Lasting mesothalamic dopamine imbalance and altered exploratory behavior in rats after a mild neonatal hypoxic event
- 1Department of Biology, University of Zagreb Faculty of Science, Zagreb, Croatia
- 2Croatian Institute for Brain Research, University of Zagreb School of Medicine, Zagreb, Croatia
- 3Department of Biology, University of Zagreb School of Medicine, Zagreb, Croatia
- 4Department for Anatomy and Clinical Anatomy, University of Zagreb School of Medicine, Zagreb, Croatia
Introduction: Adversities during the perinatal period can decrease oxygen supply to the fetal brain, leading to various hypoxic brain injuries, which can compromise the regularity of brain development in different aspects. To examine the catecholaminergic contribution to the link between an early-life hypoxic insult and adolescent behavioral aberrations, we used a previously established rat model of perinatal hypoxia but altered the hypobaric to normobaric conditions.
Methods: Exploratory and social behavior and learning abilities were tested in 70 rats of both sexes at adolescent age. Inherent vertical locomotion, sensory-motor functions and spatial learning abilities were explored in a subset of animals to clarify the background of altered exploratory behavior. Finally, the concentrations of dopamine (DA) and noradrenaline in midbrain and pons, and the relative expression of genes for DA receptors D1 and D2, and their down-stream targets (DA- and cAMP-regulated phosphoprotein, Mr 32 kDa, the regulatory subunit of protein kinase A, and inhibitor-5 of protein phosphatase 1) in the hippocampus and thalamus were investigated in 31 rats.
Results: A lesser extent of alterations in exploratory and cognitive aspects of behavior in the present study suggests that normobaric conditions mitigate the hypoxic injury compared to the one obtained under hypobaric conditions. Increased exploratory rearing was the most prominent consequence, with impaired spatial learning in the background. In affected rats, increased midbrain/pons DA content, as well as mRNA levels for DA receptors and their down-stream elements in the thalamus, but not the hippocampus, were found.
Conclusion: We can conclude that a mild hypoxic event induced long-lasting disbalances in mesothalamic DA signaling, contributing to the observed behavioral alterations. The thalamus was thereby indicated as another structure, besides the well-established striatum, involved in mediating hypoxic effects on behavior through DA signaling.
1 Introduction
There has been growing evidence that multiple epigenetic factors during gestation and the perinatal period modulate brain development, shaping individual susceptibility to various neuropsychiatric disorders later in life (Faa et al., 2014). Changes in the maternal environment or placental dysfunction may lead to lowered fetal oxygen supply, often combined with reduced blood supply, and seriously affect the developing neuronal tissue, leading to a hypoxic brain injury (HBI; Frajewicki et al., 2020; Piešová and Mach, 2020). Severe HBI, induced by perinatal hypoxia-ischemia, could result in the infant’s death or permanent neurologic deficits, such as motor disabilities, seizures, impaired muscle tone, and epilepsy (Leviton and Nelson, 1992; Pin et al., 2009). On the other hand, moderate or mild HBI may pass unnoticed at birth but could still affect normal brain development and manifest later in childhood or adolescence as cognitive problems or behavioral disorders (Gonzalez and Miller, 2006; Nalivaeva et al., 2018).
To unravel events between an early life insult and development of behavioral symptoms later in life, and to discover the underlying mechanisms of HBI, various animal models have been employed. The hypoxia-ischemia (HI) models, which combine surgically induced ischemia with exposure to a hypoxic condition, anatomically and functionally correspond to the more severe HBI in humans and are mainly used for modeling neurological disorders. The “hypoxia-only” (H) models employ acute or chronic exposure to hypoxic conditions that do not induce necrosis, correspond to a mild hypoxic injury in human fetuses and prematurely born babies, and are suitable for exploring biochemical, molecular, and structural events, leading to psychiatric/behavioral disorders (Millar et al., 2017; Hefter et al., 2018).
The exploratory behavior of a rodent in a novel environment is based on an evolutionary balance between a need to secure resources and a need to minimize the risk of predation (Thompson et al., 2018). Rearing is an essential aspect of exploratory behavior that combines sensory perception, spatial memory acquisition, and vigilance. There are two types of rearing—supported (wall-leaning) and unsupported (brief rise on hind legs), both considered to be valuable tools for collecting information about the novel space (Lever et al., 2006). Unsupported rearing provides an animal with visual information needed for spatial mapping, while supported rearing, through somatosensory perception, enables the acquisition of information about environmental boundaries (Crusio, 2001; Lever et al., 2006; Sturman et al., 2018).
The thalamus seems to bridge sensory perception and cognition with an aim to identify environmental signals bearing current behavioral relevance (Wolff et al., 2021). Some thalamic nuclei convey sensory information from the site of reception to the area of perception, playing an important role in their filtering and organization (Sherman, 2005). Other thalamic nuclei send projections to the cingulate cortex and hippocampal formation (Shibata, 1993; van Groen and Wyss, 1995; Aggleton et al., 2010) and seem to be involved in spatial learning and memory (Aggleton et al., 1991; Savage et al., 1998; van Groen et al., 2002). The thalamic relay function can be modulated depending on behavioral demands, and important modulators are the brainstem monoaminergic neurotransmitters, including dopamine (DA; Venkatraman et al., 2017).
The DA system is highly conserved among vertebrates, bearing a crucial role in the adaptation of animal behavior (Yamamoto and Vernier, 2011). The primary source of DA in the brain are the midbrain DA neurons, which send projections to various parts of the forebrain through the three main dopaminergic pathways—nigrostriatal, mesolimbic, and mesoprefrontal (Islam et al., 2021). In primates, dopaminergic afferents also project from the ventral tegmental area (VTA), periaqueductal gray, and the lateral parabrachial nucleus broadly across the thalamus (Sánchez-González et al., 2005). To a lesser extent, dopaminergic innervation of the thalamus (Papadopoulos and Parnavelas, 1990; García-Cabezas et al., 2009; Varela, 2014) as well as the presence of dopamine receptor 1 (D1; Fremeau et al., 1991; Huang et al., 1992) and dopamine receptor 2 (D2) (Khan et al., 1998; Clark et al., 2017) have also been demonstrated in rats. The binding of DA to the D1 receptor activates (via stimulatory G-protein) the effector adenylate-cyclase, raising cAMP levels and activating protein kinase A (PKA). PKA then phosphorylates two proteins: dopamine- and cAMP-regulated phosphoprotein Mr. 32 kDa (DARPP-32) and inhibitor-5 of protein phosphatase 1 (IPP5), thereby turning them into potent inhibitors of serine/threonine protein phosphatase (PP-1), which plays a significant role in dephosphorylation of eukaryotic cell proteins (Walaas et al., 2011). The binding of DA to the D2 receptor elicits the opposite effect, inhibiting the PKA/DARPP-32/PP-1 signaling.
Expression of DA receptors occurs early in development and plays an important role in shaping neuronal cytoarchitecture. During this period, various disruptors can alter DA signaling, affecting brain structure and connectivity (Money and Stanwood, 2013). Post-mortem analysis of human neonates indicated a vulnerability of mesencephalic DA neurons to prolonged neonatal hypoxia (Pagida et al., 2013), while the study on a rodent model showed that perinatal hypoxia affected early differentiation of mesencephalic DA neurons and postnatal brain DA terminal architecture (Brandon et al., 2022). These findings indicate DA dysfunction as one of the consequences of perinatal HBI that can later lead to the development of DA-related behavioral and/or cognitive deficits in infant survivors (Giannopoulou et al., 2018).
In H models, hypoxia is achieved by placing an animal in a chamber with either decreased barometric pressure (hypobaric hypoxia, HH) or reduced oxygen fraction (normobaric hypoxia, NH). Although both procedures decrease partial oxygen pressure, physiological studies on adult animals and humans revealed a more intense reaction to HH than to NH, concerning generalized hypoxemia, hypocapnia, blood alkalosis, or arterial oxygen saturation (Savourey et al., 2003). In an attempt to compare the consequences of perinatal exposure to HH and NH and to choose the more suitable animal model for further studies, we have exposed postnatal day 1 (P1) pups to both modes of hypoxia. In our previous study, we explored a HH model and observed a long-lasting reorganization of the connectivity in the cingulate cortex, accompanied by cognitive impairment and prominent alteration in exploratory behavior that persisted in adulthood (Trnski et al., 2022).
In the present study, we exposed P1 neonates to a mild NH. We hypothesized the following: (1) Milder behavioral alterations after the exposure to NH, compared to HH, could be expected, but some aspects of altered exploratory behavior, as the most prominent behavioral consequence of hypobaric HBI, should be preserved, (2) the altered exploratory behavior could be related to the impairments in sensory processing and spatial learning, and (3) the increased DA and/or noradrenaline (NA) concentrations in the midbrain/pons could be expected, affecting thereby downstream signaling in the regions involved in somatosensory processing and spatial learning (i.e., thalamus and hippocampus). To compare behavioral consequences of NH to that of HH, we submitted a large group of hypoxic and control animals of adolescent age to a set of behavioral tests investigating exploratory behavior, social behavior, and learning. In order to search for the functional background of altered exploratory behavior, we then submitted another group of animals to a set of tests specifically exploring sensory-motor function and spatial learning. Finally, to check neurochemical alterations induced by hypoxia in subcortical brain regions involved in the mediation of exploratory behavior, we measured catecholamine concentrations in the part of the brain stem encompassing the midbrain (the main source of DA) and pons (the main source of NA). Additionally, we assessed the relative expression of genes for dopamine D1 and D2 receptors and their downstream targets in the hippocampus and thalamus. Animals of both sexes were used to check the potential differential vulnerability to HBI.
2 Materials and methods
2.1 Animals
All animal experiments comply with the ARRIVE guidelines and have been carried out following the United Kingdom Animals (Scientific Procedures) Act, 1986, EU Directive 2010/63/EU, Croatian regulations for experimentation on animals, and constitutive documents: NN 102/2017 and 32/19; NN 55/2013; 39/17 and 116/2019. The ethical committee of the University of Zagreb and national ethical and animal welfare bodies (EP231/2019; UP/I-322-01/19.01/75) approved the study design and experiments. Every effort was made to reduce the number of animals in use and to minimize animal discomfort.
2.1.1 Animal housing
Animals were housed in polysulfone cages within a controlled environment with a temperature of 21 ± 2°C and humidity maintained at 65 ± 5%. A light or dark cycle of 12:12 h was employed with the light period beginning at 7 a.m. Food (4RF21C, Mucedola srl, Settimo Milanese MI, Italy) and tap water were provided ad libitum. The minimum number of animals needed for the study was determined by power analysis, and a total of 102 1-day-old Wistar Han (RccHan: WIST) rats were obtained from our breeding facility (School of Medicine, University of Zagreb, Croatia). The day of birth was considered P0 until noon of the next day when P1 began. After exposure to hypoxia, pups were permanently marked by a toe tattoo (NEO-9 Neonate Tattoo System, AgnTho’s AB, Sweden) and returned to dams until weaning at P30. After weaning, they were separated by sex and group, and 3–4 of them were kept in each cage until they were sacrificed for brain tissue collection (P50). A scheme of experiments and number of animals used can be found in Table 1.
2.1.2 Exposure of animals to normobaric hypoxia
P1 pups (without any dysmorphic features and of an average body mass of 6.68 ± 0.27 g) were randomly assigned to the hypoxic or control group, keeping both sexes equally represented. A modification of the hypoxia-inducing protocol was made according to Zhang et al. (2013). The hypoxic group (4F + 4 M per session) was placed for 2 h in a closed-heated hypoxia chamber (STEMCELL Technologies Inc. Vancouver, Canada; Cat. No. 27310) connected to the bottle containing the calibration gas mixture of 8% O2 and 92% N2 (Messer Croatia Plin d.o.o., Zaprešić, Croatia), using a spectromed cylinder pressure regulator FM41-S1 (Spectron Gas Control Systems GmbH, Langen, Germany), which enabled a continuous flow of a mixture of gases of 3.5 L/min. During the first 15 min, temperature was gradually raised from 23.4 to 30°C and then maintained at 30°C until the end of the experiment. For animal comfort and welfare, webs of cellulose fibers and bedding from the home cage were placed in the chamber. Oxygen partial pressure, temperature, pressure, and humidity were continuously monitored by an optical oxygen gas sensor—FDO2 (PyroScience GmbH, Aachen, Germany) connected to a computer. An absorbent for CO₂ was also placed in the chamber to annulate CO2 exhaled by the animals. The control group (4F + 4 M per session) was placed in a smaller cage at normoxic conditions (atmospheric air 21% O2 and 79% N2) in the above described heating regime for 2 h together with bedding from a home cage and a thermometer/hygrometer.
2.2 Behavioral testing
Testing was performed between 2 and 6 pm under illumination of 30 lx, in a randomized order, by experimenters unaware of the rat’s treatment group. The apparatuses were thoroughly cleaned after each animal or each trial to remove odor. Sessions were filmed using a camera placed above the apparatus and analyzed using EthoVisionXT13 software (Noldus Information Technology Inc., Leesburg, VA, SAD).
2.2.1 Initial behavioral tests
A set of initial behavioral tests was performed on 34 control (18 female rats and 16 male rats) and 36 hypoxic (18 female rats and 18 male rats) animals, from P33 to P43, in the following order: open field, hole-board test, T-maze, and social choice. Apparatuses and procedures have been described in detail in our previous research (Blazevic et al., 2012; Trnski et al., 2022).
An open field (of) enclosure was used to measure a novel space exploration behavior consisting of horizontal movement interrupted by occasional stopping, head scanning, and rearing. It is influenced by the inherent locomotor activity reflected as ambulation in an open field, exploratory tendency reflected as rearing frequency, and anxiety level reflected as the amount of time spent on a less safe location of the testing apparatus (Thompson et al., 2018). Distance covered (DCof in cm), time spent in movement (TMof in s), and number of rearings (Rof) were recorded during a 5-min session.
Same enclosure was turned into a hole board (hb) and used to study head dipping (both eyes in a hole) as a form of novel object exploration behavior involving orienting toward, touching, and sniffing a novel object, which provides animal information on the potential usefulness or threat of an unfamiliar item (Brown and Nemes, 2008). The total number of holes visited (THVhb) and the percentage of inner holes (%INhb) were recorded during a 5-min session.
Reward-motivated spatial learning was tested in a T-maze (tm) as an attempt to find a reward (food pellet) according to a Win/Stay strategy. The number of correct choices (CCtm) in sessions of 10 60-s trials during 5 consecutive days was recorded. During the entire testing period, rats had access to food only for 1 h daily after the session.
Sociability represents a degree of interest in an unknown conspecific and can be measured as the time spent exploring a conspecific in a social choice test (sc). A testing rat was placed into the central compartment and, after 3 min of habituation, was allowed to freely explore side compartments, containing either an inanimate object or a same-sex conspecific placed in a wire, during a 5-min period. Latency to approach the rat (LRsc) and time spent exploring the rat (TRsc) were recorded as measures of sociability. Time out of the middle chamber (TOMCsc in s), number of rearings (Rsc), and time spent exploring an object (TOsc) were recorded as additional measures of anxiety level, novel space exploration, and novel object exploration, respectively.
2.2.2 Additional behavioral tests
To check which functional aspect of exploratory rearing has been potentially changed, additional tests were performed on another group of 16 control (eight female rats and eight male rats) and 16 hypoxic (eight female rats and eight male rats) animals, from P33 to P41, in the following order: cylinder test, object location memory, and adhesive removal test.
The level of spontaneous vertical locomotion was examined in the cylinder test (CT), developed for assessing forelimb use asymmetry during spontaneous rearing in rodent models of neurological deficits (Schallert et al., 2000; Magno et al., 2019; Penny et al., 2021). The rat was placed in a glass cylinder, separated from the experimenters by a black curtain, and the number of rearings in the cylinder (Rc) during a 10-min period was recorded.
Retention of spatial memory was examined by the object location memory (OLM) test measuring the rat’s preference for an object in a novel versus familiar location (Gerstein et al., 2013). OLM was tested in the open field for 3 consecutive days. On day 1, each rat underwent two rounds of 5-min habituation in an empty arena. On day 2, the rat was allowed to explore two identical objects (LEGO blocks) placed in two corners of the arena (locations A and B) for a 10-min period. On day 3, in another 10-min session, rats were presented with the same location of one object (location A) and a novel location of the other object (location C). Frequencies of entry (FE) in the zone of the object at locations A, B, and C were recorded, and the frequency of entry ratios (FER) FEB/FEA and FEC /FEA were counted as discrimination factors between the two locations.
Sensorimotor function was assessed by the adhesive removal test (ART) (Komotar et al., 2007; Bouet et al., 2009). The test was performed in an empty transparent cage for 5 consecutive days. After 1 min of habituation, the rat was picked up by one experimenter, while the other attached an adhesive (1 cm2 of collage paper, sticky on one side) on its forepaws and returned it to the cage. The latency time to the beginning of removal (time-to-contact) and the time needed to remove the adhesive from the paws (time-to–remove) were recorded on the 1st day (initial response) and on the 5th day (after training). Average values for the left and right paw were used as the time-to-contact (TC in s) and time-to-remove (TR in s) which implied, respectively, paw and mouth sensitivity (time-to-contact) and dexterity (time-to-remove).
2.3 Tissue collection and homogenization
The tissue was collected from the subgroup of rats exposed to the initial set of behavioral tests. On P50, 16 rats (eight female rats and eight male rats), from the group exposed to hypoxia, and 15 rats (eight female rats and seven male rats), from the control group, were sacrificed in a randomized order. After administering isoflurane-provoked anesthesia (Abbott), the animal was decapitated, and the brain was quickly removed from the skull and dissected on a cold plate. The brain was cut in the midsagittal plane, and the hippocampus and thalamus were sampled separately, while mesencephalon and pons were isolated as a united sample (Supplementary Figure 1). Each collected sample was placed in a microtube and briefly weighed. The midbrain/pons tissue was kept on dry ice until homogenized with an ultrasonic homogenizer (Bandelin Sonopuls, Germany) in five volumes of deproteinizing solution (0.01 N HCl, 1 mM EDTA, 4 mM Na2S2O5) and stored at −20°C for the monoamine concentrations measurement. The thalamic and hippocampal tissue was kept in liquid nitrogen until disrupted and homogenized with the ultrasonic homogenizer in 12 volumes of lysis solution (Demeditec Diagnostics GmbH, Germany) and stored at −80°C for RNA isolation. To obtain the optimal amount of tissue for the isolation protocol, the thalamus was taken from one (left) hemisphere and the hippocampus from both hemispheres.
2.4 ELISA
Tissue homogenates were thawed, centrifuged at 24,000 × g and 4°C for 20 min, and an aliquot of the clear supernatant was used for the measurements of dopamine (DA), noradrenaline (NA), and adrenaline (ADR) concentrations using the 3-CAT Research ELISA (Demeditec Diagnostics GmbH, Germany) according to the kit instructions. After determining the optimal sample concentration for the reliable measurements of both DA and NA (ADR concentration in an aliquot was too low), samples were assayed in duplicates in a single assay (one for DA and one for NA). Intra-assay coefficients of variation, calculated from replicate standard curve vials, were 3.0% for DA and 4.2% for NA. A calibration curve was drawn based on the absorbance measured at 450 nm on a microplate reader (Bio-Rad, Germany) and known concentrations of the standard solutions. The concentration values of samples were obtained by interpolating them onto the calibration curve, using four-parameter non-linear regression curve fitting. The results were expressed in pg of DA/NA per mg of the wet brain tissue.
2.5 RNA isolation and qPCR
Total RNA was isolated using the phenol-free RNAqueous-4PCR kit (Ambion, Inc., Austin, TX, United States), and genomic DNA was removed, according to the manufacturer’s instructions. RNA concentration and quality were measured in a spectrophotometer (Biochrome) and assessed through agarose gel electrophoresis. From 1 μg of total RNA, mRNA was reversely transcribed using MuLV reverse transcriptase (Applied Biosystems, Foster City, CA, United States) and oligo dT primers (Applied Biosystems, Foster City, CA, United States), following the manufacturer’s instructions in a total volume of 20 μL. The performance of the reverse transcription was assessed through PCR using positive intron-spanning primers provided in the isolation kit. cDNA was stored at −20°C until further processing.
Data for five genes of interest (GOI)—Drd1, Drd2, Ppp1r1b, Prkar2a, and Ppp1r1c—and two endogenous references (ER)—Actb and Hprt1—are listed in Supplementary Table 1. To determine starting cDNA concentrations and to test the efficiency of amplification for all genes (efficiency between 90 and 110% is considered acceptable), 5-fold dilutions of pooled cDNA were used. The relative expression of genes was assessed through qPCR using the TaqMan gene expression master mix (Applied Biosystems, Foster City, CA, United States) according to the manufacturer’s instructions. The final volume of 20 μL contained 10 μL of master mix, 1 μL of the primers and probes for the reference gene (VIC labeled, primer-limited, Applied Biosystems) or 1 μL of the primers and probes for the gene of interest (FAM labeled, Applied Biosystems), 7 μL of nuclease-free H2O, and 2 μL of cDNA in the range of 50–60 ng per reaction. Samples were run in triplicates in singleplex reactions (for each sample, one GOI and both ERs were run on the same plate). One sample was randomly chosen as a calibrator and was loaded on each plate to correct experimental differences among consecutive PCR runs. The qPCR setup in the qTower3 PCR System (Analytik Jena, Germany) was 2 min at 50°C, 10 min at 95°C, followed by 40 cycles of 95°C for 15 s and 60°C for 60 s. The amplification results were analyzed with qPCRsoft 4.0 software (Analytik Jena, Germany). By using the equation 2-ΔΔCt, threshold cycles (Ct) of GOI and ERs were normalized to the corresponding threshold cycles of a calibrator, and the levels of GOI mRNAs were expressed relative to an average of the two endogenous references, i.e., in arbitrary units (AU).
2.6 Statistical analyses
Statistical analyses were performed using Prism8 (GraphPad Software, Inc., La Jolla, CA, United States) and JMP 11.2 (SAS Institute Inc., Cary, NC, United States). The normality of distribution was assessed by the Kolmogorov–Smirnov test. Values differing more than two standard deviations from the mean were considered outliers and were excluded from the statistical analyses if they did not comply with methodological standards (degrees of freedom indicating the analyzed number of samples for each test are listed in Supplementary Tables 2–4). The univariate split-plot approach was used to analyze CCtm, FER, TC, and TR by repeated measure ANOVA, with hypoxia and sex representing between-subject variables, and testing day/location representing within-subject variables. Kenward–Roger first-order approximation was used to calculate the degree of freedom of the denominator in case of the missing values. An independent two-way ANOVA was used, on original or transformed values, to check for the influence of hypoxia, sex, and their interaction on all other behavioral parameters as well as on catecholamine concentrations and relative GOI expression. If a significant effect was revealed, Tukey’s honest significance test was used for post-hoc analyses. Correlation between the parameters was calculated using Pearson or Spearman correlation coefficient, depending on the normality of distribution. A p value of <0.05 (two-tailed) was considered to be statistically significant. Values in the text were expressed as mean ± standard error of the mean (SEM).
3 Results
3.1 Behavioral alterations
The results of the initial set of behavioral tests are displayed in Figure 1, and all numerical values and statistical parameters are shown in Supplementary Table 2.
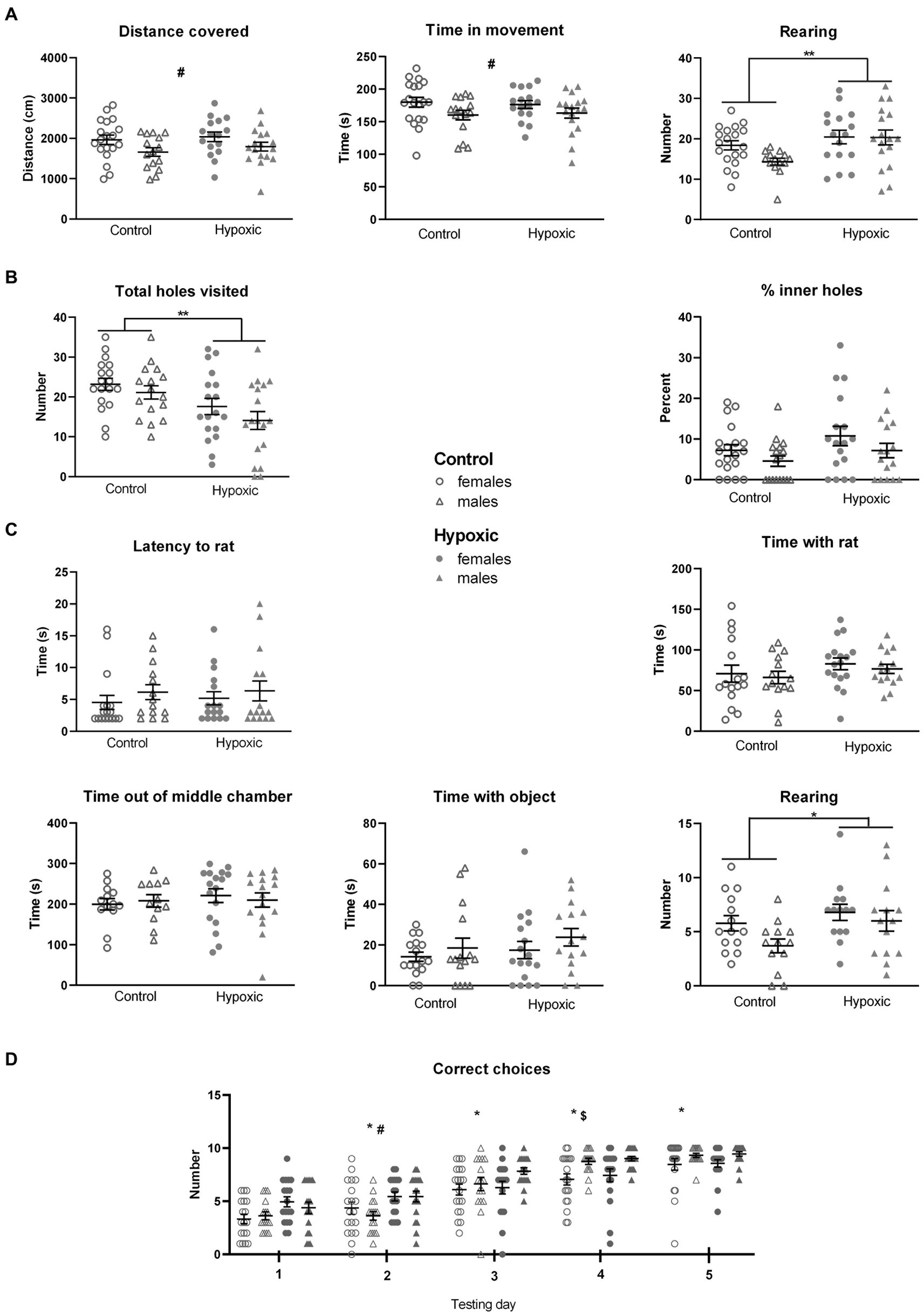
Figure 1. Behavior of 34 control (18 female rats and 16 male rats) and 36 hypoxic (18 female rats and 18 male rats) animals, at adolescent age, measured in (A) Open field test. Two-way ANOVA revealed significant effects of sex on distance covered and time in movement (#p < 0.05) and significant effects of treatment on the number of rearings (**p < 0.01). (B) Hole-board test. Two-way ANOVA revealed a significant influence of treatment on the total number of visited holes (**p < 0.01). (C) Social choice test. The only significant effect revealed by two-way ANOVA was the influence of treatment on the number of rearings (*p < 0.05). (D) T-maze test. Three-way repeated measure ANOVA revealed significant influences of treatment, testing day, sex × day interaction, and indicative influence of treatment × day interaction on the number of correct choices. Tukey’s honest significance post hoc test revealed significant differences among each testing day (*), between control and hypoxic animals on the second testing day (#), and between female and male rats on the fourth testing day ($). Results are shown as mean ± standard error.
The perinatal hypoxia did not have a significant effect on locomotor activity, measured as distance covered (F1,63 = 0.00, p = 0.96) and time in movement (F1,63 = 0.83, p = 0.37) in an open field test (Figure 1A). On the other hand, this was the only aspect of behavior with significant sex influence, with female rats being more prone to horizontal movement than male rats (F1,63 = 5.74, p = 0.02 for DC, and F1,63 = 4.97, p = 0.03 for TM). A significant effect of hypoxia was observed in exploratory rearing. Compared to the controls, the affected rats displayed a significantly higher number of rearings when placed in a novel space (F1,62 = 7.60, p = 0.008).
The rats subjected perinatally to mild NH displayed significantly decreased novel object exploration tendency, measured as a reduced number of holes visited in a hole-board test (F1,66 = 10.8, p = 0.002), while the anxiety-like behavior, measured as a percentage of inner holes visited in a hole board, was not significantly affected by hypoxia (F1,66 = 3.23, p = 0.08; Figure 1B).
In a social choice test (Figure 1C), experimental groups did not differ with respect to sociability, measured as latency to approach the conspecific (F1,63 = 0.20, p = 0.66) and as the time spent in their exploration in a social choice test (F1,59 = 2.57, p = 0.11). Time spent out of the familiar middle chamber, representing a measure of anxiety-like behavior, and the time spent in an object exploration were not significantly affected by mild NH (F1,63 = 1.98, p = 0.17, and F1,59 = 1.03, p = 0.31, respectively). On the other hand, a tendency for novel space exploration, reflected in the number of rearings, was again significantly higher in the hypoxia-exposed group (F1,54 = 4.52, p = 0.04).
Reward-motivated spatial learning, tested as a number of correctly chosen T-maze arms containing food, during the 5 consecutive days (Figure 1D), was significantly affected by the perinatal hypoxia (F1,66 = 6.95, p = 0.01) and testing day (F4,264 = 155, p < 0.0001). Sex x day interaction was also significant (F4,264 = 5.14, p = 0.0005), while the p value for the treatment × day interaction was close to the threshold of 0.05 (F1,63 = 2.31, p = 0.06). The integral group of animals significantly improved their performance during the consecutive days. Interestingly, hypoxic animals had a higher number of correct choices than control animals during the first 2 days of testing and then reached similar values as the testing progressed. On the other hand, male rats had similar numbers of correct choices as female rats during the first 3 days of testing but outperformed the female rats toward the end of testing.
Sex × treatment interaction did not significantly affect any of the measured parameter, indicating similar consequences of the perinatal hypoxia in both sexes.
3.1.1 Functional aspects of altered exploratory rearing
Since increased exploratory rearing emerged as the most prominent behavioral alteration in hypoxic animals, we further examined possible alterations in its functional aspects—spontaneous locomotion, somatosensory processing, and spatial learning. All numerical values and statistical parameters are shown in Supplementary Table 3.
Dividing the total number of rearings into unsupported (UR) and supported (SR) revealed that approximately 85% of all rearings were SR and only approximately 15% were UR (Figure 2). Accordingly, a significant effect of mild NH was observed only on supported rearing (F1,62 = 13.3, p = 0.0005, and F1,62 = 1.61, p = 0.21, respectively). We therefore measured the number of supported rearings in the cylinder test to check whether the hypoxic animals display a general increase in vertical locomotion when there is no open space to explore. Hypoxia did not significantly affect spontaneous upright movement measured as the number of supported rearings in a cylinder (F1,25 = 0.01, p = 0.92; Figure 3A).
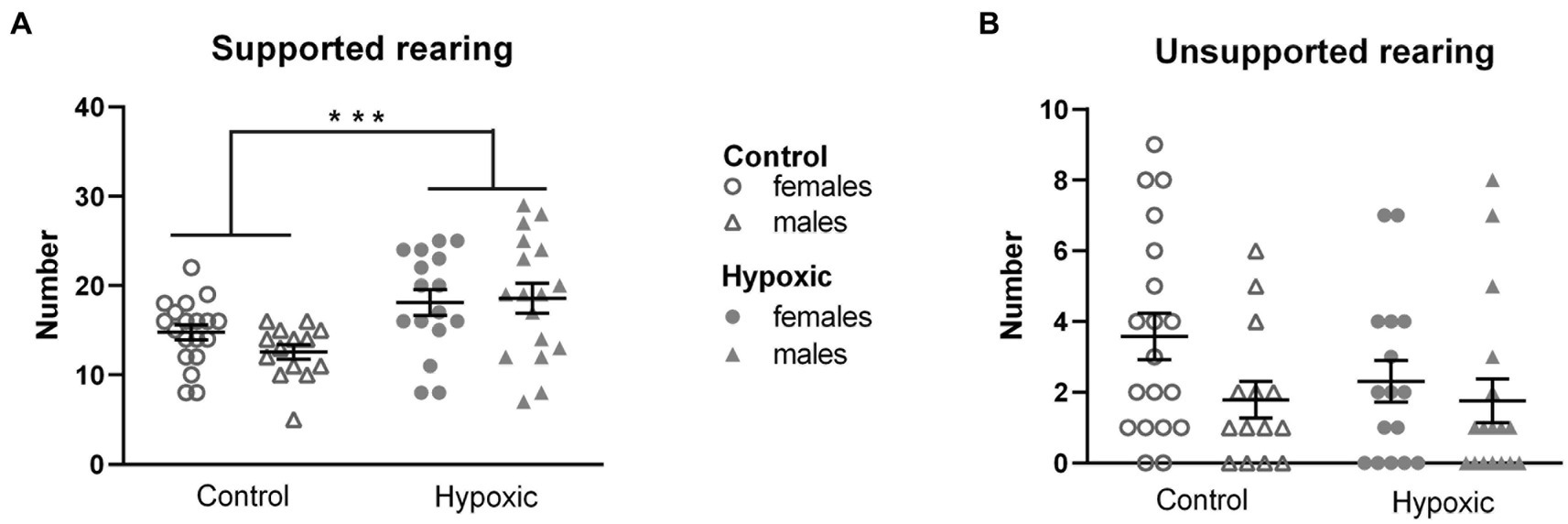
Figure 2. Rearing in open field of 34 control (18 females, 16 males) and 36 hypoxic (18 females, 18 males) rats divided into (A) supported rearing and (B) unsupported rearing. Two-way ANOVA revealed significant influence of treatment on SR (***p < 0.001). Results are shown as mean ± standard error.
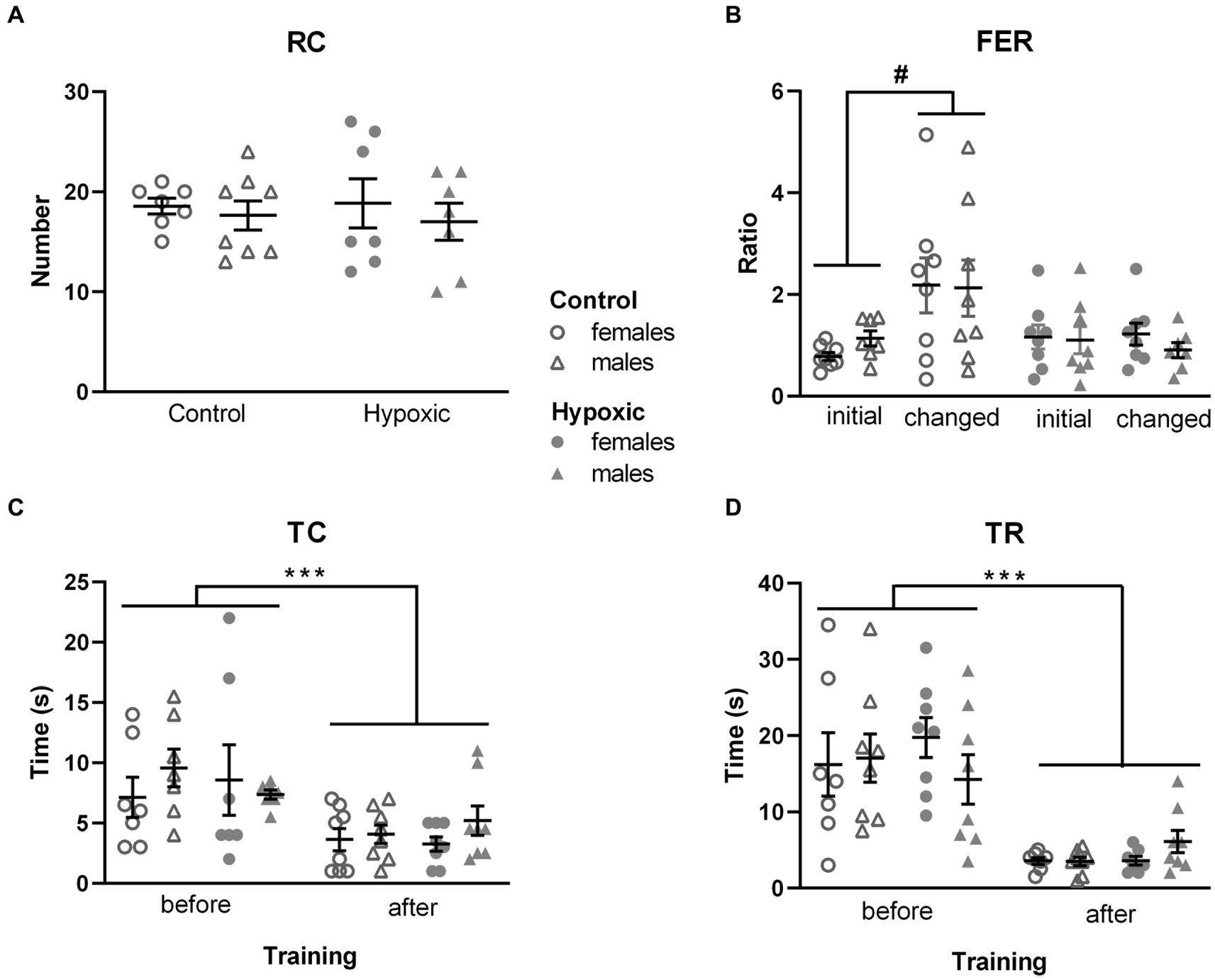
Figure 3. Behavior of 16 control (8F, 8 M) and 16 hypoxic (8F, 8 M) animals in additional behavioral tests intended to reveal the origin of increased rearing. (A) Spontaneous vertical locomotion was examined in a cylinder test as the number of rearings, RC. (B) Spatial memory was examined in the object location memory test as a ratio between frequencies of entry (FER) into the two object zones. After three-way repeated measure ANOVA showed significant effects of treatment x location interaction, Tukey’s honest significance post hoc test revealed significant differences in the object location discrimination in control but not in hypoxic rats (#p < 0.05). (C) Time to contact the adhesive (TC) and (D) time to remove the adhesive (TR) attached to the front paws were used to examine sensory-motor function in the adhesive removal test. Three-way repeated measure ANOVA revealed only significant effects of training on both TC and TR (***p < 0.0001).
In our study, perinatal hypoxic incident significantly impaired spatial memory (Figure 3B), which was reflected in significant hypoxia × location interaction (F1,28 = 7.99, p = 0.009). For both hypoxic and control animals, the frequency of entries into the zones of the two objects placed in an open field was similar in the initial test (FER = 0.96 for control and FER = 1.13 for hypoxic group). However, when one of the objects was displaced in the second test, control animals visited the object twice as many times as the object placed in the familiar location (FER = 2.15), while this shift did not occur in the hypoxic group (FER = 1.06).
As alterations in the novel object exploration after perinatal hypoxia indicated the possibility of altered somatosensory processing, we assessed the sensorimotor function in the adhesive removal test. Mild perinatal hypoxia did not affect sensory function, measured as the time to contact the adhesive attached to the front paws (F1,27 = 0.00, p = 0.96; Figure 3C), nor the dexterity, registered as the time to remove the adhesive (F1,27 = 0.22, p = 0.64; Figure 3D). Both parameters were influenced only by training (F1,27 = 17.4, p = 0.0003 for TC and F1,27 = 63.0, p < 0.0001 for TR) as all four subgroups were more efficient in performing the tasks after 3 training days.
None of the tested parameters was significantly affected either by sex or treatment × sex interaction.
3.2 Alterations in catecholamine neurotransmission pathways
The subsequent question that arose was whether the found alterations in exploratory rearing were catecholamine-mediated. We therefore measured NA and DA concentrations in the region abundant with dopaminergic neurons (Supplementary Figure 1) in a subgroup of animals (Figure 4). All numerical values and statistical parameters for the catecholamine-related parameters are shown in Supplementary Table 4. While hypoxia did not significantly affect NA concentrations (F1,27 = 0.76, p = 0.30), mean DA concentrations were approximately three times higher in the hypoxic than in the control group (F1,27 = 25.8, p < 0.0001). Sex and sex × hypoxia interaction did not significantly affect catecholamine concentrations. Interestingly, statistically significant positive correlations were observed between midbrain/pons DA concentrations and number of exploratory rearings (r = 0.466, p = 0.008) and, more specifically, supported rearings (r = 0.474, p = 0.007).
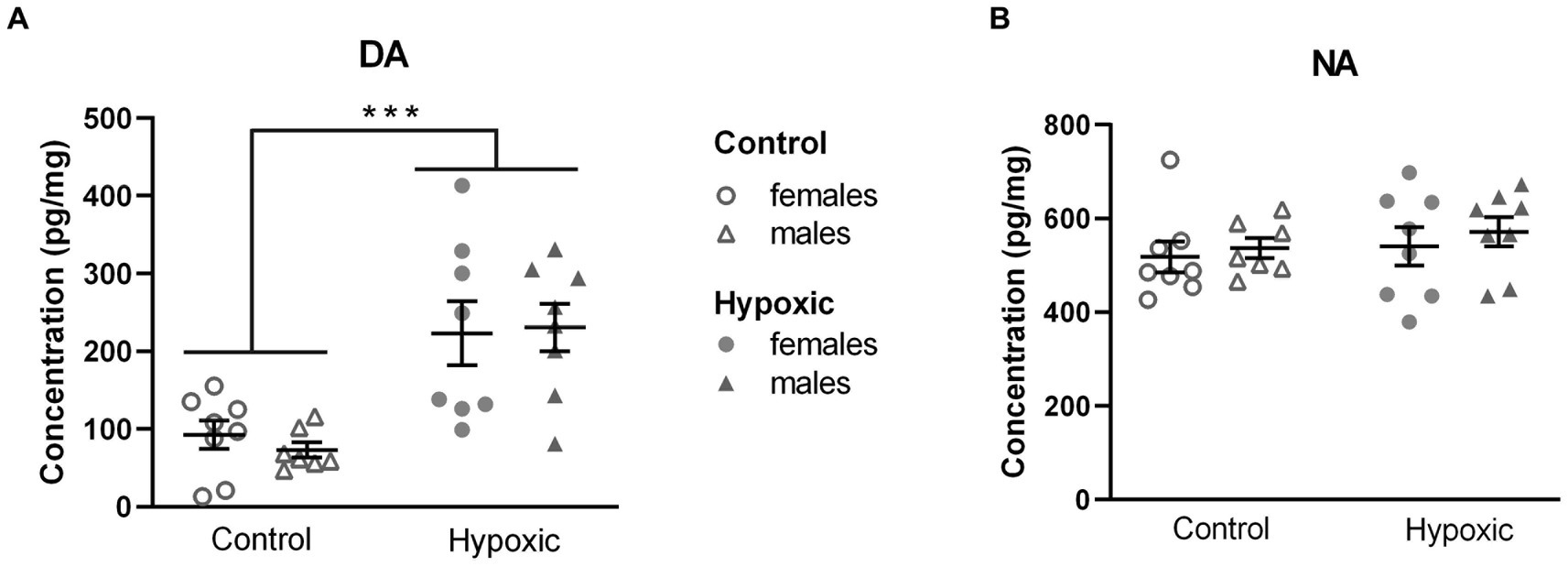
Figure 4. Midbrain/pons concentration of (A) dopamine (DA) and (B) noradrenaline (NA), in pg/mg, in 15 control (8F, 7 M) and 16 hypoxic (8F, 8 M) animals. Results are shown as mean ± standard error. ***p < 0.0001, effect of treatment by two-way ANOVA.
Considering that our hypoxic animals displayed spatial memory deficits, we further explored the influence of increased midbrain/pons DA content on the DA neurotransmission in two regions involved in spatial processing—the thalamus and hippocampus. Possible alterations in DA signaling were determined by relative expression analysis of the genes for D1 and D2 receptors (Drd1, Drd2; Figure 5). While hypoxia did not significantly affect the relative expression of Drd1 (F1,25 = 0.01, p = 0.91) and Drd2 (F1,23 = 0.05, p = 0.82) in the hippocampus (Figure 5A), the expression of both genes was significantly upregulated (F1,25 = 7.59, p = 0.01 and F1,23 = 7.44, p = 0.01, respectively) in the thalamus (Figure 5B). To confirm altered DA signaling in the thalamus, we further analyzed the relative expression of the genes for the receptors’ downstream targets: the regulatory subunit of protein kinase A, PKArs (Prkar2a), the dopamine- and cAMP-regulated phosphoprotein, DARPP-32 (Ppp1r1b), and inhibitor-5 of protein phosphatase 1, IPP5 (Ppp1r1c) (Figure 5C). Changes in the expression of all three genes pointed in the same direction, with a significant increase in the hypoxic group compared to the control group (F1,27 = 6.17, p = 0.02 for Prkar2a, F1,27 = 5.56, p = 0.03 for Ppp1r1b, and F1,63 = 20.0, p = 0.0001 for Ppp1r1c). Sex had a significant effect on the expression of Drd1 in the hippocampus (F1,25 = 4.74, p = 0.04) and the expression of Drd2 in the hippocampus (F1,23 = 9.51, p = 0.005) and thalamus (F1,23 = 6.95, p = 0.02), with higher expression in female rats. The expression of other genes was not affected by sex. Hypoxia × sex interaction did not significantly affect the relative expression of the mentioned genes.
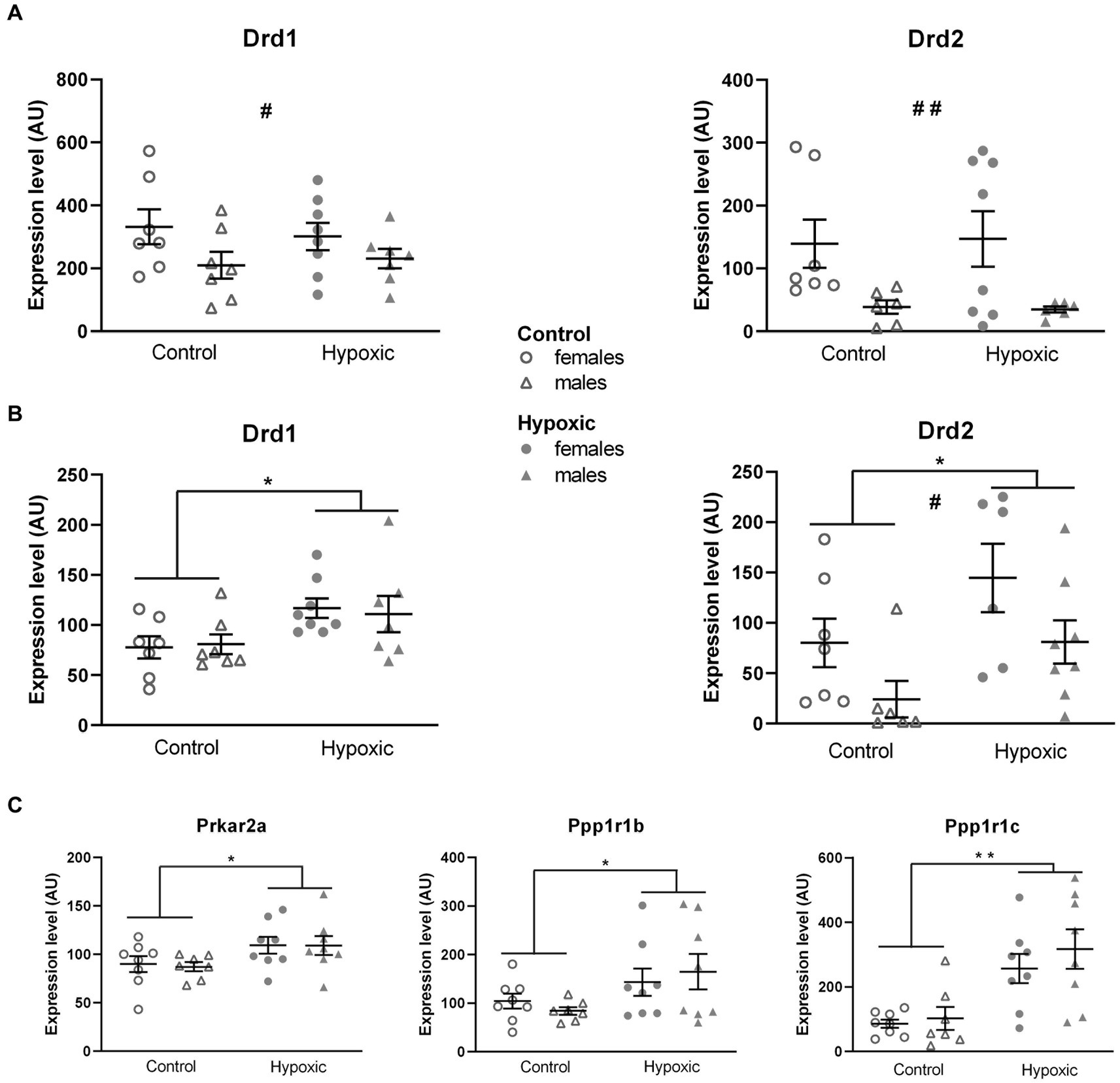
Figure 5. Relative expression of the genes for dopamine receptors D1 (Drd1) and D2 (Drd2) in the hippocampus (A) and thalamus (B) and for D1 and D2 downstream signalization molecules: regulatory subunit of protein kinase A, PKArs (Prkar2a) dopamine- and cAMP-regulated phosphoprotein, DARP-32 (Ppp1r1b), and inhibitor-5 of protein phosphatase 1, IPP5 (Ppp1r1c) in the thalamus (C), of 15 control (8F, 7 M) and 16 hypoxic (8F, 8 M) animals. mRNA levels are expressed in arbitrary units (AU), i.e., normalized to a calibrator and relative to an average of the two endogenous references, as 2-ΔΔCt × 100. Results are shown as mean ± standard error. *p < 0.05 and **p < 0.001, significant influence of treatment; #p < 0.05 and ##p < 0.001, significant effect of sex; two-way ANOVA.
4 Discussion
We have studied behavioral alterations, catecholamine content, and gene expression involved in dopaminergic signaling in adolescent rats that underwent mild normobaric hypoxia at P1. The study elucidates a high vulnerability of the immature dopaminergic neurotransmitter system. An increase in the midbrain/pons DA content and elevated expression of genes for the thalamic DA receptors and downstream signaling proteins was accompanied by deviated exploratory rearing behavior with impaired spatial learning.
4.1 Milder behavioral alterations were found in rats with HBI induced in normobaric compared to hypobaric conditions
To examine the behavioral effects of mild normobaric hypoxia and compare it to those of moderate hypobaric hypoxia observed in our previous study (Trnski et al., 2022), exploratory behavior, sociability, and cognitive performance, reported in literature as vulnerable to perinatal hypoxic events, were studied on a large group of adolescent rats in a set of behavioral tests.
Perinatal exposure to hypoxia has been frequently reported to affect locomotor activity and exploratory tendency, as the aspects of novel space exploration, without affecting anxiety. Increased ambulation in an open field was observed in juvenile rats exposed to intermittent hypoxia at P7-11 (Decker et al., 2005; Yamada et al., 2014), chronic NH at P0-21 (Mikati et al., 2005), as well as anoxia at P2 (Rogalska et al., 2004) and P4 (Shimomura and Ohta, 1988). A significant increase in both ambulation and rearing was reported in pre-pubertal rats after P2 NH (Ordyan et al., 2017) and juvenile rats after P0 anoxia (Speiser et al., 1983; Dell'Anna et al., 1991; Iuvone et al., 1996). No differences in open field and/or zero-maze thigmotaxis were observed in juvenile and/or adult rats after an invasive HI at P7 (Arteaga et al., 2015) or after a severe P0 asphyxia (Galeano et al., 2011; Herrera et al., 2018; Vázquez-Borsetti et al., 2019). In our laboratory setup, previously performed HH also evoked a significant increase in locomotor activity and exploratory tendency without affecting the anxiety level. In contrast, the exposure to NH in this study significantly affected only exploratory rearing both in the open field and social choice apparatus. Assuming that NH represents a milder insult than HH, we can conclude that rearing might be the aspect of novel space exploration that is most vulnerable to a hypoxic event.
An increase in novel object exploration after perinatal exposure to hypoxia has been reported as an increased frequency of head-dipping in the hole-board after a P7 HI (Arteaga et al., 2015) or as an increased time of object exploration after P1 anoxia (Laviola et al., 2004). HH in our previous study affected novel object exploration very mildly—only in a hole board and only in male rats. As opposed to previously reported findings (Trnski et al., 2022), the hole-board test in this study revealed a significant decrease in the number of visited holes in the entire group exposed to NH. This finding may be explained by an observation that hypoxic rats frequently dipped their head in a hole only partially, not fulfilling the criterion for scoring. Regardless of the underlying cause, which may include increased cautiousness or altered sensory processing, the hole-board test did not suggest an increase in novel object exploration. The lack of influence on the time spent exploring an object in the social choice test points in the same direction.
Sociability seems to be less vulnerable to milder hypoxic events. In adolescent rats, P12 ischemia did not alter social interaction (Tejkalová et al., 2007), P0 asphyxia selectively decreased play soliciting while leaving other aspects of social interaction intact (Vázquez-Borsetti et al., 2019), and only HI at P7 significantly lowered social preference (Ji et al., 2015) and at P0 increased social avoidance (Driscoll et al., 2018). In our studies, previous HH reduced sociability only in female rats, while the current NH did not affect sociability measured either as a latency to approach or as a time to explore a conspecific in a social choice test.
Cognitive alterations induced by perinatal hypoxia/anoxia have been described as impairments in working or reference memory in various spatial navigation tasks (Dell'Anna et al., 1991; Buwalda et al., 1995; Balduini et al., 2000; Decker et al., 2003; Mikati et al., 2005; Raveendran and Skaria, 2013). In our previous study, HH induced transient sex-specific learning impairment, with a significantly decreased number of correct choices in a T-maze in juvenile hypoxic male rats compared to the control male rats. On the contrary, NH in this study did not seem to impair T-maze performance. It is possible that our experimental conditions were mild compared to the moderate conditions used in the abovementioned studies. Similarly, Mikati et al. (2005) showed that acute hypoxia (4% O2) at P10, but not mild chronic hypoxia (10% O2) at P0–P21, decreased performance in the Morris water maze. Surprisingly, our hypoxic animals outperformed control rats during the first 2 days of testing but then leveled up with the controls for the rest of the testing period. This phenomenon might be explained by increased motivation for a reward in the hypoxic group that improved working memory in the early testing phase and compensated for a potential deficit in reference memory as the testing continued. Another possible explanation points to the opposite—while the spontaneous alteration of the T-maze arms (Richman et al., 1986) was suppressed in the control group by repeated presence of the reward in the same arm, the hypoxic group might not have associated the correct arm choice with the reward (Win/Stay strategy) in the early phase of testing and kept displaying spontaneous alteration. Indeed, within a 10-trial session during the first 2 days of testing, hypoxic animals would always walk to either arm of the maze (being correct or false), while the control animals would stop in many trials at the branching point and head-scanned until the testing time of a trial was over, rendering the outcome a false choice. The latter behavior was observed in our previous studies, and increasing the trial time did not result in an arm choice.
As opposed to our previous study in which exposure to hypobaric HBI differentially affected locomotor behavior and spatial learning in a sex-dependent manner, normobaric HBI in this study seemed to affect male and female pups equally, indicating that the sex-specific adverse effects of hypoxia may become detectable only after a more serious insult. Statistically significant sex-related differences in locomotor activity and spatial learning were noticed only generally between female and male rats regardless of treatment. This is in accord with published data reporting a higher level of locomotion in female rodents and a better spatial learning in male rodents (Belviranli et al., 2012; Chen et al., 2020), presumably underpinned by anatomical differences in the corresponding neuronal circuits induced by the neonatal testosterone surge in male rats (Clarkson and Herbison, 2016).
4.2 The impaired spatial learning might explain increased exploratory rearing
The possible functional relevance of altered exploratory rearing was examined by additional behavioral tests (CT, ART, and OLM).
Rearing in an open field is a measure of novel space exploration and represents a joint outcome of several separate aspects—inherent locomotor activity, exploratory tendency, and anxiety level. When a rat is placed in a narrow cylinder, there is no possibility of ambulation (hence, no space to explore and map), and no distinction between open space and walls, so rearing in the cylinder is considered to be the only result of inherent locomotor activity. The number of spontaneous rearings in CT was not altered in the hypoxic group, showing that, in our study, the inherent vertical locomotor activity, similar to the horizontal one, has not been affected by NH.
The lack of significant differences in ART between control and hypoxic animals indicated that NH did not affect sensory-motor processing. Similarly, no change in ART performance in adult rats was reported after P11 asphyxia (Gailus et al., 2021), P3 HI (Sanches et al., 2017), and adult NH (Yan et al., 2011). On the other hand, unilateral severe P7 HI was reported to increase adhesive removal time from both the affected and unaffected paw (Lowe et al., 2017). ART also revealed that both experimental groups significantly improved performance after 2 days of training, indicating that implicit learning in our hypoxic animals remained intact.
In the OLM test, hypoxic rats did not prefer exploring an object moved to a novel location, which was clearly seen in the control group. Several factors, such as locomotor activity, motivation, or anxiety level might have affected the performance of the hypoxic group in the OLM test. However, the initial set of behavioral tests did not show hypoxia-related alterations in the locomotor activity and anxiety level. In addition, the influence of the mentioned factors would have been reflected in the total frequencies of entry (FEA + FEB and FEA + FEC), but they did not differ between the groups (65 vs. 61 total entries in the first test and 66 vs. 68 total entries in the second test for control and hypoxic rats, respectively). Therefore, we consider the lack of preference for an object moved to a new location to be a sign of impairment in spatial memory. This result is in accordance with findings of decreased exploratory preference for an object in a non-familiar location after neonatal hipoxia-ischemia in P7 rats (Tata et al., 2015) and P10 mice (Spahic et al., 2022). It is also consistent with reports of impaired habituation memory in juvenile rats in the open field test (Sab et al., 2013) and an inability to discriminate between the new and familiar object in the novel object recognition memory test (Cunha-Rodrigues et al., 2018) after prenatal hipoxia-ischemia, suggesting that the exposure to NH affected retention of spatial memory 24 h after the initial test. Slightly different paradigms of testing may explain the distinction between the results obtained in OLM and T-maze tests. Unlike the T-maze test, which combines working memory, reference memory, and reward-based motivation, OLM uses natural rodent preference for novelty and does not depend on the retention of a rule or responsivity to reward (Ennaceur et al., 1997), representing a more sensitive measure of impairments in spatial learning.
Although rearing has been reported as an aspect of exploration affected by various hypoxic events, none of the studies mentioned in Section 4.1 distinguished between SR and UR. While UR is agreed upon to represent a hippocampus-mediated spatial mapping behavior, the function of SR is yet to be unraveled and has been suggested to represent the noradrenaline-mediated escape attempt, dopamine-mediated general increase in locomotion, or way of acquiring somatosensory space perception (Lever et al., 2006). In our study, rats reared predominantly by leaning against the wall, while unsupported rearings were scarce in both groups. Considering that rats are prone to thigmotaxic behavior, characterized by avoiding the center of open field apparatus, such a high portion of SR in the total number of rearings is not unexpected. Since the number of UR was too low for reliable statistical comparison, we can only claim a statistically significant effect of hypoxia on SR, which corresponded with the level of retention of spatial memory, but not with the inherent locomotor activity and sensory-motor processing.
4.3 Increased midbrain/pons DA content and altered thalamic DA signaling are observed in hypoxic rats
We further examined a possible catecholaminergic background of the increased exploratory rearing by measuring the midbrain/pons NA and DA content. The lack of differences in NA levels between the hypoxic and control groups diminished the possibility of explaining the increased SR as the NA-mediated escape attempt. On the other hand, DA levels increased threefold in hypoxic compared to control animals and significantly correlated with a number of exploratory rearings, pointing at a dopaminergic mediation of this behavior. Our results align with those obtained by Seidler and Slotkin (1990), who reported increased DA but not NA content after P1 hypoxia. An increase in the midbrain DA content and the number of DA-containing neuronal cell bodies was found after chronic intermittent hypoxia (Raghuraman et al., 2009) and after neonatal asphyxia (Bjelke et al., 1991; Chen et al., 1997). Alterations of the midbrain DA homeostasis after perinatal hypoxia were also observed in the human brain post-mortem (Pagida et al., 2013) and in the mesencephalic cell culture following exposure to non-damaging hypoxia during in vitro days 1–3 (Gross et al., 1999).
Hypoxia-induced rise in the midbrain/pons DA content could be expected to affect dopaminergic neurotransmission in the target regions. Alterations in DA signaling have been predominantly studied in the main midbrain DA targets—basal ganglia, for which an increase in dopaminergic innervation (Burke et al., 1991), extracellular DA concentration (Akiyama et al., 1991), and expression of DA-regulating elements (Boksa et al., 2002; Decker et al., 2003) has been reported, while the target regions involved in spatial learning have been far less explored. The hippocampus, already established as a key component of spatial processing (Bird and Burgess, 2008), receives the DA input from dopaminergic neurons in the VTA and substantia nigra (Gasbarri et al., 1994) as well as from noradrenergic neurons in the locus coeruleus (LC) (Kempadoo et al., 2016). The thalamus, particularly its anterior part containing head direction cells and dense interconnections with the limbic cortex (in which our previous study of HBI under hypobaric conditions revealed structural changes) and hippocampal formation, has been recently recognized as a critical mediator of spatial orientation (Jankowski et al., 2013).
Although recent optogenetic studies showed that midbrain DA communication with the hippocampus modulates the acquisition of long-term memory (McNamara et al., 2014; Rosen et al., 2015), this study did not indicate hippocampal changes in DA signalization, measured as relative mRNA levels for D1 and D2 receptors. Since we used RNA isolated from the whole hippocampus, discrete changes in Drd1 and/or Drd2 expression in specific regions could have gone unnoticed and should not be ruled out. Nevertheless, our current results do not support the involvement of dopaminergic signaling in the hippocampus in the hypoxia-induced memory deficit. Indeed, memory impairments in animal models, induced by H and HI, have been linked to the long-term hippocampal alterations in excitatory/inhibitory balance. An increase in extracellular acetyl-choline and a decrease in extracellular glutamate concentrations have been reported in adolescent rats after P7 hypoxia (López-Pérez et al., 2012). Additionally, an increase in the extracellular GABA level has been observed in adult rats after P10 hypoxia (Pozdnyakova et al., 2011), along with a higher rate of GABA synthesis in young rats after prenatal HI (Cunha-Rodrigues et al., 2018).
On the other hand, we are the first to report that dopaminergic alterations in the thalamus accompany cognitive impairment following a mild hypoxic event. Altered dopaminergic signaling in the thalamus, indicated by the significant increase in Drd1 and Drd2 expression, was confirmed by the corresponding increase in mRNA levels for the main downstream proteins in the D1/D2 signaling pathway: the regulatory subunit of PKA and the two inhibitors of PP-1: DARPP-32 and IPP5. An increased abundance of DA-pathway mRNAs in the thalamic tissue may represent the consequence of a long-lasting upregulation of dopaminergic pathway genes in the thalamic neurons following neonatal hypoxia. The expression of D1/D2 downstream elements after hypoxia has been scarcely investigated. A 2-fold increase in DARPP-32 abundance was noted in rat cortex following P7 hypoxia (Hu et al., 2006), but no reports of the long-term effects on expression could be found. Increased Prkar2a expression, related to a low-oxygen/high-altitude adaptation, was observed in the hearts of Tibetan pigs in comparison to low-land pigs (Zhang et al., 2019), while the expression of Ppp1r1c has been studied only in tumor hypoxia. Alternatively, increased abundance of DA-pathway mRNAs may result from structural changes induced by an increased dopaminergic innervation of the thalamus. The latter possibility is supported by the findings of a long-lasting increase in DA release sites in the rat striatum and nucleus accumbens after neonatal exposure to HI (Burke et al., 1991) as well as in the mouse striatum after prenatal exposure to hypoxia (Brandon et al., 2022).
By controlling the state of phosphorylation of a variety of downstream physiological effectors, the PKA/DARPP-32/PP-1 cascade was shown to regulate the functional state of neurons in the striatum (Greengard et al., 1998; Svenningsson et al., 2004), the activity of proteins involved in the MAPK signaling pathway in the prefrontal cortex (Nagai et al., 2007), and the activity of several transcription factors, including CREB, in the caudate putamen (Di Benedetto et al., 2007). It is, therefore, possible to presume that the hypoxia-induced alteration in DA signaling might have affected thalamic function and its communication with other brain regions involved in the mediation of exploratory behavior.
5 Conclusion
Mild perinatal HBI obtained under normobaric conditions presented in this study has generally affected similar behavioral aspects as previously presented HBI obtained under hypobaric conditions (exploration and cognition) but to a much lesser extent. This aligns with more severe physiological effects of HH than NH found in adult animals and humans (Savourey et al., 2003).
The set of initial behavioral tests pointed out exploratory rearing as the most prominent alteration induced by perinatal hypoxia regardless of hypo- or normobaric conditions. Our search for the functional aspect of rearing that contributed to the observed increase in this type of behavior identified lasting impairment of spatial learning, rather than impaired somatosensory processing or increased inherent vertical locomotion, in animals exposed to hypoxia.
Altered exploratory rearing was paralleled by a considerable increase of DA content in the midbrain/pons and by significant alterations in the thalamic dopaminergic signaling. The increased mRNA levels for DA receptors and their downstream elements likely resulted in increased levels of their protein products, which, depending on the regional D1 and D2 distribution, may have enhanced or attenuated short-term and long-term effects of DA in the thalamus. Altered DA signaling in the thalamus could have affected its communication with the target regions, including the hippocampus and cingulate cortex, indirectly contributing to the alterations in spatial processing.
We can conclude that NH is the least possible perinatal intervention on an animal model that can still induce measurable behavioral, neurochemical, and molecular alterations, and is therefore suitable for studying mild prenatal hypoxic events in humans. Further studies on our model, aimed at structural and molecular changes in particular thalamic nuclei as well as in the thalamocortical and thalamo-hippocampal projections, should give a closer insight into the role of mesothalamic and mesolimbic dopaminergic pathways in hypoxia, opening a possibility for studying new approaches to treat long-term behavioral outcomes after a hypoxic–ischemic insult.
Data availability statement
The original contributions presented in the study are included in the article/Supplementary material, further inquiries can be directed to the corresponding authors.
Ethics statement
The animal study was approved by the ethical committee of the University of Zagreb and national ethical and animal welfare bodies (EP231/2019; UP/I-322-01/19.01/75). The study was conducted in accordance with the local legislation and institutional requirements.
Author contributions
BN: Investigation, Methodology, Visualization, Writing – review & editing. ST-L: Investigation, Methodology, Visualization, Writing – review & editing. KK: Investigation, Writing – review & editing. MD: Visualization, Writing – review & editing. IB: Formal Analysis, Writing – review & editing. DH: Conceptualization, Formal Analysis, Supervision, Writing – original draft. NJ-M: Conceptualization, Funding acquisition, Project administration, Supervision, Writing – review & editing.
Funding
The author(s) declare financial support was received for the research, authorship, and/or publication of this article. This study was supported by the Croatian Science Foundation (projects: IP-2019-04-3182, DOK-01-3771 and DOK-02-5988), University of Zagreb School of Medicine Support (10106-22-3116 and10106-23-2487), University of Zagreb Faculty of Science Support (20285123 and 20285822), and the European Regional Development Fund, project “Experimental and clinical research of hypoxic–ischemic damage in perinatal and adult brain” (GA KK01.1.1.01.0007).
Acknowledgments
The authors would like to thank Ivica Kostović and Miloš Judaš for their encouragement to develop an animal model of perinatal hypoxia and for their valuable advice and help in that respect. The authors would also like to thank Nataša Kuretic and Valentina Ban-Lugarić for their dedication, care, and knowledge in using animals in research.
Conflict of interest
The authors declare that the research was conducted in the absence of any commercial or financial relationships that could be construed as a potential conflict of interest.
Publisher’s note
All claims expressed in this article are solely those of the authors and do not necessarily represent those of their affiliated organizations, or those of the publisher, the editors and the reviewers. Any product that may be evaluated in this article, or claim that may be made by its manufacturer, is not guaranteed or endorsed by the publisher.
Supplementary material
The Supplementary material for this article can be found online at: https://www.frontiersin.org/articles/10.3389/fnint.2023.1304338/full#supplementary-material
References
Aggleton, J. P., Keith, A. B., and Sahgal, A. (1991). Both fornix and anterior thalamic, but not mammillary, lesions disrupt delayed nonmatching-to-position memory in rats. Behav. Brain Res. 44, 151–161. doi: 10.1016/S0166-4328(05)80020-8
Aggleton, J. P., O'Mara, S. M., Vann, S. D., Wright, N. F., Tsanov, M., and Erichsen, J. T. (2010). Hippocampal-anterior thalamic pathways for memory: uncovering a network of direct and indirect actions. Eur. J. Neurosci. 31, 2292–2307. doi: 10.1111/j.1460-9568.2010.07251
Akiyama, Y., Koshimura, K., Ohue, T., Lee, K., Miwa, S., Yamagata, S., et al. (1991). Effects of hypoxia on the activity of the dopaminergic neuron system in the rat striatum as studied by in vivo brain microdialysis. J. Neurochem. 57, 997–1002. doi: 10.1111/j.1471-4159.1991.tb08249.x
Arteaga, O., Revuelta, M., Urigüen, L., Álvarez, A., Montalvo, H., and Hilario, E. (2015). Pretreatment with resveratrol prevents neuronal injury and cognitive deficits induced by perinatal hypoxia-ischemia in rats. PLoS One 10:e0142424. doi: 10.1371/journal.pone.0142424
Balduini, W., De Angelis, V., Mazzoni, E., and Cimino, M. (2000). Long-lasting behavioral alterations following a hypoxic/ischemic brain injury in neonatal rats. Brain Res. 859, 318–325. doi: 10.1016/s0006-8993(00)01997-1
Belviranli, M., Atalik, K. E., Okudan, N., and Gökbel, H. (2012). Age and sex affect spatial and emotional behaviors in rats: the role of repeated elevated plus maze test. Neuroscience 227, 1–9. doi: 10.1016/j.neuroscience.2012.09.036
Bird, C., and Burgess, N. (2008). The hippocampus and memory: insights from spatial processing. Nat. Rev. Neurosci. 9, 182–194. doi: 10.1038/nrn2335
Bjelke, B., Andersson, K., Ogren, S. O., and Bolme, P. (1991). Asphyctic lesion: proliferation of tyrosine hydroxylase-immunoreactive nerve cell bodies in the rat substantia nigra and functional changes in dopamine neurotransmission. Brain Res. 543, 1–9. doi: 10.1016/0006-8993(91)91041-x
Blazevic, S., Colic, L., Culig, L., and Hranilovic, D. (2012). Anxiety-like behavior and cognitive flexibility in adult rats perinatally exposed to increased serotonin concentrations. Behav. Brain Res. 230, 175–181. doi: 10.1016/j.bbr.2012.02.001
Boksa, P., Zhang, Y., and Bestawros, A. (2002). Dopamine D1 receptor changes due to caesarean section birth: effects of anesthesia, developmental time course, and functional consequences. Exp. Neurol. 175, 388–397. doi: 10.1006/exnr.2002.7896
Bouet, V., Boulouard, M., Toutain, J., Divoux, D., Bernaudin, M., Schumann-Bard, P., et al. (2009). The adhesive removal test: a sensitive method to assess sensorimotor deficits in mice. Nat. Protoc. 4, 1560–1564. doi: 10.1038/nprot.2009.125
Brandon, A., Cui, X., Luan, W., Ali, A. A., Pertile, R. A. N., Alexander, S. A., et al. (2022). Prenatal hypoxia alters the early ontogeny of dopamine neurons. Transl. Psychiatry 12:238. doi: 10.1038/s41398-022-02005-w
Brown, G. R., and Nemes, C. (2008). The exploratory behaviour of rats in the hole-board apparatus: is head-dipping a valid measure of neophilia? Behav. Process. 78, 442–448. doi: 10.1016/j.beproc.2008.02.019
Burke, R. E., Kent, J., Kenyon, N., and Karanas, A. (1991). Unilateral hypoxic-ischemic injury in neonatal rat results in a persistent increase in the density of striatal tyrosine hydroxylase immunoperoxidase staining. Dev. Brain Res. 58, 171–179. doi: 10.1016/0165-3806(91)90003-2
Buwalda, B., Nyakas, C., Vosselman, H. J., and Luiten, P. G. M. (1995). Effects of early postnatal anoxia on adult learning and emotion in rats. Behav. Brain Res. 67, 85–90. doi: 10.1016/0166-4328(94)00108-R
Chen, Y., Herrera-Marschitz, M., Bjelke, B., Blum, M., Gross, J., and Andersson, K. (1997). Perinatal asphyxia-induced changes in rat brain tyrosine hydroxylase-immunoreactive cell body number: effects of nicotine treatment. Neurosci. Lett. 221, 77–80. doi: 10.1016/s0304-3940(96)13293-6
Chen, W., Liu, B., Li, X., Wang, P., and Wang, B. (2020). Sex diferences in spatial memory. Neuroscience 443, 140–147. doi: 10.1016/j.neuroscience.2020.06.016
Clark, A. M., Leroy, F., Martyniuk, K. M., Feng, W., McManus, E., Bailey, M. R., et al. (2017). Dopamine D2 receptors in the paraventricular thalamus attenuate cocaine locomotor sensitization. eNeuro 4:ENEURO.0227-17.2017. doi: 10.1523/ENEURO.0227-17.2017
Clarkson, J., and Herbison, A. E. (2016). Hypothalamic control of the male neonatal testosterone surge. Philos. Trans. R. Soc. Lond. Ser. B Biol. Sci. 371:20150115. doi: 10.1098/rstb.2015.0115
Crusio, W. E. (2001). Genetic dissection of mouse exploratory behaviour. Behav. Brain Res. 125:127. doi: 10.1016/s0166-4328(01)00280-7
Cunha-Rodrigues, M. C., Do Nascimento Balduci, C. T., Tenório, F., and Barradas, P. C. (2018). GABA function may be related to the impairment of learning and memory caused by systemic prenatal hypoxia-ischemia. Neurobiol. Learn. Mem. 149, 20–27. doi: 10.1016/j.nlm.2018.01.004
Decker, M. J., Hue, G. E., Caudle, W. M., Miller, G. W., Keating, G. L., and Rye, D. B. (2003). Episodic neonatal hypoxia evokes executive dysfunction and regionally specific alterations in markers of dopamine signaling. Neuroscience 117, 417–425. doi: 10.1016/s0306-4522(02)00805-9
Decker, M. J., Jones, K. A., Solomon, I. G., Keating, G. L., and Rye, D. B. (2005). Reduced extracellular dopamine and increased responsiveness to novelty: neurochemical and behavioral sequelae of intermittent hypoxia. Sleep 28, 169–176. doi: 10.1093/sleep/28.2.169
Dell'Anna, M. E., Calzolari, S., Molinari, M., Iuvone, L., and Calimici, R. (1991). Neonatal anoxia induces transitory hyperactivity, permanent spatial memory deficits and CA1 cell density reduction in developing rats. Behav. Brain Res. 45, 125–134. doi: 10.1016/s0166-4328(05)80078-6
Di Benedetto, M., D'Addario, C., Candeletti, S., and Romualdi, P. (2007). Alterations of CREB and DARPP-32 phosphorylation following cocaine and monoaminergic uptake inhibitors. Brain Res. 1128, 33–39. doi: 10.1016/j.brainres.2006.10.062
Driscoll, D. J. O., Felice, V. D., Kenny, L. C., Boylan, G. B., and O'Keeffe, G. W. (2018). Mild prenatal hypoxia-ischemia leads to social deficits and central and peripheral inflammation in exposed offspring. Brain Behav. Immun. 69, 418–427. doi: 10.1016/j.bbi.2018.01.001
Ennaceur, A., Neave, N., and Aggleton, J. P. (1997). Spontaneous object recognition and object location memory in rats: the effects of lesions in the cingulate cortices, the medial prefrontal cortex, the cingulum bundle and the fornix. Exp. Brain Res. 113, 509–519. doi: 10.1007/pl00005603
Faa, G., Marcialis, M. A., Ravarino, A., Piras, M., Pintus, M. C., and Fanos, V. (2014). Fetal programming of the human brain: is there a link with insurgence of neurodegenerative disorders in adulthood? Curr. Med. Chem. 21, 3854–3876. doi: 10.2174/0929867321666140601163658
Frajewicki, A., Laštůvka, Z., Borbélyová, V., Khan, S., Jandová, K., Janišová, K., et al. (2020). Perinatal hypoxic-ischemic damage: review of the current treatment possibilities. Physiol. Res. 69, S379–S401. doi: 10.33549/physiolres.934595
Fremeau, R. T. Jr., Duncan, G. E., Fornaretto, M. G., Dearry, A., Gingrich, J. A., Breese, G. R., et al. (1991). Localization of D1 dopamine receptor mRNA in brain supports a role in cognitive, affective, and neuroendocrine aspects of dopaminergic neurotransmission. Proc. Natl. Acad. Sci. U. S. A. 88, 3772–3776. doi: 10.1073/pnas.88.9.3772
Gailus, B., Naundorf, H., Welzel, L., Johne, M., Römermann, K., Kaila, K., et al. (2021). Long-term outcome in a noninvasive rat model of birth asphyxia with neonatal seizures: cognitive impairment, anxiety, epilepsy, and structural brain alterations. Epilepsia 62, 2826–2844. doi: 10.1111/epi.17050
Galeano, P., Blanco Calvo, E., Madureira de Oliveira, D., Cuenya, L., Kamenetzky, G. V., Mustaca, A. E., et al. (2011). Long-lasting effects of perinatal asphyxia on exploration, memory and incentive downshift. Int. J. Dev. Neurosci. 29, 609–619. doi: 10.1016/j.ijdevneu.2011.05.002
García-Cabezas, M. A., Martínez-Sánchez, P., Sánchez-González, M. A., Garzón, M., and Cavada, C. (2009). Dopamine innervation in the thalamus: monkey versus rat. Cereb. Cortex 19, 424–434. doi: 10.1093/cercor/bhn093
Gasbarri, A., Verney, C., Innocenzi, R., Campana, E., and Pacitti, C. (1994). Mesolimbic dopaminergic neurons innervating the hippocampal formation in the rat: a combined retrograde tracing and immunohistochemical study. Brain Res. 668, 71–79. doi: 10.1016/0006-8993(94)90512-6
Gerstein, H., Hullinger, R., Lindstrom, M. J., and Burger, C. (2013). A behavioral paradigm to evaluate hippocampal performance in aged rodents for pharmacological and genetic target validation. PLoS One 8:e62360. doi: 10.1371/journal.pone.0062360
Giannopoulou, I., Pagida, M. A., Briana, D. D., and Panayotacopoulou, M. T. (2018). Perinatal hypoxia as a risk factor for psychopathology later in life: the role of dopamine and neurotrophins. Hormones 17, 25–32. doi: 10.1007/s42000-018-0007-7
Gonzalez, F. F., and Miller, S. P. (2006). Does perinatal asphyxia impair cognitive function without cerebral palsy? Archives of disease in childhood. Arch. Dis. Child. Fetal Neonatal Ed. 91, F454–F459. doi: 10.1136/adc.2005.092445
Greengard, P., Nairn, A. C., Girault, J. A., Ouimet, C. C., Snyder, G. L., Fisone, G., et al. (1998). The DARPP-32/protein phosphatase-1 cascade: a model for signal integration. Brain Res. Rev. 26, 274–284. doi: 10.1016/s0165-0173(97)00057-x
Gross, J., Ungethüm, U., Andreeva, N., Heldt, J., Gao, J., Marschhausen, G., et al. (1999). Hypoxia during early developmental period induces long-term changes in the dopamine content and release in a mesencephalic cell culture. Neuroscience 92, 699–704. doi: 10.1016/s0306-4522(98)00760-x
Hefter, D., Marti, H. H., Gass, P., and Inta, D. (2018). Perinatal hypoxia and ischemia in animal models of schizophrenia. Front. Psychol. 9:106. doi: 10.3389/fpsyt.2018.00106
Herrera, M. I., Udovin, L. D., Toro-Urrego, N., Kusnier, C. F., Luaces, J. P., and Capani, F. (2018). Palmitoylethanolamide ameliorates hippocampal damage and behavioral dysfunction after perinatal asphyxia in the immature rat brain. Front. Neurosci. 12:145. doi: 10.3389/fnins.2018.00145
Hu, X., Rea, H. C., Wiktorowicz, J. E., and Perez-Polo, J. R. (2006). Proteomic analysis of hypoxia/ischemia-induced alteration of cortical development and dopamine neurotransmission in neonatal rat. J. Proteome Res. 5, 2396–2404. doi: 10.1021/pr060209x
Huang, Q., Zhou, D., Chase, K., Gusella, J. F., Aronin, N., and DiFiglia, M. (1992). Immunohistochemical localization of the D1 dopamine receptor in rat brain reveals its axonal transport, pre- and postsynaptic localization, and prevalence in the basal ganglia, limbic system, and thalamic reticular nucleus. Proc. Natl. Acad. Sci. U. S. A. 89, 11988–11992. doi: 10.1073/pnas.89.24.11988
Islam, K. U. S., Meli, N., and Blaess, S. (2021). The development of the Mesoprefrontal dopaminergic system in health and disease. Front. Neural Circuits 15:746582. doi: 10.3389/fncir.2021.746582
Iuvone, L., Geloso, M. C., and Dell’Anna, E. (1996). Changes in open field behavior, spatial memory, and hippocampal parvalbumin immunoreactivity following enrichment in rats exposed to neonatal anoxia. Exp. Neurol. 139, 25–33. doi: 10.1006/exnr.1996.0077
Jankowski, M. M., Ronnqvist, K. C., Tsanov, M., Vann, S. D., Wright, N. F., Erichsen, J. T., et al. (2013). The anterior thalamus provides a subcortical circuit supporting memory and spatial navigation. Front. Syst. Neurosci. 7:45. doi: 10.3389/fnsys.2013.00045
Ji, G., Liu, M., Zhao, X. F., Liu, X. Y., Guo, Q. L., Guan, Z. F., et al. (2015). NF-κB signaling is involved in the effects of Intranasally engrafted human neural stem cells on Neurofunctional improvements in neonatal rat hypoxic-ischemic encephalopathy. CNS Neurosci. Ther. 21, 926–935. doi: 10.1111/cns.12441
Kempadoo, K. A., Mosharov, E. V., Choi, S. J., Sulzer, D., and Kandel, E. R. (2016). Dopamine release from the locus coeruleus to the dorsal hippocampus promotes spatial learning and memory. Proc. Natl. Acad. Sci. U. S. A. 113, 14835–14840. doi: 10.1073/pnas.1616515114
Khan, Z. U., Gutiérrez, A., Martín, R., Peñafiel, A., Rivera, A., and De La Calle, A. (1998). Differential regional and cellular distribution of dopamine D2-like receptors: an immunocytochemical study of subtype-specific antibodies in rat and human brain. J. Comp. Neurol. 402, 353–371. doi: 10.1002/(sici)1096-9861(19981221)402:3<353::aid-cne5>3.0.co;2-4
Komotar, R. J., Kim, G. H., Sughrue, M. E., Otten, M. L., Rynkowski, M. A., Kellner, C. P., et al. (2007). Neurologic assessment of somatosensory dysfunction following an experimental rodent model of cerebral ischemia. Nat. Protoc. 2, 2345–2347. doi: 10.1038/nprot.2007.359
Laviola, G., Adriani, W., Rea, M., Aloe, L., and Alleva, E. (2004). Social withdrawal, neophobia, and stereotyped behavior in developing rats exposed to neonatal asphyxia. Psychopharmacology 175, 196–205. doi: 10.1007/s00213-004-1800-3
Lever, C., Burton, S., and O’Keefe, J. (2006). Rearing on hind legs, environmental novelty, and the hippocampal formation. Rev. Neurosci. 17, 111–133. doi: 10.1515/revneuro.2006.17.1-2.111
Leviton, A., and Nelson, K. B. (1992). Problems with definitions and classifications of newborn encephalopathy. Pediatr. Neurol. 8, 85–90. doi: 10.1016/0887-8994(92)90026-u
López-Pérez, S. J., Morales-Villagrán, A., Ventura-Valenzuela, J., and Medina-Ceja, L. (2012). Short- and long-term changes in extracellular glutamate and acetylcholine concentrations in the rat hippocampus following hypoxia. Neurochem. Int. 61, 258–265. doi: 10.1016/j.neuint.2012.03.009
Lowe, D. W., Fraser, J. L., Rollins, L. G., Bentzley, J., Nie, X., Martin, R., et al. (2017). Vitamin D improves functional outcomes in neonatal hypoxic ischemic male rats treated with N-acetylcysteine and hypothermia. Neuropharmacology 123, 186–200. doi: 10.1016/j.neuropharm.2017.06.004
Magno, L. A. V., Collodetti, M., Tenza-Ferrer, H., and Romano-Silva, M. A. (2019). Cylinder test to assess sensory-motor function in a mouse model of Parkinson's disease. Bio. Protoc. 9:e3337. doi: 10.21769/BioProtoc.3337
McNamara, C. G., Tejero-Cantero, Á., Trouche, S., Campo-Urriza, N., and Dupret, D. (2014). Dopaminergic neurons promote hippocampal reactivation and spatial memory persistence. Nat. Neurosci. 17, 1658–1660. doi: 10.1038/nn.3843
Mikati, M. A., Zeinieh, M. P., Kurdi, R. M., Harb, S. A., El Hokayem, J. A., Daderian, R. H., et al. (2005). Long-term effects of acute and of chronic hypoxia on behavior and on hippocampal histology in the developing brain. Brain Res. Dev. Brain Res. 157, 98–102. doi: 10.1016/j.devbrainres.2005.03.007
Millar, L. J., Shi, L., Hoerder-Suabedissen, A., and Molnár, Z. (2017). Neonatal hypoxia Ischaemia: mechanisms, models, and therapeutic challenges. Front. Cell. Neurosci. 11:78. doi: 10.3389/fncel.2017.00078
Money, K. M., and Stanwood, G. D. (2013). Developmental origins of brain disorders: roles for dopamine. Front. Cell. Neurosci. 7:260. doi: 10.3389/fncel.2013.00260
Nagai, T., Takuma, K., Kamei, H., Ito, Y., Nakamichi, N., Ibi, D., et al. (2007). Dopamine D1 receptors regulate protein synthesis-dependent long-term recognition memory via extracellular signal-regulated kinase 1/2 in the prefrontal cortex. Learn. Mem. 14, 117–125. doi: 10.1101/lm.461407
Nalivaeva, N. N., Turner, A. J., and Zhuravin, I. A. (2018). Role of prenatal hypoxia in brain development, cognitive functions and neurodegeneration. Front. Neurosci. 12:825. doi: 10.3389/fnins.2018.00825
Ordyan, N. E., Akulova, V. K., Mironova, V. I., and Otellin, V. A. (2017). Behavior disorders caused by perinatal hypoxia in juvenile rats and their correction with GABA derivative. Bull. Exp. Biol. Med. 164, 118–122. doi: 10.1007/s10517-017-3938-0
Pagida, M. A., Konstantinidou, A. E., Tsekoura, E., Mangoura, D., Patsouris, E., and Panayotacopoulou, M. T. (2013). Vulnerability of the mesencephalic dopaminergic neurons of the human neonate to prolonged perinatal hypoxia: an immunohistochemical study of tyrosine hydroxylase expression in autopsy material. J. Neuropathol. Exp. Neurol. 72, 337–350. doi: 10.1097/NEN.0b013e31828b48b3
Papadopoulos, G. C., and Parnavelas, J. G. (1990). Distribution and synaptic organization of dopaminergic axons in the lateral geniculate nucleus of the rat. J. Comp. Neurol. 294, 356–361. doi: 10.1002/cne.902940305
Penny, T. R., Pham, Y., Sutherland, A. E., Smith, M. J., Lee, J., Jenkin, G., et al. (2021). Optimization of behavioral testing in a long-term rat model of hypoxic ischemic brain injury. Behav. Brain Res. 409:113322. doi: 10.1016/j.bbr.2021.113322
Piešová, M., and Mach, M. (2020). Impact of perinatal hypoxia on the developing brain. Physiol. Res. 69, 199–213. doi: 10.33549/physiolres.934198
Pin, T. W., Eldridge, B., and Galea, M. P. (2009). A review of developmental outcomes of term infants with post-asphyxia neonatal encephalopathy. Eur. J. Paediatr. Neurol. 13, 224–234. doi: 10.1016/j.ejpn.2008.05.001
Pozdnyakova, N., Yatsenko, L., Parkhomenko, N., and Himmelreich, N. (2011). Perinatal hypoxia induces a long-lasting increase in unstimulated gaba release in rat brain cortex and hippocampus. The protective effect of pyruvate. Neurochem. Int. 58, 14–21. doi: 10.1016/j.neuint.2010.10.004
Raghuraman, G., Rai, V., Peng, Y. J., Prabhakar, N. R., and Kumar, G. K. (2009). Pattern-specific sustained activation of tyrosine hydroxylase by intermittent hypoxia: role of reactive oxygen species-dependent downregulation of protein phosphatase 2A and upregulation of protein kinases. Antioxid. Redox Signal. 11, 1777–1789. doi: 10.1089/ars.2008.2368
Raveendran, A. T., and Skaria, P. C. (2013). Learning and cognitive deficits in hypoxic neonatal rats intensified by BAX mediated apoptosis: protective role of glucose, oxygen, and epinephrine. Int. J. Neurosci. 123, 80–88. doi: 10.3109/00207454.2012.731457
Richman, C. L., Dember, W. N., and Kim, P. (1986). Spontaneous alternation behavior in animals: a review. Curr. Psychol. Res. Rev. 5, 358–391. doi: 10.1007/BF02686603
Rogalska, J., Caputa, M., Wentowska, K., and Nowakowska, A. (2004). Stress-induced behaviour in juvenile rats: effects of neonatal asphyxia, body temperature and chelation of iron. Behav. Brain Res. 154, 321–329. doi: 10.1016/j.bbr.2004.02.020
Rosen, Z. B., Cheung, S., and Siegelbaum, S. A. (2015). Midbrain dopamine neurons bidirectionally regulate CA3-CA1 synaptic drive. Nat. Neurosci. 18, 1763–1771. doi: 10.1038/nn.4152
Sab, I. M., Ferraz, M. M., Amaral, T. A., Resende, A. C., Ferraz, M. R., Matsuura, C., et al. (2013). Prenatal hypoxia, habituation memory and oxidative stress. Pharmacol. Biochem. Behav. 107, 24–28. doi: 10.1016/j.pbb.2013.04.004
Sanches, E. F., Durán-Carabali, L. E., Tosta, A., Nicola, F., Schmitz, F., Rodrigues, A., et al. (2017). Pregnancy swimming causes short- and long-term neuroprotection against hypoxia-ischemia in very immature rats. Pediatr. Res. 82, 544–553. doi: 10.1038/pr.2017.110
Sánchez-González, M. A., García-Cabezas, M. A., Rico, B., and Cavada, C. (2005). The primate thalamus is a key target for brain dopamine. J. Neurosci. 25, 6076–6083. doi: 10.1523/JNEUROSCI.0968-05.2005
Savage, L. M., Castillo, R., and Langlais, P. J. (1998). Effects of lesions of thalamic intralaminar and midline nuclei and internal medullary lamina on spatial memory and object discrimination. Behav. Neurosci. 112, 1339–1352. doi: 10.1037//0735-7044.112.6.1339
Savourey, G., Launay, J. C., Besnard, Y., Guinet, A., and Travers, S. (2003). Normo- and hypobaric hypoxia: are there any physiological differences? Eur. J. Appl. Physiol. 89, 122–126. doi: 10.1007/s00421-002-0789-8
Schallert, T., Fleming, S. M., Leasure, J. L., Tillerson, J. L., and Bland, S. T. (2000). CNS plasticity and assessment of forelimb sensorimotor outcome in unilateral rat models of stroke, cortical ablation, parkinsonism and spinal cord injury. Neuropharmacology 39, 777–787. doi: 10.1016/s0028-3908(00)00005-8
Seidler, F. J., and Slotkin, T. A. (1990). Effects of acute hypoxia on neonatal rat brain: regionally selective, long-term alterations in catecholamine levels and turnover. Brain Res. Bull. 24, 157–161. doi: 10.1016/0361-9230(90)90200-j
Sherman, S. M. (2005). Thalamic relays and cortical functioning. Prog. Brain Res. 149, 107–126. doi: 10.1016/S0079-6123(05)49009-3
Shibata, H. (1993). Efferent projections from the anterior thalamic nuclei to the cingulate cortex in the rat. J. Comp. Neurol. 330, 533–542. doi: 10.1002/cne.903300409
Shimomura, C., and Ohta, H. (1988). Behavioral abnormalities and seizure susceptibility in rat after neonatal anoxia. Brain Dev. 10, 160–163. doi: 10.1016/s0387-7604(88)80020-2
Spahic, H., Parmar, P., Miller, S., Emerson, P. C., Lechner, C., St Pierre, M., et al. (2022). Dysregulation of ErbB4 signaling pathway in the dorsal Hippocampus after neonatal hypoxia-ischemia and late deficits in PV+ interneurons, synaptic plasticity and working memory. Int. J. Mol. Sci. 24:508. doi: 10.3390/ijms24010508
Speiser, Z., Korczyn, A. D., Teplitzky, I., and Gitter, S. (1983). Hyperactivity in rats following postnatal anoxia. Behav. Brain Res. 7, 379–382. doi: 10.1016/0166-4328(83)90028-1
Sturman, O., Germain, P. L., and Bohacek, J. (2018). Exploratory rearing: a context- and stress-sensitive behavior recorded in the open-field test. Stress 21, 443–452. doi: 10.1080/10253890.2018.1438405
Svenningsson, P., Nishi, A., Fisone, G., Girault, J. A., Nairn, A. C., and Greengard, P. (2004). DARPP-32: an integrator of neurotransmission. Annu. Rev. Pharmacol. Toxicol. 44, 269–296. doi: 10.1146/annurev.pharmtox.44.101802.121415
Tata, D. A., Markostamou, I., Ioannidis, A., Gkioka, M., Simeonidou, C., Anogianakis, G., et al. (2015). Effects of maternal separation on behavior and brain damage in adult rats exposed to neonatal hypoxia-ischemia. Behav. Brain Res. 280, 51–61. doi: 10.1016/j.bbr.2014.11.033
Tejkalová, H., Kaiser, M., Klaschka, J., and Stastný, F. (2007). Does neonatal brain ischemia induce schizophrenia-like behavior in young adult rats? Physiol. Res. 56, 815–823. doi: 10.33549/physiolres.931056
Thompson, S. M., Berkowitz, L. E., and Clark, B. J. (2018). Behavioral and neural subsystems of rodent exploration. Learn. Motiv. 61, 3–15. doi: 10.1016/j.lmot.2017.03.009
Trnski, S., Nikolić, B., Ilic, K., Drlje, M., Bobic-Rasonja, M., Darmopil, S., et al. (2022). The signature of moderate perinatal hypoxia on cortical organization and behavior: altered PNN-Parvalbumin interneuron connectivity of the cingulate circuitries. Front. Cell Dev. Biol. 10:810980. doi: 10.3389/fcell.2022.810980
van Groen, T., Kadish, I., and Wyss, J. M. (2002). The role of the laterodorsal nucleus of the thalamus in spatial learning and memory in the rat. Behav. Brain Res. 136, 329–337. doi: 10.1016/s0166-4328(02)00199-7
van Groen, T., and Wyss, J. M. (1995). Projections from the anterodorsal and anteroventral nucleus of the thalamus to the limbic cortex in the rat. J. Comp. Neurol. 358, 584–604. doi: 10.1002/cne.903580411
Varela, C. (2014). Thalamic neuromodulation and its implications for executive networks. Front. Neural Circuits 8:69. doi: 10.3389/fncir.2014.00069
Vázquez-Borsetti, P., Peña, E., Rojo, Y., Acuña, A., and Loidl, F. C. (2019). Deep hypothermia reverses behavioral and histological alterations in a rat model of perinatal asphyxia. J. Comp. Neurol. 527, 362–371. doi: 10.1002/cne.24539
Venkatraman, A., Edlow, B. L., and Immordino-Yang, M. H. (2017). The brainstem in emotion: a review. Front. Neuroanat. 11:15. doi: 10.3389/fnana.2017.00015
Walaas, S. I., Hemmings, H. C. Jr., Greengard, P., and Nairn, A. C. (2011). Beyond the dopamine receptor: regulation and roles of serine/threonine protein phosphatases. Front. Neuroanat. 5:50. doi: 10.3389/fnana.2011.00050
Wolff, M., Morceau, S., Folkard, R., Martin-Cortecero, J., and Groh, A. (2021). A thalamic bridge from sensory perception to cognition. Neurosci. Biobehav. Rev. 120, 222–235. doi: 10.1016/j.neubiorev.2020.11.013
Yamada, K. Y., Satake, S. Y., Perry, J. C., Garcia, K. O., D'Almeida, V., Tufik, S., et al. (2014). Effect of postnatal intermittent hypoxia on locomotor activity and neuronal development in rats tested in early adulthood. Psychol. Neurosci. 7, 125–130. doi: 10.3922/j.psns.2014.02.03
Yamamoto, K., and Vernier, P. (2011). The evolution of dopamine systems in chordates. Front. Neuroanat. 5:21. doi: 10.3389/fnana.2011.00021
Yan, E. B., Hellewell, S. C., Bellander, B. M., Agyapomaa, D. A., and Morganti-Kossmann, M. C. (2011). Post-traumatic hypoxia exacerbates neurological deficit, neuroinflammation and cerebral metabolism in rats with diffuse traumatic brain injury. J. Neuroinflammation 8:147. doi: 10.1186/1742-2094-8-147
Zhang, B., Ban, D., Gou, X., Zhang, Y., Yang, L., Chamba, Y., et al. (2019). Genome-wide DNA methylation profiles in Tibetan and Yorkshire pigs under high-altitude hypoxia. J. Anim. Sci. Biotechnol. 10:25. doi: 10.1186/s40104-019-0316-y
Keywords: catecholamine, brainstem, thalamus, non-invasive model, DARPP-32, cognitive impairment
Citation: Nikolic B, Trnski-Levak S, Kosic K, Drlje M, Banovac I, Hranilovic D and Jovanov-Milosevic N (2024) Lasting mesothalamic dopamine imbalance and altered exploratory behavior in rats after a mild neonatal hypoxic event. Front. Integr. Neurosci. 17:1304338. doi: 10.3389/fnint.2023.1304338
Edited by:
Denise Manahan-Vaughan, Ruhr University Bochum, GermanyReviewed by:
Jong-Hyun Son, University of Scranton, United StatesPenha Cristina Barradas, Rio de Janeiro State University, Brazil
Copyright © 2024 Nikolic, Trnski-Levak, Kosic, Drlje, Banovac, Hranilovic and Jovanov-Milosevic. This is an open-access article distributed under the terms of the Creative Commons Attribution License (CC BY). The use, distribution or reproduction in other forums is permitted, provided the original author(s) and the copyright owner(s) are credited and that the original publication in this journal is cited, in accordance with accepted academic practice. No use, distribution or reproduction is permitted which does not comply with these terms.
*Correspondence: Dubravka Hranilovic, dubravka.hranilovic@biol.pmf.unizg.hr; Natasa Jovanov-Milosevic, njovanov@hiim.hr
†These authors have contributed equally to this work and share first authorship