- Molecular Medicine Branch, National Institute of Diabetes and Digestive and Kidney Diseases, National Institutes of Health, Bethesda, MD, United States
Erythropoietin (EPO) receptor (EPOR) determines EPO response. High level EPOR on erythroid progenitor cells gives rise to EPO regulated production of red blood cells. Animal models provide evidence for EPO activity in non-hematopoietic tissue mediated by EPOR expression. Beyond erythropoiesis, EPO activity includes neuroprotection in brain ischemia and trauma, endothelial nitric oxide production and cardioprotection, skeletal muscle wound healing, and context dependent bone remodeling affecting bone repair or bone loss. This review highlights examples of EPO protective activity in select non-hematopoietic tissue with emphasis on metabolic response mediated by EPOR expression in fat and brain and sex-specific regulation of fat mass and inflammation associated with diet induced obesity. Endogenous EPO maintains glucose and insulin tolerance and protects against fat mass accumulation and inflammation. Accompanying the increase in erythropoiesis with EPO treatment is improved glucose tolerance and insulin response. During high fat diet feeding, EPO also decreases fat mass accumulation in male mice. The increased white adipose tissue inflammation and macrophage infiltration associated with diet induced obesity are also reduced with EPO treatment with a shift toward an anti-inflammatory state and decreased inflammatory cytokine production. In female mice the protective effect of estrogen against obesity supersedes EPO regulation of fat mass and inflammation, and requires estrogen receptor alpha activity. In brain, EPOR expression in the hypothalamus localizes to proopiomelanocortin neurons in the arcuate nucleus that promotes a lean phenotype. EPO stimulation of proopiomelanocortin neurons increases STAT3 signaling and production of proopiomelanocortin. Cerebral EPO contributes to metabolic response, and elevated brain EPO reduces fat mass and hypothalamus inflammation during diet induced obesity in male mice without affecting EPO stimulated erythropoiesis. Ovariectomy abrogates the sex-specific metabolic response of brain EPO. The sex-dimorphic EPO metabolic response associated with fat mass accumulation and inflammation during diet induced obesity provide evidence for crosstalk between estrogen and EPO in their anti-obesity potential in female mice mediated in part via tissue specific response in brain and white adipose tissue. Endogenous and exogenous EPO response in non-hematopoietic tissue demonstrated in animal models suggests additional activity by which EPO treatment may affect human health beyond increased erythropoiesis.
Introduction
Erythropoietin (EPO) is a glycoprotein produced in the adult kidney in a hypoxia responsive manner and functions primarily to regulate erythropoiesis in the bone marrow (Semenza, 2009; Bunn, 2013; Pugh and Ratcliffe, 2017). EPO acts by binding to its cell surface EPO receptor (EPOR) that is expressed at the highest levels on erythroid progenitor cells resulting from transactivation by EPO induced erythroid transcription factors, GATA1 and TAL1 (Broudy et al., 1991; Zon et al., 1991; Kassouf et al., 2010; Rogers et al., 2012). EPO stimulation of erythroid progenitor cells activates JAK2/STAT and other signaling pathways to promote cell survival, proliferation and differentiation, resulting in the production of 200 billion new red blood cells daily in the human body (Witthuhn et al., 1993; Kuhrt and Wojchowski, 2015; Bhoopalan et al., 2020). Following the cloning of the human EPO gene (Lin et al., 1985; Jacobs et al., 1985), recombinant human EPO was approved for clinical use by the United States Food and Drug Administration in 1989 primarily for treatment of anemia associated with chronic renal disease. For three decades, EPO treatment in hemodialysis patients has reduced exposure to blood transfusions and associated with improved physical performance (Paoletti and Cannella, 2006; Jelkmann, 2013; Wright et al., 2015).
Targeted deletion of EPO (EPO−/−) or EPOR (EPOR−/−) in mice results in embryonic death due to severe anemia (Wu et al., 1995; Lin et al., 1996). Although EPOR expression in erythroid tissue is required for life (Suzuki et al., 2002), EPOR expression is not restricted to erythroid tissue. EPO response in animal models provides evidence for EPOR mediated EPO activity in non-hematopoietic tissues that include EPO response to ischemia and injury in brain, heart and skeletal muscle, and EPO stimulated bone remodeling (Suresh et al., 2020a). This review focuses on the metabolic activity of EPO such as regulation of fat mass and inflammation during diet induced obesity and sex-dimorphic EPO response in fat and brain (Table 1).
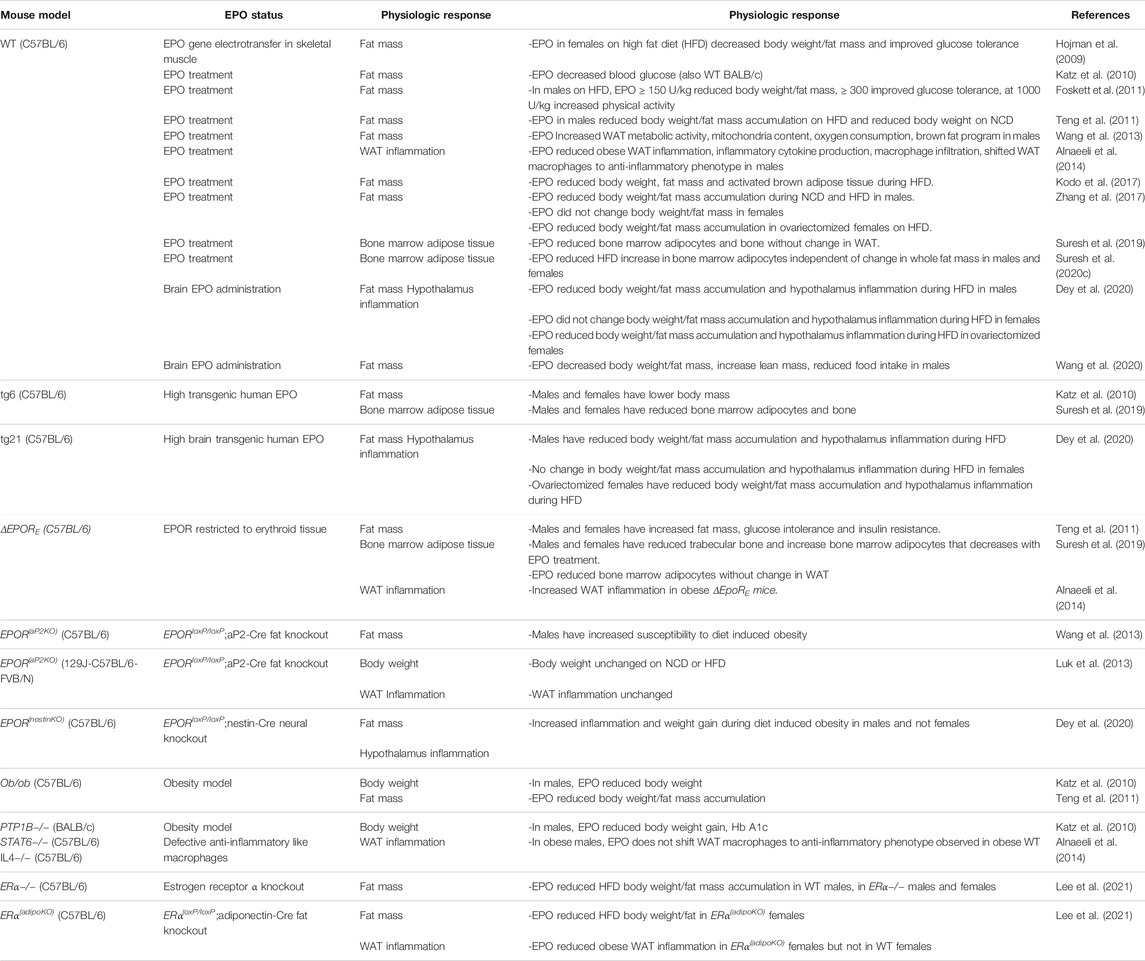
TABLE 1. Erythropoietin regulation of fat mass and inflammation in mice during diet induced obesity.
Erythropoietin Activity in Erythroid Development and Non-hematopoietic Tissue
EPO regulates survival, proliferation, and differentiation of erythroid progenitor/precursor cells. Erythropoiesis in mammals begins with primitive erythropoiesis in blood islands in the extraembryonic yolk sac at E7.5 in mice and erythroid cells transiently circulate as large, nucleated cells that ultimately enucleate (Palis et al., 1999). Primitive erythroid progenitors exhibit an aerobic glycolytic gene expression profile characteristic of cancer and rapidly proliferating cells and form larger sized colonies in culture at low oxygen (Isern et al., 2011). EPO signaling is not required for formation of primitive erythropoiesis but is required for appropriate rate of terminal maturation of primitive erythroid precursors (Malik et al., 2013). EPO support for maturation of primitive erythroid precursors may be produced in the yolk sac and/or neuroepithelial cells (Yasuda et al., 2002; Suzuki et al., 2013). EPO produced in the fetal liver and adult kidney promotes definitive erythropoiesis in the fetal liver and after birth in the bone marrow giving rise to circulating, enucleated mature red blood cells (Koury et al., 1988). During definitive erythropoiesis, EPO signaling is required for erythroid progenitor/precursor cell survival, proliferation and differentiation beyond the colony forming unit-erythroid (CFU-E) stage. EPO−/− and EPOR−/− mice die in utero at E13.5 due to lack of definitive erythropoiesis and the resultant severe anemia (Wu et al., 1995; Lin et al., 1996).
While EPO activity was initially thought to be limited to regulation of erythropoiesis, animal studies indicate activity of endogenous EPO and exogenous EPO in non-erythroid tissues such as neurons and brain (Masuda et al., 1993; Sakanaka et al., 1998; Yu et al., 2002; Tsai et al., 2006), vascular endothelium and heart (Anagnostou et al., 1990; Kertesz et al., 2004; Cai and Semenza, 2004), myoblasts and skeletal muscle (Ogilvie et al., 2000; Jia et al., 2012), fat (Teng et al., 2011; Alnaeeli et al., 2014; Zhang et al., 2017), and bone (Figure 1) (Holstein et al., 2007; Hiram-Bab et al., 2015; Suresh et al., 2019). During development, EPOR is expressed in non-hematopoietic tissue such as heart and brain and hypoplasia in embryonic heart and brain of EPOR−/− mice add further support for direct non-hematopoietic tissue response to EPO (Kertesz et al., 2004; Yu et al., 2001; Yu et al., 2002; Suresh et al., 2020a).
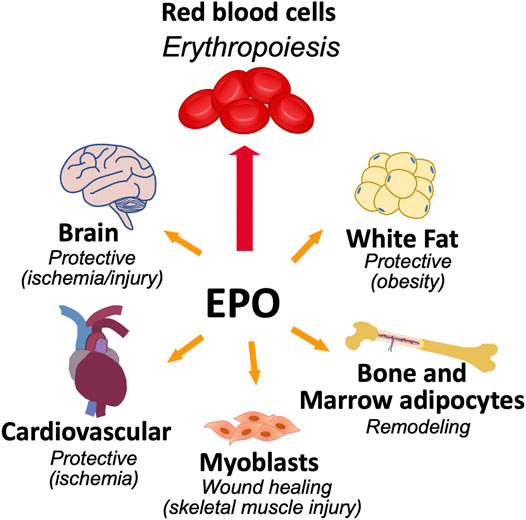
FIGURE 1. Erythropoietin activity in erythroid and select non-hematopoietic tissues. The primary function of EPO is to regulate the production of red blood cells from erythroid progenitor cells that express high level of EPOR. EPOR expression in non-hematopoietic tissue provides for EPO response that includes brain for neuroprotection and metabolic response, cardiovascular system for cardioprotection and endothelial regulation of vascular tone and oxygen delivery, skeletal muscle maintenance and wound healing, bone remodeling, and metabolic response in white adipose tissue including sex-dimorphic regulation of fat mass and inflammation associated with diet induced obesity.
Erythropoietin Regulation of Fat Mass by Erythropoietin Receptor Expression in Nonerythroid Tissue
Mice With Erythropoietin Receptor Restricted to Erythroid Cells
Endogenous EPO is involved in the regulation of fat mass (Teng et al., 2011), and EPOR is highly expressed in white adipose tissue (WAT) including adipocytes and stromal vascular fraction (SVF) in WT mice (Alnaeeli and Noguchi, 2015). A transgene with EPOR expression restricted to erythroid tissue is able to rescue EPOR−/− mice from severe embryonic anemia and gives rise to viable ΔEPORE mice with EPOR restricted to erythroid tissue (Suzuki et al., 2002). ΔEPORE mice develop obesity and glucose intolerance but exhibit normal erythropoiesis (Figure 2; Table 1) (Teng et al., 2011). In WT mice, EPO treatment reduces fat mass and body weight in males fed normal chow and decreased fat mass gain and body weight accumulation in males on high fat diet (Figure 2) (Teng et al., 2011; Zhang et al., 2017). This provides evidence that exogeneous EPO reduces fat mass accumulation in males. EPO regulation of fat mass could be related to inhibition of preadipocyte differentiation by EPO-stimulated PPARγ phosphorylation (Teng et al., 2011). Brown adipose tissue activation has also been suggested to mediate EPO stimulated increase in oxygen consumption, improved glucose tolerance and reduction in body weight and fat mass in young, male mice on high fat diet treated (Kodo et al., 2017). EPO was also associated with improved diabetes-associated cognitive dysfunction in male rodents (Wang et al., 2017; Othman et al., 2018). EPO administration in male WT mice increased energy expenditure and total activity and reduced food intake in WT while loss of endogenous EPO activity in non-hematopoietic tissue in ΔEPORE mice led to decreased energy expenditure with decreased total activity compared with WT mice (Teng et al., 2011).

FIGURE 2. Erythropoietin regulation of fat mass in mice is sex-specific. Male and female ΔEPORE mice with EPOR restricted to erythroid tissue exhibit increased gain in body weight and fat mass on normal chow diet and become obese. Male, but not female, wild type (C57BL/6) mice fed high fat diet become obese while male mice on high fat diet and treated with EPO show increase hematocrit without change in fat mass. Ovariectomy eliminates the protective effect of estrogen against diet induced obesity in females and ovariectomized mice fed high fat diet become obese, but not ovariectomized mice treated with EPO.
Mice With Deletion of Erythropoietin Receptor in Adipose Tissue
The EPO induced metabolic activity is related to promotion of brown fat like feature in white adipose tissue, which is mediated by PPARα coordinating with Sirt1 (Wang et al., 2013). The direct adipocyte EPO response was determined using mice with adipocyte-specific deletion of EPO receptor (EPORE(aP2KO)). EPORE(aP2KO) male mice on a C57BL/6 background exhibited obesity and glucose intolerance during high fat diet feeding (Table 1) (Wang et al., 2013). EPORE(aP2KO) mice showed decreased AKT phosphorylation in white adipose tissue while EPO treatment increased AKT phosphorylation in WT mice, suggesting EPO activity regulates AKT activation, which may contribute to glucose and energy homeostasis via insulin signaling (Jordan et al., 2011; Wang et al., 2013). In addition to EPOR expression in white adipose tissue mediating direct metabolic EPO activity, background strain also appears to affect metabolic response of EPORE(aP2KO) mice (Luk et al., 2013).
Erythropoietin Regulation of Fat Mass Accumulation is Sex Specific
Compared with males, female ΔEPORE mice become obese earlier with a greater proportionate accumulation of body fat mass suggesting sex specific metabolic effects of EPO in mice with the loss of EPOR in nonerythroid tissues (Teng et al., 2011). Increased circulating EPO by EPO administration in male mice or by over-expression in skeletal muscle via gene electrotransfer in female mice reduced body weight and fat mass during high fat diet induced obesity (Hojman et al., 2009; Foskett et al., 2011). In mouse models of diabetes and obesity, EPO treatment in male mice with protein Tyr phosphatase knock out (PTP1B−/−) and in ob/ob mice decreased blood glucose and body weight gain (Teng et al., 2011; Katz et al., 2010). While EPO treatment decreased fat mass accumulation in WT male mice and stimulated expression of mitochondrial oxidative genes in white adipose tissue, this was not observed in WT female mice (Table 1) (Zhang et al., 2017; Katz et al., 2010). Of note, the increased hematocrit levels and improved glucose tolerance by exogeneous EPO were observed in both male and female WT mice (Zhang et al., 2017). The EPO associated sex-dimorphic fat mass regulation can be linked to estrogen production in female mice, which contributes to energy metabolism, insulin sensitivity, and lipid metabolism (Xu and López, 2018). After ovariectomy surgery, mice on high fat diet treated with EPO showed decreased fat mass gain, while estradiol supplementation abrogated this EPO response (Figure 2) (Zhang et al., 2017). Estrogen dependent sex-specific EPO activity has also been demonstrated in mice for EPO induction in the uterine endometrium contributing to angiogenesis during the estrus cycle (Yasuda et al., 1998) and for hypoxia induced carotid body dependent EPO ventilatory response (Soliz et al., 2012).
Estrogen receptor alpha (ERα) knockout (ERα−/−) mice were shown to have similar phenotypes with menopausal women who have symptoms such as weight gain and reduction in metabolism (Arao et al., 2018). Female ERα−/− mice showed similar body weight compared with male WT and male ERα−/− mice but the body weights of ERα−/− mice were greater compared to female WT at 4 months on normal chow diet (Lee et al., 2021). EPO administration increased hematocrit levels and did not change fat mass in female ERα−/− and WT mice on normal chow diet, which is consistent with EPO activity in ovariectomized mice on normal chow diet (Zhang et al., 2017; Lee et al., 2021). In contrast, on high fat diet, EPO metabolic regulation of body weight, fat mass, and glucose homeostasis was observed in ERα−/− mice and in mice with ERα deleted in adipose tissue (ERα(adipoKO)) (Table 1) (Lee et al., 2021). The exogenous EPO treatment reduced fat mass in female ERα−/− but there was no change in fat mass in female WT mice during high fat diet feeding (Lee et al., 2021). The EPO fat regulation in ERα−/− mice is related to browning of white adipocyte, mediated by decreased white fat associated genes, Psat1, Wdnm1-like, Sepina3K and induced brown fat specific uncoupling protein 1 (UCP1) (Lee et al., 2021). EPO stimulated increase in hematocrit was comparable in all mouse groups indicating EPO regulation of fat mass is independent of EPO-induced red blood cell production. These results suggest cross talk between EPO and estrogen and that direct ERα response in adipose tissue to modulates EPO action in metabolic regulation beyond erythropoiesis, especially in female mice.
In humans, hypoxia results in increased EPO level and increased erythropoiesis and iron utilization with elevated blood hemoglobin and hematocrit as individuals ascend to high altitude (Smith et al., 2008; Gassmann and Muckenthaler, 2015). EPO regulation of metabolism and fat mass in mice (Teng et al., 2011; Zhang et al., 2017) may explain in part the association of reduced incidence of obesity in military recruits in the United States (∼94% male) that reside at high altitude (Voss et al., 2014). Full-heritage Pima Indians exhibit a high prevalence of obesity and type 2 diabetes (Smith et al., 1996; Pavkov et al., 2007), and assessment of endogenous plasma EPO level in a subset 79 individuals showed the expected negative association with hemoglobin (p = 0.005) (Reinhardt et al., 2016). In addition, males exhibited an inverse association of endogenous EPO level with percent weight change per year (p = 0.02) while females exhibited a positive association (p = 0.02). This provides evidence for sex specific correlation of endogenous EPO with weight change associated with weight loss in men and weight gain in women and independent of EPO regulation of erythropoiesis (Reinhardt et al., 2016).
Erythropoietin Promotes Bone Marrow Fat and Bone Remodeling in Male and Female Mice
In mice, EPO stimulated erythropoiesis is accompanied by decrease bone marrow adipocytes independent of changes in fat mass in white adipose tissue and by bone loss (Suresh et al., 2019). EPO activity in bone remodeling is context dependent and in animal models EPO can increase bone healing or promote bone loss during EPO stimulated erythropoiesis (Hiram-Bab et al., 2017; Suresh et al., 2020a). EPO increases bone healing in mouse fracture models (Holstein et al., 2007; Garcia et al., 2011; Shiozawa et al., 2010), and accelerates new bone formation in rats and rabbits (Li et al., 2015; Omlor et al., 2016; Mihmanli et al., 2009). However, trabecular bone loss accompanies EPO stimulated erythropoiesis in mice treated with EPO or expressing high level of transgenic EPO (Suresh et al., 2019; Hiram-Bab et al., 2015; Singbrant et al., 2011). Bone loss associated with EPO treatment is mediated via EPOR expression in osteoblasts and B-cells (Suresh et al., 2019; Suresh et al., 2020b; Deshet-Unger et al., 2020). Mice expressing high transgenic EPO produce increased osteoclast numbers in the femur, and osteoclasts and calvarial osteoblasts exhibit increased differentiation in culture, consistent with the significant reduction in trabecular bone observed in vivo (Hiram-Bab et al., 2015; Suresh et al., 2019).
The potential for endogenous EPO to affect bone health in human was observed in a study of bone fracture in elderly Swedish men with normal renal function that revealed high endogenous levels of EPO correlated with increased fracture risk (Kristjansdottir et al., 2019). Although incidence of hip fractures decreased among the general population in the United States after 1990, hip fracture incidence increased among hemodialysis patients coincident with EPO use and dose escalation up to 2004 (Suresh et al., 2021). Average EPO dose per week then decreased relating to reports of adverse cardiovascular events in clinical trials designed to achieve normal hemoglobin levels and the FDA “Black Box” warning against EPO use for hemoglobin targets over 12 g/dl, and to changes in Medicare/Medicaid reimbursement in 2007. Incidence of hip fracture among hemodialysis patients also decreased after 2004. Multivariable analysis of United States Renal Data System (USRDS) datasets for 1997–2013 revealed EPO treatment as a previously unrecognized independent risk factor for hip fracture in hemodialysis patients (Suresh et al., 2021). These findings illustrate the potential of EPO studies in animal models to be informative for EPO use and human health.
Erythropoietin Receptor Expression in Brain and Brain Erythropoietin Regulates Fat Mass During High Fat Diet Feeding
EPOR in brain detected by a transgenic reporter gene is highly expressed in mice at mid-gestation in the neural tube, and decreases with developmental age to 1–3% of hematopoietic tissue at birth (Liu et al., 1997). EPOR was shown to be expressed in neurons and EPO binding was demonstrated for neural cells contributing to a neuroprotective effect, and in select locations in the mouse brain including the hippocampus, cortex and midbrain areas (Masuda et al., 1993; Morishita et al., 1997; Digicaylioglu et al., 1995).
EPOR−/− mice show changes in genes associated with regulation of neural progenitor cell proliferation, maturation, and survival (Sollinger et al., 2017). Human EPO and EPOR expression was detected in the developing central nervous system and in brain as early as 5 weeks post-conception and specific distribution changed with development (Juul et al., 1998; Juul et al., 1999). EPO expressed in human, non-human primate and murine brain by astrocytes and neurons is upregulated by hypoxia providing further support for the biological potential of EPO activity on the brian side of the blood brain barrier (Masuda et al., 1994; Marti et al., 1996). Even prior to severe anemia, embryonic EPOR−/− mice show reduced neuroepithelium and neural progenitor cells, increased brain apoptosis and neural cells with increased sensitivity to hypoxia (Liu et al., 1997; Yu et al., 2002). Mice that retain EPOR expression in hematopoietic tissue but lack EPOR expression in neural cells exhibit no gross morphological defects but show reduced neural cell proliferation and viability, sensitivity to glutamate damage and post-stroke neurogenesis (Tsai et al., 2006; Chen et al., 2007). EPO treatment in adult animal models shows increase in newly generated interneurons and protection with EPO preconditioning prior to ischemia or middle cerebral artery occlusion (Sakanaka et al., 1998; Sadamoto et al., 1998; Bernaudin et al., 1999; Shingo et al., 2001). EPO protective activity in brain is suggested to be associated with neurogenesis and revascularization as described for a rodent model of neonatal hypoxia/ischemia (Iwai et al., 2007). Demonstration of EPO for neuroprotection in clinical trials for stroke (Ehrenreich et al., 2002; Ehrenreich et al., 2009; Ehrenreich et al., 2011) or for very preterm infants (Natalucci et al., 2020; Juul et al., 2020a) remains elusive. High dose EPO in very preterm infants reduced the transfusion needs in this population, but at 2 years of age lower risk of severe neurodevelopment impairment was not observed (Juul et al., 2020a; Juul et al., 2020b).
The hypothalamus is a master regulatory site of appetite and energy expenditure via the function of orexigenic agouti-related peptide (AgRP)/neuropeptideY (NPY)-producing neurons and anorexigenic proopiomelanocortin (POMC)-producing neurons. The POMC neurons are localized in the arcuate nucleus region of the hypothalamus. Ablation of POMC neurons and loss of POMC-derived neurotransmitters lead to obesity, underscoring the importance of POMC neurons in regulation of energy homeostasis (Yaswen et al., 1999; Xu et al., 2005). Leptin, a hormone predominantly produced from the white adipose tissue, plays a critical role in hunger suppression by activating the POMC neurons. EPO and leptin are both members of the class-I cytokine superfamily (Ouyang and He, 2003; Frühbeck, 2006) and act through the JAK/STAT signaling pathway (Constantinescu et al., 2001; Mancour et al., 2012). LEPR and EPOR are expressed in the POMC neurons and both leptin and EPO stimulation leads to increase in POMC expression in the hypothalamus. Both leptin and EPO function is mediated via STAT3 activation and results in decreased food intake (Teng et al., 2011; Bates et al., 2003; Dey et al., 2016). Moreover, optimum POMC neuron stimulation by leptin requires the presence of EPOR and ΔEPORE mice show lower POMC expression, contributing to the obesity, insulin resistance, and glucose intolerance seen in these mice (Teng et al., 2011).
High Fat Diet Feeding in Mice With Deletion of Erythropoietin Receptor in Neural Cells
EPO regulation of metabolism through its function in the neuronal cells has been studied in mice by EPOR gene knock out in the nestin expressing cells (EPOR(nestinKO); Table 1) (Dey et al., 2020). Differences between WT and EPOR(nestinKO) mice are visible primarily during metabolic stress conditions. EPOR(nestinKO) mice show greater high fat-diet induced weight gain and glucose intolerance, particularly in male mice. In contrast, females exhibit estrogen protection to diet induced obesity and high fat diet does not increase weight gain and glucose intolerance in female EPOR(nestinKO) (Dey et al., 2020).
Regulation of Metabolism by Elevated Brain Erythropoietin is Sex-Dimorphic
In mice, transgenic over-expression of EPO in the brain (tg21) provides protection from metabolic stress resulting from high fat-diet feeding (Table 1) (Dey et al., 2020). Transgenic PDGF B-chain promoter driving expression of human EPO in tg21 mice provides a model to study EPO over-expression in the brain (Kilic et al., 2005). Male and female tg21 mice show improved glucose tolerance on normal chow and high fat diet feeding, and male tg21 mice on high fat-diet feeding show lower weight gain and fat mass gain compared to wild type control mice with normal EPO levels (Dey et al., 2020). In comparison to male mice, female mice are protected from high fat-diet induced weight gain and metabolic stress. Ovariectomized control wild type mice lose the protective estrogen effect against high fat diet induced weight and show increased fat mass accumulation, while ovariectomized tg21 mice can still prevent this metabolic stress due to the elevated EPO levels in the brain (Dey et al., 2020). Administration of recombinant human EPO in the brain via intracerebroventricular osmotic pump or via a cannula implanted into the hypothalamus can also protect the male mice from gaining weight and fat during high fat diet feeding (Dey et al., 2020; Wang et al., 2020).
Erythropoietin Regulation of Adrenocorticotropic Hormone in the Pituitary Gland
In the pituitary gland, the level of EPOR expression is comparable to that in the hypothalamus, and EPO functions in regulating the secretion of the adrenocorticotropic hormone (ACTH) from the pituitary (Dey et al., 2015). Both endogenous EPO and EPO treatment affect serum ACTH levels (Dey et al., 2015). ACTH is derived from the POMC precursor peptide in the pituitary and EPO has been found to regulate its secretion by controlling the Ca2+ signaling pathways. EPO decreases the intracellular Ca2+ levels, thereby reducing the secretion of ACTH from the pituitary gland. Accordingly, the ΔEPORE mice show higher serum ACTH levels even in young mice at 3 weeks of age, that contributes to the development of the metabolic syndrome in these mice (Dey et al., 2015). The hypothalamus and the pituitary gland are part of the hypothalamus-pituitary-adrenal gland (HPA) axis that regulate the stress response pathways. With EPO produced by cells in the brain, such as astrocytes, and with EPO-stimulated POMC expression in the hypothalamus and EPO-inhibited ACTH secretion in the pituitary, EPO signaling contributes to the hypothalamic–pituitary axis as a major regulator of glucose metabolism and energy homeostasis.
Erythropoietin Regulation of Inflammation During Diet Induced Obesity
Sex Dimorphic Erythropoietin Regulation of Inflammation in Obese White Adipose Tissue
Obesity-induced insulin resistance increases the risk to develop type 2 diabetes and causes macrophage infiltration in white adipose tissue through chronic inflammation (Guilherme et al., 2008). With ongoing white adipose tissue inflammation, M1 pro-inflammatory macrophage infiltration increases, and the macrophage population in white adipose tissue shifts from anti-inflammatory M2-like cells to predominantly pro-inflammatory M1-like cells. M1 macrophages release the pro-inflammatory cytokines, which interfere with insulin signaling (Lauterbach and Wunderlich, 2017). In addition to white adipocytes, macrophages in the stromal vascular fraction of white adipose tissue express EPOR, especially in obese mice (Alnaeeli et al., 2014). Crown-like structures are known as indicators of proinflammatory process in adipose tissue, which are macrophages mostly derived from monocytes surrounding necrotic adipocytes. Estrogen in female mice is protective against obesity and female mice on high fat diet show a blunted increase in adiposity and inflammatory response compared with male mice (Singer et al., 2015). High fat diet feeding for 16 weeks showed increase crown-like structures only in male mice. Two weeks of EPO treatment in obese mice (10 weeks of high fat diet-feeding) did not affect body weight but dramatically improved inflammation, reduced the number of crown-like structures, and decreased macrophage infiltration in male WT mice (Table 1) (Alnaeeli et al., 2014). These anti-inflammatory effects are attributed to direct EPOR response in macrophage via STAT3 phosphorylation, which is associated with reduced proinflammatory gene expression with increased anti-inflammatory cytokine, IL-10 expression. EPO also stimulated macrophage subtype shift toward the anti-inflammatory M2-like cells, which requires IL-4/STAT6 signaling (Table 1). This suggests that the EPO anti-inflammatory activity in obese white adipose tissue is independent from EPO regulation of body weight and fat mass. Endogenous EPO in white adipose tissue contributes to an anti-inflammatory phenotype in male mice. During high fat diet induced obesity, ∆EPORE male mice exhibited higher circulating inflammatory monocyte numbers, increased macrophage inflammatory infiltrates, and enhanced crown like structures although their body weight and fat mass were similar to WT males (Alnaeeli et al., 2014).
In obese female WT mice (10 weeks of high fat diet feeding), EPO treatment for 2 weeks did not promote the anti-inflammatory response in white adipose tissue observed in male mice (Lee et al., 2021). However, EPO treatment for 2 weeks in obese female ERαadipoKO decreased pro-inflammatory associated genes, TNFα and iNOS in white adipose tissue, and 4 weeks EPO treatment in female ERα−/− mice on high fat diet reduced white adipose tissue TNFα expression (Table 1) (Lee et al., 2021). Thus, these findings suggest that estrogen activity in female mice interferes with the EPO anti-inflammatory activity in white adipose tissue associated with diet induced obesity and that loss of adipocyte specific ERα allows for the anti-inflammatory response by EPO.
Sex-specific Erythropoietin Regulation of Inflammation in Hypothalamus During High Fat Diet Feeding
Male mice on high fat diet exhibit chronic low-grade hypothalamus inflammation, activation of microglial cells and increased proinflammatory cytokine expression (Valdearcos et al., 2015). On normal chow, EPOR(nestinKO) mice show minimal difference in hypothalamus inflammation compared with wild-type mice. Endogenous EPO is protective against obesity induced hypothalamus inflammation (Dey et al., 2020). Male EPOR(nestinKO) mice on high fat diet exhibit greater inflammatory stress, microglial cell activation in the hypothalamus, and recruitment of peripheral myeloid cells compared with wild-type male mice (Table 1). High fat-diet feeding in wild type mice results in increase in metabolic stress with increase in serum ACTH, corticosterone, and C-reactive protein levels. tg21 mice expressing high transgenic EPO in brain are protected from these adverse effects of high fat-diet and show a better physiological control of ACTH, corticosterone, and C-reactive protein (Dey et al., 2020). The male tg21 mice also show lower inflammatory stress markers such as microglial activation, as detected by induction of Iba1 expression, and TNFα secretion in the hypothalamus (Table 1). In comparison to male mice, female mice are protected from high fat-diet induced weight gain and metabolic stress (Dey et al., 2020). In female mice, lack of EPOR in the neuronal cells (EPOR(nestinKO)) does not worsen the effect of high fat diet and EPO over-expression in the brain (tg21) does not provide any additional protection under such circumstances (Figure 3) (Dey et al., 2020). Estrogen plays a critical role in providing protection and ovariectomy in WT mice abrogates the protective effect against high fat diet induced weight and fat gain and hypothalamus inflammation. In contrast, this metabolic stress and increased hypothalamus inflammation is still prevented in ovariectomized female tg21 mice due to the elevated EPO levels in the brain (Figure 3) (Dey et al., 2020). Female tg21 mice show no difference in serum ACTH, corticosterone, and C-reactive protein during either normal or high fat-diet. Administration of recombinant human EPO in the brain via intracerebroventricular osmotic pump also protects the male mice from gaining weight and fat during high fat diet feeding concomitant with reduced expression of inflammatory markers in the hypothalamus.
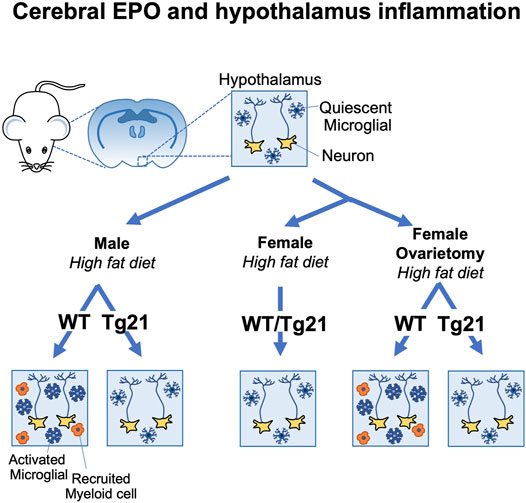
FIGURE 3. Brain erythropoietin reduction of hypothalamus inflammation during high fat diet induced obesity in mice is sex-specific. Male wild type (WT) (C57BL/6) mice fed high fat diet show increased inflammation in the arcuate region of the hypothalamus with increase microglial activation and recruitment of inflammatory myeloid cells. Male tg21 mice with elevated transgenic EPO production in brain are protected from hypothalamus inflammation associated with high fat diet feeding. Estrogen is protective in female mice against hypothalamus inflammation associated with high fat diet feeding that is only apparent in WT mice after ovariectomy and the protective effects of elevated brain EPO becomes evident in ovariectomized tg21 mice.
Brain Specific Erythropoietin and Glucose Tolerance
EPO effect in the brain can improve physiological glucose regulation under normal conditions. Under both normal diet and high fat diet, EPOR(nestinKO) mice show worse glucose tolerance, while the tg21 mice show improved glucose tolerance under similar conditions (Table 1) (Dey et al., 2020). Additionally, this effect is seen in both male and female mice, suggesting that the EPO-mediated control is independent of the protective effect of estrogen. Intracerebroventricular administration of recombinant human EPO can also reduce fasting blood glucose levels. Studies done with the tg21 mouse model suggest that regulation of glucose metabolism is probably carried out by regulation of Fgf21 and adiponectin. Fgf21, a cytokine produced primarily in liver, is involved in regulation of glucose metabolism and insulin sensitivity, and one of the downstream targets of Fgf21 is adiponectin production from white adipose tissue. Increased Fgf21 during diet induced obesity in WT mice was accompanied by lower adiponectin production from white adipose tissue, possibly due to downregulation of the Fgf21 coreceptor βKlotho (Dey et al., 2020). Adiponectin contributes to the metabolic benefits of Fgf21 in both liver and skeletal muscles (Lin et al., 2013). Fgf21 can also cross the blood-brain barrier and act as a messenger between the liver and hypothalamus, by regulating corticotropin releasing factor (CRF) expression and adrenal corticosterone levels (Liang et al., 2014). The release of CRF from the hypothalamus induces secretion of ACTH from the pituitary, that in turn results in the release of corticosterone from the adrenal gland. Although this effect is seen in both male and female mice, the hypothalamus response of Fgf21 with respect to CRF expression showed sexual dimorphism without any difference in Fgf21 receptor and βKlotho expression (Dey et al., 2020). Higher CRF expression in the hypothalamus of male WT mice could ultimately cause higher ACTH and corticosterone levels in the serum.
Conclusion
EPO response is determined by the extent of EPOR expression and animal models have been useful in demonstrating EPO stimulation of EPOR in ischemic and metabolic stress or injury beyond EPO stimulated erythropoiesis. Metabolic response to endogenous EPO and elevated EPO in the circulation or brain in mice suggest that EPO improves glucose metabolism and provides regulation of fat mass and inflammation associated with diet induced obesity, especially in males. In females, EPO effect in regulation of metabolism is comparable to that in males in the absence of estrogen effects. Relevance of metabolic EPO responses to human is indicated by subset analysis of full-heritage Pima Indians that shows a negative association of endogenous EPO level and percent weight change per year in males and a positive association in females (Reinhardt et al., 2016). The metabolic benefit of elevated EPO and EPO induction with increasing altitude may contribute to the reduced incidence of obesity in military recruits in the United States associated with residence at high altitude (Voss et al., 2014). Animal models provide insight on EPO activity in non-hematopoietic tissue such as bone loss accompanying EPO stimulated erythropoiesis that led to multivariable analysis of USRDS data sets which unexpectedly showed EPO dose as an independent risk factor for hip fracture in hemodialysis patients. EPO treatment in animal models show EPO regulation of glucose homeostasis and obesity associated fat mass and inflammation with contributions from genetic background, sex and expression of EPOR in non-hematopoietic tissue such as fat and brain, and support the potential benefit of EPO in metabolic regulation for human health.
Author Contributions
All authors listed have made substantial, direct, and intellectual contribution to the work, and approved it for publication.
Funding
This work was supported by the Intramural Research Program at the National Institute of Diabetes and Digestive and Kidney Diseases at the National Institutes of Health.
Conflict of Interest
The authors declare that the research was conducted in the absence of any commercial or financial relationships that could be construed as a potential conflict of interest.
Publisher’s Note
All claims expressed in this article are solely those of the authors and do not necessarily represent those of their affiliated organizations, or those of the publisher, the editors and the reviewers. Any product that may be evaluated in this article, or claim that may be made by its manufacturer, is not guaranteed or endorsed by the publisher.
References
Alnaeeli, M., and Noguchi, C. T. (2015). Erythropoietin and Obesity-Induced white Adipose Tissue Inflammation: Redefining the Boundaries of the Immunometabolism Territory. Adipocyte 4, 153–157. doi:10.4161/21623945.2014.978654
Alnaeeli, M., Raaka, B. M., Gavrilova, O., Teng, R., Chanturiya, T., and Noguchi, C. T. (2014). Erythropoietin Signaling: a Novel Regulator of white Adipose Tissue Inflammation during Diet-Induced Obesity. Diabetes 63, 2415–2431. doi:10.2337/db13-0883
Anagnostou, A., Lee, E. S., Kessimian, N., Levinson, R., and Steiner, M. (1990). Erythropoietin Has a Mitogenic and Positive Chemotactic Effect on Endothelial Cells. Proc. Natl. Acad. Sci. U S A. 87, 5978–5982. doi:10.1073/pnas.87.15.5978
Arao, Y., Hamilton, K. J., Lierz, S. L., and Korach, K. S. (2018). N-terminal Transactivation Function, AF-1, of Estrogen Receptor Alpha Controls Obesity through Enhancement of Energy Expenditure. Mol. Metab. 18, 68–78. doi:10.1016/j.molmet.2018.09.006
Bates, S. H., Stearns, W. H., Dundon, T. A., Schubert, M., Tso, A. W., Wang, Y., et al. (2003). STAT3 Signalling Is Required for Leptin Regulation of Energy Balance but Not Reproduction. Nature 421, 856–859. doi:10.1038/nature01388
Bernaudin, M., Marti, H. H., Roussel, S., Divoux, D., Nouvelot, A., MacKenzie, E. T., et al. (1999). A Potential Role for Erythropoietin in Focal Permanent Cerebral Ischemia in Mice. J. Cereb. Blood Flow Metab. 19, 643–651. doi:10.1097/00004647-199906000-00007
Bhoopalan, S. V., Huang, L. J., and Weiss, M. J. (2020). Erythropoietin Regulation of Red Blood Cell Production: from Bench to Bedside and Back. F1000Res 9, F1000. doi:10.12688/f1000research.26648.1
Broudy, V. C., Lin, N., Brice, M., Nakamoto, B., and Papayannopoulou, T. (1991). Erythropoietin Receptor Characteristics on Primary Human Erythroid Cells. Blood 77, 2583–2590. doi:10.1182/blood.v77.12.2583.bloodjournal77122583
Bunn, H. F. (2013). Erythropoietin. Cold Spring Harbor Perspect. Med. 3, a011619. doi:10.1101/cshperspect.a011619
Cai, Z., and Semenza, G. L. (2004). Phosphatidylinositol-3-kinase Signaling Is Required for Erythropoietin-Mediated Acute protection against Myocardial Ischemia/reperfusion Injury. Circulation 109, 2050–2053. doi:10.1161/01.CIR.0000127954.98131.23
Chen, Z. Y., Asavaritikrai, P., Prchal, J. T., and Noguchi, C. T. (2007). Endogenous Erythropoietin Signaling Is Required for normal Neural Progenitor Cell Proliferation. J. Biol. Chem. 282, 25875–25883. doi:10.1074/jbc.M701988200
Constantinescu, S. N., Huang, L. J., Nam, H., and Lodish, H. F. (2001). The Erythropoietin Receptor Cytosolic Juxtamembrane Domain Contains an Essential, Precisely Oriented, Hydrophobic Motif. Mol. Cel. 7, 377–385. doi:10.1016/s1097-2765(01)00185-x
Deshet-Unger, N., Kolomansky, A., Ben-Califa, N., Hiram-Bab, S., Gilboa, D., Liron, T., et al. (2020). Erythropoietin Receptor in B Cells Plays a Role in Bone Remodeling in Mice. Theranostics 10, 8744–8756. doi:10.7150/thno.45845
Dey, S., Cui, Z., Gavrilova, O., Zhang, X., Gassmann, M., and Noguchi, C. T. (2020). Sex-specific Brain Erythropoietin Regulation of Mouse Metabolism and Hypothalamic Inflammation. JCI Insight 5, e134061. doi:10.1172/jci.insight.134061
Dey, S., Li, X., Teng, R., Alnaeeli, M., Chen, Z., Rogers, H., et al. (2016). Erythropoietin Regulates POMC Expression via STAT3 and Potentiates Leptin Response. J. Mol. Endocrinol. 56, 55–67. doi:10.1530/JME-15-0171
Dey, S., Scullen, T., and Noguchi, C. T. (2015). Erythropoietin Negatively Regulates Pituitary ACTH Secretion. Brain Res. 1608, 14–20. doi:10.1016/j.brainres.2015.02.052
Digicaylioglu, M., Bichet, S., Marti, H. H., Wenger, R. H., Rivas, L. A., Bauer, C., et al. (1995). Localization of Specific Erythropoietin Binding Sites in Defined Areas of the Mouse Brain. Proc. Natl. Acad. Sci. U S A. 92, 3717–3720. doi:10.1073/pnas.92.9.3717
Ehrenreich, H., Hasselblatt, M., Dembowski, C., Cepek, L., Lewczuk, P., Stiefel, M., et al. (2002). Erythropoietin Therapy for Acute Stroke Is Both Safe and Beneficial. Mol. Med. 8, 495–505. doi:10.1007/bf03402029
Ehrenreich, H., Kästner, A., Weissenborn, K., Streeter, J., Sperling, S., Wang, K. K., et al. (2011). Circulating Damage Marker Profiles Support a Neuroprotective Effect of Erythropoietin in Ischemic Stroke Patients. Mol. Med. 17, 1306–1310. doi:10.2119/molmed.2011.00259
Ehrenreich, H., Weissenborn, K., Prange, H., Schneider, D., Weimar, C., Wartenberg, K., et al. (2009). Recombinant Human Erythropoietin in the Treatment of Acute Ischemic Stroke. Stroke 40, e647–56. doi:10.1161/STROKEAHA.109.564872
Foskett, A., Alnaeeli, M., Wang, L., Teng, R., and Noguchi, C. T. (2011). The Effects of Erythropoietin Dose Titration during High-Fat Diet-Induced Obesity. J. Biomed. Biotechnol. 2011, 373781. doi:10.1155/2011/373781
Frühbeck, G. (2006). Intracellular Signalling Pathways Activated by Leptin. Biochem. J. 393, 7–20. doi:10.1042/BJ20051578
Garcia, P., Speidel, V., Scheuer, C., Laschke, M. W., Holstein, J. H., Histing, T., et al. (2011). Low Dose Erythropoietin Stimulates Bone Healing in Mice. J. Orthop. Res. 29, 165–172. doi:10.1002/jor.21219
Gassmann, M., and Muckenthaler, M. U. (2015). Adaptation of Iron Requirement to Hypoxic Conditions at High Altitude. J. Appl. Physiol. (1985) 119, 1432–1440. doi:10.1152/japplphysiol.00248.2015
Guilherme, A., Virbasius, J. V., Puri, V., and Czech, M. P. (2008). Adipocyte Dysfunctions Linking Obesity to Insulin Resistance and Type 2 Diabetes. Nat. Rev. Mol. Cel Biol. 9, 367–377. doi:10.1038/nrm2391
Hiram-Bab, S., Liron, T., Deshet-Unger, N., Mittelman, M., Gassmann, M., Rauner, M., et al. (2015). Erythropoietin Directly Stimulates Osteoclast Precursors and Induces Bone Loss. FASEB J. 29, 1890–1900. doi:10.1096/fj.14-259085
Hiram-Bab, S., Neumann, D., and Gabet, Y. (2017). Erythropoietin in Bone - Controversies and Consensus. Cytokine 89, 155–159. doi:10.1016/j.cyto.2016.01.008
Hojman, P., Brolin, C., Gissel, H., Brandt, C., Zerahn, B., Pedersen, B. K., et al. (2009). Erythropoietin Over-expression Protects against Diet-Induced Obesity in Mice through Increased Fat Oxidation in Muscles. PLoS One 4, e5894. doi:10.1371/journal.pone.0005894
Holstein, J. H., Menger, M. D., Scheuer, C., Meier, C., Culemann, U., Wirbel, R. J., et al. (2007). Erythropoietin (EPO): EPO-Receptor Signaling Improves Early Endochondral Ossification and Mechanical Strength in Fracture Healing. Life Sci. 80, 893–900. doi:10.1016/j.lfs.2006.11.023
Isern, J., He, Z., Fraser, S. T., Nowotschin, S., Ferrer-Vaquer, A., Moore, R., et al. (2011). Single-lineage Transcriptome Analysis Reveals Key Regulatory Pathways in Primitive Erythroid Progenitors in the Mouse Embryo. Blood 117, 4924–4934. doi:10.1182/blood-2010-10-313676
Iwai, M., Cao, G., Yin, W., Stetler, R. A., Liu, J., and Chen, J. (2007). Erythropoietin Promotes Neuronal Replacement through Revascularization and Neurogenesis after Neonatal Hypoxia/ischemia in Rats. Stroke 38, 2795–2803. doi:10.1161/STROKEAHA.107.483008
Jacobs, K., Shoemaker, C., Rudersdorf, R., Neill, S. D., Kaufman, R. J., Mufson, A., et al. (1985). Isolation and Characterization of Genomic and cDNA Clones of Human Erythropoietin. Nature 313, 806–810. doi:10.1038/313806a0
Jelkmann, W. (2013). Physiology and Pharmacology of Erythropoietin. Transfus. Med. Hemother 40, 302–309. doi:10.1159/000356193
Jia, Y., Suzuki, N., Yamamoto, M., Gassmann, M., and Noguchi, C. T. (2012). Endogenous Erythropoietin Signaling Facilitates Skeletal Muscle Repair and Recovery Following Pharmacologically Induced Damage. FASEB J. 26, 2847. doi:10.1096/fj.11-196618
Jordan, S. D., Krüger, M., Willmes, D. M., Redemann, N., Wunderlich, F. T., Brönneke, H. S., et al. (2011). Obesity-induced Overexpression of miRNA-143 Inhibits Insulin-Stimulated AKT Activation and Impairs Glucose Metabolism. Nat. Cel Biol. 13, 434–446. doi:10.1038/ncb2211
Juul, S. E., Anderson, D. K., Li, Y., and Christensen, R. D. (1998). Erythropoietin and Erythropoietin Receptor in the Developing Human central Nervous System. Pediatr. Res. 43, 40–49. doi:10.1203/00006450-199801000-00007
Juul, S. E., Comstock, B. A., Wadhawan, R., Mayock, D. E., Courtney, S. E., Robinson, T., et al. (2020). A Randomized Trial of Erythropoietin for Neuroprotection in Preterm Infants. N. Engl. J. Med. 382, 233–243. doi:10.1056/NEJMoa1907423
Juul, S. E., Vu, P. T., Comstock, B. A., Wadhawan, R., Mayock, D. E., Courtney, S. E., et al. (2020). Effect of High-Dose Erythropoietin on Blood Transfusions in Extremely Low Gestational Age Neonates: Post Hoc Analysis of a Randomized Clinical Trial. JAMA Pediatr. 174, 933–943. doi:10.1001/jamapediatrics.2020.2271
Juul, S. E., Yachnis, A. T., Rojiani, A. M., and Christensen, R. D. (1999). Immunohistochemical Localization of Erythropoietin and its Receptor in the Developing Human Brain. Pediatr. Dev. Pathol. 2, 148–158. doi:10.1007/s100249900103
Kassouf, M. T., Hughes, J. R., Taylor, S., McGowan, S. J., Soneji, S., Green, A. L., et al. (2010). Genome-wide Identification of TAL1's Functional Targets: Insights into its Mechanisms of Action in Primary Erythroid Cells. Genome Res. 20, 1064–1083. doi:10.1101/gr.104935.110
Katz, O., Stuible, M., Golishevski, N., Lifshitz, L., Tremblay, M. L., Gassmann, M., et al. (2010). Erythropoietin Treatment Leads to Reduced Blood Glucose Levels and Body Mass: Insights from Murine Models. J. Endocrinol. 205, 87–95. doi:10.1677/JOE-09-0425
Kertesz, N., Wu, J., Chen, T. H., Sucov, H. M., and Wu, H. (2004). The Role of Erythropoietin in Regulating Angiogenesis. Dev. Biol. 276, 101–110. doi:10.1016/j.ydbio.2004.08.025
Kilic, E., Kilic, U., Soliz, J., Bassetti, C. L., Gassmann, M., and Hermann, D. M. (2005). Brain-derived Erythropoietin Protects from Focal Cerebral Ischemia by Dual Activation of ERK-1/-2 and Akt Pathways. FASEB J. 19, 2026–2028. doi:10.1096/fj.05-3941fje
Kodo, K., Sugimoto, S., Nakajima, H., Mori, J., Itoh, I., Fukuhara, S., et al. (2017). Erythropoietin (EPO) Ameliorates Obesity and Glucose Homeostasis by Promoting Thermogenesis and Endocrine Function of Classical Brown Adipose Tissue (BAT) in Diet-Induced Obese Mice. PLoS One 12, e0173661. doi:10.1371/journal.pone.0173661
Koury, M. J., Bondurant, M. C., Graber, S. E., and Sawyer, S. T. (1988). Erythropoietin Messenger RNA Levels in Developing Mice and Transfer of 125I-Erythropoietin by the Placenta. J. Clin. Invest. 82, 154–159. doi:10.1172/JCI113564
Kristjansdottir, H. L., Lewerin, C., Lerner, U. H., Herlitz, H., Johansson, P., Johansson, H., et al. (2019). High Plasma Erythropoietin Predicts Incident Fractures in Elderly Men with Normal Renal Function: The MrOS Sweden Cohort. J. Bone Miner Res. 35, 298. doi:10.1002/jbmr.3900
Kuhrt, D., and Wojchowski, D. M. (2015). Emerging EPO and EPO Receptor Regulators and Signal Transducers. Blood 125, 3536–3541. doi:10.1182/blood-2014-11-575357
Lauterbach, M. A., and Wunderlich, F. T. (2017). Macrophage Function in Obesity-Induced Inflammation and Insulin Resistance. Pflugers Arch. 469, 385–396. doi:10.1007/s00424-017-1955-5
Lee, J., Walter, M. F., Korach, K. S., and Noguchi, C. T. (2021). Erythropoietin Reduces Fat Mass in Female Mice Lacking Estrogen Receptor Alpha. Mol. Metab. 45, 101142. doi:10.1016/j.molmet.2020.101142
Li, C., Shi, C., Kim, J., Chen, Y., Ni, S., Jiang, L., et al. (2015). Erythropoietin Promotes Bone Formation through EphrinB2/EphB4 Signaling. J. Dent Res. 94, 455–463. doi:10.1177/0022034514566431
Liang, Q., Zhong, L., Zhang, J., Wang, Y., Bornstein, S. R., Triggle, C. R., et al. (2014). FGF21 Maintains Glucose Homeostasis by Mediating the Cross Talk between Liver and Brain during Prolonged Fasting. Diabetes 63, 4064–4075. doi:10.2337/db14-0541
Lin, C. S., Lim, S. K., D'Agati, V., and Costantini, F. (1996). Differential Effects of an Erythropoietin Receptor Gene Disruption on Primitive and Definitive Erythropoiesis. Genes Dev. 10, 154–164. doi:10.1101/gad.10.2.154
Lin, F. K., Suggs, S., Lin, C. H., Browne, J. K., Smalling, R., Egrie, J. C., et al. (1985). Cloning and Expression of the Human Erythropoietin Gene. Proc. Natl. Acad. Sci. U S A. 82, 7580–7584. doi:10.1073/pnas.82.22.7580
Lin, Z., Tian, H., Lam, K. S., Lin, S., Hoo, R. C., Konishi, M., et al. (2013). Adiponectin Mediates the Metabolic Effects of FGF21 on Glucose Homeostasis and Insulin Sensitivity in Mice. Cell Metab. 17, 779–789. doi:10.1016/j.cmet.2013.04.005
Liu, C., Shen, K., Liu, Z., and Noguchi, C. T. (1997). Regulated Human Erythropoietin Receptor Expression in Mouse Brain. J. Biol. Chem. 272, 32395–32400. doi:10.1074/jbc.272.51.32395
Luk, C. T., Shi, S. Y., Choi, D., Cai, E. P., Schroer, S. A., and Woo, M. (2013). In Vivo knockdown of Adipocyte Erythropoietin Receptor Does Not Alter Glucose or Energy Homeostasis. Endocrinology 154, 3652–3659. doi:10.1210/en.2013-1113
Malik, J., Kim, A. R., Tyre, K. A., Cherukuri, A. R., and Palis, J. (2013). Erythropoietin Critically Regulates the Terminal Maturation of Murine and Human Primitive Erythroblasts. Haematologica 98, 1778–1787. doi:10.3324/haematol.2013.087361
Mancour, L. V., Daghestani, H. N., Dutta, S., Westfield, G. H., Schilling, J., Oleskie, A. N., et al. (2012). Ligand-induced Architecture of the Leptin Receptor Signaling Complex. Mol. Cel. 48, 655–661. doi:10.1016/j.molcel.2012.09.003
Marti, H. H., Wenger, R. H., Rivas, L. A., Straumann, U., Digicaylioglu, M., Henn, V., et al. (1996). Erythropoietin Gene Expression in Human, Monkey and Murine Brain. Eur. J. Neurosci. 8, 666–676. doi:10.1111/j.1460-9568.1996.tb01252.x
Masuda, S., Nagao, M., Takahata, K., Konishi, Y., Gallyas, F., Tabira, T., et al. (1993). Functional Erythropoietin Receptor of the Cells with Neural Characteristics. Comparison with Receptor Properties of Erythroid Cells. J. Biol. Chem. 268, 11208–11216. doi:10.1016/s0021-9258(18)82112-3
Masuda, S., Okano, M., Yamagishi, K., Nagao, M., Ueda, M., and Sasaki, R. (1994). A Novel Site of Erythropoietin Production. Oxygen-dependent Production in Cultured Rat Astrocytes. J. Biol. Chem. 269, 19488–19493. doi:10.1016/s0021-9258(17)32195-6
Mihmanli, A., Dolanmaz, D., Avunduk, M. C., and Erdemli, E. (2009). Effects of Recombinant Human Erythropoietin on Mandibular Distraction Osteogenesis. J. Oral Maxillofac. Surg. 67, 2337–2343. doi:10.1016/j.joms.2008.06.082
Morishita, E., Masuda, S., Nagao, M., Yasuda, Y., and Sasaki, R. (1997). Erythropoietin Receptor Is Expressed in Rat Hippocampal and Cerebral Cortical Neurons, and Erythropoietin Prevents In Vitro Glutamate-Induced Neuronal Death. Neuroscience 76, 105–116. doi:10.1016/s0306-4522(96)00306-5
Natalucci, G., Latal, B., Koller, B., Rüegger, C., Sick, B., Held, L., et al. (2020). Neurodevelopmental Outcomes at Age 5 Years after Prophylactic Early High-Dose Recombinant Human Erythropoietin for Neuroprotection in Very Preterm Infants. JAMA 324, 2324–2327. doi:10.1001/jama.2020.19395
Ogilvie, M., Yu, X., Nicolas-Metral, V., Pulido, S. M., Liu, C., Ruegg, U. T., et al. (2000). Erythropoietin Stimulates Proliferation and Interferes with Differentiation of Myoblasts. J. Biol. Chem. 275, 39754–39761. doi:10.1074/jbc.M004999200
Omlor, G. W., Kleinschmidt, K., Gantz, S., Speicher, A., Guehring, T., and Richter, W. (2016). Increased Bone Formation in a Rabbit Long-Bone Defect Model after Single Local and Single Systemic Application of Erythropoietin. Acta Orthop. 87, 425–431. doi:10.1080/17453674.2016.1198200
Othman, M. A. M., Rajab, E., AlMubarak, A., AlNaisar, M., Bahzad, N., and Kamal, A. (2018). Erythropoietin Protects against Cognitive Impairment and Hippocampal Neurodegeneration in Diabetic Mice. Behav. Sci. (Basel) 9, 4. doi:10.3390/bs9010004
Ouyang, S., and He, F. (2003). Phylogeny of a Growth Hormone-like Cytokine Superfamily Based upon 3D Structure. J. Mol. Evol. 56, 131–136. doi:10.1007/s00239-002-2385-2
Palis, J., Robertson, S., Kennedy, M., Wall, C., and Keller, G. (1999). Development of Erythroid and Myeloid Progenitors in the Yolk Sac and Embryo Proper of the Mouse. Development 126, 5073–5084. doi:10.1242/dev.126.22.5073
Paoletti, E., and Cannella, G. (2006). Update on Erythropoietin Treatment: Should Hemoglobin Be Normalized in Patients with Chronic Kidney Disease? J. Am. Soc. Nephrol. 17, S74–S77. doi:10.1681/ASN.2005121325
Pavkov, M. E., Hanson, R. L., Knowler, W. C., Bennett, P. H., Krakoff, J., and Nelson, R. G. (2007). Changing Patterns of Type 2 Diabetes Incidence Among Pima Indians. Diabetes Care 30, 1758–1763. doi:10.2337/dc06-2010
Pugh, C. W., and Ratcliffe, P. J. (2017). New Horizons in Hypoxia Signaling Pathways. Exp. Cel Res. 356, 116–121. doi:10.1016/j.yexcr.2017.03.008
Reinhardt, M., Dey, S., Tom Noguchi, C., Zhang, Y., Krakoff, J., and Thearle, M. S. (2016). Non-hematopoietic Effects of Endogenous Erythropoietin on Lean Mass and Body Weight Regulation. Obesity (Silver Spring) 24, 1530–1536. doi:10.1002/oby.21537
Rogers, H., Wang, L., Yu, X., Alnaeeli, M., Cui, K., Zhao, K., et al. (2012). T-cell Acute Leukemia 1 (TAL1) Regulation of Erythropoietin Receptor and Association with Excessive Erythrocytosis. J. Biol. Chem. 287, 36720–36731. doi:10.1074/jbc.M112.378398
Sadamoto, Y., Igase, K., Sakanaka, M., Sato, K., Otsuka, H., Sakaki, S., et al. (1998). Erythropoietin Prevents Place Navigation Disability and Cortical Infarction in Rats with Permanent Occlusion of the Middle Cerebral Artery. Biochem. Biophys. Res. Commun. 253, 26–32. doi:10.1006/bbrc.1998.9748
Sakanaka, M., Wen, T. C., Matsuda, S., Masuda, S., Morishita, E., Nagao, M., et al. (1998). In Vivo evidence that Erythropoietin Protects Neurons from Ischemic Damage. Proc. Natl. Acad. Sci. U S A. 95, 4635–4640. doi:10.1073/pnas.95.8.4635
Semenza, G. L. (2009). Involvement of Oxygen-Sensing Pathways in Physiologic and Pathologic Erythropoiesis. Blood 114, 2015–2019. doi:10.1182/blood-2009-05-189985
Shingo, T., Sorokan, S. T., Shimazaki, T., and Weiss, S. (2001). Erythropoietin Regulates the In Vitro and In Vivo Production of Neuronal Progenitors by Mammalian Forebrain Neural Stem Cells. J. Neurosci. 21, 9733–9743. doi:10.1523/jneurosci.21-24-09733.2001
Shiozawa, Y., Jung, Y., Ziegler, A. M., Pedersen, E. A., Wang, J., Wang, Z., et al. (2010). Erythropoietin Couples Hematopoiesis with Bone Formation. PLoS One 5, e10853. doi:10.1371/journal.pone.0010853
Singbrant, S., Russell, M. R., Jovic, T., Liddicoat, B., Izon, D. J., Purton, L. E., et al. (2011). Erythropoietin Couples Erythropoiesis, B-Lymphopoiesis, and Bone Homeostasis within the Bone Marrow Microenvironment. Blood 117, 5631–5642. doi:10.1182/blood-2010-11-320564
Singer, K., Maley, N., Mergian, T., DelProposto, J., Cho, K. W., Zamarron, B. F., et al. (2015). Differences in Hematopoietic Stem Cells Contribute to Sexually Dimorphic Inflammatory Responses to High Fat Diet-Induced Obesity. J. Biol. Chem. 290, 13250–13262. doi:10.1074/jbc.M114.634568
Smith, C. J., Nelson, R. G., Hardy, S. A., Manahan, E. M., Bennett, P. H., and Knowler, W. C. (1996). Survey of the Diet of Pima Indians Using Quantitative Food Frequency Assessment and 24-hour Recall. Diabetic Renal Disease Study. J. Am. Diet. Assoc. 96, 778–784. doi:10.1016/s0002-8223(96)00216-7
Smith, T. G., Robbins, P. A., and Ratcliffe, P. J. (2008). The Human Side of Hypoxia-Inducible Factor. Br. J. Haematol. 141, 325–334. doi:10.1111/j.1365-2141.2008.07029.x
Soliz, J., Khemiri, H., Caravagna, C., and Seaborn, T. (2012). Erythropoietin and the Sex-Dimorphic Chemoreflex Pathway. Adv. Exp. Med. Biol. 758, 55–62. doi:10.1007/978-94-007-4584-1_8
Sollinger, C., Lillis, J., Malik, J., Getman, M., Proschel, C., and Steiner, L. (2017). Erythropoietin Signaling Regulates Key Epigenetic and Transcription Networks in Fetal Neural Progenitor Cells. Sci. Rep. 7, 14381. doi:10.1038/s41598-017-14366-0
Suresh, S., Alvarez, J. C., Dey, S., and Noguchi, C. T. (2020). Erythropoietin-Induced Changes in Bone and Bone Marrow in Mouse Models of Diet-Induced Obesity. Int. J. Mol. Sci. 21, 1657. doi:10.3390/ijms21051657
Suresh, S., de Castro, L. F., Dey, S., Robey, P. G., and Noguchi, C. T. (2019). Erythropoietin Modulates Bone Marrow Stromal Cell Differentiation. Bone Res. 7, 21. doi:10.1038/s41413-019-0060-0
Suresh, S., Lee, J., and Noguchi, C. T. (2020). Erythropoietin Signaling in Osteoblasts Is Required for normal Bone Formation and for Bone Loss during Erythropoietin-Stimulated Erythropoiesis. FASEB J. 34, 11685–11697. doi:10.1096/fj.202000888R
Suresh, S., Rajvanshi, P. K., and Noguchi, C. T. (2020). The Many Facets of Erythropoietin Physiologic and Metabolic Response. Front. Physiol. 10, 1534. doi:10.3389/fphys.2019.01534
Suresh, S., Wright, E. C., Wright, D. G., Abbott, K. C., and Noguchi, C. T. (2021). Erythropoietin Treatment and the Risk of Hip Fractures in Hemodialysis Patients. J. Bone Miner Res. 36, 1211. doi:10.1002/jbmr.4297
Suzuki, N., Hirano, I., Pan, X., Minegishi, N., and Yamamoto, M. (2013). Erythropoietin Production in Neuroepithelial and Neural Crest Cells during Primitive Erythropoiesis. Nat. Commun. 4, 2902. doi:10.1038/ncomms3902
Suzuki, N., Ohneda, O., Takahashi, S., Higuchi, M., Mukai, H. Y., Nakahata, T., et al. (2002). Erythroid-specific Expression of the Erythropoietin Receptor Rescued its Null Mutant Mice from Lethality. Blood 100, 2279–2288. doi:10.1182/blood-2002-01-0124
Teng, R., Gavrilova, O., Suzuki, N., Chanturiya, T., Schimel, D., Hugendubler, L., et al. (2011). Disrupted Erythropoietin Signalling Promotes Obesity and Alters Hypothalamus Proopiomelanocortin Production. Nat. Commun. 2, 520. doi:10.1038/ncomms1526
Tsai, P. T., Ohab, J. J., Kertesz, N., Groszer, M., Matter, C., Gao, J., et al. (2006). A Critical Role of Erythropoietin Receptor in Neurogenesis and post-stroke Recovery. J. Neurosci. 26, 1269–1274. doi:10.1523/JNEUROSCI.4480-05.2006
Valdearcos, M., Xu, A. W., and Koliwad, S. K. (2015). Hypothalamic Inflammation in the Control of Metabolic Function. Annu. Rev. Physiol. 77, 131–160. doi:10.1146/annurev-physiol-021014-071656
Voss, J. D., Allison, D. B., Webber, B. J., Otto, J. L., and Clark, L. L. (2014). Lower Obesity Rate during Residence at High Altitude Among a Military Population with Frequent Migration: a Quasi Experimental Model for Investigating Spatial Causation. PLoS One 9, e93493. doi:10.1371/journal.pone.0093493
Wang, L., Teng, R., Di, L., Rogers, H., Wu, H., Kopp, J. B., et al. (2013). PPARα and Sirt1 Mediate Erythropoietin Action in Increasing Metabolic Activity and browning of white Adipocytes to Protect against Obesity and Metabolic Disorders. Diabetes 62, 4122–4131. doi:10.2337/db13-0518
Wang, M., Yan, W., Liu, Y., Hu, H., Sun, Q., Chen, X., et al. (2017). Erythropoietin Ameliorates Diabetes-Associated Cognitive Dysfunction In Vitro and In Vivo. Sci. Rep. 7, 2801. doi:10.1038/s41598-017-03137-6
Wang, Z., Khor, S., and Cai, D. (2020). Regulation of Muscle and Metabolic Physiology by Hypothalamic Erythropoietin Independently of its Peripheral Action. Mol. Metab. 32, 56–68. doi:10.1016/j.molmet.2019.12.001
Witthuhn, B. A., Quelle, F. W., Silvennoinen, O., Yi, T., Tang, B., Miura, O., et al. (1993). JAK2 Associates with the Erythropoietin Receptor and Is Tyrosine Phosphorylated and Activated Following Stimulation with Erythropoietin. Cell 74, 227–236. doi:10.1016/0092-8674(93)90414-l
Wright, D. G., Wright, E. C., Narva, A. S., Noguchi, C. T., and Eggers, P. W. (2015). Association of Erythropoietin Dose and Route of Administration with Clinical Outcomes for Patients on Hemodialysis in the United States. Clin. J. Am. Soc. Nephrol. 10, 1822–1830. doi:10.2215/CJN.01590215
Wu, H., Liu, X., Jaenisch, R., and Lodish, H. F. (1995). Generation of Committed Erythroid BFU-E and CFU-E Progenitors Does Not Require Erythropoietin or the Erythropoietin Receptor. Cell 83, 59–67. doi:10.1016/0092-8674(95)90234-1
Xu, A. W., Kaelin, C. B., Morton, G. J., Ogimoto, K., Stanhope, K., Graham, J., et al. (2005). Effects of Hypothalamic Neurodegeneration on Energy Balance. PLoS Biol. 3, e415. doi:10.1371/journal.pbio.0030415
Xu, Y., and López, M. (2018). Central Regulation of Energy Metabolism by Estrogens. Mol. Metab. 15, 104–115. doi:10.1016/j.molmet.2018.05.012
Yasuda, Y., Masuda, S., Chikuma, M., Inoue, K., Nagao, M., and Sasaki, R. (1998). Estrogen-dependent Production of Erythropoietin in Uterus and its Implication in Uterine Angiogenesis. J. Biol. Chem. 273, 25381–25387. doi:10.1074/jbc.273.39.25381
Yasuda, Y., Okano, M., Nagao, M., Masuda, S., Fujita, Y., and Sasaki, R. (2002). Erythropoietin and Erythropoietin-Receptor Producing Cells Demonstrated by In Situ Hybridization in Mouse Visceral Yolk Sacs. Anat. Sci. Int. 77, 58–63. doi:10.1046/j.0022-7722.2002.00007.x
Yaswen, L., Diehl, N., Brennan, M. B., and Hochgeschwender, U. (1999). Obesity in the Mouse Model of Pro-opiomelanocortin Deficiency Responds to Peripheral Melanocortin. Nat. Med. 5, 1066–1070. doi:10.1038/12506
Yu, X., Lin, C. S., Costantini, F., and Noguchi, C. T. (2001). The Human Erythropoietin Receptor Gene Rescues Erythropoiesis and Developmental Defects in the Erythropoietin Receptor Null Mouse. Blood 98, 475–477. doi:10.1182/blood.v98.2.475
Yu, X., Shacka, J. J., Eells, J. B., Suarez-Quian, C., Przygodzki, R. M., Beleslin-Cokic, B., et al. (2002). Erythropoietin Receptor Signalling Is Required for normal Brain Development. Development 129, 505–516. doi:10.1242/dev.129.2.505
Zhang, Y., Rogers, H. M., Zhang, X., and Noguchi, C. T. (2017). Sex Difference in Mouse Metabolic Response to Erythropoietin. FASEB J. 31, 2661–2673. doi:10.1096/fj.201601223RRR
Keywords: erythropoietin, erythropoietin receptor, gender-specific, obesity, inflammation, hypothalamus, microglial
Citation: Dey S, Lee J and Noguchi CT (2021) Erythropoietin Non-hematopoietic Tissue Response and Regulation of Metabolism During Diet Induced Obesity. Front. Pharmacol. 12:725734. doi: 10.3389/fphar.2021.725734
Received: 15 June 2021; Accepted: 31 August 2021;
Published: 15 September 2021.
Edited by:
Edith Marianne Schneider Gasser, University of Zurich, SwitzerlandReviewed by:
Drorit Neumann, Tel Aviv University, IsraelMarkus Thiersch, University of Zurich, Switzerland
Copyright © 2021 Dey, Lee and Noguchi. This is an open-access article distributed under the terms of the Creative Commons Attribution License (CC BY). The use, distribution or reproduction in other forums is permitted, provided the original author(s) and the copyright owner(s) are credited and that the original publication in this journal is cited, in accordance with accepted academic practice. No use, distribution or reproduction is permitted which does not comply with these terms.
*Correspondence: Constance T. Noguchi, connien@niddk.nih.gov