wnt3a/β-Catenin Pathway. Eur. J. Med. Chem. 143, 1345–1360. doi:10.1016/j.ejmech.2017.10.034
Rasmussen, M. K., Daujat-Chavanieu, M., and Gerbal-Chaloin, S. (2017). Activation of the Aryl Hydrocarbon Receptor Decreases Rifampicin-Induced CYP3A4 Expression in Primary Human Hepatocytes and HepaRG. Toxicol. Lett. 277, 1–8. doi:10.1016/j.toxlet.2017.05.029
Remillard, R., and Bunce, N. J. E. h. p. (2002). Linking Dioxins to Diabetes: Eidemiology Biology Plausibility. Environ. Health Perspect 110 (9), 853–858. doi:10.1289/ehp.02110853
Sari, Z., Miko, E., Kovacs, T., Janko, L., Csonka, T., Lente, G., et al. (2020). Indolepropionic Acid, a Metabolite of the Microbiome, Has Cytostatic Properties in Breast Cancer by Activating AHR and PXR Receptors and Inducing Oxidative Stress. Cancers (Basel) 12 (9), 2411. doi:10.3390/cancers12092411
Schanz, O., Chijiiwa, R., Cengiz, S. C., Majlesain, Y., Weighardt, H., Takeyama, H., et al. (2020). Dietary AhR Ligands Regulate AhRR Expression in Intestinal Immune Cells and Intestinal Microbiota Composition. Int. J. Mol. Sci. 21 (9), 3189. doi:10.3390/ijms21093189
Schroeder, B. O., and Backhed, F. (2016). Signals from the Gut Microbiota to Distant Organs in Physiology and Disease. Nat. Med. 22 (10), 1079–1089. doi:10.1038/nm.4185
Scott, S. A., Fu, J., and Chang, P. V. (2020). Microbial Tryptophan Metabolites Regulate Gut Barrier Function via the Aryl Hydrocarbon Receptor. Proc. Natl. Acad. Sci. U S A. 117 (32), 19376–19387. doi:10.1073/pnas.2000047117
Shimada, Y., Kinoshita, M., Harada, K., Mizutani, M., Masahata, K., Kayama, H., et al. (2013). Commensal Bacteria-dependent Indole Production Enhances Epithelial Barrier Function in the colon. PLoS One 8 (11), e80604. doi:10.1371/journal.pone.0080604
Smith, H. G., Smith, W. R., and Jepson, J. B. (1968). Interconversions of Indolic Acids by Bacteria and Rat Tissue-Ppossible Relevance to Hartnup Disorder. Clin. Sci. 34 (2), 333–342.
Sobhonslidsuk, A., Chanprasertyothin, S., Pongrujikorn, T., Kaewduang, P., Promson, K., Petraksa, S., et al. (2018). The Association of Gut Microbiota with Nonalcoholic Steatohepatitis in Thais. Biomed. Res. Int. 2018, 9340316. doi:10.1155/2018/9340316
Takaki, M., Mawe, G. M., Barasch, J. M., Gershon, M. D., and Gershon, M. D. (1985). Physiological Responses of guinea-pig Myenteric Neurons Secondary to the Release of Endogenous Serotonin by Tryptamine. Neuroscience 16 (1), 223–240. doi:10.1016/0306-4522(85)90059-4
Tossou, M. C., Liu, H., Bai, M., Chen, S., Cai, Y., Duraipandiyan, V., et al. (2016). Effect of High Dietary Tryptophan on Intestinal Morphology and Tight Junction Protein of Weaned Pig. Biomed. Res. Int. 2016, 2912418. doi:10.1155/2016/2912418
Tripathi, A., Debelius, J., Brenner, D. A., Karin, M., Loomba, R., Schnabl, B., et al. (2018). The Gut-Liver axis and the Intersection with the Microbiome. Nat. Rev. Gastroenterol. Hepatol. 15 (7), 397–411. doi:10.1038/s41575-018-0011-z
Tsavkelova, E., Oeser, B., Oren-Young, L., Israeli, M., Sasson, Y., Tudzynski, B., et al. (2012). Identification and Functional Characterization of Indole-3-Acetamide-Mediated IAA Biosynthesis in Plant-Associated Fusarium Species. Fungal Genet. Biol. 49 (1), 48–57. doi:10.1016/j.fgb.2011.10.005
Ulaszewska, M. M., Koutsos, A., Trost, K., Stanstrup, J., Garcia-Aloy, M., Scholz, M., et al. (2020). Two Apples a Day Modulate Human:microbiome Co-metabolic Processing of Polyphenols, Tyrosine and Tryptophan. Eur. J. Nutr. 59 (8), 3691–3714. doi:10.1007/s00394-020-02201-8
Van Benschoten, A. H. (1979). Indole-3-lactic Acid as a Tryptophan Metabolite Produced by Bifidobacterium Spp. Appl. Environ. Microbiol. 38, 544–546.
Venkatesh, M., Mukherjee, S., Wang, H., Li, H., Sun, K., Benechet, A. P., et al. (2014). Symbiotic Bacterial Metabolites Regulate Gastrointestinal Barrier Function via the Xenobiotic Sensor PXR and Toll-like Receptor 4. Immunity 41 (2), 296–310. doi:10.1016/j.immuni.2014.06.014
Virtue, A. T., McCright, S. J., Wright, J. M., Jimenez, M. T., Mowel, W. K., Kotzin, J. J., et al. (2019). The Gut Microbiota Regulates white Adipose Tissue Inflammation and Obesity via a Family of microRNAs. Sci. Transl Med. 11 (496), eaav1892. doi:10.1126/scitranslmed.aav1892
Vogel, C. F. A., Van Winkle, L. S., Esser, C., and Haarmann-Stemmann, T. (2020). The Aryl Hydrocarbon Receptor as a Target of Environmental Stressors - Implications for Pollution Mediated Stress and Inflammatory Responses. Redox Biol. 34, 101530. doi:10.1016/j.redox.2020.101530
Vyhlidalova, B., Bartonkova, I., Jiskrova, E., Li, H., Mani, S., and Dvorak, Z. (2020a). Differential Activation of Human Pregnane X Receptor PXR by Isomeric Mono-Methylated Indoles in Intestinal and Hepatic In Vitro Models. Toxicol. Lett. 324, 104–110. doi:10.1016/j.toxlet.2020.02.010
Vyhlidalova, B., Krasulova, K., Pecinkova, P., Marcalikova, A., Vrzal, R., Zemankova, L., et al. (2020b). Gut Microbial Catabolites of Tryptophan Are Ligands and Agonists of the Aryl Hydrocarbon Receptor: A Detailed Characterization. Int. J. Mol. Sci. 21 (7), 2614. doi:10.3390/ijms21072614
Wang, Y., Li, J., Chen, C., Lu, J., Yu, J., Xu, X., et al. (2020). Targeting the Gut Microbial Metabolic Pathway with Small Molecules Decreases Uremic Toxin Production. Gut Microbes 12 (1), 1–19. doi:10.1080/19490976.2020.1823800
Watanabe, T., and Snell, E. E. (1972). Reversibility of the Tryptophanase Reaction: Synthesis of Tryptophan from Indole, Pyruvate, and Ammonia. Proc. Natl. Acad. Sci. U S A. 69 (5), 1086–1090. doi:10.1073/pnas.69.5.1086
Wesoly, R., and Weiler, U. (2012). Nutritional Influences on Skatole Formation and Skatole Metabolism in the Pig. Animals (Basel) 2 (2), 221–242. doi:10.3390/ani2020221
Wilck, N., Matus, M. G., Kearney, S. M., Olesen, S. W., Forslund, K., Bartolomaeus, H., et al. (2017). Salt-responsive Gut Commensal Modulates TH17 axis and Disease. Nature 551 (7682), 585–589. doi:10.1038/nature24628
Williams, B. B., Van Benschoten, A. H., Cimermancic, P., Donia, M. S., Zimmermann, M., Taketani, M., et al. (2014). Discovery and Characterization of Gut Microbiota Decarboxylases that Can Produce the Neurotransmitter Tryptamine. Cell Host Microbe 16 (4), 495–503. doi:10.1016/j.chom.2014.09.001
Wlodarska, M., Luo, C., Kolde, R., d'Hennezel, E., Annand, J. W., Heim, C. E., et al. (2017). Indoleacrylic Acid Produced by Commensal Peptostreptococcus Species Suppresses Inflammation. Cell Host Microbe 22 (1), 25–37.e26. doi:10.1016/j.chom.2017.06.007
Wyeth, F. J. (1919). The Effects of Acids, Alkalies, and Sugars on the Growth and Indole Formation of Bacillus Coli: A Report to the Medical Research Committee. Biochem. J. 13 (1), 10–24. doi:10.1042/bj0130010
Xiao, H. W., Cui, M., Li, Y., Dong, J. L., Zhang, S. Q., Zhu, C. C., et al. (2020). Gut Microbiota-Derived Indole 3-propionic Acid Protects against Radiation Toxicity via Retaining Acyl-CoA-Binding Protein. Microbiome 8 (1), 69. doi:10.1186/s40168-020-00845-6
Xue, P., Fu, J., and Zhou, Y. (2018). The Aryl Hydrocarbon Receptor and Tumor Immunity. Front. Immunol. 9, 286. doi:10.3389/fimmu.2018.00286
Yang, J., Chawla, R., Rhee, K. Y., Gupta, R., Manson, M. D., Jayaraman, A., et al. (2020a). Biphasic Chemotaxis of Escherichia coli to the Microbiota Metabolite Indole. Proc. Natl. Acad. Sci. U S A. 117 (11), 6114–6120. doi:10.1073/pnas.1916974117
Yang, W., Yu, T., Huang, X., Bilotta, A. J., Xu, L., Lu, Y., et al. (2020b). Intestinal Microbiota-Derived Short-Chain Fatty Acids Regulation of Immune Cell IL-22 Production and Gut Immunity. Nat. Commun. 11 (1), 4457. doi:10.1038/s41467-020-18262-6
Yanofsky, M. T., Horn, V., and Gollnick, P. (1991). Physiological Studies of Tryptophan Transport and Tryptophanase Operon Induction in Escherichia Coli. J Bacteriol 173 (19), 6009–6017. doi:10.1128/jb.173.19.6009-6017.1991
Yokoyama, M. T., and Carlson, J. R. (1979). Microbial Metabolites of Tryptophan in the Intestinal Tract with Special Reference to Skatole. Am. J. Clin. Nutr. 32 (1), 173–178. doi:10.1093/ajcn/32.1.173
Zelante, T., Iannitti, R. G., Cunha, C., De Luca, A., Giovannini, G., Pieraccini, G., et al. (2013). Tryptophan Catabolites from Microbiota Engage Aryl Hydrocarbon Receptor and Balance Mucosal Reactivity via Interleukin-22. Immunity 39 (2), 372–385. doi:10.1016/j.immuni.2013.08.003
Zelante, T., Puccetti, M., Giovagnoli, S., and Romani, L. (2021). Regulation of Host Physiology and Immunity by Microbial Indole-3-Aldehyde. Curr. Opin. Immunol. 70, 27–32. doi:10.1016/j.coi.2020.12.004
Zhang, P., Jin, T., Kumar Sahu, S., Xu, J., Shi, Q., Liu, H., et al. (2019). The Distribution of Tryptophan-dependent Indole-3-Acetic Acid Synthesis Pathways in Bacteria Unraveled by Large-Scale Genomic Analysis. Molecules 24 (7), 1411. doi:10.3390/molecules24071411
Zhang, X., Gan, M., Li, J., Li, H., Su, M., Tan, D., et al. (2020). Endogenous Indole Pyruvate Pathway for Tryptophan Metabolism Mediated by IL4I1. J. Agric. Food Chem. 68 (39), 10678–10684. doi:10.1021/acs.jafc.0c03735
Zhang, X., Coker, O. O., Chu, E. S., Fu, K., Lau, H. C. H., Wang, Y. X., et al. (2021). Dietary Cholesterol Drives Fatty Liver-Associated Liver Cancer by Modulating Gut Microbiota and Metabolites. Gut 70 (4), 761–774. doi:10.1136/gutjnl-2019-319664
Zhao, L., Zhang, F., Ding, X., Wu, G., Lam, Y. Y., Wang, X., et al. (2018). Gut Bacteria Selectively Promoted by Dietary Fibers Alleviate Type 2 Diabetes. Science 359 (6380), 1151–1156. doi:10.1126/science.aao5774
Zhao, Z. H., Xin, F. Z., Xue, Y., Hu, Z., Han, Y., Ma, F., et al. (2019). Indole-3-propionic Acid Inhibits Gut Dysbiosis and Endotoxin Leakage to Attenuate Steatohepatitis in Rats. Exp. Mol. Med. 51 (9), 1–14. doi:10.1038/s12276-019-0304-5
Keywords: indole, indole derivates, intestinal inflammation, liver diseases, tryptophan metabolites
Citation: Li X, Zhang B, Hu Y and Zhao Y (2021) New Insights Into Gut-Bacteria-Derived Indole and Its Derivatives in Intestinal and Liver Diseases. Front. Pharmacol. 12:769501. doi: 10.3389/fphar.2021.769501
Received: 02 September 2021; Accepted: 17 November 2021;
Published: 13 December 2021.
Edited by:
Ralf Weiskirchen, RWTH Aachen University, GermanyReviewed by:
Shailesh K. Shahi, The University of Iowa, United StatesPragyan Acharya, All India Institute of Medical Sciences, New Delhi, India
Copyright © 2021 Li, Zhang, Hu and Zhao. This is an open-access article distributed under the terms of the Creative Commons Attribution License (CC BY). The use, distribution or reproduction in other forums is permitted, provided the original author(s) and the copyright owner(s) are credited and that the original publication in this journal is cited, in accordance with accepted academic practice. No use, distribution or reproduction is permitted which does not comply with these terms.
*Correspondence: Yu Zhao, cathy150@139.com
†These authors have contributed equally to this work