- 1PET Center, Huashan Hospital, Fudan University, Shanghai, China
- 2Laboratory of Molecular Neural Biology, School of Life Sciences, Shanghai University, Shanghai, China
- 3Department of Neurosurgery, Ruijin Hospital, Shanghai Jiao Tong University School of Medicine, Shanghai, China
Diabetes mellitus (DM) and Parkinson’s disease (PD) have been and will continue to be two common chronic diseases globally that are difficult to diagnose during the prodromal phase. Current molecular genetics, cell biological, and epidemiological evidences have shown the correlation between PD and DM. PD shares the same pathogenesis pathways and pathological factors with DM. In addition, β-cell reduction, which can cause hyperglycemia, is a striking feature of DM. Recent studies indicated that hyperglycemia is highly relevant to the pathologic changes in PD. However, further correlation between DM and PD remains to be investigated. Intriguingly, polycystic monoamine transporter 2 (VMAT2), which is co-expressed in dopaminergic neurons and β cells, is responsible for taking up dopamine into the presynaptic vesicles and can specifically bind to the β cells. Furthermore, we have summarized the specific molecular and diagnostic functions of VMAT2 for the two diseases reported in this review. Therefore, VMAT2 can be applied as a target probe for positron emission tomography (PET) imaging to detect β-cell and dopamine level changes, which can contribute to the diagnosis of DM and PD during the prodromal phase. Targeting VMAT2 with the molecular probe 18F-FP-(+)-DTBZ can be an entry point for the β cell mass (BCM) changes in DM at the molecular level, to clarify the potential relationship between DM and PD. VMAT2 has promising clinical significance in investigating the pathogenesis, early diagnosis, and treatment evaluation of the two diseases.
Introduction
Parkinson’s disease (PD) is the most common progressive neurodegenerative disorder and is characterized by severe motor and non-motor symptoms including uncontrollable tremor, bradykinesia, rigidity, and sleep disturbances. More than 6 million individuals worldwide have PD (Armstrong and Okun, 2020). However, in the prodromal phase, diagnosis based on the clinical profiles is difficult. Diabetes mellitus (DM) is a metabolic disorder characterized by an absolute or relative deficiency of β cell mass (BCM), which manifests as persistent hyperglycemia (Jonietz, 2012; Cinti et al., 2016). DM can be largely classified into type I and type II. According to a 2019 epidemiological survey, the global prevalence of diabetes was an estimated 9.3% (463 million people) at publication, and it was predicted to rise to 10.2% (578 million) by 2030 and 10.9% (700 million) by 2045. The prevailing evidence points to diabetes accounting for a considerable global burden of chronic illness in aging societies (Saeedi et al., 2019; Sinclair et al., 2020).
Both PD and DM are highly prevalent. Previous research suggests that DM predisposes toward a Parkinson-like pathology and induces a more aggressive phenotype in patients already ill with PD (Pagano et al., 2018). In addition, studies have shown that these two diseases exhibit common pathogenic and pathological changes. Moreover, studies have demonstrated that hyperglycemia, which is caused by decreased BCM in DM patients, may lead to the occurrence of PD or aggravate PD symptoms.
The β-cell marker, vesicular monoamine transporter-2 (VMAT2), is closely linked to the occurrence of PD. Therefore, detecting progressive changes in BCM can both assist in the diagnosis of PD and clarify the pathogenesis of DM. Positron–emission tomography (PET) imaging using probes targeting VMAT2 has been applied to the diagnosis of PD and DM. Because of the low abundance of β cells, researchers use a notably sensitive tracer, an 18F-labeled dihydrotetrabenazine derivative (18F-FP-(+)-DTBZ), in their study (Wu et al., 2015). Researchers have used VMAT2 imaging with 18F-FP-(+)-DTBZ to identify DM as a risk factor for PD. In summary, this probe may, in the future, reveal the pathogenesis of DM and permit the early diagnosis of PD.
Molecular Genetics, Cell Biology, and Epidemiology: Correlation Between PD and DM
Studies in molecular genetics, cell biology, and epidemiology have shown that the pathogeneses of PD and DM have common characteristics. Approximately 60% of patients with PD have impaired insulin signaling and impaired glucose tolerance (Santiago and Potashkin, 2014). Moreover, 62% of patients with PD and dementia have insulin resistance, and 30% of these patients also have impaired glucose tolerance (Bosco et al., 2012). PD is aggravated if the onset of comorbid DM is earlier than that of PD (Cereda et al., 2012). In the pathogenesis and progression of PD and DM, insulin and dopamine are regulated mutually, and hypoinsulinemia induced by streptozotocin can reduce the levels of dopamine transporter (DAT) and tyrosine hydroxylase (TH) transcription in the substantia nigra pars compacta (Lima et al., 2014). In addition, reduction of dopamine in the striatum can attenuate insulin signaling in the basal ganglia region. Pathways common to both diseases are mitochondrial dysfunction, endoplasmic reticulum stress, inflammatory response, vitamin D deficiency, and ubiquitin-protease/autophagy-lysosomal-system dysfunction (Table 1). Peroxisome proliferator-activated receptor gamma coactivator 1-alpha (PGC-1α) is a key regulator of the enzymes involved in the mitochondrial respiratory chain and of insulin resistance and plays an important role in the pathogenesis of both DM and PD. In addition, ATP-sensitive K+ channels, AMP-activated protein kinases, glucagon-like peptide-1, and dipeptidyl peptidase enzyme 4 show common pathological changes in DM and PD. Hyperglycemia can cause disturbances in the energy metabolism in the brain, damage neurons through various injury mechanisms, and lead to abnormal expression of proteins in the striatum and hippocampus. Consequently, recent studies have focused on the relationship between PD and hyperglycemia. One study found that thioredoxin-interacting protein (TXNIP), an endogenous inhibitor of reactive oxygen species elimination, regulates Parkin/PINK1-mediated mitophagy in dopaminergic neurons under high-glucose conditions (Su et al., 2020), which revealed the neuronal impact of mitochondrial dysfunction caused by hyperglycemia and hyperglycemia-induced oxidative stress. In addition, the preferential occurrence of nigrostriatal dopaminergic neurodegeneration in long-term hyperglycemia suggests that hyperglycemia causes premature aging of the central nervous system, fostering the development of age-related neurodegenerative diseases (Renaud et al., 2018). However, these studies did not sufficiently clarify the molecular link between hyperglycemia and PD. Further research on this topic will help elucidate the pathogenesis of PD, which is important for better clinical diagnosis, prevention, and treatment.
Both type 2 diabetes (T2DM) and PD are involved in the accumulation of misfolded proteins in amyloid aggregates. In diabetic patients, the accumulation of islet amyloid polypeptide (IAPP) in pancreatic cells can cause cell dysfunction. In patients with PD, α-synuclein eventually aggregates into the Lewy bodies (Wang and Raleigh, 2014; Bridi and Hirth, 2018). Researchers have found that IAPP and α-synuclein cross-interact in the two diseases, and IAPP in patients with T2DM can promote α-synuclein aggregation, which leads to the occurrence of PD (Horvath and Wittung-Stafshede, 2016). Another study showed that insulin-degrading enzymes (IDE) can bind to synuclein oligomers to prevent further aggregation. Moreover, insulin resistance can inhibit IDE and promote the formation of α-synuclein fibrils, which may enhance PD progression (Sharma et al., 2015).
1-Methyl-4-phenyl-1,2,3,6-tetrahydropyridine (MPTP) can lead to neuronal death by inhibiting the mitochondrial respiratory chain enzyme, which is a PD animal model inducer (Jing et al., 2017). In addition, studies have shown that mitochondrial dysfunction occurs in patients with DM and PD, and insulin resistance in diabetic mice can lead to mitochondrial destruction and dopaminergic neuron degeneration (Khang et al., 2015).
According to many case–control studies, longitudinal studies, and meta-analyses, DM is a risk factor for PD (some of these results are shown in Table 2). DM can accelerate the development and progression of PD, especially in young women aged between 8 and 12 years (Sun et al., 2012; Aviles-Olmos et al., 2013a; Ahn et al., 2015; Santiago et al., 2017; Pagano et al., 2018). Several studies in the late 1990s showed that, in the diabetic mouse, the levels of DAT in the V9 and V10 regions (Del Pino et al., 2017) and in the medial forebrain bundle of the midbrain (Petrisic et al., 1997) were decreased significantly. A positive correlation was established between insulin reduction in DM and decreases in the levels of DAT, VMAT (mainly VMAT2), and TH. Insulin regulates DAT and VMAT2 levels through the PI3K/AKT pathway, and insulin deficiency leads to DAT and VMAT2 dysfunction. Dopamine D2 receptors can also regulate DAT and VMAT2 expression through the ERK signaling pathway (Carvelli et al., 2002; Owens et al., 2012; Samandari et al., 2013; Bini et al., 2019).
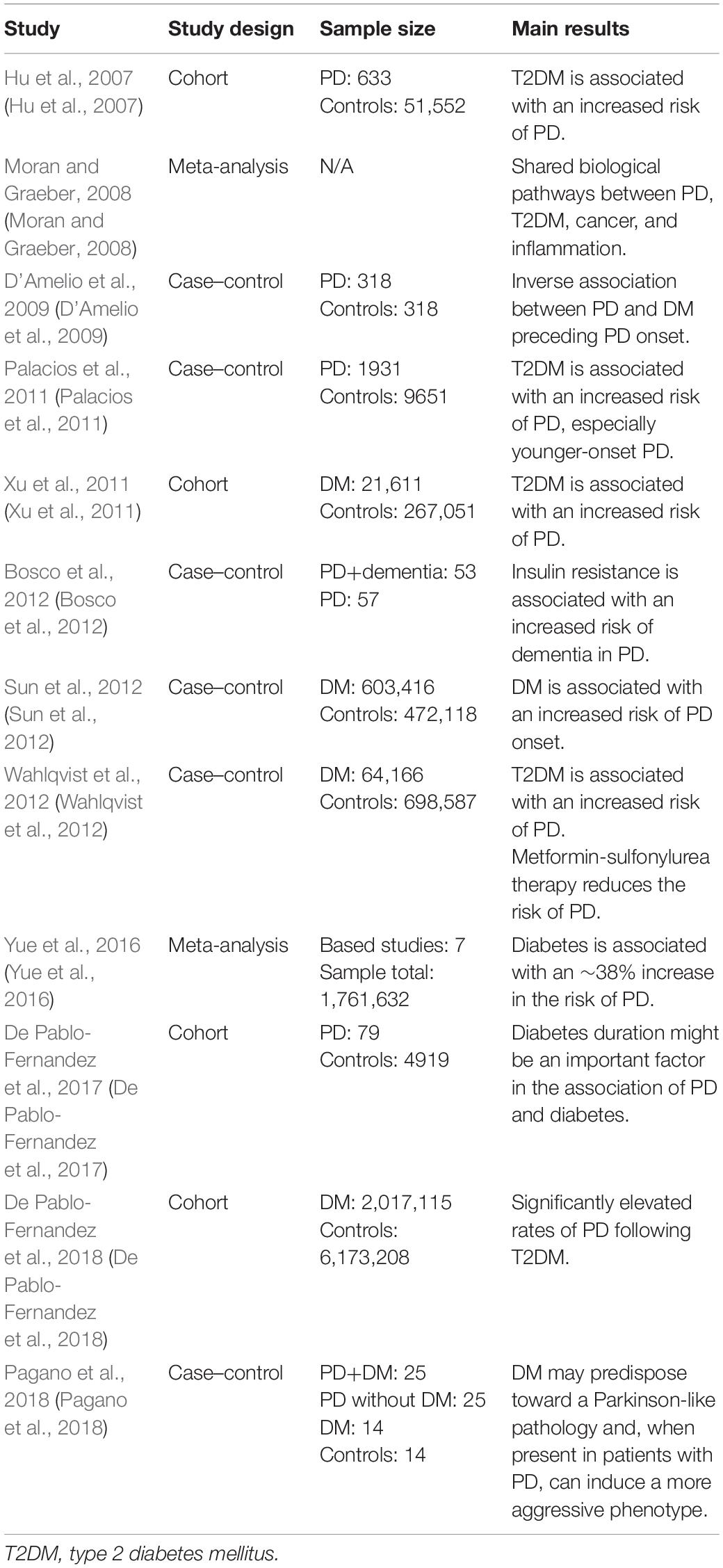
Table 2. Recent studies on the correlation between diabetes mellitus (DM) and Parkinson’s disease (PD).
There are also other molecular targets that tightly link PD and DM. For example, glucose-dependent insulin-promoting polypeptide (GIP) is not only an endogenous hormone of the incretin family but also a neurotrophic factor. It activates cell proliferation and protects the neurons by promoting cell repair and preventing apoptosis, which enhances the survival ability of β-cells in the pancreas and neurons in the brain (Maino et al., 2014; Altmann et al., 2016). Besides, the GIP receptor is a G protein-coupled receptor that belongs to the glucagon family and increases insulin secretion during the onset of hyperglycemia (Park et al., 2013). In addition, studies have revealed that treatment with GIP analogs can increase the level of reduced TH in PD patients (Li et al., 2016).
Another incretin hormone, glucagon-like peptide-1 (GLP-1), which is functionally similar to GIP, also plays a role in DM and PD pathogenesis. The GLP-1 receptor agonists have been approved for the treatment of T2DM and have a good therapeutic effect on the clinical symptoms of PD including motor and cognitive dysfunctions. Their neuroprotective effect is shown in the protection of dopaminergic neurons in the substantia nigra, and they reduce the accumulation of synuclein proteins (Aviles-Olmos et al., 2013b; Ji et al., 2016). In addition, GLP-1 treatment reduces oxidative stress and lipid peroxidation and increases the level of brain-derived neurotrophic factor (BDNF), alleviating the symptoms of neuroinflammation in the brain (Li et al., 2017). Studies on the effects of GLP1 analogs on MPTP rodents have revealed that by inhibiting inflammation and excitatory cytokines and stimulating antioxidant enzymes, striatum dopamine levels can be improved and neuronal damage can be reduced (Elbassuoni and Ahmed, 2019).
After treatment with GLP1/GIP receptor agonists, TH in the brain increases and microglial activation decreases, promoting the production of dopamine and providing neuroprotection for dopaminergic neurons. These two peptides can be used as evidence for the connection between PD and DM.
Although these studies proved that DM is a risk factor for PD, the mechanistic basis of the relationship has not been fully elucidated. In addition, because a deficiency in BCM is the absolute or relative characteristic of DM, early, effective evaluation of BCM levels in patients with DM will be of great significance for the prevention, early diagnosis, and treatment of PD. VMAT2 is expressed in brain monoaminergic neurons, especially in the striatum, which is a regulator of monoaminergic neuronal function. Besides, VMAT2 is also a potential target for the assessment of BCM, and decreases in BCM level in the human pancreases correlate with decreases in insulin level in the blood (Anlauf et al., 2003; Fu et al., 2019). Jiang et al. (2020) found a decreased VMAT2 expression in the striatum due to the β-cell impairment in a type 1 DM (T1DM) rat model. Furthermore, VMAT2 expression in the striatum was also increased with the recovery of BCM and glucose levels in DM rats after streptozotocin injection treatment (Jiang et al., 2020). VMAT2 is, therefore, a key factor in connecting PD and DM.
Considering the correlation between DM and PD, researchers have employed a molecular imaging probe for VMAT2, the PET radioligand [18F]fluoropropyl-(+)-dihydrotetrabenazine (18F-FP-(+)-DTBZ or 18F-AV-133), as a molecular marker that can be used to objectively analyze dopamine and β cells.
VMAT2 Function and Anatomical Distribution
VMAT2, a glycoprotein bound to secretory vesicle membranes and a constitutive protein in humans, is a subtype of the vesicle monoamine transporter (Stahl, 2018). A large number of studies have shown that VMAT2 is pivotal in the pathogenesis of PD (Ma et al., 2019; Shi et al., 2019), which is well known as a disease of dopamine deficiency. In endocrine islets, a large number of genes expressed by β cells have homologs in neural cells, one of which is VMAT2 (Harris et al., 2008). In 2003, Anlauf et al. (2003) determined the distribution of VMAT2 in human and monkey pancreas by immunohistochemistry and in situ hybridization. This was the first confirmation of VMAT2 localized in islet β cells. The monoamine neurotransmitters transported by VMAT2 are important paracrine and/or autocrine regulators of islet β cells and include dopamine, which inhibits insulin release via dopamine D2 receptors on β cells (Pecic et al., 2019). Recent studies have found that VMAT2 also mediates the release of the inhibitory neurotransmitter γ-aminobutyric acid (Tritsch et al., 2012), which plays a protective role by inhibiting β-cell apoptosis (Purwana et al., 2014). Exocytosis or endocytosis of the vesicles is important for the regulation of cellular signal transduction. Although glucose is an important factor in stimulating insulin secretion, VMAT2 also regulates glucose and insulin homeostasis (Raffo et al., 2008; Rodriguez-Diaz et al., 2011). Neuroendocrine cells accumulate biogenic amines and peptides, and the VMAT2 that they express serves to take up monoamines from the cytoplasm, which are then stored in the secretory vesicles for use in signaling (Bernstein et al., 2012). Accumulating evidence shows that VMAT2 is a contributor in PD progression (Ma et al., 2019; Shi et al., 2019). The accumulation of toxic α-synuclein in the prodromal phase of PD can impair VMAT2 activity, which eventually increases the cytosolic levels of dopamine (Bridi and Hirth, 2018). The metabolites of dopamine itself are cytotoxic; thus, an abnormal level of dopamine can exacerbate PD symptoms (Lotharius and Brundin, 2002). Moreover, disturbances of VMAT2 expression decrease neurogenesis in the olfactory nerve, causing hyposmia, which is another symptom of PD (Ma et al., 2019). It is known that the main histopathological change observed in PD is the selective degeneration of dopaminergic neurons in the substantia nigra pars compacta and of dopaminergic nerve endings in the striatum. A previous study identified a correlation between decreased striatal VMAT2 and multiple non-motor symptoms in patients with PD (Shi et al., 2019).
Furthermore, physiological effects of knocking out the VMAT2 mouse model were studied. Homozygous (VMAT2-/-) mice die within a few days after birth due to poor eating. The number of monoaminergic cells in the brain of knockout mice is lower than that of wild-type littermates (Fon et al., 1997). In addition, heterozygosity (VMAT2 +/−) shows low sensitization to dopamine agonist synaptic apomorphine, psychostimulant cocaine, and other neurotoxins (Wang et al., 1997). In VMAT2 knockout mice, disruption of vesicle trafficking leads to enhanced methamphetamine (METH)-induced dopaminergic neurotoxicity. METH produces greater dopamine consumption and metabolite content in the VMAT2 +/− striatum. DAT expression in the striatum of VAT2 +/− mice was further reduced, and the dopamine transporter content was lower than that of the wild type after treatment with METH (Fumagalli et al., 1999). Moreover, neurotoxin MPTP to heterozygotes’ dopamine cells is more than two times lower than that in wild-type mice (Takahashi et al., 1997). These results suggest that monoamine function, post-synaptic sensitization, and neurotoxin mechanism of action are linked to VMAT2 expression. In summary, VMAT2 may play an important role in the pathogenesis of PD.
T1DM is caused by the reductions in BCM due to an autoimmune reaction, resulting in an absolute deficiency of endogenous insulin secretion (Wilcox et al., 2016; Yu et al., 2019). T2DM stems from metabolic disorders and insulin resistance, and the toxic downstream effects of these metabolic disorders can further exacerbate BCM reduction by up to 60% (Ashcroft and Rorsman, 2012; Johnson and Olefsky, 2013; Derakhshan et al., 2015). Significant losses of BCM precede rises in blood glucose; therefore, an in-depth study of β-cell numbers can provide new clues to the pathology, early diagnosis, and treatment of DM. A non-invasive method for determining the residual BCM of patients with DM would be extremely useful. Great efforts have been devoted to improving the diagnostic accuracy of DM/BCM determination using highly β-cell-specific molecular imaging probes. Considering the necessity of tracking dynamic changes in BCM over time, PET imaging seems to be a feasible method for accessing BCM (Wei et al., 2019). Moreover, VMAT2 is one of the β-cell biomarkers, and the binding of VMAT2 with specific radioactive probe substances is well correlated with the amount of insulin secretion observed after a glucose challenge (Naganawa et al., 2018). Compared with the approach of directly determining insulin levels, determining VMAT2 as a proxy for BCM has the advantages of not being affected by insulin secretion, metabolism, inflammation, etc. (Freeby et al., 2012). In addition, multiple molecular probes are available for imaging β cells, including the voltage-dependent Ca2+ channel (VDCC), the glucose transporter (GLUT), the radiotracer 18F-fluorodeoxyglucose (18F-FDG), VMAT2, dihydrotetrabenazine (DTBZ), 5-hydroxytryptophan (5-HTP), and GPL-1 receptor (GLP-1R) (Wei et al., 2019). VMAT2 has high specific binding and a high density in β cells, which makes it a promising target for BCM imaging (Yang et al., 2017). Dopaminergic neuron damage in PD primarily begins with the nigrostriatal neurons, subsequently affecting the nigrostriatal pathway and finally the mesolimbic system. The striatum has the highest VMAT2 level (Hsiao et al., 2014). VMAT2 is located in the presynaptic neurons, which are responsible for storing and packing the neurotransmitter to regulate the cytoplasmic dopamine level (Figure 1; Hefti et al., 2010). Moreover, previous studies have delineated a strong correlation between the density of striatal VMAT2 and the non-motor symptoms of PD (Shi et al., 2019). Therefore, VMAT2 is promising as an imaging target for the diagnosis PD.
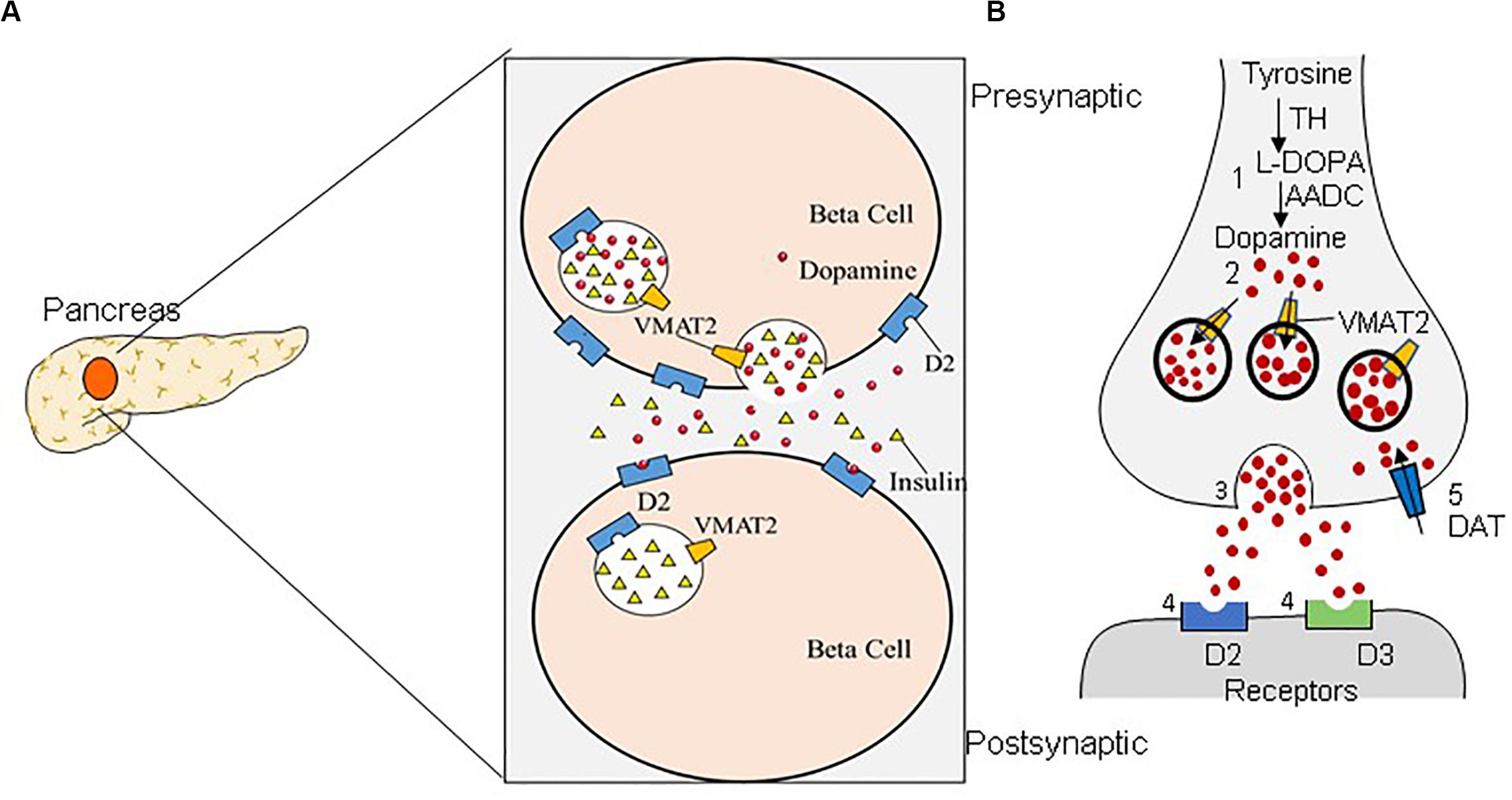
Figure 1. Dual distribution of the vesicular monoamine transporter-2 (VMAT2). VMAT2 is expressed in both β cells (A) and dopaminergic neurons (B). (A) In pancreatic β cells, VMAT2 can accumulate cytoplasmic insulin in secretory vesicles. (B) VMAT2 is also responsible for accumulating cytoplasmic dopamine in synaptic vesicles in the classical dopaminergic neuron in the central nervous system.
Imaging the Monoaminergic System: VMAT2
Role in PD Research
At present, the positron–emission molecular probes used in the diagnosis of PD mainly employ dopaminergic targets and are divided into presynaptic and post-synaptic imaging agents according to their binding targets. Targets on the presynaptic membrane include the following: (1) The dopamine transporter (DAT) of dopaminergic neurons, which transports DA from the synaptic gap back to the presynaptic compartment. Its signal is closely related to the number of dopaminergic neurons. Therefore, DAT imaging can assess the function of dopaminergic nerve endings. 11C-methyl-N-2b-carbomethoxy-3b-(4-fluorophenyl)tropane (11C-CFT) is a DAT radiotracer widely used in clinical practice. However, DAT is commonly downregulated in the early stages of disease, resulting in an overestimation of the amount of degeneration (Lee et al., 2000). (2) L-Aromatic amino acid decarboxylase (AADC) is one of the most important enzymes in dopamine synthesis, being responsible for converting levodopa into dopamine. The activity of AADC is measured by the tracer L-3,4-dihydroxy-6-18F-fluorophenylalanine (18F-DOPA) (Stormezand et al., 2020). (3) VMAT2 is responsible for taking up cytoplasmic dopamine into presynaptic vesicles. The main tracers used are 11C-dihydrotetrabenazine (11C-DTBZ) and 18F-FP-(+)-DTBZ (Bohnen et al., 2006). Furthermore, post-synaptic dopamine receptors are mainly in the D1 family (D1 and D5) and D2 family (D2, D3, and D4). The main tracers used are 11C-FLB 457, 18F-fallypride, and 11C-raclopride (11C-RAC), which bind to D2 (Laruelle, 2000; Ray et al., 2012), and 11C-PHNO, which binds to D3 (Figure 2; Payer et al., 2016).
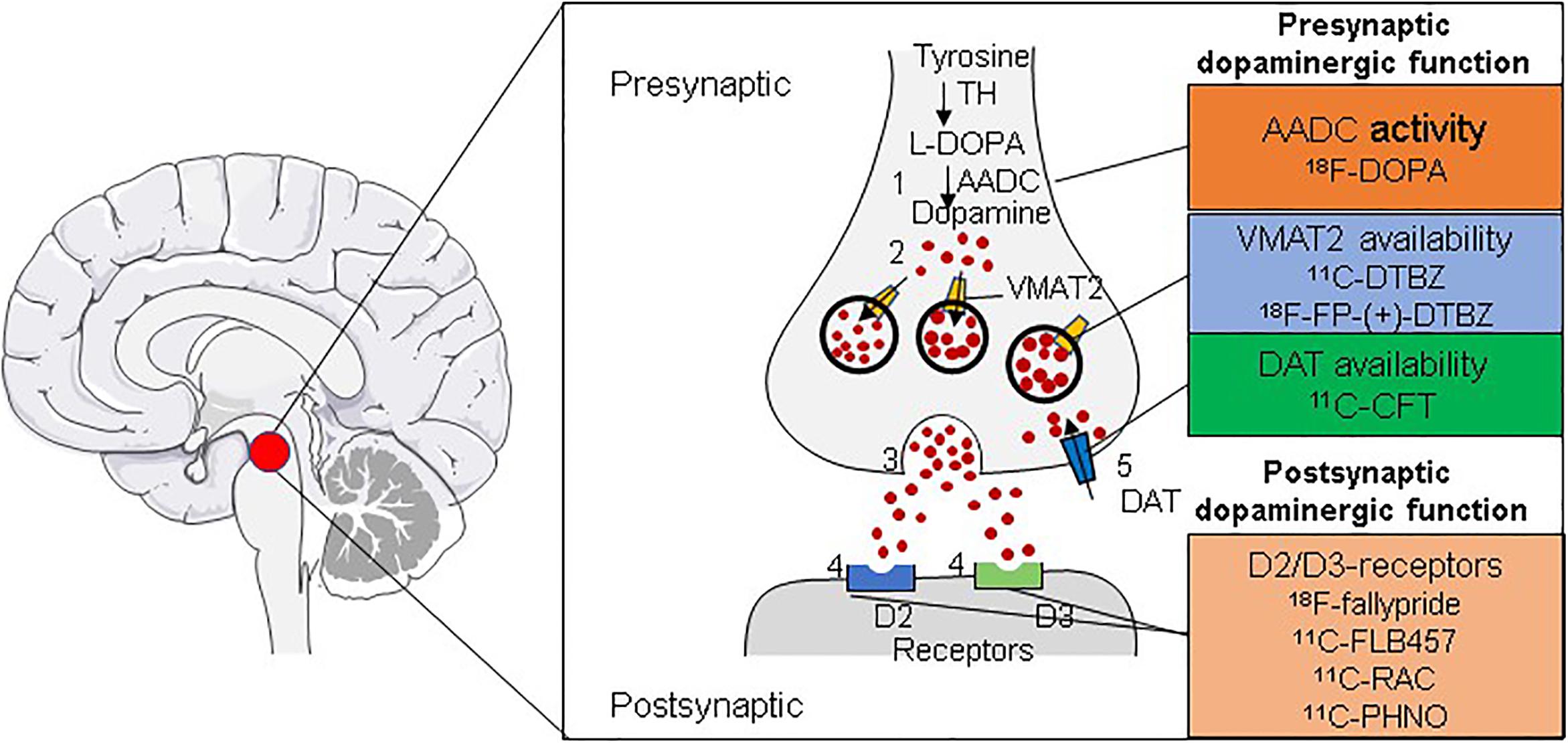
Figure 2. Radiotracers for imaging the dopaminergic system. Shown are the radiotracers used for the in vivo imaging of the dopaminergic system for the diagnosis of PD. Tracers can be used for imaging either presynaptic (AADC, VMAT2, or DAT) or post-synaptic (D2/D3 receptors) dopaminergic function through positron–emission tomography (PET).
The diagnostic imaging agent most used to assess DAT expression in cases of PD is 11C-CFT, but its use is limited by the short half-life of 11C (20 min). Recent studies have shown that, in the primate PD model, the changes in DAT and VMAT2 binding sites in the striatum of surviving substantia nigra neurons are similar, suggesting that targeting VMAT2 with the 18F-labeled dihydrotetrabenazine derivative (18F-FP-(+)-DTBZ) for imaging can provide results similar to those of the DAT imaging presently used to diagnose PD (Lin et al., 2014; Wood, 2014; Cho et al., 2019), but with the further advantage that 18F-FP-(+)-DTBZ imaging can also be used to assess the severity of PD (Hsiao et al., 2014). Another advantage is that it is less affected by compensation or pharmacological regulation (Wilson and Kish, 1996).
18F-FP-(+)-DTBZ in the Diagnosis of DM
11C-Dihydrotetrabenazine (11C-DTBZ) is a specific VMAT2 radioligand currently used in PD research and in clinical imaging of the brain for the diagnosis of PD (Lin et al., 2013). In rodents, a model of type-1 DM showed a good correlation between 11C-DTBZ uptake in the pancreas and blood glucose homeostasis (Naganawa et al., 2016).
A limitation of PET technology in VMAT2 imaging is its low spatial resolution. Moreover, β cells account for a small proportion (1–2%) of the pancreas and are relatively dispersed. To overcome these challenges, it is necessary to further improve the signal-to-noise ratio of the images provided by the radiotracer. Therefore, the 18F-labeled dihydrotetrabenazine derivative 18F-FP-(+)-DTBZ (18F-AV-133), which improves upon certain properties of 11C-DTBZ, is preferred. Compared with 11C-DTBZ, 18F-FP-(+)-DTBZ has better affinity for VMAT2 and lower fat solubility, which translates into less non-specific binding (Wu et al., 2015). In addition, 18F has a longer half-life than 11C, making it suitable for a wider range of applications.
In the most recent primate experiments, the renal cortex, which contains no VMAT2, has been used as a control for non-specific binding in estimations of the specific binding ability of 18F-FP-(+)-DTBZ to VMAT2. Recent experiments on sputum have confirmed that the probe’s specific binding capacity to such samples can reach 85% of that in the striatum, and pancreatic BCM has been successfully reevaluated by PET scanning in humans (Naganawa et al., 2016). 18F-FP-(+)-DTBZ can be both precise and accurate. A quantitative display of PET results, therefore, can be used as a non-invasive means to effectively quantify the BCM and thus the secretion of insulin, which has broad application in the diagnosis, treatment, and monitoring of DM (Singhal et al., 2011). The 18F-FP-(+)-DTBZ-standardized uptake value (SUV), the total volume of distribution, and the binding potential in the pancreas were shown to be reduced by 38, 20, and 40%, respectively, in patients with T1DM compared with those in healthy controls (Normandin et al., 2012). Moreover, the new procedure reduced the patient’s exposure to radioactivity. In addition, the extent of tracer uptake correlated with the rate of insulin secretion. The PET Center of Huashan Hospital in China conducted a study in baboons on the use of 18F-FP-(+)DTBZ for imaging the pancreas and reached similar conclusions (Naganawa et al., 2016).
VMAT2 Imaging Reveals a Correlation Between PD and DM
Using 18F-FP-(+)DTBZ imaging of rat models of T1DM and T2DM, previous studies at the Huashan Hospital PET Center demonstrated that the uptake of 18F-FP-(+)DTBZ in the striatum and the fasting blood glucose of the two groups were significantly negatively correlated. This indicates that BCM is closely related to VMAT2 expression in the brain. At the same time, T2DM caused a decrease in the expression of VMAT2 in the dopaminergic pathway in the brain. In addition, it was found that in the dopamine neurological abnormalities caused by T2DM, the abnormal expression of VMAT2 appeared earlier than that of DAT, and to a greater degree. These results suggest that DM and PD have a common pathogenesis.
Furthermore, imaging of the pancreas in patients with T1DM, patients with T2DM, and normal controls revealed that the uptake of 18F-FP-(+)-DTBZ was significantly lower in T1DM and T2DM than in controls (Figures 3, 4; Donglang et al., 2018; Jianfei et al., 2019). Quantification of 18F-FP-(+)-DTBZ can be used to evaluate BCM in the pancreas of diabetic patients. 18F-FP-(+)-DTBZ imaging in the caudate nucleus and putamen of patients with comorbid PD and T2DM showed that the standardized uptake value ratio (SUVR) of the caudate nucleus in the comorbid group was significantly lower than that of PD patients and normal controls. The SUVR was also lower in the putamen in the comorbid group than in PD patients. This result indicates that T2DM exacerbates the decline in VMAT2 expression in the caudate and putamen of the brain (Figure 5; Jiang et al., 2020). Previous clinical studies have also found that DM aggravates the symptoms of PD (Yue et al., 2016).
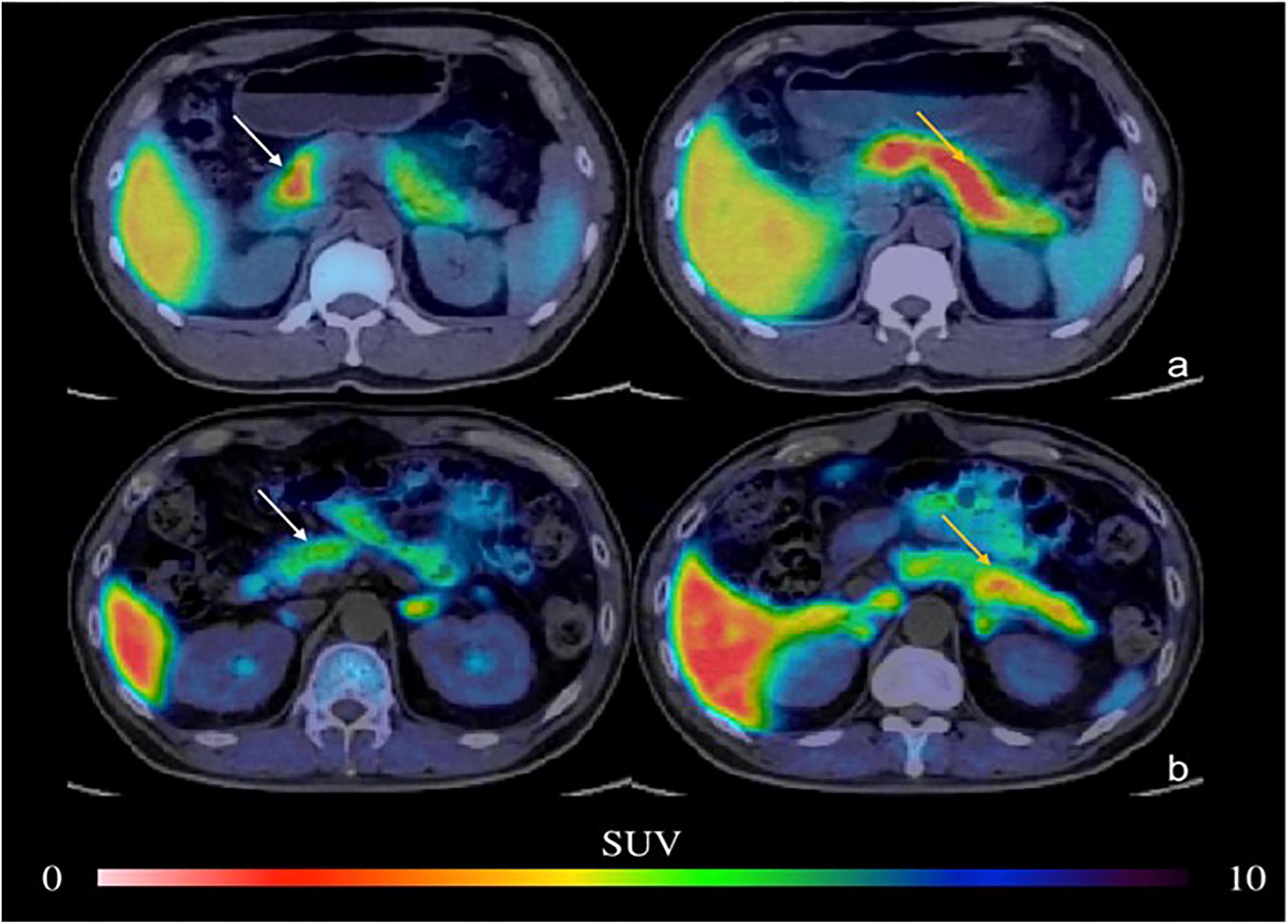
Figure 3. PET imaging of the pancreas in type 2 DM (Donglang et al., 2018) (E-produced/adapted from Chin J Endocrinol Metab, used with permission) Representative PET imaging of the pancreatic head, body, and tail in the baboon in type 2 DM and in healthy controls. (a) Normal pancreas; (b) type 2 DM; white arrow, pancreatic head; yellow arrow, pancreatic body and tail; SUV, standardized uptake value.
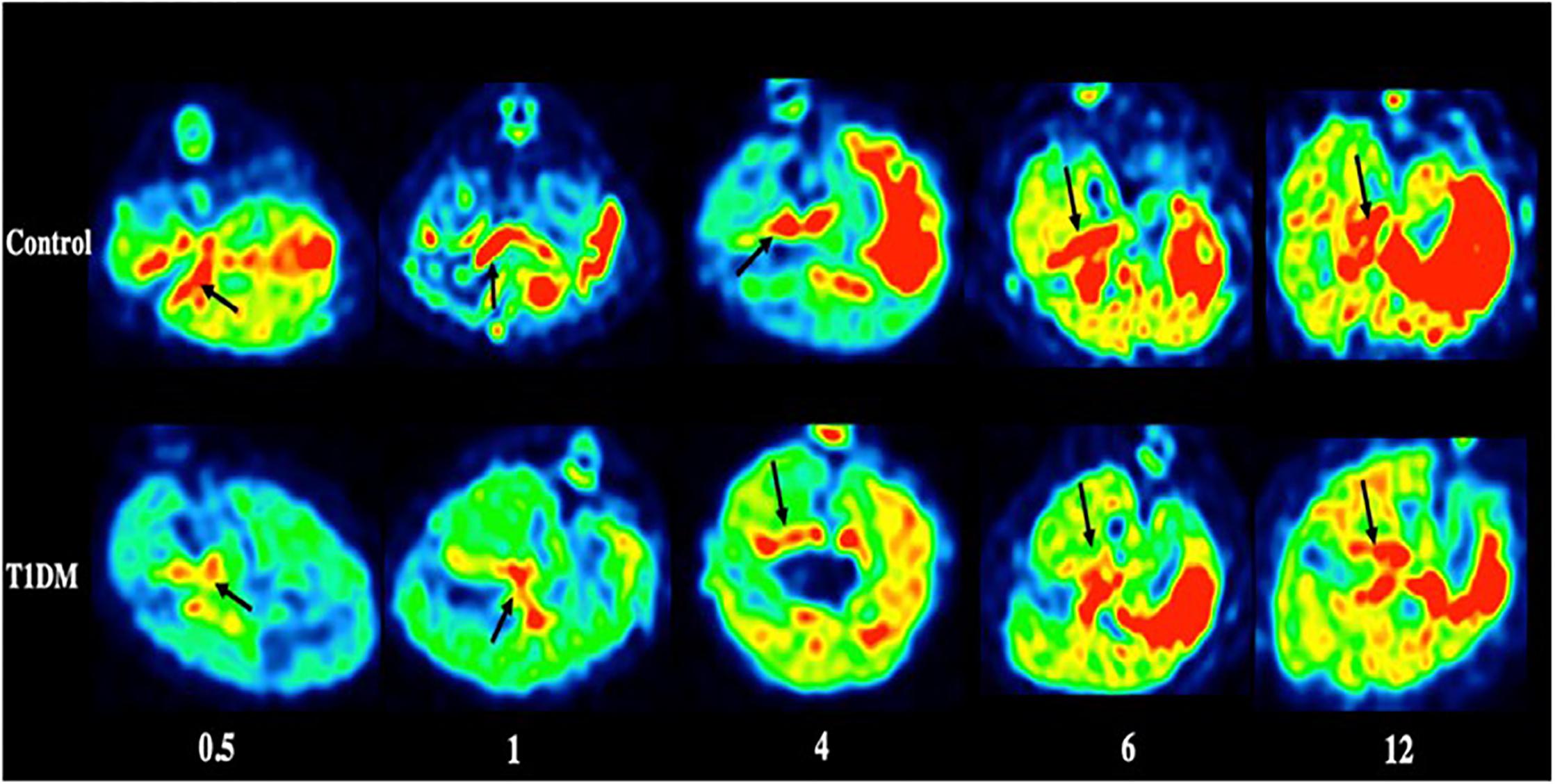
Figure 4. PET imaging of the pancreas in type 1 DM (Jianfei et al., 2019) (E-produced/adapted from Chin J Endocrinol Metab, used with permission) Shown are representative 18F-PF-(+)-DTBZ PET images of the pancreas (black arrows) of rats with type 1 diabetes mellitus (T1DM) and control rats, 0.5, 1, 4, 6, and 12 months after model induction.
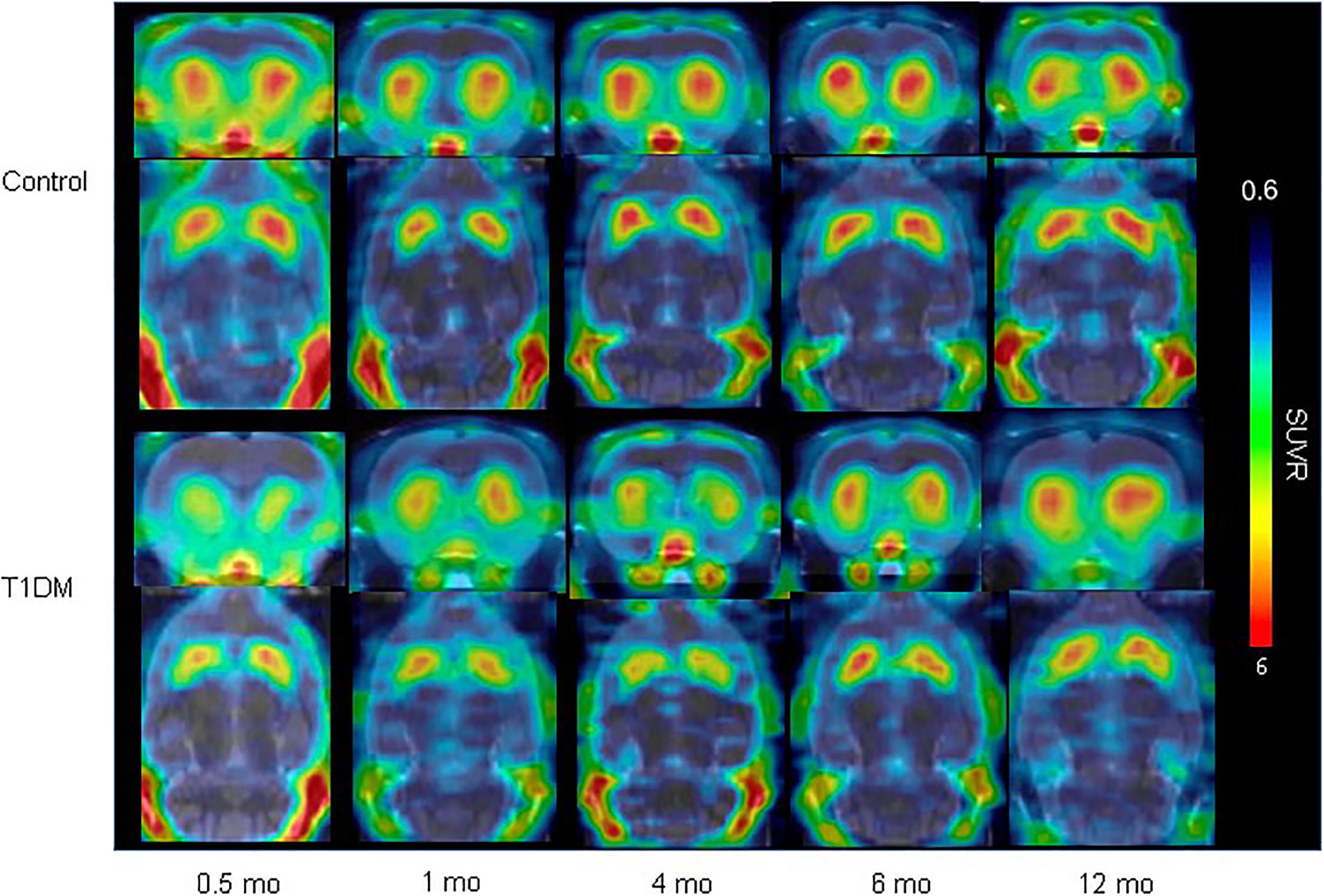
Figure 5. VMAT2 expression in the striatum in type 1 DM (Jiang et al., 2020) (E-produced/adapted from Nuclear Medicine and Biology, used with permission) Shown is the decreased VMAT2 expression in the striatum in a rat model of T1DM, and in control rats, 0.5, 1, 4, 6, and 12 months after model induction, as imaged by 18F-FP-(+)-DTBZ-PET/CT; SUVR, standardized uptake ratio.
As a protein that transports neurotransmitters, the distribution and expression of VMAT2 in human pancreas and its presence in sputum cells have clinical applications. The homology of the human, mouse, and rat VMAT2 amino acid sequences can be above 90% (German et al., 2015; Wu et al., 2016). Thus, studies based on diverse animal models suggest a correlation between BCM reduction and PD in humans (Jiang et al., 2020). In summary, diabetes is a demonstrated risk factor for PD, and a correlation between DM and PD has been established by the above experiments.
Future Perspectives
The physiological function of VMAT2 in islet β cells needs further study to determine the molecular mechanisms affecting BCM and insulin secretion and how VMAT2 participates in regulating the secretion of neurotransmitters. This information will provide new molecular therapeutic targets and an important theoretical basis for the early prediction, diagnosis, and treatment of DM. Future research should use PET scans to compare VMAT2 distributions among patients with T2DM, patients with obesity but without DM, and participants without DM. Undoubtedly, the advantages of using VMAT2 as a BCM marker will lead to useful information.
Positron emission tomography has shown prominent features compared with other molecular imaging approaches. PET can trace suspicious malignant lesions and provide functional imaging of those areas, which is useful for early diagnosis of diseases (Kang et al., 2004; Machtens et al., 2004). Although magnetic resonance imaging (MRI) has a higher resolution, the specificity of its related contrast agents for β cells is not as good as PET imaging. Moreover, the radiation exposure of PET imaging is much lower than that of CT scan. In addition, the evaluation of the BCM level has a significant effect through PET imaging (Wei et al., 2019; Yang et al., 2019). However, the high cost of PET has limited its promotion.
Molecular imaging allows qualitative and quantitative studies of biological processes in vivo at the cellular and molecular levels. The establishment of a functional imaging platform based on PET technology to observe physiological and pathological changes in the BCM can objectively, intuitively, and quantitatively reveal the key factors affecting insulin secretion and insulin resistance. Such a platform will advance our understanding of the pathogenesis of DM and the evaluation of the efficacy of experimental treatments, resulting in great clinical and social benefits.
Conclusion
Targeting VMAT2 with the molecular probe 18F-FP-(+)-DTBZ provides an entry point for revealing the relationship between DM and PD. Interest in the use of imaging methods in research on BCM determination and DM has steadily increased because of their practicality, non-invasiveness, and safety. At present, radionuclide labeling remains the most sensitive imaging method for human β cells. This approach serves to clarify the role of BCM in the pathogenesis and progression of DM and has practical, clinical, and social value for the early diagnosis and treatment of DM.
Author Contributions
YK conceived and designed the idea for the review. YK, HZ, HF, and JZ searched and reviewed the PD and DM literature and drafted the manuscript. YK, JW, and CZ further revised the manuscript. JW, YG, and BS reviewed and edited the manuscript. All authors read and approved the final manuscript.
Funding
This study was supported by the National Natural Science Foundation of China (Project Nos. 81571345 and 81701732), Shanghai Municipal Science and Technology Major Project (No. 2018SHZDZX01) and ZJLab, Shanghai Municipal Key Clinical Specialty (shslczdzk03402).
Conflict of Interest
The authors declare that the research was conducted in the absence of any commercial or financial relationships that could be construed as a potential conflict of interest.
Supplementary Material
The Supplementary Material for this article can be found online at: https://www.frontiersin.org/articles/10.3389/fnins.2020.00682/full#supplementary-material
Abbreviations
11C-CFT, 11C-methyl-N-2b-carbomethoxy-3b-(4-fluorophenyl) tropane; 11C-DTBZ, 11C-dihydrotetrabenazine; 11C-DTBZ, 11C-dihydrotetrabenazine; 11C-RAC, 11C-raclopride; 18F-DOPA, L-3,4-dihydroxy-6-18F-fluorophenylalanine; 18F-FP-(+)-DTBZ, 18F-fluoropropyl-(+)-dihydrotetrabenazine; AADC, L-aromatic amino acid decarboxylase; BCM, β cell mass; DA, dopamine; DAT, dopamine transporter; DM, diabetes mellitus; DTBZ, dihydrotetrabenazine; PD, Parkinson’s disease; PET, positron-emission tomography; SUVR, standardized uptake value ratio; T1DM, type 1 DM; T2DM, type 2 DM; TH, tyrosine hydroxylase; VMAT2, vesicular monoamine transporter-2.
References
Ahn, H. J., Yoo, W. K., Park, J., Ma, H. I., and Kim, Y. J. (2015). Cognitive dysfunction in drug-induced parkinsonism caused by prokinetics and antiemetics. J. Korean Med. Sci. 30, 1328–1333.
Allan, S. M., and Rothwell, N. J. (2001). Cytokines and acute neurodegeneration. Nat. Rev. Neurosci. 2, 734–744. doi: 10.1038/35094583
Altmann, V., Schumacher-Schuh, A. F., Rieck, M., Callegari-Jacques, S. M., Rieder, C. R., and Hutz, M. H. (2016). Val66Met BDNF polymorphism is associated with Parkinson’s disease cognitive impairment. Neurosci. Lett. 615, 88–91. doi: 10.1016/j.neulet.2016.01.030
Anlauf, M., Eissele, R., Schafer, M. K., Eiden, L. E., Arnold, R., Pauser, U., et al. (2003). Expression of the two isoforms of the vesicular monoamine transporter (VMAT1 and VMAT2) in the endocrine pancreas and pancreatic endocrine tumors. J. Histochem. Cytochem. 51, 1027–1040. doi: 10.1177/002215540305100806
Armstrong, M. J., and Okun, M. S. (2020). Diagnosis and treatment of parkinson disease: a review. JAMA 323, 548–560.
Ashcroft, F. M., and Rorsman, P. (2012). Diabetes mellitus and the beta cell: the last ten years. Cell 148, 1160–1171. doi: 10.1016/j.cell.2012.02.010
Aviles-Olmos, I., Dickson, J., Kefalopoulou, Z., Djamshidian, A., Ell, P., Soderlund, T., et al. (2013a). Exenatide and the treatment of patients with Parkinson’s disease. J. Clin. Invest. 123, 2730–2736.
Aviles-Olmos, I., Limousin, P., Lees, A., and Foltynie, T. (2013b). Parkinson’s disease, insulin resistance and novel agents of neuroprotection. Brain 136(Pt 2), 374–384. doi: 10.1093/brain/aws009
Bernstein, A. I., Stout, K. A., and Miller, G. W. (2012). A fluorescent-based assay for live cell, spatially resolved assessment of vesicular monoamine transporter 2-mediated neurotransmitter transport. J. Neurosci. Methods 209, 357–366. doi: 10.1016/j.jneumeth.2012.06.002
Bini, J., Sanchez-Rangel, E., Gallezot, J. D., Naganawa, M., Nabulsi, N. B., Lim, K., et al. (2019). PET imaging of pancreatic dopamine D3/D2 receptor density with (11)C (+)-PHNO in Type-1 Diabetes Mellitus. J. Nucl. Med. 61, 570–576. doi: 10.2967/jnumed.119.234013
Bohnen, N. I., Albin, R. L., Koeppe, R. A., Wernette, K. A., Kilbourn, M. R., Minoshima, S., et al. (2006). Positron emission tomography of monoaminergic vesicular binding in aging and Parkinson disease. J. Cereb. Blood Flow Metab. 26, 1198–1212. doi: 10.1038/sj.jcbfm.9600276
Bonnard, C., Durand, A., Peyrol, S., Chanseaume, E., Chauvin, M. A., Morio, B., et al. (2008). Mitochondrial dysfunction results from oxidative stress in the skeletal muscle of diet-induced insulin-resistant mice. J. Clin. Invest. 118, 789–800.
Bosco, D., Plastino, M., Cristiano, D., Colica, C., Ermio, C., De Bartolo, M., et al. (2012). Dementia is associated with insulin resistance in patients with Parkinson’s disease. J. Neurol. Sci. 315, 39–43.
Boucher, B. J., John, W. G., and Noonan, K. (2004). Hypovitaminosis D is associated with insulin resistance and beta cell dysfunction. Am. J. Clin. Nutr. 80, 1666; author rely1666–1667.
Bridi, J. C., and Hirth, F. (2018). Mechanisms of α-Synuclein induced synaptopathy in Parkinson’s disease. Front. Neurosci. 12:80. doi: 10.3389/fnins.2018.00080
Carvelli, L., Moron, J. A., Kahlig, K. M., Ferrer, J. V., Sen, N., Lechleiter, J. D., et al. (2002). PI 3-kinase regulation of dopamine uptake. J. Neurochem. 81, 859–869. doi: 10.1046/j.1471-4159.2002.00892.x
Cereda, E., Barichella, M., Cassani, E., Caccialanza, R., and Pezzoli, G. (2012). Clinical features of Parkinson disease when onset of diabetes came first: a case-control study. Neurology 78, 1507–1511. doi: 10.1212/wnl.0b013e3182553cc9
Chen, H., O’Reilly, E. J., Schwarzschild, M. A., and Ascherio, A. (2008). Peripheral inflammatory biomarkers and risk of Parkinson’s disease. Am. J. Epidemiol. 167, 90–95. doi: 10.1093/aje/kwm260
Cho, S. S., Christopher, L., Koshimori, Y., Li, C., Lang, A. E., Houle, S., et al. (2019). Decreased pallidal vesicular monoamine transporter type 2 availability in Parkinson’s disease: the contribution of the nigropallidal pathway. Neurobiol. Dis. 124, 176–182. doi: 10.1016/j.nbd.2018.11.022
Cinti, F., Bouchi, R., Kim-Muller, J. Y., Ohmura, Y., Sandoval, P. R., Masini, M., et al. (2016). Evidence of beta-Cell dedifferentiation in human type 2 diabetes. J. Clin. Endocrinol. Metab. 101, 1044–1054.
Cuervo, A. M., Stefanis, L., Fredenburg, R., Lansbury, P. T., and Sulzer, D. (2004). Impaired degradation of mutant alpha-synuclein by chaperone-mediated autophagy. Science 305, 1292–1295. doi: 10.1126/science.1101738
D’Amelio, M., Ragonese, P., Callari, G., Di Benedetto, N., Palmeri, B., Terruso, V., et al. (2009). Diabetes preceding Parkinson’s disease onset. A case-control study. Parkinson. Relat. Disord. 15, 660–664. doi: 10.1016/j.parkreldis.2009.02.013
De Pablo-Fernandez, E., Goldacre, R., Pakpoor, J., Noyce, A. J., and Warner, T. T. (2018). Association between diabetes and subsequent Parkinson disease: a record-linkage cohort study. Neurology 91, e139–e142. doi: 10.1212/wnl.0000000000005771
De Pablo-Fernandez, E., Sierra-Hidalgo, F., Benito-Leon, J., and Bermejo-Pareja, F. (2017). Association between Parkinson’s disease and diabetes: data from NEDICES study. Acta Neurol. Scand. 136, 732–736. doi: 10.1111/ane.12793
Del Pino, J., Moyano, P., Ruiz, M., Anadon, M. J., Diaz, M. J., Garcia, J. M., et al. (2017). Amitraz changes NE, DA and 5-HT biosynthesis and metabolism mediated by alterations in estradiol content in CNS of male rats. Chemosphere 181, 518–529. doi: 10.1016/j.chemosphere.2017.04.113
Derakhshan, A., Tohidi, M., Arshi, B., Khalili, D., Azizi, F., and Hadaegh, F. (2015). Relationship of hyperinsulinaemia, insulin resistance and beta-cell dysfunction with incident diabetes and pre-diabetes: the Tehran lipid and glucose study. Diabet Med. 32, 24–32. doi: 10.1111/dme.12560
Derex, L., and Trouillas, P. (1997). Reversible parkinsonism, hypophosphoremia, and hypocalcemia under vitamin D therapy. Mov. Disord. 12, 612–613. doi: 10.1002/mds.870120424
Donglang, J., Yanyan, K., Xiuhong, L., Ming, L., Weiyan, Z., and Fengchun, H. (2018). Evaluation of β-cell mass in type 2 diabetic patients with [18F]-FP-(+)-DTBZ, a vesicular monoamine transporter type 2 molecular probe. Chin. J. Endocrinol. Metab. 34, 638–642.
Elbassuoni, E. A., and Ahmed, R. F. (2019). Mechanism of the neuroprotective effect of GLP-1 in a rat model of Parkinson’s with pre-existing diabetes. Neurochem. Int. 131:104583. doi: 10.1016/j.neuint.2019.104583
Evatt, M. L., Delong, M. R., Khazai, N., Rosen, A., Triche, S., et al. (2008). Prevalence of vitamin d insufficiency in patients with Parkinson disease and Alzheimer disease. Arch. Neurol. 65, 1348–1352.
Fon, E. A., Pothos, E. N., Sun, B. C., Killeen, N., Sulzer, D., and Edwards, R. H. (1997). Vesicular transport regulates monoamine storage and release but is not essential for amphetamine action. Neuron 19, 1271–1283. doi: 10.1016/s0896-6273(00)80418-3
Freeby, M., Ichise, M., and Harris, P. E. (2012). Vesicular monoamine transporter, type 2 (VMAT2) expression as it compares to insulin and pancreatic polypeptide in the head, body and tail of the human pancreas. Islets 4, 393–397. doi: 10.4161/isl.22995
Fu, J. F., Klyuzhin, I., McKenzie, J., Neilson, N., Shahinfard, E., Dinelle, K., et al. (2019). Joint pattern analysis applied to PET DAT and VMAT2 imaging reveals new insights into Parkinson’s disease induced presynaptic alterations. Neuroimage Clin. 23:101856. doi: 10.1016/j.nicl.2019.101856
Fumagalli, F., Gainetdinov, R. R., Wang, Y. M., Valenzano, K. J., Miller, G. W., and Caron, M. G. (1999). Increased methamphetamine neurotoxicity in heterozygous vesicular monoamine transporter 2 knock-out mice. J. Neurosci. 19, 2424–2431. doi: 10.1523/jneurosci.19-07-02424.1999
German, C. L., Baladi, M. G., McFadden, L. M., Hanson, G. R., and Fleckenstein, A. E. (2015). Regulation of the dopamine and vesicular monoamine transporters: pharmacological targets and implications for disease. Pharmacol. Rev. 67, 1005–1024. doi: 10.1124/pr.114.010397
Harris, P. E., Ferrara, C., Barba, P., Polito, T., Freeby, M., et al. (2008). VMAT2 gene expression and function as it applies to imaging beta-cell mass. J. Mol. Med. 86, 5–16. doi: 10.1007/s00109-007-0242-x
Hefti, F. F., Kung, H. F., Kilbourn, M. R., Carpenter, A. P., Clark, C. M., and Skovronsky, D. M. (2010). 18F-AV-133: a selective VMAT2-binding radiopharmaceutical for PET imaging of dopaminergic neurons. PET Clin. 5, 75–82. doi: 10.1016/j.cpet.2010.02.001
Horvath, I., and Wittung-Stafshede, P. (2016). Cross-talk between amyloidogenic proteins in type-2 diabetes and Parkinson’s disease. Proc. Natl. Acad. Sci. U.S.A. 113, 12473–12477. doi: 10.1073/pnas.1610371113
Hsiao, I. T., Weng, Y. H., Hsieh, C. J., Lin, W. Y., Wey, S. P., Kung, M. P., et al. (2014). Correlation of Parkinson disease severity and 18F-DTBZ positron emission tomography. JAMA Neurol. 71, 758–766.
Hu, G., Jousilahti, P., Bidel, S., Antikainen, R., and Tuomilehto, J. (2007). Type 2 diabetes and the risk of Parkinson’s disease. Diabetes Care 30, 842–847.
Ji, C., Xue, G. F., Lijun, C., Feng, P., Li, D., Li, L., et al. (2016). A novel dual GLP-1 and GIP receptor agonist is neuroprotective in the MPTP mouse model of Parkinson’s disease by increasing expression of BNDF. Brain Res. 1634, 1–11. doi: 10.1016/j.brainres.2015.09.035
Jianfei, X., Donglang, J., Shuhua, R., Qi, H., Fang, X., Yihui, G., et al. (2019). Quantification of β cell mass using18F-FP-(+)-DTBZ, a vesicular monoamine transporter type 2 radiotracer: a longitudinal study in type 1 diabetic rats. Chin. J. Endocrinol. Metab. 35, 494–498.
Jiang, D., Kong, Y., Ren, S., Cai, H., Zhang, Z., Huang, Z., et al. (2020). Decreased striatal vesicular monoamine transporter 2 (VMAT2) expression in a type 1 diabetic rat model: a longitudinal study using micro-PET/CT. Nucl. Med. Biol. 8, 89–95. doi: 10.1016/j.nucmedbio.2020.02.011
Jing, H., Wang, S., Wang, M., Fu, W., Zhang, C., and Xu, D. (2017). Isobavachalcone attenuates MPTP-induced Parkinson’s disease in mice by inhibition of microglial activation through NF-κB pathway. PLoS One 12:e0169560. doi: 10.1371/journal.pone.0169560
Johnson, A. M., and Olefsky, J. M. (2013). The origins and drivers of insulin resistance. Cell 152, 673–684. doi: 10.1016/j.cell.2013.01.041
Kang, D. E., White, R. L. Jr., Zuger, J. H., Sasser, H. C., and Teigland, C. M. (2004). Clinical use of fluorodeoxyglucose F 18 positron emission tomography for detection of renal cell carcinoma. J. Urol. 171, 1806–1809. doi: 10.1097/01.ju.0000120241.50061.e4
Khang, R., Park, C., and Shin, J. H. (2015). Dysregulation of parkin in the substantia nigra of db/db and high-fat diet mice. Neuroscience 294, 182–192. doi: 10.1016/j.neuroscience.2015.03.017
Laruelle, M. (2000). Imaging synaptic neurotransmission with in vivo binding competition techniques: a critical review. J. Cereb. Blood Flow Metab. 20, 423–451. doi: 10.1097/00004647-200003000-00001
Lee, C. S., Samii, A., Sossi, V., Ruth, T. J., Schulzer, M., Holden, J. E., et al. (2000). In vivo positron emission tomographic evidence for compensatory changes in presynaptic dopaminergic nerve terminals in Parkinson’s disease. Ann. Neurol. 47, 493–503. doi: 10.1002/1531-8249(200004)47:4<493::aid-ana13>3.0.co;2-4
Li, Y., Liu, W., Li, L., and Hölscher, C. (2016). Neuroprotective effects of a GIP analogue in the MPTP Parkinson’s disease mouse model. Neuropharmacology 101, 255–263. doi: 10.1016/j.neuropharm.2015.10.002
Li, Y., Liu, W., Li, L., and Holscher, C. (2017). D-Ala2-GIP-glu-PAL is neuroprotective in a chronic Parkinson’s disease mouse model and increases BNDF expression while reducing neuroinflammation and lipid peroxidation. Eur. J. Pharmacol. 797, 162–172. doi: 10.1016/j.ejphar.2016.11.050
Lima, M. M., Targa, A. D., Noseda, A. C., Rodrigues, L. S., Delattre, A. M., dos Santos, F. V., et al. (2014). Does Parkinson’s disease and type-2 diabetes mellitus present common pathophysiological mechanisms and treatments? CNS Neurol. Disord. Drug Targets 13, 418–428. doi: 10.2174/18715273113126660155
Lin, K. J., Weng, Y. H., Hsieh, C. J., Lin, W. Y., Wey, S. P., Kung, M. P., et al. (2013). Brain imaging of vesicular monoamine transporter type 2 in healthy aging subjects by 18F-FP-(+)-DTBZ PET. PLoS One 8:e75952. doi: 10.1371/journal.pone.0075952
Lin, S. C., Lin, K. J., Hsiao, I. T., Hsieh, C. J., Lin, W. Y., Lu, C. S., et al. (2014). In vivo detection of monoaminergic degeneration in early Parkinson disease by (18)F-9-fluoropropyl-(+)-dihydrotetrabenzazine PET. J. Nucl. Med. 55, 73–79. doi: 10.2967/jnumed.113.121897
Lotharius, J., and Brundin, P. (2002). Pathogenesis of Parkinson’s disease: dopamine, vesicles and alpha-synuclein. Nat. Rev. Neurosci. 3, 932–942. doi: 10.1038/nrn983
Ma, K., Han, C., Zhang, G., Guo, X., Xia, Y., Wan, F., et al. (2019). Reduced VMAT2 expression exacerbates the hyposmia in the MPTP model of Parkinson’s disease. Biochem. Biophys. Res. Commun. 513, 306–312. doi: 10.1016/j.bbrc.2019.03.159
Machtens, S., Boerner, A. R., Hofmann, M., Knapp, W. H., et al. (2004). [Positron emission tomography (PET) for diagnosis and monitoring of treatment for urological tumors]. Urol. A 43, 1397–1409.
Maino, B., Ciotti, M. T., Calissano, P., and Cavallaro, S. (2014). Transcriptional analysis of apoptotic cerebellar granule neurons following rescue by gastric inhibitory polypeptide. Int. J. Mol. Sci. 15, 5596–5622. doi: 10.3390/ijms15045596
Matos, M., Dublecz, K., Grafl, B., Liebhart, D., and Hess, M. (2018). Pancreatitis is an important feature of broilers suffering from inclusion body hepatitis leading to dysmetabolic conditions with consequences for zootechnical performance. Avian. Dis. 62, 57–64.
Moran, L. B., and Graeber, M. B. (2008). Towards a pathway definition of Parkinson’s disease: a complex disorder with links to cancer, diabetes and inflammation. Neurogenetics 9, 1–13. doi: 10.1007/s10048-007-0116-y
Moroo, I., Yamada, T., Makino, H., Tooyama, I., McGeer, P. L., McGeer, E. G., et al. (1994). Loss of insulin receptor immunoreactivity from the substantia nigra pars compacta neurons in Parkinson’s disease. Acta Neuropathol. 87, 343–348. doi: 10.1007/bf00313602
Naganawa, M., Lim, K., Nabulsi, N. B., Lin, S. F., Labaree, D., Ropchan, J., et al. (2018). Evaluation of pancreatic VMAT2 binding with active and inactive enantiomers of [(18)F]FP-DTBZ in healthy subjects and patients with type 1 diabetes. Mol. Imaging Biol. 20, 835–845. doi: 10.1007/s11307-018-1170-6
Naganawa, M., Lin, S. F., Lim, K., Labaree, D., Ropchan, J., Harris, P., et al. (2016). Evaluation of pancreatic VMAT2 binding with active and inactive enantiomers of (18)F-FP-DTBZ in baboons. Nucl. Med. Biol. 43, 743–751. doi: 10.1016/j.nucmedbio.2016.08.018
Normandin, M. D., Petersen, K. F., Ding, Y. S., Lin, S. F., Naik, S., Fowles, K., et al. (2012). In vivo imaging of endogenous pancreatic beta-cell mass in healthy and type 1 diabetic subjects using 18F-fluoropropyl-dihydrotetrabenazine and PET. J. Nucl. Med. 53, 908–916. doi: 10.2967/jnumed.111.100545
Ogama, N., Sakurai, T., Kawashima, S., Tanikawa, T., Tokuda, H., Satake, S., et al. (2018). Postprandial hyperglycemia is associated with white matter hyperintensity and brain atrophy in older patients with Type 2 diabetes mellitus. Front. Aging Neurosci. 10:273. doi: 10.3389/fnagi.2018.00273
Owens, W. A., Williams, J. M., Saunders, C., Avison, M. J., Galli, A., and Daws, L. C. (2012). Rescue of dopamine transporter function in hypoinsulinemic rats by a D2 receptor-ERK-dependent mechanism. J. Neurosci. 32, 2637–2647. doi: 10.1523/jneurosci.3759-11.2012
Pagano, G., Polychronis, S., Wilson, H., Giordano, B., Ferrara, N., Niccolini, F., et al. (2018). Diabetes mellitus and Parkinson disease. Neurology 90, e1654–e1662.
Palacios, N., Gao, X., McCullough, M. L., Jacobs, E. J., Patel, A. V., Mayo, T., et al. (2011). Obesity, diabetes, and risk of Parkinson’s disease. Mov. Disord. 26, 2253–2259.
Park, C. R., Moon, M. J., Park, S., Kim, D. K., Cho, E. B., Millar, R. P., et al. (2013). A novel glucagon-related peptide (GCRP) and its receptor GCRPR account for coevolution of their family members in vertebrates. PLoS One 8:e65420. doi: 10.1371/journal.pone.0065420
Parker, W. D. Jr., Parks, J. K., et al. (2008). Complex I deficiency in Parkinson’s disease frontal cortex. Brain Res. 1189, 215–218. doi: 10.1016/j.brainres.2007.10.061
Payer, D. E., Guttman, M., Kish, S. J., Tong, J., Adams, J. R., Rusjan, P., et al. (2016). D3 dopamine receptor-preferring [11C]PHNO PET imaging in Parkinson patients with dyskinesia. Neurology 86, 224–230. doi: 10.1212/wnl.0000000000002285
Pecic, S., Milosavic, N., Rayat, G., Maffei, A., and Harris, P. E. (2019). A novel optical tracer for VMAT2 applied to live cell measurements of vesicle maturation in cultured human beta-cells. Sci. Rep. 9:5403.
Petrisic, M. S., Augood, S. J., and Bicknell, R. J. (1997). Monoamine transporter gene expression in the central nervous system in diabetes mellitus. J. Neurochem. 68, 2435–2441. doi: 10.1046/j.1471-4159.1997.68062435.x
Purwana, I., Zheng, J., Li, X., Deurloo, M., Son, D. O., Zhang, Z., et al. (2014). GABA promotes human beta-cell proliferation and modulates glucose homeostasis. Diabetes 63, 4197–4205. doi: 10.2337/db14-0153
Raffo, A., Hancock, K., Polito, T., Xie, Y., Andan, G., Witkowski, P., et al. (2008). Role of vesicular monoamine transporter type 2 in rodent insulin secretion and glucose metabolism revealed by its specific antagonist tetrabenazine. J. Endocrinol. 198, 41–49. doi: 10.1677/joe-07-0632
Ray, N. J., Miyasaki, J. M., Zurowski, M., Ko, J. H., Cho, S. S., Pellecchia, G., et al. (2012). Extrastriatal dopaminergic abnormalities of DA homeostasis in Parkinson’s patients with medication-induced pathological gambling: a [11C] FLB-457 and PET study. Neurobiol. Dis. 48, 519–525. doi: 10.1016/j.nbd.2012.06.021
Renaud, J., Bassareo, V., Beaulieu, J., Pinna, A., Schlich, M., Lavoie, C., et al. (2018). Dopaminergic neurodegeneration in a rat model of long-term hyperglycemia: preferential degeneration of the nigrostriatal motor pathway. Neurobiol. Aging 69, 117–128. doi: 10.1016/j.neurobiolaging.2018.05.010
Rodriguez-Diaz, R., Abdulreda, M. H., Formoso, A. L., Gans, I., Ricordi, C., Berggren, P. O., et al. (2011). Innervation patterns of autonomic axons in the human endocrine pancreas. Cell. Metab. 14, 45–54. doi: 10.1016/j.cmet.2011.05.008
Saeedi, P., Petersohn, I., Salpea, P., Malanda, B., Karuranga, S., Unwin, N., et al. (2019). Global and regional diabetes prevalence estimates for 2019 and projections for 2030 and 2045: results from the International Diabetes Federation Diabetes Atlas. Diabetes Res. Clin. Pract. 157:107843. doi: 10.1016/j.diabres.2019.107843
Samandari, R., Chizari, A., Hassanpour, R., Mousavi, Z., and Haghparast, A. (2013). Streptozotocin-induced diabetes affects the development and maintenance of morphine reward in rats. Neurosci. Lett. 543, 90–94. doi: 10.1016/j.neulet.2013.03.024
Santiago, J. A., Bottero, V., and Potashkin, J. A. (2017). Biological and clinical implications of comorbidities in Parkinson’s disease. Front. Aging Neurosci. 9:394. doi: 10.3389/fnagi.2017.00394
Santiago, J. A., and Potashkin, J. A. (2014). System-based approaches to decode the molecular links in Parkinson’s disease and diabetes. Neurobiol. Dis. 72(Pt A), 84–91. doi: 10.1016/j.nbd.2014.03.019
Sharma, A. N., Ligade, S. S., Sharma, J. N., Shukla, P., Elased, K. M., and Lucot, J. B. (2015). GLP-1 receptor agonist liraglutide reverses long-term atypical antipsychotic treatment associated behavioral depression and metabolic abnormalities in rats. Metab. Brain Dis. 30, 519–527. doi: 10.1007/s11011-014-9591-7
Shi, X., Zhang, Y., Xu, S., Kung, H. F., Qiao, H., Jiang, L., et al. (2019). Decreased striatal vesicular monoamine transporter type 2 correlates with the nonmotor symptoms in parkinson disease. Clin. Nucl. Med. 44, 707–713. doi: 10.1097/rlu.0000000000002664
Sinclair, A., Saeedi, P., Kaundal, A., Karuranga, S., Malanda, B., and Williams, R. (2020). Diabetes and global ageing among 65-99-year-old adults: findings from the International Diabetes Federation Diabetes Atlas. Diabetes Res. Clin. Pract. 162:108078. doi: 10.1016/j.diabres.2020.108078
Singhal, T., Ding, Y. S., Weinzimmer, D., Normandin, M. D., Labaree, D., Ropchan, J., et al. (2011). Pancreatic beta cell mass PET imaging and quantification with [11C]DTBZ and [18F]FP-(+)-DTBZ in rodent models of diabetes. Mol. Imaging Biol. 13, 973–984. doi: 10.1007/s11307-010-0406-x
Stahl, S. M. (2018). Mechanism of action of vesicular monoamine transporter 2 (VMAT2) inhibitors in tardive dyskinesia: reducing dopamine leads to less “go” and more “stop” from the motor striatum for robust therapeutic effects. CNS Spectr. 23, 1–6. doi: 10.1017/s1092852917000621
Stormezand, G. N., Chaves, L. T., Vallez Garcia, D., Doorduin, J., De Jong, B. M., Leenders, K. L., et al. (2020). Intrastriatal gradient analyses of 18F-FDOPA PET scans for differentiation of Parkinsonian disorders. Neuroimage Clin. 25:102161. doi: 10.1016/j.nicl.2019.102161
Su, C. J., Shen, Z., Cui, R. X., Huang, Y., Xu, D. L., Zhao, F. L., et al. (2020). Thioredoxin-Interacting Protein (TXNIP) Regulates Parkin/PINK1-mediated Mitophagy in dopaminergic neurons under high-glucose conditions: implications for molecular links between Parkinson’s disease and diabetes. Neurosci. Bull. 36, 346–358. doi: 10.1007/s12264-019-00459-5
Sun, X., Han, F., Yi, J., Han, L., and Wang, B. (2011). Effect of aspirin on the expression of hepatocyte NF-kappaB and serum TNF-alpha in streptozotocin-induced type 2 diabetic rats. J. Korean Med. Sci. 26, 765–770.
Sun, Y., Chang, Y. H., Chen, H. F., Su, Y. H., Su, H. F., and Li, C. Y. (2012). Risk of Parkinson disease onset in patients with diabetes: a 9-year population-based cohort study with age and sex stratifications. Diabetes Care 35, 1047–1049. doi: 10.2337/dc11-1511
Takahashi, N., Miner, L. L., Sora, I., Ujike, H., Revay, R. S., Kostic, V., et al. (1997). VMAT2 knockout mice: heterozygotes display reduced amphetamine-conditioned reward, enhanced amphetamine locomotion, and enhanced MPTP toxicity. Proc. Natl. Acad. Sci. U.S.A. 94, 9938–9943. doi: 10.1073/pnas.94.18.9938
Ter Horst, K. W., Lammers, N. M., Trinko, R., Opland, D. M., Figee, M., Ackermans, M. T., et al. (2018). Striatal dopamine regulates systemic glucose metabolism in humans and mice. Sci. Transl. Med. 10:eaar3752. doi: 10.1126/scitranslmed.aar3752
Tritsch, N. X., Ding, J. B., and Sabatini, B. L. (2012). Dopaminergic neurons inhibit striatal output through non-canonical release of GAB. Nature A 490, 262–266. doi: 10.1038/nature11466
Wahlqvist, M. L., Lee, M. S., Hsu, C. C., Chuang, S. Y., Lee, J. T., and Tsai, H. N. (2012). Metformin-inclusive sulfonylurea therapy reduces the risk of Parkinson’s disease occurring with Type 2 diabetes in a Taiwanese population cohort. Parkins. Relat. Disord. 18, 753–758. doi: 10.1016/j.parkreldis.2012.03.010
Wang, C., Huang, X., Tian, S., Huang, R., Guo, D., Lin, H., et al. (2020). High plasma resistin levels portend the insulin resistance-associated susceptibility to early cognitive decline in patients with type 2 diabetes mellitus. J. Alzheimers Dis. 75, 807–815. doi: 10.3233/jad-200074
Wang, H., and Raleigh, D. P. (2014). The ability of insulin to inhibit the formation of amyloid by pro-islet amyloid polypeptide processing intermediates is significantly reduced in the presence of sulfated glycosaminoglycans. Biochemistry 53, 2605–2614. doi: 10.1021/bi4015488
Wang, Y. M., Gainetdinov, R. R., Fumagalli, F., Xu, F., Jones, S. R., Bock, C. B., et al. (1997). Knockout of the vesicular monoamine transporter 2 gene results in neonatal death and supersensitivity to cocaine and amphetamine. Neuron 19, 1285–1296. doi: 10.1016/s0896-6273(00)80419-5
Webb, J. L., Ravikumar, B., Atkins, J., Skepper, J. N., and Rubinsztein, D. C. (2003). Alpha-Synuclein is degraded by both autophagy and the proteasome. J. Biol. Chem. 278, 25009–25013. doi: 10.1074/jbc.m300227200
Wei, W., Ehlerding, E. B., Lan, X., Luo, Q. Y., and Cai, W. (2019). Molecular imaging of beta-cells: diabetes and beyond. Adv. Drug Deliv. Rev. 139, 16–31. doi: 10.1016/j.addr.2018.06.022
Wilcox, N. S., Rui, J., Hebrok, M., and Herold, K. C. (2016). Life and death of beta cells in Type 1 diabetes: a comprehensive review. J. Autoimmun. 71, 51–58. doi: 10.1016/j.jaut.2016.02.001
Wilson, J. M., and Kish, S. J. (1996). The vesicular monoamine transporter, in contrast to the dopamine transporter, is not altered by chronic cocaine self-administration in the rat. J. Neurosci. 16, 3507–3510. doi: 10.1523/jneurosci.16-10-03507.1996
Wood, H. (2014). Parkinson disease: 18F-DTBZ PET tracks dopaminergic degeneration in patients with Parkinson disease. Nat. Rev. Neurol. 10:305. doi: 10.1038/nrneurol.2014.81
Wu, Q., Xu, H., Wang, W., Chang, F., Jiang, Y., et al. (2016). Retrograde trafficking of VMAT2 and its role in protein stability in non-neuronal cells. J. Biomed. Res. 30, 502–509.
Wu, X., Zhou, X., Zhang, S., Zhang, Y., Deng, A., Han, J., et al. (2015). Brain uptake of a non-radioactive pseudo-carrier and its effect on the biodistribution of [(18)F]AV-133 in mouse brain. Nucl. Med. Biol. 42, 630–636. doi: 10.1016/j.nucmedbio.2015.03.009
Xu, Q., Park, Y., Huang, X., Hollenbeck, A., Blair, A., Schatzkin, A., et al. (2011). Diabetes and risk of Parkinson’s disease. Diabetes Care 34, 910–915.
Yang, C. T., Ghosh, K. K., Padmanabhan, P., Langer, O., Liu, J., Halldin, C., et al. (2017). PET probes for imaging pancreatic islet cells. Clin. Transl. Imaging 5, 507–523. doi: 10.1007/s40336-017-0251-x
Yang, J., Zhang, L. J., Wang, F., Hong, T., and Liu, Z. (2019). Molecular imaging of diabetes and diabetic complications: beyond pancreatic beta-cell targeting. Adv. Drug Deliv. Rev. 139, 32–50. doi: 10.1016/j.addr.2018.11.007
Yu, M. G., Keenan, H. A., Shah, H. S., Frodsham, S. G., Pober, D., He, Z., et al. (2019). Residual beta cell function and monogenic variants in long-duration type 1 diabetes patients. J. Clin. Invest. 129, 3252–3263. doi: 10.1172/jci127397
Keywords: diabetes mellitus, β-cell mass, Parkinson’s disease, dopamine, VMAT2
Citation: Kong Y, Zhou H, Feng H, Zhuang J, Wen T, Zhang C, Sun B, Wang J and Guan Y (2020) Elucidating the Relationship Between Diabetes Mellitus and Parkinson’s Disease Using 18F-FP-(+)-DTBZ, a Positron-Emission Tomography Probe for Vesicular Monoamine Transporter 2. Front. Neurosci. 14:682. doi: 10.3389/fnins.2020.00682
Received: 08 March 2020; Accepted: 03 June 2020;
Published: 14 July 2020.
Edited by:
Nicola Toschi, University of Rome Tor Vergata, ItalyReviewed by:
Chengxiang Qiu, University of Washington, United StatesDelia Cabrera DeBuc, University of Miami, United States
Copyright © 2020 Kong, Zhou, Feng, Zhuang, Wen, Zhang, Sun, Wang and Guan. This is an open-access article distributed under the terms of the Creative Commons Attribution License (CC BY). The use, distribution or reproduction in other forums is permitted, provided the original author(s) and the copyright owner(s) are credited and that the original publication in this journal is cited, in accordance with accepted academic practice. No use, distribution or reproduction is permitted which does not comply with these terms.
*Correspondence: Bomin Sun, sbm11224@rjh.com.cn; Jiao Wang, jo717@shu.edu.cn; Yihui Guan, guanyihui@hotmail.com