Lactate Is Answerable for Brain Function and Treating Brain Diseases: Energy Substrates and Signal Molecule
- 1Department of Rehabilitation Medicine, Shanghai University of Medicine and Health Sciences Affiliated Zhoupu Hospital, Shanghai, China
- 2Bio-X Institutes, Shanghai Jiao Tong University, Shanghai, China
- 3Department of Physical Education, Shanghai University of Medicine and Health Sciences, Shanghai, China
- 4Central Lab, Shanghai Pudong New Area People's Hospital, Shanghai, China
- 5College of Rehabilitation Sciences, Shanghai University of Medicine and Health Sciences, Shanghai, China
- 6Key Laboratory of Exercise and Health Sciences of Ministry of Education, Shanghai University of Sport, Shanghai, China
Research to date has provided novel insights into lactate's positive role in multiple brain functions and several brain diseases. Although notable controversies and discrepancies remain, the neurobiological role and the metabolic mechanisms of brain lactate have now been described. A theoretical framework on the relevance between lactate and brain function and brain diseases is presented. This review begins with the source and route of lactate formation in the brain and food; goes on to uncover the regulatory effect of lactate on brain function; and progresses to gathering the application and concentration variation of lactate in several brain diseases (diabetic encephalopathy, Alzheimer's disease, stroke, traumatic brain injury, and epilepsy) treatment. Finally, the dual role of lactate in the brain is discussed. This review highlights the biological effect of lactate, especially L-lactate, in brain function and disease studies and amplifies our understanding of past research.
Introduction
Brain lactate, as a well-known metabolite, primarily roots in astrocytic glycolysis from blood glucose, glycogen, and blood lactate. Recently, the role of the “good guy” has gradually superseded the traditional concept of metabolic waste in medical literature in neuroscience (1). The most interesting dimension of this is the physiological character of lactate's role in mediating brain function (2). These canonical function involves learning and memory (3), cerebral blood flow (4), neurogenesis (5, 6) and cerebral microangiogenesis (7), energy metabolism (8), neuronal activity (9–11), and neuroprotection (12–15). Therefore, lactate is competent to be a potential therapy for ameliorating the pathological process of some brain diseases associated with impaired brain function. In mammals, lactate exists as two enantiomers. The structure of asymmetrical C2 carbon leads to the two stereoisomers of lactate that are designated as L-lactate and D-lactate (16). L-lactate is the major enantiomer found in the brain and blood whereas D-lactate is normally present in very low concentrations under healthy physiological conditions (17). D-lactate is also considered as the rivalrous inhibitor of L-lactate since it competitively inhibits L-lactate transport (18). In different brain disease patterns, L- and D-lactate is reported to exert a similar or distinct effect on brain function. The involving mechanisms are far more complex than originally thought. For the most part, L-lactate can be utilized as a preferred energy substrate of neurons for meeting the energy demand (19, 20) or act as the novel hormone-like effect called lactormone (21, 22). But current research about D-lactate's role in brain function and brain-related disease is sparse and debatable. D-lactate-mediated mechanisms are also unclear.
In this narrative review, we aim to provide a comprehensive and profound summary of the role of lactate in brain function and related diseases. Consequently, we expound the food source of lactate intake, discuss the lactate enantiomers and their metabolism manner in the brain, compare the influence of L- and D-lactate on brain functions, expound on the effect of L- and D-lactate replenishment on several common brain diseases, and summarize the mechanisms of L-lactate.
Lactate Enantiomers
Lactate in mammals exists as two enantiomers: the most common form of L-isomers (known as L-lactate) is produced during mammalian glucose metabolism, and a quite small quantity of D-isomers (known as D-lactate) is generated from carbohydrates by bacterial metabolism (23, 24). The L-isomer of lactate is believed to have biological metabolic activity, while the D-isomer is too low in the body to activate the relevant enzymes for catabolism (25).
The Formation of Lactate in the Brain
L-Lactate
In the brain, the main source of L-lactate is astrocytic glycolysis from blood glucose, glycogen, and blood lactate (26, 27). In astrocytes, glucose can be converted directly to L-lactate by glycolysis or be stored in the form of glycogen (28). Glycogen is almost exclusively localized in astrocytes (20). As the neuronal activity intensifies, astrocytic glycogen is mobilized to supply neurons in case of neuronal glucose dissatisfying the energetic demand (29). In consequence, besides maintaining the astrocytes itself energy demand, L-lactate also supports neuronal activity by providing ATP (30, 31). Furthermore, the elevated blood L-lactate can also cross the blood-brain barrier into the brain via the monocarboxylate transporter 1 (MCT1) in some conditions (32, 33), such as vigorous exercise (34, 35) and fermentative (36) or fiber-containing foods (37).
D-Lactate
D-lactate, as the stereoisomer of L-lactate, is barely found in the brain and does not participate in energy production (23). A tiny amount of methylglyoxal (MG), the metabolic intermediary product, can be produced during glycolysis (38). The glyoxalase system, mostly located in astrocytes, allows bulk MG to convert into endogenic D-lactate or glutathione (GSH) (39).
The Metabolism of Lactate in the Brain
L-Lactate
The hypothesis of astrocyte-neuron lactate shuttle (ANLS) describing L-lactate shuttling between astrocytes and neurons is linked to glutamatergic signaling by Pellerin and Magistretti (40). A model opens the new insight for the L-lactate role in the brain and perfectly elaborates the mechanism of L-lactate how to serve as energy substrates. The ANLS switches on glutamate released by neuronal terminals and then taken up by astrocytes via the excitatory amino acid transporters 1 and 2 (EAAT1 and 2) to convert into glutamine or glutathione, which is activated by a gradient. This process stimulates the Na+-K+ pump to favor astrocytic mitochondrial acidification for launching glycolysis (21). Phosphofructokinase-2/fructose-2,6-bisphosphatase 3 (Pfkfb3) is a key positive modulator of glycolysis. Its expression and activity are high in astrocytes but not in neurons. These cell-specific expression and activity profiles result in the capacity of glycolysis being more active in astrocytes than neurons (26). The stored glycogen first decomposes into glucose-6-Phosphate (glucose-6P) catalyzed by glycogen phosphorylase. Then the glucose-6P gives rise to two molecules of pyruvate. Finally, pyruvate can be either converted to acetyl coenzyme A (acetyl-CoA) to be used directly by the astrocytic TCA cycle or transformed into L-lactate by lactate dehydrogenase 5 (LDH5) (26, 39).
Monocarboxylate transporters (MCTs) belong to the SLC16 gene family and facilitate the transmembrane H+-linked monocarboxylate, like lactate, pyruvate, and ketone bodies, to shuttle between astrocytes and neurons for the brain metabolic demands (26, 41). MCT1 is mainly located at the membrane of astrocytes, oligodendrocytes, and endothelial cells of blood vessels (42–44). The Km value of MCT1 for L-lactate approximates 3.5 mM. MCT4 is widely expressed at the membrane of astrocytes to cooperate with MCT1 in L-lactate efflux transport, in which the Km value for L-lactate is 34 mM. Compared with MCT1 and MCT4, MCT2 is specific in the membrane of neurons (45, 46) and has a higher affinity for L-lactate with the Km value of 0.7 mM (20, 47). The L-lactate from astrocytes or blood will be released into intercellular substances through MCT1 and/or MCT4, transported by MCT2 into neurons (30, 48), and then oxidized at the Krebs cycle (21).
The L-lactate oxidation in neuronal cytoplasm into pyruvate by LDH1 is the first step to metabolic removal (1, 38). The newly generated pyruvate later crosses the mitochondrial inner membrane in favor of presumptive pyruvate transporters MPC (MPC1 and MPC2) and/or MCT1 (16, 49), to transform into acetyl-CoA for entering the tricarboxylic acid cycle (TCA) and therefore producing 14–17 ATPs per lactate molecule for maintaining neuronal activity (26). But the remarkable thing is that the MPC is only identified in yeast, drosophila, and humans (49). The molecular masses of MPC1 (15 kD) and MPC2 (14 kD) are akin to the findings in the experiment performed in rat liver and heart (50). That is to say, deficient evidence shows that the MPC exists in the neurons so far. The localization of MCT1 in mitochondrial inner membrane is not only verified in rat heart and muscle (51–53) but also in the rat cortical, hippocampal, and thalamic neurons (54). Besides deductive pyruvate transporters, L-lactate may be directly oxidized by mitochondria (in heart, muscle, liver, spermatozoa, and brain) (22, 55) in the wake of the concept “cytosol-mitochondria lactate shuttle” proposed by Brooks' team (53, 56–58). In this model, L-lactate enters into the mitochondrial intermembrane space through porins in the outer mitochondrial membrane. Then it will be oxidized by putative mitochondrial LDH (mLDH) (located at the inner mitochondrial membrane) into pyruvate (59). Several studies have confirmed that L-lactate can be taken up and metabolized by the mLDH in both astrocytes (human astrocytoma cells) (60) and neurons (rat cerebellar granule cells, cortex neurons, and hippocampal neurons) (54, 61). The evidence implies that cytosol-mitochondria lactate shuttle may occur in the brain (Figure 1).
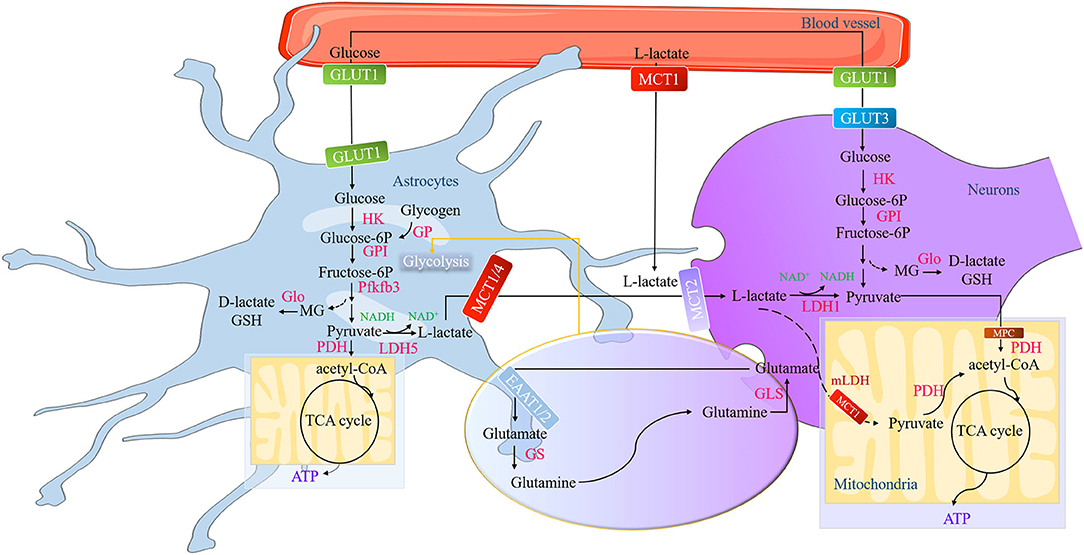
Figure 1. The formation and metabolism of lactate in the brain. The action of the glutamate-glutamine cycle switches on the glycolysis in the astrocytes, a process of glutamate released by neuronal terminals and then is taken up by astrocytes via the EAATs to convert into glutamine or GSH. Pfkfb3 (a key positive modulator of glycolysis) is a high expression in astrocytes and low in neurons, while the activity of PDH is low in astrocytes and low in neurons. These characteristics determine that astrocytes have strong glycolysis ability and neurons have strong aerobic oxidation ability. Astrocytic glycogen first decomposes into glucose-6P catalyzed by GP and finally into pyruvate through a series of catalytic reactions. During this process, a tiny amount of MG generates and then converts into endogenic D-lactate or GSH. The newly formed pyruvate can be either converted to acetyl-CoA for the astrocytic TCA cycle or transformed into L-lactate by LDH5. The L-lactate in the astrocytes and in the brain blood vessel is transported into the neurons through MCTs (MCT1, MCT2, and MCT4) and then oxidized at the Krebs cycle. EAATs, excitatory amino acid transporters; GSH, glutathione; Pfkfb3, phosphofructokinase-2/fructose-2,6-bisphosphatase 3; PDH, pyruvate dehydrogenase; Glucose-6P, glucose-6-Phosphate; GP, glycogen phosphorylase; MG, methylglyoxal; acetyl-CoA, acetyl coenzyme A; lactate dehydrogenase 5, LDH5; Monocarboxylic acid transporters, MCTs.
D-Lactate
D-lactate exerts metabolically inert in mammals (62) because of the absence of specific cytosolic lactate dehydrogenase (D-LDH) to metabolize D-lactate into pyruvate (63, 64). This enzyme barely exists in mammals (24). Some evidence shows that MCTs can transport D-lactate to inhibit L-lactate out of astrocytes or into neurons despite the Km value of MCTs for D-lactate being far higher than L-lactate (65, 66). The rate of oxidation of D-lactate in the brain is considerably slower than that of L-lactate due to the rather low expression levels of D-LDH in the brain (36, 67). Since interfering with more efficient energy substrates (pyruvate and L-lactate) for mitochondrial use, the physiological function of D-lactate is now known as the competitive inhibitor of L-lactate (18). Moreover, D-lactate is confirmed to interfere with the pyruvate metabolism in the brain, which eventually impairs in state 3 and state 4 of mitochondrial respiration (63), its accumulation may have an adverse impact on energy metabolism and thus lead to toxicity (Figure 1).
Lactate Intakes From the Food
Lactate, as the predominant end-product of lactose fermentation in the food (68), is produced by the lactic acid bacteria, namely, gram-positive and catalase-negative microorganisms. Such microorganisms are involved in the fermentation of a range of foods and beverages, such as dairy products, meat, fish, vegetable, sourdough, wine, and cider (68), which creates the specific flavors and aromas for the food and benefits human health (69). People can uptake lactate from cheese, yogurt, wine, fermented vegetables (69), and fermented oyster extract (70). Thereby, lactate will appear in the gut via the consumption of fermented foods. In addition, prebiotic, fiber-containing foods also are the way of the lactate intake, such as broccoli, brussels sprouts, cabbage, cauliflower, collard greens, kale, radish, and rutabaga (37). Since gut microbiota is likely to produce a racemic mixture, it is not surprising that L- and D-lactate are generated simultaneously following the above food intake (37). The disposal of lactate in the lower gut can be converted to acetate, butyrate, propionate, and succinate. Alternatively, the unverified idea of “gut-soma lactate shuttle” is another way for the disposal of lactate in the gut, in which the lactate production in the gut releases into the systemic circulation (37). The discovery of the sodium-dependent monocarboxylate transporter (SMCT) (including SMCT1 and SMCT2) is favorable evidence for supporting this hypothesis, which is located in the mouse digestive tract and involved in the transport of food-derived monocarboxylates, such as lactate (71). The other implicit clue is the phenomenon of the rise in the blood L-lactate after a carbohydrate diet (37, 72). Tappy's team finds that dietary fructose or the co-mixture (fructose and glucose) facilitate the L-lactate release into the systemic circulation (73, 74). Furthermore, glucose rooted in the oxidation of carbohydrate only provides 10–20% energy, while other carbohydrate energy sources like glycogen and L-lactate accounts for 70–80% in the condition of exercise (75). That is to say when blood glucose is supported by hepatic glycogenolysis and gluconeogenesis, L-lactate plays important role in carbohydrate energy substrate distribution (72). As blood L-lactate can be transported into the brain via the MCT1 (32, 33), net L-lactate uptake directly provides 12% of brain fuel (37, 75, 76). Besides, gluconeogenesis provides 45% of brain fuel. Thereby, L-lactate comprises 57% of the total brain energy source (75). Regarding D-lactate, it can be excreted in urine by renal (64) or feces by gut (37) and cannot be detected in the blood under normal physiological conditions (64). Its excessive accumulation can result in acidosis and irritation of the lower bowel. Furthermore, the release of D-lactate into circulation also cause neurotoxic effect (37). The clinical presentation of D-lactic acidosis is characterized by episodes of encephalopathy and metabolic acidosis (24).
The Role of Lactate in Regulating Brain Functions
Lactate is reported to participate in the regulation of various brain functions in the terms of learning and memory (3), cerebral blood flow (4), neurogenesis (5, 6) and cerebral microangiogenesis (7), energy metabolism (8), neuronal activity (9–11), and neuroprotection (12–15). L-lactate and D-lactate are reported to exert similar or distinct effects on those functions.
The Distinction Between L-Lactate and D-Lactate for Influencing Learning and Memory
The Effect of L-Lactate on Learning and Memory
The glycogen is necessary for long-term potentiation maintenance in the mouse Shaffer collateral-CA1 synapse (77). Shima et al. find that 4-week exercise can increase glycogen reserve, along with the elevated transport rate of L-lactate into the neurons, to ameliorate memory dysfunction in diabetic rats (78). Extracellular L-lactate rapidly increases in the rat hippocampus during spontaneous alternation. Intrahippocampal 50 nM L-lactate can not only enhance the memory in this task but will rescue impaired memory in the condition of glycogenolysis inhibition (27). This evidence shows that the use of L-lactate metabolically coupled astrocytes and neurons depends on the character of high-energy demands for memory consolidation and storage (30). The provision of L-lactate by astrocytes is proved to be a more generally important element of learning and memory processing than memory consolidation (29). Harris et al. find that the L-lactate produced by glycolysis, is required for memory acquisition but not for established memory in mice at the age of 9 months (79), suggesting that the main function of L-lactate is regulating the process of learning (80). Recent studies reveal the fact that L-lactate transporters are necessary for L-lactate mediating the memory process, especially MCT2. Inhibition of MCT1 or MCT2 can impair the rat reconsolidation of cocaine memory (81) or the mouse long-term memory formation (82). L-lactate replenishment will reverse the memory impairment in MCT1 or MCT4 knockdown of rat hippocampus (77). By contrast, L-lactate replenishment or even glucose supplement will not rescue the impaired memory once blocking MCT2 activity, which transports L-lactate into neurons (27, 77). In these studies, L-lactate in neurons is seen as the pyruvate donor, which produces ATP for neuronal energy demand (see section “Serves as an energy substrate for neurons”). Therefore, the transfer of L-lactate from astrocytes into neurons to support neuronal functions is necessary for memory consolidation (77, 83). The mechanisms may involve sustaining synaptic transmission and function (84–88) and regulating synaptic plasticity-related genes and proteins expression (89, 90). In facilitating the synaptic transmission and function, Tang et al. find that injections of 2 mM L-lactate into the locus coeruleus can activate an unidentified Gs receptor to evoke the NEergic neuronal excitability for norepinephrine release (85). Schurr et al. first find that L-lactate can replace glucose as a sole energy substrate for sustaining the normal synaptic function in rat hippocampal slices for hours (87). Lucas et al. declare that the physiological concentration of L-lactate can be utilized by presynaptic terminals as the energy for meeting the demand of maintaining functional presynaptic release sites (84). Herrera-Lo'pez et al. find that 1–2 mM L-lactate can induce glutamatergic synapse potentiation to promote the memory formation process in rat hippocampal CA3 pyramidal cells (86). As for modulating the synaptic plasticity genes and proteins expression, Margineanu et al. find that 20 mM L-lactate treatment can promote various plastic plasticity and plastic activity-related genes in mouse cortical neurons through RNA sequence (89). Yang et al. find that L-lactate from 2.5 to 20 mM will potentiate the NMDAR activity to improve the immediate early genes (IEGs) expression in mouse cortical neurons, which benefits the form of learning and memory (90). Hayek et al. find that intraperitoneal injection physiological concentration of L-lactate (117 or 180 mg/Kg) for 1 month can promote the proteins and genes expression of brain-derived neurotrophic factor (BDNF) and IEGs to improve the mouse learning and memory ability (3) (Figure 2).
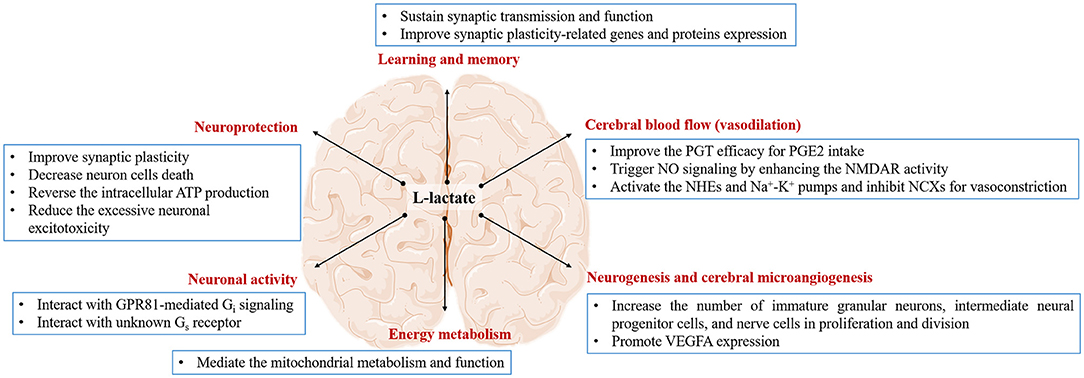
Figure 2. The effect of L-lactate and its possible mechanism on the brain. L-lactate is capable of regulating brain function in the terms of learning and memory, cerebral blood flow, neurogenesis and cerebral microangiogenesis, energy metabolism, neuronal activity, and neuroprotection via different pathways.
The Effect of D-Lactate on Learning and Memory
D-lactate is proved to impair the neonatal chick memory process in the way of intracranial injection (23, 91). The reasons ascribe inhibition of L-lactate uptake into neurons and interference with the astrocytic metabolism. Baker and Edwards find that bilateral administration of D-lactate in the lower dosage range of 1.75–2.25 mM inhibits memory retention after discrimination avoidance task from 40 min and onward, and the inhibitory effect sustains 140 min. And the scope of the validity period is from 10 min before to 20 min after the task (91). This time window is similar to the dosage of 10 nM (23). The observation indicates that the inhibitory effect of D-lactate on memory is valid for a certain period (23). Further to support this idea is the study of rodents. Michael et al. find that intracranial injection of a higher concentration of D-lactate (18 mM) at 30 min before the Y maze task seems to not affect memory retention (92). Scavuzzo et al. even find that subcutaneous injections of D-lactate (1 g/kg) impair memory at 15 min before the inhibitory avoidance (IA) task, whereas it significantly enhances the rat memory at 2 min after the training (80) (Table 1). Thereby, it is still debatable that the different concentration and time-point of D-lactate usage lead to the distinct results for memory up to date. The conflict results are puzzling what is the physiological role of D-lactate in learning and memory. In short, it will be interesting to figure out the mechanism of D-lactate on the memory at the level of molecules and physiology. More interestingly, the results of intracranial unilateral injection by Gibbs and Hertz also show that D-lactate injection into left intermediate medial mesopallium inhibits memory formation from 10 min before task, whereas injection into the right hemisphere works from 10 min after task (23), implying that the time window of D-lactate on inhibiting memory formation varies from the part of the brain hemisphere.
L-Lactate Increases Cerebral Blood Flow (Vasodilation)
For the last few years, the function of L-lactate regulating cerebral blood flow (CBF) has been proposed in several studies (4, 95–98). First, a hypoxia-induced increase of L-lactate can improve the prostaglandin transporter (PGT) efficacy for vasodilator prostaglandin E2 (PGE2) intake in the brain (4). Second, exercise is reported to trigger the N-methyl D-aspartate (NMDA) receptor/nitric oxide (NO) signaling for realizing the hippocampal functional hyperemia during neural activation (99). Previous evidence indicates the effect of L-lactate on enhancing the NMDAR activity in cortex neurons (90). Moreover, when the porcine second-order retinal arterioles are pressurized to no flow, 10 mM L-lactate can induce the release of NO synthase to activate the downstream guanylyl cyclase/cGMP signaling, which opens the KATP channels for vasodilation in retinal arterioles (100). Contemplating all this, it is possible for L-lactate to dilate the brain microvessels for increasing CBF via NO biological effect. During hypoglycemia, the CBF increase can be considered neuroprotective since it is an attempt to increase capillary glucose concentration for improved glucose supply to the brain (101). Third, L-lactate has the autoregulatory vascular function in switching the pericyte response from contraction to dilation in the retinal vasculature. In the normal physiological state, L-lactate activates the Na+/H+ exchangers, inhibits Na+/Ca2+ exchangers, and excites Na+-K+ pumps to trigger pericyte vasoconstriction regardless of the L-lactate concentration (Figure 2). While under the hypoxic condition, 20 mM L-lactate induces mural cell relaxation throughout the retinal vasculature, from the arterioles to the capillaries (102). Exploring the L-lactate-induced vascular reaction when dealing with the physiological and pathological situation, may be crucial for aiding in our understanding of neurovascular coupling, enhancing brain functions, and even treating multiple brain diseases.
L-Lactate Benefits the Process of Neurogenesis and Cerebral Microangiogenesis
Neurogenesis is important for memory and learning. Lambertus et al. find that the 7-week exercise or 18 mM L-lactate can induce neurogenesis in the mouse ventricular-subventricular zone but not in the hippocampus (5). Notably, the author mentioned that neurogenesis in this zone contributes to the olfactory memory, which may provide contextual clues to the spatial-visual memory controlled by the hippocampus (5). Controversially, Vachnish et al. find that 6-week 13–17 mM L-lactate can promote mice hippocampal neurogenesis (6). The distinct results are probably related to the selection of neurogenic markers. The former refers to DCX and Ki-67 to represent neurogenesis, which shows immature granular neurons and intermediate neural progenitor cells, respectively. The latter chooses BrdU as the neurogenic marker, which shows nerve cells in proliferation and division. The different markers are on behalf of different stages of neurogenesis (103). The above evidence suggests that the neurogenic effect of L-lactate is tissue-specific. For example, the effect of L-lactate on the ventricular-subventricular zone depends on the GPR81 action but the hippocampus depends on the MCT2 metabolic action. Since neurogenesis is highly correlated with cerebral microangiogenesis, there is no doubt that angiogenesis also may be influenced by L-lactate in the brain. The direct evidence is supported by Moland's team. The study declares that 7-week 18 mM L-lactate is capable of increasing the density of microvessels in the dentate gyrus of the hippocampus along with a higher VEGFA expression. Furthermore, this effect depends on the GPR81 action (7) (Figure 2). In the further study, figuring out the approaches of L-lactate how to deal with neurogenesis and cerebral microangiogenesis (metabolic pathway, lactormone action, or both), may be worthiness for understanding the physiological role of L-lactate in brain function regulation.
L-Lactate Influences Brain Energy Metabolism
Mitochondria are best known for their role in the generation of ATP that supplies eukaryotes with energy to serve their cellular needs (104). Recent studies report that L-lactate can also mediate various mitochondria-related genes. A novel discovery is first reported by Brooks's team in L6 cells (105). They find that 20 mM L-lactate can upregulate 79 genes involved in mitochondrial metabolism (MFN1, MFN2, PGC-1α, NRF2, LDHb, ATP5g1, NADH-dh, SDH, and TIM) and oxidative stress (GPX1, Glrx2, Glrx5, Prdx2, and Txndc12) (105). The evidence is also verified in the brain. For example, intraperitoneal injection of 18 mM L-lactate for 14 consecutive days will promote the PRC mRNA expression and the mtDNA levels (8). 20 mM L-lactate pretreats SY5Y cells can reverse high-concentration hydrogen peroxide (H2O2)-induced oxidative stress injury, including NRF2 expression improvement and mitochondrial membrane potential potentiation (106). The biological effect of L-lactate mediating mitochondrial metabolism and function is also in the primary mouse neurons. A total of 15–20 mM L-lactate can improve mitochondrial fusion (OPA1, MFN1, and MFN2), inhibit mitochondrial fission (DRP1 and FIS1), and promote biogenesis (PGC-1α, NRF2, TFAM, and mtDNA) (3, 107) (Figure 2). Besides, “cytosol-mitochondria lactate shuttle” also elaborates the role of L-lactate as the substrate source of mitochondrial ATP production (53, 56–58). Thus, L-lactate ought to have a close relationship with mitochondria in the brain. Considering the fact that multiple brain diseases in a large part are also associated with the energy crisis, the application of L-lactate in an animal-related experiment to shed light on the effect and mitochondria mechanism on brain energy metabolism as early as possible should have a great significance of the novel treatment in clinic brain diseases.
L-Lactate and D-Lactate Influence Neuronal Activity
The previous study demonstrates that lactate interacting with its receptor GPR81 operates negative feedback on neuronal activity. Application of 5 mM L- or D-lactate reversibly diminishes the calcium transient frequency by more than 50% in both principal and GABAergic neurons (Table 1). Moreover, the activation of GPR81 can also mimic a similar potency as the lactate (9). In fact, lactate can bind to GPR81 to couple with the Giα subunit to inhibit the intracellular adenylate cyclase (AC)-cyclic adenosine monophosphate (cAMP) cascade signal that contributes to the reduction of exocytosis (10, 11). In addition, the GPR81 activation can bind to the Giβ subunit to regulate the activity of phospholipase C (PLC) and thus inhibit neuronal excitability (10) by inducing a hyperpolarization for potassium (K+) indrawal or activating the GABA receptor for exocytosis reduction (9). Interestingly, 5 mM L-lactate and 0.56 mM 3,5-dihydroxybenzoic acid (3,5-DHBA) (one of the GPR81 agonists) reduce the firing frequency of CA1 pyramidal cells, whereas a higher level of L-lactate (30 mM) and 3,5-DHBA (3.1 mM) increase the firing frequency (11). In the locus coeruleus, 2 mM L-lactate has rather an excitatory effect on the NEergic neurons by activating an unidentified Gs receptor, which will be abolished by the D-lactate (85) (Figure 2). Given these, we speculate that the effect of L-lactate on neuronal activity may depend on the L-lactate concentration, the types of neurons, and the types of receptors. Knowledge of the physiological effects of different concentrations and lactate isomers on the activity of different neurons, application of lactate is a potential therapeutic way to improve the complex neurological symptoms of epilepsy, which disease is characterized by neuronal hyperexcitability and sudden, synchronized electrical discharges (47, 108). In fact, some studies have focused on the application of L-lactate in treating epilepsy (see section Epilepsy).
The Neuroprotective Role of L-Lactate and D-Lactate
Lactate has been reported the role of neuroprotection in multiple brain disease models, however, the effect of the isomer of L- and D-lactate is split depending on the disease patterns. As known, transient middle cerebral artery occlusion (tMCAO) in vivo and oxygen and glucose deprivation (OGD) in vitro are the desired models for stroke. In the tMCAO model, L-lactate treatment can ameliorate neurological deficits after ischemia by inducing sustained neuroprotection for up to two weeks (14, 15). And as for the D-lactate treatment, it is also reported to exert neuroprotection by decreasing the infarct volume and ameliorating neurological deficits by working as the energy substrate or activating the receptor GPR81 (12). In the OGD model, the effect of ameliorating neuronal injury is possessed by both L-lactate and D-lactate (12–14). Hence, L- and D-lactate seem to exert a neuroprotective role in stroke.
In the human and rodent traumatic brain injury (TBI) model, evidence shows that endogenous produced brain L-lactate or exogenous L-lactate replenishment can facilitate neurologic recovery by improving synaptic plasticity (109) and regulating the brain metabolic state (76, 110–114) (see section “TBI”). However, the level of blood D-lactate is adopted as the biomarker of TBI degree. This is because that D-lactate is one of the microbe-dependent metabolites which will pass through the destroyed intestinal barrier into the blood when TBI causes gut dysbiosis (115, 116).
In addition, L-lactate but not D-lactate can reduce the excessive NMDAR-induced neuronal excitotoxicity, which is common in some acute brain pathologies. 10 mM L-lactate can improve the intracellular ATP production to stimulate metabotropic purinergic receptor P2Y for activating the PI3K pathway and opening the KATP channels, as a result of decreasing the intracellular Ca2+ concentration (93) (Figure 2). Furthermore, in the high concentration of glutamate-induced neurotoxicity model, infusion of 6 mM L-lactate can obviously reduce the rat cortex lesion from 6.05 ± 0.64 mm3 to 4.16 ± 0.43 mm3. On the contrary, the same concentration of D-lactate aggravates the lesion area from 2.7 ± 0.4 mm3 to 4.4 ± 0.5 mm3 (18) (Table 1). With a view to the metabolism of lactate and the universality of neuroprotective effects in the brain, L-lactate is a preferred research direction and practical value of the application and treatment in neuroprotection.
Lactate Is the Potential Therapy for Multiple Brain Diseases
In the preceding decades, lactate was long considered as a waste product or even a sign of cerebral harm. As the proposal of the novel opinion such as “lactate shuttles” (37) and “lactormone” (22), these theories transform our stereotype and gradually unlock the mystery of lactate in physiological and pathological states. Recently, lactate (especially L-lactate) is postulated to protect the brain in several pathologic conditions, such as diabetic encephalopathy, Alzheimer's disease (AD), stroke, TBI, and epilepsy.
Diabetic Encephalopathy
Diabetes mellitus often hurts brain health, which accompanies the symptoms of brain functional decline (117–119), especially cognitive impairment and vascular dementia (120). This symptom is called “diabetic encephalopathy” (121). Evidence shows that the brain lactate falls in response to hypoglycemia in diabetes (122, 123). Intravenous injection of L-lactate is capable of reducing the symptomatic and adrenaline responses to hypoglycemia and simultaneously alleviating hypoglycemia-induced cognitive dysfunction in diabetic patients (124–127). In addition, intracerebroventricular administration of 200 nM L-lactate can also mitigate the deterioration of memory consolidation via recovering the amplitude of sharp-wave ripples (SWRs) in diabetic mice (128). These results directly demonstrate that the L-lactate supplement benefits diabetic brain function. Another indirect evidence is that an increase of L-lactate transporter MCTs in the cortex and/or the hippocampus, especially MCT2 (transport L-lactate into neurons), is observed to be capable of ameliorating the cognitive impairment in diabetic encephalopathy (32, 78, 129). It is conceivable that L-lactate uptake enhancement supported by MCTs may improve diabetic encephalopathy. However, some studies show the phenomenon that the L-lactate increases in the brain regions of corpus callosum and hippocampus in diabetic encephalopathy (130–132). One possibility is the low utilization rate of L-lactate during diabetic encephalopathy (133, 134). The convincing evidence listed is that the L-lactate is secreted to increase in astrocytes but not in neurons in the condition of a high-glucose environment (134, 135), implying that the transport of L-lactate into neurons is hampered and thus the elevated brain L-lactate is observed in diabetic encephalopathy.
AD
Alzheimer's disease (AD) is one of the most common forms of brain disease (136), characterized by a series of neuropathological changes, such as cognitive dysfunction. Cognitive impairment in AD is associated with recessionary energy metabolism (137). Whereas, L-lactate seems like an important energy substrate for memory formation in connection with energy supply (19, 27). In fact, decreased L-lactate content is observed in the cerebral cortex and hippocampus in AD rodent models (138–140). Nevertheless, elevated L-lactate levels occur in aging models (94, 141, 142) and AD patients (143). The increased lactate dehydrogenase A (LDH-A)/lactate dehydrogenase B (LDH-B) gene activity ratios (94) and downregulated MCTs (MCT1, MCT2, and MCT4) are due for the opposite results (139, 140). This is because that increased brain L-lactate production owing to the LDH-A/LDH-B ratio but blockage of L-lactate transport from glia to neurons, resulting in L-lactate deficit in neurons. Inferior L-lactate utilization rate leads to energy deficiency in neurons and thereby exacerbates the progression of neuronal injury, including cognitive impairment during the pathological process of AD or aging.
Regarding D-lactate, a study reports that D-LDH, located at the inner side of the rat liver mitochondrial inner membrane (MIM), oxidizes D-lactate to pyruvate in the mitochondrial matrix (144). This process will improve oxidative phosphorylation (OXPHOS) efficiency, synthesis, and efflux of biosynthetic precursors from mitochondria, balance the cytosolic and mitochondrial GSH pools, and enhance the NADPH production in the cytosol. All of these can contribute to mitochondrial energy production (145). Nevertheless, the D-LDH isoforms in both human and mouse brains show a rather weak signal (67). Based on this evidence, we speculate that moderate D-lactate combined with D-LDH supplement may target a novel mitochondrial energetic mechanism for AD treatment.
Stroke
Stroke, one of the important factors causing neurological morbidity and mortality, is most characterized by ischemia and infarcts involving the territory of the middle cerebral artery (146). Berthet et al. adopt different concentrations of L-lactate treatment after OGD in the rat hippocampal slices. The results show that, immediately after OGD, the low dose treatment of L-lactate (4 mM) significantly inhibits the hippocampal neuron death, while L-lactate at the 20 mM dose aggravates the neuronal injury (14). Whereas, during OGD, only a high dose of L-lactate at 20 mM is the capacity of reversing the ATP decline to prevent cell death in primary cultured neurons and N2A cells, but not the low dose at 1 mM (13). Hence, the neuroprotective effect of L-lactate on stroke may be related to the time points for intervention or the types of neuron cells. Further study shows that L-lactate can reduce the size of the lesion area to promote neurological rehabilitation in the way of intracerebroventricular injection of 100 mM L-lactate (14) or tail vein injection of 200 mM L-lactate (15) immediately after tMCAO. They speculate that the ischemia-induced lactate increase may initially have a neuroprotective effect on the brain, but also may cause lactic acidosis when the lactate concentration reaches higher levels (14). Therefore, different concentrations of L-lactate may have different regulatory effects on brain metabolism and function under different physiological metabolic states. The complex metabolic characteristics of L-lactate bring immense challenges for revealing its role in brain function (14). Soon afterward, their team finds that similar neuroprotection occurs in D-lactate. The results suggest that intravenous injection of 1 μmol/g D-lactate will decrease about half of the infarct volume in tMCAO of the mice. Moreover, in the OGD model, 4 mM D-lactate treatment can also reduce about 50% neuronal cell death in the rat hippocampal slices (12). Future studies need further excellently clarify the mechanism of how lactate performs neuroprotection although it has fully considered the dual role of lactate as the energy substrate (pyruvate replacement) or signal molecular (receptor agonist replacement). So far, two results are responsible for explaining the neuroprotective effect of lactate on stroke. First, the accumulated lactate is transported by MCTs into the neurons and utilized as the alternative energy substrate immediately postischemia (147) in favor of sustaining neuronal integrity (148) and delaying the neuronal damage (149). Second, lactate may interact with the known receptor-GPR81 or unknown putative Gs-type receptors to resist ischemic injury. It is worth noting that the evidence and characterization of GPR81 are still disputed in stroke. For example, Castillo et al. find the 3,5-DHBA treatment, one of the GPR81 agonists, can reduce neuronal cell death in OGD (12). In contrast with the study, Shen et al. find that inhibition of the GPR81 activity can promote the ERK1/2 signal to copy with apoptosis in neurons (13). Moreover, a newly published study also reports that the GPR81 agonists (3Cl-5OH-BA and 3,5-DHBA) do exert no neuroprotective effect on stroke mice whether intravenous administration or intracerebroventricular administration (150). Applying the genetic tools for GPR81 knockout may be a preferred protocol to definite the role and the mechanism of this receptor in the stroke.
TBI
Traumatic brain injury (TBI) is a type of brain injury acquired from an external force that inflicts devastating effects on the brain vasculature and neurons (116). It is always reported to face an energy crisis in the brain in the fact of glucose uptake suppression following cerebral injury. Although glucose is the preferred fuel of the brain, it may result in odious insults, such as hyperglycemia and infection or mortality events increment (151–153). As in previous studies, many TBI patients have observed the increased net L-lactate uptake after-hours postinjury (76, 154) without hyperglycemia (110), implying the protective function of L-lactate in TBI. Increasing blood arterial L-lactate to supraphysiologic range (4–5 mmol/l) in the early phase of brain injury can accelerate the recovery of neural function through cerebral perfusion and brain glucose availability improvement (155). Brooks and Martin find that endogenous L-lactate, induced by TBI, can support brain metabolism indirectly or directly (76). On the one hand, endogenous lactate is reported to be related to accelerating hepatic and renal gluconeogenesis to make glucose available for essential organs like the brain. On the other hand, elevated blood L-lactate may provide substrate directly to the injured brain in link with the L-lactate shuttle (114). Through isotope tracer, ~70% carbohydrate (direct lactate uptake and indirect glucose uptake from lactate) is provided for the TBI brain (113), suggesting the importance of endogenous L-lactate generation as the energy supply in TBI. In addition, exogenous intraperitoneal injection of 500 mg/kg L-lactate before 30 min of TBI is reported to alleviate the brain injury-induced neurological deficits by promoting the neuronal plasticity proteins (PSD95, GAP43, and BDNF) (109). Intravenous infusion of 5 mM L-lactate is also verified to utilize with sparing of cerebral glucose along with a reduction of brain glutamate and intracranial pressure after TBI, which implies the benefit of cerebral metabolic and hemodynamic effects (110). A total of 100 mM L-lactate infusion is shown reduced lesion volume at day 2 after cerebral cortical impact through slightly regulating cerebral blood flow (111). Hence, L-lactate, as the alternative source of glucose, has dream prospects to improve the outcome of posttraumatic brain damage.
Recently, the lactate receptor GPR81 is also reported to potentially participate in the pathological process of TBI. The evidence is that the increased GPR81 gene expression lasts at least 28 days after TBI injury in lesion areas of the cerebral cortex and hippocampus (156). Coincidentally, L-lactate further enhances the expression of GPR81 in the ipsilateral cortex and hippocampus 24 h after TBI (109). These results show that L-lactate may not only be the brain fuel, but also the possible upstream molecule that interacts with the receptor to activate or inhibit the downstream molecular signals for neuroprotection in the TBI.
Epilepsy
Epilepsy is a common neurological emergency with considerable associated healthcare costs, morbidity, and mortality, which is often causing neuronal and glial damage (157). During epilepsy, L-lactate is observed to rise in the brain regions of cerebral gray and white matter (158), cerebrospinal fluid (159), cerebral cortex (160), and hippocampus (161). Previously, one research has proved that the elevated L-lactate level in tissues can persist for approximately 1 h (161). In some cases, the L-lactate level can even reach 6 mM (162). Jorwal and Sikdar find that 6 mM L-lactate application reduces the spike frequency and hyperpolarizes the subicular neurons in rat hippocampal slices, suggesting an anticonvulsant effect of L-lactate. Further electrophysiological recordings reveal that L-lactate induces the Giβγ subunit activity to expedite the inwardly rectifying potassium (GIRK) channel. They also point out an interesting phenomenon that normal brain concentration of L-lactate (2 mM) and even up to 6 mM have few effects on rat hippocampus neural activity in the absence of epileptiform activity. This fact reminds us that the important role of elevated brain L-lactate in epilepsy (163). It is worth thinking that since there is no negative effect of excessive brain L-lactate on the mean spike frequency and membrane potential of subicular pyramidal neurons in the normal physical condition, what physiological effects can this L-lactate have after being metabolized or working as the neurotransmitter? Considering the fact that and concentration of 6 mM L-lactate is sufficient to activate its receptor GPR81, which is also known to couple to Giβγ subunit to inhibit the neuronal activity (10). Another function of GPR81 is to couple to the Giα subunit to inhibit the AC-cAMP cascade signal, as a result, the decrease of neuronal excitement (11). However, unlike L-lactate, 3,5-DHBA does not result in hyperpolarizing subicular neurons (10). Therefore, it is likely that L-lactate may interact with other unknown Gi-type proteins. L-lactate-receptor signals may be the novel target for antiepileptic treatment.
The Neurobiological Role of L-Lactate in the Brain
Serves as an Energy Substrate for Neurons
Astrocytic derived-L-lactate has been proposed to serve as an energy pool for neurons (40). In the presence or absence of oxygen, glycogen in the astrocytes can be broken down into L-lactate (30). An interesting phenomenon that manifests the role of L-lactate in neuronal energy metabolism is that the levels of glycogen in various brain regions (cortices, hippocampus, brainstem, epencephalon, and hypothalamus) are constant during the first hour of moderate-intensity exercise, while the levels of glycogen will decrease approximately 50% in the several hours that follow. Accompanied with the decreased glycogen levels are the elevated L-lactate levels in these regions (164). A similar phenomenon exists in the exhaustive swimming model, especially in the hippocampus (165). L-lactate oxidation metabolism is preferentially located in neurons by using the 3-[13C] lactate label (166). Furthermore, when increasing the plasma L-lactate levels, the cerebrum tends to reduce glucose utilization and prefers to utilize L-lactate by using the 1-[11C]L-lactate and [18F]fluorodeoxyglucose (FDG) for local cerebral metabolism measurement (148). The formed L-lactate in the brain is a crucial aerobic energy substrate that enables neurons to endure activation (167). Another evidence for L-lactate is the energy substrate of neurons is that the capacity of sustaining the presynaptic transmission function in the lack of glucose. The interesting note is that the transport of L-lactate into neurons is significant for ATP production to satisfy the energy demand under physiological or hypoxic conditions (28, 84, 168) (Figure 3).
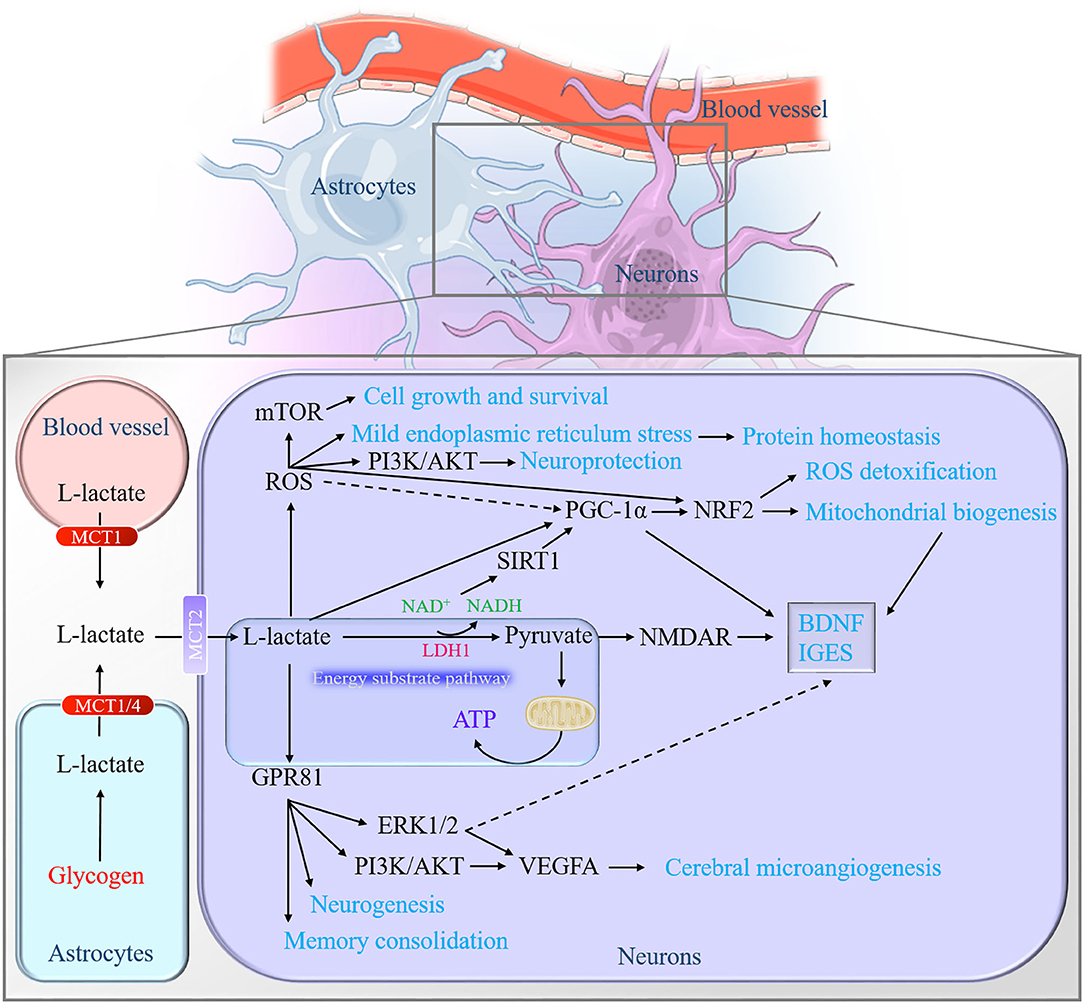
Figure 3. Graphical summary of the neurobiological role of L-lactate in the brain. On the one hand, L-lactate serves as an energy pool for neurons to facilitate mitochondrial energy production through converting into pyruvate. On the other hand, L-lactate may act as the role like the hormone, defined as lactormone. It triggers multiple downstream signaling cascades via influencing the intracellular NADH/NAD+ ratio, mediating the ROS generation, and activating the receptor GPR81. NADH, nicotinamide adenine dinucleotide; ROS, reactive oxygen species; GPR81, Gi-protein-coupled receptor 81.
Signal Pathways Regulation as a Lactormone
In addition to being an energy substrate, L-lactate is also suggested its role as a signal regulator molecule or neurotransmitter since the proposal of “lactormone” (22) and the confirmation of its endogenous receptor GPR81 in mice brain (169).
Influences the Intracellular NADH/NAD+ Ratio
The catalytic action of LDH1 in neurons allows L-lactate to convert into pyruvate. This process of reducing NAD+ to NADH is supposed to influence NMDAR excitability (16, 93, 170). It is L-lactate, but not pyruvate, that generates NADH to enhance the NMDAR activity, which increases the intracellular Ca2+ and then promotes the expression of plasticity-associated genes (90). Along with the changes of redox state (NADH/NAD+ ratio), L-lactate is permissible to activate the NAD+-dependent histone deacetylase Sirtuin1 (SIRT1) (3, 171). SIRT1 deacetylates and amplifies the activity of the peroxisome proliferator-activated receptor-gamma coactivator-1 alpha (PGC-1α), which is known to mediate the BDNF expression (3) and mitochondrial biogenesis (172, 173). For example, Hayek et al. find that L-lactate can induce the expression of SITRT1 to activate the PGC1-1α, which will contribute to BDNF signals and some immediate early gene (IEGs) as a result in the mouse neurons (3). Our experiment also finds that a high concentration of L-lactate promotes the PGC-1-NRF2 signal axis and subsequently boosts mitochondrial biogenesis in mouse primary hippocampal cells (107) (Figure 3). However, Lezi et al. find that 14 consecutive-day-intraperitoneal injections of 18 mM L-lactate do not affect the PCG-1α-mediated mitochondrial biogenesis in the mouse brain (8). This may relate to the short administration time or the examination of the whole brain.
Activates Reactive Oxygen Species-Related Signals
It is well-known that excessive reactive oxygen species (ROS) production is considered a cause of several pathological conditions, which are harmful to the cells. Yet, low ROS production may be relevant to cellular activity under physiological conditions (174). Moreover, the moderate elevation of ROS can even protect against oxidative damage through the induction of antioxidant and detoxification enzymes (175).
Hashimoto et al. first report that L-lactate is able to stimulate some of the ROS-sensitive transcription factors' activity in L6 cells. Their research also reports that the PGC1α, known as a master coordinator of mitochondrial biogenesis, is activated in a high concentration of L-lactate treatment which is believed to be regulated by ROS and H2O2. Then the activated PGC1α interacts with transcription factors for mitochondrial gene expression, including cAMP-response element-binding protein (CREB) and nuclear respiratory factor (NRF)-2 in L6 cells (105). L-lactate metabolism also potentiates the phosphorylation of adenosine 5'-monophosphate (AMP)-activated protein kinase (AMPK) to activate PCG-1α signals for mitochondrial biogenesis and inhibits the phosphorylation of mTOR for mitophagy via mild production of ROS in skin fibroblasts (175). Recently, a similar phenomenon occurs in neurons. In SY5Y cells, 20 mM L-lactate is proved to induce moderate ROS for antioxidant stress and cellular homeostasis improvement, for instance, activating mTOR signaling for cell growth and survival, phosphatidylinositol 3-kinase (PI3-K)/protein kinase B (AKT) signaling for neuroprotection, mild endoplasmic reticulum stress (ERS) for protein homeostasis, and NRF2 signaling for ROS detoxification (Figure 3). Interestingly, it is an extremely high concentration of L-lactate (100 mM) but not lower or physiological concentration of L-lactate (50 and 10 mM) exerts antiaging phenotypes when Caenorhabditis elegans suffer from oxidative stress. Furthermore, 10 mM L-lactate suffice to resist stress and increase their lifespan (106).
Noteworthy, it is not known how L-lactate is ready-witted enough to mediate and maintain moderate ROS production for safeguarding cells when a high concentration of H2O2 provokes excessive ROS. One of the reasons may be associated with the potential ability of L-lactate sensing and decreasing the mitochondrial H2O2 production accurately to ensure moderate ROS production (175). Another hypothesis of L-lactate mitochondrial metabolism may explain the ROS signals activation. Bari et al. find that L-lactation can generate the H2O2 production via the inner membrane of putative L-lactate oxidase (LOX) in the pure rat liver mitochondria (176). Hence, L-lactate metabolism in mitochondria is likely to produce partial ROS and then activate its related signals if there exists analogous oxidase located at the mitochondrial inner membrane in the neurons. The evidence supports this hypothesis is that a mitochondrial lactate oxidation complex (mLOC) (including MCT1 or MCT2, LDH, and COX) is present in the neurons discovered by Hashimoto et al. (54). However, how L-lactate metabolism in neurons produces and regulates ROS in the subsequent process remains to be further investigated.
Interacts With the Receptor GPR81
GPR81 is a type of G protein (Gi) coupled receptor and involves the metabolic process of some tissues and cells (177–179). So far, recent research has revealed that GPR81 regulates multiple signal pathways in tissues and cells, such as the extracellular regulated protein kinases (ERK1/2) (7, 180–182), nod-like receptor family pyrin domain-containing 3 (NLRP3)/ nuclear factor kappa-B (NF-κB) inflammation (183, 184), peroxisome proliferator-activated receptor γ (PPARγ) (185, 186) and Wnt signaling pathway (187, 188).
In the brain, it expresses in the regions of the pituitary (177), hippocampus, cerebellum, and brain stem (169). Cell localization indicates that it widely distributes at neuronal synaptic membranes (169), implying that GPR81 may have an important role in regulating brain function. It is known as the endogenic lactate receptor in 2008 (189, 190). The efficiency of lactate on GPR81 has a wide range from 0.2 to 30 mM (191). Furthermore, partial activation of GPR81 by lactate requires only 0.2–1 mM (192). Therefore, lactate absolutely has the ability to activate the GPR81 in the physiological state (human blood lactate concentration range is 0.5–2 mM) (193). Needless to say, in the conditions of intensive exercise and hypoxia, the blood lactate level can even reach 20–30 mM (194, 195). In neuronal activity, the activation of GPR81 inhibits the excitability of neurons (9, 10). In cellular energy, the astrocytic glycolysis rates accelerate ATP production to meet the energy requirements of neurons when the GPR81 agonists stimulate the cells (196). In terms of cerebral microangiogenesis and neurogenesis, L-lactate and exercise enhance the PI3K/AKT and ERK1/2 signaling pathway to promote VEGFA protein expression and vascular density in the dentate gyrus of mice via GPR81 (7). In addition, the neurogenesis effect of both L-lactate and exercise on the ventriculosubventricular region is dependent on the GPR81 in the mice (5). As for the learning and memory, intraperitoneal injection of GPR81 agonist 3,5-DHBA before the inhibitory avoidance task will impair the memory of rats, while the injection after the training contributes to memory consolidation. The distinct effects may be attributed to the reasons that the activation of GPR81 in the forebrain after training, can promote slow-wave activity (SWA) and enhance the consolidation of previous experience, but it also impairs the ongoing learning process at the same time (80). The results suggest that GPR81 activation at different stages of training may have different functions of influencing learning and memory. Synaptic plasticity, induced by appropriate synapses, is both necessary and sufficient for the information storage of memory (197). Previous studies revealed that L-lactate could promote plastic plasticity-related proteins and genes expression via enhancing the NMDAR-mediated phosphorylation of ERK1/2 (89, 90). The interesting thing is the fact that GPR81 is upstream of the ERK1/2 (7) (Figure 3). Hence, GPR81 is likely to act as a sensor for L-lactate metabolism in neurons and participate in L-lactate-regulated synaptic plasticity (198).
Conclusion
To summarize the current evidence, this review shows that lactate participates in coordinating various brain functions in both the healthy and diseased states. The involving mechanisms are far more complex than originally thought and further knowledge of the lactate operating, as outlined in this article, is a drop in the bucket to our understanding of brain physiological and pathological mysteries. L-lactate not only serves as the energy substrate but also acts as lactormone in the regulation of downstream cascaded signaling pathways. Considering the consensus that lactate is a common metabolite and readily available (food intake or exercise), knowledge of lactate's role in the brain may provide precise tactics for brain diseases involving lactate metabolism. It also may set new insight and ideas for studying the relationship between lactate metabolism and brain function improvement.
Author Contributions
MC and HW drafted the manuscript. HS, RY, and LW assisted with drafting the tables and figures. XX suggested valuable advice for the article. WS and JH conceptualized the article and revised the final version. All authors read and approved the final manuscript.
Funding
This work was sponsored by Shanghai Sailing Program (22YF1441600), the grant of the funding of Youth Fund Project of Research Planning Foundation on Humanities and Social Sciences of the Ministry of Education (20YJCZH001), China Postdoctoral Science Foundation (2021M702128), and the Scientific Research Foundation of SUMHS (SSF-21-03-008).
Conflict of Interest
The authors declare that the research was conducted in the absence of any commercial or financial relationships that could be construed as a potential conflict of interest.
Publisher's Note
All claims expressed in this article are solely those of the authors and do not necessarily represent those of their affiliated organizations, or those of the publisher, the editors and the reviewers. Any product that may be evaluated in this article, or claim that may be made by its manufacturer, is not guaranteed or endorsed by the publisher.
References
1. Mason S. Lactate shuttles in neuroenergetics-homeostasis, allostasis and beyond. Front Neurosci. (2017) 11:43. doi: 10.3389/fnins.2017.00043
2. Todd JJ. Lactate: valuable for physical performance and maintenance of brain function during exercise. Biosci Horiz. (2014). doi: 10.1093/biohorizons/hzu001
3. El Hayek L, Khalifeh M, Zibara V, Abi Assaad R, Emmanuel N, Karnib N, et al. Lactate mediates the effects of exercise on learning and memory through SIRT1-dependent activation of hippocampal brain-derived neurotrophic factor (BDNF). J Neurosci. (2019) 39:2369–82. doi: 10.1523/JNEUROSCI.1661-18.2019
4. Gordon GR, Choi HB, Rungta RL, Ellis-Davies GC, MacVicar BA. Brain metabolism dictates the polarity of astrocyte control over arterioles. Nature. (2008) 456:745–9. doi: 10.1038/nature07525
5. Lambertus M, Overberg LT, Andersson KA, Hjelden MS, Hadzic A, Haugen OP, et al. L-lactate induces neurogenesis in the mouse ventricular-subventricular zone via the lactate receptor HCA1. Acta Physiol. (2021) 231:e13587. doi: 10.1111/apha.13587
6. Lev-Vachnish Y, Cadury S, Rotter-Maskowitz A, Feldman N, Roichman A, Illouz T, et al. L-Lactate promotes adult hippocampal neurogenesis. Front Neurosci. (2019) 13:403. doi: 10.3389/fnins.2019.00403
7. Morland C, Andersson KA, Haugen OP, Hadzic A, Kleppa L, Gille A, et al. Exercise induces cerebral VEGF and angiogenesis via the lactate receptor HCAR1. Nat Commun. (2017) 8:15557. doi: 10.1038/ncomms15557
8. E L, Lu J, Selfridge JE, Burns JM, Swerdlow RH. Lactate administration reproduces specific brain and liver exercise-related changes. J Neurochem. (2013) 127:91–100. doi: 10.1111/jnc.12394
9. Bozzo L, Puyal J, Chatton JY. Lactate modulates the activity of primary cortical neurons through a receptor-mediated pathway. PLoS ONE. (2013) 8:e71721. doi: 10.1371/journal.pone.0071721
10. de Castro Abrantes H, Briquet M, Schmuziger C, Restivo L, Puyal J, Rosenberg N, et al. The lactate receptor HCAR1 modulates neuronal network activity through the activation of galpha and gbetagamma subunits. J Neurosci. (2019) 39:4422–33. doi: 10.1523/JNEUROSCI.2092-18.2019
11. Herrera-Lopez G, Galvan EJ. Modulation of hippocampal excitability via the hydroxycarboxylic acid receptor 1. Hippocampus. (2018) 28:557–67. doi: 10.1002/hipo.22958
12. Castillo X, Rosafio K, Wyss MT, Drandarov K, Buck A, Pellerin L, et al. A probable dual mode of action for both L- and D-lactate neuroprotection in cerebral ischemia. J Cereb Blood Flow Metab. (2015) 5:1561–9. doi: 10.1038/jcbfm.2015.115
13. Shen Z, Jiang L, Yuan Y, Deng T, Zheng YR, Zhao YY, et al. Inhibition of G protein-coupled receptor 81 (GPR81) protects against ischemic brain injury. CNS Neurosci Ther. (2015) 21:271–9. doi: 10.1111/cns.12362
14. Berthet C, Lei H, Thevenet J, Gruetter R, Magistretti PJ, Hirt L. Neuroprotective role of lactate after cerebral ischemia. J Cereb Blood Flow Metab. (2009) 29:1780–9. doi: 10.1038/jcbfm.2009.97
15. Berthet C, Castillo X, Magistretti PJ, Hirt L. New evidence of neuroprotection by lactate after transient focal cerebral ischaemia: extended benefit after intracerebroventricular injection and efficacy of intravenous administration. Cerebrovasc Dis. (2012) 34:329–35. doi: 10.1159/000343657
16. Ferguson BS, Rogatzki MJ, Goodwin ML, Kane DA, Rightmire Z, Gladden LB. Lactate metabolism: historical context, prior misinterpretations, and current understanding. Eur J Appl Physiol. (2018) 118:691–728. doi: 10.1007/s00421-017-3795-6
17. Kondoh Y, Kawase M, Kawakami Y, Ohmori S. Concentrations of D-lactate and its related metabolic intermediates in liver, blood, and muscle of diabetic and starved rats. Res Exp Med. (1992) 192:407–14. doi: 10.1007/BF02576298
18. Ros J, Pecinska N, Beat Alessandri, Landolt H, Fillenz M. Lactate reduces glutamate-induced neurotoxicity in rat cortex. J Neurosci Res. (2001) 66:790–4. doi: 10.1002/jnr.10043
19. Bouzier-Sore AK, Voisin P, Canioni P, Magistretti PJ, Pellerin L. Lactate is a preferential oxidative energy substrate over glucose for neurons in culture. J Cereb Blood Flow Metab. (2003) 23:1298–306. doi: 10.1097/01.WCB.0000091761.61714.25
20. Pellerin L, Magistretti PJ. Sweet sixteen for ANLS. J Cereb Blood Flow Metab. (2012) 32:1152–66. doi: 10.1038/jcbfm.2011.149
21. Sobral-Monteiro-Junior R, Maillot P, Gatica-Rojas V, Avila WRM, de Paula AMB, Guimaraes ALS, et al. Is the “lactormone” a key-factor for exercise-related neuroplasticity? A hypothesis based on an alternative lactate neurobiological pathway. Med Hypotheses. (2019) 123:63–6. doi: 10.1016/j.mehy.2018.12.013
22. Brooks GA. Cell-cell and intracellular lactate shuttles. J Physiol. (2009) 587:5591–600. doi: 10.1113/jphysiol.2009.178350
23. Gibbs ME, Hertz L. Inhibition of astrocytic energy metabolism by d-lactate exposure impairs memory. Neurochem Int. (2008) 52:1012–8. doi: 10.1016/j.neuint.2007.10.014
24. Uribarri J, Oh MS, Carroll HJ. D-lactic acidosis. A review of clinical presentation, biochemical features, and pathophysiologic mechanism. Medicine. (1998) 77:73–82. doi: 10.1097/00005792-199803000-00001
25. Robergs RA, Ghiasvand F, Parker D. Biochemistry of exercise-induced metabolic acidosis. Am J Physiol Regul Integr Comp Physiol. (2004) 287:R502–16. doi: 10.1152/ajpregu.00114.2004
26. Magistretti PJ, Allaman I. A cellular perspective on brain energy metabolism and functional imaging. Neuron. (2015) 86:883–901. doi: 10.1016/j.neuron.2015.03.035
27. Newman LA, Korol DL, Gold PE. Lactate produced by glycogenolysis in astrocytes regulates memory processing. PLoS ONE. (2011) 6:e28427. doi: 10.1371/journal.pone.0028427
28. Sickmann HM, Walls AB, Schousboe A, Bouman SD, Waagepetersen HS. Functional significance of brain glycogen in sustaining glutamatergic neurotransmission. J Neurochem. (2009) 109(Suppl. 1):80–6. doi: 10.1111/j.1471-4159.2009.05915.x
29. Gold PE, Newman LA, Scavuzzo CJ, Korol DL. Modulation of multiple memory systems: from neurotransmitters to metabolic substrates. Hippocampus. (2013) 23:1053–65. doi: 10.1002/hipo.22182
30. Steinman MQ, Gao V, Alberini CM. The role of lactate-mediated metabolic coupling between astrocytes and neurons in long-term memory formation. Front Integr Neurosci. (2016) 10:10. doi: 10.3389/fnint.2016.00010
31. Matsui T, Omuro H, Liu YF, Soya M, Shima T, McEwen BS, et al. Astrocytic glycogen-derived lactate fuels the brain during exhaustive exercise to maintain endurance capacity. Proc Natl Acad Sci. (2017) 114:6358–63. doi: 10.1073/pnas.1702739114
32. Aveseh M, Nikooie R, Sheibani V, Esmaeili-Mahani S. Endurance training increases brain lactate uptake during hypoglycemia by up regulation of brain lactate transporters. Mol Cell Endocrinol. (2014) 394:29–36. doi: 10.1016/j.mce.2014.06.019
33. Masaki Takimoto TH. Acute exercise increases brain region-specific expression of MCT1, MCT2, MCT4, GLUT1, and COX IV proteins. J Appl Physiol. (2014) 116:1238–50. doi: 10.1152/japplphysiol.01288.2013
34. Hashimoto T, Tsukamoto H, Takenaka S, Olesen ND, Petersen LG, Sorensen H, et al. Maintained exercise-enhanced brain executive function related to cerebral lactate metabolism in men. Faseb J. (2018) 32:1417–27. doi: 10.1096/fj.201700381RR
35. van Hall G, Stromstad M, Rasmussen P, Jans O, Zaar M, Gam C, et al. Blood lactate is an important energy source for the human brain. J Cereb Blood Flow Metab. (2009) 29:1121–9. doi: 10.1038/jcbfm.2009.35
36. Tubbs PK. The metabolism of D-alpha-hydroxy acids in animal tissues. Ann N Y Acad Sci. (1965) 119:920–6. doi: 10.1111/j.1749-6632.1965.tb47452.x
37. Brooks GA. The science and translation of lactate shuttle theory. Cell Metab. (2018) 27:757–85. doi: 10.1016/j.cmet.2018.03.008
38. Adeva-Andany M, López-Ojén M, Funcasta-Calderón R, Ameneiros-Rodríguez E, Donapetry-García C, Vila-Altesor M, et al. Comprehensive review on lactate metabolism in human health. Mitochondrion. (2014) 17:76–100. doi: 10.1016/j.mito.2014.05.007
39. Finsterwald C, Magistretti PJ, Lengacher S. Astrocytes: new targets for the treatment of neurodegenerative diseases. Curr Pharm Des. (2015) 21:3570–81. doi: 10.2174/1381612821666150710144502
40. Pellerin L, Magistretti PJ. Glutamate uptake into astrocytes stimulates aerobic glycolysis: A mechanism coupling neuronal activity to glucose utilization. Proc Nat Acad Sci. (1994) 9:10625–9. doi: 10.1073/pnas.91.22.10625
41. Baltazar F, Afonso J, Costa M, Granja S. Lactate beyond a waste metabolite: metabolic affairs and signaling in malignancy. Front Oncol. (2020) 10:231. doi: 10.3389/fonc.2020.00231
42. Pierre K, Pellerin L, Debernardi R, Riederer BM, Magistretti PJ. Cell-specific localization of monocarboxylate transporters, MCT1 and MCT2, in the adult mouse brain revealed by double immunohistochemical. Neuroscience Vol. (2000) 100:617–27. doi: 10.1016/S0306-4522(00)00294-3
43. Gerhart DZ, Enerson BE, Zhdankina OY, Leino RL, Drewes LR. Expression of monocarboxylate transporter MCT1 by brain endothelium and glia in adult and suckling rats. Am J Physiol. (1997) 273:E207–213. doi: 10.1152/ajpendo.1997.273.1.E207
44. Hanu R, McKenna M, O'Neill A, Resneck WG, Bloch RJ. Monocarboxylic acid transporters, MCT1 and MCT2, in cortical astrocytes in vitro and in vivo. Am J Physiol Cell Physiol. (2000) 278:C921–30. doi: 10.1152/ajpcell.2000.278.5.C921
45. Rafiki A, Boulland JL, Halestrap AP, Ottersen OP, Bergersen L. Highly differential expression of the monocarboxylate transporters MCT2 and MCT4 in the developing rat brain. Neuroscience. (2003) 122:677–88. doi: 10.1016/j.neuroscience.2003.08.040
46. Bergersen LH. Is lactate food for neurons? Comparison of monocarboxylate transporter subtypes in brain and muscle. Neuroscience. (2007) 145:11–9. doi: 10.1016/j.neuroscience.2006.11.062
47. Dienel GA. The metabolic trinity, glucose–glycogen–lactate, links astrocytes and neurons in brain energetics, signaling, memory, and gene expression. Neurosci Lett. (2017) 637:18–25. doi: 10.1016/j.neulet.2015.02.052
48. Pierre K, Pellerin L. Monocarboxylate transporters in the central nervous system: distribution, regulation and function. J Neurochem. (2005) 94:1–14. doi: 10.1111/j.1471-4159.2005.03168.x
49. Bricker DK, Taylor EB, Schell JC, Orsak T, Boutron A, Chen YC, et al. A mitochondrial pyruvate carrier required for pyruvate uptake in yeast, Drosophila, and humans. Science. (2012) 337:96–100. doi: 10.1126/science.1218099
50. Thomas AP, Halestrap AP. Identification of the protein responsible for pyruvate transport into rat liver and heart mitochondria by specific labelling with [3H]N-phenylmaleimide. Biochem J. (1981) 196:471–9. doi: 10.1042/bj1960471
51. Brooks GA, Brown MA, Butz CE, Sicurello JP, Dubouchaud H. Cardiac and skeletal muscle mitochondria have a monocarboxylate transporter MCT1. J Appl Physiol (1985). (1999) 87:1713–8. doi: 10.1152/jappl.1999.87.5.1713
52. Butz CE, McClelland GB, Brooks GA. MCT1 confirmed in rat striated muscle mitochondria. J Appl Physiol(1985). (2004) 97:1059–66. doi: 10.1152/japplphysiol.00009.2004
53. Hashimoto T, Hussien R, Brooks GA. Colocalization of MCT1, CD147, and LDH in mitochondrial inner membrane of L6 muscle cells: evidence of a mitochondrial lactate oxidation complex. Am J Physiol Endocrinol Metab. (2006) 290:E1237–44. doi: 10.1152/ajpendo.00594.2005
54. Hashimoto T, Hussien R, Cho HS, Kaufer D, Brooks GA. Evidence for the mitochondrial lactate oxidation complex in rat neurons: demonstration of an essential component of brain lactate shuttles. PLoS ONE. (2008) 3:e2915. doi: 10.1371/journal.pone.0002915
55. Passarella S, de Bari L, Valenti D, Pizzuto R, Paventi G, Atlante A. Mitochondria and L-lactate metabolism. FEBS Lett. (2008) 582:3569–76. doi: 10.1016/j.febslet.2008.09.042
56. Stainsby WN, Brooks GA. Control of lactic acid metabolism in contracting muscles and during exercise. Exerc Sport Sci Rev. (1990) 18:29–63. doi: 10.1249/00003677-199001000-00005
57. Hashimoto T, Brooks GA. Mitochondrial lactate oxidation complex and an adaptive role for lactate production. Med Sci Sports Exerc. (2008) 40:486–94. doi: 10.1249/MSS.0b013e31815fcb04
58. Brooks GA. Intra- and extra-cellular lactate shuttles. Med Sci Sports Exerc. (2000) 32:790–9. doi: 10.1097/00005768-200004000-00011
59. Rogatzki MJ, Ferguson BS, Goodwin ML, Gladden LB. Lactate is always the end product of glycolysis. Front Neurosci. (2015) 9:22. doi: 10.3389/fnins.2015.00022
60. Lemire J, Mailloux RJ, Appanna VD. Mitochondrial lactate dehydrogenase is involved in oxidative-energy metabolism in human astrocytoma cells (CCF-STTG1). PLoS ONE. (2008) 3:e1550. doi: 10.1371/journal.pone.0001550
61. Atlante A, de Bari L, Bobba A, Marra E, Passarella S. Transport and metabolism of L-lactate occur in mitochondria from cerebellar granule cells and are modified in cells undergoing low potassium dependent apoptosis. Biochim Biophys Acta. (2007) 1767:1285–99. doi: 10.1016/j.bbabio.2007.08.003
62. Tekkök SB, Brown AM, Westenbroek R, Pellerin L, Ransom BR. Transfer of glycogen-derived lactate from astrocytes to axons via specific monocarboxylate transporters supports mouse optic nerve activity. J Neurosci Res. (2005) 81:644–52. doi: 10.1002/jnr.20573
63. Ling B, Peng F, Alcorn J, Lohmann K, Bandy B, Zello GA. D-Lactate altered mitochondrial energy production in rat brain and heart but not liver. Nutr Metab. (2012) 9:6. doi: 10.1186/1743-7075-9-6
64. Connor H, Woods HF, Ledingham JGG. Comparison of the kinetics and utilisation of D(-)-and L(+)-sodium lactate in normal man. Ann Nutr Metab. (1983) 27:481–7. doi: 10.1159/000176723
65. Jackson VN, Halestrap AP. The kinetics, substrate, and inhibitor specificity of the monocarboxylate (Lactate) transporter of rat liver cells determined using the fluorescent intracellular pH indicator, 2′,7′-Bis(carboxyethyl)-5(6)-carboxyfluorescein. J Biol Chem. (1996) 271:861–8. doi: 10.1074/jbc.271.2.861
66. Halestrap AP. The monocarboxylate transporter family–Structure and functional characterization. IUBMB Life. (2012) 64:1–9. doi: 10.1002/iub.573
67. Flick MJ, Konieczny SF. Identification of putative mammalian D-lactate dehydrogenase enzymes. Biochem Biophys Res Commun. (2002) 295:910–6. doi: 10.1016/S0006-291X(02)00768-4
68. Liu S. Practical implications of lactate and pyruvate metabolism by lactic acid bacteria in food and beverage fermentations. Int J Food Microbiol. (2003) 83:115–31. doi: 10.1016/S0168-1605(02)00366-5
69. Sousa MA, Rama GR, Volken de Souza CF, Granada CE. Acid lactic lactobacilli as a biotechnological toll to improve food quality and human health. Biotechnol Prog. (2020) 36:e2937. doi: 10.1002/btpr.2937
70. Reid SNS, Park JH, Kim Y, Kwak YS, Jeon BH. In vitro and in vivo effects of fermented oyster-derived lactate on exercise endurance indicators in mice. Int J Environ Res Public Health. (2020). 17:8811. doi: 10.3390/ijerph17238811
71. Teramae H, Yoshikawa T, Inoue R, Ushida K, Takebe K, Nio-Kobayashi J, et al. The cellular expression of SMCT2 and its comparison with other transporters for monocarboxylates in the mouse digestive tract. Biomed Res. (2010) 31:239–49. doi: 10.2220/biomedres.31.239
72. Brooks GA, Arevalo JA, Osmond AD, Leija RG, Curl CC, Tovar AP. Lactate in contemporary biology: a phoenix risen. J Physiol. (2022) 600:1229–51. doi: 10.1113/JP280955
73. Lecoultre V, Benoit R, Carrel G, Schutz Y, Millet GP, Tappy L, et al. Fructose and glucose co-ingestion during prolonged exercise increases lactate and glucose fluxes and oxidation compared with an equimolar intake of glucose. Am J Clin Nutr. (2010) 92:1071–9. doi: 10.3945/ajcn.2010.29566
74. Theytaz F, de Giorgi S, Hodson L, Stefanoni N, Rey V, Schneiter P, et al. Metabolic fate of fructose ingested with and without glucose in a mixed meal. Nutrients. (2014) 6:2632–49. doi: 10.3390/nu6072632
75. Brooks GA. The precious few grams of glucose during exercise. Int J Mol Sci. (2020) 21:5733. doi: 10.3390/ijms21165733
76. Brooks GA, Martin NA. Cerebral metabolism following traumatic brain injury: new discoveries with implications for treatment. Front Neurosci. (2014) 8:408. doi: 10.3389/fnins.2014.00408
77. Suzuki A, Stern SA, Bozdagi O, Huntley GW, Walker RH, Magistretti PJ, et al. Astrocyte-neuron lactate transport is required for long-term memory formation. Cell. (2011) 144:810–23. doi: 10.1016/j.cell.2011.02.018
78. Shima T, Matsui T, Jesmin S, Okamoto M, Soya M, Inoue K, et al. Moderate exercise ameliorates dysregulated hippocampal glycometabolism and memory function in a rat model of type 2 diabetes. Diabetologia. (2017) 60:597–606. doi: 10.1007/s00125-016-4164-4
79. Harris RA, Lone A, Lim H, Martinez F, Frame AK, Scholl TJ, et al. Aerobic glycolysis is required for spatial memory acquisition but not memory retrieval in mice. eNeuro. (2019). doi: 10.1523/ENEURO.0389-18.2019
80. Scavuzzo CJ, Rakotovao I, Dickson CT. Differential effects of L- and D-lactate on memory encoding and consolidation: Potential role of HCAR1 signaling. Neurobiol Learn Mem. (2020) 168:107151. doi: 10.1016/j.nlm.2019.107151
81. Zhang Y, Xue Y, Meng S, Luo Y, Liang J, Li J, et al. Inhibition of lactate transport erases drug memory and prevents drug relapse. Biol Psychiatry. (2016) 79:928–9. doi: 10.1016/j.biopsych.2015.07.007
82. Tadi M, Allaman I, Lengacher S, Grenningloh G, Magistretti PJ. Learning-induced gene expression in the hippocampus reveals a role of neuron -astrocyte metabolic coupling in long term memory. PLoS ONE. (2015) 10:e0141568. doi: 10.1371/journal.pone.0141568
83. Alberini CM, Cruz E, Descalzi G, Bessières B, Gao V. Astrocyte glycogen and lactate: New insights into learning and memory mechanisms. Glia. (2018) 66:1244–62. doi: 10.1002/glia.23250
84. Lucas SJ, Michel CB, Marra V, Smalley JL, Hennig MH, Graham BP, et al. Glucose and lactate as metabolic constraints on presynaptic transmission at an excitatory synapse. J Physiol. (2018) 596:1699–721. doi: 10.1113/JP275107
85. Tang F, Lane S, Korsak A, Paton JF, Gourine AV, Kasparov S, et al. Lactate-mediated glia-neuronal signalling in the mammalian brain. Nat Commun. (2014) 5:3284. doi: 10.1038/ncomms4284
86. Herrera-Lo'pez G, Griego E, Galva'n EJ. Lactate induces synapse-specific potentiation on CA3 pyramidal cells of rat hippocampus. PLoS ONE. (2020) 15:e0242309. doi: 10.1371/journal.pone.0242309
87. Schurr A, West CA, Rigor BM. Lactate-supported synaptic function in the rat hippocampal slice preparation. Science. (1988) 240: 1326–8. doi: 10.1126/science.3375817
88. Brown AM, Tekkök SB, Ransom BR. Glycogen regulation and functional role in mouse white matter. J Physiol. (2003) 549:501–12. doi: 10.1113/jphysiol.2003.042416
89. Margineanu MB, Mahmood H, Fiumelli H, Magistretti PJ. L-lactate regulates the expression of synaptic plasticity and neuroprotection genes in cortical neurons: a transcriptome analysis. Front Mol Neurosci. (2018) 11:375. doi: 10.3389/fnmol.2018.00375
90. Yang Y, Ruchti E, Petit JM, Jourdain P, Magistretti PJ. Lactate promotes plasticity gene expression by potentiating NMDA signaling in neurons. Proc Natl Acad Sci U S A. (2014) 111:12228–33. doi: 10.1073/pnas.1322912111
91. Baker KD, Edwards TM. D-Lactate inhibition of memory in a single trial discrimination avoidance task in the young chick. Neurobiol Learn Mem. (2007) 88:269–76. doi: 10.1016/j.nlm.2007.06.004
92. Ragozzino ME, Hellems K, Robert C, Lennartz Gold PE. Pyruvate infusions into the septal area attenuate spontaneous alternation impairments induced by intraseptal morphine injections. Behav Neurosci. (1995) 109:T074–1080. doi: 10.1037/0735-7044.109.6.1074
93. Jourdain P, Allaman I, Rothenfusser K, Fiumelli H, Marquet P, Magistretti PJ. L-Lactate protects neurons against excitotoxicity: implication of an ATP-mediated signaling cascade. Sci Rep. (2016) 6:21250. doi: 10.1038/srep21250
94. Ross JM, Oberg J, Brene S, Coppotelli G, Terzioglu M, Pernold K, et al. High brain lactate is a hallmark of aging and caused by a shift in the lactate dehydrogenase A/B ratio. Proc Natl Acad Sci U S A. (2010) 107:20087–92. doi: 10.1073/pnas.1008189107
95. langer MB, Allaman I, Magistretti PJ. Brain energy metabolism_ focus on astrocyte neuron metabolic cooperation. Cell Metab. (2011) 14:724–38. doi: 10.1016/j.cmet.2011.08.016
96. Dienel GA, McKenna MC. A dogma-breaking concept: glutamate oxidation in astrocytes is the source of lactate during aerobic glycolysis in resting subjects. J Neurochem. (2014) 131:395–8. doi: 10.1111/jnc.12835
97. Dienel GA. Brain lactate metabolism: the discoveries and the controversies. J Cereb Blood Flow Metab. (2011) 32:1107–38. doi: 10.1038/jcbfm.2011.175
98. Vafaee MS, Vang K, Bergersen LH, Gjedde A. Oxygen consumption and blood flow coupling in human motor cortex during intense finger tapping: implication for a role of lactate. J Cereb Blood Flow Metab. (2012) 32:1859–68. doi: 10.1038/jcbfm.2012.89
99. Nishijima T, Okamoto M, Matsui T, Kita I, Soya H. Hippocampal functional hyperemia mediated by NMDA receptor/NO signaling in rats during mild exercise. J Appl Physiol (1985). (2012) 54:197–203. doi: 10.1152/japplphysiol.00763.2011
100. Hein TW, Xu W, Kuo L. Dilation of retinal arterioles in response to lactate: role of nitric oxide, guanylyl cyclase, and ATP-sensitive potassium channels. Invest Ophthalmol Vis Sci. (2006) 47:693–9. doi: 10.1167/iovs.05-1224
101. Falkowska A, Gutowska I, Goschorska M, Nowacki P, Chlubek D, Baranowska-Bosiacka I. Energy metabolism of the brain, including the cooperation between astrocytes and neurons, especially in the context of glycogen metabolism. Int J Mol Sci. (2015) 16:25959–81. doi: 10.3390/ijms161125939
102. Yamanishi S, Katsumura K, Kobayashi T, Puro DG. Extracellular lactate as a dynamic vasoactive signal in the rat retinal microvasculature. Am J Physiol Heart Circ Physiol. (2006) 290:H925–934. doi: 10.1152/ajpheart.01012.2005
103. Wojtowicz JM, Kee N. BrdU assay for neurogenesis in rodents. Nat Protoc. (2006) 1:1399–405. doi: 10.1038/nprot.2006.224
104. Roger AJ, Munoz-Gomez SA, Kamikawa R. The origin and diversification of mitochondria. Curr Biol. (2017) 27:R1177–92. doi: 10.1016/j.cub.2017.09.015
105. Hashimoto T, Hussien R, Oommen S, Gohil K, Brooks GA. Lactate sensitive transcription factor network in L6 cells: activation of MCT1 and mitochondrial biogenesis. FASEB J. (2007) 21:2602–12. doi: 10.1096/fj.07-8174com
106. Tauffenberger A, Fiumelli H, Almustafa S, Magistretti PJ. Lactate and pyruvate promote oxidative stress resistance through hormetic ROS signaling. Cell Death Dis. (2019) 10:653. doi: 10.1038/s41419-019-1877-6
107. Hu J, Cai M, Shang Q, Li Z, Feng Y, Liu B, et al. Elevated lactate by high-intensity interval training regulates the hippocampal bdnf expression and the mitochondrial quality control system. Front Physiol. (2021) 12:629914. doi: 10.3389/fphys.2021.629914
108. Lauritzen F, Eid T, Bergersen LH. Monocarboxylate transporters in temporal lobe epilepsy: roles of lactate and ketogenic diet. Brain Struct Funct. (2015) 220:1–12. doi: 10.1007/s00429-013-0672-x
109. Zhai X, Li J, Li L, Sun Y, Zhang X, Xue Y, et al. L-lactate preconditioning promotes plasticity-related proteins expression and reduces neurological deficits by potentiating GPR81 signaling in rat traumatic brain injury model. Brain Res. (2020) 1746:146945. doi: 10.1016/j.brainres.2020.146945
110. Bouzat P, Sala N, Suys T, Zerlauth JB, Marques-Vidal P, Feihl F, et al. Cerebral metabolic effects of exogenous lactate supplementation on the injured human brain. Intensive Care Med. (2014) 40:412–21. doi: 10.1007/s00134-013-3203-6
111. Alessandri B, Schwandt E, Kamada Y, Nagata M, Heimann A, Kempski O. The neuroprotective effect of lactate is not due to improved glutamate uptake after controlled cortical impact in rats. J Neurotrauma. (2012) 29:2181–91. doi: 10.1089/neu.2011.2067
112. Dienel GA. Lactate shuttling and lactate use as fuel after traumatic brain injury: metabolic considerations. J Cereb Blood Flow Metab. (2014) 34:1736–48. doi: 10.1038/jcbfm.2014.153
113. Glenn TC, Martin NA, Horning MA, McArthur DL, Hovda DA, Vespa P, et al. Lactate: brain fuel in human traumatic brain injury: a comparison with normal healthy control subjects. J Neurotrauma. (2015) 32:820–32. doi: 10.1089/neu.2014.3483
114. Glenn TC, Martin NA, McArthur DL, Hovda DA, Vespa P, Johnson ML, et al. Endogenous nutritive support after traumatic brain injury: peripheral lactate production for glucose supply via gluconeogenesis. J Neurotrauma. (2015) 32:811–19. doi: 10.1089/neu.2014.3482
115. Ma Y, Liu T, Fu J, Fu S, Hu C, Sun B, et al. Lactobacillus acidophilus exerts neuroprotective effects in mice with traumatic brain injury. J Nutr. (2019) 149:1543–52. doi: 10.1093/jn/nxz105
116. Li H, Sun J, Du J, Wang F, Fang R, Yu C, et al. Clostridium butyricum exerts a neuroprotective effect in a mouse model of traumatic brain injury via the gut-brain axis. Neurogastroenterol Motil. (2018) 30:e13260. doi: 10.1111/nmo.13260
117. Mijnhout GS, Scheltens P, Diamant M, Biessels GJ, Wessels AM, Simsek S, et al. Diabetic encephalopathy: a concept in need of a definition. Diabetologia. (2006) 49:1447–8. doi: 10.1007/s00125-006-0221-8
118. McCrimmon RJ, Ryan CM, Frier BM. Diabetes and cognitive dysfunction. Lancet. (2012) 379:2291–9. doi: 10.1016/S0140-6736(12)60360-2
119. Biessels GJ, van der Heide LP, Kamal A, Bleys RL, Gispen WH. Ageing and diabetes implications for brain function. Eur J Pharmacol. (2002) 441:1–14. doi: 10.1016/S0014-2999(02)01486-3
120. Yi SS. Effects of exercise on brain functions in diabetic animal models. World J Diabetes. (2015) 6:583–97. doi: 10.4239/wjd.v6.i4.583
121. Fazeli SA. Neuroprotection in diabetic encephalopathy. Neurodegener Dis. (2009) 6:213–8. doi: 10.1159/000278694
122. Wiegers EC, Rooijackers HM, Tack CJ, Heerschap A, de Galan BE, van der Graaf M. Brain lactate concentration falls in response to hypoglycemia in patients with type 1 diabetes and impaired awareness of hypoglycemia. Diabetes. (2016) 65:1601–5. doi: 10.2337/db16-0068
123. Wiegers EC, Rooijackers HM, Tack2 CJ, Groenewoud HJMM, Heerschap A, Galan BEd, et al. Effect of exercise-induced lactate elevation on brain lactate levels during hypoglycemia in patients with type 1 diabetes and impaired awareness of hypoglycemia. Diabetes. (2017) 66:3105–10. doi: 10.2337/db17-0794
124. King P, Kong MF, Parkin H, MacDonald IA, Barber C, Tattersall RB. Intravenous lactate prevents cerebral dysfunction during hypoglycaemia in insulin-dependent diabetes mellitus. Clin Sci. (1998) 94:157–63. doi: 10.1042/cs0940157
125. Maran A, Crepaldi C, Trupiani S, Lucca T, Jori E, Macdonald IA, et al. Brain function rescue effect of lactate following hypoglycaemia is not an adaptation process in both normal and type I diabetic subjects. Diabetologia. (2000) 43:733–41. doi: 10.1007/s001250051371
126. Wiegers EC, Rooijackers HM, Tack CJ, Philips BW, Heerschap A, van der Graaf M, et al. Effect of lactate administration on brain lactate levels during hypoglycemia in patients with type 1 diabetes. J Cereb Blood Flow Metab. (2019) 39:1974–82. doi: 10.1177/0271678X18775884
127. Chan O, Paranjape S, Horblitt A, Zhu W, Sherwin RS. Lactate-induced release of GABA in the ventromedial hypothalamus contributes to counterregulatory failure in recurrent hypoglycemia and diabetes. Diabetes. (2013) 62:4239–46. doi: 10.2337/db13-0770
128. Kobayashi R, Maruoka J, Norimoto H, Ikegaya Y, Kume K, Ohsawa M. Involvement of l-lactate in hippocampal dysfunction of type I diabetes. J Pharmacol Sci. (2019) 141:79–82. doi: 10.1016/j.jphs.2019.09.004
129. Mason GF, Petersen KF, Lebon V, Rothman DL, Shulman GI. increased brain monocarboxylic acid transport and utilization in type 1 diabetes. Diabetes. (2006) 55:929–34. doi: 10.2337/diabetes.55.04.06.db05-1325
130. Hackett MJ, Hollings A, Majimbi M, Brook E, Cochran B, Giles C, et al. Multimodal imaging analyses of brain hippocampal formation reveal reduced Cu and lipid content and increased lactate content in non-insulin-dependent diabetic mice. ACS Chem Neurosci. (2019) 10:2533–40. doi: 10.1021/acschemneuro.9b00039
131. Li J, Liu B, Cai M, Lin X, Lou S. Glucose metabolic alterations in hippocampus of diabetes mellitus rats and the regulation of aerobic exercise. Behav Brain Res. (2019) 364:447–56. doi: 10.1016/j.bbr.2017.11.001
132. Choi Y-S, Song JE, Lee JE, Kim E, Kim CH, Kim D-H, et al. Hyperpolarized [1-13C] lactate flux increased in the hippocampal region in diabetic mice. Molecular Brain. (2019) 12:88. doi: 10.1186/s13041-019-0505-9
133. De Feyter HM, Mason GF, Shulman GI, Rothman DL, Petersen KF. Increased brain lactate concentrations without increased lactate oxidation during hypoglycemia in type 1 diabetic individuals. Diabetes. (2013) 62:3075–80. doi: 10.2337/db13-0313
134. Zhao L, Dong M, Ren M, Li C, Zheng H, Gao H. Metabolomic analysis identifies lactate as an important pathogenic factor in diabetes-associated cognitive decline rats. Mol Cell Proteomics. (2018) 17:2335–46. doi: 10.1074/mcp.RA118.000690
135. Wang D, Zhao L, Zheng H, Dong M, Pan L, Zhang X, et al. Time-dependent lactate production and amino acid utilization in cultured astrocytes under high glucose exposure. Mol Neurobiol. (2018) 55:1112. doi: 10.1007/s12035-016-0360-y
136. Tapeinos C, Battaglini M, Ciofani G. Advances in the design of solid lipid nanoparticles and nanostructured lipid carriers for targeting brain diseases. J Control Release. (2017) 264:306–32. doi: 10.1016/j.jconrel.2017.08.033
137. Yin F, Sancheti H, Patil I, Cadenas E. Energy metabolism and inflammation in brain aging and Alzheimer's disease. Free Radic Biol Med. (2016) 100:108–22. doi: 10.1016/j.freeradbiomed.2016.04.200
138. Lu WT, Sun SQ, Li Y, Xu SY, Gan SW, Xu J, et al. Curcumin ameliorates memory deficits by enhancing lactate content and MCT2 expression in APP/PS1 transgenic mouse model of Alzheimer's disease. Anat Rec. (2019) 302:332–8. doi: 10.1002/ar.23969
139. Lu W, Huang J, Sun S, Huang S, Gan S, Xu J, et al. Changes in lactate content and monocarboxylate transporter 2 expression in Abeta(2)(5)(-)(3)(5)-treated rat model of Alzheimer's disease. Neurol Sci. (2015) 36:871–6. doi: 10.1007/s10072-015-2087-3
140. Zhang M, Cheng X, Dang R, Zhang W, Zhang J, Yao Z. Lactate deficit in an alzheimer disease mouse model: the relationship with neuronal damage. J Neuropathol Exp Neurol. (2018) 77:1163–76. doi: 10.1093/jnen/nly102
141. Datta S, Chakrabarti N. Age related rise in lactate and its correlation with lactate dehydrogenase (LDH) status in post-mitochondrial fractions isolated from different regions of brain in mice. Neurochem Int. (2018) 118:23–33. doi: 10.1016/j.neuint.2018.04.007
142. Long DM, Frame AK, Reardon PN, Cumming RC, Hendrix DA, Kretzschmar D, et al. Lactate dehydrogenase expression modulates longevity and neurodegeneration in Drosophila melanogaster. Aging. (2020) 12:10041–58. doi: 10.18632/aging.103373
143. Mullins R, Reiter D, Kapogiannis D. Magnetic resonance spectroscopy reveals abnormalities of glucose metabolism in the Alzheimer's brain. Ann Clin Transl Neurol. (2018) 5:262–72. doi: 10.1002/acn3.530
144. de Bari L, Atlante A, Guaragnella N, Principato G, Passarella S. D-Lactate transport and metabolism in rat liver mitochondria. Biochem J. (2002) 365:391–403. doi: 10.1042/bj20020139
145. de Bari L, Atlante A, Armeni T, Kalapos MP. Synthesis and metabolism of methylglyoxal, S-D-lactoylglutathione and D-lactate in cancer and Alzheimer's disease. Exploring the crossroad of eternal youth and premature aging. Ageing Res Rev. (2019) 53:100915. doi: 10.1016/j.arr.2019.100915
146. Maida CD, Norrito RL, Daidone M, Tuttolomondo A, Pinto A. Neuroinflammatory mechanisms in ischemic stroke: focus on cardioembolic stroke, background, and therapeutic approaches. Int J Mol Sci. (2020) 21:6454. doi: 10.3390/ijms21186454
147. Hyacinthe JN, Buscemi L, Le TP, Lepore M, Hirt L, Mishkovsky M. Evaluating the potential of hyperpolarised [1-(13)C] L-lactate as a neuroprotectant metabolic biosensor for stroke. Sci Rep. (2020) 10:5507. doi: 10.1038/s41598-020-62319-x
148. Wyss MT, Jolivet R, Buck A, Magistretti PJ, Weber B. In vivo evidence for lactate as a neuronal energy source. J Neurosci. (2011) 31:7477–85. doi: 10.1523/JNEUROSCI.0415-11.2011
149. Schurr A, Payne RS, Miller JJ, Tseng MT, Rigor BM. Rigor. Blockade of lactate transport exacerbates delayed neuronal damage in__a rat model of cerebral ischemia. Brain Res. (2001) 895:268–72. doi: 10.1016/S0006-8993(01)02082-0
150. Buscemi L, Blochet C, Magistretti PJ, Hirt L. Hydroxycarboxylic acid receptor 1 and neuroprotection in a mouse model of cerebral ischemia-reperfusion. Front Physiol. (2021) 12:689239. doi: 10.3389/fphys.2021.689239
151. Moro N, Ghavim S, Harris NG, Hovda DA, Sutton RL. Glucose administration after traumatic brain injury improves cerebral metabolism and reduces secondary neuronal injury. Brain Res. (2013) 1535:124–36. doi: 10.1016/j.brainres.2013.08.044
152. Bergsneider M, Hovda DA, Shalmon E, Kelly DF, Vespa PM, Martin NA, et al. Cerebral hyperglycolysis following severe traumatic brain injury in humans: a positron emission tomography study. J Neurosurg. (1997) 86:241–51. doi: 10.3171/jns.1997.86.2.0241
153. Glenn TC, Kelly DF, Boscardin WJ, McArthur DL, Vespa P, Oertel M, et al. Energy dysfunction as a predictor of outcome after moderate or severe head injury: indices of oxygen, glucose, and lactate metabolism. J Cereb Blood Flow Metab. (2003) 23:1239–50. doi: 10.1097/01.WCB.0000089833.23606.7F
154. Jalloh I, Helmy A, Shannon RJ, Gallagher CN, Menon DK, Carpenter KL, et al. Lactate uptake by the injured human brain: evidence from an arteriovenous gradient and cerebral microdialysis study. J Neurotrauma. (2013) 30:2031–7. doi: 10.1089/neu.2013.2947
155. Carteron L, Solari D, Patet C, Quintard H, Miroz JP, Bloch J, et al. Hypertonic lactate to improve cerebral perfusion and glucose availability after acute brain injury. Crit Care Med. (2018) 46:1649–55. doi: 10.1097/CCM.0000000000003274
156. Zhou J, Burns MP, Huynh L, Villapol S, Taub DD, Saavedra JM, et al. Temporal changes in cortical and hippocampal expression of genes important for brain glucose metabolism following controlled cortical impact injury in mice. Front Endocrinol. (2017) 8:231. doi: 10.3389/fendo.2017.00231
157. Betjemann JP, Lowenstein DH. Status epilepticus in adults. Lancet Neurol. (2015) 14:615–24. doi: 10.1016/S1474-4422(15)00042-3
158. Thoresen M, Hallstrom A, Whitelaw A, Puka-Sundvall M, Loberg EM, Satas S, et al. Lactate and pyruvate changes in the cerebral gray and white matter during posthypoxic seizures in newborn pigs. Pediatr Res. (1998) 44:746–54. doi: 10.1203/00006450-199811000-00018
159. Mariani CL, Nye CJ, Ruterbories L, Tokarz DA, Green L, Lau J, et al. Cerebrospinal fluid lactate concentrations in dogs with seizure disorders. J Vet Intern Med. (2020) 34:2562–70. doi: 10.1111/jvim.15953
160. Slais K, Vorisek I, Zoremba N, Homola A, Dmytrenko L, Sykova E. Brain metabolism and diffusion in the rat cerebral cortex during pilocarpine-induced status epilepticus. Exp Neurol. (2008) 209:145–54. doi: 10.1016/j.expneurol.2007.09.008
161. During MJ, Fried I, Leone P, Katz A, Spencer DD. Direct measurement of extracellular lactate in the human hippocampus during spontaneous seizures. J Neurochem. (1994) 62:2356–61. doi: 10.1046/j.1471-4159.1994.62062356.x
162. Cavus I, Kasoff WS, Cassaday MP, Jacob R, Gueorguieva R, Sherwin RS, et al. Extracellular metabolites in the cortex and hippocampus of epileptic patients. Ann Neurol. (2005) 57:226–35. doi: 10.1002/ana.20380
163. Jorwal P, Sikdar SK. Lactate reduces epileptiform activity through HCA1 and GIRK channel activation in rat subicular neurons in an in vitro model. Epilepsia. (2019) 60:2370–85. doi: 10.1111/epi.16389
164. Matsui T, Soya S, Okamoto M, Ichitani Y, Kawanaka K, Soya H. Brain glycogen decreases during prolonged exercise. J Physiol. (2011) 589:3383–93. doi: 10.1113/jphysiol.2011.203570
165. Matsui T, Soya S, Kawanaka K, Soya H. Brain glycogen decreases during intense exercise without hypoglycemia: the possible involvement of serotonin. Neurochem Res. (2015) 40:1333–40. doi: 10.1007/s11064-015-1594-1
166. Bouzier AK, Thiaudiere E, Biran M, Rouland R, Canioni P, Merle M. The metabolism of [3-(13)C]lactate in the rat brain is specific of a pyruvate carboxylase-deprived compartment. J Neurochem. (2000) 75:480–6. doi: 10.1046/j.1471-4159.2000.0750480.x
167. Schurr A, Miller JJ, Payne RS, Rigor BM. An increase in lactate output by brain tissue serves to meet the energy needs of glutamateactivated neurons. J Neurosci. (1999) 19:34–9. doi: 10.1523/JNEUROSCI.19-01-00034.1999
168. Genc S, Kurnaz IA, Ozilgen M. Astrocyte-neuron lactate shuttle may boost more ATP supply to the neuron under hypoxic conditions–in silico study supported by in vitro expression data. BMC Syst Biol. (2011) 5:162. doi: 10.1186/1752-0509-5-162
169. Lauritzen KH, Morland C, Puchades M, Holm-Hansen S, Hagelin EM, Lauritzen F, et al. Lactate receptor sites link neurotransmission, neurovascular coupling, and brain energy metabolism. Cereb Cortex. (2014) 24:2784–95. doi: 10.1093/cercor/bht136
170. Magistretti PJ, Allaman I. Lactate in the brain: from metabolic end-product to signalling molecule. Nat Rev Neurosci. (2018) 19:235–49. doi: 10.1038/nrn.2018.19
171. Brooks GA. Lactate as a fulcrum of metabolism. Redox Biol. (2020) 35:101454. doi: 10.1016/j.redox.2020.101454
172. Fernandez-Marcos PJ, Auwerx J. Regulation of PGC-1α, a nodal regulator of mitochondrial biogenesis. Am J Clin Nutr. (2011) 93:884S−90S. doi: 10.3945/ajcn.110.001917
173. Gureev AP, Shaforostova EA, Popov VN. Regulation of mitochondrial biogenesis as a way for active longevity: interaction between the Nrf2 and PGC-1alpha signaling pathways. Front Genet. (2019) 10:435. doi: 10.3389/fgene.2019.00435
174. Galardo MaN, Regueira M, Riera MaF, Pellizzari EH, Cigorraga SB, Meroni SB. Lactate regulates rat male germ cell function through reactive oxygen species. PLoS ONE. (2014) 9:e88024. doi: 10.1371/journal.pone.0088024
175. Zelenka J, Dvorak A, Alan L. L-lactate protects skin fibroblasts against aging-associated mitochondrial dysfunction via mitohormesis. Oxid Med Cell Longev. (2015) 2015:351698. doi: 10.1155/2015/351698
176. Bari LD, Valenti D, Atlante A, Passarella S. L-Lactate generates hydrogen peroxide in purified rat liver mitochondria due to the putative L-lactate oxidase localized in the intermembrane space. FEBS Letters. (2010) 584:2285–90. doi: 10.1016/j.febslet.2010.03.038
177. Ge H, Weiszmann J, Reagan JD, Gupte J, Baribault H, Gyuris T, et al. Elucidation of signaling and functional activities of an orphan GPCR, GPR81. J Lipid Res. (2008) 49:797–803. doi: 10.1194/jlr.M700513-JLR200
178. Ahmed K, Tunaru S, Tang C, Muller M, Gille A, Sassmann A, et al. An autocrine lactate loop mediates insulin-dependent inhibition of lipolysis through GPR81. Cell Metab. (2010) 11:311–19. doi: 10.1016/j.cmet.2010.02.012
179. Brooks GA. Role of the heart in lactate shuttling. Front Nutr. (2021) 8:663560. doi: 10.3389/fnut.2021.663560
180. Li G, Wang HQ, Wang LH, Chen RP, Liu JP. Distinct pathways of ERK1/2 activation by hydroxycarboxylic acid receptor-1. PLoS ONE. (2014) 9:e93041. doi: 10.1371/journal.pone.0093041
181. Ohno Y, Oyama A, Kaneko H, Egawa T, Yokoyama S, Sugiura T, et al. Lactate increases myotube diameter via activation of MEK/ERK pathway in C2C12 cells. Acta Physiol. (2018) 223:e13042. doi: 10.1111/apha.13042
182. Wu Y, Wang M, Zhang K, Li Y, Xu M, Tang S, et al. Lactate enhanced the effect of parathyroid hormone on osteoblast differentiation via GPR81-PKC-Akt signaling. Biochem Biophys Res Commun. (2018) 503:737–43. doi: 10.1016/j.bbrc.2018.06.069
183. Hoque R, Farooq A, Ghani A, Gorelick F, Mehal WZ. Lactate reduces liver and pancreatic injury in Toll-like receptor- and inflammasome-mediated inflammation via GPR81-mediated suppression of innate immunity. Gastroenterology. (2014) 146:1763–74. doi: 10.1053/j.gastro.2014.03.014
184. Harun-Or-Rashid M, Inman DM. Reduced AMPK activation and increased HCAR activation drive anti-inflammatory response and neuroprotection in glaucoma. J Neuroinflammation. (2018) 15:313. doi: 10.1186/s12974-018-1346-7
185. Jeninga EH, Bugge A, Nielsen R, Kersten S, Hamers N, Dani C, et al. Peroxisome proliferator-activated receptor gamma regulates expression of the anti-lipolytic G-protein-coupled receptor 81 (GPR81/Gpr81). J Biol Chem. (2009) 284:26385–93. doi: 10.1074/jbc.M109.040741
186. Wanders D, Graff EC, Judd RL. Effects of high fat diet on GPR109A and GPR81 gene expression. Biochem Biophys Res Commun. (2012) 425:278–83. doi: 10.1016/j.bbrc.2012.07.082
187. Madaan A, Chaudhari P, Nadeau-Vallée M, Hamel D, Zhu T, Mitchell G, et al. Müller cell–localized G-protein–coupled receptor 81 (hydroxycarboxylic acid receptor 1) regulates inner retinal vasculature via Norrin/Wnt pathways. Am J Pathol. (2019) 189:1878–96. doi: 10.1016/j.ajpath.2019.05.016
188. Lee Y-S, Kim T-Y, Kim Y, Lee S-H, Kim S, Kang SW, et al. Microbiota-derived lactate accelerates intestinal stem-cell-mediated epithelial development. Cell Host Microbe. (2018) 24:833–46.e6. doi: 10.1016/j.chom.2018.11.002
189. Cai TQ, Ren N, Jin L, Cheng K, Kash S, Chen R, et al. Role of GPR81 in lactate-mediated reduction of adipose lipolysis. Biochem Biophys Res Commun. (2008) 377:987–91. doi: 10.1016/j.bbrc.2008.10.088
190. Liu C, Wu J, Zhu J, Kuei C, Yu J, Shelton J, et al. Lactate inhibits lipolysis in fat cells through activation of an orphan G-protein-coupled receptor, GPR81. J Biol Chem. (2009) 284:2811–22. doi: 10.1074/jbc.M806409200
191. Alarcon P, Manosalva C, Carretta MD, Hidalgo AI, Figueroa CD, Taubert A, et al. Fatty and hydroxycarboxylic acid receptors: The missing link of immune response and metabolism in cattle. Vet Immunol Immunopathol. (2018) 201:77–87. doi: 10.1016/j.vetimm.2018.05.009
192. Morland C, Lauritzen KH, Puchades M, Holm-Hansen S, Andersson K, Gjedde A, et al. The lactate receptor, G-protein-coupled receptor 81/hydroxycarboxylic acid receptor 1: expression and action in brain. J. Neurosci Res. (2015) 93:1045–55. doi: 10.1002/jnr.23593
193. Huckabee WE. Relationship of pyruvate and lactate during anaerobic metabolism. V coronary adequacy. Am J Physiol Physiol. (1961) 200:1169. doi: 10.1152/ajplegacy.1961.200.6.1169
194. Osnes JB, Hermansen L. Acid-base balance after maximal exercise of short duration. J Appl Physiol. (1972) 32:59–63. doi: 10.1152/jappl.1972.32.1.59
195. Kreisberg RA. Lactate homeostasis and lactic acidosis. Ann Intern Med. (1980) 92:227. doi: 10.7326/0003-4819-92-2-227
196. Vardjan N, Chowdhury HH, Horvat A, Velebit J, Malnar M, Muhic M, et al. Enhancement of astroglial aerobic glycolysis by extracellular lactate-mediated increase in cAMP. Front Mol Neurosci. (2018) 11:148. doi: 10.3389/fnmol.2018.00148
197. Martin SJ, Grimwood PD, Morris RG. Synaptic plasticity and memory: an evaluation of the hypothesis. Annu Rev Neurosci. (2000) 23:649–711. doi: 10.1146/annurev.neuro.23.1.649
Keywords: lactate, brain function, brain diseases, energy substrates, signal molecule
Citation: Cai M, Wang H, Song H, Yang R, Wang L, Xue X, Sun W and Hu J (2022) Lactate Is Answerable for Brain Function and Treating Brain Diseases: Energy Substrates and Signal Molecule. Front. Nutr. 9:800901. doi: 10.3389/fnut.2022.800901
Received: 24 October 2021; Accepted: 18 March 2022;
Published: 28 April 2022.
Edited by:
Andrew Scholey, Swinburne University of Technology, AustraliaReviewed by:
Romana Stark, Monash University, AustraliaGeorge A. Brooks, University of California, Berkeley, United States
Copyright © 2022 Cai, Wang, Song, Yang, Wang, Xue, Sun and Hu. This is an open-access article distributed under the terms of the Creative Commons Attribution License (CC BY). The use, distribution or reproduction in other forums is permitted, provided the original author(s) and the copyright owner(s) are credited and that the original publication in this journal is cited, in accordance with accepted academic practice. No use, distribution or reproduction is permitted which does not comply with these terms.
*Correspondence: Wanju Sun, sunwanju2021@163.com; Jingyun Hu, jingyunhu121@126.com
†These authors have contributed equally to this work and share first authorship